- Department of Geography, University of Zurich Winterthurerstrasse, Zürich, Switzerland
Afforestation has been the dominant land-use change in the Swiss Alps during the last decades which has not only the potential to increase soil organic carbon sequestration, but it has also the potential to alter soil organic matter (SOM) dynamics through the vegetation shift and change in organic matter (OM) input into soils. The effects of afforestation on SOM dynamics, however, are still not fully understood as specific sources of OM and modifications of soil processes influencing decomposition and preservation remain largely unknown on alpine to subalpine slopes. Within this study we aimed to identify the potential sources and the decomposition of OM in a subalpine afforestation chrono-sequence (0–130 years) with Norway spruce (Picea abies L.) on a former pasture by using a multi-proxy molecular marker approach. We observed that leaf-derived OM plays an essential role in the pasture areas, while root-derived OM only plays a minor role in pasture and forest areas. Needle-derived OM represents the dominant source of SOM with increasing forest age, while understory shrubs and moss also contribute to the OM input in younger forest stand ages. However, needle litter and buildup of organic layers and subsequently less input of fresh OM from organic horizons to mineral soil can result in increased OM decomposition in mineral soils rather than contributing to additional SOM stabilization in mineral soils. This was most pronounced in the oldest forest stand (130-year-old) in the investigated afforestation sequence, particularly in deeper soil horizons (10–45 cm). Thereby, our study provides new insights into SOM dynamics following afforestation, especially with respect to the long-term SOM sequestration potential of afforestation of subalpine pasture soils.
1 Introduction
In forests, soil store approximately 44% of their carbon (C) in the soil (1 m depth), 42% in the living biomass, and 14% in the litter pad (Pan et al., 2011). On a global scale, temperate forest ecosystems account for 14% of the current C sink (Pan et al., 2011). In Switzerland, currently 30% of the total land area is covered by forests (Gehrig-Fasel et al., 2007) of which 60% are located in alpine ecosystems (Brändli, 2010). Specifically in alpine settings, forest expansion is likely to continue during the next decades (Bolliger et al., 2008), because of abandonment of meadows and treeline upward movement. Afforestation is also a well-known and promising strategy to increase carbon sequestration and is therefore highly encouraged to compensate for CO2 emissions (Strand et al., 2021). While the increase in the aboveground biomass following afforestation is well documented (Lal, 2005; Thuille and Schulze, 2006; Risch et al., 2008) the effects of afforestation on the belowground biomass and carbon stocks in soils vary from sink to source, depending on climate conditions, tree species, and forest age (Paul et al., 2002). It therefore remains an open question to which extend an increased C stock in the aboveground biomass can coincide with increased C sequestration belowground and soils, as soils may have finite capacities to store additional C (Lajtha and Bowden, 2014).
Soil organic carbon (SOC) is typically considered to originate mainly from plant biomass (Kögel-Knabner, 2002) and either derives from above- or belowground plant biomass (Angst et al., 2016). The quality as well as the quantity of plant litter input is directly linked to the composition of soil organic matter (SOM) in forest soils (Crow et al., 2009a) and is assumed to drive its turnover and stabilization (Kögel-Knabner, 2002; Chabbi et al., 2009). Hence, plant residues play an important role in SOC accumulation in forest soils (Dai et al., 2022). Twigs, leaves, needles, and seeds are typical aboveground sources of SOM in forests (Nadelhoffer et al., 2004) and with ongoing time, they are incorporated in the organic horizons, where they are processed and finally translocated into the mineral soil either as dissolved OM (Kalbitz et al., 2000) or as particulate matter via the soil fauna (Pulleman et al., 2005). This plant debris is the principal source for the formation of SOM in the mineral topsoil (Kögel-Knabner, 2002). Root-derived litter, including root exudates (Nadelhoffer et al., 2004; Dennis et al., 2010) and particulate OM, are belowground sources of SOM and are directly supplied within subsoil horizons (Angst et al., 2016), which becomes more relevant with increasing soil depth. The different origin (above- vs. belowground plant biomass) of SOM not only results in a different SOM composition, but it can also result in SOM components that differ in their stability (Pisani et al., 2016). This highlights the importance of knowing the origin of SOM, as this determines the fate of plant-derived carbon in soil (Angst et al., 2016). To date, specifically in alpine and subalpine soils, knowledge with respect to the proportion of aboveground- and root-derived carbon and how this is incorporated into the soil and may contribute to stable SOM (Guidi et al., 2023) is still limited.
One approach to identify different plant-derived sources of SOM is the analysis of solvent-extractable lipids (Naafs et al., 2004) as the molecular composition varies between above- and belowground plant biomass (Kögel-Knabner, 2002) and different plant species (Teunissen van Manen et al., 2020). Solvent-extractable lipids are a heterogeneous group of compounds and include, e.g., n-fatty acids, n-alkanes, and n-alcohols (Jansen and Wiesenberg, 2017). Especially aliphatic straight-chain lipids with a chain-length in the range of C20 to C36 are indicative for leaf waxes of terrestrial higher plants (Eglinton and Hamilton, 1967; Jansen and Wiesenberg, 2017). For example, n-C27 and n-C29 alkanes are typically interpreted as indicative for tree and shrub plants, whereas n-C31 and n-C33 alkanes are often more abundant in grass and herb plants (Jansen et al., 2006; Schäfer et al., 2016). As these plant-derived lipids account for a major part of SOM, they can be used as a molecular proxy for tracing sources of plant-derived organic matter (OM) in soils (Jansen and Wiesenberg, 2017). Compounds originating from leaf waxes as well as from roots are typically characterized by an odd-over-even carbon predominance of n-alkanes and an even-over-odd carbon predominance of the other component class, such as n-alcohols and n-fatty acids (Jansen et al., 2006). Leaf waxes are considered as the dominant source of solvent-extractable lipids in the topsoil (Angst et al., 2016; Jansen and Wiesenberg, 2017), whereas it is still an open question whether root-derived carbon or carbon from aboveground biomass that is translocated vertically through the profile is the dominant source of carbon in the subsoil (Kalbitz et al., 2000; Rasse et al., 2005).
The current study aims to investigate whether OM input or decomposition is more important in soils of an afforestation sequence on a former pasture – 0 to 130-years – with Norway spruce (Picea abies L.) in a subalpine setting at Jaun (Switzerland; Hiltbrunner et al., 2013; Speckert et al., 2023). A major objective was to determine the potential sources of OM (above- vs. belowground plant litter) and how the sources vary with increasing soil depth and from pasture to forest with increasing forest age. With increasing soil depth, we expect more fine root-derived OM as the predominant source, especially in the pasture area, as grass species can have a more profound rooting system (Hodge et al., 2009) than coniferous trees. With increasing forest age, we expect more likely to find needle-derived OM in the forest soil due to the low substrate quality of spruce litter which favors its accumulation, especially in the organic horizons (Poeplau and Don, 2013). As coniferous trees are characterized by shallow rooting systems (Puhe, 2003) that consist mainly of woody roots which are thicker than the roots of the pasture vegetation, we expect less contribution of root-derived OM in the forest than in the pasture soil. The second objective is to assess the decomposition of OM with increasing soil depth and forest age. With increasing forest age, we expect less decomposition, specifically in organic horizons and surface mineral soils as the organic horizons in old forest stand ages are characterized by higher C:N ratios compared to younger forest stand ages (Speckert et al., 2023). Furthermore, a decrease in pH with increasing forest age (Hiltbrunner et al., 2013) hampers decomposition of OM in soils. To investigate the potential shifts in the OM composition as well as its decomposition, we analyzed long-chain n-fatty acids, n-alkanes, and n-alcohols and derived molecular markers, e.g., average chain length (ACL) and carbon preference index (CPI) of straight chain lipids, which carry information regarding source and decomposition of plant-derived OM in soils (Wiesenberg et al., 2010; Angst et al., 2016).
2 Materials and methods
2.1 Description of the study site
The study site is located in Jaun, in the Canton of Fribourg, Switzerland [7°15′54 E, 46°37′17 N]. The afforestation sequence is located on a south-exposed slope between 1,450 and 1,600 m above sea level. The mean air temperature is 11.4°C during summer and 0.6°C during winter with a mean annual precipitation of 1,250 mm (Hiltbrunner et al., 2013). Between 1954 and 1968, several severe snow avalanches occurred around the village of Jaun. To protect the village against future avalanches, the pasture located above the village was gradually afforested with Norway spruce (Picea abies L.). The oldest forest stand represents an area that was forested before 130 years with the age being verified by aerial photographs, historical maps and counting of tree rings. The plant community on pasture soils mainly consists of grass species with ribgrass (Plantago lanceolata L.) and reed fescue (Festuca arundinacea Schreb.). In the forest, Norway spruce (Picea abies L.) was the dominant species in all forest stand ages. In our study, we focused on four different forest ages in the afforestation sequence: Pasture (0-year-old forest) as control and forest stand ages of 40 years, 55 years, and at least 130 years. Our field design represents a space-for-time approach with a study site with presumably homogeneous and comparable soil properties, slope, and exposition among the different forest stand ages, which allows to investigate changes in the SOM composition over several decades.
2.2 Sampling and sample preparation
The sampling campaign was conducted in July 2020. For each forest stand age, five individual plots were placed in a line over the entire area of the respective forest age and pasture, respectively (see Speckert et al., 2023). Spruce needles and moss samples were collected in forests of all stand ages [n = 3 (n = number of replicates)] and most dominant grass species (F. arundinacea and P. lanceolata) were collected (n = 1) in the pasture area. Material of organic horizons (O-horizons) was collected on three plots in the 40-year-old, the 55-year-old, and the 130-year-old forest stands (n = 2). The samples of the O-horizons were separated into Oi (slightly decomposed organic material), Oe (moderately decomposed organic material), and Oa (highly decomposed organic material; Jahn et al., 2006). Five soil pits in the pasture area (0-year-old) as well as three soil pits for each forest stand age (40-, 55-, and 130-year-old) were prepared with dimensions of at least 100 cm width x 50 cm depth. The mineral soil samples were taken with two volumetric steel cylinders (100 cm3) in these profiles on slope-parallel levels that were incrementally increased by 5 cm to a maximum depth of 45 cm (pasture n = 5; forest areas n = 3). All soil samples were stored in open plastic bags until their arrival in the laboratory, where they were stored at −20°C.
Mineral soil samples, plant samples, and samples of organic horizons were freeze-dried to constant weight. Soil samples were sieved through a 2 mm sieve. Roots were manually removed from the fine earth (<2 mm) and combined with the roots >2 mm. Root samples (0–5 mm) were afterwards washed with deionized water to remove attached soil particles, followed by drying at 40°C in an oven until constant weight. Dried samples of the mineral soil, aboveground plant tissues, roots, and organic horizons were ground in a ball mill (MM400, Retsch, Haan, Germany). A subsample of the milled and homogenized mineral soil was acidified with HCl to remove carbonates, washed with deionized water, and afterwards dried in the oven at 40°C (Volk et al., 2018).
2.3 Analysis of free extractable lipids
To identify potential sources of SOM as well as its quality, quantity, and decomposition, several lipid biomarkers (n-fatty acids, n-alkanes, n-alcohols) were analyzed according to Wiesenberg and Gocke (2017). The analysis of the lipid biomarkers was performed in duplicate (n = 2; field replicates) for all investigated samples with the exception of root samples in the 130-year-old forest due to a low amount of material. This means that we analyzed two spots each, for the pasture area and for each forest stand age. For mineral soil samples, 10 – 15 g were extracted. For spruce needles, moss, roots and organic horizons, 0.5 – 5 g were extracted. The root samples were combined into topsoil (0–5 cm, 5–10 cm) and subsoil roots (10–45 cm) for the following reasons: (1) To investigate the strongly rooted topsoil separately from the less rooted subsoil; (2) to achieve enough material for the analysis as less root material was available in the deeper soil horizons due to the parent soil material; and (3) to obtain a comparable and representative result between the two different land-use (pasture vs. forest) types. Extraction of free extractable lipids was performed using Soxhlet extraction with a mixture of dichloromethane:methanol (DCM:MeOH; 93:7, v/v). The total lipid extracts were evaporated until dryness to gravimetrically determine the total lipid concentration. Afterwards, the extracts were sequentially separated by solid phase extraction using KOH-coated silica gel (SiO2 60 Å, 5% KOH) into neutral lipid, fatty acid, and polar lipid fractions, respectively. The neutral lipid fractions were separated into n-alkanes, aromatic hydrocarbons and low polar heterocompound fractions by solid phase extraction using activated SiO2 (100 Å). Deuterated tetracosane (D50C24) was added as an internal standard to the n-alkane fraction prior to gas chromatographic (GC) analysis. An aliquot of the n-fatty acid fractions (1–2 mg) was split apart and deuterated eicosanoic acid (D39C20) was added as an internal standard for quantification. Thereafter, the fatty acids were derivatized to fatty acid methyl esters (FAME) using boron trifluoride:methanol (BF3:MeOH) prior to the GC analysis. Deuterated octadecanol (D37C18) was used as an internal standard in an aliquot (1–2 mg) of the low polar heterocompound fraction (containing n-alcohols) that was subsequently silylated with N,O-Bis(trimethylsilyl)-acetamide (BSA) before GC analysis.
2.4 Identification and quantification of individual lipids
The identification of the individual lipids was performed on an Agilent 6890 N GC equipped with split/splitless injector coupled to an Agilent 5973 mass selective detector (MS). Quantification was performed on a GC (Agilent 7890B) equipped with a multi-mode inlet (MMI) and flame ionization detector (FID). The identification of the individual lipids was done by comparison with mass spectra of external standards as well as with the NIST mass spectra library. Both GC instruments were equipped with a J&W DB-5MS narrow-bore capillary column (50 m × 0.2 mm, 0.33 μm film thickness) and a deactivated precolumn (1.5 m). Helium was used as carrier gas. For the n-alkane analysis, the GC oven temperature increased from 70°C (held for 4 min) to 320°C (held for 20 min) at a rate of 5°C min−1. The GC oven temperature for fatty acids and for the low-polar heterocompound fractions started at 50°C (held for 4 min) and increased to 150°C at a rate of 4°C min−1 and finally increased to 320°C (held for 40 min) at a rate of 3°C min−1. The temperature of the MMI started 10°C above the respective oven temperature, kept constant for 0.5 min and then ramped at 800°C/min to 400°C and kept isothermal for 2 min. Afterwards, MMI temperature was reduced to 250°C and remained constant until the end of the run.
2.5 Molecular proxies
To assess the source and degradation of the SOM, several molecular proxies, such as the average chain length (ACL) and the carbon preference index (CPI) were applied (Wiesenberg and Gocke, 2017). The ACL is widely used as a proxy for OM derived from higher terrestrial plants (Cranwell, 1973) and is characterized by longer chain-lengths than microorganism-derived OM (Wiesenberg et al., 2010). The ACL is the average carbon chain-length of selected n-fatty acids (C22 – C32), n-alkanes (C21 – C33), and n-alcohols (C22 – C32), respectively, and was calculated using the following equation according to Wiesenberg and Gocke (2017):
where n is the number of carbon atoms and represents the concentrations of the respective compounds.
The CPI has been applied for n-fatty acids, n-alkanes and n-alcohols and is used to determine the biological origin of OM (Herrera-Herrera et al., 2020). The CPI provides information about the even-over-odd (and odd-over-even) predominance of carbon chain lengths (Wiesenberg and Gocke, 2017). Fresh plant material is characterized by odd-over-even predominance for n-alkanes and even-over-odd dominance for n-fatty acids and n-alcohols. The CPI thus indicates, if SOM is consisting of fresh (CPI > 2) or degraded plant-derived OM (CPI close to 1; Angst et al., 2016). The CPI was calculated based on the following equation according to Wiesenberg and Gocke (2017) for n-fatty acids (CPIFA), n-alkanes (CPIALK), and for n-alcohols (CPIALC), respectively.
where Z represent the concentrations of the compounds with even or odd carbon chain lengths, respectively.
2.6 Statistics and calculation
Data analysis was performed using the R Software v.4.3.2 (R Core Team, 2020). Samples of the organic horizons, aboveground biomass, and root samples, as well as mineral soil samples were analyzed separately. To test, whether there is a significant difference in the lipid concentration (including all quantified n-fatty acids, n-alkanes, and n-alcohols) as well as in the molecular proxies (ACL and CPI) between land-use (pasture vs. forest) and between different forest ages (0 to 130-year-old) a three-way analysis of variance (ANOVA, p < 0.05) followed by a post-hoc Tukey HSD test (p adj < 0.95) was applied. The ACL and CPI values of mineral soils, organic horizons and root samples were given as average ± SE (standard error).
Principal component analysis (PCA) was performed using the FactoMineR package to determine the chemical composition of SOM and to identify potential SOM sources in relation to land-use (pasture vs. forest) and different forest ages (0 to 130-year-old). Given the strong even-over-odd carbon predominance of n-fatty acids and n-alcohols and the strong odd-over-even carbon dominance of n-alkanes (Jansen et al., 2006), only long-chain and even number n-fatty acids (C20 – C32) and n-alcohols (C22 – C32) and odd numbered n-alkanes (C21 – C33) were included in the PCA. The PCA comprised 529 data points for pasture, 782 data points for the 40-year-old, 736 data points for the 55-year-old, and 759 data points for the 130-year-old forest and 24 variables for all observed forest stand ages (0-130-years-old). All variables were standardized before PCA analysis.
For the correlation analysis the corrplot package was used to identify correlation within and between ACL and CPI values of the different lipid classes, i.e., n-fatty acids, n-alkanes, and n-alcohols. The correlation analysis was performed separately for topsoil (0–10 cm) and subsoil (10–45 cm) mineral soil samples to retrieve more detailed information regarding decomposition of OM. The correlation matrix included 24 data points for the topsoil, 72 data points for the subsoil in pasture, and 84 data points for the subsoil of each forest stand age.
3 Results
3.1 Lipid concentrations
3.1.1 Mineral soil horizons
In all investigated mineral soil samples, the n-fatty acid concentration was significantly higher (p = 0.01) compared to the n-alkane and n-alcohol concentrations (Figure 1A). The n-fatty acid concentration was highest in the topsoil (0 – 5 cm) in the pasture and lowest in the subsoil (10–45 cm) of the 130-year-old forest (Supplementary Table S1). The n-fatty acids n-C24:0 and n-C26:0 were the two most dominant homologues in the mineral soil of both, pasture and forests of all stand ages (Figures 2A–J). The n-alkane concentration significantly (p = 0.02) differed between pasture and forest soils with highest concentration in the topsoil (0–5 cm) of pastures and lowest concentration in the topsoil of the 130-year-old forest (Figure 1A). The n-alkane concentration decreased with increasing soil depth, most prominent in the pasture and the 40-year-old forest (Figure 1A). The n-alkanes n-C29 and n-C31 were the two most abundant homologues in the mineral soil of pasture and forest stand ages (Figures 2B–K). There was a decrease in the n-alcohol concentration with increasing soil depth in all land-uses, which was only significant (p = 0.01) in the pasture soil (Figure 1A). The Cmax (homologues or maximum 2 homologues with the largest concentration) of n-alcohols was characterized by n-C26 in the mineral soil of pastures over the entire soil profile (Figure 2C and Supplementary Table S2). In all forest stand ages, the n-alcohol n-C26 was the most abundant in the topsoil (0 – 10 cm) while n-C32 became more abundant in deeper soil horizons (Figures 2F,I,L and Supplementary Tables S2–S4).
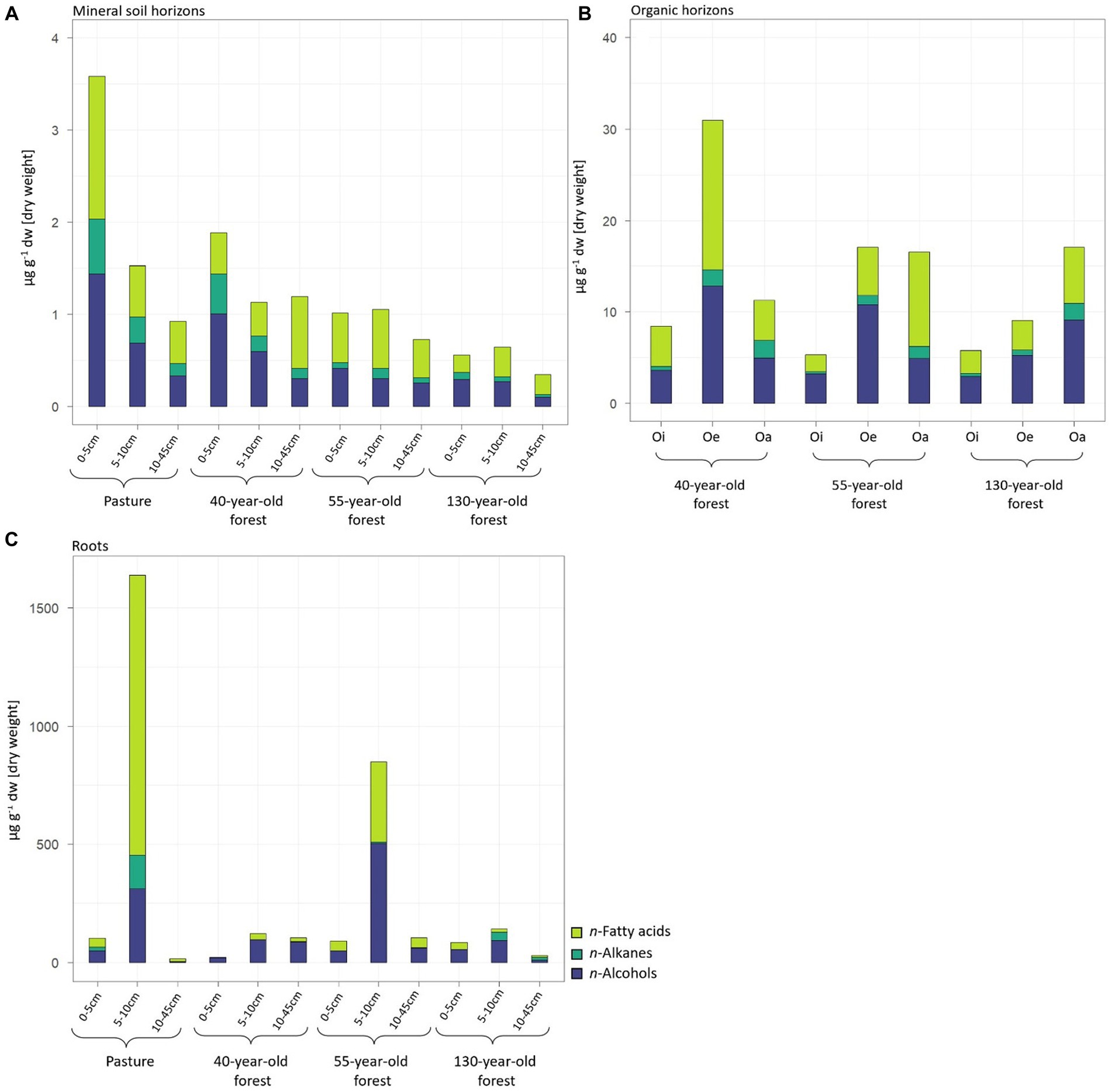
Figure 1. n-Fatty acid, n-alkane, and n-alcohol concentrations of the mineral soil horizons (A) of the Oi-, Oe-, and Oa-horizons (B) and of the root biomass (C) of pasture and different forest stand ages. Values represent average value.
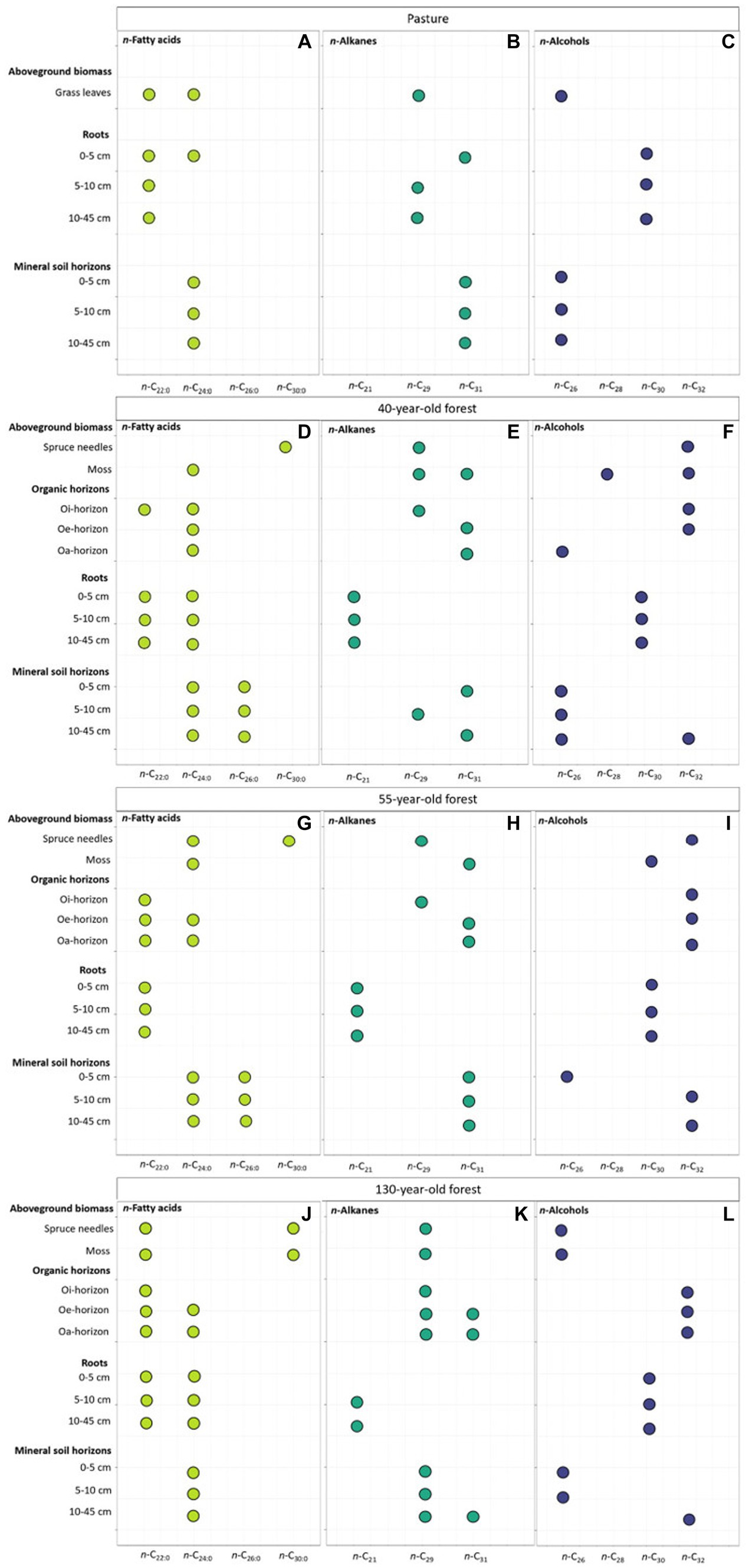
Figure 2. Most abundant lipids (Cmax) of n-fatty acids (n-C22:0, n-C24:0, n-C26:0, n-C30:0; A,D,G,J), of n-alkanes (n-C21, n-C29, n-C31; B,E,H,K), and of n-alcohols (n-C26, n-C28, n-C30, n-C32; C,F,I,L) of aboveground biomass, roots, organic horizons and mineral soil horizons of pastures and of different forest stand ages.
3.1.2 Organic horizons
The concentration of quantified lipids significantly differed (p < 0.001) between the different forest ages in the order 40-year-old > 55-year-old > 130-year-old forest (Figure 1B and Supplementary Table S1). In all investigated samples, the n-fatty acid (FA) concentration was significantly higher (p < 0.001) in relation to the n-alkane (ALK) concentration. The n-fatty acid and n-alcohol concentrations increased from Oi-to the Oa-horizons in all forest stand ages with highest values in the Oe-horizon in the 40-year-old forest. The organic horizons of all forest stand ages were characterized by a Cmax of n-C22:0 and n-C24:0 fatty acids, n-C29 and n-C31 alkanes, and n-C32 alcohol (Figures 2D–L).
3.1.3 Aboveground biomass
Grass leaves were characterized by a Cmax of n-C22:0 and n-C24:0 fatty acids, n-C29 alkane, and the n-C26 alcohol (Figure 2A). All investigated spruce needles showed a Cmax of the n-C30:0 fatty acid while moss samples were characterized by a Cmax of n-C24:0 fatty acid (Figures 2D,G,J). While the Cmax of n-C29 and n-C31 alkanes was similar in spruce needles and moss samples, the Cmax of alcohols differed between spruce needles (n-C32) and moss samples (n-C28, n-C30) (Figures 2F,I). The only exception was the 130-year-old forest with the same Cmax of alcohols (n-C26) for spruce needles and moss samples (Figure 2L).
3.1.4 Root biomass
Pasture and spruce roots of the 55-year-old forest located in the topsoil (5 – 10 cm) showed higher (p = 0.54) n-fatty acid and n-alcohol concentrations (p = 0.68) compared to spruce roots of the 40-year-old and 130-year-old forests (Figure 1C). While the Cmax of fatty acids (n-C22:0, n-C24:0) and alcohols (n-C30) were similar in pasture (Figures 2A,B) and spruce roots of all forest stand ages (Figures 2D–L), the Cmax of alkanes in spruce roots differed in comparison to pasture roots with n-C21 in spruce roots (Figures 2E,H,K) and n-C29 in pasture roots (Figure 2B and Supplementary Tables S2–S5).
3.2 Principal component analysis
In the pasture area, PC1 (38.0%) is dominated by n-C24:0 and n-C26:0 fatty acid concentrations, by n-C24 and n-C26 alcohols, and by n-C21 alkanes (Figure 3A). PC2 (20.0%) is mainly influenced by the n-C29 alkane concentration. In the 40-year-old forest, n-C24:0 and n-C26:0 fatty acids as well as n-C29 and n-C31alkanes contribute the most to PC1 (33.0%, Figure 3B). PC2 (20.8%) is mainly characterized by n-C30 alcohol, the n-C34:0 fatty acid, and by the n-C21 alkane, the latter being more abundant in forest roots. In the 55-year-old forest PC1 (36.5%) is defined by n-C28:0 and n-C32:0 fatty acid concentrations, by n-C21 and n-C31 alkanes, and by n-C20 and n-C30 alcohols (Figure 3C). The n-C22:0 fatty acid, n-C23 and n-C29 alkanes, and n-C22 and n-C34 alcohols mainly contribute to PC2 (21.3%). In the 130-year-old forest, PC1 (34.6%) is affected by the n-C26:0 fatty acid, n-C27 alkane, and n-C24 alcohol, whereas PC2 (17.2%) is influenced by the n-C22:0 fatty acid, the n-C29 alkane, and n-C30 alcohol concentrations (Figure 3D).
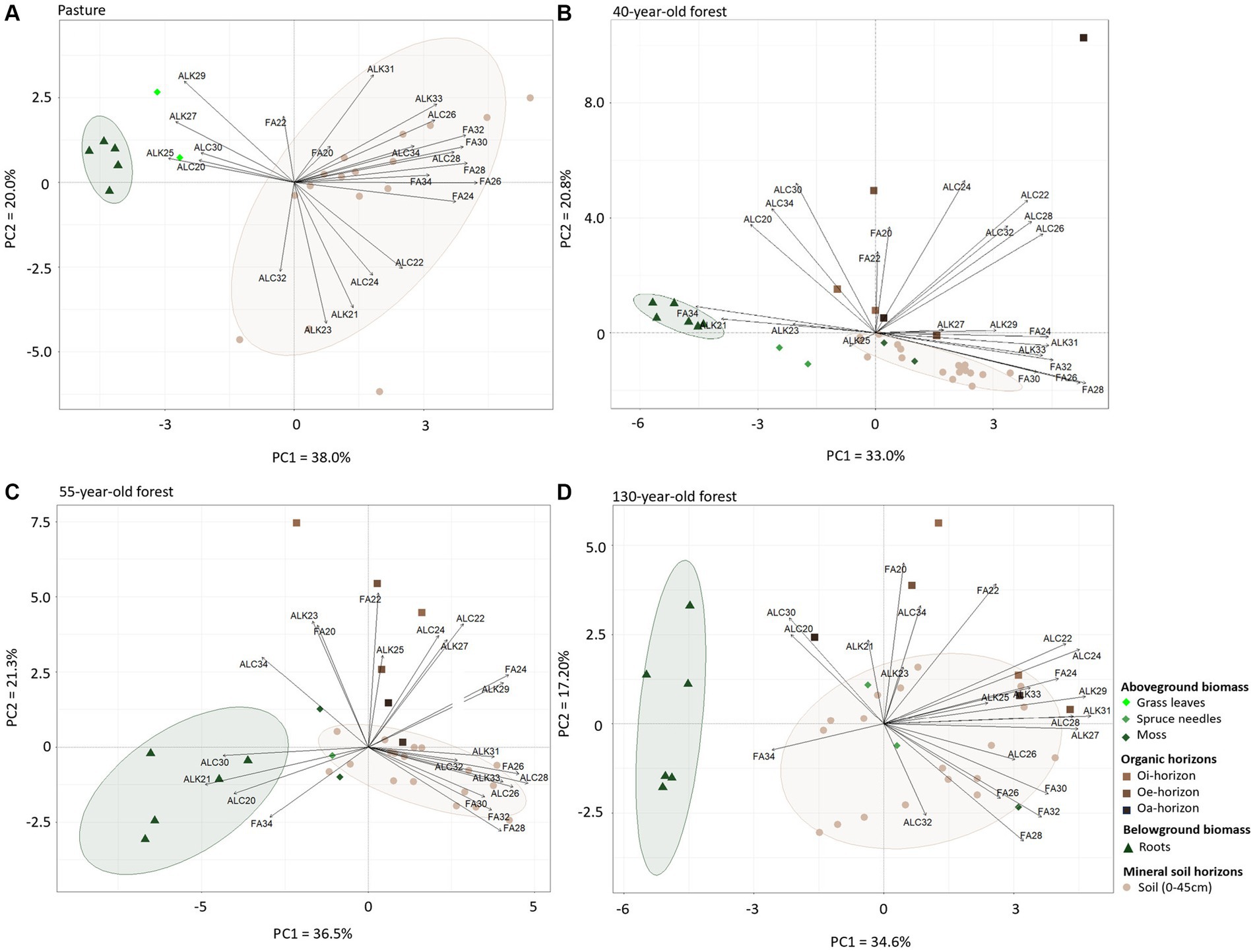
Figure 3. Principal components analysis (PCA) of the n-fatty acid, n-alkane, and n-alcohol composition in the pasture (A), the 40-year-old (B), the 55-year-old (C), and in the 130-year-old forest (D) soil horizons and plant samples. PC1 and PC2 explain the respective variation of the data. FA = n-fatty acids, ALK = n-alkanes, ALC = n-alcohols. Circles around points indicate clusters similarity.
3.3 Molecular proxies
3.3.1 Mineral soil horizons
The ACLFA values of the mineral soil decreased (p = 0.02) with increasing forest age from pasture to the 130-year-old forest (Figure 4A). A decrease (p = 0.04) of the ACLFA values was observed in mineral soil samples with increasing soil depth, especially in the pasture area. Similar to the observed ACLFA values, ACLALK values significantly decreased with increasing soil depth (p < 0.001) and with increasing forest age (p < 0.001) (Figure 4B). In contrast to the decreasing trends of the ACLFA and ACLALK values, the ACLALC values increased (p < 0.001) with increasing soil depth as well as with increasing forest age (p < 0.001) with peak values in the 130-year-old forest (Figure 4C). The CPIFA only significantly decreased with increasing soil depth in the mineral forest soil (p < 0.001), most prominently in the 130-year-old forest (Figure 4D). The CPIALk values decreased with increasing soil depth (p < 0.001) in the mineral soil of pasture and forest areas, except for the 55-year-old forest (Figure 4E). The CPIALC values increased with increasing soil depth (p < 0.001) in the mineral soil in all forest stand ages and decreased with increasing soil depth in the pasture soil (Figure 4F).
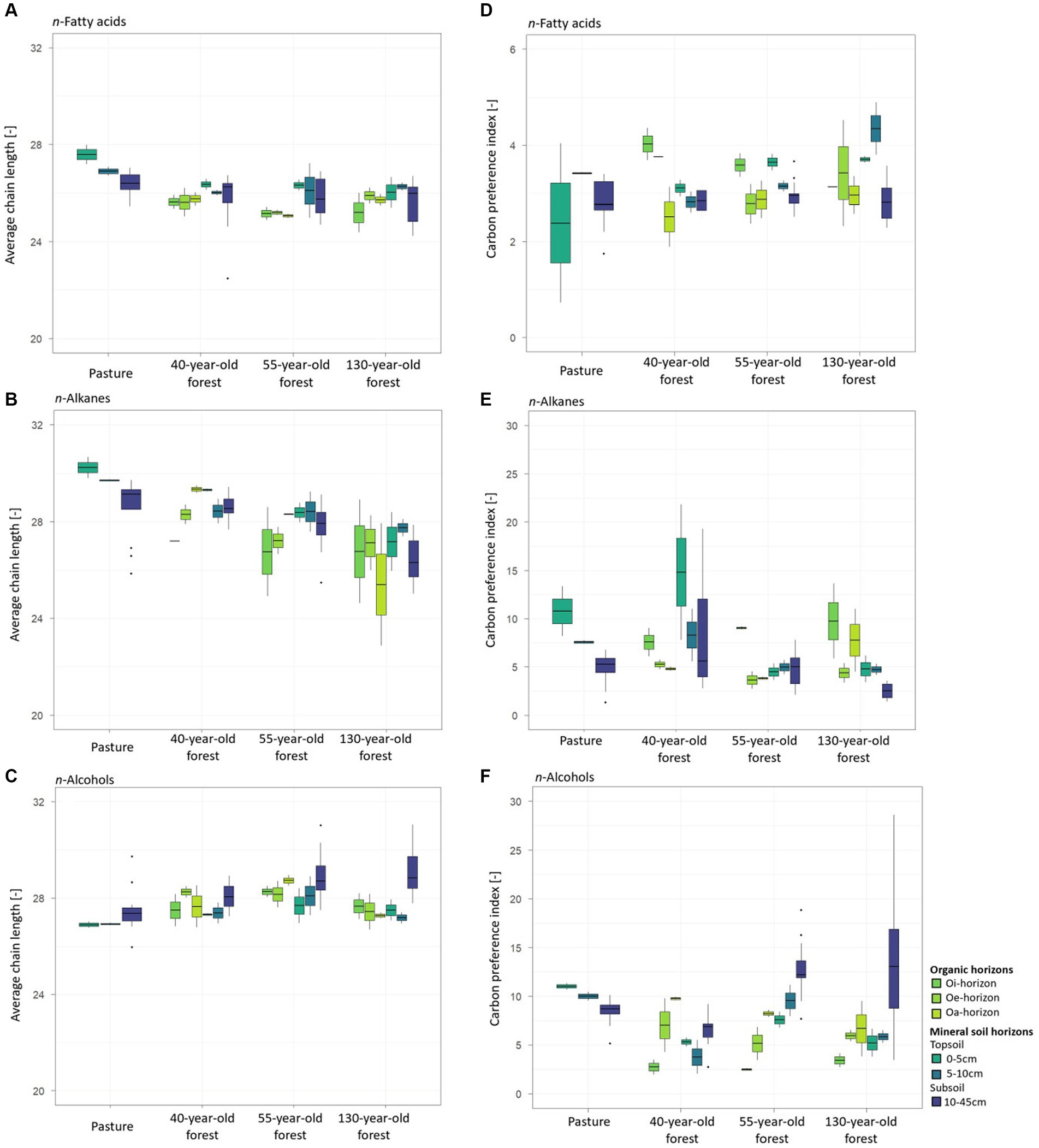
Figure 4. Average chain length (ACL) and carbon preference index (CPI) values of n-fatty acids (A,D), n-alkanes (B,E), and n-alcohols (C,F), of the Oi-, Oe-, and Oa-horizons and of the mineral soil horizons of pasture and the different forest stand ages.
3.3.2 Organic horizons
There was no significant difference in the ACLFA and CPIFA values between the organic horizons of different forest ages (ACLFA: p = 0.24; CPIFA: p = 0.60) or between the different organic horizons within individual forests (ACLFA: p = 0.74; CPIFA: p = 0.13; Figures 4A,D). Increasing ACLALK values (p = 0.28) were observed in the 40-year-old and 55-year-old forest (Figure 4B) from the Oi- toward the Oa-horizons. The opposite trend occurred for the 130-year-old forest (Figure 4B). Decreasing CPIALK values (p = 0.04) from the Oi- to the Oa-horizons were obtained for all forest stand ages but were most prominent in the 55-year-old forest (Figure 4E). The ACLALC values showed no significant difference between the different forest ages (p = 0.15) and between the different organic horizons (p = 0.95; Figure 4C).
3.3.3 Roots
In root samples of the 40-year-old and 55-year-old forests, an increase of the ACLFA values was obtained with increasing soil depth while the opposite was observed for pasture roots and spruce roots of the 130-year-old forest (Figure 5A). The ACLALK decreased (p < 0.001) with soil depth in root samples of both, pasture and forest areas and with increasing forest age (Figure 5B). Decreasing ACLALC values with increasing soil depth were also observed for pasture and spruce roots, except for spruce roots of the 130-year-old forest with an increasing trend (p = 0.14) (Figure 5C). For spruce roots, CPIALK values decreased with increasing soil depth (p < 0.001) in all forest stand ages (Figure 5E), especially in the 40-year-old and in the 130-year-old forests. No clear trend was observed for CPIFA (Figure 5D) and CPIALC (Figure 5F) in relation to soil depth and increasing forest age.
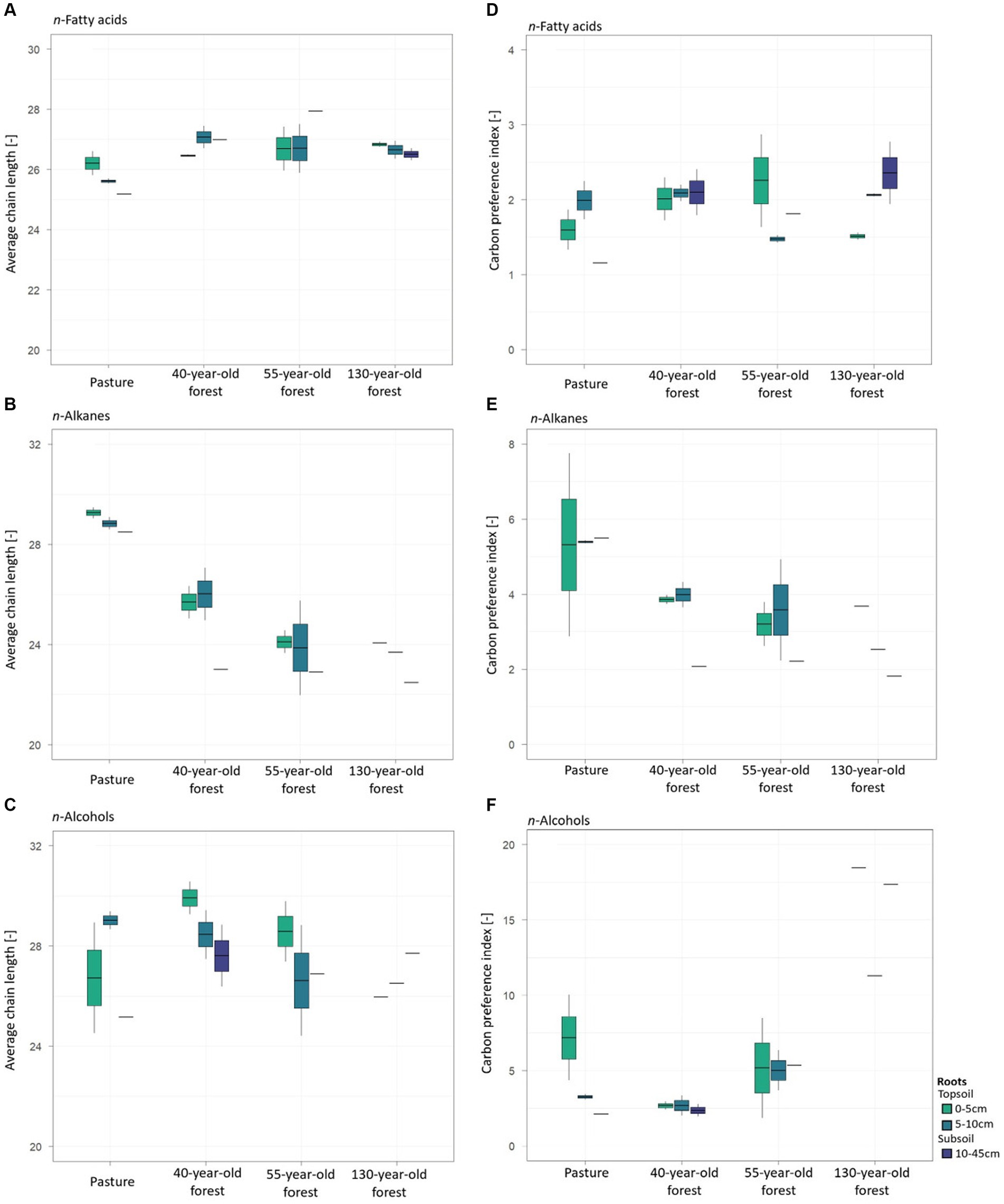
Figure 5. Average chain length (ACL) and carbon preferences index (CPI) values of n-fatty acids (A,D), n-alkanes (B,E), and n-alcohols (C,F) of the root biomass of pasture and of the different forest stand ages.
3.4 Correlation between ACL and CPI values in the mineral soil horizons
In the topsoil (0 – 10 cm) of the pasture, a positive correlation (R2 = 0.91) was observed between the ACLALC and the CPIFA values (Figures 6A,B). In the topsoil of the forests, a negative correlation was observed between the ACLALC and CPIFA values (Figures 6C–H). With increasing forest age, the correlation became less pronounced (forest40: R2 = −0.74; forest55: R2 = −0.70; forest130: R2 = −0.67). A strong negative correlation was observed in the subsoil (10 – 45 cm) between ACLALC and ACLALK values in all forest stand ages (forest40: R2 = −0.82; forest55: R2 = −0.54; forest130: R2 = −0.89). In the 55-year-old forest, there was a strong negative correlation (R2 = −0.86) in the subsoil between ACLALC and CPIFA. A positive correlation between CPIALC and ACLALC was obtained in the 55-year-old (R2 = 0.83) and 130-year-old forests (R2 = 0.91) in the subsoil. A lower negative correlation (R2 = −0.50) was observed in the subsoil of the pasture between ACLALC and CPIALK, specifically in comparison with the 130-year-old forest. In contrast, a strong positive correlation (R2 = 0.97) was found in the pasture soil between ACLALK and CPIALK (Supplementary Tables S6A–H).
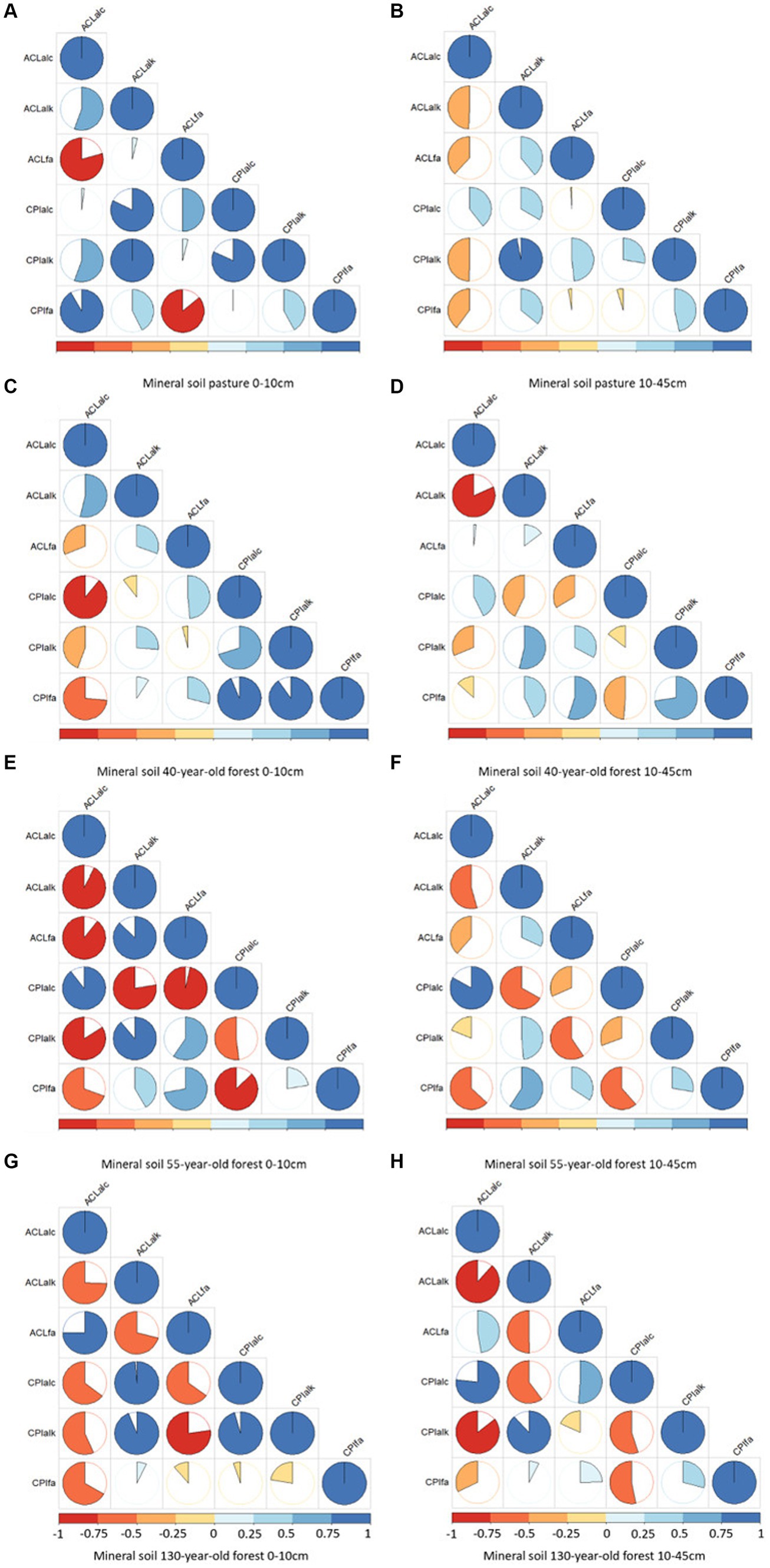
Figure 6. Correlation matrices of the mineral topsoil (0–10 cm) and subsoil (10–45 cm) between the average chain length (ACL) and carbon preference index (CPI) values of n-fatty acids, n-alkanes, and n-alcohols of pasture (topsoil: A and subsoil: B), of the 40-year-old (topsoil: C and subsoil: D), of the year 55-year-old (topsoil: E and subsoil: F), and of the 130-year-old forest stand age (topsoil: G and subsoil: H).
4 Discussion
130 years of afforestation with Norway spruce on a former pasture not only resulted in a change of the aboveground biomass but also of the belowground biomass quality and quantity (Hiltbrunner et al., 2013; Speckert et al., 2023). This thereby caused an alteration of the composition and decomposition of SOM in the soils, which we traced at a molecular level.
4.1 Aboveground biomass as the dominant source of forest soil organic matter
The Cmax of n-fatty acids and n-alkanes in grass leaves and roots of the pasture were identical with chain lengths of n-C24:0 and n-C29, respectively. We found the same homologues to be dominant in the mineral soil of the pasture, which thereby did not enable a clear distinction between aboveground biomass- or root-derived OM input. In contrast to n-fatty acids and n-alkanes, the observed Cmax of n-alcohols differed between grass leaves and pasture roots with n-C26 being the most dominant in grass leaves and n-C30 being the most abundant in grass roots. Such a high abundance of n-C26 alcohol in grass leaves and grassland soils was previously observed in other studies (van Bergen et al., 1997; Jansen et al., 2006; Trendel et al., 2010). The higher proportion of n-C26 over n-C30 alcohols observed in this study (Supplementary Table S2), suggests that grass leaves contribute more to the OM in pasture soils than roots, which contradicts our expectation of rather root-derived OM in pasture soils, particularly in the subsoil. The high abundance of n-C31 alkane, however, which is present in grass roots and becomes more prominent in the subsoil compared to n-C29 (Supplementary Table S2) could be evidence for root-derived OM. Further, an increase in long-chain n-alkanes can be a sign of root-derived OM as some grass roots can produce a significant amount of long-chain n-alkanes (Jansen and Wiesenberg, 2017). This was previously also described by Huang et al. (2011) with a larger abundance of long-chain n-alkanes in roots than in the leaves of two herbaceous plant species (M. trifoliata and C. dimorpholepis) in a subalpine region in central China. The combined predominance of the Cmax of n-C26 alcohols and n-C31 alkanes partially supports our hypothesis of potentially root-derived OM in the subsoil of the pasture, with n-alkanes being indicative for OM input mainly via roots in the subsoil and n-alcohols being indicative for leaf-derived OM in the topsoil.
Similar to grass leaves, the moss and spruce needles investigated in this study were characterized by a clear even-over-odd predominance of n-fatty acids with chain lengths of n-C22:0 to n-C30:0. This is in good agreement with other studies who reported a strong even-over-odd predominance of fatty acids with chain lengths between n-C22:0 and n-C30:0 in grasslands (Jansen et al., 2006) and coniferous forests (Hirave et al., 2020). The same predominant fatty acid homologues were found in the corresponding organic horizons (Supplementary Tables S3–S5). As n-C24:0 being more dominant in moss than in spruce needles in the 40-year-old forest and a Cmax of n-C24:0 being prominent in the organic horizons, this suggests moss-derived OM input as a potential source for SOM in the 40-year-old forest. The Cmax of n-C22:0 in spruce needles and organic horizons in the 130-year-old forest further indicate spruce needle-dominated, rather than moss-dominated OM input. As n-C22:0 was dominant in spruce needles and n-C24:0 was more prominent in moss, this might indicate a shift in the potential source of OM input between the different forest ages from rather moss-dominated OM in the young forest stand (40-year-old) towards more spruce-dominated OM in the old forest stand age (130-year-old). This is supported by the findings by Bona et al. (2013), who pointed out the importance of moss-derived OM in a black spruce forest, with an organic horizon rich in moss-derived OM and its contribution to carbon accumulation in the mineral soil. At the current study site, the dense 40-year-old forest compared to the thinned older forests might lead to more moist soils that enable more moss growth. However, soil moisture has not been monitored at this site.
The observed negative correlation between the n-alcohols n-C26 and n-C30 in the 130-year-old forest with a dominant n-C26 signal particularly in the Oi-horizon and in the upper 10 cm of the mineral soil, further indicate an OM input dominated by spruce needles and a minor role of root-derived OM input in forest areas in the present study. Our observations thus agree with the findings by Crow et al. (2009b), who showed the importance of aboveground litter as OM source in coniferous forest soils in the Cascade Mountains (west-central Oregon, USA) because needle-derived aliphatic compounds, in contrast to root-derived aliphatic compounds, are selectively preserved in soil. On the contrary, Rasse et al. (2005) reported root-derived OM becoming dominant over aboveground OM input after 66 years in Scots pine and after 90 years in beech forest soils in a temperate forest in Belgium. They assessed the shoot- and root-derived litter production and found substantial more carbon produced by root turnover in the soils in comparison to aboveground carbon originating from leaves or branches. In this context, it would be beneficial to analyze additional plant polymers, such as cutin and suberin polymers to be able to make a clearer distinction between shoot- vs. root-derived OM (Mendez-Millan et al., 2011).
The observed Cmax of the n-C29 alkane in spruce needles of all forest stand ages in this study has been frequently observed for coniferous tree species (Bush and McInerney, 2013; Diefendorf and Freimuth, 2017; Hirave et al., 2020). This n-alkane signal is transferred into the organic horizons in all forest stand ages with an increasing n-alkane concentration from the Oi-towards the Oa-horizon in the younger forest stand ages and finally detectable in the mineral soil of all forest stand ages. This observed n-C29 predominance in the mineral soil of forest stand ages is in line with other studies, who reported n-C29 being indicative for soils under coniferous tree species (Lavrieux et al., 2012; Norris et al., 2013). We also found a high concentration of n-C31 alkane in the mineral soil of all forest stand ages which is rather indicative for grass and shrub plants (Bush and McInerney, 2013). However, it was most prominent only in the 40-year-old forest and indicates additional input of understorey vegetation (Rowland et al., 2009) or remains of OM derived from the grass vegetation of the previous pasture (Speckert et al., 2023). Conclusively, among our Norway spruce afforestation chrono-sequence we observed a shift in the OM input with increasing forest age of mainly grass leaf-derived OM in the pasture (0-year-old) to OM input of grass remains, mosses and spruce needles in younger forest stand ages (40-and 55-year-old) towards mainly needle-derived OM in old growth forest stands (130-year-old), which confirms our expectation of more needle-derived OM with increasing forest age.
4.2 Increasing decomposition of organic matter with increasing forest age
The decrease in the CPIALK values from the Oi- to the Oa-horizon in the 130-year-old forest indicates a preferential decomposition of long-chain odd n-alkanes within the organic horizons. This reduction in the odd-over-even predominance of n-alkanes during the transfer from organic horizons into the mineral soil as an indication of OM decomposition was also reported by others (Teunissen van Manen et al., 2020). The higher ACLALK values in the underlying soil horizons, in contrast, show evidence for a preferential decomposition of short-chain n-alkanes and a preferential preservation of long-chain n-alkanes (> n-C27; Lichtfouse et al., 1998), which is in line with previous observations (Marseille et al., 1999; Anokhina et al., 2018; Hirave et al., 2020). The increased ACLALK of the Oi-to the Oa-horizon in the 40-year-old and 55-year-old forests and the high ACLALK values in the underlying mineral soil suggests preferential preservation of long-chain n-alkanes in both, organic and mineral soil horizons. This might be related to potential selective preservation of some fragments of needle polymers like cutin (Kögel-Knabner, 2002; Crow et al., 2009a), where the alkyl chains of n-alkanes are potential intermediate decomposition products. Probably, also the low quality of the needle substrate, together with low pH and low nitrogen concentration of the soil limits microbial activity and thus prevents more complete decomposition (Hamer et al., 2004; Barbier et al., 2008; Vancampenhout et al., 2009). Particularly low soil pH has the potential to preserve organic biomolecules by limiting microbial decomposition (van Bergen et al., 1998). The large lipid concentrations in the 40-year-old forest compared to the pasture and to the 130-year-old forest is most likely related to additional OM input from aboveground litter (Pisani et al., 2016) such as mosses (Nierop et al., 2001). The decrease of the total lipid concentration in the mineral soil from the 40-year-old towards the 130-year-old indicates either a decrease in the OM input or an enhanced decomposition of OM. Additionally, the strong correlation between ACLALC and CPIFA in the mineral soil of the 130-year-old forest supports our observation of a rather enhanced OM decomposition than a lower OM input. Another evidence for the enhanced decomposition in the 130-year-old forest is the increase in n-fatty acid concentrations with increasing soil depth (Supplementary Figure S1A). This increase in the n-fatty acid concentration may be due to the additional decomposition of root-derived suberin compounds (Pisani et al., 2016) presumably resulting in higher n-fatty acid and n-alcohol concentrations as decomposition products of suberin polymers. Additionally, the strong negative correlation between ACLALC and ACLALK and ACLACL and CPIALK in the subsoil (10–45 cm) in the 130-year-old forest further confirms an enhanced decomposition in the subsoil, which was less prominent in the 40-year-old and 55-year-old forests. Given the less easily decomposable character of spruce needles as a major OM source in the 130-year-old forest, this predominant source of OM hampers translocation of OM within the soil profile. As less fresh OM is incorporated into deeper soil horizons, the soil microbial community might rely in more decomposed SOM, which is often located in the subsoil (Rumpel and Kögel-Knabner, 2011). This could be one explanation for the observed enhanced OM decomposition in the subsoil of the 130-year-old forest compared to the younger forest stand ages within this study. This observation is in line with other studies on the same study site, who observed carbon loss particularly in the subsoil with increasing forest stand age (Hiltbrunner et al., 2013). This further suggests a different microbial community between pasture and forest stands (Ortiz et al., 2022).
These results of enhanced OM decomposition in context of spruce needle input in an old forest stand, however, contradicts our second hypothesis of a lower OM decomposition with increasing forest age, but it confirms the observations of Crow et al. (2009b) that coniferous litter might stimulate microbial respiration, particularly in the organic horizons and thereby prime OM decomposition. Moreover, the work of Rasmussen et al. (2007) showed an increased soil C mineralization after addition of Pinus ponderosa litter to temperate coniferous soils in an incubation experiment. Thus, the addition of recalcitrant litter has the potential to prime C mineralization, especially if the present SOM is already recalcitrant (Rasmussen et al., 2008), which is in line to our old forest stand. However, this effect was not observed in the current study in the 40-year-old and 55-year-old forest, as both show evidence for additional OM input of grass, moss, and understorey shrubs.
4.3 Implications of molecular proxies for assessing sources and decomposition of plant-derived organic matter in soils
In the present study, the predominant n-fatty acids were less distinctive and thus not useful for identifying the potential source of OM in comparison to n-alkane and n-alcohol homologues. This was already described by Jansen et al. (2006), who observed that the n-alkane and n-alcohol signal in grass leaves and roots is more specific for individual plant-derived sources of OM than the respective n-fatty acid signal. Generally, the n-alkane pattern produced by leaves and roots of the same plant species might differ substantially (Teunissen van Manen et al., 2020), specifically in trees and shrubs (Jansen and Wiesenberg, 2017). Grass leaves and roots shared the same n-alkane homologues n-C29 and n-C31 which makes them less specific to discern the different sources of plant-derived OM, specifically roots and aboveground biomass. Additionally, not only grass leaves and roots shared the same predominant homologues, but also grass leaves, moss and spruce needles share the same Cmax of n-fatty acids (n-C24:0) and n-alkanes (n-C29, n-C31) in the current study, which challenges the identification of the OM input particularly in young forests, i.e., the 40-year-old forest in the current study. The n-alcohol signal was more specific for identifying potential sources of OM, particularly in pasture areas given n-C26 as the dominant n-alcohol in grass leaves and grassland soils (van Bergen et al., 1997; Jansen et al., 2006; Trendel et al., 2010). In the same line, the n-alcohol signal was helpful for the differentiation between spruce needle- and root-derived OM as both showed different Cmax of n-C26 and n-C32 in spruce needles and a Cmax of n-C30 in spruce roots, respectively. However, the both homologues n-C26 and n-C30 are similarly dominant in grass leaves and spruce needles and in grass roots and spruce roots, which makes the n-alcohol homologues only unique if only one land-use type (pasture vs. forest) is considered in the current study. The n-alkane n-C21 was observed to be predominant exclusively in spruce roots within this study and was therefore the only compound that was useful to differentiate between above- and belowground OM input within the two land-use types. Although the transformation of OM from aboveground biomass to the organic horizons into the mineral soil results in a lower n-alkane concentration in the mineral soil, the distribution pattern remains the same. Additionally, the observed n-alkane pattern in the mineral soil within this study still reflects the one of higher terrestrial plants (Eglinton and Hamilton, 1967), which implies that the n-alkane pattern does not considerably change during decomposition. This is an observation that has been already made by several studies, who investigated the n-alkane transformation from leaf to soil in grasslands (Schäfer et al., 2016; Hirave et al., 2020; Teunissen van Manen et al., 2020). This confirms our observation that n-alkanes are more specific for source identification of OM than n-fatty acids, which might be partially related to the lower degradability of n-alkanes compared with n-fatty acids (Wiesenberg et al., 2004). In summary, if one would consider only one compound class, complementary information of additional compound classes might be missing (Li et al., 2018), thus leading to misguiding conclusions. Therefore, the combination of multiple compound classes as in this study (i.e., n-fatty acids, n-alkanes, and n-alcohols) allows to draw a better conclusions regarding potential sources of SOM.
To identify the potential decomposition of plant-derived OM in soils, molecular proxies such as ACL and CPI values were used in the present study. The ACL is often used to identify potential sources and decomposition of OM whereas the CPI is used to identify the changes in the odd-over-even predominance and decomposition of OM (Wiesenberg and Gocke, 2017). The decrease in both, ACL and CPI values, is generally accepted to be indicative for decomposition (Marzi et al., 1993). In the present study, CPI values of all three substance classes (i.e., n-fatty acids, n-alkanes, and n-alcohols) were observed to be indicative for decomposition. The strong correlation between ACLFA, ACLALK and ACLALC with increasing forest age further proved an increased OM decomposition with increasing forest age in our study. Compared to other molecular proxies, CPIALC was found to be less indicative for OM decomposition due to its high variability within samples and due to no clear trend in context of different land-use and increasing forest age.
5 Conclusion
In this study, we identified a shift in the organic matter sources in a subalpine afforestation sequence with Norway spruce on a former pasture by using a multi-proxy molecular marker approach. With increasing forest age (0–130 years), the organic matter input changed from grass leaf-derived over moss- to spruce needle-derived organic matter. In contrast, root-derived organic matter seems to play a minor role in organic matter input in both, pasture and forest areas. This highlights the importance of aboveground biomass as major contribution to soil organic matter in subalpine pasture and forest soils. Additionally, molecular proxies such as average chain-length (ACL) and carbon-preference index (CPI) values of long-chain n-alkanes, n-fatty acids and n-alcohols indicated an increased decomposition of organic matter with increasing forest age, particularly in the subsoil (10–45 cm). This is of interest particularly in alpine and subalpine ecosystems, where SOM losses are expected to become greater with increasing temperatures in the future.
To better understand the connection between organic matter input and decomposition and the resulting effects on the soil organic matter dynamic following afforestation, future research should include plant polymers as important source of some of the monomeric substances investigated in the current study. Furthermore, the analysis of cutin and suberin polymers can help to disentangle the importance of root- vs. shoot-derived organic matter and their contribution to the stable soil organic matter pool in alpine ecosystems. In addition to an improved characterization of plant-derived organic matter, the characterization of the microbial community and related processes influencing organic matter decomposition would facilitate a better assessment of related processes.
Data availability statement
The original contributions presented in the study are included in the article/Supplementary material, further inquiries can be directed to the corresponding author.
Author contributions
TS: Conceptualization, Data curation, Formal analysis, Investigation, Methodology, Software, Visualization, Writing – original draft. GW: Funding acquisition, Supervision, Writing – review & editing, Methodology, Project administration, Resources.
Funding
The author(s) declare financial support was received for the research, authorship, and/or publication of this article. We acknowledge funding by the Swiss National Science Foundation (SNSF) under contract 188684 of the IQ-SASS project (Improved Quantitative Source Assessment of organic matter in Soils and Sediments using molecular markers and inverse modeling).
Acknowledgments
We thank Barbara Siegfried, Yves Brügger, and Dmitry Tichomirov for support during lab work and Thomy Keller, Binyan Sun, and Dr. Maziar Mohammadi for their helpful advice.
Conflict of interest
The authors declare that the research was conducted in the absence of any commercial or financial relationships that could be construed as a potential conflict of interest.
The author(s) declared that they were an editorial board member of Frontiers, at the time of submission. This had no impact on the peer review process and the final decision.
Publisher’s note
All claims expressed in this article are solely those of the authors and do not necessarily represent those of their affiliated organizations, or those of the publisher, the editors and the reviewers. Any product that may be evaluated in this article, or claim that may be made by its manufacturer, is not guaranteed or endorsed by the publisher.
Supplementary material
The Supplementary material for this article can be found online at: https://www.frontiersin.org/articles/10.3389/ffgc.2023.1290922/full#supplementary-material
References
Angst, G., John, S., Mueller, C. W., Kögel-Knabner, I., and Rethemeyer, J. (2016). Tracing the sources and spatial distribution of organic carbon in subsoils using a multi-biomarker approach. Sci. Rep. 6, 1–12. doi: 10.1038/srep29478
Anokhina, N. A., Demin, V. V., and Zavgorodnyaya, Y. A. (2018). Compositions of n-alkanes and n-methyl ketones in soils of the forest-park zone of Moscow. Eurasian Soil Sci. 51, 637–646. doi: 10.1134/S1064229318060030
Barbier, S., Gosselin, F., and Balandier, P. (2008). Influence of tree species on understory vegetation diversity and mechanisms involved – a critical review for temperate and boreal forests. For. Ecol. Manag. 254, 1–15. doi: 10.1016/j.foreco.2007.09.038
Bolliger, J., Hagedorn, F., Leifeld, J., Böhl, J., Zimmermann, S., Soliva, R., et al. (2008). Effects of land-use change on carbon stocks in Switzerland. Ecosystems 11, 895–907. doi: 10.1007/s10021-008-9168-6
Bona, K. A., Fyles, J. W., Shaw, C., and Kurz, W. A. (2013). Are mosses required to accurately predict upland black spruce forest soil carbon in national-scale forest C accounting models? Ecosystems 16, 1071–1086. doi: 10.1007/s10021-013-9668-x
Brändli, U. B. (2010). Schweizerisches Landesforstinventar. Ergebnisse der dritten Erhebung 2004–2006. Birmensdorf, Eidgenössische Forschungsanstalt für Wald, Schnee und Landschaft WSL. Bern: Bundesamt für Umwelt, BAFU.
Bush, R. T., and McInerney, F. A. (2013). Leaf wax n-alkane distributions in and across modern plants: implications for paleoecology and chemotaxonomy. Geochim. Cosmochim. Acta 117, 161–179. doi: 10.1016/j.gca.2013.04.016
Chabbi, A., Kögel-Knabner, I., and Rumpel, C. (2009). Stabilised carbon in subsoil horizons is located in spatially distinct parts of the soil profile. Soil Biol. Biochem. 41, 256–261. doi: 10.1016/j.soilbio.2008.10.033
Cranwell, P. A. (1973). Chain-length distribution of n-alkanes from lake sediments in relation to post-glacial environmental change. Freshw. Biol. 3, 259–265. doi: 10.1111/j.1365-2427.1973.tb00921.x
Crow, S. E., Lajtha, K., Bowden, R. D., Yano, Y., Brant, J. B., Caldwell, B. A., et al. (2009b). Increased coniferous needle inputs accelerate decomposition of soil carbon in an old-growth forest. For. Ecol. Manag. 258, 2224–2232. doi: 10.1016/j.foreco.2009.01.014
Crow, S. E., Lajtha, K., Filley, T. R., Swanston, C. W., Bowden, R. D., and Caldwell, B. A. (2009a). Sources of plant-derived carbon and stability of organic matter in soil: implications for global change. Glob. Chang. Biol. 15, 2003–2019. doi: 10.1111/j.1365-2486.2009.01850.x
Dai, G., Zhu, S., Cai, Y., Zhu, E., Jia, Y., Ji, C., et al. (2022). Plant-derived lipids play a crucial role in forest soil carbon accumulation. Soil Biol. Biochem. 168:108645. doi: 10.1016/j.soilbio.2022.108645
Dennis, P. G., Miller, A. J., and Hirsch, P. R. (2010). Are root exudates more important than other sources of rhizodeposits in structuring rhizosphere bacterial communities? FEMS Microb. Ecol. 72, 313–327. doi: 10.1111/j.1574-6941.2010.00860.x
Diefendorf, A. F., and Freimuth, E. J. (2017). Extracting the most from terrestrial plant-derived n-alkyl lipids and their carbon isotopes from the sedimentary record: a review. Org. Geochem. 103, 1–21. doi: 10.1016/j.orggeochem.2016.10.016
Eglinton, G., and Hamilton, R. J. (1967). Leaf epicuticular waxes. Science 156, 1322–1335. doi: 10.1126/science.156.3780.1322
Gehrig-Fasel, J., Guisan, A., and Zimmermann, N. E. (2007). Tree line shifts in the Swiss Alps: climate change or land abandonment? J. Veg. Sci. 18, 571–582. doi: 10.1111/j.1654-1103.2007.tb02571.x
Guidi, C., Lehmann, M. M., Meusburger, K., Saurer, M., Vitali, V., Peter, M., et al. (2023). Tracing sources and turnover of soil organic matter in a long-term irrigated dry forest using a novel hydrogen isotope approach. Soil Biol. Biochem. 184:109113. doi: 10.1016/j.soilbio.2023.109113
Hamer, U., Marschner, B., Brodowski, S., and Amelung, W. (2004). Interactive priming of black carbon and glucose mineralisation. Org. Geochem. 35, 823–830. doi: 10.1016/j.orggeochem.2004.03.003
Herrera-Herrera, A. V., Leierer, L., Jambrina-Enríquez, M., Connolly, R., and Mallol, C. (2020). Evaluating different methods for calculating the carbon preference index (CPI): implications for palaeoecological and archaeological research. Org. Geochem. 146:104056. doi: 10.1016/j.orggeochem.2020.104056
Hiltbrunner, D., Zimmermann, S., and Hagedorn, F. (2013). Afforestation with Norway spruce on a subalpine pasture alters carbon dynamics but only moderately affects soil carbon storage. Biogeochemistry 115, 251–266. doi: 10.1007/s10533-013-9832-6
Hirave, P., Wiesenberg, G. L. B., Birkholz, A., and Alewell, C. (2020). Understanding the effects of early degradation on isotopic tracers: implications for sediment source attribution using compound-specific isotope analysis (CSIA). Biogeosciences 17, 2169–2180. doi: 10.5194/bg-17-2169-2020
Hodge, A., Berta, G., Doussan, C., Merchan, F., and Crespi, M. (2009). Plant root growth, architecture and function. Plant Soil 321, 153–187. doi: 10.1007/s11104-009-9929-9
Huang, X., Wang, C., Zhang, J., Wiesenberg, G. L., Zhang, Z., and Xie, S. (2011). Comparison of free lipid compositions between roots and leaves of plants in the Dajiuhu Peatland, central China. Geochemical Journal, 45, 365–373. doi: 10.2343/geochemj.1.0129
Jahn, R., Blume, H. P., Asio, V., Spaargaren, O., and Schad, P. (2006). Guidelines for soil description. 4th edition. Rome: Food and Agriculture Organization of the United Nations.
Jansen, B., Nierop, K. G., Hageman, J. A., Cleef, A. M., and Verstraten, J. M. (2006). The straight-chain lipid biomarker composition of plant species responsible for the dominant biomass production along two altitudinal transects in the Ecuadorian Andes. Org. Geochem. 37, 1514–1536. doi: 10.1016/j.orggeochem.2006.06.018
Jansen, B., and Wiesenberg, G. L. B. (2017). Opportunities and limitations related to the application of plant-derived lipid molecular proxies in soil science. Soil 3, 211–234. doi: 10.5194/soil-3-211-2017
Kalbitz, K., Solinger, S., Park, J.-H., Michalzik, B., and Matzner, E. (2000). Controls on the dynamics of dissolved organic matter in soils: a review. Soil Sci. 165, 277–304. doi: 10.1097/00010694-200004000-00001
Kögel-Knabner, I. (2002). The macromolecular organic composition of plant and microbial residues as inputs to soil organic matter. Soil Biol. Biochem. 34, 139–162. doi: 10.1016/S0038-0717(01)00158-4
Lajtha, K., and Bowden, R. D. (2014). Litter and root manipulations provide insights into soil organic matter dynamics and stability. Soil Sci. Soc. Am. J. 78, S261–S269. doi: 10.2136/sssaj2013.08.0370nafsc
Lal, R. (2005). Forest soils and carbon sequestration. For. Ecol. Manag. 220, 242–258. doi: 10.1016/j.foreco.2005.08.015
Lavrieux, M., Breheret, J., Disnar, J., Jacob, J., Le Milbeau, C., and Zocatelli, R. (2012). Preservation of an ancient grassland biomarker signature in a forest soil from the French massif central. Org. Geochem. 51, 1–10. doi: 10.1016/j.orggeochem.2012.07.003
Li, X., Anderson, B. J., Vogeler, I., and Schwendenmann, L. (2018). Long-chain n-alkane and n-fatty acid characteristics in plants and soil-potential to separate plant growth forms, primary and secondary grasslands? Sci. Total Environ. 645, 1567–1578. doi: 10.1016/j.scitotenv.2018.07.105
Lichtfouse, E., Chenu, C., Baudin, F., Leblond, C., Da Silva, M., Béhar, F., et al. (1998). A novel pathway of soil organic matter formation by selective preservation of resistant straight-chain biopolymers: chemical and isotope evidence. Org. Geochem. 28, 411–415. doi: 10.1016/S0146-6380(98)00005-9
Marseille, F., Disnar, J. R., Guillet, B., and Noack, Y. (1999). N-alkanes and free fatty acids in humus and A1 horizons of soils under beech, spruce and grass in the massif-central (Mont-Lozère), France. Eur. J. Soil Sci. 50, 433–441. doi: 10.1046/j.1365-2389.1999.00243.x
Marzi, R., Torkelson, B. E., and Olson, R. K. (1993). A revised carbon preference index. Org. Geochem. 20, 1303–1306. doi: 10.1016/0146-6380(93)90016-5
Mendez-Millan, M., Dignac, M. F., Rumpel, C., and Derenne, S. (2011). Can cutin and suberin biomarkers be used to trace shoot and root-derived organic matter? A molecular and isotopic approach. Biogeochemistry 106, 23–38. doi: 10.1007/s10533-010-9407-8
Naafs, D. F., van Bergen, P. F., Boogert, S. J., and de Leeuw, J. W. (2004). Solvent-extractable lipids in an acid andic forest soil; variations with depth and season. Soil Biol. Biochem. 36, 297–308. doi: 10.1016/j.soilbio.2003.10.005
Nadelhoffer, K. J., Boone, R. D., Bowden, R. D., Canary, J. D., Kaye, J., Micks, P., et al. (2004). “The DIRT experiment: litter and root influences on forest soil organic matter stocks and function” in Forests in time: the environmental consequences of 1000 years of change in New England. eds. D. R. Foster and J. D. Aber (New Haven: Yale University Press), 300–315.
Nierop, K. G. J., van Lagen, B., and Buurman, P. (2001). Composition of plant tissues and soil organic matter in the first stages of a vegetation succession. Geoderma 100, 1–24. doi: 10.1016/S0016-7061(00)00078-1
Norris, C. E., Dungait, J. A., Joynes, A., and Quideau, S. A. (2013). Biomarkers of novel ecosystem development in boreal forest soils. Org. Geochem. 64, 9–18. doi: 10.1016/j.orggeochem.2013.08.014
Ortiz, C., Fernández-Alonso, M. J., Kitzler, B., Diaz-Pines, E., Saiz, G., Rubio, A., et al. (2022). Variations in soil aggregation, microbial community structure and soil organic matter cycling associated to long-term afforestation and woody encroachment in a Mediterranean alpine ecotone. Geoderma 405:115450. doi: 10.1016/j.geoderma.2021.115450
Pan, Y., Birdsey, R. A., Fang, J., Houghton, R., Kauppi, P. E., Kurz, W. A., et al. (2011). A large and persistent carbon sink in the world’s forests. Science 333, 988–993. doi: 10.1126/science.1201609
Paul, K. I., Polglase, P. J., Nyakuengama, J. G., and Khanna, P. K. (2002). Change in soil carbon following afforestation. For. Ecol. Manag. 168, 241–257. doi: 10.1016/S0378-1127(01)00740-X
Pisani, O., Lin, L. H., Lun, O. O., Lajtha, K., Nadelhoffer, K. J., Simpson, A. J., et al. (2016). Long-term doubling of litter inputs accelerates soil organic matter degradation and reduces soil carbon stocks. Biogeochemistry 127, 1–14. doi: 10.1007/s10533-015-0171-7
Poeplau, C., and Don, A. (2013). Sensitivity of soil organic carbon stocks and fractions to different land-use changes across Europe. Geoderma 192, 189–201. doi: 10.1016/j.geoderma.2012.08.003
Puhe, J. (2003). Growth and development of the root system of Norway spruce (Picea abies) in forest stands - a review. For. Ecol. Manag. 175, 253–273. doi: 10.1016/S0378-1127(02)00134-2
Pulleman, M. M., Six, J., Uyl, A., Marinissen, J. C. Y., and Jongmans, A. G. (2005). Earthworms and management affect organic matter incorporation and microaggregate formation in agricultural soils. Appl. Soil Ecol. 29, 1–15. doi: 10.1016/j.apsoil.2004.10.003
R Core Team (2020). A language and environment for statistical computing. R foundation for statistical computing, Vienna, Austria. Available at: http://www.R-project.org/
Rasmussen, C., Southard, R. J., and Horwath, W. R. (2007). Soil mineralogy affects conifer forest soil carbon source utilization and microbial priming. Soil Sci. Soc. Am. J. 71, 1141–1150. doi: 10.2136/sssaj2006.0375
Rasmussen, C., Southard, R. J., and Horwath, W. R. (2008). Litter type and soil minerals control temperate forest soil carbon response to climate change. Glob. Chang. Biol. 14, 2064–2080. doi: 10.1111/j.1365-2486.2008.01639.x
Rasse, D. P., Rumpel, C., and Dignac, M. F. (2005). Is soil carbon mostly root carbon? Mechanisms for a specific stabilisation. Plant Soil 269, 341–356. doi: 10.1007/s11104-004-0907-y
Risch, A. C., Jurgensen, M. F., Page-Dumroese, D. S., Wildi, O., and Schütz, M. (2008). Long-term development of above-and below-ground carbon stocks following land-use change in subalpine ecosystems of the Swiss National Park. Can. J. For. Res. 38, 1590–1602. doi: 10.1139/X08-014
Rowland, S. M., Prescott, C. E., Grayston, S. J., Quideau, S. A., and Bradfield, G. E. (2009). Recreating a functioning forest soil in reclaimed oil sands in Northern Alberta: an approach for measuring success in ecological restoration. J. Environ. Qual. 38, 1580–1590. doi: 10.2134/jeq2008.0317
Rumpel, C., and Kögel-Knabner, I. (2011). Deep soil organic matter - a key but poorly understood component of terrestrial C cycle. Plant Soil 338, 143–158. doi: 10.1007/s11104-010-0391-5
Schäfer, I. K., Lanny, V., Franke, J., Eglinton, T. I., Zech, M., Vysloužilová, B., et al. (2016). Leaf waxes in litter and topsoils along a European transect. Soil 2, 551–564. doi: 10.5194/soil-2-551-2016
Speckert, T. C., Suremann, J., Gavazov, K., Santos, M. J., Hagedorn, F., and Wiesenberg, G. L. B. (2023). Soil organic carbon stocks did not change after 130 years of afforestation on a former Swiss alpine pasture. EGUsphere 2023, 1–20. doi: 10.5194/egusphere-2023-645
Strand, L. T., Fjellstad, W., Jackson-Blake, L., and De Wit, H. A. (2021). Afforestation of a pasture in Norway did not result in higher soil carbon, 50 years after planting. Landsc. Urban Plan. 207:104007. doi: 10.1016/j.landurbplan.2020.104007
Teunissen van Manen, M. L., Jansen, B., Cuesta, F., León-Yánez, S., and Gosling, W. D. (2020). From leaf to soil: n-alkane signal preservation, despite degradation along an environmental gradient in the tropical Andes. Biogeosciences 17, 5465–5487. doi: 10.5194/bg-17-5465-2020
Thuille, A., and Schulze, E. D. (2006). Carbon dynamics in successional and afforested spruce stands in Thuringia and the Alps. Glob. Chang. Biol. 12, 325–342. doi: 10.1111/j.1365-2486.2005.01078.x
Trendel, J. M., Schaeffer, P., Adam, P., Ertlen, D., and Schwartz, D. (2010). Molecular characterisation of soil surface horizons with different vegetation in the Vosges massif (France). Org. Geochem. 41, 1036–1039. doi: 10.1016/j.orggeochem.2010.04.014
Van Bergen, P. F., Bull, I. D., Poulton, P. R., and Evershed, R. P. (1997). Organic geochemical studies of soils from the Rothamsted classical experiments - I. Total lipid extracts, solvent insoluble residues and humic acids from Broadbalk wilderness. Org. Geochem. 26, 117–135. doi: 10.1016/S0146-6380(96)00134-9
Van Bergen, P. F., Nott, C. J., Bull, I. D., Poulton, P. R., and Evershed, R. P. (1998). Organic geochemical studies of soils from the Rothamsted classical experiments-IV. Preliminary results from a study of the effect of soil pH on organic matter decay. Org. Geochem. 29, 1779–1795. doi: 10.1016/S0146-6380(98)00188-0
Vancampenhout, K., Wouters, K., De Vos, B., Buurman, P., Swennen, R., and Deckers, J. (2009). Differences in chemical composition of soil organic matter in natural ecosystems from different climatic regions–a pyrolysis–GC/MS study. Soil Biol. Biochem. 41, 568–579. doi: 10.1016/j.soilbio.2008.12.023
Volk, M., Bassin, S., Lehmann, M. F., Johnson, M. G., and Andersen, C. P. (2018). 13C isotopic signature and C concentration of soil density fractions illustrate reduced C allocation to subalpine grassland soil under high atmospheric N deposition. Soil Biol. Biochem. 125, 178–184. doi: 10.1016/j.soilbio.2018.07.014
Wiesenberg, G. L. B., and Gocke, M. I. (2017). “Analysis of lipids and polycyclic aromatic hydrocarbons as indicator of past and present (micro-) biological activity” in Hydrocarbon and lipid microbiology protocols – petroleum, hydrocarbon and lipid analysis. eds. T. J. McGenity, N. T. K. Nogales, and B. Fernández (Berlin, Heidelberg: Springer), 61–91.
Wiesenberg, G. L. B., Gocke, M., and Kuzyakov, Y. (2010). Fast incorporation of root-derived lipids and fatty acids into soil–evidence from a short term multiple 14CO2 pulse labelling experiment. Org. Geochem. 41, 1049–1055. doi: 10.1016/j.orggeochem.2009.12.007
Keywords: soil organic matter, afforestation, Picea abies L., subalpine ecosystem, n-fatty acids, n-alkanes, n-alcohols
Citation: Speckert TC and Wiesenberg GLB (2023) Source or decomposition of soil organic matter: what is more important with increasing forest age in a subalpine setting? Front. For. Glob. Change. 6:1290922. doi: 10.3389/ffgc.2023.1290922
Edited by:
Marie Spohn, Swedish University of Agricultural Sciences, SwedenReviewed by:
Jörg Luster, Swiss Federal Institute for Forest, Snow and Landscape Research (WSL), SwitzerlandNicasio T. Jiménez-Morillo, University of Évora, Portugal
Copyright © 2023 Speckert and Wiesenberg. This is an open-access article distributed under the terms of the Creative Commons Attribution License (CC BY). The use, distribution or reproduction in other forums is permitted, provided the original author(s) and the copyright owner(s) are credited and that the original publication in this journal is cited, in accordance with accepted academic practice. No use, distribution or reproduction is permitted which does not comply with these terms.
*Correspondence: Tatjana Carina Speckert, dGF0amFuYWNhcmluYS5zcGVja2VydEBnZW8udXpoLmNo