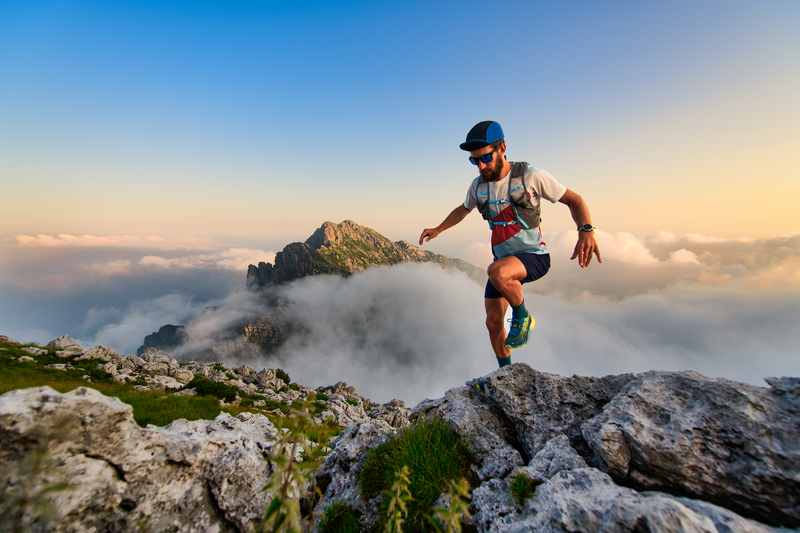
95% of researchers rate our articles as excellent or good
Learn more about the work of our research integrity team to safeguard the quality of each article we publish.
Find out more
ORIGINAL RESEARCH article
Front. For. Glob. Change , 31 May 2024
Sec. Forest Soils
Volume 7 - 2024 | https://doi.org/10.3389/ffgc.2024.1419023
This article is part of the Research Topic Understanding the Relationship between Extreme Climate Events and Forest Soil Hydrology: Implications for Ecosystem Functions View all 6 articles
Larix gmelinii is an important ecological construction tree species in northern China, and its carbon storage and distribution characteristics are of great significance for evaluating the carbon balance and climate effect of forest ecosystems. However, at present, there is a lack of systematic research on the carbon storage of L. gmelinii forests and its change with forest age. In this paper, the biomass and carbon density of L. gmelinii forests at different ages and the distribution of carbon storage in vegetation and soil were analyzed by means of sample plot investigation and model simulation in the northern forest area of Daxing’anling, Inner Mongolia. The influence of forest age on the carbon storage and carbon pool distribution characteristics of L. gmelinii forests and the mechanism of influencing factors were also discussed. Results show that: (1) As forest age increased, the total amount of carbon pools initially increased and then decreased, and the distribution structure of carbon pools showed a trend of transferring from soil to trees. The proportion of soil carbon pools gradually decreased (72.72–51.87%), while the proportion of tree carbon pools gradually increased (23.98–39.33%). The proportion of shrub and grass carbon pools was also relatively stable (0.51–0.53%). (2) Soil carbon pool was affected by the input and output of soil organic matter, soil depth, soil carbon content, and soil bulk density, shrub–grass carbon pool was affected by undergrowth light conditions and soil moisture, litter carbon pool was affected by litter input and output, and the carbon pool of trees was affected by the growth rate and carbon balance of trees. This study provides scientific basis and management suggestions for the carbon storage capacity of L. gmelinii forests and the mitigation of climate change.
The forest is the most important carbon sink in the terrestrial ecosystem that can absorb carbon dioxide in the atmosphere through photosynthesis, convert it into organic carbon, and store it in plants and soil (Terrer et al., 2021). The global forest carbon storage is estimated at 861PgC, among which vegetation and soil carbon storages account for 436PgC and 425PgC, respectively (Chen et al., 2018). The size and distribution of forest carbon storage are affected by forest types, climatic conditions, soil properties, human disturbance, and other factors (Wei et al., 2015; Aponte et al., 2020). Studying forest carbon storage and its characteristics has important theoretical significance and practical value for revealing the carbon cycle process of forest ecosystems, evaluating the response and adaptation ability of forests to climate change, and formulating policies and measures for forest carbon emission reduction (Hofhansl et al., 2020). Larix gmelinii is one of the northernmost conifer species in the world that is distributed in Northeast China and the Far East of Russia and is the main conifer species in the Daxing’anling forest area (Gao et al., 2023). L. gmelinii natural forest occupies more than 70% of the Daxing’anling forest area, making it the most typical forest type in this area (Wei et al., 2015). L. gmelinii is also an important carbon sink and source that plays an important role in mitigating and adapting to climate change (Wang et al., 2023). However, due to climate change, human disturbance, natural disasters, and other factors, the dynamic changes and driving mechanisms of biomass and carbon storage in L. gmelinii natural forests remain unclear and warrant further study (Lange et al., 2015; Sun and Liu, 2019).
Moerdaoga, Jinhe, and Genhe are affiliated enterprises of the key state-owned forest administration in Daxing’anling, Inner Mongolia. These enterprises are located in the northwest foot of Daxing’anling facing Russia across the Ergun River and have rich forest resources and ecological value. These three forestry bureaus have a total area of over 1.5 million ha and a forest coverage rate of over 90%, making these enterprises an important part of the ecological functional area of Daxing’anling. These bureaus are located in the core part of the northern forest area of Daxing’anling and are typical L. gmelinii forest distribution areas with high biodiversity. These areas are also national key forest areas with high biomass and carbon density. The L. gmelinii forests in these bureaus are affected by human disturbance and natural disasters to different degrees, such as harvesting, grazing, and fires. Therefore, the dynamic changes and driving mechanisms of biomass and carbon storage in these forests warrant an extensive investigation.
Many studies have investigated the carbon pool of L. gmelinii forests in Daxing’anling, especially its size, distribution, change, and influencing factors, from different angles and levels. For instance, Xiao et al. (2020) compared the carbon and nitrogen stocks of three typical L. gmelinii forest ecosystems in northern Daxing’anling and identified soil as their main component, accounting for 64.61–83.20% of the total carbon stocks. Khan et al. (2019) studied the effects of climatic factors on the stem biomass and carbon pool of L. gmelinii and Betula platyphylla in Daxing’anling and the spatial changes in the carbon storage of these two tree species. Results show that the stem biomass and carbon pool of L. gmelinii decreased with increasing altitude, that the stem biomass and carbon pool of L. gmelinii were higher than those of B. platyphylla, and that the stem biomass and carbon pool significantly differ between these two species across various slope directions and gradients. Meng et al. (2019) found that the aboveground biomass of natural L. gmelinii forests initially increased and then decreased with increasing forest age mainly due to stem biomass, while the biomass of branches and leaves was relatively small. The effects of stand density and diameter at breast height (DBH) on aboveground biomass interact with stand age, thereby suggesting that these effects vary across different stand ages. Zhu et al. (2017) studied the plant residue carbon pool of L. gmelinii forests across different succession stages in northeast China, its contributions to the ecosystem carbon pool, and its influencing factors. Results show that the carbon pool of plant residues in L. gmelinii forests initially increased and then decreased along with changing succession stage mainly due to litter carbon pool, while the carbon pool of dead wood was relatively small. Meng et al. (2017) found that the carbon pool of natural L. gmelinii forests initially increased and then decreased with increasing forest age mainly due to arbor and soil carbon pools, while the undergrowth and litter carbon pools were relatively small. The spatial distribution of carbon pools is affected by topography, climate, and soil and are mainly concentrated in the northern and central regions. The dynamic change in carbon pool is affected by forest fires, forestry activities, and climate change, which can also reflect an increase or decrease in carbon pool. Qi et al. (2013) studied the soil organic carbon pool and layered distribution characteristics of L. gmelinii plantations with different forest ages in Daxing’anling and those factors affecting the soil organic carbon pool. Results show that the soil organic carbon pool of L. gmelinii plantations initially increased and then decreased with increasing forest age and was mainly distributed in the 0 cm–20 cm soil layer. Soil organic carbon pool is significantly correlated with soil physical and chemical properties, stand structure, and undergrowth vegetation. Guang et al. (2016) studied the carbon pool (along with its distribution characteristics) of L. gmelinii plantations and natural Korean pine broad-leaved forests of different ages in northeast China and its influencing factors. Results show that the carbon pool of these plantations initially increased and then decreased with increasing forest age mainly due to arbor and soil carbon pools, while the undergrowth and litter carbon pools were relatively small. The carbon pool of natural Korean pine broad-leaved forests is significantly higher than that of L. gmelinii plantations mainly due to arbor carbon pool. The distribution characteristics of carbon pool are affected by forest age, stand density, DBH, tree height, and soil organic carbon content.
In this paper, three typical forestry bureaus in the north of Daxing’anling were selected as the study areas, and natural L. gmelinii forests of different forest ages were selected as the study objects. The biomass, standing stock, carbon content, carbon density, and carbon storage of the trees, undergrowth, standing trees, litter, and soil of natural L. gmelinii forests were measured in detail by means of standard plot investigation, laboratory analysis, and model simulation. The carbon pool distribution characteristics of these forests were also analyzed, and the relationship between their carbon storage and certain factors, such as forest age, stand density, and soil depth, was discussed. This study provides basic data and reference for further investigations into the carbon cycle of L. gmelinii natural forests in northern Daxing’anling.
The study area is located in three forestry bureaus in the north of Daxing’anling, namely, the Moerdaoga Forestry Bureau, Jinhe Forestry Bureau, and Genhe Forestry Bureau. Moerdaoga Forestry Bureau is located in the western slope of the northern section of the Daxing’anling. The geographical coordinates are N51°03′16′′ to 52°06′00 ′′ and E120°00′26′′to 121°19′31′′. The forest coverage was 94.97%. The annual average temperature in the region is −5.0°C, the extreme minimum temperature in January is −44.5°C, and the extreme maximum temperature in July is 33.9°C. The average annual precipitation is 360 mm, and the growth period is about 85 days. The terrain is higher in the south and east, and lower in the west and north. The climate is temperate monsoon climate and temperate continental climate. The forest coverage rate is as high as 94.91%. The main rainfall season is from July to September. The soil types are mountainous shallow gray loam and zonal brown coniferous forest soil.
Jinhe Forestry Bureau is located in the northwest slope of the northern section of the Daxing’anling. The forest coverage was 87.97%. The geographical coordinates are N51°20′02′′ to 51°49′48′′and E122°23′34′′ to 122° 52′46′′. The area belongs to the arid continental climate, the annual average temperature is −5.5°C, the extreme maximum temperature is 36.0°C, and the extreme minimum temperature is −58.0°C. The average annual precipitation is 480 mm, the main rainfall season is from June to August, the growth period is about 60 days, the forest coverage is 90%, and the soil type is brown coniferous forest soil.
The Genhe Forestry Bureau is located on the western slope of the northern section of the Daxing’anling. The geographical coordinates are N50°25′30′′ to 51°17′00′′and E120°41′30 ′′ to122° 42′30′′. The forest coverage was 83.76%. The area is a cold temperate monsoon coniferous forest climate. The annual average temperature is −5.3°C, the extreme maximum temperature is 35.0°C, and the extreme minimum temperature is −58.0°C. The average annual precipitation is 530 mm, and the growth period is 90 to 110 days. The forest coverage rate is 83.76%. The climate is cold and humid. The winter is long and the summer is short. There is a permafrost layer in the territory. The soil type is brown coniferous forest soil (see Figure 1).
From July to September 2020, sample plots were set up in the aforementioned bureaus mainly aimed at the forest types of L. gmelinii, including young, middle-aged, near-mature, mature, and over-mature forests. Each sample plot had an area of 20 m × 20 m, and the canopy density conditions in the sample plots were similar. In each sample plot, five survey points were selected along the two diagonal lines and the central position of the sample plot. At each survey point, three small squares were set up for investigation as follows: (1) five samples of 2 m × 2 m shrub small squares, whose shrub species, number of plants, height, ground diameter, and coverage were recorded; five 1 m × 1 m small samples of herbs whose species, plant number, height, coverage, and other factors were recorded; and (3) five samples of 0.5 m × 0.5 m litter, which were investigated according to decomposed layer and undecomposed layer. All trees in each sample plot were investigated along with their species, DBH, height, survival status, and other factors. All dead standing trees were also examined along with their coordinates, species, survival status, DBH, height, and other factors. All fallen trees were investigated via a sample plot survey, and each tree was measured from left to right and from bottom to top in turn. The horizontal and ordinate, decay degree, length, big head diameter, small head diameter, and central diameter of these trees were recorded.
The forest age of the sample plot was determined according to the vegetation information of the main forest layer of the sample plot, the forest phase data of the local forestry bureau, and other relevant data. Sample plots were randomly selected, and 3 repeated sample plots not belonging to the same forest class were set up, amounting to a total of 45 sample plots. Table 1 presents the details of these sample plots.
Three standing trees were randomly selected from each sample plot, and trunk, bark, branch, leaf, and root samples were collected. To protect the trees, only a little destructive collection was carried out on the trunk. The dead parts of shrubs and herbs were removed, and these shrubs and samples were divided into branches, leaves, and roots (shrubs) or aboveground and underground parts (herbs). All dead and fallen trees were collected. Three samples were randomly selected from five samples of 0.5 m × 0. 5 m in each sample plot to collect litter. Stones and some fresh plants were screened out and divided into decomposed and undecomposed layers. The weight of all samples exceeded 100 g. The fresh weight in the field was weighed, brought into a room, and let dry at 65°C to constant weight. The dry weight it was then weighed and recorded. The total dry weight of the field sample was converted from the calculated moisture content, which was then used to calculate the on-hand quantity. The soil profile method was adopted, and one soil profile was dug from each sample plot. Given the thin soil layer in the study area, only three layers of 0 cm–10 cm, 10 cm–20 cm, and 20 cm–30 cm were collected. Three soil samples were collected from each layer. The roots and gravels in the soil samples were picked out and brought into a room for air drying to determine their carbon content. Ring knife soil samples were taken from each soil layer to determine the soil bulk density.
According to the same organs, 225 standing tree samples, 135 shrub samples, 90 herb samples, 45 dead standing tree samples, and 135 fallen tree samples were obtained. The surface litter samples were divided into decomposed and undecomposed layers, amounting to a total of 90 samples. All these samples were dried and ground into powder and passed through a 0.2 mm (80 mesh) sieve, and the carbon content was determined using the K2Cr2O2 + H2SO4 oxidation method. A total of 540 soil samples were retained for the analysis. The air-dried soil samples were crushed with wooden sticks and passed through 2 mm (10 mesh) sieve. A proper amount of samples was then obtained using the quartering method and ground through a 0.149 mm (100 mesh) sieve. The carbon content of the treated soil samples was calculated via titration analysis with the K2Cr2O2 + H2SO4 external heating method. The carbon content of all samples was sent to the Experimental Research Center of Shagu Fenglin Environmental Technology Co., Ltd. in Inner Mongolia for determination. In order to avoid the experimental error caused by the long storage of the samples, all the samples were measured within 1 month after collection.
The biomass or standing stock of the standing trees, shrubs, herbs, dead trees, fallen trees, and ground litter was estimated through a model simulation. Table 2 presents the calculation formulas. The carbon storage in this study includes arbor, shrub, grass, litter, and soil carbon storage. After calculating the biomass and standing stock and obtaining the carbon density, the carbon storage and total carbon storage of each component were obtained by multiplying the area of different forest ages and carbon density in Table 1. After these calculations, the distribution characteristics of the carbon pool can be analyzed.
All data in this paper were measured by the average of the results of each treatment. The influence of forest age on carbon storage of vegetation and soil and the difference in soil carbon storage at different depths were measured by one-way ANOVA and least significant difference. The significance level was set to a = 0. 05. The data analysis software was SPSS 22, and the mapping was based on Origin 2022.
Figure 2 shows the biomass of different organs of L. gmelinii at different forest ages in the three forestry bureaus. The biomass of these organs showed different trends in general. Specifically, the biomass in A increased with forest age, that in B initially decreased and then increased, and that in C slightly changed across each forest age and reached its peak in the middle forest age. The trunk biomass occupied the majority of the biomass of each organ (58.26–68.78% of the total biomass), followed by root biomass (11.38–16.12%), branch (5.58–9.62%), bark (4.62–8.76%), and leaf (0.96–3.82%). In sum, the biomass distribution of L. gmelinii forests was mainly concentrated in the aboveground part and was relatively small in the underground part. The biomass of A was higher than those of B and C, especially in mature and over-mature forests. Specifically, the biomass of A was 139.03 t/hm2 and 178.29 t/hm2, while those of B and C were 73.14 t/hm2 and 70.13 t/hm2 and 57.12 t/hm2 and 61.15 t/hm2, respectively. This result may be ascribed to the geographical location, climatic conditions, soil types, forest fire interference, and other factors of the three bureaus. Trunk, root, branch, leaf, and bark showed different trends across various forestry scales but showed similar trends in the same forestry scale, which may be due to the stand structure, DBH, height, and stand density of L. gmelinii forests.
Figure 2. Biomass of different organs of L. gmelinii standing trees of different ages. (A) denotes the Moerdaoga Forestry Bureau, (B) denotes the Jinhe Forestry Bureau, and (C) denotes the Genhe Forestry Bureau.
Figure 3 shows the standing stock of dead components and the biomass of undergrowth vegetation layer at the scale of the three forestry bureaus. On the whole, with increasing forest age, the standing stock of dead standing trees and fallen trees initially increased and then decreased, among which the standing stock of mature and over-mature forests accounted for the largest proportion and the standing stock of young forests accounted for the smallest proportion. This result is consistent with the characteristics of forest fire disturbance and natural regeneration of L. gmelinii forests. In the standing stock of dead standing trees and fallen trees, the standing stock of fallen trees occupied the main part (54.01–100% of the total standing stock), while that of dead standing trees only occupied a relatively small part (0–45.99%). This result indicates that the dead wood of L. gmelinii forests mainly exists in the form of fallen wood and is relatively few. The standing stock of standing trees in A was higher than those in B and C, especially in middle-aged and over-mature forests. Specifically, the standing stock of A was 14.80 t/hm2 and 16.29 t/hm2, while those of B and C were 0.55 t/hm2 and 0.92 t/hm2 and 3.67 t/hm2 and 0 t/hm2, respectively. This result may be related to forest fire frequency, forest fire intensity, forest fire types, and other factors in different forestry bureaus. In the standing stock of surface litter and litter layer, the standing stock of the litter layer accounted for the main part (50.00–71.43% of the total standing stock), while that of surface litter occupied a relatively small part (28.57–50.00%). This result indicates that the litter of L. gmelinii forests mainly exists in the form of a litter layer, whereas the form of the surface litter is relatively small. Among the three bureaus, the standing amount of litter and litter layer of A was generally higher than those of B and C, especially in over-mature forests, where the standing amount of A was 23.17 t/hm2 while those of B and C were 8.34 t/hm2 and 3.26 t/hm2, respectively. This result may be related to the stand structure, DBH, tree height, stand density, and other factors of these bureaus.
Figure 3. Standing stock of dead components and biomass of undergrowth vegetation at different ages. (A) denotes the Moerdaoga Forestry Bureau, (B) denotes the Jinhe Forestry Bureau, and (C) denotes the Genhe Forestry Bureau.
In the biomass of shrubs and herbs, the biomass of herbs occupied the main part (50.00–83.33% of the total biomass), while that of shrubs only occupied a relatively small proportion of 16.67–50.00%. This result indicates that the undergrowth vegetation of L. gmelinii forests mainly exists in the form of herbs, and the form of shrubs is relatively few. The biomass of shrubs and herbs in B was higher than those in A and C, especially in young and mature forests. Specifically, the biomass of B was 2.44 t/hm2 and 2.84 t/hm2, while those of A and C was 2.02 t/hm2 and 0.49 t/hm2 and 2.83 t/hm2 and 2.30 t/hm2, respectively. This result may be related to the soil quality, soil moisture, soil nutrients, and other factors in the three bureaus.
Figure 4 shows the distribution characteristics of carbon content of each component. The carbon content of living, fallen, and dead trees increased along with forest age, among which mature and over-mature forests had the highest carbon content, while young forests had the lowest carbon content. This result is related to the growth characteristics of L. gmelinii forests and the decomposition speed of dead wood. In the carbon content of standing and fallen trees, the carbon content of fallen trees was higher than that of standing trees, accounting for 51.06–100% of the total carbon content, while the carbon content of standing trees only accounted for 0–48.94%. This result indicates that the fallen trees of L. gmelinii forests play an important role in carbon storage. Among the three bureaus, fallen trees were observed in the five forest ages of A, and no dead standing trees were observed in the near-mature forest. Fallen trees were observed in the middle, mature, and over-mature forests of B, and no dead standing trees were observed across the five forest ages. Fallen trees were only observed in the near-mature forests of C, and dead standing trees were observed in its middle, near-mature, and mature forests. These characteristics may be due to the stand structure, DBH, tree height, and stand density of the three bureaus. Figure 4 shows that the carbon content of dead standing trees and fallen trees is higher than that of the other components, and some of these trees even have a higher carbon content than the living standing trees.
For shrubs and herbs, the carbon content of shrubs was higher than that of herbs, thereby suggesting that shrubs in L. gmelinii forests play an important role in carbon storage. The carbon content of shrubs and herbs under the L. gmelinii forests in B was generally higher than those of shrubs and herbs in A and C, which may be due to the soil quality, soil moisture, soil nutrients, and other factors in these bureaus. Meanwhile, the carbon content of surface litter increased along with forest age. The carbon content of litter was the highest in mature and over-mature forests and was the lowest in young and middle forests. This result may be related to the characteristics of litter generation and decomposition in L. gmelinii forests. The carbon content of litter in A was generally higher than those in B and C, especially in over-mature forests, which may be due to litter quality and the decomposition speed in these bureaus.
Figure 5 shows the carbon content of different soil layers. The carbon content of these soil layers generally decreased with increasing soil depth, among which the carbon content of the 0 cm–10 cm layer was the highest, while that of the 20 cm–30 cm layer was the lowest. This result may be due to the distribution characteristics of soil organic carbon. Among different age groups, the carbon content of soil layer increased along with forest age, with the mature and over-mature forests accounting for the highest carbon content and the young forests accounting for the lowest. This result may be explained by the influence of forest age on soil organic carbon. The carbon content of the soil layer of L. gmelinii forests in C was generally higher than that of the soil layers of A and B, especially in near-mature and mature forests, which may be due to the soil types, soil fertility, soil moisture, and other factors in these bureaus.
Figure 5. Carbon content in different soil layers. (A) denotes the Moerdaoga Forestry Bureau, (B) denotes the Jinhe Forestry Bureau, and (C) denotes the Genhe Forestry Bureau.
Figure 6 shows the carbon density of different organs of standing trees of different ages. The carbon density of standing trees increased along with forest age, with the mature and over-mature forests having the highest carbon density, while the middle-aged forest having the lowest (except in C). This result is related to the growth characteristics and carbon storage capacity of L. gmelinii forests. Among all organs of standing trees, the carbon density of trunk accounted for the largest proportion of total carbon density, followed by roots, bark, branches, and leaves. This result indicates that the trunk of L. gmelinii forests plays an important role in carbon storage. The carbon density of standing trees of L. gmelinii forests in A was generally higher than that of standing trees in B and C, especially in mature and over-mature forests. The carbon density of A was 62.37 t/hm2 and 80.40 t/hm2, while those of B and C were 32.77 t/hm2 and 30.80 t/hm2 and 28.40 t/hm2 and 28.52 t/hm2, respectively. This result may be related to the stand structure, DBH, tree height, stand density, and other factors in the forestry bureaus.
Figure 6. Carbon density of different organs of standing trees of different ages. (A) denotes the Moerdaoga Forestry Bureau, (B) denotes the Jinhe Forestry Bureau, and (C) denotes the Genhe Forestry Bureau.
Figure 7 shows the carbon density of dead components and the undergrowth vegetation layer. The carbon density of dead components increased along with forest age, with the over-mature forest having the highest carbon density and the young forest having the lowest. This result is related to the quantity and quality of dead components. Among the dead components, the carbon density of ground litter accounted for the largest proportion, followed by that of fallen and dead standing trees. This result indicates that surface litter plays an important role in carbon storage. The carbon density of the dead component of A was generally higher than those of the dead components of B and C, especially in middle-aged and over-mature forests. The carbon density of A was 4.94 t/hm2 and 6.10 t/hm2, while those of B and C were 2.01 t/hm2 and 2.36 t/hm2 and 0.67 t/hm2 and 1.14 t/hm2, respectively. This result may be related to the quantity and quality of dead components across the bureaus. Meanwhile, the carbon density of the undergrowth layer decreased along with increasing forest age, with the young forest having the highest carbon density and the near-mature forest having the lowest. This result is related to the growth and competition of the undergrowth vegetation layer. In the understory vegetation layer, the carbon density of herbs accounted for the largest proportion, followed by shrubs. Therefore, herbs play an important role in carbon storage. The carbon density of the undergrowth layer of B was higher than those of the undergrowth layers of A and C, especially in young and over-mature forests. The carbon density of B was 0.99 t/hm2 and 1.17 t/hm2, while those of A and C were 0.71 t/hm2 and 0.16 t/hm2 and 0.66 t/hm2 and 0.51 t/hm2, respectively. This result may be related to the quantity and quality of undergrowth vegetation in these bureaus.
Figure 7. Carbon density of dead components and the understory vegetation layer. (A) denotes the Moerdaoga Forestry Bureau, (B) denotes the Jinhe Forestry Bureau, and (C) denotes the Genhe Forestry Bureau.
Figure 8 shows the carbon density of different soil layers. The soil carbon density increased along with forest age, with the mature forest showing highest carbon density and the young forest showing the lowest. This result is related to the accumulation and decomposition of soil organic matter. Among all soil layers, the 0 cm–10 cm soil layer accounted for the largest proportion of total carbon density, followed by the 10 cm–20 cm and 20 cm–30 cm layers. This result highlights the important role of topsoil in carbon storage. The soil carbon density of B was generally higher than those of A and C, especially in mature and over-mature forests. Specifically, the carbon density of B was 251.69 t/hm2 and 238.20 t/hm2, while those of A and C were 120.64 t/hm2 and 106.03 t/hm2 and 235.40 t/hm2 and 208.03 t/hm2, respectively. This result may be related to soil types, fertility, moisture, and other factors in these bureaus.
Figure 8. Carbon density across different soil layers. (A) denotes the Moerdaoga Forestry Bureau, (B) denotes the Jinhe Forestry Bureau, and (C) denotes the Genhe Forestry Bureau.
Figure 9 shows the distribution characteristics of carbon pool in L. gmelinii natural forests. The proportion of soil carbon pool to total carbon pool decreased along with increasing forest age, while that of arbor carbon pool increased along with forest age. This result is related to the growth characteristics and carbon storage capacity of L. gmelinii forests. Among all carbon pools, soil carbon pools accounted for the largest proportion, followed by arbor, litter, and shrub and grass carbon pools. This result shows that soil and trees play an important role in carbon storage. The proportion of soil carbon pool in B was generally higher than that in A and C, especially in middle-aged and near-mature forests. Specifically, the proportion of soil carbon pool in B was 91.78 and 88.93%, while those in A and C were 74.50 and 83.88% and 76.92 and 88.15%, respectively. This observation may be related to the soil types, fertility, moisture, and other factors in different forestry bureaus. The proportion of arbor carbon pool in A was generally higher than that in B and C, especially in mature and over-mature forests. The proportion in A was 32.32 and 39.33%, while those in B and C were 11.18 and 11.20% and 10.56 and 11.89%, respectively. This result may be related to the stand structure, DBH, tree height, stand density, and other factors in the bureaus.
Figure 9. Distribution characteristics of carbon pool in natural L. gmelinii forests. (A) denotes the Moerdaoga Forestry Bureau, (B) denotes the Jinhe Forestry Bureau, and (C) denotes the Genhe Forestry Bureau.
Figure 10 shows the carbon storage of different age groups in the three forestry bureaus. The change in carbon storage along with forest age showed an M shape, with the mature and middle-aged forests having the highest carbon storage and the young forest having the lowest. This observation is directly related to the area of different forest ages, their growth characteristics, and their carbon storage capacity. The carbon storage of C was generally higher than those of A and B, especially in middle-aged and near-mature forests. Specifically, the carbon storage of C was 5550.09 Mt. and 1709.30 Mt., while those of A and B were 3310.63 Mt. and 4150.99 Mt. and 743.42 Mt. and 1487.95 Mt., respectively. This result may be related to the stand structure, DBH, tree height, stand density, and other factors in the forestry bureaus. The carbon storage of A was generally lower than that of B and C, especially in mature and over-mature forests. Specifically, the carbon storage of A was 2455.90 Mt. and 490.89 Mt., while those of B and C were 4158.65 Mt. and 872.82 Mt. and 3041.10 Mt. and 1618.61 Mt., respectively. This finding may be related to the growth rate, mortality rate, and regeneration rate of trees in different forestry bureaus.
In this study, we found that the biomass and carbon density of living trees showed complex trends in different ages of L. gmelinii forests (He et al., 2018; Ying et al., 2019). In general, the standing trees of over-mature forests have higher dry matter content and carbon content due to their larger individuals and high lignin and cellulose content. In contrast, middle-aged standing trees have smaller individuals, lower lignin and cellulose content, trees are in a rapid growth period, with rapid cell division and expansion, but the degree of lignification has not yet reached a high level, and therefore lower biomass and carbon density (Peng et al., 2019; Bārdule et al., 2021). The carbon content of standing trees was relatively stable in different forest ages, which was related to the growth rate, lignification degree and lignocellulose content of trees. The living standing trees of mature forest are in the peak period of growth, with large quantity and high quality, while the number of living standing trees of young forest is small and the quality is low. The lignification degree of standing trees in middle-aged and near-mature forests is higher, and the carbon content is also higher, while the lignification degree of standing trees in young and near-mature forests is lower, and the carbon content is also lower (Kim et al., 2017; Dong et al., 2018). These findings are important for understanding the dynamics and management of forest carbon pools.
In L. gmelinii forest, with the increase of forest age, shrub biomass and carbon content showed an increasing trend, especially in over-mature forest, which was related to canopy density and light conditions (Guo et al., 2022). Light is an important factor in plant photosynthesis. With the increase of forest age, the canopy becomes sparse and the light conditions are improved, thus promoting the growth of shrub layer. Herb biomass increased first and then decreased, while carbon content fluctuated, reflecting the effects of species, structure, water content and decomposition degree of herbaceous plants (Jiang et al., 2016). In the stand with small forest age, herbaceous plants grow vigorously due to sufficient light. With the increase of forest age, the light conditions become worse, and the biomass of herbaceous plants begins to decrease. The number and thickness of surface litter increased with the increase of forest age, but the carbon content decreased, which may be related to the decomposition rate of litter. The shrub biomass of mature and over-mature forests was larger, while the shrub biomass of near-mature forests was the smallest, which may be related to canopy openness and soil fertility (Neupane and Sharma, 2014). Herb biomass is the largest in the near-mature forest, while the carbon content of the mature forest is the highest, which reflects the differences in plant growth conditions under different forest ages (Huang and Di, 2011). The surface litter layer is the thickest in the over-mature forest, and the carbon content is the lowest. This may be because the trees in the over-mature forest grow slowly, and the accumulation rate of fallen leaves and branches is greater than the decomposition rate (Li et al., 2014). In general, the increase of forest age had a significant effect on the biomass and carbon content of shrubs and herbs, while the change of surface litter was more affected by the decomposition process.
In different forest ages of L. gmelinii forest inDaxing’anling, the standing stock and carbon content of dead standing trees and fallen trees show a change rule closely related to the growth, death, decomposition and weathering of trees (Mac Nally et al., 2002). The standing stock of dead standing trees increased with forest age, reflecting the relationship between the increase of natural mortality and the accumulation of dead standing trees. The standing stock of dead standing trees of A was the highest, which may be due to its more over-mature forests in the forest land, high mortality rate of old trees and slow decomposition rate. On the contrary, the lack of dead standing trees in woodland B indicates that it may have taken effective forestry management measures to clean up dead trees and reduce forest fire risk and carbon emissions. The carbon content of dead standing trees decreased with the increase of forest age, and the carbon content of dead standing trees of C was the lowest, indicating that the dead standing trees had experienced a long period of decomposition and weathering. The standing stock of fallen trees also increased with the increase of forest age, and the standing stock of fallen trees in C was the highest, which may be related to the fact that there were more near-mature forests in the forest land, and the biomass and carbon storage of trees were large but the canopy was unstable and vulnerable to natural disasters. The standing crop of fallen wood of B is the lowest, which may be because its forest land has strong wind resistance and is not easy to fall wood. The carbon content of the fallen wood decreased with the age of the forest, and the carbon content of the fallen wood of B was the highest, which may be due to the slow decomposition rate of the fallen wood and less carbon loss.
The effects of dead standing trees and fallen trees on ecosystems are complex (Kang et al., 2012). They provide organic matter and nutrients for forest land, increase soil fertility, promote understory vegetation growth, increase biodiversity, provide habitats for animals, maintain ecological balance, and promote forest land renewal and natural restoration. However, they also occupy space, affect forest growth, increase combustibles, increase forest fire risk, release greenhouse gases, and exacerbate climate change (Lv et al., 2014). In the process of decomposition, they also release carbon dioxide and methane, which have a negative impact on global warming. Therefore, it is necessary to take appropriate management measures to make full use of its ecological value, prevent ecological risks, and achieve sustainable development, including regular clean-up, rational utilization, manual control and ecological restoration (Kirby et al., 1991). These measures should be adjusted according to factors such as forest age, forest type, topography and climate to ensure the health of forest land and the stability of ecosystem. This integrated management strategy helps to balance the ecological value and risk of forest land and promote its long-term ecological health and carbon storage capacity.
Forest age is an important factor affecting soil organic carbon accumulation. However, there is still no unified conclusion on the effect of forest age on soil carbon storage. In this study, it was found that soil carbon content and carbon density showed complex changes with soil depth and forest age in different age soils of L. gmelinii forest. The surface soil (0-10 cm) was most affected by vegetation cover and litter, and the soil carbon content of mature forest and near mature forest was higher. The sub-surface soil (10-20 cm) is greatly affected by soil type and structure. The carbon content of the shallow ash loam in the mountain is usually higher than that of the zonal brown coniferous forest soil, This is due to its better water retention and lower decomposition rate. The deep soil (20-30 cm) is most affected by climatic conditions. Low temperature and snow in winter lead to reduced soil microbial activity, resulting in higher soil carbon content. The change of soil carbon density is closely related to soil carbon content, and is affected by vegetation, soil type, climatic conditions and other factors (Wang et al., 2021). When evaluating the carbon storage capacity and carbon cycle function of L. gmelinii forest, these factors need to be considered comprehensively, rather than simply based on forest age. Specifically, the soil carbon content of A was the highest in the surface layer of mature forest, while in the deep soil, the carbon content of near mature forest was the highest. The soil carbon content and carbon density of B increased with the increase of forest age, and the over-mature forest was the highest in the surface and sub-surface soil, while the mature forest was the highest in the deep soil. The carbon content and carbon density of mature forest were the highest in surface and sub-surface soil, while the carbon content and carbon density of middle-aged forest were the highest in deep soil.
These changes reflected the accumulation and decomposition process of soil organic matter under different forest ages, as well as the effects of soil microbial activity and soil moisture status. The high vegetation coverage and litter amount of mature and near-mature forests promoted the accumulation of soil organic matter, while the low temperature and snow in winter inhibited the activity of soil microorganisms and reduced the decomposition of soil organic matter. In addition, soil type and structure also have significant effects on soil carbon content. For example, acidic soils and shallower soils are not conducive to the decomposition and transformation of soil organic matter (Wang et al., 2013).
In short, the soil carbon storage capacity and carbon cycle function of L. gmelinii forest are affected by many factors such as forest age, soil type and climatic conditions. These factors together determine the variation of soil carbon content and carbon density. Therefore, in order to more accurately assess and manage the carbon storage capacity of these forests, multiple considerations and integrated management strategies are needed (Wang et al., 2012). This includes monitoring changes in soil carbon content and carbon density, understanding the process of accumulation and decomposition of soil organic matter, and considering the potential impact of climate change on the soil carbon cycle, which can better understand and utilize the potential of L. gmelinii forest as a carbon sink to promote its ecological health and long-term carbon storage capacity.
Soil accounted for the greatest share of carbon pool across all three bureaus, indicating that soil is one of the most important carbon pools in the L. gmelinii forest ecosystem and a long-term carbon reservoir. This proportion showed different trends along with increasing forest age, which may be related to the input and output of soil organic matter (Yaozhan and Mingxi, 2015). Specifically, the proportion of soil carbon pool in A initially increased and then decreased because with an increasing forest age, the soil organic matter content and soil fertility of the forest land also increase, thus subsequently increasing the soil carbon storage. However, when forest age reaches a certain level, the decomposition and loss rate of soil carbon exceed the input rate of soil carbon, thus reducing the soil carbon storage (Webb et al., 2017). The proportion of soil carbon pool in B initially decreased and then increased. With increasing forest age, the soil organic matter content and soil fertility of forest land initially decrease and then increase, thus affecting soil carbon storage. The proportion of soil carbon pool in C showed a relatively stable distribution probably due to the relatively balanced input and output of soil organic matter, thus maintaining soil carbon storage.
Shrub and grass accounted for the lowest share of the carbon pool in the three bureaus, indicating that shrub and grass do not significantly contribute to the carbon storage of the L. gmelinii forest ecosystem. This result also reflects the inhibiting effect of dominant tree species in L. gmelinii forests on shrub and grass. Such proportion demonstrated different trends along with increasing forest age due to undergrowth light conditions and soil moisture. Specifically, with an increasing forest age, the forest land canopy gradually forms, thus reducing the light and temperature in the forest, which is not conducive to the growth of shrub and grass. However, when the forest age reaches a certain level, gaps start appearing in the canopy, thus allowing light and warmth into the forest, which benefit the growth of shrub and grass.
Litter carbon also accounted for a low share of the carbon pool in the three bureaus, indicating that litter does not significantly contribute to the carbon storage of the L. gmelinii forest ecosystem yet remains an important source of soil carbon pool and an important link in the carbon cycle (Huang et al., 2013). The proportion of litter carbon pool showed different trends with increasing forest age, which may be related to the input and output of litter. Specifically, the proportion of litter carbon pool in A and B initially increased and then decreased due to the increasing litter input, which ultimately increases the litter carbon storage. However, when the forest age reaches a certain level, the decomposition and transformation rate of litter carbon would exceed its input speed, thus reducing litter carbon storage. The proportion of litter carbon pool in C had a relatively stable distribution due to the balanced litter input and output in the bureau, thus maintaining litter carbon storage.
Arbor accounted for a high proportion of the carbon pool in all bureaus, indicating that arbor is one of the most important carbon pools in the L. gmelinii forest ecosystem and a rapid carbon reservoir (Wei et al., 2014). As the forest age increased, the proportion of arbor carbon pool initially decreased before increasing due to the different growth rates and carbon balance of trees. Specifically, the younger the forest, the faster the growth of trees. Moreover, the fixed rate of carbon is less than the release rate of carbon, thus resulting in a relatively low carbon pool of trees. Trees need to consume large amounts of carbon as an energy source during their growth, and their respiration also releases part of their consumed carbon. In this case, trees have a relatively low carbon storage that accounts for a small proportion of their total carbon pool. When the forest age reaches a certain level, the growth rate of trees gradually slows down, and the fixed rate of carbon exceeds the release rate of carbon, thus gradually increasing the proportion of arbor carbon pool. Trees can still fix a certain amount of carbon through photosynthesis during their growth, and their respiration will weaken. In this case, trees have a relatively high carbon storage that accounts for a large proportion of their total carbon pool (Klein and Hoch, 2015). When the forest is too old, the growth of trees almost stops, and the fixed rate of carbon is equal to the release rate. Therefore, the proportion of carbon pool of trees is stable or slightly decreased because they can no longer fix more carbon through photosynthesis during their growth, and their respiration will not consume too much carbon. In this case, the carbon storage of trees is relatively stable, and its proportion in the total carbon pool is stable or slightly decreased.
As the forest age increased, the total carbon pool initially increased and then decreased, where OMF > PMF > MF > YF > MAF. Specifically, over-mature forests had the largest total carbon pool, while middle-aged forests had the lowest. This result may be due to the slow growth of trees in over-mature forests, and the carbon fixation rate in these forests is greater than their carbon release rate, thus increasing their carbon pool. Meanwhile, the trees in middle-aged forests grow rapidly, and the fixed rate of carbon is less than the release rate of carbon, thus resulting in a low carbon pool (Wani and Qaisar, 2014). The total carbon pool of mature and near-mature forests also initially increased and then decreased due to the different growth rates and carbon balance of trees with different ages, which affect the formation and change in the total carbon pool.
As the forest age increased, the carbon pool distribution structure was transferred from the soil to the trees. Specifically, the proportion of soil carbon pool gradually decreased while that of tree carbon pool gradually increased, and the proportion of shrub and grass carbon and litter carbon pools was relatively stable. These results, are consistent with the findings of Qi et al. (2011), may be attributed to the fact that as the forest age increases, the soil carbon pool of the forest land gradually reaches saturation, while its arbor carbon pool gradually increases, thus changing the carbon pool distribution structure. The shrub and grass and litter carbon pools are affected by several factors, such as canopy shading, litter covering, and water supply. Therefore, the change in the distribution structure of these carbon pools is not obvious.
The distribution characteristics of the carbon pool in A demonstrated a certain regularity that is closely related to the change in forest age (Pregitzer and Euskirchen, 2004). To improve the carbon storage capacity of forest land and mitigate climate change, the following measures are proposed: properly adjust the age structure of forest land, keep a certain proportion of mature and near-mature forests, prevent the excessive growth of over-mature and middle-aged forests, and improve the total amount and stability of the carbon pool of forest land. A reasonable utilization of dead and fallen trees should not only give full play to their ecological value but also prevent ecological risks so as to improve the efficiency and safety of carbon pool allocation in forest land. The management and protection of forest land should be strengthened, the loss of carbon pool due to forest fires, diseases, pests, man-made destruction, and other factors should be prevented, the biodiversity of the forest land should be enhanced, and its ecological balance and sustainable development should be promoted. A large number of studies have shown that China’s forest ecosystem is mainly characterized by carbon sinks over the past 30 years mainly due to the increase in plantation areas and the restoration of natural forests in the country (Fang et al., 2001; Pan et al., 2004). Implementing natural forest protection projects also plays an important role in this process.
In this study, the effect of forest age on the carbon storage of L. gmelinii natural forests was investigated by replacing time with space. Using data of different forest age sample plots in the Moerdaoga, Jinhe, and Genhe Forestry Bureaus, the effect of forest age on the carbon storage and carbon pool distribution characteristics of L. gmelinii natural forests was analyzed. Results show that: (1) with increasing forest age, the total amount of carbon pools initially increased and then decreased, and their distribution structure was transferred from the soil to the trees. The proportion of soil carbon pools gradually decreased, while that of shrub and grass and litter carbon pools gradually increased, The proportion of shrub and grass carbon pools was also relatively stable; and (2) soil carbon pool is affected by the input and output of soil organic matter, soil depth, soil carbon content, and soil bulk density, the shrub and grass carbon pool is affected by undergrowth light conditions and soil moisture, the litter carbon pool is affected by litter input and output, and the carbon pool of trees is affected by their growth rate and carbon balance. The carbon sequestration potential of forest ecosystems in this area is mainly related to arbor carbon storage. Therefore, strengthening the management of forest ecosystem in this area, especially the management of arbor carbon pool, is of great significance for increasing the forest carbon sink in this area in the future. This study provides scientific basis and management suggestions for enhancing the carbon storage capacity and mitigating the effects of climate change on L. gmelinii forests.
Through a field investigation, the carbon storage of trees, shrubs, grasses, litter, and soil in L. gmelinii natural forests was systematically estimated on the sample plot scale, and the carbon content of each component was the actual value sent for inspection, thus greatly improving the estimation accuracy of carbon storage in forest ecosystems. The soil profile method was used to estimate the soil carbon storage of L. gmelinii natural forests. The soil occurrence layer in the Daxing’anling forest area is shallow and has many gravels, which introduce some uncertainty to the estimation of soil correlation value. In addition, the selection of sample plots in the process of field investigation also has some subjectivity, thus restricting the accuracy of the carbon storage estimation results.
The data analyzed in this study is subject to the following licenses/restrictions: The data in this paper are obtained by paid experiments. If you need to refer to, please contact the first author (KZ) or the corresponding author (YY). Requests to access these datasets should be directed to NDkzMDIwNTk2QHFxLmNvbQ==.
KZ: Methodology, Software, Visualization, Writing – original draft, Writing – review & editing. YY: Conceptualization, Methodology, Project administration, Supervision, Writing – review & editing. FQ: Writing – review & editing. LHai: Conceptualization, Resources, Supervision, Writing – review & editing. LY: Data curation, Investigation, Writing – review & editing. PZ: Project administration, Resources, Writing – review & editing. LHao: Data curation, Project administration, Resources, Writing – review & editing. YS: Data curation, Formal analysis, Funding acquisition, Writing – review & editing. YZ: Data curation, Formal analysis, Methodology, Supervision, Writing – review & editing. LL: Project administration, Resources, Supervision, Writing – review & editing. RH: Investigation, Software, Writing – review & editing. YX: Formal analysis, Investigation, Software, Writing – review & editing.
The author(s) declare that financial support was received for the research, authorship, and/or publication of this article. This research was funded by The Inner Mongolia Natural Science Foundation Major Project ‘Natural Science Foundation-Study on the Mechanism of Gully Slope Erosion in Pisha Sandstone Area of the Yellow River Basin ‘(2021ZD07); Research on key technologies of carbon sink in Hohhot section of the Yellow River Basin-Research and demonstration of key technologies for carbon sink potential evaluation and capacity improvement of typical ecosystems in Hohhot section of the Yellow River Basin (2022-She-Zhong-4-2). The basic scientific research project of colleges and universities ‘Study on the spatial and temporal variation characteristics of hydraulic erosion under different slope vegetation patterns in the coarse sand area of the Yellow River Basin ‘(BR220109); Inner Mongolia Autonomous Region directly under the university basic scientific research business fee ‘Inner Mongolia Yellow River Basin sandy coarse sand area of forest and grass vegetation quality and efficiency of technological innovation team ‘(BR22-13-10), The Inner Mongolia Autonomous Region Science and Technology Plan ‘Ecological Suitability Evaluation and Improvement Key Technology of Low Quality and Low Efficiency Plantation in the Southern Margin of Horqin Sandy Land ‘(2022YFDZ0030); Study on multi-functional management technology of larch plantation in Greater Khingan Mountains based on carbon sink (NSGKJ2022 No. 14).
The completion of this paper is inseparable from the help of YY, FQ, LHai, PZ, LHao, YS, YZ, and LL teachers. The teachers are very careful from the topic selection to the guidance of the paper. LY, RH, and YX students provide field investigation and internal processing for this paper.
The authors declare that the research was conducted in the absence of any commercial or financial relationships that could be construed as a potential conflict of interest.
All claims expressed in this article are solely those of the authors and do not necessarily represent those of their affiliated organizations, or those of the publisher, the editors and the reviewers. Any product that may be evaluated in this article, or claim that may be made by its manufacturer, is not guaranteed or endorsed by the publisher.
Aponte, C., Kasel, S., Nitschke, C. R., Tanase, M. A., Vickers, H., Parker, L. R., et al. (2020). Structural diversity underpins carbon storage in Australian temperate forests. Glob. Ecol. Biogeogr. 29, 789–802. doi: 10.1111/geb.13038
Bārdule, A., Liepiņš, J., Liepiņš, K., Stola, J., Butlers, A., and Lazdiņš, A. (2021). Variation in carbon content among the major tree species in hemiboreal forests in Latvia. Forests 12:1292. doi: 10.3390/f12091292
Chen, S., Wang, W., Xu, W., Wang, Y., Wan, H., Chen, D., et al. (2018). Plant diversity enhances productivity and soil carbon storage. Proc. Natl. Acad. Sci. 115, 4027–4032. doi: 10.1073/pnas.1700298114
Dong, L., Zhang, L., and Li, F. (2018). Additive biomass equations based on different dendrometric variables for two dominant species (Larix gmelini Rupr. And Betula platyphylla Suk.) in natural forests in the eastern Daxing’an mountains, Northeast China. Forests 9:261. doi: 10.3390/f9050261
Fang, J., Chen, A., Peng, C., Zhao, S., and Ci, L. (2001). Changes in Forest biomass carbon storage in China between 1949 and 1998. Science 292, 2320–2322. doi: 10.1126/SCIENCE.1058629
Gao, M., Zhao, G., Zhang, S., Wang, Z., Wen, X., Liu, L., et al. (2023). Priority conservation area of Larix gmelinii under climate change: application of an ensemble modeling. Front. Plant Sci. 14:1177307. doi: 10.3389/fpls.2023.1177307
Guang, Q., Hua, C., Li, Z., Xinchuang, W., Zhou, W., Lin, Q., et al. (2016). Carbon stock of larch plantations and its comparison with an old-growth forest in Northeast China. Chin. Geogr. Sci. 26, 10–21. doi: 10.1007/S11769-015-0772-Z
Guo, Z., Chen, W., Chen, Q., Liu, X., Hong, S., Zhu, X., et al. (2022). Biomass distribution pattern and stoichiometric characteristics in main shrub ecosystems in Central Yunnan, China. PeerJ 10:e13005. doi: 10.7717/peerj.13005
He, H., Zhang, C., Zhao, X., Fousseni, F., Wang, J., Dai, H., et al. (2018). Allometric biomass equations for 12 tree species in coniferous and broadleaved mixed forests, Northeastern China. PloS One 13:e0186226. doi: 10.1371/journal.pone.0186226
Hofhansl, F., Chacón-Madrigal, E., Fuchslueger, L., Jenking, D., Morera-Beita, A., Plutzar, C., et al. (2020). Climatic and edaphic controls over tropical forest diversity and vegetation carbon storage. Sci. Rep. 10:5066. doi: 10.1038/s41598-020-61868-5
Huang, J. S., and Di, X. Y. (2011). Aboveground biomass estimation model of six shrubs in Maoershan area. J. Northeast For. Univ. 5, 54–57. doi: 10.13759/j.cnki.dlxb.2011.05.037
Huang, Z. S., Fu, Y. H., and Yu, L. F. (2013). Evolution of litter standing crop and carbon pool characteristics in natural restoration of karst forest vegetation. For. Sci. Res. 1, 8–14. doi: 10.13275/j.cnki.lykxyj.2013.01.009
Jiang, P., Cao, Y., and Chen, Y. (2016). C, N, P stoichiometric characteristics of tree, shrub, herb leaves and litter in forest community of Shaanxi Province, China. J. Appl. Ecol. 27, 365–372. doi: 10.13287/j.1001-9332.201602.036
Kang, L., Gao, R. H., Zhang, D. M., Qin, L., Xin, W. W., and Leng, Y. R. (2012). Study on the decomposition rate and nutrient content of fallen wood in Da Hinggan Mountains. Inner Mongolia Forestry Science and Technology 3, 1–4. doi: 10.3969/j.issn.1007-4066.2012.03.001
Khan, D., Muneer, M. A., Nisa, Z. U., Shah, S., Amir, M., Saeed, S., et al. (2019). Effect of climatic factors on stem biomass and carbon stock of Larix gmelinii and Betula platyphylla in Daxing’anling mountain of Inner Mongolia, China. Adv. Meteorol. 2019, 1–10. doi: 10.1155/2019/5692574
Kim, C., Yoo, B. O., Jung, S. Y., and Lee, K. S. (2017). Allometric equations to assess biomass, carbon and nitrogen content of black pine and red pine trees in southern Korea. iFor. Biogeosci. For. 10, 483–490. doi: 10.3832/ifor2164-010
Kirby, K. J., Webster, S. D., and Antczak, A. T. (1991). Effects of forest management on stand structure and the quantity of fallen dead wood: some British and polish examples. For. Ecol. Manag. 43, 167–174. doi: 10.1016/0378-1127(91)90083-8
Klein, T., and Hoch, G. (2015). Tree carbon allocation dynamics determined using a carbon mass balance approach. New Phytol. 205, 147–159. doi: 10.1111/nph.12993
Lange, M., Eisenhauer, N., Sierra, C. A., Bessler, H., Engels, C., Griffiths, R. I., et al. (2015). Plant diversity increases soil microbial activity and soil carbon storage. Nat. Commun. 6:6707. doi: 10.1038/ncomms7707
Li, Q., Zhou, D. W., and Chen, X. Y. (2014). Accumulation and decomposition of above-ground litters and their roles in terrestrial ecosystems. Acta Ecol. Sin. 34, 3807–3819. doi: 10.5846/stxb201211271684
Lv, Y. Y., Yue, Y. J., Duan, J. J., Na, L., and Wu, L. Y. (2014). Analysis of the spatial point pattern of fallen wood in typical mixed forests in Daxing ‘anling, Inner Mongolia. Northwest Agric. J. 11, 204–211. doi: 10.7606/j.issn.1004-1389.2014.11.034
Mac Nally, R., Horrocks, G., and Pettifer, L. (2002). Experimental evidence for potential beneficial effects of fallen timber in forests. Ecol. Appl. 12, 1588–1594. doi: 10.1890/1051-0761(2002)012[1588:EEFPBE]2.0.CO;2
Meng, S., Jia, Q., Liu, Q., Zhou, G., Wang, H., and Yu, J. (2019). Aboveground biomass allocation and additive Allometric models for natural Larix gmelinii in the Western Daxing’anling mountains, Northeastern China. Forests 10:150. doi: 10.3390/F10020150
Meng, S., Liu, Q., Jia, Q., Zhuang, H., Qi, Y., Lu, C., et al. (2017). Spatial distribution and dynamics of carbon storage in natural Larix gmelinii forest in Daxing’anling mountains of Inner Mongolia, northeastern China. J. Mt. Sci. 14, 1633–1641. doi: 10.1007/s11629-016-3844-3
Neupane, B., and Sharma, R. P. (2014). An assessment of the effect of vegetation size and type, and altitude on above ground plant biomass and carbon. J. Agric. Crop Res. 2, 44–50.
Pan, Y., Luo, T., Birdsey, R., Hom, J., and Melillo, J. M. (2004). New estimates of carbon storage and sequestration in China’S forests: effects of age–class and method on inventory-based carbon estimation. Clim. Chang. 67, 211–236. doi: 10.1007/S10584-004-2799-5
Peng, D., Zhang, H., Liu, L., Huang, W., Huete, A. R., Zhang, X., et al. (2019). Estimating the aboveground biomass for planted forests based on stand age and environmental variables. Remote Sens. 11:2270. doi: 10.3390/rs11192270
Pregitzer, K. S., and Euskirchen, E. S. (2004). Carbon cycling and storage in world forests: biome patterns related to forest age. Glob. Chang. Biol. 10, 2052–2077. doi: 10.1111/j.1365-2486.2004.00866.x
Qi, G., Wang, Q. L., Wang, X. C., Lin, Q. I., Wang, Q. W., Ye, Y. J., et al. (2011). Vegetation carbon storage of Larix gmelinii plantation in Daxing 'anling forest region. Appl. Ecol. J. 2, 273–279. doi: 10.13287/j.1001-9332.2011.0072
Qi, G., Wang, Q., Wang, X., Yu, D., Zhou, L., Zhou, W., et al. (2013). Soil organic carbon storage in different aged Larix gmelinii plantations in great Xing' an mountains of Northeast China. J. Appl. Ecol. 24, 10–16. Available at: http://www.cjae.net/EN/Y2013/V24/I1/10
Sun, W., and Liu, X. (2019). Review on carbon storage estimation of forest ecosystem and applications in China. For. Ecosyst. 7, 1–4. doi: 10.1186/s40663-019-0210-2
Terrer, C., Phillips, R. P., Hungate, B. A., Rosende, J., Pett-Ridge, J., Craig, M. E., et al. (2021). A trade-off between plant and soil carbon storage under elevated CO2. Nature 591, 599–603. doi: 10.1038/s41586-021-03306-8
Wang, B., Hao, S., and Zhang, Q. (2023). Protection mechanisms and influencing factors of soil organic carbon pools in the Larix gmelinii forests. Ecol. Indic. 150:110242. doi: 10.1016/j.ecolind.2023.110242
Wang, W., Su, D., Qiu, L., Wang, H., An, J., Zheng, G., et al. (2013). Concurrent changes in soil inorganic and organic carbon during the development of larch, Larix gmelinii, plantations and their effects on soil physicochemical properties. Environ. Earth Sci. 69, 1559–1570. doi: 10.1007/s12665-012-1990-7
Wang, H., Wang, W., Ling, Q., Su, D., Jing, A., Zheng, G., et al. (2012). Differences in biomass,litter layer mass and SOC storage changing with tree growth in Larix gmelinii plantations in Northeast China. Acta Ecol. Sin. 32, 833–843. doi: 10.5846/STXB201108311276
Wang, B., Zhang, P. J., and Zhang, Q. L. (2021). Characteristics of the soil aggregate and its organic carbon in different Larix gmelinii forest types. J. Nanjing For. Univ. 45, 15–24. doi: 10.12302/j.issn.1000-2006.202009067
Wani, N. R., and Qaisar, K. N. (2014). Carbon per cent in different components of tree species and soil organic carbon Pool under these tree species in Kashmir Valley. Curr. World Environ. 9, 174–181. doi: 10.12944/CWE.9.1.24
Webb, E. E., Heard, K. E., Natali, S. M., Bunn, A. G., Alexander, H. D., Berner, L. T., et al. (2017). Variability in above- and belowground carbon stocks in a Siberian larch watershed. Biogeosciences 14, 4279–4294. doi: 10.5194/BG-14-4279-2017
Wei, Y. W., Yu, D., Lewis, B. J., Zhou, L., Zhou, W., Fang, X., et al. (2014). Forest carbon storage and tree carbon pool dynamics under natural forest protection program in northeastern China. Chin. Geogr. Sci. 24, 397–405. doi: 10.1007/s11769-014-0703-4
Wei, Y. W., Zhou, W. M., Zhou, L., Yu, D. P., Zhou, Y. B., Qin, S. J., et al. (2015). Carbon storage and carbon pool allocation characteristics of natural larch forest in larix dahurica. Acta Ecol. Sin. 35, 189–195. doi: 10.5846/stxb201407271520
Xiao, R., Man, X., and Duan, B. (2020). Carbon and nitrogen stocks in three types of Larix gmelinii forests in Daxing’an mountains, Northeast China. Forests 11:305. doi: 10.3390/f11030305
Yaozhan, X., and Mingxi, J. (2015). Forest carbon pool characteristics and advances in the researches of carbon storage and related factors. Acta Ecol. Sin. 35, 926–933. doi: 10.5846/STXB201304190745
Ying, J., Weng, Y., Oswald, B. P., and Zhang, H. (2019). Variation in carbon concentrations and allocations among Larix olgensis populations growing in three field environments. Ann. For. Sci. 76, 1–14. doi: 10.1007/s13595-019-0877-0
Keywords: Larix gmelinii, biomass, forest ecosystem, forest age, carbon storage and allocation, model simulation
Citation: Zhao K, Yue Y, Qin F, Hai L, Yi L, Zhao P, Hao L, Shu Y, Zheng Y, Li L, He R and Xu Y (2024) Carbon storage and carbon pool characteristics of Larix gmelinii forest in Daxing’anling, Inner Mongolia, China. Front. For. Glob. Change. 7:1419023. doi: 10.3389/ffgc.2024.1419023
Received: 17 April 2024; Accepted: 22 May 2024;
Published: 31 May 2024.
Edited by:
Quanhou Dai, Guizhou University, ChinaCopyright © 2024 Zhao, Yue, Qin, Hai, Yi, Zhao, Hao, Shu, Zheng, Li, He and Xu. This is an open-access article distributed under the terms of the Creative Commons Attribution License (CC BY). The use, distribution or reproduction in other forums is permitted, provided the original author(s) and the copyright owner(s) are credited and that the original publication in this journal is cited, in accordance with accepted academic practice. No use, distribution or reproduction is permitted which does not comply with these terms.
*Correspondence: Yongjie Yue, d29sb25neXVlQDEyNi5jb20=
Disclaimer: All claims expressed in this article are solely those of the authors and do not necessarily represent those of their affiliated organizations, or those of the publisher, the editors and the reviewers. Any product that may be evaluated in this article or claim that may be made by its manufacturer is not guaranteed or endorsed by the publisher.
Research integrity at Frontiers
Learn more about the work of our research integrity team to safeguard the quality of each article we publish.