- 1Institute for Alpine Environment, Eurac Research, Bolzano, Italy
- 2D.R.E.Am Italia, Pratovecchio-Stia, Italy
- 3Faculty of Resource Management, University of Applied Sciences and Arts Hildesheim/Holzminden/Göttingen (HAWK), Göttingen, Germany
- 4Department of Botany, Universität Innsbruck, Innsbruck, Austria
- 5Department of Ecology, Universität Innsbruck, Innsbruck, Austria
Introduction: Climate change affects the vitality of mountain forests through increasing temperatures and decreasing water availability due to changing precipitation patterns, earlier snowmelt, and increasing evaporative demand. Depending on species characteristics, tree growth might therefore increase in cold habitats near the forest line but decrease in water-limited conditions at low elevation.
Methods: We analyzed the tree-ring widths of five conifers (Picea abies, Larix decidua, Pinus sylvestris, Pinus nigra, and Pinus cembra) along an elevational gradient from 1,000 m to 2,320 m above sea level (a.s.l.) in Vinschgau/Val Venosta Valley in Northern Italy, one of the driest regions of the Alps (mean annual precipitation of 682 mm at 1,310 m a.s.l.).
Results: Our aim was to estimate the species-specific growth response to changing climate conditions along an elevational gradient. At low elevations, we observed a significant response to water availability not only during the actual growing season but also throughout the previous autumn for all species present. At mid-elevation, the correlation coefficients to precipitation and drought indices (SPEI) were highest for Picea abies. At high elevations, the positive correlation of growth with temperature was smaller than expected for Pinus cembra. In contrast, Larix decidua responded positively to temperature and grew faster in recent decades.
Discussion: Considering that a further increase in temperatures will reduce plant water availability during the growing season, our space-for-time approach provides an outlook on future growth conditions of conifers in larger regions of the European Alps. Water limitation will affect tree growth and vitality not only at low elevation in the valleys but also at mid elevation on mountain slopes, potentially impacting timber production and protective and recreative functions of forests. Near the forest line, the different capabilities of tree species to benefit from higher temperatures might lead to changes in species composition.
1 Introduction
Mountain forests provide essential functions and ecosystem services to humanity. In addition to timber production and CO2 sequestration, protective function and recreational value are especially important in the European Alps (Perzl et al., 2021; Winkel et al., 2022). Currently, the Alps are experiencing a stronger temperature increase than the surrounding lowlands with inconsistent precipitation trends (Auer et al., 2007; Kotlarski et al., 2022). These climatic changes and their effects on the composition, structure, and vitality of mountain forests might jeopardize the above-mentioned services and functions.
Rising temperatures can benefit tree growth at high elevation by reducing energy limitations and increasing growing season length (Babst et al., 2013). On the other hand, they can also lead to growth limitations by increasing air vapor pressure deficit and decreasing soil water availability due to higher evapotranspiration (Babst et al., 2019; Etzold et al., 2021; Zweifel et al., 2021). These limitations were stronger at low elevations but were also found to affect trees up to the forest line (Obojes et al., 2022). Furthermore, climate change is expected to impact tree growth by increasing the frequency and intensity of extreme events such as droughts, heat waves, and storms and promoting mass outbreaks and range expansion of pests such as bark beetles (Netherer et al., 2019; Senf and Seidl, 2021). To adapt forest management to these challenges, it is necessary to predict future tree growth and vitality including their reaction to changing climate conditions. Past climate-growth relationships recorded in tree rings provide a foundation to predict future growth dynamics at the site level (Charney et al., 2016). In the case of climate predictions that exceed the recorded range found in the available tree rings, a space-for-time approach, with several stands along an elevational gradient, can provide further data. In this approach, low-elevation sites correspond to warmer and drier future growth conditions (Becker et al., 2007; Körner, 2007). In the inner alpine Vinschgau/Val Venosta region, our study area is particularly well suited for this approach due to its steep elevational gradients, comparatively dry conditions at low elevation, and an interesting set of both native and introduced conifers.
Conifers are the dominant plant functional type in the Central Alps in the high montane and subalpine belt and usually constitute the forest line (Vacik et al., 2010a; Leuschner and Ellenberg, 2017). In the last centuries, their natural dominance has additionally been promoted by human use. Norway spruce (Picea abies L., in the following PA) is by far the most abundant tree species at mid and high elevations in the Alps due to its competitiveness and importance for timber production (Vacik et al., 2010a; Caudullo et al., 2016). However, PA is vulnerable to extreme events such as drought and windthrow both directly and by increasing its susceptibility to bark beetles. Such forest declines have been predominantly observed in plantations in lowlands but are increasingly reported at lower and mid-elevation sites across the Alps (Pasztor et al., 2014; Hlásny et al., 2021; Jaime et al., 2022). After spruce, European larch (Larix decidua MILL., in the following LD) and Scots pine (Pinus sylvestris L., in the following PS) are the most abundant conifer species in the Alps. Under natural conditions, LD is the most abundant near the forest line in avalanche tracks and erosion channels but may also substitute beech (Fagus sylvatica L.) in dry montane forests (Staffler and Karrer, 2001; Vacik et al., 2010b; Da Ronch et al., 2016). Additionally, LD was promoted by humans in silvopastoral larch meadows (Motta and Lingua, 2005; Fontana et al., 2014). Due to their light-demanding early life stages, a lack of disturbance or management leads to regeneration of PA underneath older LD trees (Garbarino et al., 2011; Moris et al., 2017). Deciduous by nature, LD has to be photosynthetically active also in drier conditions (Anfodillo et al., 1998; Wieser, 2010; Leo et al., 2014). This is achieved by maintaining high water consumption through its deep heart-root system and osmotic adjustments under dry conditions (Badalotti et al., 2000). Thus, hardly any large-scale drought-related dieback of LD is known in the Alps, even though several studies suggest that LD is close to its limits in dry areas (Eilmann and Rigling, 2012; Lévesque et al., 2013). PS is the most abundant conifer in the world (Durrant et al., 2016). In the Alps, due to its low competitiveness compared with PA, PS grows mainly in dry inner-Alpine valleys at low elevation and on marginal soils (Oberhuber et al., 2014). While also a light-demanding pioneer, PS is an isohydric species with strict control of stomatal conductance (Oberhuber et al., 2015). Though PS is considered a drought-resistant species, severe drought led to large-scale diebacks of stands growing at marginal conditions (Vacchiano et al., 2012; Hunziker et al., 2022; Lemaire et al., 2022). The Swiss stone pine (Pinus cembra L., in the following PC) is a slow-growing, late-successional pine species that is restricted to the upper subalpine forests near the forest line as it is outcompeted by PA at elevations below 1,700 m a.s.l. (Motta et al., 2006; Caudullo and de Rigo, 2016). Its abundance is limited to the more continental Central Alps as sapling mortality, which is induced by snow fungi, preventing its regeneration in snowy regions (Holtmeier, 1990; Barbeito et al., 2013). Finally, we also sampled black pine (Pinus nigra J. F. ARNOLD in the following PN), a Mediterranean conifer that was introduced to the region 130 to 50 years ago for erosion control on degraded south-exposed, low-elevation slopes (Vacik et al., 2010b). PN is considered an isohydric, drought-resistant species (Enescu et al., 2016).
In this study, we compare the climate-growth relationships of these five conifer species with different physiological backgrounds at different elevations within their local distribution range. Our objective is to determine how their growth reacts to changing environments from water-limited to temperature-limited conditions. We hypothesize that at low elevation, isohydric, drought-resistant pines (PN and PS) will be less water-limited than drought-vulnerable PA and deciduous LD. We also anticipate that growth conditions for LD and PA will be ideal at mid-elevation. At high elevation and the forest line, we expected a stronger temperature response of fast-growing LD and PA in comparison to PC. Thus, we predict higher growth rates for LD and PA due to the increasing temperatures observed in recent decades.
2 Materials and methods
2.1 Site description
Our study was conducted within the LTSER platform “Matsch|Mazia” (LTER_EU_IT_097), which is located in a side valley of the Vinschgau/Val Venosta Valley in the Province of Bozen/Bolzano, Italy. Three to nine sites per species were sampled ranging from the valley bottom at 1,070 m above sea level (a.s.l.) to the current forest line (2,250 m a.s.l. at the South Slope and 2,320 m a.s.l. at the North Slope). Variable environmental conditions along the elevational gradient but also past and present land use cause differences in tree age, forest structure, and composition (see Table 1). Low-elevation sites are located close to the floor of Vinschgau/Val Venosta at the entrance of the Matsch/Mazia Valley and are the low-elevation forests in the area. Mid-elevation sites include the highest PN stands and LD, PA, and PS stands up to 1,800 m relatively close to the village of Matsch/Mazia. High-elevation sites include the highest PS stand and otherwise LD, PA, and PC stands in subalpine forests typical for the region. Finally, forest line sites were located at the uppermost limits of the closed forests. At the sampled forest stands at low elevation, trees were mostly planted starting in the 1890s, but also in the 1950s and 1960s, leading to a uniform age distribution. At high elevation, natural regeneration is more important, resulting in a more variable age distribution. Some sites are still used as pasture for sheep, horses, and/or cattle.
2.2 Climate
Long-term temperature and precipitation records (monthly data since 1858, daily precipitation data since 1924, and daily temperature since 1967) for the region are available from the climate station Marienberg/Monte Maria operated by the Hydrographic Office of the Autonomous Province of South Tyrol (Auer et al., 2007). This station is located 4.5 km northwest of the lowest elevation sites at 1,310 m a.s.l. These records are still continuously updated. For climate-growth analysis, we used data until 2013 in accordance with our tree-ring data. As a reference period for climate comparisons, we used the climate normal period of 1981–2010. Daily temperature data from 1924 to 1967 were estimated using data from the respective grid of the E-OBS data set (Cornes et al., 2018), which was corrected using a linear regression of gridded and station temperatures from 1967 to 2021. To this end, we calculated separate linear regressions for daily maximum (Tmax,station = Tmax,grid * 0.98 + 4.5, r2 = 0.87) and daily minimum (Tmin,station = Tmin,grid * 0.93 + 4.81, r2 = 0.95) temperatures and applied these equations to the period before 1967. We then calculated the daily mean temperatures for the whole period as the arithmetic mean between daily maximum and minimum temperatures. To combine temperature and precipitation data and indicate water availability and drought conditions, we used the standardized precipitation-evapotranspiration index (SPEI), which integrates precipitation and potential evapotranspiration (Beguería et al., 2014). To analyze the effects of short-term versus long-term dry periods, we calculated the SPEI on 1 month, 3 months, and 12 months basis using the spei()-function of the R-package SPEI (Begueria et al., 2017). To this end, according to Thornthwaite, we estimated the potential evapotranspiration with the thornthwaite()-function of the same R package.
2.3 Methods
To investigate climate-growth relationships, two core samples with a diameter of 5 mm were extracted at breast height (1.3 m) at opposite sides of 10 to 16 trees per site. Trees from all diameter classes present at the sites were sampled. To avoid sampling of reaction wood and growth irregularities, trees were cored parallel to the slope. Trees at PC-sites and at most LD-sites were sampled in August 2014 (Meurer, 2015), at all other sites during the summer of 2017 (Buscarini, 2018). Tree-ring analysis focused on the common period until 2013 for all sites. In the laboratory, core samples were fixed on a holder, and their surface was smoothed by cutting with a razor blade. Early wood and late wood ring widths were measured using a digital measuring table (LINTAB 4, Rinntech, Heidelberg, Germany) and the tree-ring program TSAP-Win (Rinntech, Heidelberg, Germany) or were scanned with an Epson Perfection V600 photo scanner with 2,400 dpi resolution and measured with CooRecorder 9.8 (Cybis Elektronik & Data AB, Sweden, Maxwell and Larsson, 2021). Early wood and late wood tree-ring sections were defined visually (cell size and darkening). For species with a gradual transition between earlywood and latewood (Norway spruce), we defined the early wood/late wood boundaries as the middle of the transition zone. Ring width series were crossdated visually in TSAP-Win (Rinntech, Heidelberg, Germany) or Cybis CDendro 9.8 (Cybis Elektronik & Data AB) and statistically in Cofecha (Holmes, 1983) and in R using the dplR package (Bunn et al., 2016). For further analysis, we calculated tree means from the two cores selected per tree. Site mean chronologies were derived using the dplR package, thereby trees with a correlation coefficient of less than 0.5 with the mean site chronology after crossdating were excluded from the site chronologies. Standard chronology statistics and Gleichläufigkeit between chronologies were also calculated with the dplR package. To visualize long-term growth trends, we calculated basal area increment (BAI), which depends less on tree age than tree-ring widths (Fritts, 1976; Schuster and Oberhuber, 2013a), using the bai.out()-function of the R package dplR. This analysis was performed by taking measured diameters at breast height (DBH) into account. For cores missing the pith, the missing rings were estimated in CooRecorder or from the difference between core length and stem radius calculated from measured DBH considering the average width of the five innermost measured rings of the core. For climate-growth correlations, we calculated the ring width index (RWI) by removing low-frequency variability related to tree aging and forest stand development using a spline with a frequency response of 0.5 at a wavelength of 30 years. To remove the remaining temporal autocorrelation and emphasize the high-frequency signal (year-to-year variability), the RWI was pre-whitened using a first-order autoregressive model before calculating climate-growth correlations. To visualize differences in tree growth patterns between different species and elevations, tree-ring chronologies and single tree-ring width data were compared using hierarchical cluster analysis (HCA) and principal component gradient analysis (PCGA) from the dendRolAB package (Buras et al., 2016) for a common period from 1971 to 2013.
Static seasonal climate-growth relationships were calculated using the dcc()-function (method: “correlation”) of the R package treeclim (Zang and Biondi, 2015), which we used to calculate seasonal correlations between tree-ring proxies and climatic drivers (temperature, precipitation, and SPEI) measured from spring (March to May) of the previous year to autumn (September to November) of the current year. Daily climate-growth correlations were determined from 1924 to 2013 using the function daily_response() in the R package dendrotools (Jevšenak and Levanič, 2018). This function searches for the time period with the highest correlation between the chosen tree-ring proxy and the respective climatic driver at a daily resolution. We allowed for a range of 21 to 270 days for this optimal cumulative time window within a period from 1st April of the previous year to 1st October of the current year. Temporal stability of daily correlations for the optimal time periods was checked using the same function, with a fixed-window approach and a 30 years window.
3 Results
3.1 Climate
Due to the sheltering effect of the surrounding mountains, the study area is one of the driest in the Alps with a mean annual temperature of 6.38°C and a mean annual precipitation of 683 mm. As a comparison, the average precipitation in the Alpine region was 1,100 mm during the late 20th century (Frei and Schär, 1998). During the period for which instrumental records are available, the mean annual air temperature at the Marienberg/Monte Maria climate station increased from 5.4°C in 1861 to 1870 (the first full decade available) to 6.7°C in 2001 to 2010 (the last full decade before tree-ring sampling). Overall, the temperature increased since the 1980s and, in particular, during summer and spring (Figure 1A). The precipitation was highest in summer (average 251 mm from 1981–2010), followed by autumn (195 mm), spring (136 mm), and winter (101 mm). No significant long-term trend was observed in precipitation since the 1860s (Figure 1B). According to SPEI12, 2002 was the driest since the start of the measurements at Marienberg/Monte Maria (Figure 1C). Other rather dry periods were from the mid-1980s to late 1990s, the early 1970s, and the 1940s. In contrast, a short period from 1999 to 2001, the late 1970s to early 1980s, the late 1960s, and, in general, until the mid-1930s were more humid. SPEI3 and SPEI1 provide information on short-term variability within these dry and wet periods. For instance, during the recent dry period since 2003, spring and early summer (March to June) were particularly dry while autumn and winter were dry in the early 1970s.
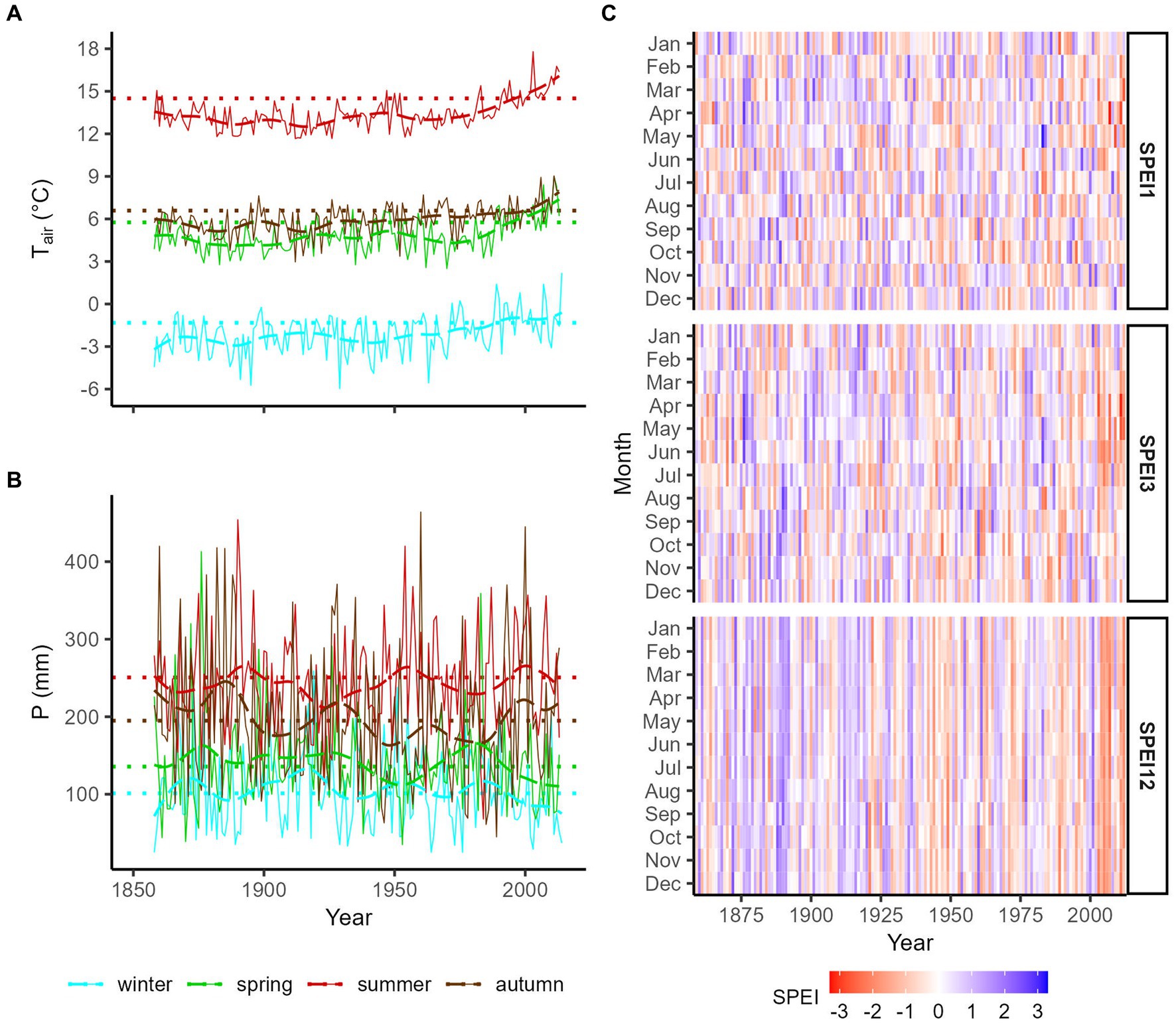
Figure 1. Long-term seasonal (winter = previous December to February, spring = March to May, summer = June to August, and autumn = September to November) mean temperatures (A) and precipitation sums (B) and 30 years means (1981–2010 climate normal period, dotted lines) and 30 years splines (dashed) from the Marienberg/Monte Maria climate station close to the research area. (C) Heatmaps of 1 month (SPEI1), 3 months (SPEI3), and 12 months (SPEI12) standardized precipitation-evapotranspiration index indicate dry (negative values) and humid conditions (positive values).
3.2 Tree growth
Tree age was very uniform (standard deviation lower than 10, see Table 1) at low-exposed (<1,200 m a.s.l.) and most south-exposed mid-elevation (1,200–1,800 m a.s.l.) stands, which were established either approximately 45 or between 110 and 130 years ago. Age distribution became less uniform (s.d. 10–80) at high elevation and on the North Slope with an average tree age between 70 and 140 years. Only PC stands at the north-exposed, high elevation (1,800–2,200 m a.s.l.), and forest line (>2,200 m a.s.l.) sites were older with a mean age of 190 years. In general, DBH was highest at mid-elevation and lowest at low elevation. Within the analyzed tree species, DBH was highest for PA, similar for PS, LD, and PN, and lowest for PC. Tree height was highest at mid-elevation and lowest at the forest line. Averaged by species, tree height was again highest for PA, followed by LD and PN and lowest for PC and PS.
Individual site chronologies showed the lowest mean tree-ring width at three of the four PC sites and at the lowest elevation sites (Table 2; Supplementary Table S1). In contrast, the highest mean ring widths were found at sites with low tree age at low-elevation and mid-elevation. Summarized by species, the mean total ring width was highest for PN (where trees at two of three sites were less than 45 years old), followed by PA, PS, and LD, and lowest for PC, which was restricted to high elevation. Early wood (EW) width had the same pattern as total ring width, while late wood (LW) width was relatively low in PA and very low in PC. The high first-order autocorrelation for TR and EW in PC was related to its constant growth. In contrast, the low autocorrelation for the same parameters found in LD and PN indicated a strong reaction to the respective climatic conditions and other factors driving growth. In addition, high values of signal-to-noise-ratio (SNR), expressed population signal (EPS), mean inter-series correlation (rbar), and overall inter-series correlation (OIC) at low elevation (especially for TR and EW) indicated similar growth behavior within sites due to strong climate forcing. These effects decreased at high elevation.
Another indicator of a strong climatic forcing of tree growth at low elevation is the high Gleichläufigkeit (GLK) between these site chronologies, even between different species. This tendency decreased at mid-elevation and increased again slightly toward the forest line (Supplementary Figure S1). The values and pattern of GLK were similar to TR and EW chronologies but were mostly lower for LW, indicating a stronger climatic influence earlier in the year and more species-specific patterns in LW formation. In general, BAI has shown increasing growth since the mid-1970s at most high-elevation and forest line PA and LD sites. However, BAI did not increase in recent decades for PC and at south-exposed LD and PA sites near 2,000 m a.s.l. (Figure 2). A sudden increase of BAI in the late 1970s at PS-1870-SW coincided with an increase in sampling depth (Supplementary Figure S2). Most mid-elevation sites (except for PA-1800-NW) and the younger low-elevation sites showed higher growth from the 1970s to 2000. At most low-elevation and mid-elevation sites, growth rates were higher in the 1970s and 1980s than in previous decades. The 2003 heatwave led to a clear growth reduction in all of these sites that persisted for several years. Recovery was only observed around 2010.

Figure 2. Time course of basal area increment (BAI) chronologies from 1880 to 2013 arranged by species and elevation (low <1,200 m, mid = 1,200 to 1,800 m, high = 1,800 to 2,200 m, and forest line >2,200 m a.s.l.). Sampling depth: > 4 trees per site. The grey dashed line marks the year 2003.
Overall, the high-frequency growth patterns of 30 years spline-detrended RWI displayed with HCA showed a distinct separation of all low-elevation sites (and LD.1160.SW), which was independent of the tree species (Figure 3). Most mid-elevation sites also formed a separate group. In contrast, at high-elevation, the forest line LD sites were clearly separated from pine (PC, PS) and spruce (PA) sites. Early wood HCA patterns were very similar to those obtained for total ring width. Unlike early wood, late wood HCA chronologies also showed a separation between low-elevation sites but allowed the distinction of different more elevation- and less species-related patterns at high elevations (Supplementary Figure S3).
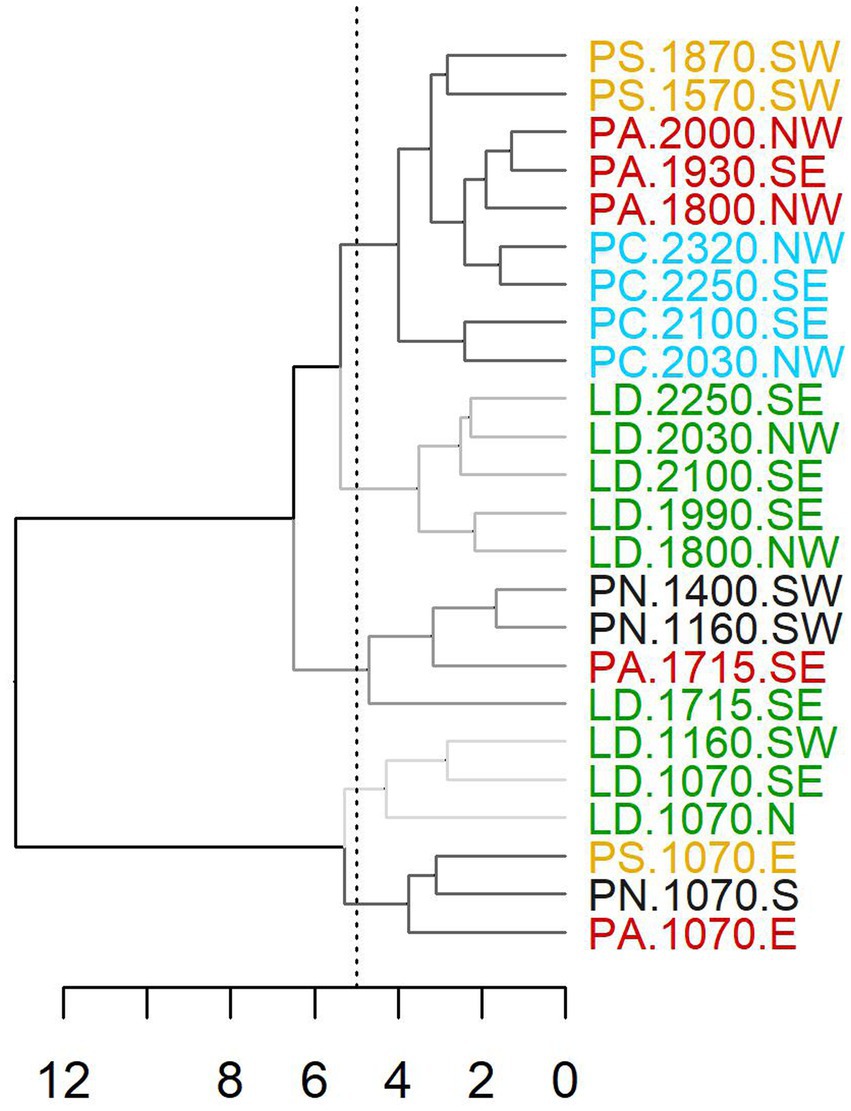
Figure 3. Hierarchical cluster analysis of RWI site chronologies of total ring width. (LD, Larix decidua; PA, Picea abies; PN, Pinus nigra; PS, Pinus sylvestris; PC, Pinus cembra).
A separation along the elevational gradient is also clearly displayed in PCGA-biplots (Figure 4A), where it corresponds to the second principal component. In contrast, the first principal component showed some separation between species, for instance, between PS and most PA sites at mid-elevation or for late wood LD and PC at high elevation and the forest line. Nonetheless, the first principal component was overall harder to interpret. Comparing PCGA ranks, low-elevation sites differed significantly from high-elevation and forest line sites, with mid-elevation sites in between (Figure 4B). In contrast, significant differences between species were found only between PN and PC, where PN was restricted to mid-elevation and low-elevation, while PC occurred at high elevation and the forest line (Figure 4C). Variability of tree growth within species was highest for LD with sites ranging across the whole elevational gradient. PA and PS, which were also distributed from low to high elevation, were less variable. In general, TR and EW patterns were similar, while LW chronologies differed. Especially, late LW chronologies of PC were separated from high-elevation/forest line LD sites. To obtain more detail on intra-species and intra-site versus inter-site variability, we applied PCGA at tree level for total ring width (Figure 5; Supplementary Figure S4). LD and PA showed a significant separation between low-elevation and high-elevation/forest line sites. PS separated quite clearly along the elevational gradient, while PN, which occurred at a narrower elevation range, separated quite less along the elevational gradient. For PC, high-elevation and forest line sites differed considerably. Across species, sites approximately 2,000 m a.s.l. showed a higher variability than low-elevation and forest line sites.
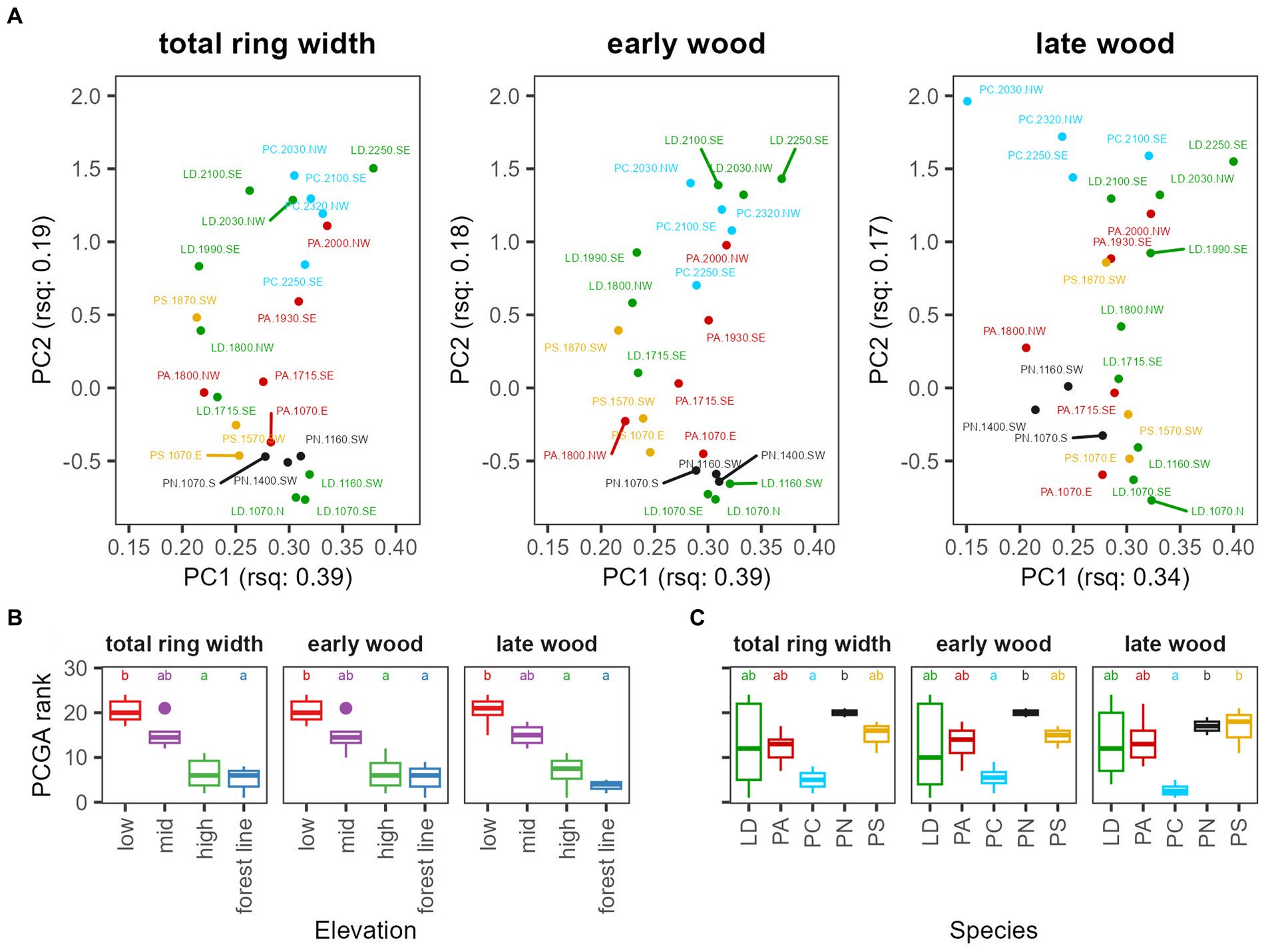
Figure 4. Comparison of indexed site chronologies of total ring width (TR), early wood (EW), and late wood (LW) using PCGA. The top row (A) displays the PCA-biplots, numbers in the axis titles (rsq) denote the variance explained by the component. The bottom row shows boxplots of the PCGA ranks of site chronologies grouped by elevation (B, low <1,200 m, mid = 1,200 to 1,800 m, high = 1,800 to 2,200 m, forest line >2,200 m a.s.l.) and species (C, LD, Larix decidua; PA, Picea abies; PN, Pinus nigra; PS, Pinus sylvestris; PC, Pinus cembra). In the boxplots, the upper and lower hinges correspond to the first and third quartiles, the whiskers extend to a maximum of 1.5 times of the inter-quartile range, and points are outliers. Different letters in the boxplots indicate significant differences between elevations or species (p < 0.05).
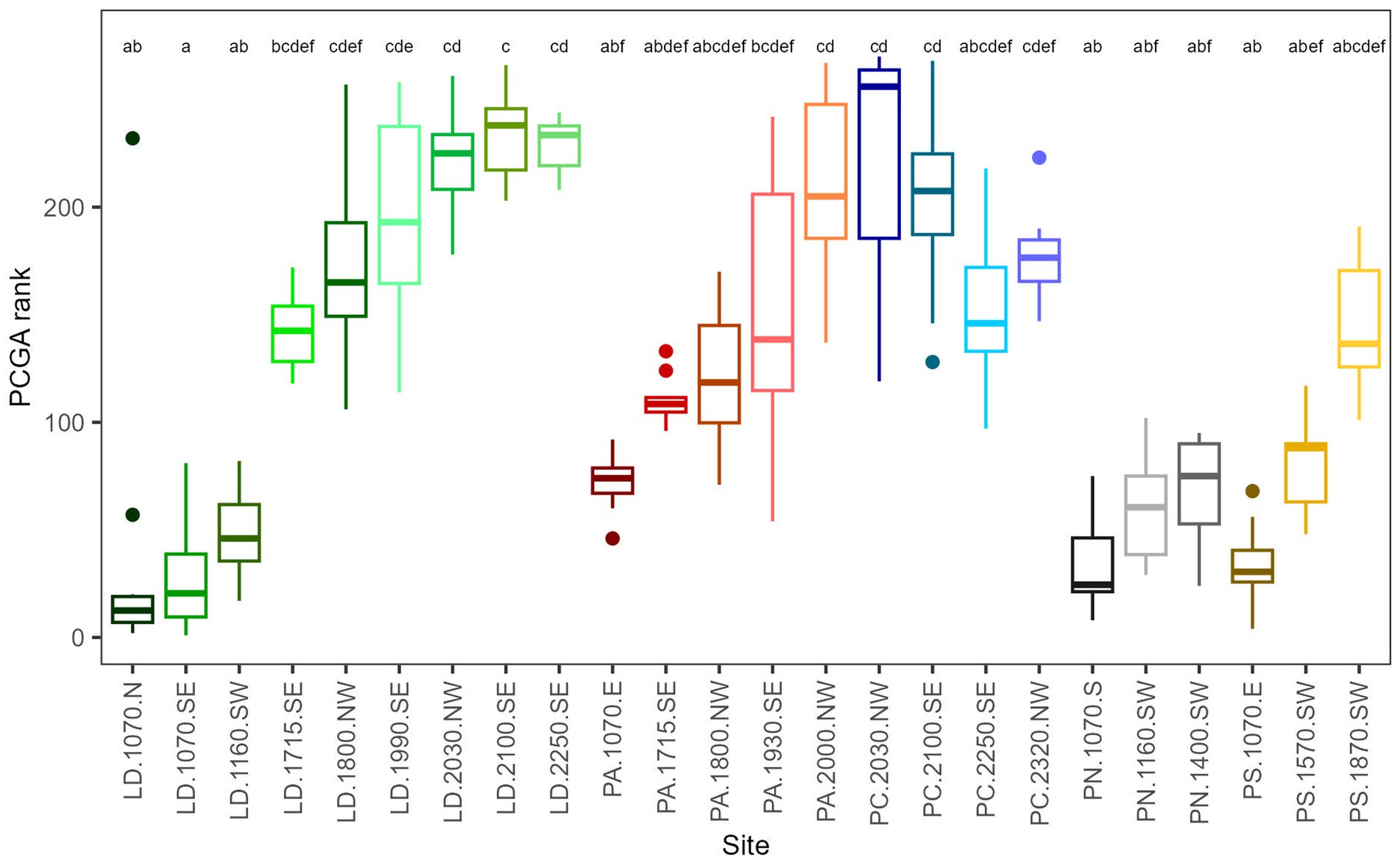
Figure 5. Boxplots of PCGA ranks for a tree level PCGA using 30 years spline-detrend, total ring width RWI (for PCGA biplot, see Supplementary Figure S4). The upper and lower hinges correspond to the first and third quartiles, the whiskers extend to a maximum of 1.5 times of the inter-quartile range, and points are outliers. Different letters above the boxplots indicate significant differences between species or elevations (p < 0.05). In the site names (x-axis), LD, Larix decidua; PA, Picea abies; PN, Pinus nigra; PS, Pinus sylvestris; PC, Pinus cembra, numbers are the elevation, and the last letters the aspect.
3.3 Climate-growth relationships
Static seasonal climate-growth relationships showed by far more positive than negative correlations, mainly with precipitation and SPEI at low-elevation and mid-elevation (Figure 6; Supplementary Figures S5–S7), indicating the importance of water availability. Within SPEI correlations, those correlations with SPEI12 were higher than with SPEI3 and SPEI1, showing that constant, long-term water supply was essential. In general, significant temperature correlations were less frequent. Positive ones were observed mainly in summer of the current year and previous autumn temperatures at high elevations and the forest line. In contrast, significant negative correlations were found with previous summer and autumn temperatures at low-, mid-, and high elevations. Most sites with significant climate correlations and the highest correlation coefficients were found for current summer and current spring, followed by correlations with previous autumn and winter conditions. Growth correlations with previous summer and previous spring were rare.
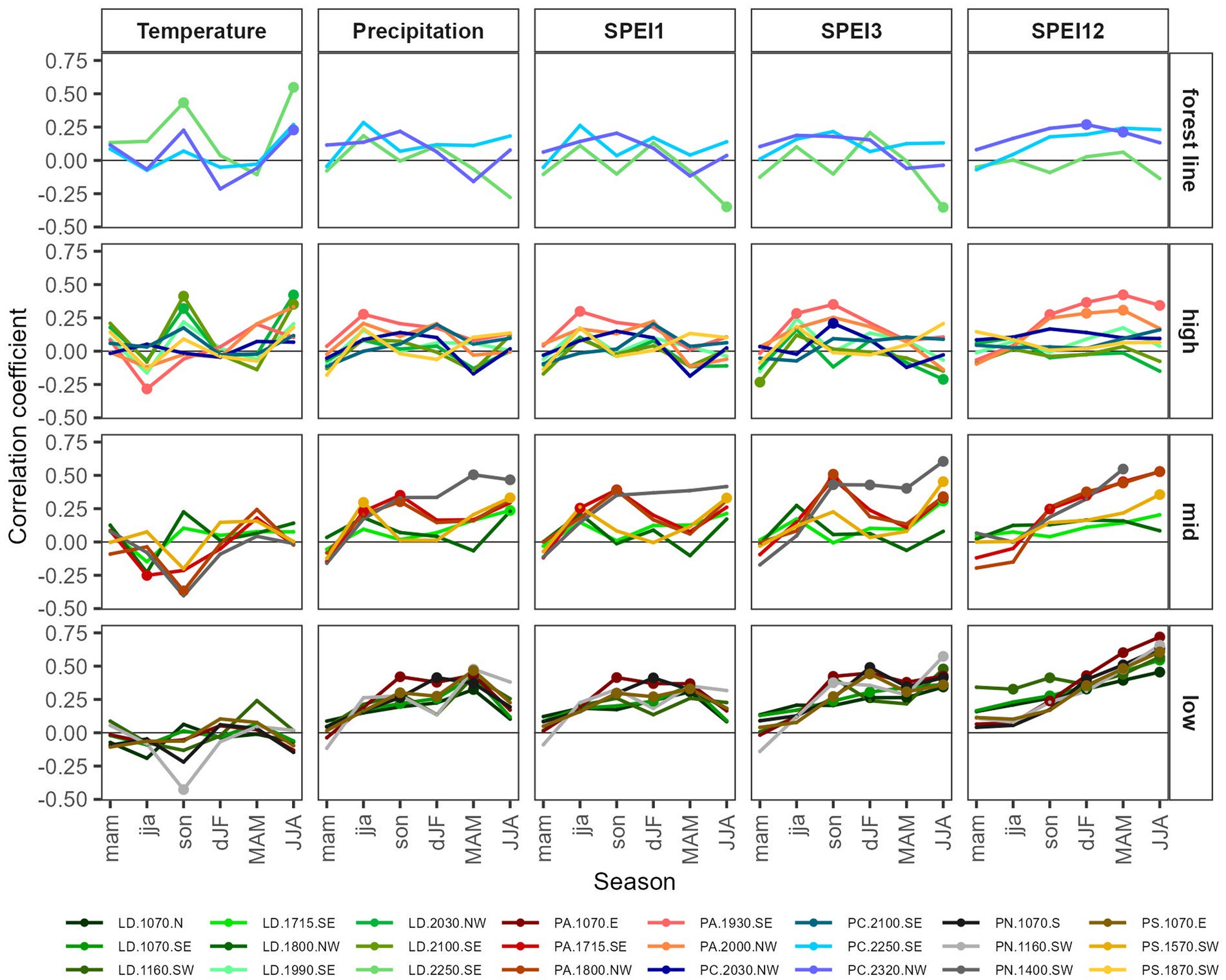
Figure 6. Seasonal static correlations between pre-whitened residual total tree-ring chronologies (calculated using 30 years splines) and seasonal mean temperature, total precipitation, and mean 1 month, 3 months, and 12 months SPEI (standardized precipitation-evapotranspiration index) for years with a sampling depth of at least five trees per site. Seasons: mam = previous spring, jja = previous summer, son = previous autumn, dJF = current winter, MAM = current spring, JJA = current summer. Full circles indicate significant relationships at p < 0.01. See Supplementary Figures S5, S6 for climate correlations of early wood and late wood.
Overall the highest positive correlation coefficients were observed with current spring- and summer SPEI and precipitation. At species level,they were strongest for low- and mid-elevation PN, followed by PA, PS and LD (Supplementary Figure S7). Positive growth correlation with temperature was significant for LD for summer of the current year and previous autumn at high elevation and the forest line and for PC at the North Slope forest line. Strongly negative correlation coefficients were found in previous autumn temperature for PN and PA. More negative growth correlations were observed with current summer SPEI1 and SPEI3 for LD at high elevation and the forest line but also with previous spring SPEI for LD at high elevation and PA at mid elevation and high elevation. Finally, significant negative correlations were also found with previous summer temperatures for PA at mid-elevation and high elevation. Climate correlations of early wood were similar to those of total ring width. In contrast, late wood showed less significant growth correlations with the previous year and current spring conditions but showed more significant growth correlations with current summer climate variables (Supplementary Figure S7).
In contrast to seasonal or monthly climate-growth correlations which use a-priori defined, arbitrary time windows, the daily climate data approach provides the exact start date and end date of the time period with the highest correlation between the respective climate (temperature and precipitation) and growth (total ring, early wood, and late wood widths) variables. As such, daily climate data should display any differences more explicitly in climate-growth correlations between species or along the elevational gradient. The highest correlations were found for precipitation at low-elevation for all species and at mid-elevation PN sites. The optimal time periods for these mainly started from October to November of the previous year and lasted until June to August of the current year (Figure 7). When considering only current year data, the correlation coefficients were mostly slightly lower, and the optimal time periods started from mid-March to 1st May of the current year and lasted again until June to August. At sites higher than 1,700 m a.s.l., in general, correlations with precipitation were decreased. The exception is PA for which the correlation remained relatively strong. Optimal periods at high elevations were shorter and most sites covered only the previous growing season. A significant positive correlation with precipitation in the current year occurred up to 1,900 m, covering the entire growing season. Above that elevation, correlations with precipitation were found only at one LD and at two PC sites at the South Slope. Significant negative correlations with precipitation were rare. When detected, they were found mainly at three high-elevation/forest line LD sites for periods in the early current growing season.
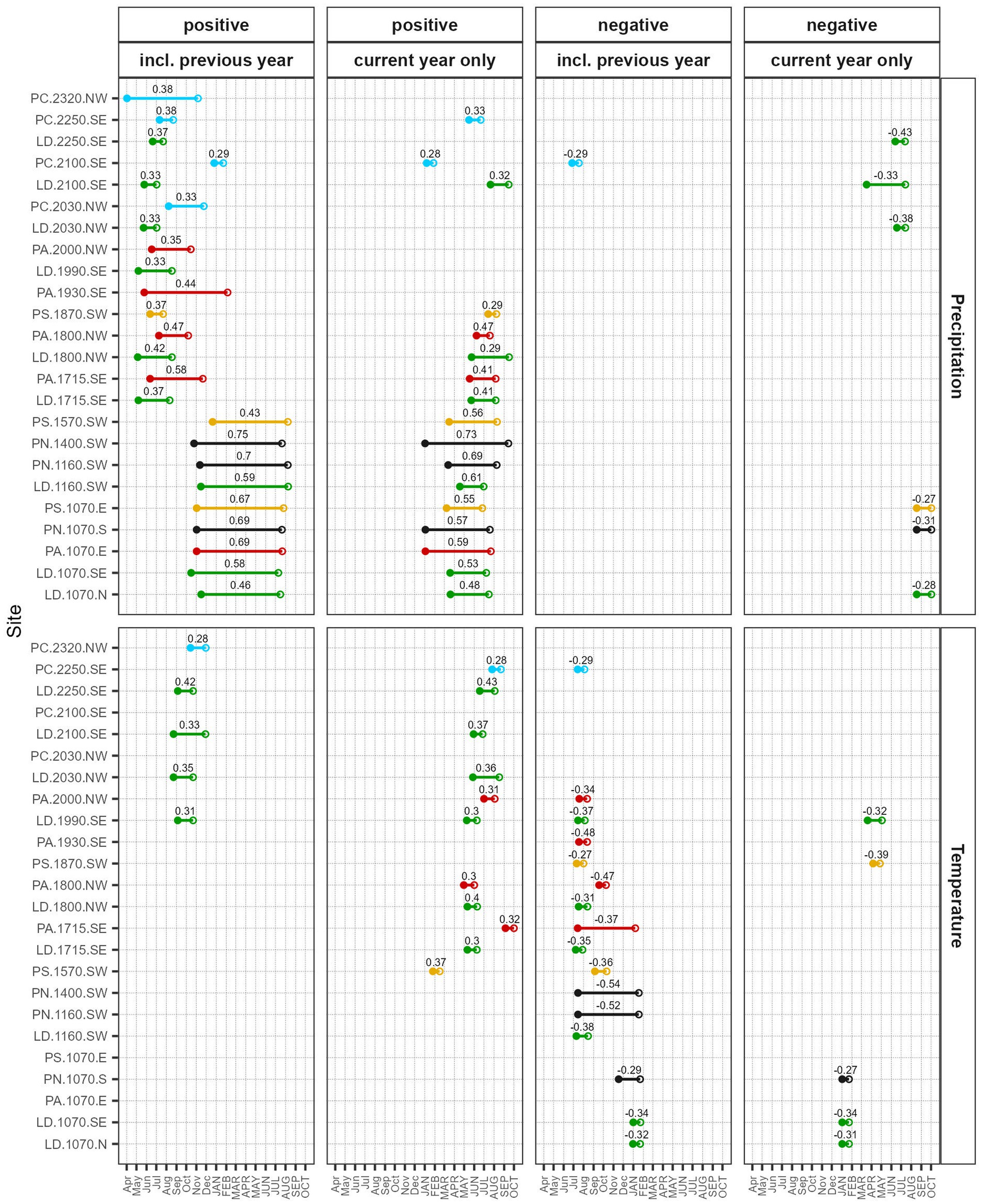
Figure 7. Optimal window width (line), starting (full circle), and end day (empty circle) for the highest positive and negative climate-growth correlations (numbers in the plot) of total ring width with temperature and precipitation using daily data. Both optimal periods starting in the previous year and for the current year only were calculated. Months in lower case letters at the x-axis indicate the previous year, capital letters indicate the current year. Only significant (p < 0.01) correlations are shown.
Significant positive correlations with previous year temperatures occurred almost exclusively at high-elevation and forest line LD-sites (Figure 7). Significant positive correlations with current year temperatures were found only above 1,570 m a.s.l., again mainly for LD but also for PA, and for short periods during the growing season. In contrast, significant negative correlations were observed more often with previous than with current year temperatures. At mid-elevation and high-elevation sites, these negative correlations were highest for periods covering the growing season of the previous year. At lower sites, winter temperatures were negatively correlated with tree growth.
Overall, daily correlations with early wood width were similar to the ones to total ring withs with slightly earlier optimal periods (Supplementary Figure S8). In contrast, daily correlations with late wood width were often lower for previous year and higher for current year conditions and had later optimal periods (Supplementary Figure S9). Growth correlations with precipitation were mostly stable, especially for current year optimal periods (Supplementary Figure S10). When including periods starting in the previous year, growth correlations decreased after the mid-1990s at low-elevation PA and LD sites but increased at some mid-elevation, high-elevation, and forest line sites (Supplementary Figure S11). Both positive and negative temperature correlations got stronger for 30 years ending after the 1960s at some low-elevations sites but decreased in recent years, especially at some high-elevation sites (Supplementary Figures S12, S13).
4 Discussion
4.1 Patterns of tree growth
The low variability of tree age observed at low elevation and mid elevation for LD, PA, PN, and PS was a consequence of anthropogenic reforestation in the region from the 1890s to the 1960s, which was conducted mainly for erosion control on formerly pastured slopes (Vacik et al., 2010b). Only at high elevations above 2,000 m (for PS, which is rather rare in the study area, also at 1,570 m a.s.l.), a more diverse age structure indicated a prevalence of natural regeneration or selective logging in the past. Nonetheless, the relatively young age of most trees present at high-elevation sites was due to forest management and the reforestation of pastures. Especially on the South Slope, pasturing was more intense in previous centuries and was partially abandoned in the last decades (Vittoz et al., 2008). DBH and tree height varied at even-aged sites, highlighting the importance of competition and microhabitat conditions (Wieser et al., 2019; Schmid et al., 2021). Interestingly, mean DBH and tree heights of PA were among the highest of all species. This occurred even though PA is generally considered susceptible to drought (Caudullo et al., 2016), and its growth was strongly correlated with water supply at all investigated sites. In contrast, low species-specific growth rates of PS and PC were indicated by comparatively low average tree heights and DBH (Caudullo and de Rigo, 2016). For PC, generally harsh growing conditions in its high-elevation habitats were an additional reason for slow growth.
In general, the mean ring width for all species increased with elevation and reached the highest values at nearly 2,000 m a.s.l., corresponding to LD and PA sites at the South Slope. There were two exceptions to this trend: some very young stands at low elevation with age-related wide rings (Speer, 2010) and PS with a maximum at relatively low 1,570 m a.s.l. In contrast to our findings, other tree-ring studies along elevational gradients at more humid sites in the Swiss (Affolter et al., 2010) and German Alps (Hartl-Meier et al., 2014a) found decreasing mean ring widths at least above 1,000 m a.s.l. for LD, PA, and PS. Additionally, we observed higher mean ring widths at the South Slope than at the North Slope for LD, PA, and, especially, PC. Conversely, little differences between opposing slopes were observed by King et al. (2013) in the Swiss Alps.
Generally, higher SNR, EPS, OIC, and rbar values at low elevations indicate a strong common driver determining tree growth at these sites. This was further supported by high GLK values between low-elevation sites even between different species. The most noticeable trends in BAI were an increase in growth since the 1970s which was found at most high-elevation and forest line LD sites at the North Slope high-elevation PA site but not for PC sites. Such high-elevation growth increments in recent decades for PA and LD but not for PC were also observed in the Central Eastern Alps (Oberhuber et al., 2020) and in the French Alps (Rolland et al., 1998; Rozenberg et al., 2020). Additionally, a growth increase in PA near the forest line in recent decades was also found in the Northern Limestone Alps in Germany and Austria (Hartl-Meier et al., 2014b) and in the Sudetes and Carpathians (Ponocna et al., 2016). In addition to rising temperatures, several additional factors could contribute to increasing tree growth rates at high elevation detected over the last decades. These supplementary factors include (Wieser et al., 2016), the increasing atmospheric deposition of nutrients such as nitrogen (Braun et al., 2010), and for LD the absence of regular outbreaks of larch budmoth (Zeiraphera diniana Guénée) after the 1980s (Peters et al., 2017). The lack of growth increment of PC found over the same period may be related to increasing stand densities (Gruber et al., 2022) but also to the strict limitation of daily tree growth by VPD using automatic dendrometers, as observed in an earlier study in the region (Obojes et al., 2022). Similarly, Saulnier et al. (2011) found evidence for water limitation of PC at high-elevation sites in the southern French Alps, and Vittoz et al. (2008) observed drought stress at the lower limits of PC occurrence approximately 2,100 to 2,200 m a.s.l. in southwestern Switzerland.
The beginning of a period with high BAI from the 1970s to early 2000s at most mid-elevation and some low-elevation sites coincided with more humid conditions. In addition to climatic reasons, management interventions could also be a reason for temporary growth releases at some of these sites. The 2003 heatwave and the following dry years led to a distinct growth reduction at all low elevation and mid elevation and even some south-exposed high-elevation sites. Similar growth declines during and after drought years were also observed in other studies. These include PA at sites below 1,400 m a.s.l. in the humid German and Austrian Northern Limestone Alps (Hartl-Meier et al., 2014b) and LD stands at a dry montane site in the Austrian Alps (Schuster and Oberhuber, 2013b). Negative growth reactions to drought were also previously observed for pine species. Specifically, they were reported for PS at low-elevation sites in the rather dry southwestern Swiss Alps (Affolter et al., 2010) and Western Italian Alps (Gonthier et al., 2010). For PN, drought impact on growth was found in the submontane zone in Eastern Austria (Leal et al., 2007), and for dense, planted stands, drought impact on growth was found in southern Spain (Sánchez-Salguero et al., 2013).
Clustering growth patterns via HCA and PCGA resulted in a clear separation of sites along the elevation transect. This was driven by decreasing temperatures and increasing precipitation/moisture availability with increasing elevation (Körner, 2007; Babst et al., 2013). Changing tree growth patterns are commonly observed in studies along elevational gradients (Affolter et al., 2010; Hartl-Meier et al., 2014b; Rozenberg et al., 2020; Jevšenak et al., 2021). Surprisingly, neither HCA nor PCGA found different growth patterns between species at low-elevation sites. Apparently, water limitation was so strong that all species were affected. With increasing elevation and better water supply, a certain separation between LD and the evergreen species could be observed in HCA. In contrast, other studies found distinct differences between species also at low elevation, for instance between LD and PA (Hartl-Meier et al., 2014a) or PN and PS (Marqués et al., 2016).
4.2 Climate impact versus species effects
The frequent and strong positive growth correlations with precipitation and SPEI for all species at low elevation confirmed the water-limitation of growth at these sites. The highest correlation coefficients were observed for PA which was expected to be the most drought-susceptible species. Similar uniform positive precipitation correlations among species in dry, low-elevation conditions were also observed in other studies; for PA, LD, and PS, similar uniform positive precipitation correlations were observed in the inner Alps (Schuster and Oberhuber, 2013b); for PN, PS, and PA, similar uniform positive precipitation correlations were observed in France (Lebourgeois et al., 2010, 2011); and for PA and LD, similar uniform positive precipitation correlations were observed in Switzerland (King et al., 2013). In contrast, Hartl-Meier et al. (2014a) found a different growth reaction of water-limited PA and indifferent LD at low elevation in the montane belt in the more humid northern Alps, while correlations with water supply were highest during the actual growing season, and also winter and previous autumn conditions were impacting tree growth. In fact, water availability for longer periods appeared to have the biggest influence on tree growth. This was reflected by stronger correlations with SPEI12 and SPEI3 compared with SPEI1 and precipitation as well as by the long optimal windows observed for positive precipitation correlations using daily data. Thereby, summer precipitation and SPEI1 mainly impacted LW at low elevation, while previous autumn water supply was correlated stronger with TR and EW. As expected, deciduous LD benefited most from carbohydrate reserves, which was assimilated in favorable conditions in autumn of the previous year (Kagawa et al., 2006; King et al., 2013; Jochner et al., 2018). However, positive growth correlations with water availability in autumn of the previous year were also found for all other present species (PA, PS, and PN) at low elevation. This was also observed in other dry environments for PS (Oberhuber et al., 1998; Rigling et al., 2002; Weber et al., 2007; Bouriaud and Popa, 2009) and PN (Martin-Benito et al., 2013; Sánchez-Salguero et al., 2013). A possible reason mentioned in the literature is stronger bud formation, enhancing photosynthesis in the following year (Takahashi et al., 2003; Weber et al., 2007). Winter precipitation, also expressed by higher winter SPEI, should increase soil moisture in the following spring either directly or after snowmelt. As a consequence, these factors positively correlated with growth at our low-elevation sites, a phenomenon which was also observed for different conifers in previous studies (Rigling et al., 2002; Lévesque et al., 2013; Saulnier et al., 2019).
At mid elevation and high elevation, PA still showed strong positive growth correlations with precipitation and SPEI from autumn of the previous year to summer of the current year at all sites, while they decreased for most other species. Similar differences between a strong precipitation response of PA and a weaker one of co-occurring species were found in other studies at elevations below 500–1,500 m a.s.l. (Wilson and Hopfmueller, 2001; Frank and Esper, 2005; Hartl-Meier et al., 2014a; Tumajer et al., 2017; Jevšenak et al., 2021). In addition, such differences extended up to the high-elevation distribution limit of LD and PA in the inner Alps (King et al., 2013). The surprising positive growth correlation with previous autumn to current spring SPEI12 at our highest, North Slope PC site was likely caused by protective effects of snow against soil frost and winter desiccation (Oberhuber, 2004; Saulnier et al., 2011).
Interestingly, we found no negative growth correlations with current year temperatures, co-occurring with positive precipitation correlations at our low-elevation and mid-elevation sites. In contrast, such a combination has been observed at lowland sites, especially for PA (Bouriaud and Popa, 2009; van der Maaten-Theunissen et al., 2013; Jevšenak et al., 2021). Apparently, higher temperatures than measured at our sites are necessary for a profound direct heat effect on physiological processes, such as photosynthesis and respiration, and consequently on tree growth (Rennenberg et al., 2006; Teskey et al., 2015). A maximum air temperature of 33.4°C at 1,070 m was estimated from temperature records at the Marienberg/Monte Maria station using a lapse rate of 0.6°C per 100 m of elevation. However, we found some negative growth correlations with previous summer and autumn temperatures, which may relate to the depletion of carbohydrate reserves due to increased respiration (Bouriaud and Popa, 2009; van der Maaten-Theunissen et al., 2013). This observation may also arise from the negative growth effects of enhanced cone/seed production, which is triggered by warm summer temperatures (Andreassen et al., 2006).
We also found less positive climate-growth correlations with temperature at high elevation and the forest line than expected based on the literature (Frank and Esper, 2005; Leal et al., 2007; Affolter et al., 2010; Hartl-Meier et al., 2014b). At our sites, they were restricted to LD at high-elevation and forest line sites and to PC at its highest and presumably coldest site. Consequently, the rising temperatures of the last decades preferentially benefitted LD growth at high-elevation and forest line sites, as indicated by an increasing BAI. The positive current summer temperature responses of LD were linked to negative precipitation and SPEI correlations. This was also found at high elevation in other studies in more humid conditions (Carrer and Urbinati, 2006; Hartl-Meier et al., 2014a; Jevšenak et al., 2021).
Climate warming was found to reduce the sensitivity of tree growth to summer temperature at cold-dry sites worldwide (Babst et al., 2019) and, specifically, in the Alps (Büntgen et al., 2012; Coppola et al., 2012; Oberhuber et al., 2020). This effect may be attributed to an earlier start of the growing season or growth depression due to lower water availability in late summer caused by earlier snowmelt (Saderi et al., 2019). In our case, we found a reduction in temperature sensitivity at high elevation for PC, which appears to be affected by drier conditions in recent years (Obojes et al., 2022). Moreover, a decreasing temperature response was also observed at some South Slope sites for LD with early snowmelt. While precipitation correlations at low elevation were consistently strong, the increasing response observed at some mid-elevation sites indicated a rising dependency on water availability. This was also found by Debel et al. (2021) at low-elevation sites in southern Germany.
5 Conclusion
Overall, our results support our first hypothesis that water availability limits growth for all conifers present at low elevation. We also found positive temperature correlations at high elevation, but they were less pronounced than expected. Growth patterns and climate-growth relationships of the study species were more similar than hypothesized, especially at low elevation. Nonetheless, we found the strongest positive growth correlations with water availability for, PA which was assumed to be the most drought-sensitive species. Our study showed that in inner-alpine areas, water availability at low elevations is a stronger limiting factor to tree growth than temperature at high elevations. Indeed, the long-term water supply including previous autumn and winter precipitation was as important as water availability during the actual growing season. Differences in climate-growth relationships between anisohydric LD and isohydric PA, PS, and PN were surprisingly small at low elevation. Observed and predicted further reductions in precipitation and snow fall/cover in the southern part of the Central European Alps are therefore anticipated as the main reason for a decline in forest growth and vitality in this region. Drought will impact trees either directly or by increasing their susceptibility to pests. In this regard, displacement of conifers with more drought-resistant broad leaves (for instances Quercus pubescens and Fraxinus ornus) at low elevations may be expected. This can already be observed in the Valais (Bigler et al., 2006; Eilmann et al., 2006; Weber et al., 2007) which displays conditions similar to the study area. The adaptation to such shifts in species compositions will be an important factor in future forest management strategies. The most stable and favorable growth conditions of the last decades were found at surprisingly high elevations approximately 2,000 m a.s.l., indicating that these might provide the best location for future timber production. The comparatively weak temperature correlations at the actual forest line can be explained by its depression due to historic and current pastures and the increasing temperatures observed in recent decades. With the ongoing abandonment of remote areas and the intensification of climate change, a rise in the forest line can be expected. However, different growth trends in PC compared with LD and PA indicate probable changes in future forest composition at the forest line, which will also be influenced by species-specific regeneration requirements.
Data availability statement
The raw data supporting the conclusions of this article will be made available by the authors, without undue reservation.
Author contributions
NO: Conceptualization, Data curation, Formal analysis, Investigation, Methodology, Project administration, Validation, Visualization, Writing – original draft, Writing – review & editing. SB: Data curation, Formal analysis, Investigation, Writing – review & editing. AM: Data curation, Formal analysis, Investigation, Writing – review & editing. ET: Conceptualization, Resources, Supervision, Writing – review & editing. WO: Conceptualization, Methodology, Resources, Supervision, Validation, Writing – review & editing. SM: Conceptualization, Resources, Supervision, Writing – review & editing. UT: Conceptualization, Funding acquisition, Resources, Supervision, Writing – review & editing.
Funding
The author(s) declare that financial support was received for the research, authorship, and/or publication of this article. This study was funded by the Autonomous Province of Bozen/Bolzano via its support of the LTSER platform Matsch/Mazia. Open Access publication costs were covered by the Department of Innovation, Research and University of the Autonomous Province of Bozen/Bolzano.
Acknowledgments
The LTSER platform Matsch/Mazia belongs to the national and international long-term ecological research networks (LTER-Italy, LTER Europe, and ILTER) and is supported financially by the Autonomous Province of Bozen/Bolzano. SM, WO, and UT are members of the research area “Mountain Regions” at the University of Innsbruck. The authors thanks the Department of Innovation, Research and University of the Autonomous Province of Bozen/Bolzano for covering the Open Access publication costs.
Conflict of interest
The authors declare that the research was conducted in the absence of any commercial or financial relationships that could be construed as a potential conflict of interest.
Publisher’s note
All claims expressed in this article are solely those of the authors and do not necessarily represent those of their affiliated organizations, or those of the publisher, the editors and the reviewers. Any product that may be evaluated in this article, or claim that may be made by its manufacturer, is not guaranteed or endorsed by the publisher.
Supplementary material
The Supplementary material for this article can be found online at: https://www.frontiersin.org/articles/10.3389/ffgc.2024.1332941/full#supplementary-material
References
Affolter, P., Büntgen, U., Esper, J., Rigling, A., Weber, P., Luterbacher, J., et al. (2010). Inner alpine conifer response to 20th century drought swings. Eur. J. For. Res. 129, 289–298. doi: 10.1007/s10342-009-0327-x
Andreassen, K., Solberg, S., Tveito, O. E., and Lystad, S. L. (2006). Regional differences in climatic responses of Norway spruce (Picea abies L. Karst) growth in Norway. For. Ecol. Manag. 222, 211–221. doi: 10.1016/j.foreco.2005.10.029
Anfodillo, T., Rento, S., Carraro, V., Furlanetto, L., Urbinati, C., and Carrer, M. (1998). Tree water relations and climatic variations at the alpine timberline: seasonal changes of sap flux and xylem water potential in Larix decidua Miller, Picea abies (L.) Karst. and Pinus cembra L. Ann. For. Sci. 55, 159–172. doi: 10.1051/forest:19980110
Auer, I., Böhm, R., Jurkovic, A., Lipa, W., Orlik, A., Potzmann, R., et al. (2007). HISTALP—historical instrumental climatological surface time series of the greater Alpine region. Int. J. Climatol. 27, 17–46. doi: 10.1002/joc.1377
Babst, F., Bouriaud, O., Poulter, B., Trouet, V., Girardin, M. P., and Frank, D. C. (2019). Twentieth century redistribution in climatic drivers of global tree growth. Sci. Adv. 5:eaat4313. doi: 10.1126/sciadv.aat4313
Babst, F., Poulter, B., Trouet, V., Tan, K., Neuwirth, B., Wilson, R., et al. (2013). Site- and species-specific responses of forest growth to climate across the European continent. Glob. Ecol. Biogeogr. 22, 706–717. doi: 10.1111/geb.12023
Badalotti, A., Anfodillo, T., and Grace, J. (2000). Evidence of osmoregulation in Larix decidua at alpine treeline and comparative responses to water availability of two co-occurring evergreen species. Ann. For. Sci. 57, 623–633. doi: 10.1051/forest:2000146
Barbeito, I., Brücker, R. L., Rixen, C., and Bebi, P. (2013). Snow fungi-induced mortality of Pinus cembra at the alpine treeline: evidence from plantations. Arct. Antarct. Alp. Res. 45, 455–470. doi: 10.1657/1938-4246-45.4.455
Becker, A., Körner, C., Brun, J.-J., Guisan, A., and Tappeiner, U. (2007). Ecological and land use studies along elevational gradients. Mt. Res. Dev. 27, 58–65. doi: 10.1659/0276-4741(2007)27[58:EALUSA]2.0.CO;2
Begueria, S., Serrano, V., and Sawasawa, H. (2017). “SPEI: calculation of standardised precipitation-evapotranspiration index” in R package version 1.7
Beguería, S., Vicente-Serrano, S. M., Reig, F., and Latorre, B. (2014). Standardized precipitation evapotranspiration index (SPEI) revisited: parameter fitting, evapotranspiration models, tools, datasets and drought monitoring. Int. J. Climatol. 34, 3001–3023. doi: 10.1002/JOC.3887
Bigler, C., Bräker, O. U., Bugmann, H., Dobbertin, M., and Rigling, A. (2006). Drought as an inciting mortality factor in Scots pine stands of the Valais, Switzerland. Ecosystems 9, 330–343. doi: 10.1007/s10021-005-0126-2
Bouriaud, O., and Popa, I. (2009). Comparative dendroclimatic study of Scots pine, Norway spruce, and silver fir in the Vrancea Range, Eastern Carpathian Mountains. Trees 23, 95–106. doi: 10.1007/s00468-008-0258-z
Braun, S., Thomas, V. F. D., Quiring, R., and Flückiger, W. (2010). Does nitrogen deposition increase forest production? The role of phosphorus. Environ. Pollut. 158, 2043–2052. doi: 10.1016/j.envpol.2009.11.030
Bunn, A., Korpela, M., Biondi, F., Campelo, F., Mérian, P., Qeadan, F., et al. (2016). dplR: Dendrochronology program library in R. Available at: https://cran.r-project.org/package=dplR
Büntgen, U., Frank, D., Neuenschwander, T., Esper, J., Büntgen, U., Frank, D., et al. (2012). Fading temperature sensitivity of alpine tree growth at its Mediterranean margin and associated effects on large-scale climate reconstructions. Clim. Change 114, 651–666. doi: 10.1007/s10584-012-0450-4
Buras, A., van der Maaten-Theunissen, M., van der Maaten, E., Ahlgrimm, S., Hermann, P., Simard, S., et al. (2016). Tuning the voices of a choir: detecting ecological gradients in time-series populations. PLoS One 11:e0158346. doi: 10.1371/journal.pone.0158346
Buscarini, S. (2018). Comparison of climate-growth relations of different conifers along elevation gradients in an inner-alpine dry valley. Bolzano: Free University of Bolzano.
Carrer, M., and Urbinati, C. (2006). Long-term change in the sensitivity of tree-ring growth to climate forcing in Larix decidua. New Phytol. 170, 861–872. doi: 10.1111/j.1469-8137.2006.01703.x
Caudullo, G., and de Rigo, D. (2016). “Pinus cembra in Europe: distribution, habitat, usage and threats,” in European atlas of forest tree species. J. San-Miguel-Ayanz, D. Rigode, G. Caudullo, T. Houston Durrant, and A. Mauri Luxembourg: European Union, e01bd9b
Caudullo, G., Tinner, W., and de Rigo, D. (2016). “Picea abies in Europe: distribution, habitat, usage and threats,” in European atlas of forest tree species. J. San-Miguel-Ayanz, D. Rigode, G. Caudullo, T. Houston Durrant, and A. Mauri Luxembourg: European Union, e012300
Charney, N. D., Babst, F., Poulter, B., Record, S., Trouet, V. M., Frank, D., et al. (2016). Observed forest sensitivity to climate implies large changes in 21st century North American forest growth. Ecol. Lett. 19, 1119–1128. doi: 10.1111/ele.12650
Coppola, A., Leonelli, G., Salvatore, M. C., Pelfini, M., and Baroni, C. (2012). Weakening climatic signal since mid-20th century in European larch tree-ring chronologies at different altitudes from the Adamello-Presanella Massif (Italian Alps). Quat. Res. 77, 344–354. doi: 10.1016/J.YQRES.2012.01.004
Cornes, R. C., van der Schrier, G., van den Besselaar, E. J. M., and Jones, P. D. (2018). An ensemble version of the E-OBS temperature and precipitation data sets. J. Geophys. Res. Atmos. 123, 9391–9409. doi: 10.1029/2017JD028200
Da Ronch, F., Caudullo, G., Tinner, W., and de Rigo, D. (2016). “Larix decidua and other larches in Europe: distribution, habitat, usage and threats,” in European atlas of forest tree species. J. San-Miguel-Ayanz, D. Rigode, G. Caudullo, T. Houston Durrant, and A. Mauri Luxembourg: European Union. e01e492.
Debel, A., Meier, W. J.-H., and Bräuning, A. (2021). Climate signals for growth variations of F. sylvatica, P. abies, and P. sylvestris in Southeast Germany over the past 50 years. Forests 12:1433. doi: 10.3390/f12111433
Durrant, T. H., Rigo, D. D., and Caudullo, G. (2016). “Pinus sylvestris in Europe: distribution, habitat, usage and threats,” in European atlas of forest tree species. J. San-Miguel-Ayanz, D. Rigode, G. Caudullo, T. Houston Durrant, and A. Mauri. Luxembourg: European Union, 132–133. Available at: https://w3id.org/mtv/FISE-Comm/v01/e016b94
Eilmann, B., and Rigling, A. (2012). Tree-growth analyses to estimate tree species’ drought tolerance. Tree Physiol. 32, 178–187. doi: 10.1093/treephys/tps004
Eilmann, B., Weber, P., Rigling, A., and Eckstein, D. (2006). Growth reactions of Pinus sylvestris L. and Quercus pubescens Willd. to drought years at a xeric site in Valais, Switzerland. Dendrochronologia 23, 121–132. doi: 10.1016/j.dendro.2005.10.002
Enescu, C. M., de Rigo, D., Caudullo, G., Mauri, A., and Houston Durrant, T. (2016). “Pinus nigra in Europe: distribution, habitat, usage and threats,” in European atlas of forest tree species. J. San-Miguel-Ayanz, D. Rigode, G. Caudullo, T. Houston Durrant, and A. Mauri. Luxembourg: European Union, e015138.
Etzold, S., Sterck, F., Bose, A. K., Braun, S., Buchmann, N., Eugster, W., et al. (2021). Number of growth days and not length of the growth period determines radial stem growth of temperate trees. Ecol. Lett. 25, 427–439. doi: 10.1111/ele.13933
Fontana, V., Radtke, A., Walde, J., Tasser, E., Wilhalm, T., Zerbe, S., et al. (2014). What plant traits tell us: consequences of land-use change of a traditional agro-forest system on biodiversity and ecosystem service provision. Agric. Ecosyst. Environ. 186, 44–53. doi: 10.1016/j.agee.2014.01.006
Frank, D., and Esper, J. (2005). Characterization and climate response patterns of a high-elevation, multi-species tree-ring network in the European Alps. Dendrochronologia 22, 107–121. doi: 10.1016/j.dendro.2005.02.004
Frei, C., and Schär, C. (1998). A precipitation climatology of the Alps from high-resolution rain-gauge observations. Int. J. Climatol. 18, 873–900. doi: 10.1002/(SICI)1097-0088(19980630)18:8<873::AID-JOC255>3.0.CO;2-9
Garbarino, M., Lingua, E., Subirà, M. M., and Motta, R. (2011). The larch wood pasture: structure and dynamics of a cultural landscape. Eur. J. For. Res. 130, 491–502. doi: 10.1007/s10342-010-0437-5
Gonthier, P., Giordano, L., and Nicolotti, G. (2010). Further observations on sudden diebacks of Scots pine in the European Alps. For. Chron. 86, 110–117. doi: 10.5558/tfc86110-1
Gruber, A., Oberhuber, W., and Wieser, G. (2022). Treeline-Quo Vadis? An ecophysiological approach. Forests 13:857. doi: 10.3390/F13060857
Hartl-Meier, C., Dittmar, C., Zang, C., and Rothe, A. (2014a). Mountain forest growth response to climate change in the northern limestone Alps. Trees 28, 819–829. doi: 10.1007/s00468-014-0994-1
Hartl-Meier, C., Zang, C., Dittmar, C., Esper, J., Göttlein, A., and Rothe, A. (2014b). Vulnerability of Norway spruce to climate change in mountain forests of the European Alps. Clim. Res. 60, 119–132. doi: 10.3354/cr01226
Hlásny, T., Zimová, S., Merganičová, K., Štěpánek, P., Modlinger, R., and Turčáni, M. (2021). Devastating outbreak of bark beetles in the Czech Republic: drivers, impacts, and management implications. For. Ecol. Manag. 490:119075. doi: 10.1016/j.foreco.2021.119075
Holmes, R. (1983). Computer-assisted quality control in tree-ring dating and measurement. Tree-Ring Bull. 43, 69–78.
Holtmeier, F.-K. (1990). Disturbance and management problems in larch-cembra pine forests in Europe. Symposium on Whitebark Pine Ecosystems: Ecology and Management of a High-Mountain Resource, 25–36.
Hunziker, S., Begert, M., Scherrer, S. C., Rigling, A., and Gessler, A. (2022). Below average midsummer to early autumn precipitation evolved into the main driver of sudden Scots pine vitality decline in the Swiss Rhône Valley. Front. For. Glob. Change 5:874100. doi: 10.3389/ffgc.2022.874100
Jaime, L., Batllori, E., Ferretti, M., and Lloret, F. (2022). Climatic and stand drivers of forest resistance to recent bark beetle disturbance in European coniferous forests. Glob. Change Biol. 28, 2830–2841. doi: 10.1111/GCB.16106
Jevšenak, J., and Levanič, T. (2018). dendroTools: R package for studying linear and nonlinear responses between tree-rings and daily environmental data. Dendrochronologia 48, 32–39. doi: 10.1016/j.dendro.2018.01.005
Jevšenak, J., Tychkov, I., Gričar, J., Levanič, T., Tumajer, J., Prislan, P., et al. (2021). Growth-limiting factors and climate response variability in Norway spruce (Picea abies L.) along an elevation and precipitation gradients in Slovenia. Int. J. Biometeorol. 65, 311–324. doi: 10.1007/s00484-020-02033-5
Jochner, M., Bugmann, H., Nötzli, M., and Bigler, C. (2018). Tree growth responses to changing temperatures across space and time: a fine-scale analysis at the treeline in the Swiss Alps. Trees 32, 645–660. doi: 10.1007/s00468-017-1648-x
Kagawa, A., Sugimoto, A., and Maximov, T. C. (2006). Seasonal course of translocation, storage and remobilization of 13C pulse-labeled photoassimilate in naturally growing Larix gmelinii saplings. New Phytol. 171, 793–804. doi: 10.1111/J.1469-8137.2006.01780.X
King, G. M., Gugerli, F., Fonti, P., and Frank, D. C. (2013). Tree growth response along an elevational gradient: climate or genetics? Oecologia 173, 1587–1600. doi: 10.1007/s00442-013-2696-6
Körner, C. (2007). The use of “altitude” in ecological research. Trends Ecol. Evol. 22, 569–574. doi: 10.1016/j.tree.2007.09.006
Kotlarski, S., Gobiet, A., Morin, S., Olefs, M., Rajczak, J., and Samacoïts, R. (2022). 21st century alpine climate change. Clim. Dyn. 60, 65–86. doi: 10.1007/s00382-022-06303-3
Leal, S., Melvin, T. M., Grabner, M., Wimmer, R., and Briffa, K. R. (2007). Tree-ring growth variability in the Austrian Alps: the influence of site, altitude, tree species and climate. Boreas 36, 426–440. doi: 10.1080/03009480701267063
Lebourgeois, F., Mérian, P., Courdier, F., Ladier, J., and Dreyfus, P. (2011). Instability of climate signal in tree-ring width in Mediterranean mountains: a multi-species analysis. Trees 26, 715–729. doi: 10.1007/s00468-011-0638-7
Lebourgeois, F., Rathgeber, C. B. K., and Ulrich, E. (2010). Sensitivity of French temperate coniferous forests to climate variability and extreme events (Abies alba, Picea abies and Pinus sylvestris). J. Veg. Sci. 21, 364–376. doi: 10.1111/j.1654-1103.2009.01148.x
Lemaire, J., Vennetier, M., Prévosto, B., and Cailleret, M. (2022, 2022). Interactive effects of abiotic factors and biotic agents on Scots pine dieback: a multivariate modeling approach in Southeast France. For. Ecol. Manag. 526:120543. doi: 10.1016/j.foreco.2022.120543
Leo, M., Oberhuber, W., Schuster, R., Grams, T. E. E., Matyssek, R., and Wieser, G. (2014). Evaluating the effect of plant water availability on inner alpine coniferous trees based on sap flow measurements. Eur. J. For. Res. 133, 691–698. doi: 10.1007/s10342-013-0697-y
Leuschner, C., and Ellenberg, H. (2017). “Abiotic conditions, flora, ecosystem functions and recent human influence” in Ecology of Central European forests: vegetation ecology of Central Europe. eds. C. Leuschner and H. Ellenberg (Cham: Springer International Publishing)
Lévesque, M., Saurer, M., Siegwolf, R., Eilmann, B., Brang, P., Bugmann, H., et al. (2013). Drought response of five conifer species under contrasting water availability suggests high vulnerability of Norway spruce and European larch. Glob. Change Biol. 19, 3184–3199. doi: 10.1111/gcb.12268
Marqués, L., Camarero, J. J., Gazol, A., and Zavala, M. A. (2016). Drought impacts on tree growth of two pine species along an altitudinal gradient and their use as early-warning signals of potential shifts in tree species distributions. For. Ecol. Manag. 381, 157–167. doi: 10.1016/j.foreco.2016.09.021
Martin-Benito, D., Beeckman, H., and Cañellas, I. (2013). Influence of drought on tree rings and tracheid features of Pinus nigra and Pinus sylvestris in a Mesic Mediterranean forest. Eur. J. For. Res. 132, 33–45. doi: 10.1007/s10342-012-0652-3
Maxwell, R. S., and Larsson, L. A. (2021). Measuring tree-ring widths using the CooRecorder software application. Dendrochronologia 67:125841. doi: 10.1016/J.DENDRO.2021.125841
Meurer, A. (2015). Dendroökologische Untersuchungen an Lärche und Zirbe im Matschertal, Südtirol. Dresden (Germany): Technische Universität Dresden.
Moris, J. V., Vacchiano, G., Ascoli, D., and Motta, R. (2017). Alternative stable states in mountain forest ecosystems: the case of European larch (Larix decidua) forests in the western Alps. J. Mt. Sci. 14, 811–822. doi: 10.1007/s11629-016-4328-1
Motta, R., and Lingua, E. (2005). Human impact on size, age, and spatial structure in a mixed European larch and Swiss stone pine forest in the Western Italian Alps. Can. J. For. Res. 35, 1809–1820. doi: 10.1139/x05-107
Motta, R., Morales, M., and Nola, P. (2006). Human land-use, forest dynamics and tree growth at the treeline in the Western Italian Alps. Ann. For. Sci. 63, 739–747. doi: 10.1051/forest:2006055
Netherer, S., Panassiti, B., Pennerstorfer, J., and Matthews, B. (2019). Acute drought is an important driver of bark beetle infestation in Austrian Norway spruce stands. Front. For. Glob. Change 2:39. doi: 10.3389/ffgc.2019.00039
Oberhuber, W. (2004). Influence of climate on radial growth of Pinus cembra within the alpine timberline ecotone. Tree Physiol. 24, 291–301. doi: 10.1093/treephys/24.3.291
Oberhuber, W., Bendler, U., Gamper, V., Geier, J., Hölzl, A., Kofler, W., et al. (2020). Growth trends of coniferous species along elevational transects in the Central European Alps indicate decreasing sensitivity to climate warming. Forests 11, 1–13. doi: 10.3390/f11020132
Oberhuber, W., Gruber, A., Kofler, W., and Swidrak, I. (2014). Radial stem growth in response to microclimate and soil moisture in a drought-prone mixed coniferous forest at an inner Alpine site. Eur. J. For. Res. 133, 467–479. doi: 10.1007/s10342-013-0777-z
Oberhuber, W., Kofler, W., Schuster, R., and Wieser, G. (2015). Environmental effects on stem water deficit in co-occurring conifers exposed to soil dryness. Int. J. Biometeorol. 59, 417–426. doi: 10.1007/s00484-014-0853-1
Oberhuber, W., Stumböck, M., and Kofler, W. (1998). Climate-tree-growth relationships of Scots pine stands (Pinus sylvestris L.) exposed to soil dryness. Trees 13, 19–27. doi: 10.1007/PL00009734
Obojes, N., Meurer, A. K., Newesely, C., Tasser, E., Oberhuber, W., Mayr, S., et al. (2022). Swiss stone pine growth benefits less from recent warming than European larch at a dry-inner alpine forest line as it reacts more sensitive to humidity. Agric. For. Meteorol. 315:108788. doi: 10.1016/j.agrformet.2021.108788
Pasztor, F., Matulla, C., Rammer, W., and Lexer, M. J. (2014). Drivers of the bark beetle disturbance regime in Alpine forests in Austria. For. Ecol. Manag. 318, 349–358. doi: 10.1016/j.foreco.2014.01.044
Perzl, F., Bono, A., Garbarino, M., Motta, R., Perzl, F., Bono, A., et al. (2021). “Protective effects of forests against gravitational natural hazards” in Protective forests as ecosystem-based solution for disaster risk reduction. Rijeka, Croatia: IntechOpen.
Peters, R. L., Klesse, S., Fonti, P., and Frank, D. C. (2017). Contribution of climate vs. larch budmoth outbreaks in regulating biomass accumulation in high-elevation forests. For. Ecol. Manag. 401, 147–158. doi: 10.1016/j.foreco.2017.06.032
Ponocna, T., Spyt, B., Kacka, R., Büntgen, U., and Treml, V. (2016). Growth trends and climate responses of Norway spruce along elevational gradients in east-Central Europe. Trees 30, 1633–1646. doi: 10.1007/s00468-016-1396-3
Rennenberg, H., Loreto, F., Polle, A., Brilli, F., Fares, S., Beniwal, R. S., et al. (2006). Physiological responses of forest trees to heat and drought. Plant Biol. 8, 556–571. doi: 10.1055/s-2006-924084
Rigling, A., Bräker, O., Schneiter, G., and Schweingruber, F. (2002). Intra-annual tree-ring parameters indicating differences in drought stress of Pinus sylvestris forests within the Erico-Pinion in the Valais (Switzerland). Plant Ecol. 163, 105–121. doi: 10.1023/A:1020355407821
Rolland, C., Petitcolas, V., and Michalet, R. (1998). Changes in radial tree growth for Picea abies, Larix decidua, Pinus cembra and Pinus uncinata near the alpine timberline since 1750. Trees 13, 40–53. doi: 10.1007/PL00009736
Rozenberg, P., Chauvin, T., Escobar-Sandoval, M., Huard, F., Shishov, V., Charpentier, J.-P., et al. (2020). Climate warming differently affects Larix decidua ring formation at each end of a French Alps elevational gradient. Ann. For. Sci. 77:54. doi: 10.1007/s13595-020-00958-w
Saderi, S., Rathgeber, C. B. K., Rozenberg, P., and Fournier, M. (2019). Phenology of wood formation in larch (Larix decidua Mill.) trees growing along a 1,000 m elevation gradient in the French Southern Alps. Ann. For. Sci. 76:89. doi: 10.1007/s13595-019-0866-3
Sánchez-Salguero, R., Camarero, J. J., Dobbertin, M., Fernández-Cancio, Á., Vilà-Cabrera, A., Manzanedo, R. D., et al. (2013). Contrasting vulnerability and resilience to drought-induced decline of densely planted vs. natural rear-edge Pinus nigra forests. For. Ecol. Manag. 310, 956–967. doi: 10.1016/j.foreco.2013.09.050
Saulnier, M., Edouard, J. L., Corona, C., and Guibal, F. (2011). Climate/growth relationships in a Pinus cembra high-elevation network in the Southern French Alps. Ann. For. Sci. 68, 189–200. doi: 10.1007/s13595-011-0020-3
Saulnier, M., Edouard, J.-L., Guibal, F., Corona, C., and Stoffel, M. (2019). Climate-growth relationships in a Larix decidua Mill. network in the French Alps. Sci. Total Environ. 664, 554–566. doi: 10.1016/j.scitotenv.2019.01.404
Schmid, U., Bigler, C., Frehner, M., and Bugmann, H. (2021). Abiotic and biotic determinants of height growth of Picea abies regeneration in small forest gaps in the Swiss Alps. For. Ecol. Manag. 490:119076. doi: 10.1016/J.FORECO.2021.119076
Schuster, R., and Oberhuber, W. (2013a). Age-dependent climate-growth relationships and regeneration of Picea abies in a drought-prone mixed coniferous forest in the Alps. Can. J. For. Res. 43, 609–618. doi: 10.1139/cjfr-2012-0426.Age-dependent
Schuster, R., and Oberhuber, W. (2013b). Drought sensitivity of three co-occurring conifers within a dry inner Alpine environment. Trees 27, 61–69. doi: 10.1007/s00468-012-0768-6
Senf, C., and Seidl, R. (2021). Storm and fire disturbances in Europe: distribution and trends. Glob. Change Biol. 27, 3605–3619. doi: 10.1111/GCB.15679
Staffler, H., and Karrer, G. (2001). Thermophilous forests in the Vinschgau valley (South-Tirol/Italy). Sauteria 11, 301–358.
Takahashi, K., Azuma, H., and Yasue, K. (2003). Effects of climate on the radial growth of tree species in the upper and lower distribution limits of an altitudinal ecotone on Mount Norikura, Central Japan. Ecol. Res. 18, 549–558. doi: 10.1046/J.1440-1703.2003.00577.X
Teskey, R., Wertin, T., Bauweraerts, I., Ameye, M., McGuire, M. A., and Steppe, K. (2015). Responses of tree species to heat waves and extreme heat events. Plant Cell Environ. 38, 1699–1712. doi: 10.1111/pce.12417
Tumajer, J., Altman, J., Štěpánek, P., Treml, V., Doležal, J., and Cienciala, E. (2017). Increasing moisture limitation of Norway spruce in Central Europe revealed by forward modelling of tree growth in tree-ring network. Agric. For. Meteorol. 247, 56–64. doi: 10.1016/j.agrformet.2017.07.015
Vacchiano, G., Garbarino, M., Borgogno Mondino, E., and Motta, R. (2012). Evidences of drought stress as a predisposing factor to Scots pine decline in Valle d’Aosta (Italy). Eur. J. For. Res. 131, 989–1000. doi: 10.1007/s10342-011-0570-9
Vacik, H., de Jel, S., Rubrecht, H., Gruber, G., Pividori, M., Del Favero, R., et al. (2010a). Waldtypisierung Südtirol Band 1 Waldtypen, Wuchsgebiete, Bestimmungsschlüssel. Lana: Autonome Provinz Bozen-Südtirol, Abteilung Forstwirtschaft, Amt für Forstplanung.
Vacik, H., de Jel, S., Rubrecht, H., Gruber, G., Pividori, M., Del Favero, R., et al. (2010b). Waldtypisierung Südtirol Band 2 Waldgruppen, Naturräume, Glossar. Lana: Autonome Provinz Bozen-Südtirol, Abteilung Forstwirtschaft, Amt für Forstplanung.
van der Maaten-Theunissen, M., Kahle, H.-P., and van der Maaten, E. (2013). Drought sensitivity of Norway spruce is higher than that of silver fir along an altitudinal gradient in southwestern Germany. Ann. For. Sci. 70, 185–193. doi: 10.1007/s13595-012-0241-0
Vittoz, P., Rulence, B., Largey, T., and Freléchoux, F. (2008). Effects of climate and land-use change on the establishment and growth of Cembran pine Pinus cembra. Arct. Antarct. Alp. Res. 40, 225–232. doi: 10.1657/1523-0430(06-010)
Weber, P., Bugmann, H., and Rigling, A. (2007). Radial growth responses to drought of Pinus sylvestris and Quercus pubescens in an inner-Alpine dry valley. J. Veg. Sci. 18, 777–792. doi: 10.1111/j.1654-1103.2007.tb02594.x
Wieser, G. (2010). Lessons from the timberline ecotone in the Central Tyrolean Alps: a review. Plant Ecol. Divers. 5, 127–139. doi: 10.1080/17550874.2010.498062
Wieser, G., Oberhuber, W., and Gruber, A. (2019). Effects of climate change at treeline: lessons from space-for-time studies, manipulative experiments, and long-term observational records in the Central Austrian Alps. Forests 10, 1–16. doi: 10.3390/f10060508
Wieser, G., Oberhuber, W., Gruber, A., Leo, M., Matyssek, R., and Grams, T. E. E. (2016). Stable water use efficiency under climate change of three sympatric conifer species at the Alpine treeline. Front. Plant Sci. 7:799. doi: 10.3389/fpls.2016.00799
Wilson, R., and Hopfmueller, M. (2001). Dendrochronological investigations of Norway spruce along an elevational transect in the Bavarian Forest, Germany. Dendrochronologia 19, 67–79.
Winkel, G., Lovrić, M., Muys, B., Katila, P., Lundhede, T., Pecurul, M., et al. (2022). Governing Europe’s forests for multiple ecosystem services: opportunities, challenges, and policy options. Forest Policy Econ. 145:102849. doi: 10.1016/J.FORPOL.2022.102849
Zang, C., and Biondi, F. (2015). Treeclim: an R package for the numerical calibration of proxy-climate relationships. Ecography 38, 431–436. doi: 10.1111/ecog.01335
Keywords: dendroecology, tree-ring analysis, climate change, mountain forests, drought, temperature gradient, precipitation gradient
Citation: Obojes N, Buscarini S, Meurer AK, Tasser E, Oberhuber W, Mayr S and Tappeiner U (2024) Tree growth at the limits: the response of multiple conifers to opposing climatic constraints along an elevational gradient in the Alps. Front. For. Glob. Change. 7:1332941. doi: 10.3389/ffgc.2024.1332941
Edited by:
Marieke van der Maaten-Theunissen, Technical University Dresden, GermanyReviewed by:
Martin Häusser, Friedrich-Alexander-Universität Erlangen-Nürnberg, GermanyIonel Popa, National Institute for research and Development in Forestry Marin Dracea (INCDS), Romania
Copyright © 2024 Obojes, Buscarini, Meurer, Tasser, Oberhuber, Mayr and Tappeiner. This is an open-access article distributed under the terms of the Creative Commons Attribution License (CC BY). The use, distribution or reproduction in other forums is permitted, provided the original author(s) and the copyright owner(s) are credited and that the original publication in this journal is cited, in accordance with accepted academic practice. No use, distribution or reproduction is permitted which does not comply with these terms.
*Correspondence: Nikolaus Obojes, bmlrb2xhdXMub2JvamVzQGV1cmFjLmVkdQ==