- 1Faculty of Forestry and Wood Sciences, Czech University of Life Sciences, Prague, Czechia
- 2ICAR-Indian Institute of Rice Research (IIRR), Hyderabad, India
Plant metabolism response to insect herbivores is the central theme of this publication. Genetically uniform individuals of European aspen (Populus tremula) were exposed to recurrent feeding by spongy moths (Lepidoptera) at specific time intervals. Changes in physiology, contents of phenolics and saccharides were quantified over the first hour. The unconventional experiment design, integrating analytical methods, and timeline led to the revealing of unexpected dynamics in plant metabolism. The time interval between herbivory initiation and sample collection revealed a pivotal moment, with induced defense activating strongly after 5 min of chewing resulting in an increase in catechin and procyanidin B1. After 10 min, a shift to a tolerant strategy occurs and induced substance concentrations return to control levels. Delayed physiological response was recorded as the first significant difference in transpiration between affected and nonaffected plants and was found after 10 min. A different strategy in exploitation of saccharides after spongy moths infestation was applied because the pool of selected saccharides was rising in the leaves but decreasing in the roots. Placing our results in the context of existing knowledge highlights the uncertain conceptual basis behind the often rigid and definitive classifications in induced plant defense or tolerance strategy.
Introduction
From a paleontological perspective, the origins of interactions between taxonomic kingdoms of plants (Plantae) and animals (Animalia), specifically within the phylum Arthropoda, extend back to the geological period of the early Devonian, as evidenced by findings in the studies of Labandeira (2007, 2013) and Fürstenberg-Hägg et al. (2013). The early Devonian period is defined by a temporal interval ranging from approximately 397 to 407 million years ago (Gerrienne et al., 2011). While phytophagous insect species, during the course of this coevolutionary relationship, adapted to exploit their host plants, plants simultaneously developed defense systems in response to herbivore attacks (Anderson and Mitchell-Olds, 2010; Johnson, 2011).
Insect herbivory triggers a cascade of processes occurring in plant tissues that can be qualified and quantified. When insects interact with plants, there is an induction of: defense proteins (Haruta et al., 2001; Fürstenberg-Hägg et al., 2013; War et al., 2021); volatile organic compounds (Holopainen and Gershenzon, 2010; Rosenkranz and Schnitzler, 2016); secondary metabolites (Smith, 2007; Wink, 2018; Khare et al., 2020); changes in gene expression (Vogel et al., 2014; Birnbaum and Abbot, 2020); changes in the level of photosynthesis and gas exchange (Garcia and Eubanks, 2018); and substance transport and resource allocation (Gomez et al., 2012; Schultz et al., 2013).
The outcome of hundreds of millions of years of coevolution between insects and plants suggests that both participants have equipped themselves with the ability for rapid temporal response. Decisions made by insects in selecting a host plant occur on a time scale of tens to hundreds of milliseconds (Bruce and Pickett, 2011). Moreover, scent plumes with an uneven structure, encounter the chemical molecules of the plants only for fractions of a second (Webster et al., 2010). However, the range of descriptions of plant responses to herbivory over time is limited. Insect herbivory in plant tissues causes the accumulation of reactive oxygen species and changes in Ca2+ concentrations in the cytoplasm, thereby triggering a chain of defense reactions (Pandey et al., 2000; Medvedev, 2005). The earliest is a change in membrane potential at the plasma membrane, immediately followed by changes in intracellular Ca2+ concentration and H2O2 formation. Within minutes, the kinases and phytohormones jasmonic acid (JA) and salicylic acid (SA) are detectable and gene activation and subsequent metabolic changes are first noticeable after about 1 h (Maffei et al., 2007).
The focal point of any discussion on carbon allocation in response to herbivory is photosynthesis, which serves as the primary source of nearly all saccharides in green plants (Zhou et al., 2015). Although the impacts of herbivory on photosynthetic efficiency are generally perceived as negative (reducing efficiency), a relatively recent meta-analysis (Garcia and Eubanks, 2018) identified 67 plant species that exhibit a certain degree of overcompensation in response to insect herbivory. Additionally, arguments advocating the implementation of management strategies to enhance market yield using insect herbivores are documented (Poveda et al., 2013, 2017). Spongy moth (Lymantria dispar) caterpillars have a substantial impact on the net photosynthetic rate (Pn) of poplar (Populus sp.) leaves and these attacks lead to reduced photosynthetic activity due to extensive defoliation, ultimately resulting in a decrease in the leaf area available for photosynthesis (Zhang et al., 2022). Hindering of stomatal conductance (Gs) and transpiration (Tr) can potentially disrupt the equilibrium between water loss and carbon dioxide absorption, and this not only impacts the water-use efficiency of poplar trees but also affects their overall physiological performance (Pilipoviš et al., 2015). Nevertheless, the response in Pn to a chewing insect is a dynamic process depending on the scale of damage. Even moderate feeding with removing of 10% of the leaf area results in 12% Pn reduction in oak (Quercus sp.) (Copolovici et al., 2017). Intracellular CO2 concentration (Ci) increases slightly in oak leaves in response to moth chewing even though Gs decreased about 50% similarly to Pn (Copolovici et al., 2017).
As Delaney (2008) points out, many studies have focused on whole plant processes and fewer studies have included analysis of changes at the physiological level – i.e. gas exchange, photosynthetic changes. According to the studies of Visakorpi et al. (2020) and Fyllas et al. (2022), a combination of several analytical approaches appears to be logically consistent for further revealing the metabolic responses of plants to the attacks of insect herbivores. Gomez et al. (2012) contribute valuable insights into the complex relationships within the primary metabolism of both aboveground and belowground plant components, emphasizing critical aspects of resource allocation essential for plant resistance and tolerance. Primary metabolism assumes a key role in plant resistance and tolerance, closely linked to the efficiency of the formation of defense substances (constitutive defense/induced defense). This link arises from the fact that products of primary metabolism, namely amino acids and saccharides, act as precursors and substrates for the biosynthesis of defense metabolites (Hanik et al., 2010a). An approach describing the dynamics of physiological and biochemical traits over time during herbivore feeding is still missing because, as it is well known, plant-insect interactions are both dynamic processes.
The research’s uniqueness of the present study stems from exploring the interplay between biochemical and physiological responses, primary and secondary metabolism, specifically within the initial hour following an insect attack. By concentrating on the timing of these responses, we aim to offer a comprehensive understanding of the complex interactions between European aspen (Populus tremula) and polyphagous spongy moth (Lymantria dispar).
Materials and methods
Plant material
For the minimization of genotype influence and standardization of experimental conditions, genetically uniform individuals of Populus tremula produced through somatic embryogenesis were employed. Populus tremula seeds were selected as the starting plant material for the experiment. The seeds were obtained through controlled crossing of parent trees aged 40–50 years, located in the Czech Republic, specifically in Sušice (Svatobor) and Krušné hory (Fláje). The controlled crossing took place in early spring 2019. Within 5–7 days after seed collection, the seed material was utilized for in vitro propagation. A total of 218 seedlings sprouted and, out of them, individual number 22 was chosen due to its superior performance in in vitro culture.
Seeds of P. tremula were washed in 200 mL distilled water with 1–2 drops of Tween 20® for 10–15 min, then sterilized in 0.1% HgCl2 for 6 min. After rinsing, seeds were placed in jars with Murashige and Skoog (MS) medium solidified with Danish® agar and supplemented with myo-inositol and 6-benzylaminopurine (BAP). The pH-adjusted medium was autoclaved, and explants were cultivated under 16/8 h light/dark with a temperature of 22 ± 1 / 20 ± 1°C. Germination occurred within 1–3 weeks. Shoots were subcultured every 2–3 weeks until sufficient material was obtained. In vitro rooting was done on segments with at least three buds using half-strength MS medium supplemented with indole-3-butyric acid (IBA). Roots developed after about 4 weeks, and after 6–8 weeks, rooted shoots were transferred ex vitro. Rooted shoots were washed and transferred to sterile substrate in plastic pots, treated with Previcur Energy®, and cultivated under controlled conditions. Humidity was gradually decreased, and plants were fertilized bi-weekly during growth. Forty elite individuals were selected from the genetically uniform in vitro culture based on phenotypic characteristics. These plants were transplanted into round flowerpots with a diameter and height of 20 cm each during the transfer from in vitro to ex vitro conditions at the somatic embryogenesis laboratory. The soil was prepared by sterilizing the substrate through steam treatment, and the plants were then placed into growth chambers. A specialized commercial mixture for sowing and growing plants was used as the soil substrate. The mixture is carefully formulated from a blend of selected light and dark peat, adjusted to achieve the desired pH level. Perlite is then added as an additional component. Furthermore, this substrate is enriched with a comprehensive range of essential and trace nutrients by adding fertilizers (Forestina, Czech Republic). The start of the experiment took place when the plants reached the age of 6 months, the leaves were fully mature and the individuals were approximately 1.2 m high. Throughout the duration of the experiment, all Populus individuals showed good health and growth was observable.
Insect breeding
The collection of spongy moths (Lymantria dispar) was delivered by Institute of Forest Entomology, Forest Pathology and Forest Protection at the University of Natural Resources and Life Sciences, Vienna from sterile laboratory cultures. Upon hatching, the larvae were provided with a nutritionally balanced agar diet (Lymantria dispar agar, Southland products Inc., United States) in sterile Petri dishes.
Lymantria dispar eggs were placed in sterile Petri dishes in a climatic chamber with a day and night regime (12 h dark/12 h light) with a temperature oscillation during the day of 24°C and a drop to 20°C at night. The moment the eggs started to hatch into caterpillars, they were given nutritionally balanced boiled agar. Once every 2–3 days, the caterpillars were transferred to new, clean Petri dishes in a sterile environment.
In the experiment, caterpillars were used after the fourth molting. To enhance feeding activity, they were incubated in darkness and deprived of food for 48 h, considering their nocturnal behavior (Hajek, 2001).
Experimental facility
For increased reproducibility and comparability of results, standardization of experimental conditions was implemented using growth chambers: Step-In FytoScope FS-SI (Photon Systems Instruments, Drasov, Czech Republic). The growth chambers were set to simulate optimal environmental conditions, including a humidity level of 75%, an average Photosynthetic Photon Flux Density of 250 μmol.m2.s−1, a CO2 concentration of 420 ppm, and a light–dark cycle of 2 h of dawn, 10 h of light, 2 h of twilight, and 10 h of darkness.
An Agilent 1,290 Infinity II (Agilent, USA) liquid chromatography system coupled with an Agilent 6,546 LC/MS quadrupole time-of-flight (qTOF) system (Agilent, USA) was used for non-volatile compounds analysis.
For gasometrical measurement, an open portable photosynthesis system with an infrared gas analyzer LI-6400 XT (LICOR, Lincoln, NE, USA) was used. The light of 1,500 μmol.m−2 s−1 overlapping the point of light saturation (usually round 450 μmol.m−2 s−1) has been measured to obtain a net photosynthetic rate (Pn), transpiration (Tr), stomatal conductance (Gs), and internal-to-ambient CO2 concentration ratio (Ci/Ca). The measurement referred to the ambient CO2 concentration of 420 μmol.m−2 s−1. A standard leaf chamber with a red/blue LED light source was used. The samples were taken from 11 am to 2 pm. Measurements were performed inside the chamber so as not to change microclimatic conditions.
Experimental design
Out of the initial pool of 40 individuals, which underwent a 6-month incubation period under optimal conditions in the growth chamber, the top 20 elite individuals were chosen for the experiment. For clarity in understanding the methodology, the experiment design (Figure 1) and procedures will be detailed for a single individual plant among the selected 20. The experimental framework drew on previous research such as the work of Boeckler et al. (2013) and Stevens and Lindroth (2005), which provided empirical support for the chosen approach.
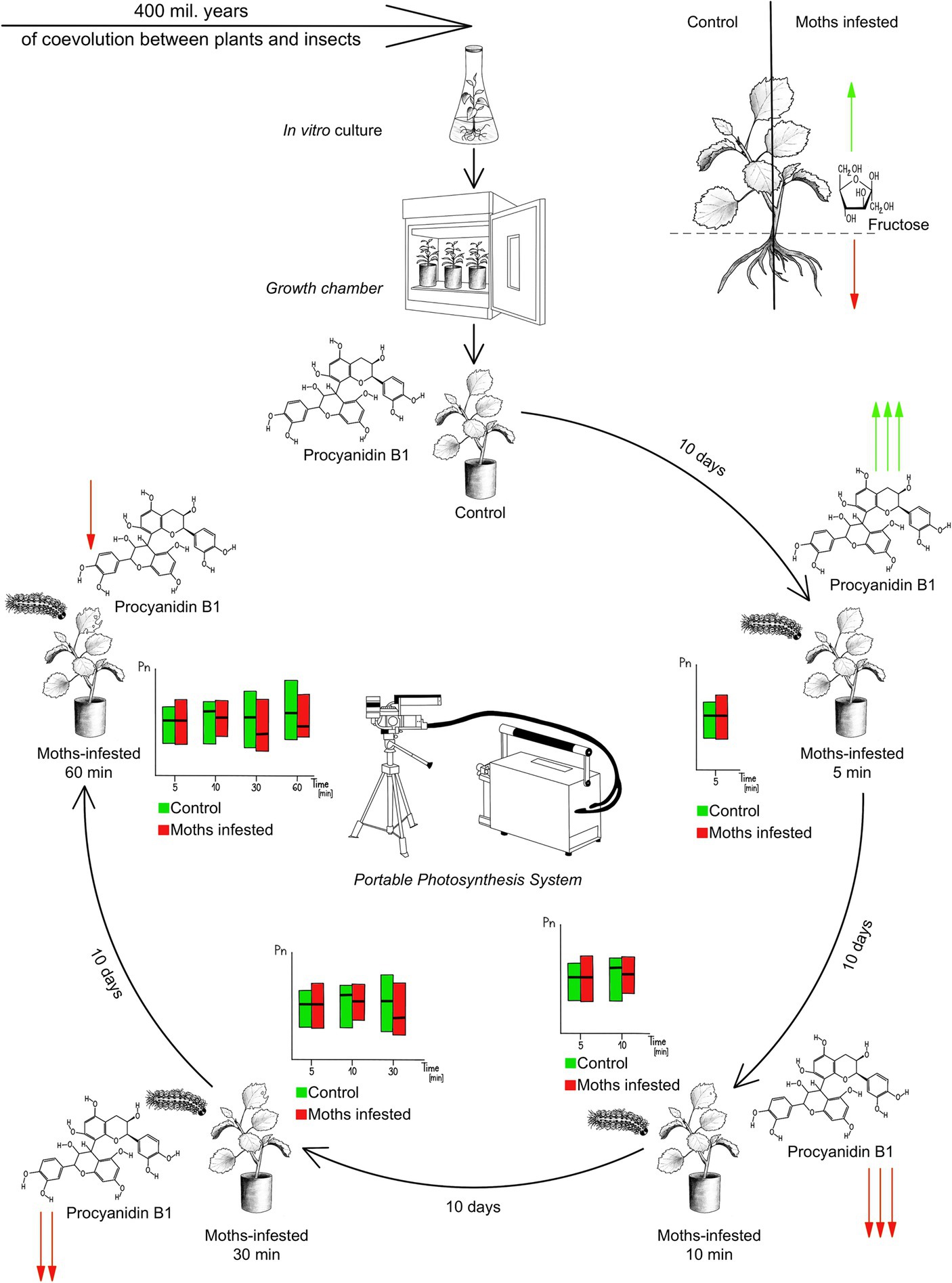
Figure 1. Experimental design. Genetically uniform poplar seedlings from in vitro culture were transplanted into pots and maintained under controlled conditions. The cycle of one plant within the experiment is shown. Early events in plant metabolism were investigated in time segments after 5, 10, 30, 60 min continuous feeding of Lymantria dispar. There were 10-day intervals between individual segments for plant recovery. Green arrows and their number graphically show the rate of increase in concentration, red arrows show the decrease in procyanidin B1 concentration over time.
The biochemical response of the Populus tree was investigated at four time moments in the first 60 min of moth caterpillar insects feeding on one individual poplar leaf. In the first phase, the selected individual was isolated for 10 days after selection (Figure 1), in order to stabilize the stress reactions to handling. Subsequently, measurements were conducted on a specific fully mature leaf located in the midsection of the trunk, using an open gasometric system. The spongy moths were put on the leaf using aseptic entomological tweezer from the Petri dishes and after chewing in individual time segments removed back from the leaf. The investigated leaves were always under the full sunlight with defined light in the growth chambers (LI-6400 XT). Immediately after the measurement, the leaf was aseptically cut with sterile scissors and placed in a sterile 50 mL test tube, which was pre-frozen in a liquid nitrogen bath. After closing the falcon tube in which the leaf was inserted, it was immediately placed in a bath of liquid nitrogen. This was followed by a 10-day rest period, allowing the metabolism to stabilize after leaf cutting.
In the second phase, another leaf of comparable quality and age was selected, and three spongy moths individuals were introduced. The precise moment of the first bite was recorded, and after 5 min of continual feeding, the caterpillars were removed. The leaf was promptly sealed in the LI-6400 XT measurement chamber. Gas exchange was assessed on intact leaves via a chamber measuring 2×3 cm, capturing CO2 uptake from the entire area. The leaf completely filled the chamber area to ensure accurate gas exchange measurements. Following completion of measurements, the leaf was cut with sterile scissors and immediately placed in a 50 mL test tube, subsequently stored in liquid nitrogen. Another 10-day period was granted to restore metabolic homeostasis. In order to eliminate the influence of the circadian rhythm of the plants, the collection of samples was always carried out at the same time of a day (i.e., from 11 am to 2 pm) at an interval of 3 h.
Subsequent stages replicated the entire process, with the only variation being the measurement time, set at 5, 10, 30, and 60 min from the first bite.
In the final step, root samples were obtained from the designated individual, both as a control and after 60 min of feeding. Throughout, the methodology was strictly followed to ensure active caterpillar feeding and their presence on the selected leaf. This procedural approach was consistently applied to all selected individuals and in all time segments. Throughout the experiment, everything was done in a growth chambers environment to eliminate the effect of handling the plant or the effect of different spectral composition of light on the plant. A total of 4 growth chambers were used, which were appropriately combined in order to eliminate the influence of intraspecific chemical communication between poplars. Before starting the laboratory work, the samples were stored in a −80°C cooling box.
For root saccharides analysis, roots were collected simultaneously with leaf control samples. Each plant was carefully removed from its pot, and approximately 50 mg of roots of various sizes were collected using sterile scissors. Following collection, the roots were promptly washed in demineralized water and stored in a microtube in liquid nitrogen for immediate preservation. The root samples were processed in the same way when the sample was harvested after 60 min of continuous feeding, when the leaf was harvested first and then the roots.
Chemical analysis
Extraction of phenolic compounds in leaves
A freeze-dried and homogenized sample (10 mg) was placed into a test tube, followed by the addition of 0.5 mL of 70% chilled methanol. After vortexing for 30 s, the test tube was then immersed in an ultrasonic bath with ice for a duration of 10 min. The resultant solution was centrifugated at 13,000 rpm and 4°C for 10 min. Prior to LC–MS-qTOF analysis, the supernatant was filtered using a 0.22 μm PTFE filter. After 5 μL in concentration of 25 μg mL−1 of internal standard indole 3-acetic acid was added. All sample handling procedures were conducted on ice. The prepared samples were stored at a temperature of −80°C.
Extraction procedure for determination of saccharides in leaves and roots
Freeze-dried homogenized sample (30 mg) was introduced into a 2-mL test tube, and 1.5 mL of methanol:water (80:20 v/v) was added. The tube was vortexing and placed into a thermoshaker for 30 min at 50°C and 1,000 rpm. Then, the samples underwent centrifugation at 12,500 rpm for 10 min. The resulting supernatant was carefully collected and subsequently filtered through a PVDF syringe filter (0.22 μm) prior to LC-qTOF-MS/MS analysis (Šulc et al., 2021).
LC-qTOF-MS/MS saccharides analysis in leaves and roots
Saccharides analysis was performed on Agilent 1,290 Infinity II liquid chromatography system, coupled with an Agilent 6,546 LC/MS quadrupole time-of-flight (qTOF) detector (Agilent, USA). for LC-qTOF-MS/MS analysis. Chromatographic separation was executed on a Supel Co apHera NH2 Polymer column (150×2 mm, 5 μm) maintained at 30°C. The mobile phase consisted of acetonitrile (A) and water (B). The gradient elution started at 80:20 (A:B) for the mobile phase, transitioning to 55:45 (A:B) from 0.5 to 13 min, and returning to 80:20 (A:B) from 14 to 15 min, at a flow rate of 0.2 mL min−1. The injection volume was set to 1 μL. The system operated in negative ionization mode (Madsen et al., 2015).
Optimized QTOF parameters, established using glucose, sucrose, fructose, and mannitol standards, were as follows: scan range of 100–1,000 m/z, drying gas temperature of 280°C, sheath gas flow rate at 12.0 L/min, sheath gas temperature of 400°C, capillary voltage set to 2.0 kV, fragmentor at 120 V, and collision energy at 10, 20, and 40 eV. MS/MS data were acquired within a scan range of 50–800 m/z. Throughout the analysis, reference masses at 112.9855 m/z and 922.0098 m/z were continuously monitored for mass correction. Agilent Mass Hunter Acquisition software was employed for data collection, while Qualitative Analysis 10.0 and Q-TOF Quantitative analysis tools were utilized for data analysis (Madsen et al., 2015). External calibration curves using standards of target compounds (fructose, glucose, sucrose and mannitol) were used for quantification.
LC-qTOF-MS/MS analysis of polyphenolic compounds in leaves
The same instrument was used for phenolics analysis as in the case of saccharide analysis, but with Zorbax Eclipse Plus C18 column (2.1×50 mm, 1.8 μm) (Agilent, United States). Mobile phase A contained 0.05% formic acid, while mobile phase B consisted of acetonitrile. The gradient elution protocol was as follows: 0–0.1 min, 95% A; 0.1–8 min, 72% A; 8–9.1 min, 25% A; 9.1–11 min, 95% A. The mobile phase flowed at a rate of 1.1 mL min−1, and the column temperature was maintained at 35°C. A 1 μL injection volume was used, and the system was operated in negative ionization mode.
Prior to analysis, qTOF parameters were optimized using standards. The qTOF parameters were set as follows: scan range of 100–1,000 m/z; drying gas temperature of 350°C; sheath gas flow rate at 12.0 L min-1; sheath gas temperature of 400°C; capillary voltage set to 5.0 kV; nozzle voltage at 0.9 kV; fragmentor set to 140 V; collision energy set at 10, 20, and 40 eV. MS/MS data were acquired within a scan range of 50–800 m/z, with a retention time window of 0.5 min, an isolation window of 1.3 amu, and an acquisition rate of 2 spectra per second. Throughout the analysis, reference masses of 112.9855 m/z and 966.0007 m/z were continuously monitored for mass correction.
Agilent Mass Hunter Acquisition software was used for data collection, while data analysis was performed using Mass Hunter Qualitative Analysis 10.0 and Q-TOF Quantitative analysis tools (Agilent, USA). External calibration curves using standards of target compounds (catechin. Epigallocatechin, ferulic acid, chlorogenic acid, procyanidin B1, gallic acid, rutin, kaempferol, quercetin, taxifolin) were used for quantification.
Statistical analysis
For the statistical analyses and data visualization, R statistical software (R Core Team, 2021) was used.
Since a single plant was measured multiple times, paired t-test and linear mixed models for repeated measures were employed.
Saccharides from roots were analyzed using paired t-test (as default by t.test function from R’s stat package).
Linear mixed models were fitted using ASReml-R v4.1. For gasometry data, the fixed effects were specified as:
where Time has 4 levels of 5 min, 10 min 30 min and 60 min of infestation and Treatment has two levels: infested and control.
Random factors were specified in a model as:
Where IDplant has 20 levels for each plant.
For each Time:Treatment combination, mean values, standard errors, and significance for pairwise comparison were extracted using predictPlus function form asremlPlus package. p-values were then adjusted using the false discovery rate method. The effect size of differences was calculated using Cohen’s d approach (difference of means divided by pooled standard deviation; so d = 1 means that means are 1 standard deviation apart).
Residuals were checked for normality and constancy of variances.
For phenolic compounds the fixed effects were specified as:
where Time has five levels of 0 (pre-infestation control), 5 min, 10 min, 30 min, and 60 min of infestation.
Repeated measures were tackled specifying heterogenous correlation in residuals specifying:
Where IDplant has 20 levels for each plant.
Again, the significance for pairwise comparison was extracted using predictPlus function form asremlPlus package. p-values were then adjusted using FDR method. Effect size of differences was calculated using Cohen’s d approach.
Results
In leaves, the concentration of fructose and glucose together with mannitol rose in moth-infested treatment in comparison to the control during the whole investigated period of 60 min. The concentration of sucrose – the most important saccharide - did not change in the investigated period and reached 253 ± 13 μg/g in moth-infested treatment and 238 ± 49 μg/g in the control group (Figure 2). Excluding sucrose, the differences between the control and moth-infested treatment were statistically significant from 10 min for glucose and mannitol. The concentrations of both monosaccharides were 5x higher after 60 min of chewing than in the control group. These shifts indicate possible saccharides transport between the roots and leaves.
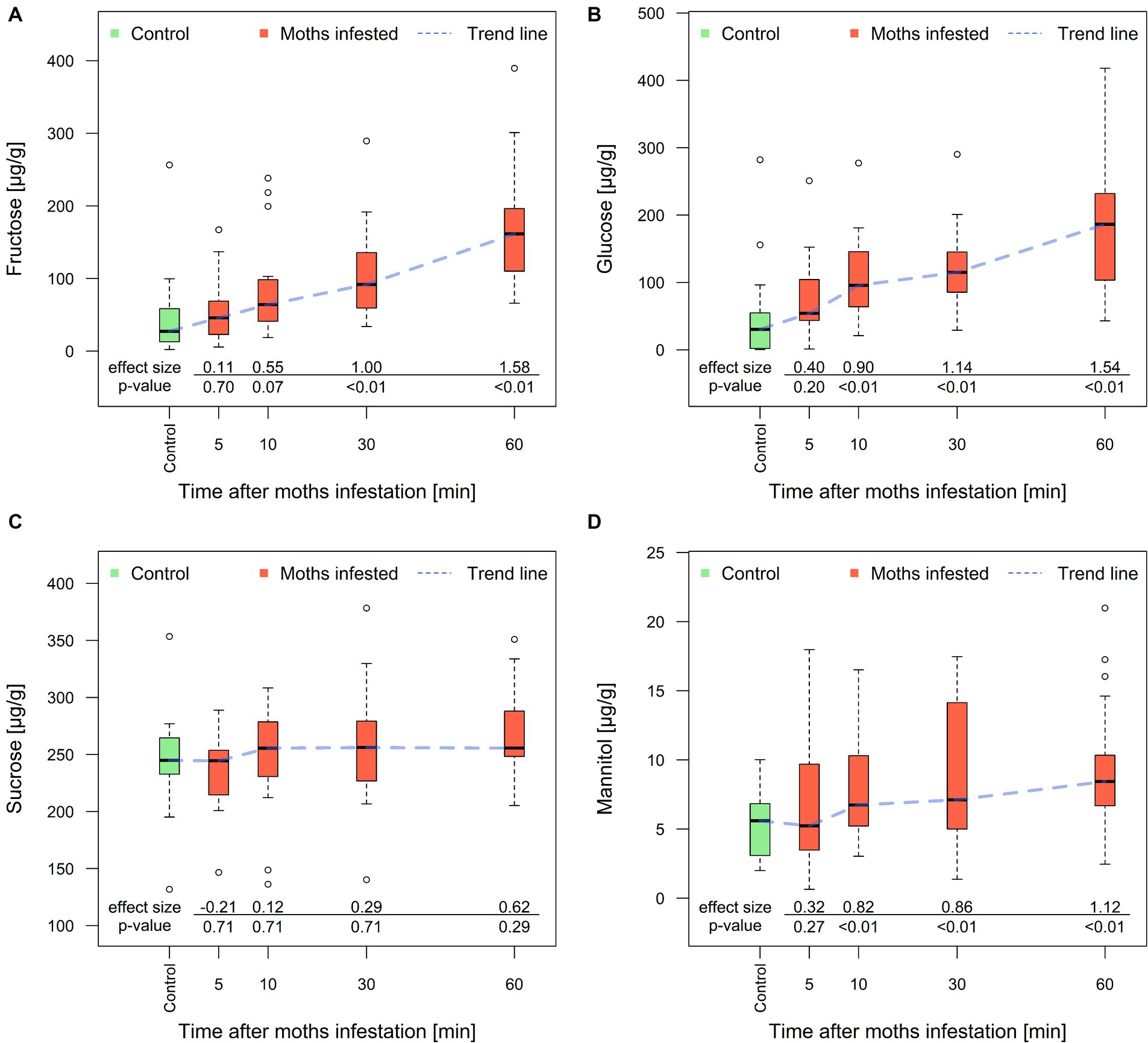
Figure 2. Concentration of fructose (A), glucose (B), sucrose (C), and mannitol (D) in poplar leaves obtained after 5, 10, 30, and 60 min of spongy moth chewing. The Y-axis represents the relative shift from the control group on a natural logarithmic (ln) scale. Thick line in the box represents median values, the box range represents lower and upper quartile value, whiskers minimum and maximum values, and circles extreme values. The effect size is a quantitative measure of the magnitude of the experimental effect (Cohen’s d).
Regarding the response of root sugar concentrations to hourly feeding by spongy moths, the results are shown in the graph (Figure 3). Sugars involved in the fructose and mannose metabolic pathway (namely glucose, fructose, and mannitol) showed a consistent pattern. Specifically, root fructose concentration significantly decreased from the control level of 66 ± 35 μg/g after feeding to 33 ± 23 μg/g, reflecting a reduction to 49% of the control value. Glucose showed an 85% decrease from the original concentration in the moth-infested treatment, while mannitol decreased to 87.5% of its concentration. In the context of the galactose metabolic pathway, there was a significant decrease in sucrose, which dropped from control values of 418 ± 66 μg/g to 368 ± 29 μg/g (12% difference between treatment and control group) after 60 min of individual leaf feeding by spongy moth.
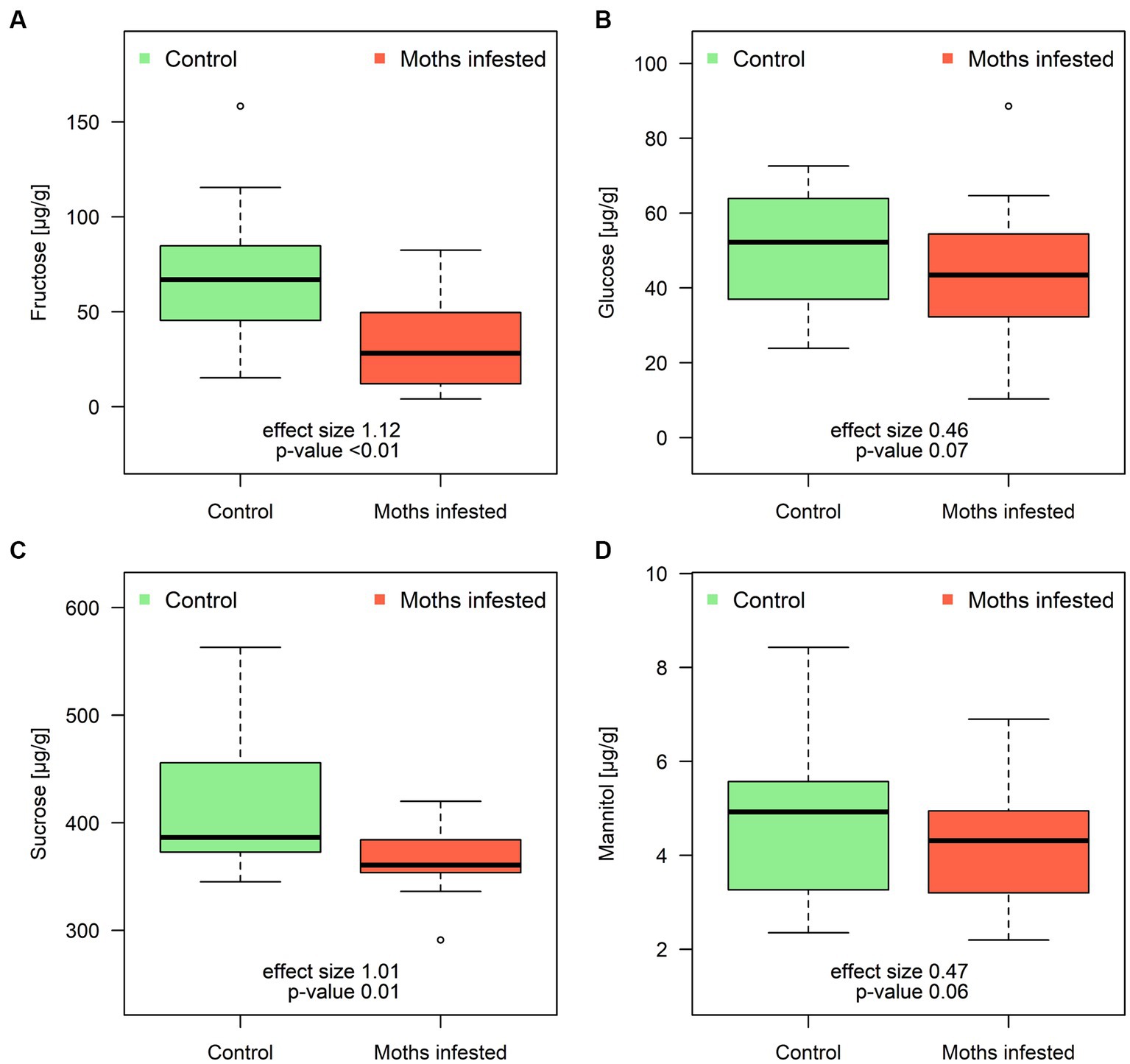
Figure 3. Concentration of fructose (A), glucose (B), sucrose (C), and mannitol (D) in poplar roots obtained after 60 min of spongy moth chewing. Thick line in the box represents median values, the box range represents lower and upper quartile value, whiskers minimum and maximum values, and circles extreme values. The effect size is a quantitative measure of the magnitude of the experimental effect (Cohen’s d).
Physiological response of Populus to the moths
The physiological response of poplar to chewing insects was very fast and maintained even after the investigated time. The Pn in damaged plants was comparable to the control group at 5 and 10 min of feeding. Nonetheless, compared to the control, a substantial reduction (p < 0.01) in Pn was noted following 30 min of insect chewing (Figure 4A). The control group maintained Pn at 11 ± 4 μmol.m−2 s−1 but the group with spongy moth damage only reached 9.9 ± 3.7 μmol.m−2 s−1. Similar trends were observed for Gs (Figure 4B) with a significant drop off at 30 min (0.50 ± 0.23 mol.m−2 s−1 in control group and 0.46 ± 0.23 mol.m−2 s−1 in moth-infested group, respectively). The significant decrease in Tr (Figure 4C) started even after 10 min of chewing (2.66 ± 0.89 mmol.m−2 s−1 and 2.58 ± 0.71 mmol.m−2 s−1, respectively). The reduction in Pn, Gs, and Tr remained similar at 30 and 60 min, ranging between 8 and 12% when compared to the control group. Interestingly, no significant changes were observed in the intercellular CO2 concentration (Ci) (Figure 4D), which implies that the ratio of Ci to ambient CO2 concentration (Ca) remained constant throughout the entire investigation period (0.85 ± 0.05 μmol CO2 mol−1). The stable Ci/Ca ratio indicates that the impact of a moth feeding on the studied physiological processes was not mediated through alterations in intercellular CO2 concentration.
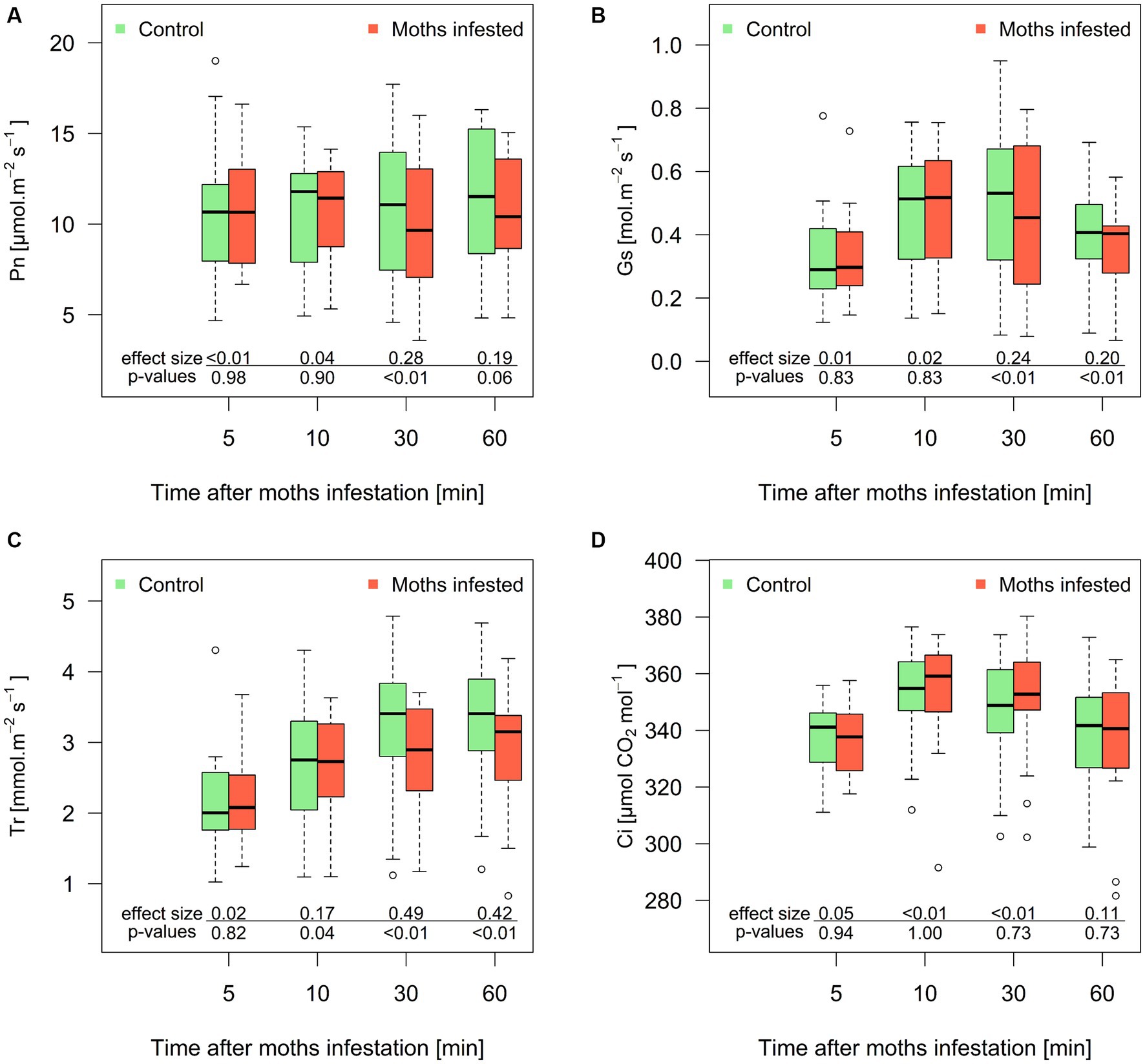
Figure 4. Course of net photosynthesis (A), stomatal conductance (B), transpiration (C), and intracellular CO2 concentration (D) in poplar leaves obtained after 5, 10, 30, and 60 min of spongy moth chewing. Values of the Y axis represent the relative shift from the control group. Thick line in the box represents median values, the box range represents lower and upper quartile value, whiskers minimum and maximum values, and circles extreme values. The effect size is a quantitative measure of the magnitude of the experimental effect (Cohen’s d).
Biochemical response of Populus to the moths
Three distinct patterns of phenolic compound production were observed in the moth-infested leaves, where the concentration of different phenolic compounds changed over time in three different ways (Figure 5) when compared to the control group:
1. The first group (Figure 5A) consisting of procyanidin B1, kaempferol, catechin, epigallocatechin, chlorogenic acid, and rutin increased its concentration several times higher after 5 min of chewing than in the control group. The standard deviations (e.g., catechin and procyanidin B1) increased almost 10 times compared to their concentration in the moths-infested treatment immediately after 5 min of chewing. There was a significant increase (43%) in chlorogenic acid (639 ± 275 μg/g and 914 ± 275 μg/g) and kaempferol (0.16 ± 0.08 μg/g and 0.28 ± 0.18 μg/g, an increase of 75% when compared to control group). Subsequently, this group of substances began to decrease in concentration from 5 min to 60 min reaching control level. In addition to catechin, chlorogenic acid and procyanidin B1 showed lower concentrations than the control group.
2. The second group (Figure 5B) contained quercetin and ferulic acid; these two phenolic compounds had exactly the inverse course in investigated time than the previous group. After the start of feeding, the concentrations started to decrease, reaching a minimum value at 30 min after the start of feeding. Between 30 and 60 min, increases in the concentration of both these phenolic compounds were recorded, reaching approximately half the concentration compared to the control group (30.7 ± 7.8 μg/g). Concentration of ferulic acid was lower during the whole investigated periods when compared to control group. Significant differences were recorded after 10 min (24.3 ± 7.2 μg/g, a decrease of 20%), 30 min (21.7 ± 6.1 μg/g, a decrease of 42%), and 60 min (23.5 ± 8.1 μg/g, an increase of 8%).
3. The third group (Figure 5C) of analyzed phenolic compounds contains gallic acid and taxifolin; the concentration of these substances was stable and did not show any significant response during investigated time segments.
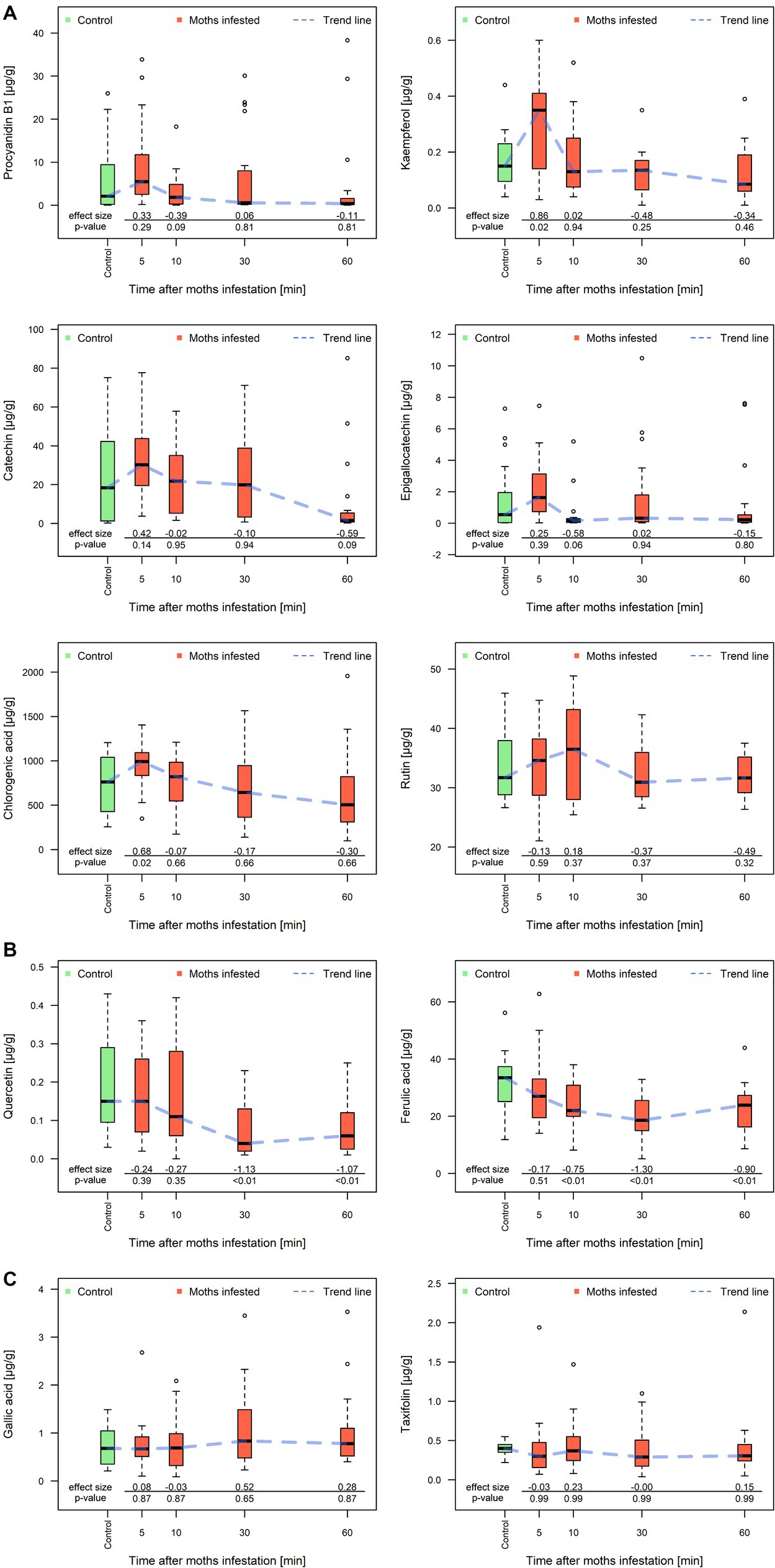
Figure 5. Concentration of phenolic compounds of the three groups according to a different patterns of development over time. First group (A) procyanidin B1, kaempferol, catechin, epigallocatechin, chlorogenic acid and rutin; second group (B) quercetin and ferulic acid; third group (C) gallic acid and taxifolin in poplar leaves obtained after 5, 10, 30, and 60 min of chewing by fungus gnats. Y-axis values represent the relative shift from the control group. The thick line in the box represents the median values, the range of the box represents the lower and upper quartile value, the minimum and maximum whisker values, and the circular extreme values. The blue dashed line represents the general trend of compound concentration over time. Effect size is a quantitative measure of the size of an experimental effect (Cohen’s d).
In Figure 6, we show the resulting chromatogram showing selected phenolic substances. By retention time on the x-axis and response rate in mass spectrometry analysis on the y-axis.
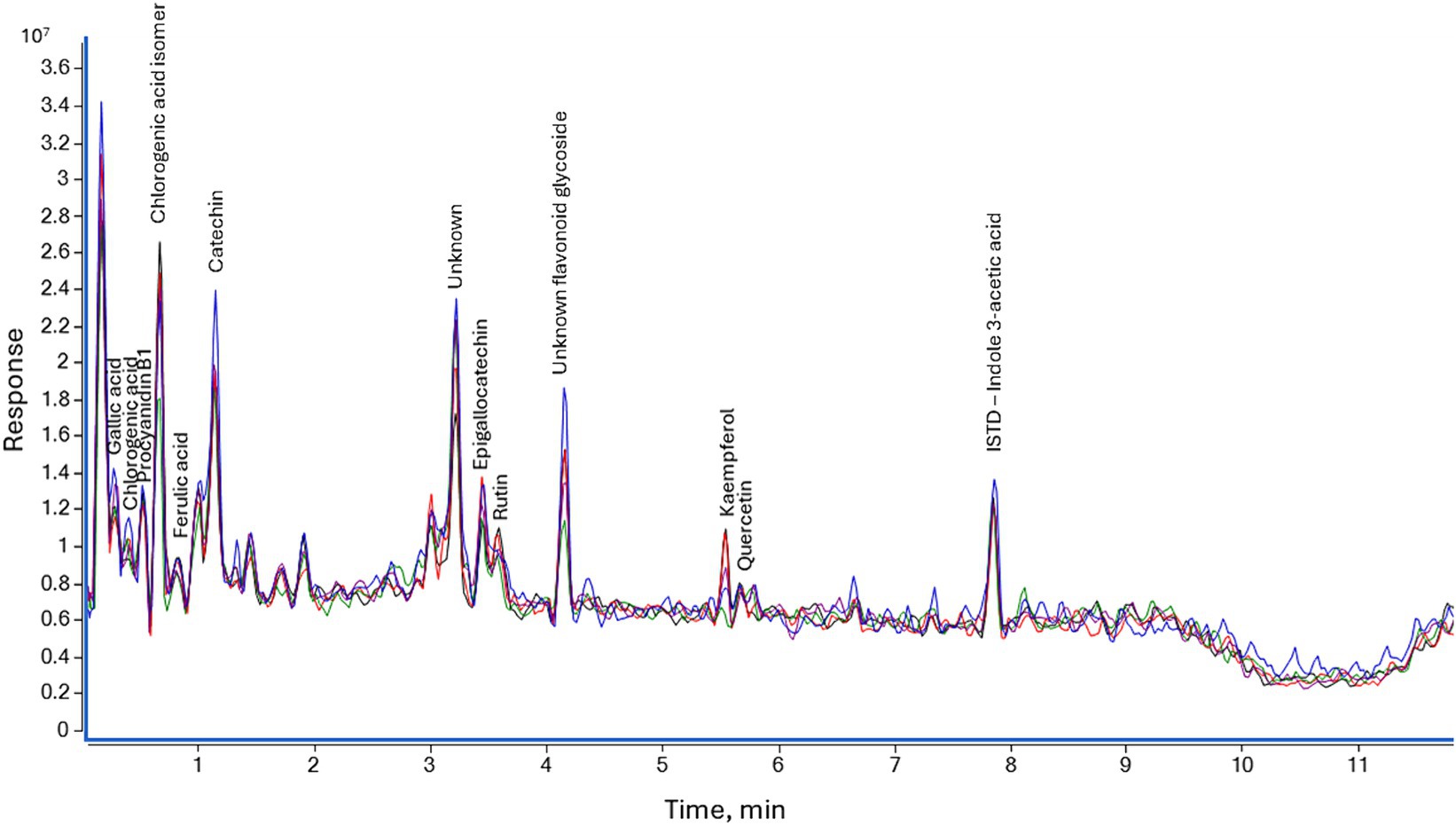
Figure 6. Total ion chromatograms of Populus tremula polyphenolic compounds: violet – control sample; green – after 5 min; black – after 10 min; red – after 30 min; blue – after 60 min.
Discussion
The current understanding of interactions between plants and insects is potentially vast, as indicated by numerous studies (Arimura, 2021; Mostafa et al., 2022; Wari et al., 2022), and supported by ample data available for meta-analyses (Zebelo and Maffei, 2014; Garcia and Eubanks, 2018; Wallis Ch and Galarneau, 2020). However, it is evident that accurately describing the dynamic relationship between a plant and an insect herbivore is highly complex and constrained. While some attention has been given to the temporal aspect of herbivory events, such as diurnal rhythms and emissions of volatile organic compounds (Kunert et al., 2002), the daily feeding patterns (Doghri et al., 2022), circadian rhythms (Jander, 2012), and early stages of interaction (Maffei et al., 2007), there is a lack of comprehensive studies considering the time perspective in the early phase of insect herbivore attacks.
In this study, we investigated the precise timing of physiological responses and changes in selected secondary metabolites to attacks by sponge moths, resulting in immediate changes in sugars and polyphenols and gasometric parameters. Our findings confirmed previous research by Allison and Schultz (2005) and Schultz et al. (2013) regarding primary metabolite redistribution from roots to damaged leaves in Populus. However, there have been studies documenting the opposite response in other plant species, suggesting that results depend on a variety of factors, including plant condition, resource availability, genetic predisposition, infestation intensity. and the specificity of the plant’s defense response (Gómez et al., 2010; Orians et al., 2011). It seems that the well-known law of compensation and growth equilibrium by Saint-Hilaire (1818) generally applies. It says: “The budget of nature is fixed; but she is free to dispose of particular sums by an appropriation that may please her. In order to spend on one side, she is forced to economize on the other side” which Schultz et al. (2013) aptly point out in their study.
Taking a closer look at the gasometrical parameter Pn beforehand, the physical damage caused by caterpillar chewing can significantly alter the structure and function of the leaf. The loss of leaf tissue and damage to cell integrity may result in malfunctioning chloroplasts related to photosynthesis, thus reducing the capacity for photosynthetic activity. According to Zhou et al. (2015), a central aspect of any discourse concerning carbon allocation in response to herbivory is photosynthesis, which serves as the primary source of nearly all carbohydrates in green plants. However, the direct effect of leaf biomass disappearance typically does not show a linear correlation with Pn (Bueno et al., 2009). The defensive reactions often incur a cost in terms of reduced photosynthesis, as resources allocated to photosynthetic activities become constrained, leading to lower photosynthetic rates. This shift in energy and resource allocation favors defense mechanisms, as discussed by Arnold et al. (2004) and Gomez et al. (2012), while also restricting carbon fixation.
Physical damage caused by caterpillar feeding results in disruption of the leaf surface. The disruption of the plant tissue thus creates openings, which is usually the explanation for the decrease in transpiration. These physical wounds, when already sealed, serve as barriers, decreasing water vapor’s ability to escape from leaf surfaces and lowering transpiration rates (Herms and Mattson, 1992).
At the level of secondary metabolism, trees of the Salicaceae family are known to be among the most prolific producers of phenolic compounds (Boeckler et al., 2013). Poplar leaves contain phenolic chemicals with an anti-herbivory function, including procyanidin B1, catechin, chlorogenic acid, quercetin, kaempferol, rutin, taxifolin, and epigallocatechin (Pietarinen et al., 2006; Sobuj et al., 2020). In addition to physiological adjustments, insect bites can induce the production and accumulation of phenolic chemicals in poplar leaves as a defense mechanism. Depending on the extent and duration of insect damage, the concentration of phenolic chemicals can change. The differences in the dynamics of concentration development in our study are in direct contrast to the studies of Lahtinen et al. (2006). They reported that levels of phenolic compounds tend to rise in response to insect feeding, with higher amounts occurring when damage lasts longer. The activation of defensive signaling pathways causes changes in the amounts of polyphenols in poplar leaves. These signaling molecules can govern the expression of genes related to defense, thereby promoting the creation of defense proteins, protease inhibitors, and various other defensive substances (Walling, 2000). The comparison of our results with the time diagram presented in the study (Maffei et al., 2007) was surprising when we cannot confirm the given values with respect to our results. In particular, metabolic changes (induction of defense substances, allocation of resources) took place in our experiment demonstrably already in the first 5 min of feeding. As part of the results from our other yet unpublished study, the dynamics of changes in gene expression show similar findings. The response of phenolic compounds in Populus leaves to spongy moth caterpillars exhibits a notable level of diversity, and the phenolic data exhibited considerable variability even between clones, which is consistent with works (Donaldson et al., 2006; Smith et al., 2011). According to studies, several Populus spp. demonstrate diverse patterns of variation in phenolic content in response to caterpillar feeding, with some showing no significant change or even a decrease (Lämke and Unsicker, 2018; Zhang et al., 2020). This significant variation underscores the intricate nature of plant defense strategies and implies that various Populus species have developed unique biochemical mechanisms to contend with herbivore assaults. What was particularly noticeable was that this same extensive variability was observed within identical Populus clones originating from in vitro plant material in the present study.
The majority of prior studies examining the ecological functions of tannins have not specifically investigated individual chemical compounds. Instead, they have relied on general precipitation or colorimetric tests to measure the levels of “total phenolic compounds” or “total hydrolyzable/condensed tannins” (Appel et al., 2001; Boeckler et al., 2013). Given the immense chemical diversity of plant polyphenols, a limiting factor may have been researchers’ attempts to pinpoint a singular (primary) effect and define it as the “raison d’être” of the entire class of polyphenols. In fact, it appears more probable that different compounds play distinct roles in plant interactions with their environment (Moctezuma et al., 2014).
Flavan-3-ols, including monomeric catechin and polymeric proanthocyanidins (referred to as condensed tannins), are prevalent phenolics in Populus spp. (Ullah et al., 2019; Bandau et al., 2021). Functionally, this group of substances has been shown to induce oxidative stress in the gut of insects (Barbehenn and Constabel, 2011). According to the original work by Feeny (1968), it was generally assumed that higher levels of condensed tannins would reduce the preference of insect herbivores (Forkner et al., 2004). However, studies by Peters and Constabel (2002), Tsai et al. (2006), and Boeckler et al. (2014) suggest that adapted insects, or certain genotypes, may be attracted to higher levels of condensed tannins. The findings of Hjältén and Axelsson (2015) suggest that through coevolution, condensed tannins may even act as stimulants for insect herbivores. Based on the above, the dynamics of tannins appear unclear and inconsistent. When considering the results of our study, which detail the concentration changes of this group of substances after Lymantria dispar attack, the situation becomes even more ambiguous.
Quercetin, a key flavonol, is widely distributed in the plant kingdom (Zhang et al., 2020). It plays a complex role in mediating interactions between herbs and insects. It can stimulate insect feeding and promote growth at low concentrations (Rahden-Staron et al., 2001); however, some studies have shown that quercetin may inhibit the activities of antioxidant and detoxification enzymes, resulting in increased mortality of insect herbivores (Gómez et al., 2020). In light of our recorded dynamics, we find agreement with the study by Jing et al. (2024), where a decrease in quercetin and a significant increase in quercetin-3-O-glucoside were observed over the course of hours. As noted by Jing et al. (2024), quercetin-3-O-glucoside has a crucial negative impact on larval development. and free quercetin had no significant effect on larval growth. Thus, it seems that glucosylation of quercetin should be the subject of further research.
While the antioxidant properties of gallic acid have been observed in other plant species, such as soybean (Glycine max), where it has been shown to reduce the level of total ascorbate and glutathione (Ozfidan-Konakci et al., 2019), a contrasting phenomenon is observed in tomato (Solanum lycopersicum), where ascorbate content increases (Farghaly et al., 2021). In our study, gallic acid together with taxifolin, belongs to the group of substances with almost no response in the response of Populus tremula to Lepidoptera attack. Regarding the evolution of gallic acid content, we agree with Zhang et al. (2020), while acknowledging the limited understanding of the impact of this polyphenolic compound on plant resistance to herbivores.
As a crucial factor, hypothetically, along with: plant health vitality, resource availability, genotypes, chemotypes and coevolutionarily given interactions, time appears to be essential for selecting appropriate strategies (and their various combinations) in response to insect herbivory.
Based on our current understanding, there appears to be numerous studies describing the impact of phenolic compounds added to the diet for Lepidoptera (Diaz Napal and Palacios, 2015; Su et al., 2017; Wang et al., 2019; Gao et al., 2022); however, no studies focusing on the response of Populus spp. mentioning the early metabolic response to Lepidopteran attack by phenolic compounds have been found. The general textbook terminology regarding the uncertain classification of plant metabolites into primary and secondary, such as that proposed by Erb and Kliebenstein (2020), appears to shape the thinking and application of approaches. Similarly, this is true according to the results of our study when using the terminology of a strict division into induced defense reaction and tolerant strategy in response to insect herbivory, which may be misleading. It is very common to overlook the dynamic development of the plant’s metabolic response. This limitation restricts the characterization of the dynamic trajectory of the metabolic response over time to the identification of a particular response type at a specific point (time) along the developmental curve of the reaction. Based on this, it can be inferred that the approach of many studies (across all taxa) does not define a metabolic strategy but rather a state at specific time - when the samples were collected.
The ability of broadleaves to recover from defoliation and adjust their chemical defense composition according to external conditions is promising in future predictive models shaping forests.
Limitations
Due to the well-documented problems associated with the inherent biological variability of living organisms, various factors were carefully considered in the creation of the experimental design. These factors included the genetic predisposition of the species studied, the potential interplay of temporal effects within individual treatment periods and the cumulative effect of herbivory, the application of multidisciplinary methodologies, and the complexity of elucidating the dynamics of interspecies interactions. Despite careful attention to detail, there is still a chance of inaccuracies or phenomena that are difficult to grasp.
To mitigate these challenges, rigorous measures were implemented: including the use of genetically uniform individuals (Populus tremula), setting optimal environmental conditions in growth chambers (especially the elimination of the influence of varying intensity of irradiance), using multiple separate growth chambers to prevent chemical communication between poplars, systematics of manual works, sample collection and laboratory protocols.
Acknowledging the inherent constraints in our study, we believe that our research provides fresh insights into the intricacies of plant-insect interactions. We hope that our findings can spur further investigation in this fascinating field.
Data availability statement
The raw data supporting the conclusions of this article will be made available by the authors, without undue reservation.
Author contributions
FP: Writing – original draft, Writing – review & editing. JČ: Writing – original draft, Writing – review & editing. AK: Writing – original draft, Writing – review & editing. KM: Writing – original draft, Writing – review & editing. JH: Writing – original draft, Writing – review & editing. JB: Writing – original draft, Writing – review & editing. IT: Writing – original draft, Writing – review & editing.
Funding
The author(s) declare that financial support was received for the research, authorship, and/or publication of this article. Supported by grant “EVA4.0,” No. CZ.02.1.01/0.0/0.0/16_019/0000803 financed by the OP RDE.
Acknowledgments
The authors are grateful to native speaker Mark Sixmith for language proofreading. The authors are grateful to Karolína Berková for graphical work (S2FyQi5hcnRzdHVkaW9AZ21haWwuY29t). KM thankfully acknowledges the Department of Biotechnology, GOI for the Ramalingaswami Re-entry grant (BT/RLF/Re-entry/11/2023).
Conflict of interest
The authors declare that the research was conducted in the absence of any commercial or financial relationships that could be construed as a potential conflict of interest.
Publisher’s note
All claims expressed in this article are solely those of the authors and do not necessarily represent those of their affiliated organizations, or those of the publisher, the editors and the reviewers. Any product that may be evaluated in this article, or claim that may be made by its manufacturer, is not guaranteed or endorsed by the publisher.
References
Allison, S. D., and Schultz, J. C. (2005). Biochemical responses of chestnut oak to a galling CYNIPID. J. Chem. Ecol. 31, 151–166. doi: 10.1007/s10886-005-0981-5
Anderson, J. T., and Mitchell-Olds, T. (2010). Ecological genetics and genomics of plant defences: evidence and approaches. Funct. Ecol. 25, 312–324. doi: 10.1111/j.1365-2435.2010.01785.x
Appel, H. M., Govenor, H. L., D'ascenzo, M., Siska, E., and Schultz, J. C. (2001). Limitations of Folin assays of foliar phenolics in ecological studies. J. Chem. Ecol. 27, 761–778. doi: 10.1023/A:1010306103643
Arimura, G. (2021). Making sense of the way plants sense herbivores. Trends Plant Sci. 26, 288–298. doi: 10.1016/j.tplants.2020.11.001
Arnold, T., Appel, H., Patel, V., Stocum, E., Kavalier, A., and Schultz, J. (2004). Carbohydrate translocation determines the phenolic content of Populus foliage: a test of the sink-source model of plant defense. New Phytol. 164, 157–164. doi: 10.1111/j.1469-8137.2004.01157.x
Bandau, F., Albrectsen, B. R., Robinson, K. M., and Gundale, M. J. (2021). European aspen with high compared to low constitutive tannin defenses grow taller in response to anthropogenic nitrogen enrichment. For. Ecol. Manag. 487, 118985–111127. doi: 10.1016/j.foreco.2021.118985
Barbehenn, R. V., and Constabel, C. P. (2011). Tannins in plant–herbivore interactions. Phytochemistry 72, 1551–1565. doi: 10.1016/j.phytochem.2011.01.040
Birnbaum, S. S. L., and Abbot, P. (2020). Gene expression and diet breadth in plant-feeding insects: summarizing trends. Trends Ecol. Evol. 35, 259–277. doi: 10.1016/j.tree.2019.10.014
Boeckler, G. A., Gershenzon, J., and Unsicker, S. B. (2013). Gypsy moth Caterpillar feeding has only a marginal impact on phenolic compounds in old-growth black poplar. J. Chem. Ecol. 39, 1301–1312. doi: 10.1007/s10886-013-0350-8
Boeckler, G. A., Towns, M., Unsicker, S. B., Mellway, R. D., Yip, L., Hilke, I., et al. (2014). Transgenic upregulation of the condensed tannin pathway in poplar leads to a dramatic shift in leaf palatability for two tree-feeding Lepidoptera. J. Chem. Ecol. 40, 150–158. doi: 10.1007/s10886-014-0383-7
Bruce, T. J. A., and Pickett, J. A. (2011). Perception of plant volatile blends by herbivorous insects – finding the right mix. Phytochemistry 72, 1605–1611. doi: 10.1016/j.phytochem.2011.04.011
Bueno, A. d. F., Bueno, R. C. O. d. F., Nabity, P. D., Higley, L. G., and Fernandes, O. A. (2009). Photosynthetic response of soybean to twospotted spider mite (Acari: Tetranychydae) injury. Braz. Arch. Biol. Technol. 52, 825–834. doi: 10.1590/s1516-89132009000400005
Copolovici, L., Pag, A., Kännaste, A., Bodescu, A., Tomescu, D., Copolovici, D., et al. (2017). Disproportionate photosynthetic decline and inverse relationship between constitutive and induced volatile emissions upon feeding of Quercus robur leaves by large larvae of gypsy moth (Lymantria dispar). Environmental and experimental botany. Crit. Rev. Plant Sci. 138, 184–192. doi: 10.1016/j.envexpbot.2017.03.014
Delaney, K. J. (2008). Injured and uninjured leaf photosynthetic responses after mechanical injury on Nerium oleander leaves, and Danaus plexippus herbivory on Asclepias curassavica leaves. Plant Ecol. 199, 187–200. doi: 10.1007/s11258-008-9423-0
Diaz Napal, G. N., and Palacios, S. M. (2015). Bioinsecticidal effect of the flavonoids pinocembrin and quercetin against Spodoptera frugiperda. J. Pest. Sci. 88, 629–635. doi: 10.1007/s10340-014-0641-z
Doghri, M., Rodríguez, V. M., Kliebenstein, D. J., and Francisco, M. (2022). Plant responses underlying timely specialized metabolites induction of Brassica crops. Front. Plant Sci. 12:807710. doi: 10.3389/fpls.2021.807710
Donaldson, J. R., Stevens, M. T., Barnhill, H. R., and Lindroth, R. L. (2006). Age-related shifts in leaf chemistry of clonal Aspen (Populus tremuloides). J. Chem. Ecol. 32, 1415–1429. doi: 10.1007/s10886-006-9059-2
Erb, M., and Kliebenstein, D. J. (2020). Plant secondary metabolites as defenses, regulators, and primary metabolites: the blurred functional trichotomy. Plant Physiol. 184, 39–52. doi: 10.1104/pp.20.00433
Farghaly, F. A., Salam, H. K., Hamada, A. M., and Radi, A. A. (2021). The role of benzoic acid, gallic acid and salicylic acid in protecting tomato callus cells from excessive boron stress. Sci. Hortic. 278:109867. doi: 10.1016/j.scienta.2020.109867
Feeny, P. P. (1968). Effect of oak leaf tannins on larval growth of the winter moth Operophtera brumata. J. Insect Physiol. 14, 805–817. doi: 10.1016/0022-1910(68)90191-1
Forkner, R. E., Marquis, R. J., and Lill, J. T. (2004). Feeny revisited: condensed tannins as anti-herbivore defences in leaf-chewing herbivore communities of Quercus. Ecol. Entomol. 29, 174–187. doi: 10.1111/j.1365-2311.2004.0590.x
Fürstenberg-Hägg, J., Zagrobelny, M., and Bak, S. (2013). Plant Defense against Insect Herbivores. Int. J. Mol. Sci. 14, 10242–10297. doi: 10.3390/ijms140510242
Fyllas, N. M., Chrysafi, D., Avtzis, D. N., and Moreira, X. (2022). Photosynthetic and defensive responses of two Mediterranean oaks to insect leaf herbivory. Tree Physiol. 42, 2282–2293. doi: 10.1093/treephys/tpac067
Gao, Y. L., Pan, Z. Y., Meng, X., Yuan, Y. F., Li, H. Y., and Chen, M. (2022). The effect of quercetin on the growth, development, nutrition utilization, and detoxification enzymes in Hyphantria cunea Drury (Lepidoptera: Arctiidae). Forests 13:1945. doi: 10.3390/f13111945
Garcia, L. C., and Eubanks, M. D. (2018). Overcompensation for insect herbivory: a review and meta-analysis of the evidence. Ecology 100:e02585. doi: 10.1002/ecy.2585
Gerrienne, P., Gensel, P. G., Strullu-Derrien, C., Lardeux, H., Steemans, P., and Prestianni, C. (2011). A simple type of wood in two early Devonian plants. Science 333:837. doi: 10.1126/science.1208882
Gómez, S., Ferrieri, R. A., Schueller, M., and Orians, C. M. (2010). Methyl jasmonate elicits rapid changes in carbon and nitrogen dynamics in tomato. New Phytol. 188, 835–844. doi: 10.1111/j.1469-8137.2010.03414.x
Gómez, J. D., Pinheiro, V. J., Silva, J. C., Romero, J. V., Merino-Cabrera, Y., Coutinho, F. S., et al. (2020). Leaf metabolic profiles of two soybean genotypes differentially affect the survival and the digestibility of Anticarsia gemmatalis caterpillars. Plant Physiol. Biochem. 155, 196–212. doi: 10.1016/j.plaphy.2020.07.010
Gomez, S., Steinbrenner, A. D., Osorio, S., Schueller, M., Ferrieri, R. A., Fernie, A. R., et al. (2012). From shoots to roots: transport and metabolic changes in tomato after simulated feeding by a specialist lepidopteran. Entomol. Exp. Appl. 144, 101–111. doi: 10.1111/j.1570-7458.2012.01268.x
Hajek, A. (2001). Larval behavior in Lymantria dispar increases risk of fungal infection. Oecologia 126, 285–291. doi: 10.1007/s004420000509
Hanik, N., Gómez, S., Best, M., Schueller, M., Orians, C. M., and Ferrieri, R. A. (2010a). Partitioning of new carbon as 11C in Nicotiana tabacum reveals insight into methyl jasmonate induced changes in metabolism. J. Chem. Ecol. 36, 1058–1067. doi: 10.1007/s10886-010-9835-x
Haruta, M., Pedersen, J. A., and Constabel, C. P. (2001). Polyphenol oxidase and herbivore defense in trembling aspen (Populus tremuloides): cDNA cloning, expression, and potential substrates. Physiol. Plant. 112, 552–558. doi: 10.1034/j.1399-3054.2001.1120413.x
Herms, D. A., and Mattson, W. J. (1992). The dilemma of plants: to grow or defend. Q. Rev. Biol. 67, 283–335. doi: 10.1086/417659
Hjältén, J., and Axelsson, E. P. (2015). GM trees with increased resistance to herbivores: trait efficiency and their potential to promote tree growth. Front. Plant Sci. 6:136916. doi: 10.3389/fpls.2015.00279
Holopainen, J. K., and Gershenzon, J. (2010). Multiple stress factors and the emission of plant VOCs. Trends Plant Sci. 15, 176–184. doi: 10.1016/j.tplants.2010.01.006
Jander, G. (2012). Timely plant defenses protect against caterpillar herbivory. Proc. Natl. Acad. Sci. 109, 4343–4344. doi: 10.1073/pnas.1201443109
Jing, T., Du, W., Qian, X., Wang, K., Luo, L., Zhang, X., et al. (2024). UGT89AC1-mediated quercetin glucosylation is induced upon herbivore damage and enhances Camellia sinensis resistance to insect feeding. Plant Cell Environ. 47, 682–697. doi: 10.1111/pce.14751
Johnson, M. T. J. (2011). Evolutionary ecology of plant defences against herbivores. Funct. Ecol. 25, 305–311. doi: 10.1111/j.1365-2435.2011.01838.x
Khare, S., Singh, N. B., Singh, A., Hussain, I., Niharika, K., Yadav, V., et al. (2020). Plant secondary metabolites synthesis and their regulations under biotic and abiotic constraints. J. Plant Biol. 63, 203–216. doi: 10.1007/s12374-020-09245-7
Kunert, M., Biedermann, A., Koch, T., and Boland, W. (2002). Ultrafast sampling and analysis of plant volatiles by a hand-held miniaturised GC with pre-concentration unit: kinetic and quantitative aspects of plant volatile production. J. Sep. Science 25, 677–684. doi: 10.1002/1615-9314(20020701)25:10/11<677::AID-JSSC677>3.0.CO;2-5
Labandeira, C. (2007). The origin of herbivory on land: initial patterns of plant tissue consumption by arthropods. Insect Science 14, 259–275. doi: 10.1111/j.1744-7917.2007.00141.x-i1
Labandeira, C. (2013). Deep-time patterns of tissue consumption by terrestrial arthropod herbivores. Naturwissenschaften 100, 355–364. doi: 10.1007/s00114-013-1035-4
Lahtinen, M., Kapari, L., Haukioja, E., and Pihlaja, K. (2006). Effects of increased content of leaf surface flavonoids on the performance of mountain birch feeding sawflies vary for early and late season species. Chemoecology 16, 159–167. doi: 10.1007/s00049-006-0343-y
Lämke, J. S., and Unsicker, S. B. (2018). Phytochemical variation in treetops: causes and consequences for tree-insect herbivore interactions. Oecologia 187, 377–388. doi: 10.1007/s00442-018-4087-5
Madsen, S. R., Kunert, G., Reichelt, M., Gershenzon, J., and Halkier, B. A. (2015). Feeding on leaves of the glucosinolate transporter mutant gtr1gtr2 reduces fitness of Myzus persicae. J. Chem. Ecol. 41, 975–984. doi: 10.1007/s10886-015-0641-3
Maffei, M. E., Mithöfer, A., and Boland, W. (2007). Before gene expression: early events in plant–insect interaction. Trends Plant Sci. 12, 310–316. doi: 10.1016/j.tplants.2007.06.001
Medvedev, S. S. (2005). Calcium signaling system in plants Russ. J. Plant Physiol. 52, 249–270. doi: 10.1007/s11183-005-0038-1
Moctezuma, C., Hammerbacher, A., Heil, M., Gershenzon, J., Méndez-Alonzo, R., and Oyama, K. (2014). Specific polyphenols and tannins are associated with defense against insect herbivores in the tropical oak Quercus oleoides. J. Chem. Ecol. 40, 458–467. doi: 10.1007/s10886-014-0431-3
Mostafa, S., Wang, Y., Zeng, W., and Jin, B. (2022). Plant responses to herbivory, wounding, and infection. Int. J. Mol. Sci. 23:7031. doi: 10.3390/ijms23137031
Orians, C. M., Thorn, A., and Gómez, S. (2011). Herbivore-induced resource sequestration in plants: why bother? Oecologia 167, 1–9. doi: 10.1007/s00442-011-1968-2
Ozfidan-Konakci, C., Yildiztugay, E., Yildiztugay, A., and Kucukoduk, M. (2019). Cold stress in soybean (Glycine max L.) roots: exogenous gallic acid promotes water status and increases antioxidant activities. Botanica Serbica. 43, 59–71. doi: 10.2298/BOTSERB1901059O
Pandey, S., Tiwari, S. B., Upadhyaya, K. C., and Sopory, S. K. (2000). Calcium signaling: Linking environmental signals to cellular functions. Crit. Rev. Plant Sci. 19, 291–318. doi: 10.1080/07352680091139240
Peters, D. J., and Constabel, C. P. (2002). Molecular analysis of herbivore-induced condensed tannin synthesis: cloning and expression of dihydroflavonol reductase from trembling aspen (Populus tremuloides). Plant J. 32, 701–712. doi: 10.1046/j.1365-313X.2002.01458.x
Pietarinen, S. P., Willför, S. M., Vikström, F. A., and Holmbom, B. R. (2006). Aspen knots, a rich source of flavonoids. J. Wood Chem. Technol. 26, 245–258. doi: 10.1080/02773810601023487
Pilipoviš, A., Drekiš, M., Orloviš, S., Poljakoviš-Pajnik, L., Nikoliš, N., and Borišev, M. (2015). “Growth and physiological response of different poplar clones on herbivory induced stress,” in Proceedings of the biennial international symposium. Forest and sustainable development. Brașov, Romania: Transilvania University Press, 121–126.
Poveda, K., Díaz, M. F., and Ramirez, A. (2017). Can overcompensation increase crop production? Ecology 99, 270–280. doi: 10.1002/ecy.2088
Poveda, K., Jimenez, M. I. G., and Kessler, A. (2013). The enemy as ally: herbivore-induced increase in crop yield. Ecol. Appl. 23, 515–522. doi: 10.1890/09-1726.1
Rahden-Staron, I., Czeczot, H., and Szumilo, M. (2001). Induction of rat liver cytochrome P450 isoenzymes CYP 1A and CYP 2B by different fungicides, nitrofurans, and quercetin. Mut. Res. 498, 57–66. doi: 10.1016/S1383-5718(01)00267-4
Rosenkranz, M., and Schnitzler, J. P. (2016). Plant Volatiles. Chichester: eLS, John Wiley & Sons, Ltd.
Schultz, J. C., Appel, H. M., Ferrieri, A. P., and Arnold, T. M. (2013). Flexible resource allocation during plant defense responses. Front. Plant Sci. 4. doi: 10.3389/fpls.2013.00324
Smith, E. (2007). Plant secondary metabolites: occurrence, structure and role in the human diet. Phytother. Res. 21:904. doi: 10.1002/ptr.2237
Smith, E. A., Collette, S. B., Boynton, T. A., Lillrose, T., Stevens, M. R., Bekker, M. F., et al. (2011). Developmental contributions to phenotypic variation in functional leaf traits within quaking aspen clones. Tree Physiol. 31, 68–77. doi: 10.1093/treephys/tpq100
Sobuj, N., Virjamo, V., Nissinen, K., Sivadasan, U., Mehtätalo, L., Nybakken, L., et al. (2020). Responses in growth and phenolics accumulation to lateral bud removal in male and female saplings of Populus tremula (L.) under simulated climate change. Sci. Total Environ. 704:135462. doi: 10.1016/j.scitotenv.2019.135462
Stevens, M. T., and Lindroth, R. L. (2005). Induced resistance in the indeterminate growth of aspen (Populus tremuloides). Oecologia 145, 297–305. doi: 10.1007/s00442-005-0128-y
Su, Q., Zhou, Z., Zhang, J., Shi, C., Zhang, G., Jin, Z., et al. (2017). Effect of plant secondary metabolites on common cutworm, Spodoptera litura (Lepidoptera: Noctuidae). Entomol. Res. 48, 18–26. doi: 10.1111/1748-5967.12238
Šulc, M., Tomášková, I., Krejzková, A., Samek, M., Diuzheva, A., Hradecký, J., et al. (2021). Trehalose determination in Norway spruce (Picea abies) roots. Analytics Matters. MethodsX 8:101280. doi: 10.1016/j.mex.2021.101280
Team, R. C. (2021). R: a language and environment for statistical computing. Published online 2020. Suppl. Inform. Ref. S 1, 371–378,
Tsai, C. J., Harding, S. A., Tschaplinski, T. J., Lindroth, R. L., and Yuan, Y. (2006). Genome-wide analysis of the structural genes regulating defense phenylpropanoid metabolism in Populus. New Phytol. 172, 47–62. doi: 10.1111/j.1469-8137.2006.01798.x
Ullah, C., Unsicker, S. B., Reichelt, M., Gershenzon, J., and Hammerbacher, A. (2019). Accumulation of Catechin and Proanthocyanidins in black poplar stems after infection by Plectosphaerella populi: hormonal regulation, biosynthesis and antifungal activity. Front. Plant Sci. 10. doi: 10.3389/fpls.2019.01441
Visakorpi, K., Riutta, T., Malhi, Y., Salminen, J. P., Salinas, N., and Gripenberg, S. (2020). Changes in oak (Quercus robur) photosynthesis after winter moth (Operophtera brumata) herbivory are not explained by changes in chemical or structural leaf traits. PLoS One 15:e0228157. doi: 10.1371/journal.pone.0228157
Vogel, H., Musser, R. O., and de la Paz Celorio-Mancera, M. (2014). Transcriptome responses in herbivorous insects towards host plant and toxin feeding. Annual Plant Rev. 47, 197–233. doi: 10.1002/9781118829783.ch6
Walling, L. L. (2000). The myriad plant responses to herbivores. J. Plant Growth Regul. 19, 195–216. doi: 10.1007/s003440000026
Wallis Ch, M., and Galarneau, E. R. (2020). Phenolic compound induction in plant-microbe and plant-insect interactions: a Meta-analysis. Front. Plant Sci. 11:580753. doi: 10.3389/fpls.2020.580753
Wang, Z., Nur, F. A., Ma, J., Wang, J., and Cao, C. (2019). Effects of poplar secondary metabolites on performance and detoxification enzyme activity of Lymantria dispar. Comp. Biochem. Physiol. Part C 225:108587. doi: 10.1016/j.cbpc.2019.108587
War, A. R., Paulraj, M. G., Ahmad, T., Buhroo, A. A., Hussain, B., Ignacimuthu, S., et al. (2021). Mechanisms of plant defense against insect herbivores. Plant Signal. Behav. 7, 1306–1320. doi: 10.4161/psb.21663
Wari, D., Aboshi, T., Shinya, T., and Galis, I. (2022). Integrated view of plant metabolic defense with particular focus on chewing herbivores. J. Integr. Plant Biol. 64, 449–475. doi: 10.1111/jipb.13204
Webster, B., Bruce, T., Pickett, J., and Hardie, J. (2010). Volatiles functioning as host cues in a blend become nonhost cues when presented alone to the black bean aphid. Anim. Behav. 79, 451–457. doi: 10.1016/j.anbehav.2009.11.028
Wink, M. (2018). Plant secondary metabolites modulate insect behavior-steps toward addiction? Front. Physiol. 9. doi: 10.3389/fphys.2018.00364
Zebelo, S. A., and Maffei, M. E. (2014). Role of early signalling events in plant-insect interactions. J. Exp. Bot. 66, 435–448. doi: 10.1093/jxb/eru480
Zhang, W. H., Schmid, B., Liu, L., and Wang, P. (2020). Phenolic responses of Populus species to spongy moth caterpillar herbivory. J. Plant Ecol. 13, 1–2. doi: 10.1093/jpe/rtaa009
Zhang, B., Zhou, L., Zhou, X., Bai, Y., Zhan, M., Chen, J., et al. (2022). Differential responses of leaf photosynthesis to insect and pathogen outbreaks: a global synthesis. Sci. Total Environ. 832:155052. doi: 10.1016/j.scitotenv.2022.155052
Keywords: induced defense, net photosynthesis, phenolics, resource allocation, spongy moth, transpiration
Citation: Pastierovič F, Čepl J, Kalyniukova A, Mogilicherla K, Hradecký J, Bláha J and Tomášková I (2024) Time is of the essence: unveiling the rapid response of Populus to insect feeding. Front. For. Glob. Change. 7:1376465. doi: 10.3389/ffgc.2024.1376465
Edited by:
Quan Lu, Chinese Academy of Forestry, ChinaReviewed by:
Jana Albrechtova, Charles University, CzechiaZheng Wang, Shandong Agricultural University, China
Copyright © 2024 Pastierovič, Čepl, Kalyniukova, Mogilicherla, Hradecký, Bláha and Tomášková. This is an open-access article distributed under the terms of the Creative Commons Attribution License (CC BY). The use, distribution or reproduction in other forums is permitted, provided the original author(s) and the copyright owner(s) are credited and that the original publication in this journal is cited, in accordance with accepted academic practice. No use, distribution or reproduction is permitted which does not comply with these terms.
*Correspondence: Ivana Tomášková, dG9tYXNrb3ZhQGZsZC5jenUuY3o=