- 1Department of Environment, Faculty of Environmental Culture and Ecotourism, Srinakharinwirot University, Bangkok, Thailand
- 2Thailand Institute of Scientific and Technological Research, Pathum Thani, Thailand
- 3The Joint Graduated School of Energy and Environment, King Mongkut's University of Technology Thonburi, Bangkok, Thailand
Soil respiration (Rs) in forest ecosystems is an important process in the global carbon cycle. The unbalanced use of forest natural resources and deforestation in the past have resulted in changes in forest structure, tree growth, and the release of carbon dioxide (CO2) emissions from Rs. Understanding Rs in both primary and secondary forests plays a crucial role in the global carbon cycle. Therefore, the purpose of this study was to estimate and compare Rs in primary dry dipterocarp forests (PDDF) and secondary dry dipterocarp forests (SDDF) in Thailand, in relation to diurnal and seasonal variations in environmental variables (air and soil temperatures, and soil moisture). CO2 flux was measured continuously from March 2019 to February 2020 in the PDDF and SDDF sites in the Nakhon Ratchasima and Ratchaburi provinces of Thailand. Using the soil gradient method, CO2 probes were employed to measure average CO2 concentrations from Rs every minute in the both sites. Additionally, air and soil temperatures and soil moisture were measured continuously to analyze the correlation between Rs and environmental variables. The average annual soil respiration rate in PDDF and SDDF were 8.16 and 8.83 tons C ha−1 yr−1, respectively. The diurnal variation of Rs in both sites changed according to air and soil temperatures. The monthly variation of the average Rs was lower in the PDDF site than in the SDDF site. Soil moisture and soil temperature were significantly correlated with the monthly variation of Rs in both sites. Rs in the PDDF and SDDF sites exhibited high emissions during the wet season, accounting for ~61 and 56% of the total annual emissions, respectively. The results indicated that soil and air temperatures were the main drivers of diurnal variation, while the combination of soil moisture and soil and air temperatures determined the seasonal variations. Additionally, litterfall production was the main carbon substrate promoting soil respiration in the SDDF site, as litterfall production was significantly lower in the PDDF site (5.32 tons dry matter ha−1 yr−1) than in the SDDF site (10.49 tons dry matter ha−1 yr−1).
Introduction
Soil respiration (Rs) is a critical component of the global carbon cycle, as it represents a major pathway for the release of carbon dioxide (CO2) from terrestrial ecosystems to the atmosphere (Bond-Lamberty et al., 2024). It is the second largest carbon flux in forest ecosystems, contributing 50–90% of the total ecosystem respiration (Janssens et al., 2001; Chambers et al., 2004; Hanpattanakit et al., 2015), and also is a key process in the global carbon cycle that supplies nutrients to forest ecosystems (Hanpattanakit, 2014). Rs is a combination of autotrophic root respiration and heterotrophic microbial respiration, consists of microbial activity and the respiration of aboveground and belowground litter (Hanpattanakit et al., 2015). Tropical forest ecosystems, which cover approximately 7% of the Earth's land surface, are responsible for a significant portion of global Rs (Hashimoto et al., 2004) and global carbon cycle by storing 45% of global terrestrial carbon stores in vegetation (IPCC, 2007). Plants serve as carbon sinks by accumulating carbon through photosynthesis. Some organic carbon compounds are used to develop biomass, while others are broken down during respiration to supply plants with energy. During this process, CO2 is released into the atmosphere from ecosystem respiration, deforestation, and animal respiration (Hanpattanakit et al., 2015). Understanding the environmental controls and the spatial and temporal variations of Rs in different forest types is essential for accurately estimating the carbon balance of these important ecosystems and predicting the effects of environmental changes on carbon dynamics (Mavrovic et al., 2023; Shao et al., 2024).
Generally, compared to boreal and temperate forests, tropical forests exhibit higher rates and greater variations in Rs (Raich and Schlesinger, 1992). A study in a temperate coniferous-deciduous forest in Belgium measured the Rs rate of 2.24 kg CO2 m−2 yr−1 (Curiel Yuste et al., 2004). The Rs rate was measured at 4.62 kg CO2 m−2 yr−1 in dry evergreen forest in Chachoengsao province, Thailand (Wiriyatangsakul et al., 2006) and 3.83 kg CO2 m−2 yr−1 in dry dipterocarp forest in Nakhon Ratchasima province, Thailand (Dorji, 2010). By comparing Rs between forest types, previous study of Panuthai et al. (2005) found that the average soil CO2 emission rate was 4.29 kg CO2 m−2 yr−1 in the Sakaerat dry evergreen forest in the Nakhon Ratchasima province of Thailand, compared to 5.07 kg CO2 m−2 yr−1 in the Maeklong mixed deciduous forest in the Kanchanaburi province of Thailand. For the primary and secondary forest, Adachi et al. (2006) found Rs rate of tropical primary and secondary forest ecosystems in Pasoh Forest Reserve on the Malaysian Peninsula were 831 ± 480 and 838 ± 143 mg CO2 m−2 h−1. Jiang et al. (2017) reported that the annual total soil C efflux was significantly higher in the tropical primary mountain rain forest (1,567 ± 205 g C m−2 yr−1) than that in the secondary forest (1,300 ± 70 g C m−2 yr−1, p < 0.05). Compared with the primary forest, the secondary forest has a lower biomass, lower soil C storage, lower litter production, and a simpler community structure (Bréchet et al., 2009; Zhou et al., 2013). This indicates that the primary forest has a higher concentration of substrate and higher microbial activity, which leads to a higher Rs rate (Wang et al., 2003). In contrasts, the studied of Wang (2006) in tropical rainforest in Bukit Timah Nature Reserve, Singapore reported that the Rs rates observed in the secondary forest was higher than that of the primary forest. However, none of the tested factors (soil temperature, soil moisture, diameter of trees at breast height, and canopy cover) were significantly influencing Rs rates. Other factors such as soil organic carbon content and species composition are more important factors influencing spatial variation of Rs between primary and secondary tropical forests (Wang, 2006).
While significant progress has been made in research on Rs in primary and secondary forests few studies have directly compared these two forest types, particularly in the context of tropical dry dipterocarp forests. Which the tropical dry dipterocarp forests are a dominant forest type in mainland Southeast Asia, including Thailand, and are characterized by a pronounced dry season and distinctive tree species composition (Hanpattanakit et al., 2009, 2015). According to the government report, the total coverage area of dry dipterocarp forests in Thailand is estimated at 15,865,260 ha (Royal Forest Department: Ministry of Natural Resources Environment, 2009). The dry dipterocarp forest is the most extensive forest type in Thailand, covering around 13.91% of the total land area of the country (Eiadthong, 2008). These forests play a crucial role in the regional and global carbon cycle, with soil respiration being a significant component of the overall carbon flux (Wangluk et al., 2013). The differences in forest structure, composition, and successional stage can lead to distinct belowground carbon dynamics, potentially resulting in divergent patterns of Rs between primary and secondary forests (Intanil et al., 2018; Raczka et al., 2023).
This study aims to estimate and compare CO2 emissions from Rs in the primary dry dipterocarp forest (PDDF) and secondary dry dipterocarp forest (SDDF) sites in Thailand, in relation to diurnal and seasonal variations in environmental variables (air and soil temperatures, and soil moisture). We anticipate significant diurnal and seasonal variations in environmental variables, which we expect will influence the rate of CO2 emissions from soil respiration (Rs). Additionally, we predict that CO2 emissions will correlate with soil temperature and moisture levels across both forest types. By comparing Rs between primary and secondary dry dipterocarp forests and investigating the influence of environmental factors, we aim to enhance our understanding of the drivers of belowground carbon dynamics in these crucial tropical ecosystems. The findings may also have implications for the management and restoration of the dry dipterocarp forests, which face threats from various anthropogenic disturbances and climate change impact.
Materials and methods
Site description
The primary dry dipterocarp forest (PDDF) in this study was located within the Sakaerat Environmental Research Station, Nakhon Ratchasima province, northeastern Thailand (latitude: 14° 30′ 29.80′' N, longitude: 101° 56′ 58.50′' E, elevation 390 m; Figure 1A). This area is part of the Sakaerat Biosphere Reserve, with a total area of 79.61 km2 and a the PDDF area of 11.80 km2 (1,180 ha), accounting for 14.8% of the total area. The average annual precipitation and air temperature over 4-year period (2013–2016) were 1,260 mm and 20.44°C, respectively (Sakaerat Environmental Research Station, 2018). The average minimum and maximum air temperatures are 21.18 and 30.39°C, respectively. The soil at this site is a sandy loam (Sonkanha et al., 2012). There are approximately 185 tree species and 49 families in this study area. The main tree species are Shorea obtuse, Xylia xylocarpa var. Kerrii, Pterocarpus macrocarpus, Lannea coromandelica, and Quercus kerrii (Kachina, 2018). The main ground cover plant species are Arundinaria pusilia, Arundinaria cililta, and Cycas siamensis Miq. (Sakaerat Environmental Research Station, 2018). The average tree height and circumference were 11.01 m and 39.19 cm, respectively. The tree basal area and density were 16.43 m2 ha−1 and 325 trees ha−1, respectively (Tammadid, 2019).
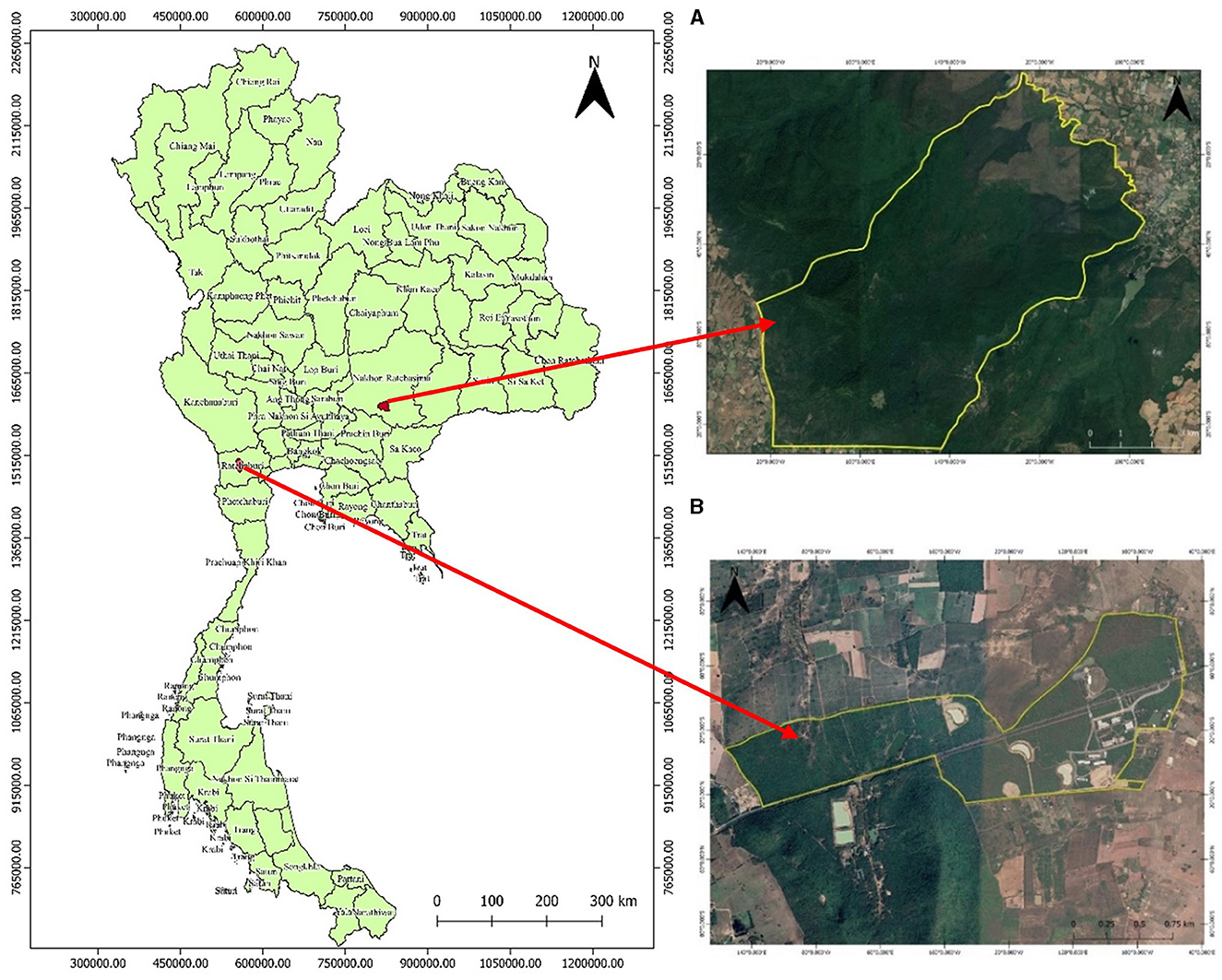
Figure 1. Location of the primary dry dipterocarp forest (PDDF) (A) at Sakaerat Environmental Research Station, Nakhon Ratchasima province and secondary dry dipterocarp forest (SDDF) (B) at King Mongkut's University of Technology Thonburi, Ratchaburi campus, Ratchaburi province in Thailand.
The secondary dry dipterocarp forest (SDDF) was located within King Mongkut's University of Technology Thonburi, Ratchaburi campus, Ratchaburi province, western Thailand (latitude: 13° 35′ 13.3′' N, longitude: 99° 30′ 3.9′' E, elevation 110 m; Figure 1B). The SDDF site has a total area of 187.2 ha, which has been preserved since 2005 after the forest was utilized by surrounding communities for energy (wood and charcoal), and building structures (menagerie and hut). As a result, most of the trees are in the recovery phase (Hanpattanakit et al., 2015). From 2011 to 2015, the average annual precipitation was 1,029 mm and the air temperature was 28.46°C. The average minimum and maximum air temperatures are 22.82 and 34.18°C, respectively (Statistical Office of Ratchaburi Province, 2017). The soil texture for the top 100 cm depth at this site is loamy sand soil, with average sand, silt and clay particle contents of 75.79, 20.86, and 3.35% respectively (Hanpattanakit, 2008). Of the ~77 tree species, the main tree species are Dipterocarpus intricatus, Dipterocarpus obtusifolius, Dipterocarpus tuberculatus, Shorea obtuse, and Shorea siamensis. The main ground cover plant species is Polyalthia debilis (Pierre) Finet and Gagnep (Phianchroen et al., 2008). The average tree height and circumference were 8.11 m and 20.48 cm, respectively. The tree basal area and density were 34.32 m2 ha−1 and 2,538 trees ha−1, respectively (Tammadid, 2019). Most plants regenerated after deforestation.
Instrumentation and CO2 flux calculations
CO2 flux from Rs was measured using a CO2 probe (GMP343: Vaisala Inc., Finland) with the soil gradient method. Two CO2 probes were buried vertically at depths of 5 and 20 cm. The average CO2 concentrations from the Rs were recorded every minute by a data logger (CR1000: Campbell Scientific, Logan, Utah, USA; Figure 2). The soil CO2 concentration from Rs in the site was continuously measured from March 2019 to February 2020. The CO2 concentrations (μmol mol−1 or μmol m−3) with environmental variables (air and soil temperatures and soil moisture) were used to calculate the CO2 flux (μmol m−2 s−1 or mg CO2 m−2 hr−1) via Equations (1–5) (Tang et al., 2003; Maier and Schack-Kirchner, 2014; Bulsathaporn et al., 2018).
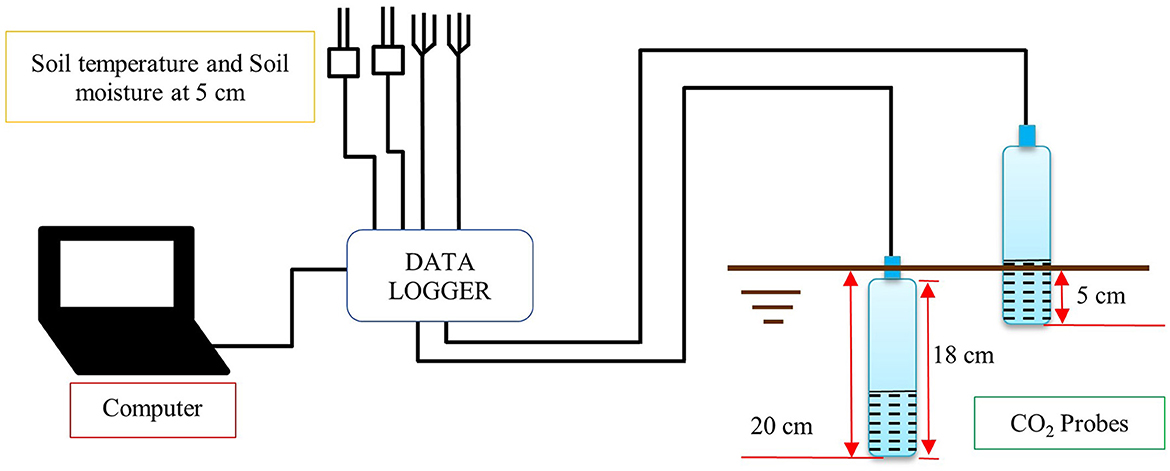
Figure 2. Placement and position of the GMP343 probes in the soil profile for soil CO2 concentration and environmental variables (air and soil temperatures, and soil moisture) measurements.
where F is the soil CO2 flux (mg CO2 m−2 hr−1), Ds is the CO2 diffusion coefficient in the soil (m2 s−1), is the vertical soil CO2 gradient, C is the CO2 concentration (μmol mol−1 or μmol m−3), and Z is the soil depth (m).
where Da is the CO2 diffusion coefficient in free air (m2 s−1) and ξ is the gas tortuosity factor.
where Da0 is the reference value of Da at 20°C (293.15 K) and 101.3 kPa, given as 14.7 × 10−3 m2 s−1, T is the air temperature (K), and P is the air pressure (kPa). There are several empirical models for computing ξ (Tang et al., 2003) as
where α is the volumetric air content and ϕ is the porosity.
where θ is the volumetric soil water content, ρb is the bulk density (g cm−3) and ρm is the particle density of the mineral soil (g cm−3). The bulk density in the PDDF and SDDF sites for calculations were 0.59 and 1.04 g cm−3, respectively. These was measured in January 2019. The particle density in the PDDF site for calculation was 2.73 g cm−3, it measured concurrently with bulk density (Tammadid, 2019). The particle density in the SDDF site was 2.68 g cm−3 reference from Bulsathaporn et al. (2018).
Air and soil temperatures, and soil moisture were measured continuously to analyze the correlation between Rs and environmental variables. Soil temperature and moisture were measured at a depth of 5 cm. Air and soil temperatures were measured using two thermocouple probes per each factor (TCAV, Campbell Scientific, Inc., USA) and soil moisture was measured using two water content reflectometers (CS615, Campbell Scientific, Inc).
Litterfall production
Litterfall production was measured from March 2019 to February 2020 using litter traps. Litter traps consisted of nylon netting and a PVC frame, each 1 m wide x 1 m long, randomly installed 1 m above ground with 12 traps per area in the PDDF and SDDF sites. Litterfall was collected every month and oven-dried at 50°C for 48 h. or until constant weight. The carbon content was calculated using dry biomass multiplied by the carbon content in the biomass analyzed via a CHN analyzer (628 series, LECO Corporation, St Joseph, Michigan, USA).
Soil properties analysis
The soil pH, soil bulk density, soil carbon and nitrogen contents, and soil organic carbon (SOC) were measured. Soil samples were collected from four soil depth levels (0–5, 5–10, 10–15, and 15–20 cm) using a soil core with a 5 cm diameter. The soil pH was analyzed with a pH meter (HI-3220, Hanna Instrument, Inc., USA) with a soil to water ratio of 1:1 (w w−1; Land Development Department, 2004). The soil was oven-dried at 105°C for 48 h and weighed to calculate the bulk density using Equation (6) (Hanpattanakit, 2013). The soil carbon and nitrogen contents were analyzed using a CHN analyzer (628 series, LECO Corporation, St Joseph, Michigan, USA) and the SOC was calculated using Equation (7) (Li et al., 2017).
where SOC is the soil organic carbon (g cm−2), C is the soil carbon content (%), BD is the soil bulk density (g cm−3), and D is the soil depth (cm).
Data analysis
CO2 emissions from Rs, litterfall production, soil properties, air and soil temperatures, and soil moisture in the PDDF and SDDF sites were statistically compared via one-way analysis of variance (ANOVA), facilitating the evaluation of mean differences among the various groups. This statistical approach served to ascertain the presence of significant disparities in CO2 emissions between the season and forest sites. The seasonal variation of Rs in the PDDF and SDDF sites was divided into changes during the dry season (November to April) and the wet season (May to October). In the dry season, the seasonal variation in daily Rs averaged from 136 and 169 days in the PDDF and SDDF sites, respectively. Then in the wet season, the seasonal variation in daily Rs averaged from 170 and 184 days in the PDDF and SDDF sites, respectively. The relationships between diurnal and daily variation in the Rs and, air and soil temperatures, and soil moisture, and the relationships between monthly variation in the Rs and litterfall production, air and soil temperatures, and soil moisture in both sites were determined via Pearson's correlation analysis and simple linear regression analysis (R2-value). In addition, the multiple relationships between daily variation in the Rs with air and soil temperature, and soil moisture were determined via the multiple linear regression analysis. The statistical analyses were conducted Statistical Package for the Social Sciences (SPSS) program version 17.0 for Windows (IBM Crop., Armonk, N.Y. USA), at a confidence level 95 and 99% (p < 0.05 and p < 0.01).
Results
Soil properties, environmental variables and litterfall production
In the PDDF site, the soil was acidic, with a pH range of 4.44–4.73 and an average of 4.54. The soil bulk density ranged from 0.55 to 0.63 g cm−3, with an average of 0.59 g cm−3. The carbon content range about 1.17–1.56%, an average around 1.35% and nitrogen content 0.10–0.13%, an average about 0.11% (Table 1). Soil organic carbon (SOC) ranged 3.70–4.29 tons C ha−1, an average and total organic carbon in the soil were 3.98–15.91 tons C ha−1 at the 4 soil depth levels in the PDDF site (Table 2). The range of the air temperatures was ~20.96–30.40 and soil temperatures range was ~20.86–29.72°C, between March-2019 to February-2020. The average annual air temperatures were 28.48°C. The maximum average was 31.38°C in March-2019 and minimum in December-2019 was 24.03°C. The average annual soil temperatures were 26.08°C. The maximum average was 28.27°C in March-2019 and minimum in December-2019 was 22.82°C. The soil moisture contain range was ~38.48–53.65% water-filled pore space (WFPS) The average annual soil moisture was 43.02% WFPS. The maximum average was 48.62% in September-2019 and minimum in February-2020 was 39.24% WFPS at the PDDF site (Figure 3A). The litterfall production was highest in January 2020 and lowest in December 2019. The total litterfall production averaged 5.32 tons dry matter ha−1 yr−1, the most of which was leaves with an average of 2.98 tons dry matter ha−1 yr−1, followed by other parts and branches with averages of 1.23–1.11 tons dry matter ha−1 yr−1 at the PDDF site (Figure 4).
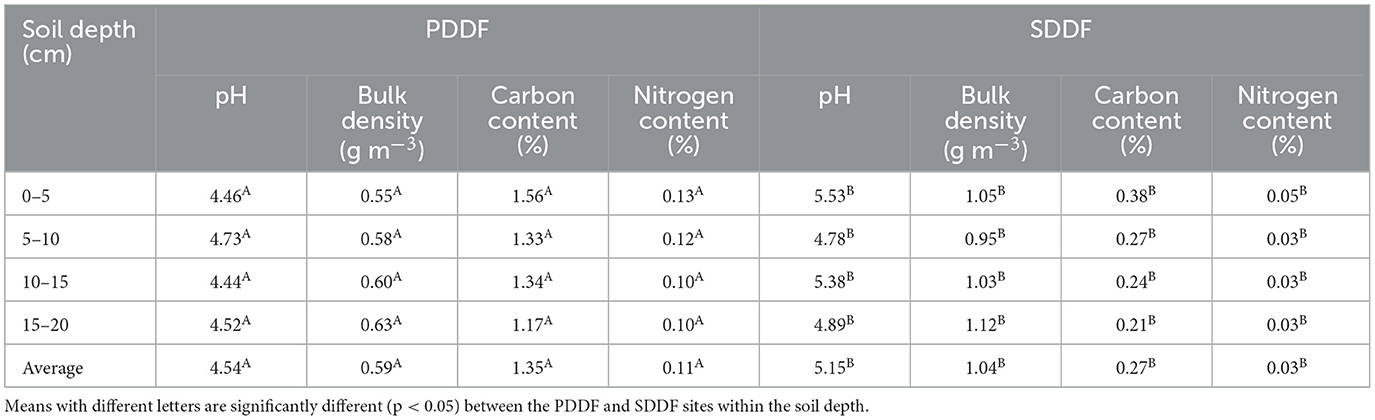
Table 1. Soil properties in primary dry dipterocarp forest (PDDF) and secondary dry dipterocarp forest (SDDF).
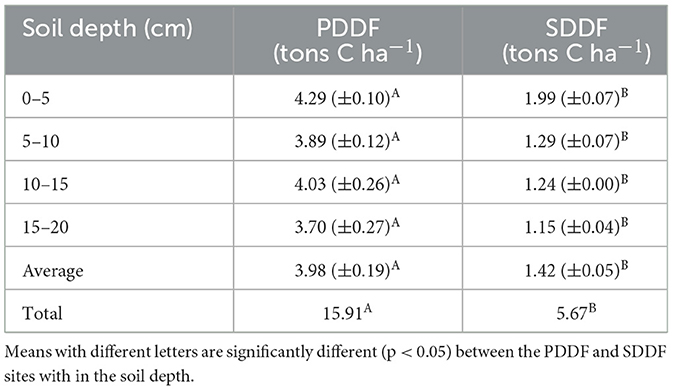
Table 2. Soil organic carbon content in primary dry dipterocarp forest (PDDF) and secondary dry dipterocarp forest (SDDF).
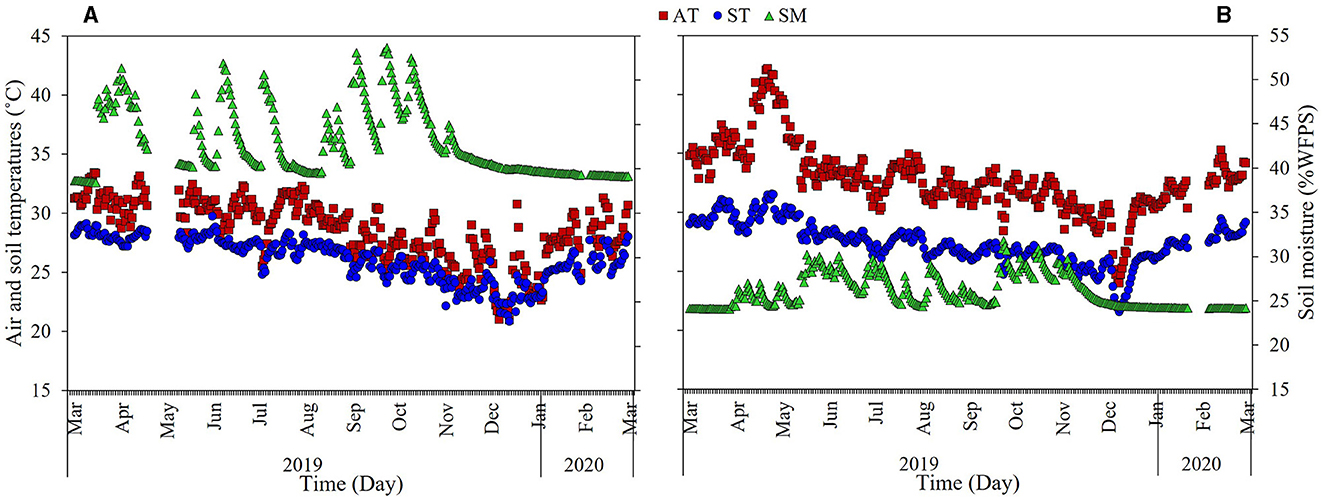
Figure 3. Daily average air (AT) and soil temperatures (ST), and soil moisture (SM) from March 2019–February 2020 in the primary dry dipterocarp forest (PDDF) (A) and secondary dry dipterocarp forest (SDDF) (B).
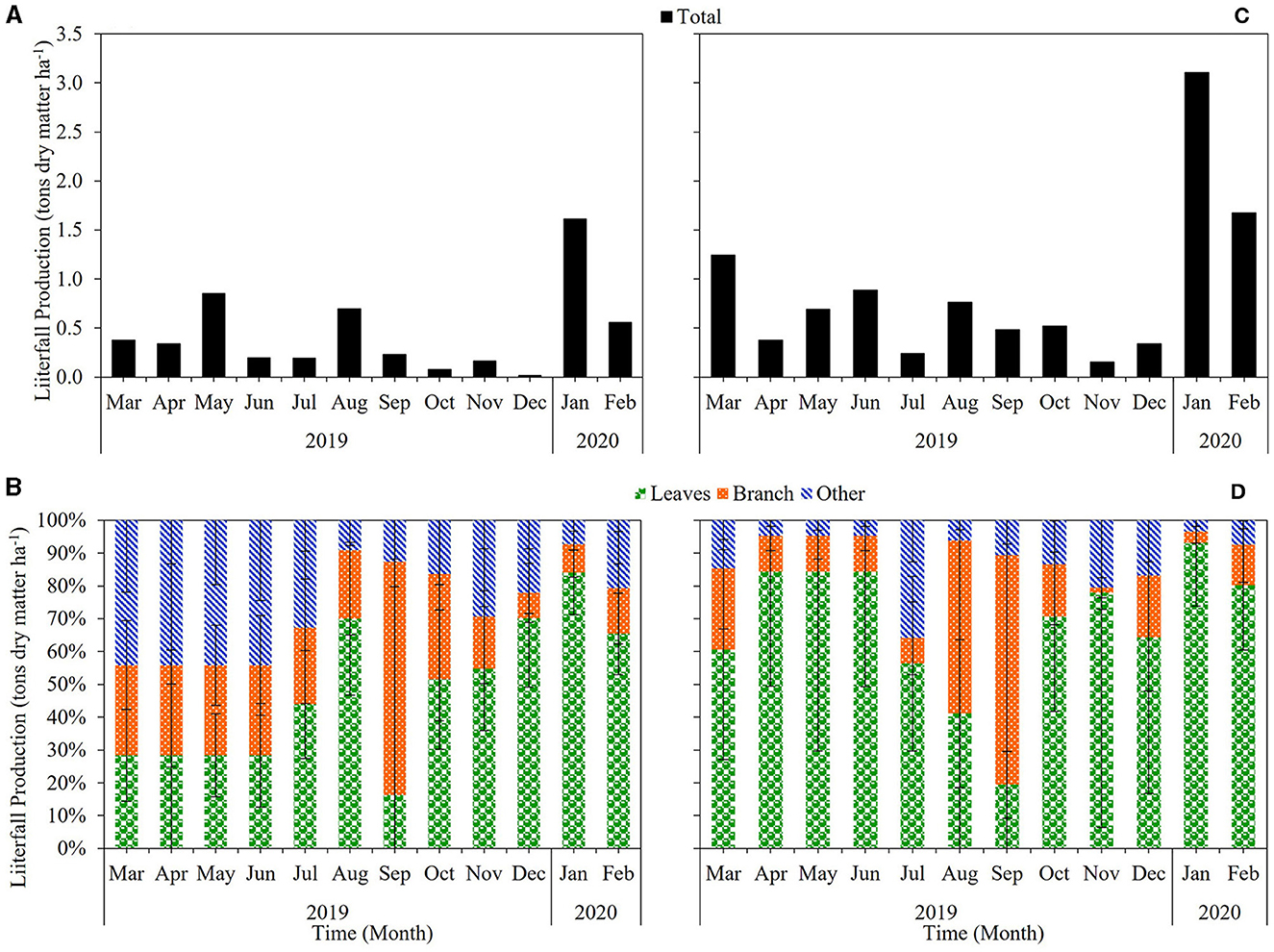
Figure 4. Litterfall production in the primary dry dipterocarp forest (PDDF) (A, B) and secondary dry dipterocarp forest (SDDF) (C, D).
While in the SDDF site, the soil was slightly less acidic, with a pH ranged of 4.78–5.53 and an average of 5.15. Soil bulk density ranged from 0.95 to 1.12 g cm−3, with an average of 1.04 g cm−3. The carbon content range about 0.21–0.38%, an average around 0.27%, and nitrogen content 0.03–0.05%, an average about 0.03% (Table 1). The soil organic carbon (SOC) ranged from 1.15 to 1.99 tons C ha−1, with average and total organic carbon of 1.42–5.67 tons C ha−1 at the 4 soil depth levels (Table 2). The range of the air temperatures was around 24.00–42.17 and the soil temperatures range was about 21.57–31.53°C, between March-2019 to February-2020. The average annual air temperatures were 32.80°C. The monthly maximum average was 38.38°C in April-2019 and minimum in December-2019 was 28.67°C. The average annual soil temperatures were 27.39°C. The monthly maximum average was 29.83°C in April-2019 and minimum in December-2019 was 24.62°C. The soil moisture contain range was ~24.08–31.82% WFPS. The average annual soil moisture was 26.09% WFPS. The monthly maximum average was 28.72% in October-2019 and minimum in March-2019 was 24.16% WFPS at the SDDF site (Figure 3B). The litterfall production was highest in January 2020 and lowest in November 2019. The total litterfall production averaged 10.49 tons dry matter ha−1 yr−1, the most of which was leaves with an average of 7.89 tons dry matter ha−1 yr−1, followed by branches and other parts, with averages of 1.75 and 0.85 tons dry matter ha−1 yr−1 in the SDDF site (Figure 4).
Moreover, the SOC decreased with increasing soil depth and was significantly greater in the PDDF site than in SDDF site (p < 0.05). The air and soil temperatures were significantly lower in the PDDF site than in the SDDF site and soil moisture was significantly greater in the PDDF site than in the SDDF site (p < 0.05). Litterfall production was significantly lower in the PDDF site than in the SDDF site (p < 0.05).
Soil respiration
Diurnal variation
The diurnal variation of the CO2 flux from Rs in both the PDDF and SDDF sites were measured and compared between the dry (March 2019) and wet (May 2019) seasons from an average of 5 consecutive days. The diurnal variation of Rs clearly changed according to the air and soil temperatures, but soil moisture was relatively stable during all days. The diurnal average Rs in the dry season in the PDDF site was 315.57 mg CO2 m−2 hr−1, with a maximum was 350.03 mg CO2 m−2 hr−1 (0.00 hour), and a minimum was 265.49 mg CO2 m−2 hr−1 (23.00 h; Figure 5A). In the wet season, the diurnal average Rs at the PDDF site was 449.04 mg CO2 m−2 hr−1, with a maximum was 471.65 mg CO2 m−2 hr−1 (14.00 h), and a minimum was 422.93 mg CO2 m−2 hr−1 (9.00 h; Figure 5B). At the SDDF site, the diurnal average Rs in the dry season was 217.36 mg CO2 m−2 hr−1, with a maximum was 244.11 mg CO2 m−2 hr−1 (22.00 h), and a minimum was 162.64 mg CO2 m−2 hr−1 (14.00 h; Figure 5C). The diurnal average Rs in the wet season was 475.98 mg CO2 m−2 hr−1, with a maximum was 501.98 mg CO2 m−2 hr−1 (2.00 h), and a minimum was 454.35 mg CO2 m−2 hr−1 (11.00 h; Figure 5D).
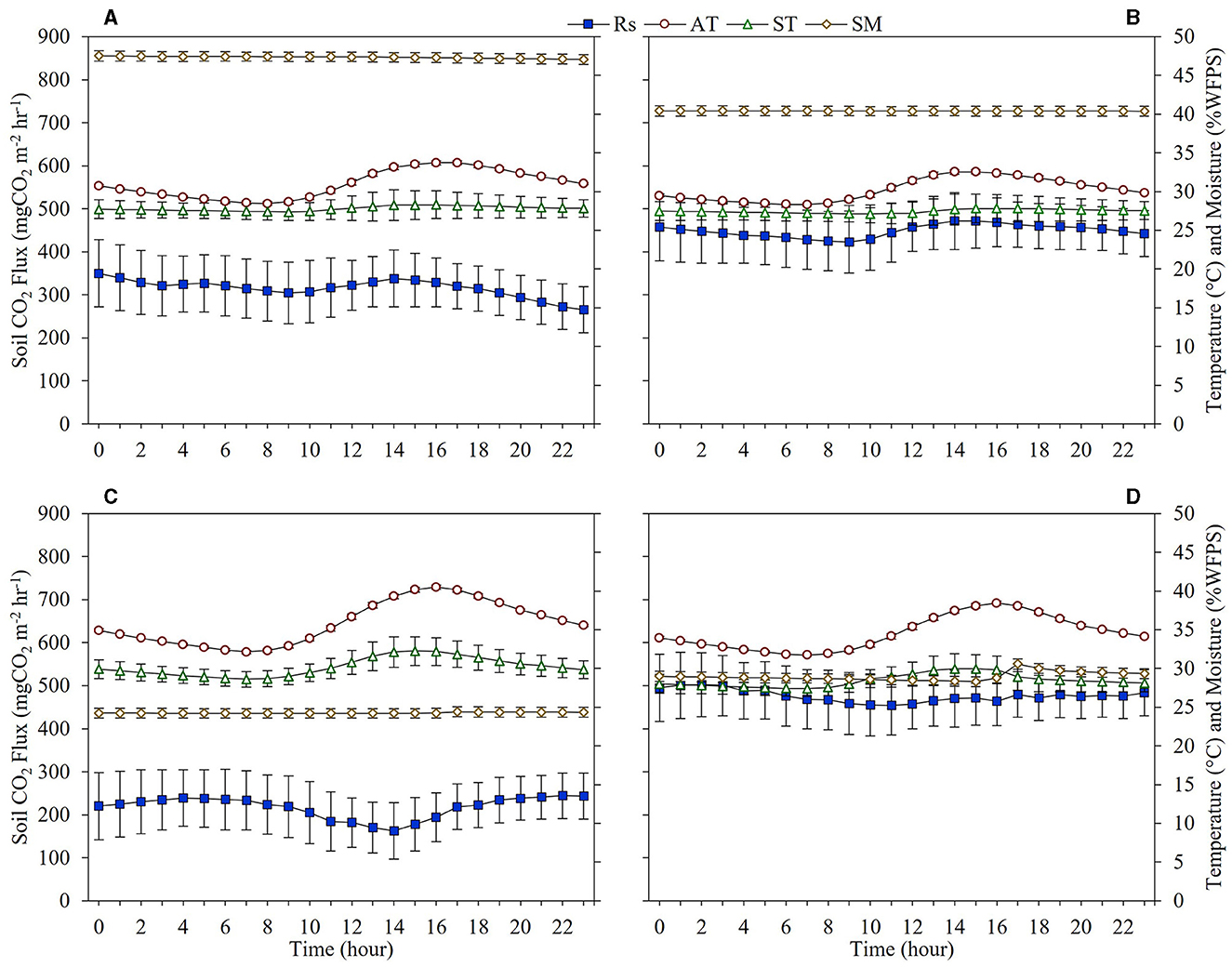
Figure 5. Diurnal variation of Rs with respect to air (AT) and soil temperatures (ST), and soil moisture (SM) in the dry season and wet seasons in the primary dry dipterocarp forest (PDDF) (A, B) and secondary dry dipterocarp forest (SDDF) (C, D).
The diurnal CO2 flux from Rs in the PDDF and SDDF sites was significantly lower in the dry season than in the wet season (p < 0.05). The diurnal variations of Rs in the PDDF site in the dry season exhibited significant positive correlations with air (R2 = 0.6114) and soil temperatures (R2 = 0.6299), and soil moisture (R2 = 0.6239; p < 0.05; Figures 6A–C). In the wet season, Rs was significantly positively correlated with air (R2 = 0.7714) and soil temperatures (R2 = 0.7088; p < 0.05; Figures 6D–F). The diurnal variations of Rs in the SDDF site in the dry season showed a significant negative correlation with air (R2 = 0.7995) and soil temperatures (R2 = 0.8075), and a significant positive correlation with soil moisture (R2 = 0.5651; p < 0.05; Figures 6A–C). In the wet season, Rs was significantly negatively correlated with soil temperature (R2 = 0.6452; p < 0.05; Figures 6D–F).
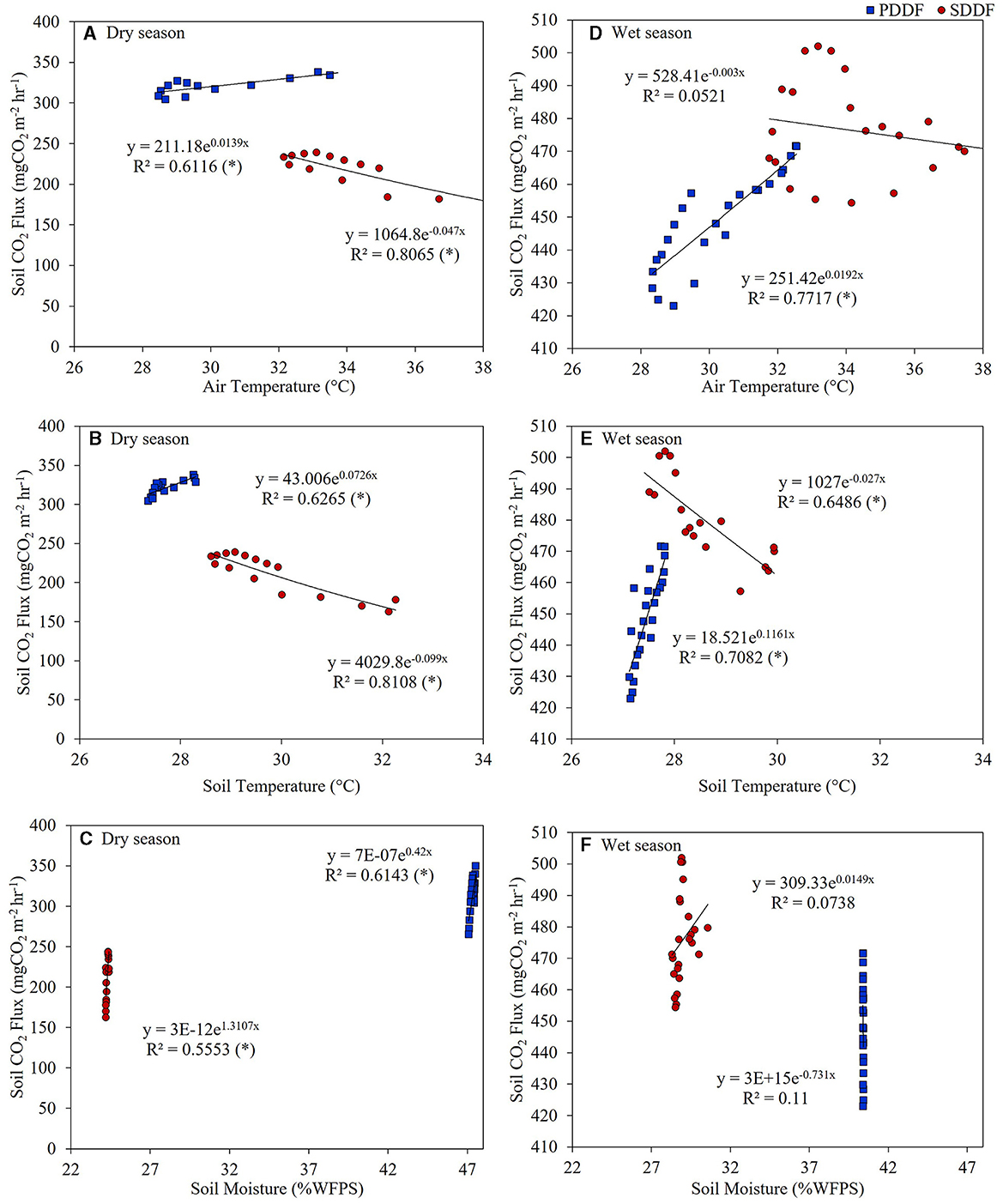
Figure 6. Relationships between diurnal variations of CO2 fluxes from Rs and air (A, D) and soil temperatures (B, E), and soil moisture (C, F) in the dry and wet seasons in the primary dry dipterocarp forest (PDDF) and secondary dry dipterocarp forest (SDDF). Asterisks (*) indicate a significant correlation (p < 0.05) between the soil CO2 flux and environmental factors.
Seasonal variation
The seasonal variation of CO2 flux from Rs changed according to soil moisture. Rs gradually increased from the beginning of the wet season to the end of the wet season and gradually decreased from the end of the wet season to the dry season. The daily average Rs in the dry and wet seasons at the PDDF site were 309.39 and 391.92 mg CO2 m−2 hr−1, respectively, accounting for 39% of the total Rs in the dry season and 61% of the total Rs in the wet season. At the SDDF site, the daily average Rs in the dry and wet seasons were 338.84 and 402.92 mg CO2 m−2 hr−1, respectively, accounting for 44% of the total Rs in the dry season and 56% of the total Rs in the wet season (Figure 7). The monthly average Rs in the dry and wet seasons at the PDDF site were 307.26 and 389.20 mg CO2 m−2 hr−1, respectively, while at the SDDF site, they were 337.73 and 402.91 mg CO2 m−2 hr−1, respectively. Rs was significantly lower in the dry season than in the wet season at both the PDDF and SDDF sites (p < 0.05). Additionally, Rs in the dry season was significantly lower at the PDDF site compared to the SDDF site (p < 0.05).
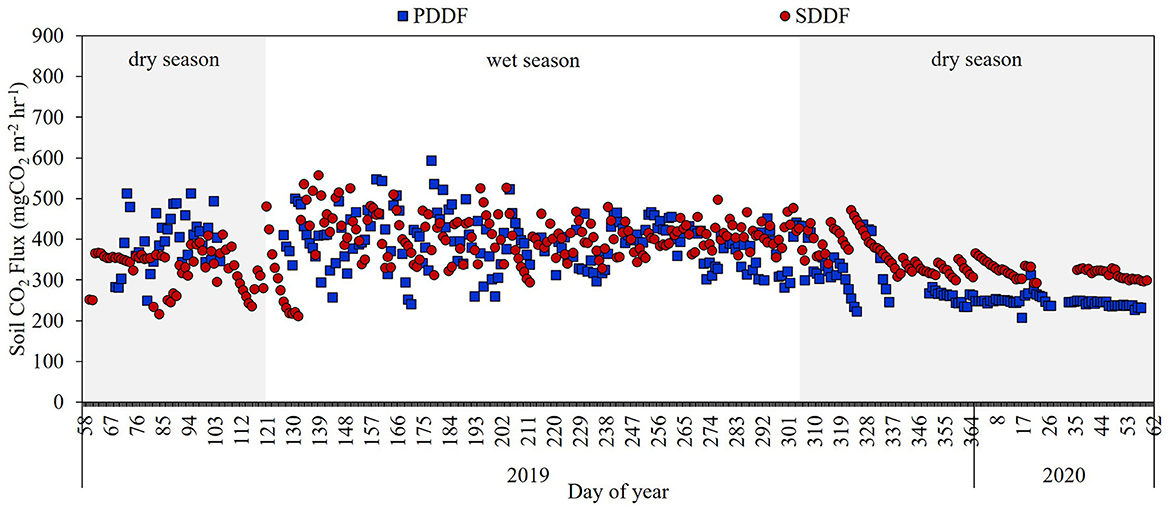
Figure 7. Average daily of CO2 flux from Rs in the primary dry dipterocarp forest (PDDF) and secondary dry dipterocarp forest (SDDF) during March 2019–February 2020.
The correlation between the seasonal variation of Rs and three factors—air temperature (AT), soil temperature (ST), and soil moisture (SM)—at the PDDF and SDDF sites was analyzed using multiple linear regression analysis. The results found that Rs in the dry season at both the PDDF and SDDF sites was significantly positively correlated with soil moisture and soil temperature, and significantly negatively correlated with air temperature (R2 = 0.50 and 0.53; p < 0.01). The multiple linear regression equations were as follows: Rs in the dry season (PDDF) = −1.3(AT) + 5.66(ST) + 16.14(SM) – 463.98, and Rs in the dry season (SDDF) = −4.94(AT) + 3(ST) + 19.49(SM) – 66.44, respectively. In the wet season at the PDDF site, Rs was significantly positively correlated with soil moisture and soil temperature (R2 = 0.50; p < 0.01), with the following equation: Rs in the wet season (PDDF) = 3.88(ST) + 16.18(SM) – 458.44. At the SDDF site in the wet season, Rs was significantly positively correlated with soil moisture, and significantly negatively correlated with air temperature and soil temperature (R2 = 0.27; p < 0.01), with the following equation: Rs in the wet season (SDDF) = −0.18(AT) – 13.26(ST) + 13.91(SM) – 393.90 (Table 3).
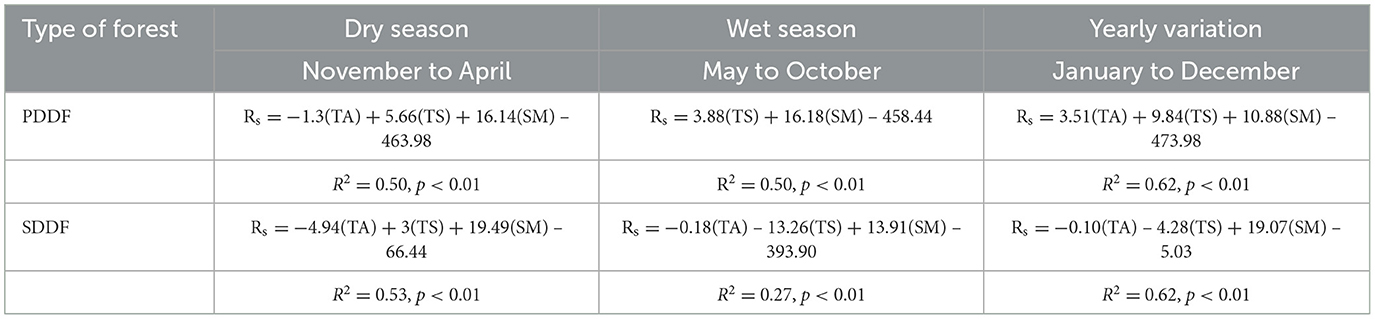
Table 3. The multiple regression in seasonal variation in primary dry dipterocarp forest (PDDF) and secondary dry dipterocarp forest (SDDF).
The daily variation of Rs at the PDDF and SDDF sites ranged from 207.87 to 593.12 mg CO2 m−2 hr−1 and 211.63 to 557.05 mg CO2 m−2 hr−1, respectively. The monthly average Rs at the PDDF site was 348.23 mg CO2 m−2 hr−1, with a monthly maximum in September (422.50 mg CO2 m−2 hr−1) and a minimum in February (241.36 mg CO2 m−2 hr−1). The monthly variations of Rs at the PDDF and SDDF sites were significantly positively correlated with soil moisture (R2 = 0.62 and 0.84, respectively) and significantly negatively correlated with litterfall production (R2 = −0.59 and −0.61, respectively; Table 4). These results indicate that soil moisture and litterfall production drive CO2 emissions in forest ecosystems and are the main factors influencing Rs in terms of the monthly variation of CO2 flux at both the PDDF and SDDF sites. However, the yearly variation (January to December) of Rs at the PDDF site was significantly positively correlated with air temperature, soil temperature, and soil moisture (R2 = 0.62; p < 0.01), as follows: Yearly Rs (PDDF) = 3.51(AT) + 9.84(ST) + 10.88(SM) – 473.98. At the SDDF site, the yearly variation of Rs was positively correlated with soil moisture and soil temperature, and significantly negatively correlated with air temperature (R2 = 0.62; p < 0.01), as follows: Yearly Rs (SDDF) = −0.10(AT) – 4.28(ST) + 19.07(SM) – 5.03 (Table 3). These results indicate that air temperature, soil temperature, and soil moisture were the drivers of Rs at the PDDF and SDDF sites, with soil moisture having a particularly strong effect and being the main factor influencing Rs in terms of monthly, seasonal, and yearly variation of CO2 flux at both sites.
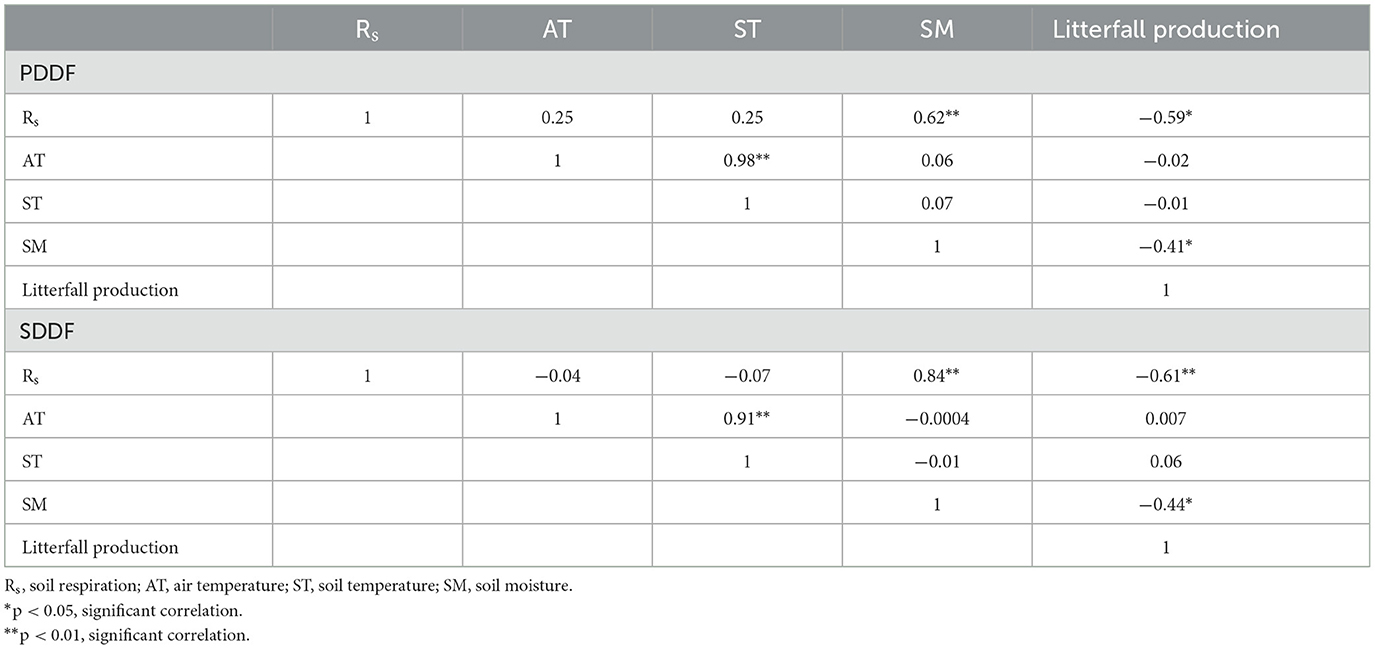
Table 4. Pearson's correlation matrix between the monthly average of Rs, litterfall production, air and soil temperatures, and soil moisture in primary dry dipterocarp forest (PDDF) and secondary dry dipterocarp forest (SDDF).
Moreover, the CO2 flux from Rs was lower at the PDDF site than at the SDDF site. In particular, the diurnal and daily CO2 fluxes from Rs at the PDDF site were significantly lower than those at the SDDF site (p < 0.05). The differences in Rs between the sites were driven by variations in air and soil temperatures, soil moisture, and litterfall production. Air and soil temperatures were significantly lower at the PDDF site than at the SDDF site, while soil moisture was significantly higher at the PDDF site than at the SDDF site (p < 0.05). Additionally, litterfall production was significantly lower at the PDDF site than at the SDDF site (p < 0.05). As a result, the cumulative annual CO2 flux from Rs at the PDDF site (2.99 kg CO2 m−2 yr−1 or 8.16 tons C ha−1 yr−1) was lower than at the SDDF site (3.24 kg CO2 m−2 yr−1 or 8.83 tons C ha−1 yr−1).
Discussion
The soil temperature is directly proportional to elevation and inversely proportional to precipitation and moisture (Zhang et al., 2022). The soil temperature decreases with increasing latitude (Edith and Charles, 1931). The average rate at which air temperature changes with increasing altitude or a rising parcel of air (environmental lapse rate) is a decreased of by ~6.5°C km−1, but this rate varies in different regions, airstreams, and seasons (Storm, 2008). Air and soil temperatures were lower in the PDDF site compared to the SDDF site, and soil moisture was greater in the PDDF site than in the SDDF site, due to differences in latitude, elevation, and precipitation. The low air and soil temperatures and high soil moisture in the wet season stimulate the activity of microbes and plant roots in the soil to increase CO2 flux from Rs (Broken et al., 1999). The diurnal CO2 flux from Rs was significantly lower in the dry season than in the wet season due to greater air and soil temperatures and lower soil moisture in the dry season (Medina and Zelwer, 1972). The diurnal pattern of Rs consisted of a gradual increase in the morning and a gradual decrease at night (after midnight; Intanil, 2017). The CO2 flux from Rs is greater in the wet season than in the dry season because the rapid increase in soil temperature in the wet season promotes root and microbial activities (Hanpattanakit et al., 2015; Hanpattanakita et al., 2021).
Soils in tropical forests are generally acidic (Suwanprapa, 2014). Topsoil (0–5 cm) has the lowest bulk density, which increases with depth due to the accumulation of organic matter from litterfall, minerals, and organic carbon in the topsoil (Kookkhunthod et al., 2018). Subsoil has less organic matter, is more compacted, and has smaller pores, which increases the bulk density of the subsoil (Wongtangprasert, 2015). SOC was significantly greater in the PDDF site than in the SDDF site (p < 0.05). The soil structure and SOC can be changed by disturbance (e.g., land-use change, and deforestation), which could help explain the difference between the PDDF and SDDF sites (Wigginton et al., 2000). The PDDF site experienced less disturbance and land-use change than in the SDDF site, which would affect forest structure, resulting in SOC and nutrients that would remain stable or change slowly.
Litterfall production was positively correlated with soil temperature and negatively correlated with soil moisture (Hanpattanakit and Chidthaisong, 2012). Litterfall production was lower in the PDDF site compared to the SDDF site, due to the lower density of trees in the PDDF site than in the SDDF site (Wongtangprasert, 2015; Kookkhunthod et al., 2018; Zhang et al., 2022). The lower soil temperature and higher soil moisture in the PDDF site is resulted in lower litterfall production in the PDDF site than in the SDDF site (Hanpattanakit and Chidthaisong, 2012). Rs was determined by litterfall production in terms of the monthly variation in both the PDDF and SDDF sites. Rs was negatively related to litterfall production at both sites (p < 0.05; Table 4). Normally, foliage and litterfall in dry dipterocarp forest were concentrated during the cool and dry period (January–March). The foliage fall on the surface implies a decrease in aboveground respiration. The litterfall production is the substrate available for microbial activity in soil respiration processes (Hanpattanakit and Chidthaisong, 2012; Hanpattanakit et al., 2012).
The diurnal CO2 flux from Rs was determined by air and soil temperatures. Low air and soil temperatures and high soil moisture in the wet season stimulate the activity of microbes and plant roots in the soil to increase CO2 flux from Rs (Broken et al., 1999). Rs rate are greater at night than during the day in arid ecosystems due to increased relative humidity and decreased soil temperature at night (Medina and Zelwer, 1972). The diurnal variation of Rs gradually increased from morning to afternoon and gradually decreased from evening to night. These results are consistent with the results of Intanil (2017), who reported that the diurnal pattern of Rs consisted of a gradual increase in the morning and a gradual decrease at night (after midnight). According to Hanpattanakit et al. (2015) the CO2 flux from Rs is greater in the wet season than in the dry season because the rapid increase in soil temperature in the wet season promotes root and microbial activities.
Seasonal patterns of Rs are largely determined by soil water availability (Davidson et al., 2000; Hanpattanakit et al., 2017). In tropical climate regimes with cold, wet winters and hot, dry summers, water usually constrains biological activity in the summer (Xu and Qi, 2001). Rs in both sites was limited during the dry season. Rs was positively correlated with soil moisture in the PDDF and SDDF sites (Figure 6C, Tables 3, 4). Soil moisture was the main factor limiting Rs in terms of daily and monthly variations of CO2 flux in both the PDDF and SDDF sites. The relationship between soil respiration and temperature is well-established, and understanding how temperature affects soil respiration is necessary for predicting soil responses to changes in climate. Air temperature and seasonal and yearly variations of Rs were negatively correlated and soil temperature was negatively correlated with seasonal and yearly variations of Rs (Tables 3, 4). Shown that, the Rs variation was concurrently influenced by air and soil temperatures, soil moisture. These similarly to the studies of Bowden et al. (1998), Fernandez et al. (1993), Hanpattanakit (2013), and Yi et al. (2007) that reported the multiple correlation between Rs and temperature and soil moisture. Air and soil temperatures were significantly lower in the PDDF site than in the SDDF site (p < 0.05) due to differences in topography, latitude, elevation, and forest characteristics between sites (Figures 3A, B). At both sites, a linear relationship was observed in which the lowest Rs coincided with the highest air and soil temperatures. However, a positive relationship was observed only when the soil temperature was < 27°C and the air temperature was < 30°C. Rs was positively correlated with low air and soil temperatures in the PDDF site and negatively correlated with high air and soil temperatures in the SDDF site.
The CO2 flux from Rs was significantly lower in the PDDF site than in the SDDF site (p < 0.05), which can be attributed to differences in biotic and abiotic factors such as climate, soil properties, litterfall production, and forest structure. Air and soil temperatures were significantly lower in the PDDF site than in the SDDF site (p < 0.05). CO2 fluxes from Rs and air and soil temperatures were positively correlated in the PDDF site and negatively correlated in the SDDF site. Rs increases linearly when air and soil temperatures are relatively low, but extreme air and soil temperatures effectively stop Rs (Intanil, 2017). In a previous study of dry dipterocarp forest, the air temperature ranged from 22.50 to 41.25°C and the soil temperature ranged from 23.75 to 33.75°C (Hanpattanakit et al., 2009). Rs was negatively correlated with soil temperature and the lowest Rs coincided with the highest soil temperature. If there had been data associated with a lower range in soil temperature, an increase in soil respiration for certain soil temperature ranges (i.e., up to 25°C) would have been observed, as suggested by Flanagan and Veum (1974). A positive relationship exists for much lower temperature ranges, such as those not found in a tropical climate. In the hilly region of Mount Taihang, Rs was positively correlated with soil temperature, which ranged from −4.98 to 26.23°C (Zeng et al., 2014).
The PDDF site had a greater soil moisture content and lower soil temperature than in the SDDF site, and was undisturbed by human activity, with a more stable forest structure and mineral and nutrient contents. The basal area and density of trees were lower in the PDDF site than in the SDDF site since the trees in the SDDF site were in the recovery phase and still growing. Rs rates are strongly correlated with elevation, temperature, precipitation, and litterfall production (Huang et al., 2017). Therefore, higher Rs rates occur at lower elevations because of increased temperature, litterfall production, and active plant growth rates and decreased precipitation (< 1,700 mm), which contribute to lower soil moisture (Kane et al., 2003; Litton et al., 2011; Berryman et al., 2014). Soil pH and soil bulk density were significantly lower in the PDDF site than in the SDDF site (p < 0.05) and soil carbon and nitrogen contents and SOC were significantly greater in the PDDF site than in the SDDF site (p < 0.05). Liu et al. (2018) reported that Rs was positively correlated with soil pH. The CO2 flux from Rs was 2–12 times less in soil with a pH of 3 than in soil with a pH of 4. Soil with a pH above 7 also release less CO2 flux from Rs (Kowalenko et al., 1978). Soil pH affects the activities of soil microbes, the main drivers of Rs. Zeng et al. (2014) reported that Rs was negatively correlated with SOC and soil nitrogen content, indicating decreased soil respiration due to increased SOC and nitrogen content. In addition, litterfall production was significantly lower in the PDDF site than in the SDDF site. Greater litterfall production tended to enhance Rs (Huang et al., 2017). According to Raich (1998), Rs increased with an increasing rate of litterfall production. Aboveground litterfall production regulates energy flow and nutrient cycling in forest ecosystems (Attiwill and Adam, 1993). Changes in aboveground litterfall production inputs can potentially affect soil respiration through the direct decomposition of litterfall and indirect effects on biological processes in the underlying soil (Bowden et al., 1993; Crow et al., 2009; Huang and Spohn, 2015).
Conclusion
Rs experienced diurnal variations corresponding to air and soil temperatures while soil moisture and litterfall production determined the seasonal variations. The seasonal variations of Rs in the primary dry dipterocarp forest (PDDF) site and secondary dry dipterocarp forest (SDDF) site were significantly positively correlated with soil moisture, and significantly negatively correlated with litterfall production. Rs produced higher CO2 emissions during the wet season at both sites. The cumulative CO2 flux from Rs was 2.99 kg CO2 m−2 yr−1 (8.16 tons C ha−1 yr−1) in the PDDF site and 3.24 kg CO2 m−2 yr−1 (8.83 tons C ha−1 yr−1) in the SDDF site. Rs was significantly lower in the PDDF site than in the SDDF site because Rs respond differently to environmental factors, such as climate, soil properties, litterfall production, and forest structure.
The research results estimating and comparing CO2 emissions from soil respiration (Rs) in primary dry dipterocarp forests (PDDF) and secondary dry dipterocarp forests (SDDF) can serve as valuable knowledge and baseline information on CO2 release from soil respiration in tropical forests. This information can help link forest carbon release data with other data from Asia. For future research, it is suggested that data collection be extended over the long term to provide clearer information on changes in carbon emissions in forest ecosystems under climate change conditions. This approach will allow for a more comprehensive understanding of the dynamics of carbon emissions and their implications for forest management and conservation.
Data availability statement
The original contributions presented in the study are included in the article/supplementary material, further inquiries can be directed to the corresponding author.
Author contributions
WT: Data curation, Formal analysis, Investigation, Methodology, Project administration, Writing – original draft. BS: Data curation, Methodology, Formal analysis, Writing – original draft. PC: Conceptualization, Formal analysis, Funding acquisition, Resources, Supervision, Writing – review & editing. AC: Conceptualization, Formal analysis, Funding acquisition, Resources, Supervision, Writing – review & editing. PH: Conceptualization, Data curation, Formal analysis, Funding acquisition, Investigation, Methodology, Project administration, Supervision, Validation, Visualization, Writing – review & editing.
Funding
The author(s) declare financial support was received for the research, authorship, and/or publication of this article. This research was successful with support funding from Thailand Institute of Scientific and Technological Research, Faculty of Environmental Culture and Ecotourism, Srinakharinwirot University (566/2564), International foundation for Science (IFS), and National Research Council of Thailand (NRCT)/N10A650841.
Acknowledgments
The authors would like to thank Sakaerat Environmental Research Station at Nakhon Ratchasima province and King Mongkut's University of Technology Thonburi at Ratchaburi province for providing access to the study plots in the PDDF and SDDF sites and support equipment for analyzing carbon dioxide emission from soil respiration of Joint Graduate School of Energy and Environment, King Mongkut's University of Technology Thonburi.
Conflict of interest
The authors declare that the research was conducted in the absence of any commercial or financial relationships that could be construed as a potential conflict of interest.
Publisher's note
All claims expressed in this article are solely those of the authors and do not necessarily represent those of their affiliated organizations, or those of the publisher, the editors and the reviewers. Any product that may be evaluated in this article, or claim that may be made by its manufacturer, is not guaranteed or endorsed by the publisher.
References
Adachi, M., Bekku, Y. S., Rashidah, W., Okuda, T., and Koizumi, H. (2006). Differences in soil respiration between different tropical ecosystems. Appl. Soil Ecol. 34, 258–265. doi: 10.1016/j.apsoil.2006.01.006
Attiwill, P. M., and Adam, M. A. (1993). Nutrient cycling in forests. New Phytol. 124, 561–582. doi: 10.1111/j.1469-8137.1993.tb03847.x
Berryman, E. M., Marshall, J. D., and Kavanagh, K. (2014). Decoupling litter respiration from whole-soil respiration along an elevation gradient in a Rocky Mountain mixed-conifer forest. Can. J. For. Res. 44, 432–440. doi: 10.1139/cjfr-2013-0334
Bond-Lamberty, B., Ballantyne, A., Berryman, E., Fluet-Chouinard, E., Jian, J. A., et al. (2024). Twenty years of progress, challenges, and opportunities in measuring and understanding soil respiration. J. Geophys. Res. 129:e2023JG007637. doi: 10.1029/2023JG007637
Bowden, R. D., Nadelhoffer, K. J., Boone, R. D., Melillo, J. M., and Garrison, J. B. (1993). Contributions of aboveground litter, belowground litter, and root respiration to total soil respiration in a temperate mixed hardwood forest. Can. J. For. Res. 23, 1402–1407. doi: 10.1139/x93-177
Bowden, R. D., Newkirk, K. M., and Rullo, G. M. (1998). Carbon dioxide and methane fluxes by a forest soil under laboratory-controlled moisture and temperature conditions. Soil Biol. Biochem. 30, 1591–1597. doi: 10.1016/S0038-0717(97)00228-9
Bréchet, L., Ponton, S., Roy, J., Freycon, V., Coûteaux, M.-M., Bonal, D., et al. (2009). Do tree species characteristics influence soil respiration in tropical forests? A test based on 16 tree species planted in monospecific plots. Plant Soil 319, 235–246. doi: 10.1007/s11104-008-9866-z
Broken, W., Xu, Y. J., Brumme, R., and Lamersdorf, N. (1999). A climate change scenario for carbon dioxide and dissolved organic carbon fluxes from a temperate forest soil. Soil Sci. Soc. Am. 63, 1848–1855. doi: 10.2136/sssaj1999.6361848x
Bulsathaporn, A., Suekhum, D., Hanpattanakit, P., and Sanwangsri, M. (2018). Soil CO2 emissions measured by closed chamber and soil gradient methods in dry dipterocarp forest and sweet sorghum plots. Sci. Asia 44, 1–10. doi: 10.2306/scienceasia1513-1874.2018.44.001
Chambers, J. Q., Tribuzy, E. S., Toledo, L. C., Crispim, E. F., Higuchi, N., and Dos Santos, J. (2004). Respiration from a tropical forest ecosystem: partitioning of sources and low carbon use efficiency. Ecol. Applicat. 14, S72–S88. doi: 10.1890/01-6012
Crow, S. E., Lajtha, K., Bowden, R. D., Yana, Y., Brant, J. B., Caldwell, B. A., et al. (2009). Increased coniferous needle inputs accelerate decomposition of soil carbon in an old-growth forest. For. Ecol. Manag. 258, 2224–2232. doi: 10.1016/j.foreco.2009.01.014
Curiel Yuste, J., Nagy, M., Janssens, I. A., and Carrara, A. (2004). Soil respiration in a mixed temperate forest and its contribution to total ecosystem respiration. Tree Physiol. 25, 609–619. doi: 10.1093/treephys/25.5.609
Davidson, E. A., Verchot, L. V., Cattânio, J. H., Ackerman, I. L., and Carvalho, J. E. M. (2000). Effects of soil water content on soil respiration in forests and cattle pastures of eastern Amazonia. Biogeochemistry 48, 53–69. doi: 10.1023/A:1006204113917
Dorji, K. (2010). The Effect of Soil Water Content and Temperature on Tropical Soil Respiration (Dissertation M. Sc.). Environmental Biology, School of Biology, Institute of Science, Suranaree University of Technology, Nakhon Ratchasima, Thailand.
Edith, M. F., and Charles, F. B. (1931). Soil temperatures in the United States. Monthly Weather Rev. 59, 6–16. doi: 10.1175/1520-0493(1931)59<6:STITUS>2.0.CO;2
Eiadthong, W. (2008). Endemic and Rare Plants in Dry Deciduous Dipterocarp Forest in Thailand. The FORTROP II: Tropical Forestry Change in a Changing World. Bangkok: Kasetsart University.
Fernandez, I. J., Son, Y., Kraske, C. R., Rustad, L. E., and David, M. B. (1993). Soil carbon dioxide characteristics under different forest types and after harvest. Soil Sci. Soc. Am. J. 57, 1115–1121. doi: 10.2136/sssaj1993.03615995005700040039x
Flanagan, P. W., and Veum, A. K. (1974). “Relationships between respiration, weight loss, temperature and moisture in organic residues on tundra,” in Proceedings of the Microbiology, Decomposition and Invertebrate Working Groups Meeting, August, 1973. Alaska: United States.
Hanpattanakit, P. (2008). In situ Measurement of CO2 Emission From Root and Soil Repiration in Dry Dipterocarp Forest (Dissertation M.Sc.). Environmental Technology, The Joint Graduate School of Energy and Enviromental at King Mongkut's University of Technology Thonburi, Bangkok, Thailand.
Hanpattanakit, P. (2013). Temporal Variations of Soil Respiration in a Dry Dipterocarp Forest (Dissertation Ph.D.). Environmental Technology, The Joint Graduate School of Energy and Environment at King Mongkut's University of Technology Thonburi, Bangkok, Thailand.
Hanpattanakit, P. (2014). The review of litterfall production and decomposition method in carbon cycle and effect to CO2 emission in tropical forest. Srinakharinwirot Univ. 6, 134–146.
Hanpattanakit, P., and Chidthaisong, A. (2012). Litter production and decomposition in dry dipterocarp forest and their responses to climatic factors. GMSARN Int. J. 6, 169–174.
Hanpattanakit, P., Leclerc, M. Y., Mcmillan, A. M. S., Limtong, P., Maeght, J.-L., Panuthai, S., et al. (2015). Multiple timescale variations and controls of soil respiration in a tropical dry dipterocarp forest, Western Thailand. Plant Soil 390, 167–181. doi: 10.1007/s11104-015-2386-8
Hanpattanakit, P., Panuthai, S., and Chidthaisong, A. (2009). Temperature and moisture controls of soil respiration in a dry dipterocarp forest Ratchaburi Province. Kasetsart J. Nat. Sci. 43, 650–661.
Hanpattanakit, P., Sanwangsri, M., and Chidthaisong, A. (2012). “Relationships among soil properties, CO2 emission and ecosystem respiration in dry dipterocarp forest, Western Thailand,” in The 2012 World Congress on Advances in Civil, Environmental, and Materials Research (ACEM' 12), August 26-30. Seoul.
Hanpattanakit, P., Wattanahemmakorn, J., Sudjarit, T., Jaiarree, S., and Taweekij, S. (2017). Soil respiration in rubber tree plantation applied with biochar. Res. J. Chem. Environ. 21, 27–34.
Hanpattanakita, P., Vanitchunga, S., Saeng-Ngamb, S., and Pearaksab, P. (2021). Effect of biochar on red chili growth and production in heavy acid soil. Chem. Eng. Transact. 83, 283–288. doi: 10.3303/CET2183048
Hashimoto, S., Tanaka, N., Suzuki, M., Inoue, A., Takizawa, H., Kosaka, I., et al. (2004). Soil respiration and soil CO2 concentration in a tropical forest, Thailand. J. For. Res. 9, 75–79. doi: 10.1007/s10310-003-0046-y
Huang, W., and Spohn, M. (2015). Effects of long-term litter manipulation on soil carbon, nitrogen, and phosphorus in a temperate deciduous forest. Soil Biol. Biochem. 83, 12–18. doi: 10.1016/j.soilbio.2015.01.011
Huang, Y. H., Hung, C. Y., Lin, I. R., Kume, T., Menyailo, O. V., and Cheng, C. H. (2017). Soil respiration patterns and rates at three Taiwanese forest plantations: dependence on elevation, temperature, precipitation, and litterfall. Botan. Stud. 58, 1–12. doi: 10.1186/s40529-017-0205-7
Intanil, P. (2017). Estimation of Soil Respiration in Dry Dipterocarp Forest, Northern Thailand (Dissertation M.Sc.). Environmental Science, University of Phayao, Mae Ka, Thailand.
Intanil, P., Boonpoke, A., Sanwangsri, M., and Hanpattanakit, P. (2018). Contribution of root respiration to soil respiration during rainy season in dry dipterocarp forest, Northern Thailand. Appl. Environ. Res. 40, 19–27. doi: 10.35762/AER.2018.40.3.3
IPCC (2007). Climate Change 2007: The Physical Science Basis, Contribution of Working Group I to the Fourth Assessment Report of the Intergovernmental Panel on Climate Change. Cambridge; New York, NY: IPCC.
Janssens, I. A., Kowalski, A. S., and Ceulemans, R. (2001). Forest floor CO2 fluxes estimated by eddy covariance and chamber-based model. Agricult. For. Meteorol. 106, 61–69. doi: 10.1016/S0168-1923(00)00177-5
Jiang, L., Ma, S., Zhou, Z., Zheng, T., Jiang, X., Cai, Q., et al. (2017). Soil respiration and its partitioning in different components in tropical primary and secondary mountain rain forests in Hainan Island, China. Plant Ecol. 10, 791–799. doi: 10.1093/jpe/rtw080
Kachina, P. (2018). Comparative of plant community and functional compositions of old-growth and secondary forest in Sakaerat Environment Research Station, Nakhon Ratchasima Province. Thai For. Ecol. Res. J. 2, 11–17.
Kane, E. S., Pregitzer, K. S., and Burton, A. J. (2003). Soil respiration along environmental gradients in Olympic National Park. Ecosystems 6, 326–335. doi: 10.1007/s10021-002-0115-7
Kookkhunthod, K., Keywjarern, P., and Kerdsaeng, P. (2018). Estimating Carbon Stock of Primary Dry Dipterocarp Forest, Nakhon Ratchasima and Secondary, Ratchaburi Provinces (Dissertation B.Sc.). Environmental Technology, Srinakharinwirot University, Bangkok, Thailand.
Kowalenko, C., Ivarson, K. C., and Cameron, R. (1978). Effect of moisture content, temperature and nitrogen fertilization on carbon dioxide evolution from field soils. Soil Biol. Biochem. 10, 417–423. doi: 10.1016/0038-0717(78)90068-8
Land Development Department (2004). The Manual of Analysis to Soil, Water, Fertilizer, Plant, Soil Amendments and Analysis for Product Standard Certification. Bangkok: Land Development Sciences Center.
Li, X., Bai, Y., Wen, W., Wang, H., Li, R., Li, G., et al. (2017). Effects of grassland degradation and precipitation on carbon storage distribuutions in a semi-arid temperate grassland of Inner Mongolia. Acta Oecol. 85, 44–52. doi: 10.1016/j.actao.2017.09.008
Litton, C. M., Giardina, C. P., Albano, J. K., Long, M. S., and Asner, G. P. (2011). The magnitude and variability of soil-surface CO2 efflux increase with mean annual temperature in Hawaiian tropical montane wet forests. Soil Biol. Biochem. 43, 2315–2323. doi: 10.1016/j.soilbio.2011.08.004
Liu, S., Luo, D., Yang, H., Shi, Z., Liu, Q., Zhang, L., et al. (2018). Fine root dynamics in three forest types with different origins in a subalpine region of the Eastern Qinghai-Tibetan Plateau. Forests 9, 1–18. doi: 10.3390/f9090517
Maier, M., and Schack-Kirchner, H. (2014). Using the gradient method to determine soil gas flux: a review. Agricult. For. Meteorol. 192–193, 78–95. doi: 10.1016/j.agrformet.2014.03.006
Mavrovic, A., Sonnentag, O., Lemmetyinen, J., Voigt, C., Rutter, N., Mann, P. J., et al. (2023). Environmental controls of winter soil carbon dioxide fluxes in boreal and tundra environments. Biogeosciences 2023:5087. doi: 10.5194/bg-20-5087-2023
Medina, E., and Zelwer, M. (1972). “Soil respiration in tropical plant communities,” in Second International Symposium on Tropical Ecology New Dehli (Athens: Georgia University), 245–260.
Panuthai, S., Junmahasatein, S., and Diloksumpun, S. (2005). Soil CO2 Emissions in the Sakaerat Dry Evergreen Forest and the Maeklong Mixed Deciduous Forest. Proceeding of Climate Change in the Forest Sector “The Potential of Forests to Support the Kyoto Protocol,” August 4–5, 2005. Bangkok: Thailand.
Phianchroen, M., Duandphakdee, O., VChanchae, P., Longkonthean, T., Sawatdee, R., Sawatpon, P., et al. (2008). Instruction of Plant in Dry Dipterocarp Forest at King Mongkut's university of Technology Thonburi at Ratchaburi Campus. Bangkok: King Mongkut's University of Technology Thonburi.
Raczka, N. C., Ho, Q. Y., Srinivasan, V., Lee, M. Y., Ko, C. W., Königer, M., et al. (2023). Greater soil carbon losses from secondary than old-growth tropical forests. Front. For. Glob. Change 6:1135270. doi: 10.3389/ffgc.2023.1135270
Raich, J. W. (1998). Aboveground production and soil respiration in three Hawaiian rain forests. For. Ecol. Manag. 107, 309–318. doi: 10.1016/S0378-1127(97)00347-2
Raich, J. W., and Schlesinger, W. H. (1992). The global carbon dioxide flux in soil respiration and its relationship to vegetation and climate. Tellus B Chem. Phys. Meteorol. 44, 88–99. doi: 10.1034/j.1600-0889.1992.t01-1-00001.x
Royal Forest Department: Ministry of Natural Resources and Environment (2009). Forestry in Thailand. Bangkok: Royal Forest Department; Ministry of Natural Resources and Environment.
Sakaerat Environmental Research Station (2018). General Condition of Sakaerat Environmental Research Station. Available online at: https://www.tistr.or.th/sakaerat/ (accessed October 20, 2018).
Shao, Y., Zhu, Q., Feng, Z., Sun, L., Yang, X., Li, X., et al. (2024). Temporal and spatial assessment of carbon flux dynamics: evaluating emissions and sequestration in the three Northern protection forest project areas supported by Google Earth Engine. Remote Sens. 16:777. doi: 10.3390/rs16050777
Sonkanha, W., Anusontpornperm, S., Thanachit, S., Kheoruenromne, I., and Artchawakom, T. (2012). Soil characteristics under various types of forest in Sakaerat Environmental Research Station. Khon Kaen Agricult. J. 40, 7–18.
Statistical Office of Ratchaburi Province (2017). Meteorological Statistics. Available online at: http://ratburi.nso.go.th/ (accessed December 24, 2017).
Storm, D. (2008). Environmental Lapse Rate: in a Dictionary of Weather. Oxford: Oxford University Press. Available online at: https://www.oxfordreference.com/view/10.1093/acref/9780199541447.001.0001/acref-9780199541447-e-2427 (accessed December 30, 2021).
Suwanprapa, W. (2014). Relationship between Soil Properties, Organic Carbon and Water-Stable Aggregates in Forest Soils of Sakaerat Environmental Research Station (Dissertation M.Sc.). Soil Science, Kasetsart University, Bangkok, Thailand.
Tammadid, W. (2019). Estimating Net Ecosystem Production (NEP) in Primary Dry Dipterocarp Forest Nakhon Ratchasima Province and Secondary Dry Dipterocarp Forest Ratchaburi Province, Thailand (Dissertation M.Sc.). Environmental Technology and Resources Management, Srinakharinwirot University, Bangkok, Thailand.
Tang, J., Baldocchi, D. D., Qi, Y., and Xu, L. (2003). Assessing soil CO2 efflux using continuous measurements of CO2 profiles in soils with small solid-state sensors. Agricult. For. Meteorol. 118, 207–220. doi: 10.1016/S0168-1923(03)00112-6
Wang, C. (2006). Soil respiration in six temperate forests in China. Glob. Change Biol. 12, 2103–2114. doi: 10.1111/j.1365-2486.2006.01234.x
Wang, W. J., Dalal, R. C., Moody, P. W., and Smith, C. J. (2003). Relationships of soil respiration to microbial biomass, substrate availability and clay content. Soil Biol. Biochem. 35, 273–284. doi: 10.1016/S0038-0717(02)00274-2
Wangluk, S., Boonyawat, S., Diloksumpun, S., and Tongdeenok, P. (2013). Role of soil temperature and moisture on soil respiration in a teak plantation and mixed deciduous forest in Thailand. J. Trop. For. Sci. 25, 339–349.
Wigginton, J. D., Lockaby, B. G., and Trettin, C. C. (2000). Soil organic matter formation and sequestration across a forested floodplain chronosequence. Ecol. Eng. 15, S141–S155. doi: 10.1016/S0925-8574(99)00080-4
Wiriyatangsakul, S., Chidthaisong, A., Tripetchkul, S., and Limtong, P. (2006). Effects of moisture and temperature on respiration in tropical forest and agricultural soil. Kasetsart J. 40, 395–409.
Wongtangprasert, O. (2015). The Study of Physical and Chemical Properties of Soil: The Case of Rice are in Chachoengsao and Chonburi Province (Dissertation M.Sc.). Environmental Science, Burapha University, Saen Suk, Thailand.
Xu, M., and Qi, Y. (2001). Soil-surface CO2 efflux and its spatial and temporal variations in a young ponderosa pine plantation in northern California. Glob. Change Biol. 7, 667–677. doi: 10.1046/j.1354-1013.2001.00435.x
Yi, Z., Fu, S., Yi, W., Zhou, G., Mo, J., Zhang, D., et al. (2007). Partitioning soil respiration of subtropical forests with different successional stages in south China. For. Ecol. Manag. 243, 178–186 doi: 10.1016/j.foreco.2007.02.022
Zeng, X., Zhang, W., Shen, H., Cao, J., and Zhao, X. (2014). Soil respiration response in different vegetation types at Mount Taihang, China. Catena 116, 78–85. doi: 10.1016/j.catena.2013.12.018
Zhang, T., Huang, J.-C., Lei, Q., Liang, X., Lindsey, S., Luo, J., et al. (2022). Empirical estimation of soil temperature and its controlling factors in Australia: implication for interaction between geographic setting and air temperature. Catena 208:105696. doi: 10.1016/j.catena.2021.105696
Keywords: soil respiration, litterfall production, air and soil temperatures, soil moisture, primary and secondary forests, dry dipterocarp forest
Citation: Tammadid W, Sangkachai B, Chanonmuang P, Chidthaisong A and Hanpattanakit P (2024) Comparison and environmental controls of soil respiration in primary and secondary dry dipterocarp forests in Thailand. Front. For. Glob. Change 7:1294942. doi: 10.3389/ffgc.2024.1294942
Received: 25 September 2023; Accepted: 26 June 2024;
Published: 12 July 2024.
Edited by:
Anna Walkiewicz, Polish Academy of Sciences, PolandReviewed by:
Apaporn Bulsathaporn, Huachiew Chalermprakiet University, ThailandChuansheng Wu, Fuyang Normal University, China
Copyright © 2024 Tammadid, Sangkachai, Chanonmuang, Chidthaisong and Hanpattanakit. This is an open-access article distributed under the terms of the Creative Commons Attribution License (CC BY). The use, distribution or reproduction in other forums is permitted, provided the original author(s) and the copyright owner(s) are credited and that the original publication in this journal is cited, in accordance with accepted academic practice. No use, distribution or reproduction is permitted which does not comply with these terms.
*Correspondence: Phongthep Hanpattanakit, cGhvbmd0aGVwQGcuc3d1LmFjLnRo