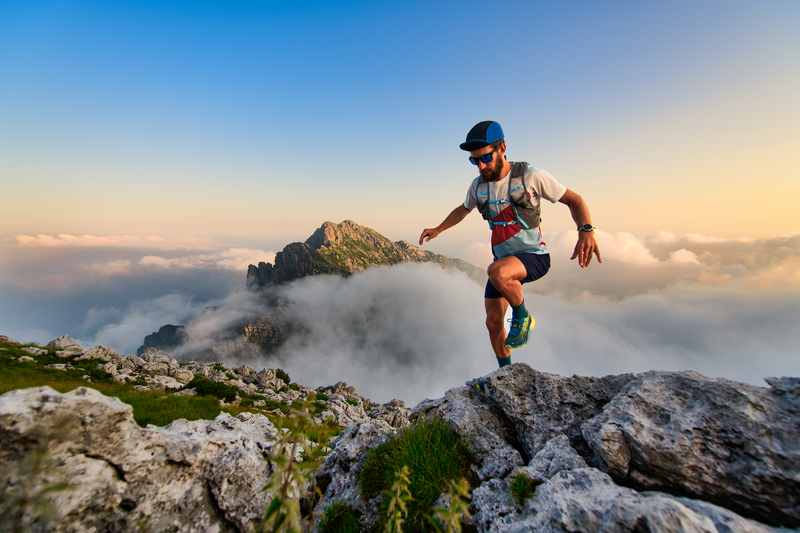
95% of researchers rate our articles as excellent or good
Learn more about the work of our research integrity team to safeguard the quality of each article we publish.
Find out more
ORIGINAL RESEARCH article
Front. For. Glob. Change , 05 January 2024
Sec. Forests and the Atmosphere
Volume 6 - 2023 | https://doi.org/10.3389/ffgc.2023.1301533
Trees can serve as effective biofilters of Particulate matter (PM) pollution, making them valuable for managing air pollution and promoting public health. Leaves of trees can reduce PM through absorption, adsorption, and fallout mechanisms, which are influenced by species-specific characteristics and environmental factors. Although several studies have explored the impact of various leaf characteristics on their ability to adsorb PM from field conditions, few have been conducted in controlled chambers to analyze the adsorption of PM on leaf surfaces and the uptake of metal and non-metal elements from PM on leaves. To fill these knowledge gaps, this study investigated the PM adsorption and leaf characteristics of two different tree species, Pinus densiflora (an evergreen coniferous species) and Quercus acutissima (a deciduous broad-leaved species) under controlled conditions in a PM exposure chamber with a target concentration of 300 μg m−3. The main aim of this study was to measure and compare the rate of PM component (metal and non-metal elements) uptake in two species and investigate the leaf characteristics that contribute to PM adsorption. We investigated the relationship between PM adsorption and physiolog, micro-morphology, and chemical properties of the leaf surface in two species. This study used a Pearson’s correlation analysis and a principal component analysis (PCA) to evaluate correlation between PM adsorption and leaf characteristics and uptake of metal/non-metal elements in PM on leaves. This result showed that leaf characteristics such as stomatal size, leaf roughness, and wax content played a crucial role in PM10 adsorption, while physiological factors like transpiration and leaf boundary layer conductance were identified as important determinants of PM2.5 adsorption on plant leaves. It also observed significant variations in the uptake of aluminum, iron, magnesium, phosphorus, and sulfur. This study not only advances our understanding of the mechanisms behind PM adsorption by tree leaves but also underscores the importance of selecting appropriate tree species based on their leaf characteristics for urban forestry and green infrastructure projects. The ability to strategically use tree species for PM pollution mitigation highlights a practical approach to enhancing environmental sustainability and public health.
Currently, environmental pollution and its adverse impacts on human health are among the most pressing global problems requiring worldwide action (Dodman et al., 2022). Especially, the effects of particulate matter (PM) on human health, visibility, the ecosystem, the weather, and the climate are extensive and are largely contingent upon the properties of the aerosols, including their number concentration, size, and chemical composition (Zhang et al., 2012). The atmospheric PM is a significant air pollutant, with an aerodynamic diameter ranging from 0.003 to 100 μm (Gilliam and Hall, 2016). PM constitutes intricate mixtures of both solid and liquid particles along with accompanying pollutants, such as nitrates, sulfates, polycyclic aromatic hydrocarbons, and metals (Zanobetti et al., 2014). PM can either be directly released into the atmosphere (primary) or generated in the atmosphere through the transformation of gases into particles (secondary). Additionally, both primary and secondary PM are subject to chemical and physical transformations, as well as transportation, processing within clouds, and removal from the atmosphere (An et al., 2019). The exact mechanisms leading to the formation of urban fine particulate matter (PM) remain largely unclear, especially with regards to the processes associated with the origin and growth of PM (Zhang et al., 2015).
Given their broad surface area, ability to respond to particles, and distinct leaf characteristics, trees can serve as effective biofilters and bioindicators to PM pollution. As such, incorporating trees into urban environments can be a valuable strategy for managing PM pollution and promoting public health (Janhäll, 2015). Furthermore, trees can function as a permeable barrier, which modifies the dispersion patterns of local air and provides a sizable surface area for PM deposition (Tomson et al., 2021). Airborne PM can be removed from the atmosphere through both dry and wet deposition, resulting in its deposition onto surfaces of trees. Dry deposition occurs through gravitational sedimentation, which is significantly affected by airflow rates and turbulence. In contrast, during wet deposition, particles and gases are initially captured by precipitation forms such as rain, snow, and fog (Wu et al., 2018).
Leaves of trees can reduce PM through several mechanisms. Firstly, absorption involves the uptake and internal storage of PM by leaves. Additionally, adsorption occurs when PM adheres to the surface of plant leaves through physical and chemical interactions. Lastly, the other mechanism is through fallout, which occurs when PM settles on the leeward side of the trees (Perini et al., 2017). The rates of adsorption and absorption of PM are influenced by a combination of species-specific characteristics, including size, shape, and leaf morphology, as well as additional factors such as location and meteorological parameters, such as precipitation (Sæbø et al., 2012).
Leaves can serve a critical function as an organ that balances protection against water loss, pathogens, and pests with the need for gas exchange to support photosynthesis reactions (Avellan et al., 2021). Although plant leaf morphology varies among species, common features of the leaf surface include trichome hairs, stomata, and hydathode pores (Avellan et al., 2021). The plant cuticle, which covers most of the leaf surface, primarily regulates transpiration but also serves several other functions, such as controlling interactions with environmental factors that can be either harmful or beneficial, including microorganisms, dust, and atmospheric pollutants (Bi et al., 2017). The cuticle also plays a crucial role in reducing PM by capturing and retaining particles through physical and chemical interactions (Read et al., 2020). The characteristics of cuticles can vary greatly among species and even cultivars within a species, and this variation is largely influenced by environmental factors (Du et al., 2015). Also, the process of PM adsorption is influenced by the interaction between the components of PM and certain compounds present on the foliage surface, such as glucosides, proteins, and wax (Avellan et al., 2019). In addition, leaves can indirectly contribute to the reduction of PM concentrations by altering air humidity and temperature and through the processes of evapotranspiration (Hofman et al., 2014; Lee et al., 2022). The interaction between PM particles and the leaf surface is largely influenced by their hygroscopicity, which is significantly impacted by air humidity levels. Changes in humidity can alter the physicochemical characteristics and dispersion state of particles, ultimately affecting their interaction with plant leaves (Burkhardt, 2010). The predominant leaf characteristic responsible for PM adsorption has not been clearly identified (Corada et al., 2021). Further investigation into the leaf characteristics that have the greatest impact on PM removal mechanisms is necessary to identify plant species that are most effective in reducing airborne PM (Chaudhary and Rathore, 2018).
While numerous research efforts have explored how different leaf traits impact particulate matter (PM) adsorption in natural field settings, there has been limited study in controlled chamber environments focusing on PM adsorption on leaf surfaces (Sæbø et al., 2012; Song et al., 2015; Li J. et al., 2021). Furthermore, while previous research has largely focused on the adsorption of particulate matter (PM) onto leaf surfaces, there is a notable lack of studies examining how leaves absorb metal and non-metal elements from PM (Liu et al., 2018; Zhang et al., 2022). To fill these knowledge gaps, this study examined the PM adsorption and leaf characteristics of two functionally distinct tree species, Pinus densiflora (a coniferous evergreen species) and Quercus acutissima (a broad-leaved deciduous species), which are typical pine-oak species in Korea (Kweon and Comeau, 2021). The two species have different morpho-physiological features that may influence their capacity for PM adsorption, including both metal and non-metal elements from atmospheric particles (Uscola et al., 2014). The clear differentiation in leaf characteristics between the two species can provide insights into the specific leaf traits that contribute to PM adsorption. The main objectives of this study were to (1) quantify and compare the rate of adsorption of PM components (Metal and non-metal elements) in P. densiflora and Q. acutissima, and (2) examine which leaf characteristics factor affect the PM adsorption. Based on our hypothesis, the wax-related characteristics, such as wax contents, surface roughness, and microclimate-related characteristics, including transpiration and leaf boundary layer, would be a significant role in determining the capture of particles by the leaf.
In this research, we used 2-year-old seedlings of two common pine-oak species, P. densiflora and Q. acutissima, which are widely distributed and abundant in South Korea, as the test plants for our study (Kweon and Comeau, 2021). Seedlings of similar heights and growth used in this study were obtained from the nursery at the National Institute of Forest Science in Seoul, Korea (37°59′34′′N, 127°04′39′′E) and transplanted into 9 L plastic pots filled with raised bed soil (Forest bed soil, Nongkyung, Incheon, Korea) for the experiment at the University of Seoul in Seoul, Korea (37°58.2′52.6′′N, 127°06.1′49.9′′E). The height and root collar diameter (RCD) of P. densiflora seedlings used in this experiment were measured to be 85.2 ± 8.46 cm and 13.97 ± 2.4 mm, respectively. For the Q. acutissima seedlings, the height and RCD measurements were 83.2 ± 3.27 cm and 11.73 ± 1.49 mm, respectively. Before the start of the experiment, the seedlings were acclimated to growth chamber conditions, which included a day/night temperature of 27/22°C and a relative humidity of 45 ± 2%. This acclimation process lasted for one week and was conducted with natural sunlight. The plants were irrigated daily to prevent water deficit and their positions in the growth chamber were rotated regularly after irrigation to avoid any positional effects. To avoid any potential canopy interference from other plants, three seedlings of each species were kept in a control chamber, while another six seedlings were placed in a treatment chamber. The topsoil of each pot was covered with transparent polyethylene bags to prevent the deposition of airborne PM on the soil surface. Leaf sampling was conducted twice: once before exposure and again 14 days after exposure (DAE). Fully expanded leaves were sampled and measured between 09:00 h and 12:00 h for analyses of physiological, morphological and microstructural characteristics, stomatal features, and leaf surface chemical properties. To assess PM adsorption on foliage, leaf samples were collected at 14 and 28 days after exposure (DAE). This sampling schedule was designed to evaluate the outcomes at both the intermediate and final stages of the study period. This experiment was conducted with three replications, with each replication consisting of three seedlings from each species per treatment. The experimental replications were conducted over a period of 28 days.
This study used growth chambers (Growth chamber, Koito Industries, Yokohama, Japan) that were equipped with a solid aerosol generator (SAG 410, Topas GmbH, Dresden, Germany) to conduct the experiment in the period between June and August of 2021. The details of the exposure system have been previously outlined in Lee et al. (2022). In the designed chamber, solid particles were transported or deposited on the surface through convective diffusion and other external forces in addition to Brownian motion and gravitational sedimentation (Figure 1). Furthermore, a triple-layer charcoal filter was installed inside the chamber to prevent external PM from entering and to inhibit the re-entry of particles that had accumulated due to convective diffusion and other external forces. Fly ash, an anthropogenic PM that is produced as a byproduct of coal combustion (Vargas Buonfiglio et al., 2017), was generated using a solid aerosol generator in order to simulate PM pollution conditions in plants. In this study, we used Fly ash test powder (JIS test powder class 10, APPIE, Kyoto, Japan), which has a particle diameter mostly under 10 μm. In the treatment chamber, the plants were exposed for 9 h to fly ash particles, maintaining a target concentration of 300 μg/m3 and a flow rate of 25 liters per minute (LPM). During the experiment period, the actual PM concentration was maintained at 292.32 ± 33.56 μg/m3, and the daily average of particulate matter was 108.83 ± 10.74 μg/m3. As a result, on 14 DAE, the total weight of PM treated in the chamber was approximately 57 mg, and on 28 DAE, it reached about 114 mg. The PM concentration used in the study was set based on the PM10 warning level specified by air quality standards in Korea (Korea Ministry of Government Legislation, 2019). And, the duration of PM exposure in this study was determined in accordance with the PM pollution data recorded in South Korea during the year 2020 (National Institute of Environmental Research, 2021). The daily variation environmental conditions in the PM-fumigated chamber are shown in Figure 2.
Figure 1. Schematic diagram of the phytotron growth chamber and its environmental control system, which was adapted from Lee et al. (2022).
Figure 2. The daily variations in actual PM concentration (solid gray line) and targeted PM concentration (dashed gray line), as well as temperature (red dotted line), relative humidity (blue dotted line), and photosynthetic photon flux density (yellow bar) in the PM-exposed chamber.
The mass of PM adsorption on leaf surface was evaluated by using a modified method derived from the works of Dzierżanowski et al. (2011) and Kwak et al. (2020). Leaves of each plant species were randomly selected for sampling at 14 and 28 DAE. The collected leaves were placed in a glass bottle containing 200 mL of deionized water and were cleaned using an ultrasonic cleaner (HSt Powersonic 620, Hwashin Technology Company, Seoul, Korea) for 1 min to remove particles from the leaf surfaces, which is referred to as ‘surface PM’. The collected water was passed through a glass filter funnel equipped with a stopper support assembly and connected to a vacuum pump (Rocker 300, Rocker Scientific Co., Ltd., Taipei, Taiwan), followed by sequential filtration through three filters having different pore sizes of 10 μm (Nylon filter, Merck Millipore, Darmstadt, Germany), 3 μm (Mixed cellulose ester (MCE) filter, Merck Millipore, Darmstadt Germany), and 0.2 μm (MCE filter, Merck Millipore, Darmstadt, Germany). Before the filtration process, the filters used for the analysis were dried for 30 min at 60°C in a dry oven (HQ-FDO 84, Coretech Korea co., Anyang, Korea). Subsequently, they were allowed to stabilize in the weighing room to attain filter humidity equilibrium before weighing. To prevent the filters from being affected by electrostatic charges, they were exposed to an antistatic ionizer (Antistatic kit, Mettler Toledo, Schwerzenbach, Switzerland) prior to weighing. The filters were pre-weighed on a microbalance (XPR2, Mettler Toledo, Schwerzenbach, Switzerland). The filters were dried and weighed again using the same procedure as the pre-weighing step to determine the mass of PM in each sample. Two fractions of particulate matter, namely surface PM10 (SPM10) (0.2–10 μm) and surface PM2.5 (SPM2.5) (0.2–3 μm), were collected on filters. The mass of PM was calculated by dividing the weight of the filtered particles by the leaf area of each species. Following the rinsing step with deionized water, the leaves were exposed to 150 mL of chloroform for 40 s to remove the epicuticular wax layer from the leaf tissues and to remove any trapped particles in the wax layer, which is referred to as “wax PM”. The method for filtering wax PM was identical to the method used for surface PM filtration. The leaf sample’s total area was determined using winFolia image software (winFolia, Regent Instruments Inc., Sainte-Foy, QC, Canada) to calculate the mass of PM in μg cm−2 leaf area. The amount of particulate matter was measured after washing both adaxial and abaxial surfaces of the leaves; however, the results were reported based on the surface area of one side of the leaf.
For the measurement of PM adsorption area, leaf samples were harvested at 14 and 28 DAE between 09:00 h and 12:00 h and free-dried using a lyophilizer (FD 8508, ilShinbiobase CO. Ltd., Dongducheon, Korea). PM adsorption area was evaluated via field emission scanning electron microscopy (FE-SEM; SU8010, Hitachi High-Tech, Tokyo, Japan). Samples of Q. acutissima leaves were cut into 5 mm × 20 mm sizes, while P. densiflora leaves were cut into 20 mm sizes, and then attached to a carbon mount in preparation for SEM analysis. The PM adsorption area per unit area was calculated as mm2 per 100 mm2.
The content of metal elements (Al, Fe, and Mg) and non-metal elements (P and S) in leaves was determined using the method described by Pequerul et al. (1993). The leaves harvested at 14 and 28 DAE were dried for 48 h at a temperature of 60°C. 0.2 g of leaf samples (dry weight) placed in a 50 mL dry flask, followed by adding 2 mL of 70% HNO3 and stirred. All the material was in a wet state when 1.6 mL of 30% H2O2 was added with caution in a well-ventilated hood, followed by a slight stirring after the addition. It was heated to 100°C on a hot plate, resulting in a strong effervescence. When the density of the brown fumes decreased after about 7–8 min, the solution was left to cool. A slightly yellow solution and a small amount of white solid in suspension remained. The solution was then filtered through a syringe filter with a 0.2 μm pore size and diluted to 10 mL using distilled water. The solution was analyzed by inductively coupled plasma optical emission spectrometry (ICP-OES) to quantify Al, Fe, Mg, P and S concentrations. The method used to determine the content of metal and non-metal elements in fly ash was the same as the method used for the leaves.
Fully developed leaves of the test plants were measured for gaseous exchange parameters, including stomatal conductance (gs), transpiration rate (Trmmol), and leaf boundary layer conductance (gbw), before exposure (0 DAE) and at 14 DAE between 09:00 h and 12:00 h by using a portable photosynthesis measurement system (Li-6400 XT, LI-COR Inc., Lincoln, NE, United States) with an LED light source chamber (6400-02B, LI-COR Inc., Lincoln, NE, United States) (Farquhar et al., 1980). The measurements were conducted with a maintained CO2 concentration of 400 μmol mol−1 and a block temperature set at 27°C, with a relative humidity (RH) range between 50 and 60%. The photosynthetically active radiation (PAR) was set to a near-saturation light intensity of 1,000 μmol m−2 s−1, and the air flow rate was held at a constant of 500 μmol s−1. The leaf boundary layer (gbw) was determined through simultaneous measurements, which included the humidity of the air inside the chamber, as well as the temperature and transpiration rate (Trmmol) from leaves, following the method outlined by Parkinson (1985) with the formular below:
(1)
In this formula, “A” represents the projected leaf area (m2) within the chamber. The term “χf” (mol mol−1) denotes the saturation humidity at the temperature of the leaves, while “χo” (mol mol−1) refers to the humidity level of the air as it exits the chamber.
Leaf samples were obtained from each chamber at 0 and 14 DAE for the assessment of micro-morphological features. The leaf area and width-to-length ratio were measured on five fully developed leaves of each tree using winFolia image software (winFolia, Regent Instruments Inc., Sainte-Foy, QC, Canada). After collecting the leaves, they were dried for 48 h at 60°C to obtain their dry weights. The specific leaf area (SLA) was then determined by dividing the leaf area by the dry weight of each leaf, following the method of Wilson et al. (1999).
The stomata traits of leaves were determined using the same procedure as that used for measuring PM adsorption area, as mentioned above. The stomatal density per leaf and size of stomata were assessed via filed emission scanning electron microscopy (FE-SEM).
After stomata traits observations by the FE-SEM, we measured the 3D topography and roughness of leaf surfaces at 50× magnification using a non-contact surface profiler (Bruker Contour GT-K™, Bruker nano GmbH, Berlin, Germany). The leaf surface measurements enabled the determination of several roughness parameters, including the average roughness (Ra), maximum profile peak height (Rp), root mean square roughness (Rq), which is the square root of the average squared deviation between height measurements, and total height of the profile on the leaf surface (Rt).
The leaf wettabilty or hydrophobicity was evaluated using a modified sessile droplet method adapted from Brewer et al. (1991). Leaf blade fragments from the adaxial and abaxial surfaces of the test plants were cut using a razor blade and mounted on glass slides with double-sided tape. Using a contact angle analyzer (UNI-CAM/m, GITsoft, Ansan, Korea), the drop contact angle of deionized water and DMF (N,N-dimethylformamide) (3 μL for P. densiflora and 7 μL for Q. acutissima) with the leaf surface was measured to determine leaf wettability. The following liquids were used for the contact angle measurements: deionized water (total surface tension: 72.8 mN m−1, dispersive component: 29.1 mN m−1, and polar component: 43.7 mN m−1) and N,N-dimethylformamide (total surface tension: 37.3 mN m−1, dispersive component: 32.42 mN m−1, and polar component: 4.88 mN m−1) (Jańczuk et al., 1989).
The wax content was analyzed by the method of Ebercon et al. (1977), which involves observing the color change that results from the reaction of acidic potassium dichromate (K2Cr2O7) with the waxes on the leaf surface. Circular leaf discs with a diameter of 8 mm and a total weight of 0.1 g were prepared from the leaves harvested at 0 and 14 DAE in each chamber, for wax extraction. The leaf discs were immersed in 10 mL of HPLC-grade chloroform for 30 s to extract the leaf wax. The duration of the immersion time was previously established to ensure complete removal of the epicuticular wax from the leaf surface (Mayeux and Jordan, 1984; Huggins et al., 2018). The extract was transferred to a clean 2 mL glass vial and dried using a nitrogen evaporator (BT1607, BT lab systems, St. Louis, MO, United States) maintained at 70°C for 30 min. The extract obtained after removing chloroform was oxidized by adding 0.3 mL of K2Cr2O7 and heating it for 30 min at 100°C. The vials were cooled down for a while. Then, 0.7 mL of deionized water was added to each vial and left for color development for 1 h. Measurements of optical density for each sample were obtained using a spectrophotometer (Synergy H1, BioTek instruments, Inc., Winooski, VT, United States) at a wavelength of 590 nm. The wax content was determined by comparing against a standard curve generated at the same wavelength using known concentrations of carnauba wax. The results were expressed as mg dm−2.
To measure surface pH, fully developed leaves of the test plants were used at 0 and 14 DAE between 09:00 h and 12:00 h, using a portable pH meter (HI99171, Hanna instruments, Padova, Italy). Surface pH of leaves was determined by placing the pH electrode at five random positions on the leaf surface of the test plants, and the results were then averaged.
All statistical analyses were performed using SPSS Statistics 26 (SPSS Inc., Chicago, IL, United States) and the statistical significance level was set at p < 0.05. The normal distribution of data was assessed using the Shapiro–Wilk test, and homogeneity of variances was checked using the Levene test. The data were then analyzed using one-way ANOVA with Tukey’s HSD (p < 0.05) as a post hoc test to investigate metal/non-metal element content on leaves among times and species. An independent t-test (p < 0.05) was used to determine significant differences in leaf characteristics at 0 DAE between P. densiflora and Q. acutissima, as well as significant differences in mass and area of leaf surface PM adsorption for each day between the two species. Pearson correlation was performed to investigate the relationship between the ability of PM adsorption (PM10 or PM2.5) and leaf characteristics of both tree species at each DAE, and a principal component analysis (PCA) was performed to identify the main factors that could potentially influence PM adsorption. The values in the figures and tables are the mean ± SD. The data were processed using Microsoft Excel (Excel 16.0, Microsoft Corporation, Redmond, Washington, DC, United States).
Figure 3 represents the different sizes and shapes of fly ash particles on the adaxial leaf surface of P. densiflora and Q. acutissima. As depicted in Figure 3, most fly ash particles exhibit a spherical shape. The spherical shape of the particles indicates that they were likely formed during high-temperature combustion processes involving coagulation, condensation, and nucleation of evaporated materials. Particles within the size range of 0.001–10 μm, from nanoscale to microscale, are commonly produced during high-temperature combustion processes (Akinyemi et al., 2019). The figure reveals obvious differences in the surface structures between the two species. The most notable distinction is that P. densiflora leaves are amphistomatous, with stomatal pores present on both sides, while Q. acutissima leaves are not. The observed differences also revealed that the adaxial surface of P. densiflora had fly ash deposits on the inner walls of stomata or the periphery of guard cells (Figures 3A,C). In Q. acutissima, fly ash deposition was observed around grooves on the leaf surface (Figures 3B,D). Different sizes and shapes of fly ash particles were observed between 14 and 28 DAE. At 28 DAE, there was a noticeable increase in the abundance of larger particles on the leaves of both species compared to 14 DAE. Large fly ash particles are primarily formed through the aggregation of smaller particles and have an irregular and loose surface. Differences in PM adsorption ability was observed between the adaxial and abaxial surfaces of both species (data not shown), possibly due to the transportation of particles via Brownian motion and gravitational sedimentation from the top to bottom in the chamber. Due to significantly lower levels of PM adsorption, compared to the adaxial surface, the images and results of PM adsorption from the abaxial surface were not included in this study.
Figure 3. Field emission scanning electron micrographs of adaxial leaf surface of P. densiflora and Q. acutissima exposed to 300 μg m−3 of fly ash particles at 14 and 28 days after the beginning of exposure (DAE). Micrographs of adaxial surfaces of P. densiflora at 14 and 28 DAE (A,C) and Q. acutissima at 14 and 28 DAE (B,D), with white dashed circles indicating fly ash particles on the leaves, and asterisks indicating stomata.
We also examined the mass of PM and PM adsorption area on the foliage of P. densiflora and Q. acutissima, which allowed us to compare their respective PM adsorption abilities (Table 1). At 14 DAE, there was a significant increase in the mass of surface PM10 and PM2.5 on the leaves of P. densiflora compared to Q. acutissima, with a difference of 43 and 75%, respectively (p < 0.01). There was no significant difference in wax PM10 and PM2.5 between the leaves of P. densiflora and Q. acutissima at 14 DAE. However, at 28 DAE, there was a significant difference in wax PM10 and PM2.5 between the leaves of P. densiflora and Q. acutissima, with a difference of 75 and 77%, respectively (p < 0.05 and p < 0.001). The trend in PM adsorption area was also similar to that of the mass of PM adsorption. P. densiflora showed a significantly higher PM adsorption area than Q. acutissima at both 14 and 28 DAE (p < 0.001). The mass of total PM10 showed a significant positive correlation with PM adsorption area on the leaves of both P. densiflora and Q. acutissima (R2 = 0.806, as shown in Figure 4).
Table 1. Mass of PM and PM adsorption area on leaves of P. densiflora and Q. acutissima exposed to 300 μg m−3 of suspended fly ash particles at 14 and 28 days after exposure began (DAE).
Figure 4. Relationship between mass of total PM10 on leaves (μg cm−2) and PM adsorption area on leaves (mm2 100 mm−2) of P. densiflora and Q. acutissima exposed to 300 μg m−3 of suspended fly ash particles at 14 and 28 DAE.
Among the elements examined in fly ash particles, the concentration of metals (Al, Fe, Mg) and non-metals (P and S) accounted for 58%, 26%, 7%, 5%, and 4%, respectively (Figure 5). Al exhibited the highest concentration in the fly ash particle, while the concentrations of Mg, P, and S were the lowest among the elements examined. Figure 5 also shows that there were differences in the foliar uptake of elements from fly ash particles between the two species. Significant differences in Al concentration on the leaves were observed between the control and treatment for both P. densiflora and Q. acutissima at both 14 and 28 DAE. Although no significant difference was observed between the treatment of P. densiflora and Q. acutissima at 14 DAE, a significant difference in the Al concentration on the leaves was found at 28 DAE, with P. densiflora exhibiting a higher concentration compared to Q. acutissima. In terms of Fe concentration on the leaves, both P. densiflora and Q. acutissima showed significant differences between the control and treatment at both 14 and 28 DAE. Similar to the Al concentration, there was no significant difference observed in Fe concentration between the treatment of P. densiflora and Q. acutissima at 14 DAE. At 28 DAE, Fe concentrations on the leaves of Q. acutissima were significantly higher than those of P. densiflora. The Mg concentration on the leaves did not show any significant differences between the control and treatment for both P. densiflora and Q. acutissima at 14 and 28 DAE, except for Q. acutissima at 28 DAE, unlike the Al and Fe concentrations. A significant difference in Mg concentration on the leaves between the control and treatment was only observed for Q. acutissima at 28 DAE. In terms of P concentration on the leaves, no significant differences were observed between P. densiflora and Q. acutissima, as well as between the control and treatment for both species at both 14 and 28 DAE. The S concentration on the leaves exhibited significant differences between the control and treatment for both P. densiflora and Q. acutissima at both 14 and 28 DAE, except for P. densiflora at 28 DAE where no significant difference was found.
Figure 5. Metal (Al, Fe, Mg) and non-metal elements (P and S) concentrations in leaves of P. densiflora and Q. acutissima exposed to 300 μg m−3 of suspended fly ash particles at 14 (A) and 28 DAE (B). Data are plotted as means ± SD (n = 3). Significant differences between treatments and species at 14 DAE and 28 DAE were indicated as lower case according to Tukey’s HSD test. Asterisks indicate significant differences between control and treatment for each tree species at 14 and 28 DAE (* p < 0.05, ** p < 0.01, *** p < 0.001). This figure (C) also displays the concentration of both metal and non-metal elements in the fly ash (n = 5).
To compare the two species, we used FE-SEM to analyze the structural characteristics of P. densiflora and Q. acutissima leaves at 0 DAE (Figure 6). The SEM images demonstrated marked distinctions in stomatal appearance between the two species. The stomatal distribution differed significantly between P. densiflora and Q. acutissima leaves, with P. densiflora exhibiting amphistomatic characteristics and Q. acutissima being non-amphistomatic. The abaxial surfaces of P. densiflora and Q. acutissima exhibited significant differences in stomatal density. Comparison of the two species showed a significant difference in stomatal density on the abaxial leaf surface, with Q. acutissima exhibiting a 79% higher density than P. densiflora (p < 0.001). Conversely, the stomatal size on the abaxial leaf surface was significantly larger in P. densiflora than in Q. acutissima, with a difference of 77% (p < 0.001) (Table 2).
Figure 6. Field emission scanning electron micrographs showing stomata traits of P. densiflora and Q. acutissima before 300 μg m−3 of suspended fly ash particles exposure (0 DAE). Abaxial surface micrographs of P. densiflora (A,C) and Q. acutissima (B,D) at 60 and 1,000 × magnification, respectively.
Table 2. Physiological characteristics (stomatal conductance, transpiration, and leaf boundary layer conductance) and structural traits (stomatal density and stomatal size) of P. densiflora and Q. acutissima before 300 μg m−3 of suspended fly ash particles exposure (0 DAE).
To compare the leaves of P. densiflora and Q. acutissima, we analyzed physiological characteristics, including stomatal conductance, transpiration, and leaf boundary layer conductance, in response to microclimate changes around the trees (Table 2). There were highly significant differences observed in all physiological parameters between the leaves of P. densiflora and Q. acutissima. In terms of stomatal conductance, transpiration, and leaf boundary layer conductance, P. densiflora exhibited significantly higher values than Q. acutissima, with increases of 42%, 46%, and 61%, respectively (p < 0.05, p < 0.01, and p < 0.001).
All contact angle values were individually determined and compared for both species in deionized water (DW) and N,N-dimethylformamide (DMF), and the summarized results are presented in Figure 7 and Table 3. A lower contact angle value, as obtained from the measurement results, indicates better surface wetting (Good, 1992). The wetting behavior of a plant leaf is multifaceted, determined by both the characteristics of the solvent and the properties of the leaf surface. Liquids with varying surface tension properties, including polar and dispersion components, can result in different contact angle values on the surface of plant leaves, as shown in Figure 7 and Table 3. Significant differences were observed in the contact angles of DW and DMF on the adaxial and abaxial leaf surfaces between the two species, with P. densiflora showing significantly higher values than Q. acutissima in all parameters (Table 3). Wax contents were also analyzed to compare the leaf surface chemical characteristics of P. densiflora and Q. acutissima (Table 3). The wax content of P. densiflora was significantly higher than that of Q. acutissima, with a 52% increase (p < 0.001). The leaves of P. densiflora and Q. acutissima did not show any significant differences in surface pH, in contrast to the significant difference in wax content (Table 3).
Figure 7. The optical images of a deionized water (DW) and N,N-dimethylformamide (DMF) droplet on adaxial and abaxial leaves of P. densiflora and Q. acutissima before 300 μg m−3 of suspended fly ash particles exposure (0 DAE). The optical images of DW droplet on adaxial and abaxial leaves of P. densiflora (A,E) and Q. acutissima (C,G), respectively. The optical images of DMF droplet on adaxial and abaxial leaves of P. densiflora (B,F) and Q. acutissima (D,H), respectively.
Table 3. Leaf wettability (deionized water (DW) and N,N-dimethylformamide (DMF) contact angle) and chemical characteristics (wax content and surface pH) of P. densiflora and Q. acutissima before 300 μg m−3 of suspended fly ash particles exposure (0 DAE).
The leaf surfaces of P. densiflora and Q. acutissima were examined for morphological and microstructural features, with results presented in Figures 8A,B, respectively. The different leaf shapes of P. densiflora and Q. acutissima are shown in Figure 8A. In terms of specific leaf area (SLA), Q. acutissima (88.89 ± 4.19 cm2 g−1) had significantly higher values compared to P. densiflora (67.93 ± 17.08 cm2 g−1) (p < 0.05) (Table 4). The width to length ratio (W/L) was significantly higher in Q. acutissima than in P. densiflora, with a difference of 89% (p < 0.001) (Table 4).
Figure 8. (A) Selected leaf images and (B) of P. densiflora and Q. acutissima before 300 μg m−3 of suspended fly ash particles exposure (0 DAE). Abaxial surface micrographs of P. densiflora (a,c) and Q. acutissima (b,d) at 60 and 1,000× magnification, respectively.
Table 4. Micro-morphological and morphological characteristics (specific leaf area and width/length ratio) of P. densiflora and Q. acutissima before 300 μg m−3 of suspended fly ash particles exposure (0 DAE).
Using a non-contact surface profiler, we captured 3D topography images of the adaxial and abaxial surfaces of P. densiflora and Q. acutissima leaves, as shown in Figure 8. 3D topography images revealed significant differences in leaf roughness between P. densiflora and Q. acutissima (Figure 8B). The presence of obvious ridges on the leaf surfaces of both P. densiflora and Q. acutissima, shown in Figure 8, indicate their high capacity for PM adsorption due to their rough leaf surfaces. The average roughness (Ra) is a parameter mostly used to quantify the roughness of leaf surfaces. In both the adaxial and abaxial surfaces of the leaves, P. densiflora showed significantly higher values of Ra than Q. acutissima, with an increase of 48 and 58%, respectively (p < 0.01) (Table 4). Similar to Ra, P. densiflora demonstrated significantly higher values of Rt (representing total height of the profile on the leaf surface) than Q. acutissima on both the adaxial and abaxial surfaces of the leaves, with increases of 26 and 47%, respectively (p < 0.01 and p < 0.001) (Table 4). However, there was no significant difference observed between the two species in Rp, which represents the maximum profile peak height.
The correlation between the absorption of PM10 and PM2.5 on leaves at 14 and 28 DAE, and the characteristics of P. densiflora and Q. acutissima leaves, are represented in Figures 9A,B. These leaf characteristics, assessed at 0 and 14 DAE, include stomatal features, leaf wettability, and gas exchange, chemical, morphological, and micromorphological attributes. The leaves of both species at 0 and 14 DAE were examined for PM adsorption over a period of two weeks to determine the amount of PM adsorbed. Wax, W/L (width-to-length ratio), SD (stomatal density), SS (stomatal size), Ra, and Rt exhibited a significant correlation with the adsorption of total PM10 on leaves. Of these, SS and Ra displayed the strongest positive correlation with TPM10 (Mass of total PM10 on foliage). However, both W/L and SD were significantly negatively correlated with TPM10. TPM2.5 (Mass of total PM2.5 on foliage) adsorbed by plant leaves was significantly correlated with Trmmol (transpiration), gbw (leaf boundary layer conductance), wax, W/L, SD, SS, Ra, and Rt. Only TPM2.5 showed significant correlation with Trmmol and gbw, while TPM10 did not show any correlation with these parameters. Ra also exhibited the strongest positive correlation with the adsorption of TPM2.5 on the leaf surfaces. Focusing on a single factor is insufficient and neglects the combined effects of multiple factors on PM adsorption by plant leaves. With an initial assessment of variable correlations, PCA was used to categorize each factor and reduce the principal components. In addition, this study also used loading plots of PCA to classify the parameters based on their relationship with metal and non-metal elements present in PM.
Figure 9. Correlations between PM adsorption [total PM10 (A) and PM2.5 (B)] and leaf characteristics of test plants. Cond = stomatal conductance; Trmmol = transpiration; gbw = leaf boundary layer conductance; CA = deionized water contact angle on adaxial leaves; SLA = specific leaf area; W/L = width-to-length ratio; SD = Stomatal density; SS = Stomatal size; Ra = the average roughness; Rp = maximum profile peak height; Rq = root mean square roughness; Rt = total height of the profile on the leaf surface. Red bar indicates significant positive relations and blue bar indicates significant negative relations. The asterisks indicate the level of significant differences based on Pearson correlation (* p < 0.05, ** p < 0.01, *** p < 0.001).
After performing PCA, three principal components were generated, which collectively accounted for 79.75% of the variance in the data (Figure 10). The first principal component (PC1) was able to explain 47.38% of the total variations, indicating its strong explanatory power. PC 1 was mainly based on width-to-length ratio of leaf (W/L), stomatal characteristics (SD and SS), and leaf roughness. The variables Ra, Wax, Cond, and SS had loading values of 0.91, 0.89, 0.81, and 0.80, respectively, and were located in the positive coordinate of PC1 (Table 5). The variables SD and W/L were located in the negative coordinate of PC1 with loading values of −0.98 and − 0.94, respectively. The second principal component (PC2), which accounted for 23.24% of the total variations, was dominated by PM adsorption including both TPM10 and TPM2.5 and metal elements on leaves such as Fe, Al, and Mg (Table 5).
Figure 10. Principal component analysis (PCA) of PM adsorption, metal/non-metal elements content, and leaf characteristics of P. densiflora and Q. acutissima. Parameters are projected onto PC1 and PC2. TPM10 = total PM10; TPM2.5 = total PM2.5; Cond = stomatal conductance; Trmmol = transpiration; gbw = leaf boundary layer conductance; CA = deionized water contact angle on adaxial leaves; SLA = specific leaf area; W/L = width-to-length ratio; SD = Stomatal density; SS = Stomatal size; Ra = the average roughness; Rp = maximum profile peak height; Rq = root mean square roughness; Rt = total height of the profile on the leaf surface.
Table 5. Principal component analysis (PCA) results for PM adsorption, metal/non-metal elements content, and leaf characteristics in P. densiflora and Q. acutissima.
In this study, we investigated the PM adsorption ability and leaf characteristics of two tree species, Pinus densiflora and Quercus acutissima, which exhibit distinct differences in leaf traits to identify the key leaf characteristic responsible for PM adsorption. In plants, leaves are the predominant location of PM adsorbed, with the structure of leaf morphology playing a crucial role in their PM capturing efficiency (Castanheiro et al., 2020). Leaf morphological traits, such as size, shape, surface texture, stomatal characteristics, epidermal wax layers, and wettability, play a crucial role in determining their proficiency in adsorbing PM (Esposito et al., 2020; Zhang et al., 2022). Some species, however, have leaves with distinct morphological characteristics, including increased roughness and a wax layer, that can elevate their efficiency in capturing particles (Li J. et al., 2021; Sharma and Saxena, 2022). Although many studies have investigated the effects of various leaf characteristics on their ability to adsorb PM in field conditions, few studies have been conducted in controlled chambers to examine PM adsorption on leaf surfaces. The adsorption of PM by leaves varies depending not only on leaf characteristics, but also on the physical and chemical properties of the particles (Burkhardt, 2010; Read et al., 2020). For this study, fly ash was chosen as the particulate matter source because it is a byproduct of coal combustion and is considered a primary source of fine PM in ambient air, thus allowing for a realistic simulation of PM adsorption by leaves (Zierold and Odoh, 2020). The adaxial leaf surfaces of P. densiflora and Q. acutissima are shown in Figure 3, displaying the various sizes and shapes of fly ash particles. The SEM image shows a significant increase in the abundance of larger particles on the leaves of both species at 28 DAE compared to 14 DAE (Figure 9). Larger particles on the leaves are typically formed through the aggregation of smaller particles over time, which is largely influenced by changes in the microclimate due to plant transpiration and the hygroscopic properties of the particles (Barwise and Kumar, 2020). The correlation between the adsorption of TPM2.5 and transpiration observed further supports the phenomenon of particle aggregation on the leaf surface over time (Figure 9). This correlation suggests that transpiration plays a role in creating changes in the microclimate of the leaf surface, which in turn leads to the aggregation of smaller particles into larger ones.
We observed that PM was able to enter the stomatal cavity and/or obstruct the stomata, based on our analysis of the SEM images of leaf surfaces of P. densiflora (Figure 3). This result is consistent with previous research indicating that particles may enter leaf tissues through the stomatal cavity and subsequently block the stomata (Przybysz et al., 2014; Song et al., 2015). Kwak et al. (2023) confirmed the attachment of PM particles inside the inner wall of stomata or the periphery of guard cells in evergreen species, which have easily distinguishable stomata compared to other tree species. From the results of the correlation analysis revealed a significant positive association between stomatal size and the adsorption of TPM10 and TPM2.5 for both species, with correlation coefficients of 0.583 and 0.501 (p < 0.01 and p < 0.05), respectively (Figure 9). The distribution of stomata on P. densiflora and Q. acutissima leaves varied significantly, with P. densiflora being amphistomatic and Q. acutissima being non-amphistomatic (Figures 3, 6). Furthermore, the size of stomata on the abaxial leaf surface was found to be significantly larger in P. densiflora compared to Q. acutissima (Table 2). As a result, our findings are consistent with previous research indicating that pine species have a greater propensity to accumulate particulate matter than broadleaved species (Beckett et al., 1998; Grantz et al., 2003; Sæbø et al., 2012; Cayuela et al., 2019). In the PCA analysis of the current study, stomatal size along with other parameters such as Ra, Trmmol, and Wax, which showed a positive correlation with PM adsorption, were located in the same group (Figure 10). By using PCA to analyze the data, we were able to consider the impact of various factors related to stomatal size on the adsorption of PM by plant leaves, rather than neglecting the role of certain stomatal size-related factors and obtaining an inaccurate assessment of the phenomenon (Tian et al., 2019). It is a widely accepted fact that stomatal size plays a crucial role in regulating physiological processes, such as transpiration and photosynthesis, which in turn affect plant growth and survival (Büssis et al., 2006; Carignato et al., 2019).
There were significant distinctions observed between the parameters that correlated with TPM10 and those that correlated with TPM2.5 (Figure 9). Physiological characteristics such as transpiration and leaf boundary layer conductance were only related to the adsorption of TPM2.5. Specifically, we found that physiological characteristics such as transpiration and leaf boundary layer conductance were only associated with the adsorption of TPM2.5. The ability of fly ash particles to absorb moisture suggests that raising the humidity levels could have increased the efficiency of PM adsorption for particles smaller than 2.5 μm (Sepahvand et al., 2022). Leaf boundary layer conductance is recognized as an essential parameter for PM adsorption since the initial deposition of particles on the leaf surface is significantly affected by Brownian motion. Following the deposition, the particles tend to wrap around the airflow and disperse along the boundary layer between the leaf and the atmosphere before eventually adhering to the leaf surface (Yin et al., 2020).
By analyzing SEM images of Q. acutissima leaves, we were able to observe that PM particles were attaching to grooves on the leaf surface (Figure 3). As reported in earlier studies, the existence of stomata, grooves, and trichomes on leaf surfaces promotes the adhesion of PM, which is supported by our findings (Liang et al., 2016; Li X. et al., 2021). Tree species that have ribbed, folded, rough, and hairy leaf surfaces have been found to be more effective in PM adsorption compared to species with unfolded, smooth surfaces (Niu et al., 2020; Zhang et al., 2023). The correlation analysis results indicated a strong positive significance between leaf roughness and the adsorption of both TPM10 and TPM2.5 (Figure 9). The stomata size of P. densiflora contributed to significantly higher values of Ra, which represents the average of leaf surface, compared to Q. acutissima on both the adaxial and abaxial surfaces of the leaves. Recent studies on the removal efficiency of vegetation for PM have mainly concentrated on investigating the correlation between leaf characteristics, microstructure, and particle accumulation (Liu et al., 2018; Read et al., 2020; Avellan et al., 2021). Additionally, various studies have concentrated primarily on the velocity of particle deposition, a factor influenced by tree canopies and the leaf area index (LAI), which plays a critical role in determining the efficiency of trees in removing PM (Han et al., 2020; Corada et al., 2021; Sonwani et al., 2022).
Besides the physical features of the leaf surface, the chemical properties of plant leaves are also closely linked to PM adsorption (Avellan et al., 2021). The attachment of particles to foliage is affected by the degree of attraction between functional groups present on the particle surface and specific compounds found on the foliage surface, including glucosides, proteins, and wax (which contains polysaccharides, fatty acids, fatty alcohols, and fatty aldehydes) (Avellan et al., 2019). Our research into the chemical properties of P. densiflora and Q. acutissima involved an examination of leaf surface wettability, surface pH, and leaf wax content to make comparisons between the two species (Table 3). Limited studies have assessed the plant surface roughness, and the distribution of chemical compounds on cuticle surfaces remains unknown. Both of these factors are expected to influence the contact phenomena of surface-deposited water, aerosol particles, or microorganisms (Barthlott et al., 2017). The wettability of leaves, which is influenced by the properties of the leaf surface cuticle and trichomes, as well as leaf shape morphology, can reduce transpiration and promote foliar uptake (Fernández et al., 2021). However, in this study, no significant relationship was found between contact angle and PM adsorption (Figure 9). The differential particle adsorption observed between the two species may have also been influenced by differences in the wax contents on their leaves, according to our results (Table 3; Figure 9). The hydrophobicity of leaves, which is associated with the degree of particle retention, is determined by the hydrocarbon contents in the epicuticular waxes (Wang et al., 2013). High hydrophilicity can lead to the development of a water film over the leaf surface, which can indirectly affect PM adsorption by altering the microclimate around the leaves (Andrade et al., 2022).
Particle size is not the only determining factor for their affinity with specific leaf characteristics; their chemical properties also play a crucial role in this regard (Kah et al., 2019). The present study not only analyzed the correlation between leaf characteristics and particle size, but also classified them based on their relationship with both metal and non-metal elements present in fly ash (Figure 10). Our results showed that there were variations in the uptake of elements from fly ash particles by the two species (Figure 5). Aluminum (Al) had the highest concentration among the elements examined in the fly ash particles, accounting for the largest proportion at 58%, followed by iron (Fe) at 26%. The concentrations of magnesium (Mg), phosphorus (P), and sulfur (S) were the lowest among the elements examined of the total concentration of elements in the fly ash particles (Figure 5). The concentrations of elements in fly ash particles have the potential to influence the uptake of these elements by plant leaves. As a result, significant differences were observed in the uptake of Al between P. densiflora and Q. acutissima. The presence of major elements such as Al, Fe, Mg, S, and Si in fly particles generated by coal combustion processes represents a significant risk to human health (Bharathi et al., 2014). Aluminum mainly enters plant leaves through the cuticle in aqueous solutions (Zhang et al., 2010). Previous research demonstrated that airborne Al also can be taken up by leaves through cuticular penetration, as evidenced by the presence of Al within the leaves following exposure to acidic wetness (Vike, 2005). Positive association was observed between Al uptake and parameters related to foliar water uptake, such as transpiration and stomatal conductance, in the PCA analysis (Figure 10). Fe in fly ash particles is predominantly present as ionic states, which can also be emitted from sources such as vehicle exhaust, brake wear debris, and road dust (Gonet and Maher, 2019). Li et al. (2017) reported that sunflower and tomato plants exhibited different levels of foliar iron absorption, which were attributed to variations in the combined thickness of the cuticle and epidermal cell wall. In this study, we also observed the foliar uptake of Fe in both P. densiflora and Q. acutissima (Figure 5). Unlike the concentration of Al and Fe on leaves, which were observed in both species throughout the study, foliar uptake of Mg was only observed in Q. acutissima at 28 DAE. Although P. densiflora showed higher PM adsorption than Q. acutissima, no significant difference was found in P. densiflora between the control and treatment groups at either duration. The exposure to fly ash may not have affected the foliar uptake of Mg, given its high mobility within plants as a highly phloem-mobile cation that can be translocated to sites with high nutrient demand (Jezek et al., 2015). While the concentration of P and S in fly ash showed no significant difference, there was a marked variation in the foliar uptake of these elements by leaves of P. densiflora and Q. acutissima at both 14 and 28 DAE. Unlike this study, numerous studies have provided evidence that leaves can absorb the macronutrient phosphate (P) (Eichert et al., 2008; Fernández et al., 2014; Görlach and Mühling, 2021). The effectiveness of foliar phosphorus (P) absorption has been reported to vary across different physiological conditions in trees. For instance, under P-deficient conditions, the reduced transpiration rates in leaves could be attributed to stomatal closure, thereby limiting the penetration of leaf-applied P solutions through stomata (Bindraban et al., 2020). Previous studies have demonstrated that particulate matter can negatively affect the photosynthetic apparatus in plants treated with different compounds (Lee et al., 2022), which can subsequently impact the efficiency of foliar phosphorus (P) absorption. Investigating the elemental composition of particles on leaves provides valuable insights into pollution sources and can inform effective monitoring and mitigation strategies (Deng et al., 2018). However, as species-specific differences in elements from PM of foliar uptake may exist, it is necessary to conduct further research to ensure accurate and comprehensive monitoring of air pollution. The results of our study provide insights into the effects of leaf characteristics on the ability of plants to capture PM by comparing two functionally distinct tree species. However, further investigations are necessary to fully understand the impacts that the leaf characteristics of different species have on PM adsorption. This could involve examining anatomical, physiological, and chemical changes in the plants, as well as conducting studies under varying environmental conditions to better understand the factors that influence PM adsorption.
This study investigated the factors that affect PM adsorption on plant leaves, with a focus on the leaf characteristics of two tree species, P. densiflora and Q. acutissima. The results showed that PM adsorption by leaves is influenced by a combination of micro-structural characteristics and physiological characteristics such as transpiration and leaf boundary layer conductance. Stomatal size, leaf roughness, and wax content were found to be important leaf characteristics in determining PM10 adsorption. Physiological characteristics, including transpiration and leaf boundary layer conductance, were identified as important factors affecting the adsorption of PM2.5 on plant leaves. The study also examined the uptake of metal and non-metal elements from fly ash particles by the two tree species, with significant variations observed in the uptake of aluminum, iron, magnesium, phosphorus, and sulfur. The findings suggest that plant species-specific differences in elements from PM of foliar uptake may exist, which highlights the need for further research to ensure accurate and comprehensive monitoring of air pollution. Overall, this study provides valuable insights into the impacts of leaf characteristics on PM adsorption by comparing two functionally distinct tree species and highlights the need for further investigations to fully understand the complex factors that influence PM adsorption by plant leaves.
The original contributions presented in the study are included in the article/Supplementary material, further inquiries can be directed to the corresponding author.
JL: Data curation, Methodology, Visualization, Writing – original draft. MK: Formal analysis, Visualization, Writing – review & editing. SW: Funding acquisition, Project administration, Supervision, Writing – review & editing.
The author(s) declare financial support was received for the research, authorship, and/or publication of this article. This research was funded by Basic Science Research Program through the National Research Foundation of Korea (NRF), grant number 2021R1A2C2013068.
The authors declare that the research was conducted in the absence of any commercial or financial relationships that could be construed as a potential conflict of interest.
All claims expressed in this article are solely those of the authors and do not necessarily represent those of their affiliated organizations, or those of the publisher, the editors and the reviewers. Any product that may be evaluated in this article, or claim that may be made by its manufacturer, is not guaranteed or endorsed by the publisher.
The Supplementary material for this article can be found online at: https://www.frontiersin.org/articles/10.3389/ffgc.2023.1301533/full#supplementary-material
Akinyemi, S. A., Gitari, W. M., Petrik, L. F., Nyakuma, B. B., Hower, J. C., Ward, C. R., et al. (2019). Environmental evaluation and nano-mineralogical study of fresh and unsaturated weathered coal fly ashes. Sci. Total Environ. 663, 177–188. doi: 10.1016/J.SCITOTENV.2019.01.308
An, Z., Huang, R. J., Zhang, R., Tie, X., Li, G., Cao, J., et al. (2019). Severe haze in northern China: a synergy of anthropogenic emissions and atmospheric processes. Proc. Natl. Acad. Sci. U. S. A. 116, 8657–8666. doi: 10.1073/pnas.1900125116
Andrade, G. C., Santana, B. V. N., Rinaldi, M. C. S., Ferreira, S. O., Silva, R. C.Da, and Silva, L. C. D. (2022). Leaf surface traits related to differential particle adsorption – a case study of two tropical legumes. Sci. Total Environ. 823,:153681. doi: 10.1016/J.SCITOTENV.2022.153681
Avellan, A., Yun, J., Morais, B. P., Clement, E. T., Rodrigues, S. M., and Lowry, G. V. (2021). Critical review: role of inorganic nanoparticle properties on their foliar uptake and in planta translocation. Environ. Sci. Technol. 55, 13417–13431. doi: 10.1021/ACS.EST.1C00178
Avellan, A., Yun, J., Zhang, Y., Spielman-Sun, E., Unrine, J. M., Thieme, J., et al. (2019). Nanoparticle size and coating chemistry control foliar uptake pathways, translocation, and leaf-to-rhizosphere transport in wheat. ACS Nano 13, 5291–5305. doi: 10.1021/ACSNANO.8B09781/ASSET/IMAGES/LARGE/NN-2018-09781X_0008.JPEG
Barthlott, W., Mail, M., Bhushan, B., and Koch, K. (2017). Plant surfaces: structures and functions for biomimetic innovations. Nanomicro. Lett. 9, 1–40. doi: 10.1007/S40820-016-0125-1
Barwise, Y., and Kumar, P. (2020). Designing vegetation barriers for urban air pollution abatement: a practical review for appropriate plant species selection. NPJ Clim. Atmos. Sci. 3, 1–19. doi: 10.1038/s41612-020-0115-3
Beckett, K. P., Freer-Smith, P. H., and Taylor, G. (1998). Urban woodlands: their role in reducing the effects of particulate pollution. Environ. Pollut. 99, 347–360. doi: 10.1016/S0269-7491(98)00016-5
Bharathi, S., Villianur, I. H. I., Pachaiappan, R., Manish, B., Abinaya, B., and Devasena, T. (2014). Coal fly ash nanoparticles induced cytotoxicity and oxidative DNA damage and apoptosis in Chang liver cells. Afr. J. Pharm. Pharmacol 8, 801–808. doi: 10.5897/AJPP2014.4088
Bi, H., Kovalchuk, N., Langridge, P., Tricker, P. J., Lopato, S., and Borisjuk, N. (2017). The impact of drought on wheat leaf cuticle properties. BMC Plant Biol. 17, 1–13. doi: 10.1186/S12870-017-1033-3/FIGURES/8
Bindraban, P. S., Dimkpa, C. O., and Pandey, R. (2020). Exploring phosphorus fertilizers and fertilization strategies for improved human and environmental health. Biol. Fertil. Soils 56, 299–317. doi: 10.1007/S00374-019-01430-2/FIGURES/4
Brewer, C. A., Smith, W. K., and Vogelmann, T. C. (1991). Functional interaction between leaf trichomes, leaf wettability and the optical properties of water droplets. Plant Cell Environ. 14, 955–962. doi: 10.1111/J.1365-3040.1991.TB00965.X
Burkhardt, J. (2010). Hygroscopic particles on leaves: nutrients or desiccants? Ecol. Monogr. 80, 369–399. doi: 10.1890/09-1988.1
Büssis, D., Groll, U., Fisahn, J., and Altmann, T. (2006). Stomatal aperture can compensate altered stomatal density in Arabidopsis thaliana at growth light conditions. Funct. Plant Biol. 33, 1037–1043. doi: 10.1071/FP06078
Carignato, A., Vázquez-Piqué, J., Tapias, R., Ruiz, F., and Fernández, M. (2019). Variability and plasticity in cuticular transpiration and leaf permeability allow differentiation of Eucalyptus clones at an early age. Forests 11:9. doi: 10.3390/F11010009
Castanheiro, A., Hofman, J., Nuyts, G., Joosen, S., Spassov, S., Blust, R., et al. (2020). Leaf accumulation of atmospheric dust: biomagnetic, morphological and elemental evaluation using SEM, ED-XRF and HR-ICP-MS. Atmos. Environ. 221:117082. doi: 10.1016/J.ATMOSENV.2019.117082
Cayuela, C., Levia, D. F., Latron, J., and Llorens, P. (2019). Particulate matter fluxes in a mediterranean mountain forest: interspecific differences between throughfall and stemflow in oak and oine stands. J. Geophys. Res. Atmos. 124, 5106–5116. doi: 10.1029/2019JD030276
Chaudhary, I. J., and Rathore, D. (2018). Suspended particulate matter deposition and its impact on urban trees. Atmos. Pollut. Res. 9, 1072–1082. doi: 10.1016/J.APR.2018.04.006
Corada, K., Woodward, H., Alaraj, H., Collins, C. M., and de Nazelle, A. (2021). A systematic review of the leaf traits considered to contribute to removal of airborne particulate matter pollution in urban areas. Environ. Pollut. 269:116104. doi: 10.1016/J.ENVPOL.2020.116104
Deng, J., Zhang, Y., Qiu, Y., Zhang, H., Du, W., Xu, L., et al. (2018). Source apportionment of PM2.5 at the Lin’an regional background site in China with three receptor models. Atmos. Res. 202, 23–32. doi: 10.1016/J.ATMOSRES.2017.11.017
Dodman, D., Hayward, B., Pelling, M., Castan Broto, V., Chow, W., Chu, E., et al. (2022). “Cities, settlements and key infrastructure” in Climate change 2022: Impacts, adaptation, and vulnerability. Contribution of working group II to the sixth assessment report of the intergovernmental panel on climate change, IPPCC (Cambridge, UK and New York, NY, USA: Cambridge University Press), 907–1040.
Du, Y., Kopittke, P. M., Noller, B. N., James, S. A., Harris, H. H., Xu, Z. P., et al. (2015). In situ analysis of foliar zinc absorption and short-distance movement in fresh and hydrated leaves of tomato and citrus using synchrotron-based X-ray fluorescence microscopy. Ann. Bot. 115, 41–53. doi: 10.1093/AOB/MCU212
Dzierżanowski, K., Popek, R., Gawrońska, H., Sæbø, A., and Gawroński, S. W. (2011). Deposition of particulate matter of different size fractions on leaf surfaces and in waxes of urban forest species. Int. J. Phytoremediation 13, 1037–1046. doi: 10.1080/15226514.2011.552929
Ebercon, A., Blum, A., and Jordan, W. R. (1977). A rapid colorimetric method for epicuticular wax contest of Sorghum leaves. Crop Sci. 17, 179–180. doi: 10.2135/CROPSCI1977.0011183X001700010047X
Eichert, T., Kurtz, A., Steiner, U., and Goldbach, H. E. (2008). Size exclusion limits and lateral heterogeneity of the stomatal foliar uptake pathway for aqueous solutes and water-suspended nanoparticles. Physiol. Plant. 134, 151–160. doi: 10.1111/J.1399-3054.2008.01135.X
Esposito, F., Memoli, V., Panico, S. C., Di Natale, G., Trifuoggi, M., Giarra, A., et al. (2020). Leaf traits of Quercus ilex L. affect particulate matter accumulation. Urban For. Urban Green. 54:126780. doi: 10.1016/J.UFUG.2020.126780
Farquhar, G. D., von Caemmerer, S., and Berry, J. A. (1980). A biochemical model of photosynthetic CO2 assimilation in leaves of C3 species. Planta 149, 78–90. doi: 10.1007/BF00386231/METRICS
Fernández, V., Gil-Pelegrín, E., and Eichert, T. (2021). Foliar water and solute absorption: an update. Plant J. 105, 870–883. doi: 10.1111/TPJ.15090
Fernández, V., Guzmán, P., Peirce, C. A. E., McBeath, T. M., Khayet, M., and McLaughlin, M. J. (2014). Effect of wheat phosphorus status on leaf surface properties and permeability to foliar-applied phosphorus. Plant Soil 384, 7–20. doi: 10.1007/S11104-014-2052-6/TABLES/4
Gilliam, J. H., and Hall, E. S. (2016). Reference and equivalent methods used to measure National Ambient air Quality Standards (NAAQS) criteria air pollutants-volume I. Washington, DC, USA: US. Environmental Protection Agency.
Gonet, T., and Maher, B. A. (2019). Airborne, vehicle-derived Fe-bearing nanoparticles in the urban environment: a review. Environ. Sci. Technol. 53, 9970–9991. doi: 10.1021/ACS.EST.9B01505/ASSET/IMAGES/MEDIUM/ES9B01505_M001.GIF
Good, R. J. (1992). Contact angle, wetting, and adhesion: a critical review. J. Adhes. Sci. Technol. 6, 1269–1302. doi: 10.1163/156856192X00629
Görlach, B. M., and Mühling, K. H. (2021). Phosphate foliar application increases biomass and P concentration in P deficient maize. J. Plant Nutr. Soil Sci. 184, 360–370. doi: 10.1002/JPLN.202000460
Grantz, D. A., Garner, J. H. B., and Johnson, D. W. (2003). Ecological effects of particulate matter. Environ. Int. 29, 213–239. doi: 10.1016/S0160-4120(02)00181-2
Han, D., Shen, H., Duan, W., and Chen, L. (2020). A review on particulate matter removal capacity by urban forests at different scales. Urban For. Urban Green. 48:126565. doi: 10.1016/J.UFUG.2019.126565
Hofman, J., Bartholomeus, H., Calders, K., Van Wittenberghe, S., Wuyts, K., and Samson, R. (2014). On the relation between tree crown morphology and particulate matter deposition on urban tree leaves: a ground-based LiDAR approach. Atmos. Environ. 99, 130–139. doi: 10.1016/J.ATMOSENV.2014.09.031
Huggins, T. D., Mohammed, S., Sengodon, P., Ibrahim, A. M. H., Tilley, M., and Hays, D. B. (2018). Changes in leaf epicuticular wax load and its effect on leaf temperature and physiological traits in wheat cultivars (Triticum aestivum L.) exposed to high temperatures during anthesis. J. Agron. Crop Sci. 204, 49–61. doi: 10.1111/JAC.12227
Jańczuk, B., Białopiotrowicz, T., and Wójcik, W. (1989). The components of surface tension of liquids and their usefulness in determinations of surface free energy of solids. J. Colloid Interface Sci. 127, 59–66. doi: 10.1016/0021-9797(89)90007-6
Janhäll, S. (2015). Review on urban vegetation and particle air pollution – deposition and dispersion. Atmos. Environ. 105, 130–137. doi: 10.1016/J.ATMOSENV.2015.01.052
Jezek, M., Geilfus, C. M., Bayer, A., and Muhling, K. H. (2015). Photosynthetic capacity, nutrient status, and growth of maize (Zea mays L.) upon MgSO4 leaf-application. Front. Plant Sci. 5:781. doi: 10.3389/FPLS.2014.00781/BIBTEX
Kah, M., Navarro, D., Kookana, R. S., Kirby, J. K., Santra, S., Ozcan, A., et al. (2019). Impact of (nano)formulations on the distribution and wash-off of copper pesticides and fertilisers applied on citrus leaves. Environ. Chem. 16, 401–410. doi: 10.1071/EN18279
Korea Ministry of Government Legislation. (2019). Available at: https://www.moleg.go.kr (Accessed May 1, 2023).
Kwak, M. J., Lee, J. K., Park, S., Kim, H., Lim, Y. J., Lee, K. A., et al. (2020). Surface-based analysis of leaf microstructures for adsorbing and retaining capability of airborne particulate matter in ten woody species. Forests 11:946. doi: 10.3390/F11090946
Kwak, M. J., Lee, J., Park, S., Lim, Y. J., Kim, H., Jeong, S. G., et al. (2023). Understanding particulate matter retention and wash-off during rainfall in relation to leaf traits of urban forest tree species. Horticulturae 9:165. doi: 10.3390/HORTICULTURAE9020165/S1
Kweon, D., and Comeau, P. G. (2021). Climate, site conditions, and stand characteristics influence maximum size-density relationships in Korean red pine (Pinus densiflora) and Mongolian oak (Quercus mongolica) stands, South Korea. For Ecol Manage 502:119727. doi: 10.1016/J.FORECO.2021.119727
Lee, J. K., Kim, D. Y., Park, S. H., Woo, S. Y., Nie, H., and Kim, S. H. (2022). Particulate matter (PM) adsorption and leaf characteristics of ornamental sweet potato (Ipomoea batatas L.) cultivars and two common indoor plants (Hedera helix L. and Epipremnum aureum Lindl. & Andre). Horticulturae 8:26. doi: 10.3390/HORTICULTURAE8010026
Li, J., Li, X., Wang, W., Wang, X., Lu, S., Sun, J., et al. (2021). Investigation on removal effects and condensation characteristics of condensable particulate matter: field test and experimental study. Sci. Total Environ. 783:146985. doi: 10.1016/J.SCITOTENV.2021.146985
Li, C., Wang, P., Menzies, N. W., Lombi, E., and Kopittke, P. M. (2017). Effects of changes in leaf properties mediated by methyl jasmonate (MeJA) on foliar absorption of Zn, Mn and Fe. Ann. Bot. 120, 405–415. doi: 10.1093/AOB/MCX063
Li, X., Zhang, T., Sun, F., Song, X., Zhang, Y., Huang, F., et al. (2021). The relationship between particulate matter retention capacity and leaf surface micromorphology of ten tree species in Hangzhou, China. Sci Total Environ 771:144812. doi: 10.1016/J.SCITOTENV.2020.144812
Liang, D., Ma, C., Wang, Y., Wang, Y., and Chen-xi, Z. (2016). Quantifying PM2.5 capture capability of greening trees based on leaf factors analyzing. Environ. Sci. Pollut. Res. 23, 21176–21186. doi: 10.1007/S11356-016-7687-9/FIGURES/8
Liu, J., Cao, Z., Zou, S., Liu, H., Hai, X., Wang, S., et al. (2018). An investigation of the leaf retention capacity, efficiency and mechanism for atmospheric particulate matter of five greening tree species in Beijing, China. Sci. Total Environ. 616-617, 417–426. doi: 10.1016/J.SCITOTENV.2017.10.314
Mayeux, H. S., and Jordan, W. R. (1984). Variation in amounts of epicuticular wax on leaves of Prosopis glandulosa. Bot. Gaz. 145, 26–32. doi: 10.1086/337421
National Institute of Environmental Research (2021). Annual report of air quality in Korea 2020. Incheon, Korea: National Institute of Environmental Research.
Niu, X., Wang, B., and Wei, W. (2020). Response of the particulate matter capture ability to leaf age and pollution intensity. Environ. Sci. Pollut. Res. 27, 34258–34269. doi: 10.1007/S11356-020-09603-5/FIGURES/9
Parkinson, K. J. (1985). A simple method for determining the boundary layer resistance in leaf cuvettes. Plant Cell Environ. 8, 223–226. doi: 10.1111/1365-3040.EP11604618
Pequerul, A., Pérez, C., Madero, P., Val, J., and Monge, E. (1993). “A rapid wet digestion method for plant analysis” in Optimization of plant nutrition, eds. M. A. C. Fragoso, M. L. Beusichem, and A. Huwers (Dordrecht, Netherlands: Springer), 3–6.
Perini, K., Ottelé, M., Giulini, S., Magliocco, A., and Roccotiello, E. (2017). Quantification of fine dust deposition on different plant species in a vertical greening system. Ecol. Eng. 100, 268–276. doi: 10.1016/J.ECOLENG.2016.12.032
Przybysz, A., Sæbø, A., Hanslin, H. M., and Gawroński, S. W. (2014). Accumulation of particulate matter and trace elements on vegetation as affected by pollution level, rainfall and the passage of time. Sci. Total Environ. 481, 360–369. doi: 10.1016/J.SCITOTENV.2014.02.072
Read, T. L., Doolette, C. L., Li, C., Schjoerring, J. K., Kopittke, P. M., Donner, E., et al. (2020). Optimising the foliar uptake of zinc oxide nanoparticles: do leaf surface properties and particle coating affect absorption? Physiol. Plant. 170, 384–397. doi: 10.1111/PPL.13167
Sæbø, A., Popek, R., Nawrot, B., Hanslin, H. M., Gawronska, H., and Gawronski, S. W. (2012). Plant species differences in particulate matter accumulation on leaf surfaces. Sci. Total Environ. 427-428, 347–354. doi: 10.1016/J.SCITOTENV.2012.03.084
Sepahvand, S., Jonoobi, M., Ashori, A., Rabie, D., Gauvin, F., Brouwers, H. J. H., et al. (2022). Modified cellulose nanofibers aerogels as a novel air filters; synthesis and performance evaluation. Int. J. Biol. Macromol. 203, 601–609. doi: 10.1016/J.IJBIOMAC.2022.01.156
Sharma, P., and Saxena, P. (2022). “Impacts and responses of particulate matter pollution on vegetation” in Airborne particulate matter: Source, chemistry and health, eds. S. Sonwani and A. Shukla (Singapore: Springer Nature), 229–264.
Song, Y., Maher, B. A., Li, F., Wang, X., Sun, X., and Zhang, H. (2015). Particulate matter deposited on leaf of five evergreen species in Beijing, China: source identification and size distribution. Atmos. Environ. 105, 53–60. doi: 10.1016/J.ATMOSENV.2015.01.032
Sonwani, S., Hussain, S., and Saxena, P. (2022). Air pollution and climate change impact on forest ecosystems in Asian region – a review. Ecosyst. Health Sustain. 8:2090448. doi: 10.1080/20964129.2022.2090448
Tian, L., Yin, S., Ma, Y., Kang, H., Zhang, X., Tan, H., et al. (2019). Impact factor assessment of the uptake and accumulation of polycyclic aromatic hydrocarbons by plant leaves: morphological characteristics have the greatest impact. Sci. Total Environ. 652, 1149–1155. doi: 10.1016/J.SCITOTENV.2018.10.357
Tomson, M., Kumar, P., Barwise, Y., Perez, P., Forehead, H., French, K., et al. (2021). Green infrastructure for air quality improvement in street canyons. Environ. Int. 146:106288. doi: 10.1016/J.ENVINT.2020.106288
Uscola, M., Villar-Salvador, P., Oliet, J., and Warren, C. R. (2014). Foliar absorption and root translocation of nitrogen from different chemical forms in seedlings of two Mediterranean trees. Environ. Exp. Bot. 104, 34–43. doi: 10.1016/J.ENVEXPBOT.2014.03.004
Vargas Buonfiglio, L. G., Mudunkotuwa, I. A., Abou Alaiwa, M. H., Vanegas Calderón, O. G., Borcherding, J. A., Gerke, A. K., et al. (2017). Effects of coal fly ash particulate matter on the antimicrobial activity of airway surface liquid. Environ. Health Perspect. 125:077003. doi: 10.1289/EHP876
Vike, E. (2005). Uptake, deposition and wash off of fluoride and aluminium in plant foliage in the vicinity of an aluminium smelter in Norway. Water Air Soil Pollut. 160, 145–159. doi: 10.1007/S11270-005-3862-1/METRICS
Wang, H., Shi, H., Li, Y., Yu, Y., and Zhang, J. (2013). Seasonal variations in leaf capturing of particulate matter, surface wettability and micromorphology in urban tree species. Front. Environ. Sci. Eng. 7, 579–588. doi: 10.1007/S11783-013-0524-1/METRICS
Wilson, P. J., Thompson, K., and Hodgson, J. G. (1999). Specific leaf area and leaf dry matter content as alternative predictors of plant strategies. New Phytol. 143, 155–162. doi: 10.1046/J.1469-8137.1999.00427.X
Wu, Y., Liu, J., Zhai, J., Cong, L., Wang, Y., Ma, W., et al. (2018). Comparison of dry and wet deposition of particulate matter in near-surface waters during summer. PLoS One 13:e0199241. doi: 10.1371/JOURNAL.PONE.0199241
Yin, S., Lyu, J., Zhang, X., Han, Y., Zhu, Y., Sun, N., et al. (2020). Coagulation effect of aero submicron particles on plant leaves: measuring methods and potential mechanisms. Environ. Pollut. 257:113611. doi: 10.1016/J.ENVPOL.2019.113611
Zanobetti, A., Austin, E., Coull, B. A., Schwartz, J., and Koutrakis, P. (2014). Health effects of multi-pollutant profiles. Environ. Int. 71, 13–19. doi: 10.1016/J.ENVINT.2014.05.023
Zhang, Z., Gong, J., Li, Y., Zhang, W., Zhang, T., Meng, H., et al. (2022). Analysis of the influencing factors of atmospheric particulate matter accumulation on coniferous species: measurement methods, pollution level, and leaf traits. Environ. Sci. Pollut. Res. 29, 62299–62311. doi: 10.1007/S11356-022-20067-7/FIGURES/8
Zhang, R., Khalizov, A., Wang, L., Hu, M., and Xu, W. (2012). Nucleation and growth of nanoparticles in the atmosphere. Chem. Rev. 112, 1957–2011. doi: 10.1021/cr2001756
Zhang, Z., Li, Y., Li, M., Meng, H., Zhang, T., Peng, Z., et al. (2023). Improving atmospheric particulate matter removal of residential green space based on landscape patterns and plant functional types. Air Qual. Atmos. Health 16, 401–413. doi: 10.1007/S11869-022-01281-1/FIGURES/6
Zhang, R., Wang, G., Guo, S., Zamora, M. L., Ying, Q., Lin, Y., et al. (2015). Formation of urban fine particulate matter. Chem. Rev. 115, 3803–3855. doi: 10.1021/acs.chemrev.5b00067
Zhang, B., Wang, X. Q., Li, X., Ni, Y. Q., and Li, H. (2010). Aluminum uptake and disease resistance in Nicotiana rustica leaves. Ecotoxicol. Environ. Saf. 73, 655–663. doi: 10.1016/J.ECOENV.2009.12.028
Keywords: gas exchange characteristics, metal/non-metal elements, Pinus densiflora , Particulate matter, PM adsorption, Quercus acutissima
Citation: Lee J, Kwak MJ and Woo SY (2024) Adsorption of particulate matter and uptake of metal and non-metal elements from PM in leaves of Pinus densiflora and Quercus acutissima: a comparative study. Front. For. Glob. Change. 6:1301533. doi: 10.3389/ffgc.2023.1301533
Received: 25 September 2023; Accepted: 12 December 2023;
Published: 05 January 2024.
Edited by:
Yasutomo Hoshika, Consiglio Nazionale delle Ricerche (CNR), ItalyCopyright © 2024 Lee, Kwak and Woo. This is an open-access article distributed under the terms of the Creative Commons Attribution License (CC BY). The use, distribution or reproduction in other forums is permitted, provided the original author(s) and the copyright owner(s) are credited and that the original publication in this journal is cited, in accordance with accepted academic practice. No use, distribution or reproduction is permitted which does not comply with these terms.
*Correspondence: Su Young Woo, d3N5QHVvcy5hYy5rcg==
Disclaimer: All claims expressed in this article are solely those of the authors and do not necessarily represent those of their affiliated organizations, or those of the publisher, the editors and the reviewers. Any product that may be evaluated in this article or claim that may be made by its manufacturer is not guaranteed or endorsed by the publisher.
Research integrity at Frontiers
Learn more about the work of our research integrity team to safeguard the quality of each article we publish.