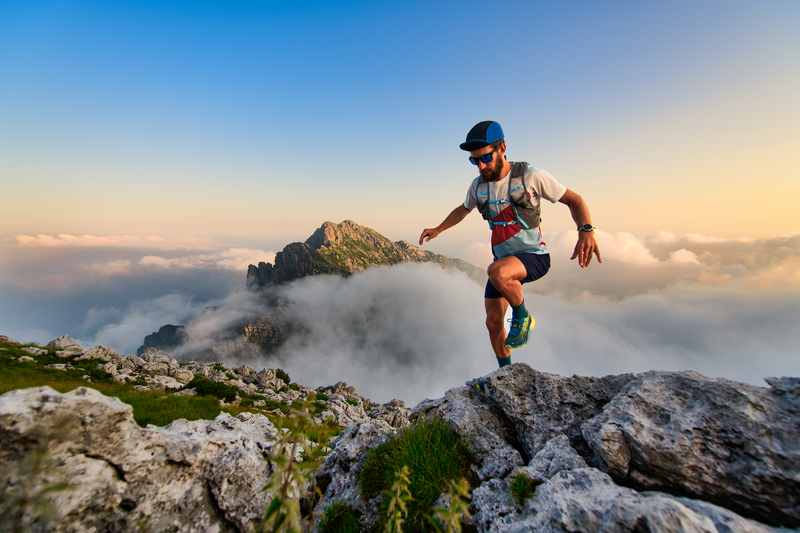
95% of researchers rate our articles as excellent or good
Learn more about the work of our research integrity team to safeguard the quality of each article we publish.
Find out more
ORIGINAL RESEARCH article
Front. For. Glob. Change , 24 July 2023
Sec. Pests, Pathogens and Invasions
Volume 6 - 2023 | https://doi.org/10.3389/ffgc.2023.1166421
This article is part of the Research Topic "Forentomics": forest pest and pathogen biology, ecology, and management using omics View all 10 articles
Introduction: Larvae of the invasive emerald ash borer [EAB, Agrilus planipennis Fairmaire (Coleoptera: Buprestidae)], kill over 99% of green ash (Fraxinus pennsylvanica Marshall) trees they infest, yet a small percentage of green ash (“lingering ash”) survive years of heavy EAB attack. In the face of an ongoing invasion that threatens multiple North American Fraxinus species with extinction, any evidence for reproducible defensive responses in the native species merits investigation.
Methods: We evaluated the capacity of three families of green ash F1 progeny to kill EAB larvae when challenged in greenhouse studies by infestation with a uniform density of EAB eggs followed by dissection 8 weeks post-infestation and comparison of the host metabolomic profiles.
Results: The mean proportions of host-killed larvae in the two families of F1 progeny from lingering ash parents were significantly higher than that of host-killed larvae in the family of F1 progeny from susceptible ash parents (p < 0.001). Untargeted metabolomics comparing F1 progeny in the quartile with the highest percent host-killed larvae (HHK) to F1 progeny in the quartile with the lowest percent host-killed larvae (LHK) and to the uninfested F1 progeny within each family revealed evidence for induced biochemical responses to EAB. Infested trees produced significantly higher levels of select secoiridoids than uninfested trees, and LHK progeny produced significantly higher levels of select secoiridoids than the HHK progeny. HHK progeny produced significantly higher abundances of three metabolites annotated as aromatic alkaloids than the LHK and uninfested individuals.
Discussion: Based on these results, we hypothesize that green ash responds to EAB infestation. However, only certain trees have the genetic capacity to tailor a response that kills enough EAB larvae to prevent lethal damage to the vascular system. Rigorous tests of this hypothesis will require 15–20 years of additional crossing, phenotyping, and omics analyses. The results of this investigation will encourage the establishment and continuation of breeding programs that, in concert with biocontrol and management, could provide trees that slow, if not halt, the decimation of the Fraxinus gene pool. At the same time, ongoing work on host-insect interaction will contribute to our understanding of how forest trees recognize and defend themselves against phloem-feeding insects.
Invasive pests and pathogens, now widely dispersed through globalization, threaten nearly two-thirds of North American forests (Potter et al., 2019). Exacerbated by climate instability, these increasingly severe infections and infestations threaten forested ecosystems and inflict billions of dollars in direct costs to individuals and local communities (Kovacs et al., 2010; Aukema et al., 2011; Hicke et al., 2012; Krist et al., 2014; Bradshaw et al., 2016; Lovett et al., 2016; Potter et al., 2019; Anderegg et al., 2020; Hoover and Riddle, 2020; Miniat et al., 2021). The emerald ash borer (EAB, Agrilus planipennis Fairmaire), a woodboring beetle native to Asia, was discovered in Michigan, United States, and Ontario, Canada, in 2002 (Poland and McCullough, 2006). EAB larvae feed on the vascular cambium, cork cambium, and phloem, inflicting severe vascular damage and consequently killing 95–99% of mature green ash [Fraxinus pennsylvanica Marshall (Lamilaes: Oleaceae) Fraxinus sect. Meliodes] trees within 3–5 years of peak infestation (Knight et al., 2013). EAB is the most damaging invasive forest insect pest ever to have invaded North America, threatening nearly all native species of Fraxinus with functional extinction (Poland and McCullough, 2006; Herms and McCullough, 2014; Aubin et al., 2015).
Green ash, the most widely distributed Fraxinus species in North America, is a diecious, diploid, and deciduous tree species native to the eastern and central United States and eastern Canada (Walters and Yawney, 2004). EAB infestation has resulted in the rapid loss of hundreds of millions of green ash, not only in forests and rural areas but also in cities, where green ash was once one of the most widely planted street and park trees in the United States (Knight et al., 2013; Hanberry, 2014). The ash mortality in EAB-infested stands can approach 100% within 6 years of local EAB detection (Knight et al., 2013).
Long-term forest plot monitoring initiated in 2005, 3 years after the initial detection of EAB in North America, revealed a small number of green ash (0.1–1%) that survive for years after all other surrounding green ash have died (Knight et al., 2012). Although the susceptibility of almost all healthy green ash suggests an absence of defensive capacity against EAB, the persistence of these few “lingering ash” in heavily infested stands suggests that innate defensive responses may be rare rather than absent. Lingering ash have been and continue to be propagated as potential sources of genetic resistance for a United States Forest Service (USFS) breeding research program (Koch et al., 2012, 2015). The USFS program identified green ash trees based on standard criteria for distinguishing Fraxinus species and selected lingering ash in natural forests based on two additional criteria: (1) a healthy canopy at least 2 years after the mortality rate of the stand exceeded 95 percent, and (2) a minimum diameter at breast height (DBH, 1.37 m above ground) of 26 cm, indicating that the tree was over the minimum size preferred by EAB when the infestation was at peak levels (Koch et al., 2015). These lingering ash trees show evidence of a less severe emerald ash borer infestation than susceptible phenotypes in the forest and maintain a healthy crown for years after local conspecifics have died (Steiner et al., 2019). Individuals meeting these criteria have been clonally propagated through grafting and subjected to EAB infestation bioassays (described below) that provided evidence of the ability of some selected lingering ash trees to mount defensive responses against EAB.
Infestation bioassay tests, conducted by placing controlled densities of EAB eggs on propagated clonal replicates of lingering green ash and then determining the fate of larvae when dissected 8 weeks post-infestation, revealed reproducible capabilities of hosts to kill EAB larvae (referred to as host-killed larvae) in most of the clonal lingering green ash selections tested (Koch et al., 2015). The reproducibility of this “proportion of host killed larvae” phenotype, detected in healthy grafted clones from lingering ash identified in forest plots, provided the first indication that the capacity to kill EAB larvae in a select group of host trees has a genetic basis (Mason et al., 2023).
Genetic variation in the profiles of plant-specialized metabolites occurs within species and even between local populations of the same species (Mithöfer and Boland, 2012; Chen et al., 2015; Gloss et al., 2022). Investigations on the role of specialized metabolites as defenses against EAB have focused on comparing cultivars and a limited number of open-pollinated seedlings from Fraxinus spp. to the naturally resistant but not immune Asian F. mandshurica cultivar ‘Mancana’ Fraxinus sect. Fraxinus (Whitehill et al., 2012). Application of methyl jasmonate in the white ash (F. americana L. Fraxinus sect. Melioides) cultivar “Autumn purple” increased the concentration of the phenylethanoid glycoside verbascoside by over 170% and suppressed EAB larval development (Whitehill et al., 2014). These studies collectively proposed a positive association between lignan glycosides and host resistance, particularly pinoresinol and verbascoside, and suggested a role for secoiridoid glycosides (Villari et al., 2016).
Many specialized metabolites, including secoiridoids, are also implicated in the response of European Ash (F. excelsior L. Fraxinus sect. Fraxinus) to ash dieback disease (ADB), causal agent Hymenoscyphus fraxineus (Baral et al., 2014). High abundances of specific secoiridoids were associated with tolerant genotypes in one study (Nemesio-Gorriz et al., 2020) and with highly susceptible genotypes in another (Sollars et al., 2017). Both groups of investigators proposed that the different levels of secoiridoids result from differential transcriptional regulation among individual trees. Similarly, investigations of resistance mechanisms in conifers have shown that some trees utilize specialized metabolite-based constitutive and induced defensive responses against wood-boring insects (Volf et al., 2015; López-Goldar et al., 2018, 2019). The abundance and profiles of specific plant specialized metabolites strongly predict resistance to the pine weevil (Hylobius abietis L.) in maritime pine (Pinus pinaster Aiton) after accounting for genetic relatedness among the host trees (López-Goldar et al., 2018). Other investigations in pine have shown that the response consists of altered synthesis rates for existing metabolites rather than the synthesis of unique compounds (Raffa and Smalley, 1995; López-Goldar et al., 2018).
The phenotypes observed in the first clonal replicates of lingering ash and susceptible ash trees under controlled larval infestations and uniform growing conditions showed that while many susceptible trees kill a few EAB larvae, lingering ash trees consistently kill more EAB larvae (Koch et al., 2015; Kelly et al., 2020). In this investigation, we combined untargeted metabolomics and intensive phenotyping on three F1 families, using an experimental design that minimizes the confounding effect of age, environment, and genetic background to investigate the response of full-sibling progeny from crosses using parents verified as lingering ash and infestation bioassays. Our design included untargeted metabolomics to investigate the chemotypes of uninfested compared to infested individuals and trees that killed the most larvae compared to those that killed the fewest, all within the same family. This investigation provides the first evidence for an induced response to EAB infestation and the first evidence for chemotypic differences associated with host response to EAB in a controlled genetic background.
Green ash is one of the most wide-ranging species in the Melioides section of the genus Fraxinus, the group of species native only to the Americas. Green ash is strictly diecious, each tree bearing only pistillate (female) or staminate (male) flowers. As trees in the Melioides section of the Fraxinus genus are sexually incompatible with the Asian Fraxinus section Fraxinus, the strong defenses of the Asian ash against EAB cannot be transferred into green ash using traditional hybrid breeding strategies. The lingering green ash parents used in this investigation were initially selected in the forest based on two criteria: (1) a healthy canopy at least 2 years after the mortality rate of the stand exceeded 95 percent, and (2) a minimum diameter at breast height (DBH, 1.37 m above ground) of 26 cm, indicating that the tree was over the minimum size preferred by EAB when the infestation was at peak levels (Koch et al., 2015). These “lingering ash” trees show evidence of less severe emerald ash borer infestation compared to susceptible phenotypes in the forest, often accompanied by evidence of vigorous wound healing, and maintaining a healthy crown for years after local conspecifics have died (Knight et al., 2012; Steiner et al., 2019). Over the last 14 years, individuals meeting these criteria have been clonally propagated through grafting and subjected to greenhouse bioassays that provided evidence of the ability of some selected lingering ash trees to mount defensive responses against EAB (Koch et al., 2015). Although there is evidence of multiple types of host defenses in Fraxinus (e.g., non-preference), this work focuses on assessing host defenses that result in larval mortality. Clonal replicates of lingering green ash genotypes, some used as parents in this study, consistently kill more larvae than the susceptible green ash controls (Koch et al., 2015).
All individuals in this investigation were generated by controlled pollination to produce full-sibling families of known parentage. Cross-pollination in green ash is only possible between trees of different sexes, and even then, not all crosses are successful. The tactical result was a limited selection of full-sibling progeny from parents known to be susceptible or lingering. A male ‘Summit’ tree was the susceptible parent for the control Family C. ‘Summit’ is a cultivar first marketed in 1957 by Summit Nursery in Stillwater, Minnesota, and was widely used as a street and park tree before the introduction of EAB. ‘Summit’ is susceptible to EAB in these environments. It was also susceptible in infestation bioassays and resulted in a proportion of host-killed larvae value of 0.08 (n = 30 ramets) (Mason et al., 2023). The female parent in Family C, Pe-97, was a field selection from a forest where EAB had yet to reach peak infestation. The lingering ash parents (Pe-53, Pe-56, and Pe-59) used in this investigation survived the initial EAB invasion, the buildup of EAB populations, and the peak infestation. The raw mean proportions of host-killed larvae for the lingering ash parents were 0.28 for Pe-53, 0.06 for Pe-56, and 0.10 for Pe-59 (Mason et al., 2023). Infestation bioassays were conducted on the parent trees in different years while calibrating EAB egg density, but ‘Summit’ was used as a susceptible control in most experiments allowing relative comparison (Mason et al., 2023). Although the proportion of host-killed larvae for Pe-56 was low, surviving larvae were typically smaller than larvae from susceptible parents. Crosses between lingering ash parents were made as part of a planned crossing design to estimate heritability. The two largest lingering ash families, Pe-Y from the cross Pe-53 x Pe-56 (Family Y) and Pe-Z from the cross Pe-53 x Pe-59 (Family Z), were selected for metabolomics experiments to take advantage of the largest pool of segregating individuals. The female parent is written first. The progeny individuals produced by these three crosses were reared in an outdoor growing area until 2 years of age, then transferred into an environmentally controlled greenhouse in the treatment year to allow acclimatization prior to the EAB infestation bioassay. At the time of the infestation bioassay, the trees were 1.5 to 2 meters tall, a height typical for green ash of this age in our growing conditions. A total of 83 trees were subjected to the infestation bioassay. We assayed 30 of the 42 trees in family Y, and set aside the remaining 12 trees as uninfested controls. We assayed 30 of 35 trees in family Z and set aside 5 trees as uninfested controls. We included 17 of the 23 assayed trees from family C and four uninfested controls in this investigation. We performed untargeted metabolomics on these 77 infested trees and 21 uninfested trees (98 trees total).
Emerald ash borer eggs were prepared as previously described (Koch et al., 2015), and twelve eggs were applied to each tree at a density of 400 eggs per square meter (Kelly et al., 2020) during June-August 2019. Individual eggs on filters were affixed to the stem beginning at the bottom and spaced at 400 per m2 until the stem diameter reached the minimum (1.0 cm) or a total of 12 eggs, whichever came first (Figure 1). The minimum number of eggs applied was nine on four trees of smaller stem diameter due to variability in the size of trees at 2 years of age. Egg hatch and EAB entry holes were marked at 3 weeks, and larval galleries were dissected 8 weeks after eggs were applied, starting at the entry hole and following the gallery path to determine the status of each larva. The 8-week interval was chosen based on the time it typically takes for newly hatched larvae to reach the final instar before pupation in susceptible trees. Recovered larvae were designated as alive, host-killed (killed by a host defense response), or killed by parasitism, cannibalism, or disease as characterized and described in detail in other studies (Duan et al., 2015, 2017; Kelly et al., 2020; Mason et al., 2023). Galleries of host-killed larvae end in callous or browning, and larvae are not parasitized, cannibalized, or diseased (Duan et al., 2015, 2017; Koch et al., 2015). Parasitoid insects are visible on or in parasitized EAB larvae. Cannibalized larvae are characterized by intersecting larval galleries with only one gallery continuing and uneaten larval parts (e.g., only the head or posterior of one larva) in the terminated gallery. Larvae with undetermined deterioration (black, shriveled insect body) are rarely found and are coded as diseased. The proportions of host-killed larvae, first or second instar (L1 + L2) larvae, and third or fourth instar (L3 + L4) larvae were calculated based on the number of larvae in each category divided by the total number of larvae that hatched and entered the tree (coded as GoodEgg). Larval instar stage was determined based on head capsule width and total body length (Chamorro et al., 2012). Average larval weight was determined based on the weights of the live larvae recovered intact (occasionally, live larvae were damaged and not weighed).
Figure 1. Infestation bioassay sequence. (A) Eggs were applied individually at a standardized density when the progeny trees were 2 years old, (B) close up of egg adhering to the coffee filter, (C) close up of the chorion of a “good egg” that had hatched and larva had entered the tree taken 3 weeks after application to the tree. The chlorion is broken to reveal frass and an exit hole in the coffee filter below the egg. The entry hole to the tree would be marked on the bark immediately below for reference when dissection begins 8 weeks after egg application. (D) Larval disection 8 weeks after egg application. Galleries are dissected and followed until the larva is located alive (left panel), or the gallery terminates with host defense reactions present (right panel) such as gallery termination with browning and callous (not all outcomes shown, see text).
We tested differences in family means for the response variable proportion host-killed larvae by a fitting generalized linear model with family as a fixed effect (logit link function) and compared differences in least squared means with a Tukey adjustment for multiplicity (SAS 9.4, PROC GLIMMIX) (Supplementary Figure 1). We used the same approach for the response variable average larval weight (with a lognormal link function). Based on the distribution of phenotypes within families for the response variable proportion of host-killed larvae (total number of host-killed larvae/GoodEgg) (Figure 2), we designated the phenotypic class of individual progeny in the lowest quartile of percent host-killed larvae as low host-killed (LHK), and individual progeny in the highest quartile of percent host-killed larvae as high host killed (HHK). As a comparison, the resistant Asian ash (F. mandshurica sect. Fraxinus Oleaceae) typically kills 80–90% of EAB larvae when tested with the infestation bioassay (Koch et al., 2015).
Figure 2. Proportion of EAB larvae killed by host defenses in Family C (one unselected and one susceptible parent) and Families Y and Z (lingering ash parents) when sampled 8 weeks post infestation. Means for Families Y and Z were both highly significantly different from the mean for Family C (p < 0.001) and means for Families Y and Z were not different from each other (p = 0.488) as assessed using GLMM (see text for model and LS means). The statistical analysis was performed on 93 infested trees, 30 trees each from families Y and Z and 33 from family C. The data shown are for the 77 infested trees used for untargeted metabolomics.
All infested and uninfested trees were destructively sampled 8 weeks after infestation with EAB eggs to assess host defense phenotype and collect tissue for metabolite analyses. A 1.5 cm section of each stem, beginning 2.5 cm above the highest EAB larval galleries, was collected and stored immediately on dry ice before being transferred to −80°C storage. This ensured the collection of the vascular cambium, the cork cambium, the phloem, and the ray parenchyma. All samples were ground under liquid nitrogen in a Spex® SamplePrep freezer mill (Metuchen, NJ, USA) and stored at −80°C before extraction.
For each sample, 1 g of frozen powdered plant tissue was extracted in 10 ml of acetonitrile/isopropanol/water (3:3:2) containing 1.00 μM telmisartan (internal standard, Sigma-Aldrich) and 0.01% formic acid in 15-mL polypropylene Falcon tubes and incubated in the dark at 4°C for 24 h. Samples were then centrifuged at 4°C and 10,000g for 10 min, supernatants were transferred to fresh tubes, and 50:1 diluted aliquots were prepared by adding deionized water. An additional aliquot of undiluted extracted sample was archived at −80°C.
Ultra-high-performance liquid chromatography-high resolution mass spectrometry (UHPLC-MS) analyses were performed using a Shimadzu LC-20AD ternary pump coupled to a SIL-5000 autosampler, column oven, and Waters Xevo G2-XS QTof mass spectrometer equipped with an electrospray ionization source. The mass spectrometer operating parameters for the positive-ion mode analyses are as previously described (Lybrand et al., 2020). A 10 μL volume of each diluted extract was analyzed using a 20-min gradient method on an Ascentis Express C18 UHPLC column (2.1 × 100 mm, 2.7 μm) with mobile phases consisting of 10 mM ammonium formate in water, adjusted to pH 2.8 with formic acid (solvent A) and acetonitrile (solvent B). The 20-min method gradient was as follows: 1% B at 0.00 to 1.00 min, then step to 5% B at 1.01 min, linear gradient to 25% B at 8.00 min, then a linear gradient to 75% B at 12.50 min, another linear gradient to 98% B at 15.00 min, and a hold at 98% B until 18.00 min, a step to 1% B at 18.01 min, and a hold at 1% B until 20.00 min.
Analyte extracts were injected in a randomized order while process blank and quality control samples were injected at regular intervals beginning with a process blank and then alternating between quality control standards and blanks following each group of 10 samples, then finishing with a quality control sample and a blank at the end of the run. All calculated peak areas were normalized to the peak area for the internal standard telmisartan utilizing Progenesis QI v2.4 software (Non-linear Dynamics Ltd., Newcastle, UK). Oleuropein, apigenin, and salidroside standards were purchased from MilliporeSigma (St. Louis, United States), dissolved in the extraction solvent, and analyzed at 5 μg/mL to provide analytic confirmation and validation.
We produced untargeted metabolite profiles from acetonitrile:isopropanol:water extractions of ash tissues using UHPLC-MS. The levels of metabolites were normalized to a constant internal standard, with a constant mass of tissue extracted. For untargeted metabolomic analysis, data were initially processed using Progenesis Ql software. Leucine enkephalin lockmass correction (m/z 556.2766) was applied during data importation, and all analyses were aligned to retention times of a pooled QC sample run automatically selected by the software from a selection of QC samples. Peak selection and deconvolution were conducted as previously described (Sadre et al., 2016). Abundances of each metabolite feature were normalized to signals of the internal telmisartan standard (m/z 515.2448). After deconvolution, 1,278 compound ions remained (Figure 3). To remove metabolite features from the dataset introduced by solvents, glassware, or instrumentation and to remove lipids, several filters were applied to the 1,278 compound ions remaining after deconvolution. This was achieved by removing compounds with the highest mean abundance in process blank samples, maximum abundance less than 0.01% of the most abundant compound in the dataset, or retention times greater than 16 min. These selection criteria reduced the total number of metabolic features to 323. This number was reduced to 147 features through manual resolution of multiple adducts and fragments from individual features (Supplementary Table 1). Further analysis and statistical comparisons of compound signals extracted by Progenesis QI software were executed using EZinfo v3.0.2 software (Umetrics®, Umeå, Sweden).
Figure 3. Data processing schematic. Flowthrough of the untargeted metabolomics workflow beginning with data generation, and highlighting the number of metabolite features at each stage of the analysis.
To test for differences in the quantity of each metabolite feature, we applied one-way analyses of variance (ANOVA) tests for each family separately, and with infestation status plus phenotypic class (Uninfested (UNI), Low Host-killed larvae (LHK), and High Host-killed larvae (HHK)] as the main effect (Figure 3). Post hoc contrasts were used to assess the significance between infestation and phenotypic classes within families using the seven following contrasts: Family C: UNI vs. LHK; Family Y: UNI vs. LHK, UNI vs. HHK, LHK vs. HHK; Family Z: UNI vs. LHK, UNI vs. HHK, LHK vs HHK. ANOVA fit was assessed by examining residuals plots. Significant features (p < 0.05) were included in pairwise orthogonal partial least squares discriminant analysis (OPLS-DA) (Figure 3, Supplementary Figure 2, and Supplementary Table 2). OPLS-DA analyses, as implemented in EZinfo, were performed using Pareto scaling. To prevent overfitting of our model, we performed a threefold cross-validation on our data and took the averages in classification outcomes as metrics of the performance of our model. For all metabolic features extracted with Progenesis QI and used in downstream statistical analyses, spectra were processed using MassLynx v4.2 software (Waters Corporation, Milford, MA, United States) as previously described (Sadre et al., 2016).
We generated electrospray ionization tandem mass spectra (ESI-MS/MS) for these metabolite features detected in our analyses and annotated them based on theoretical molecular formulae that match the measured mass and isotopic distribution of the compounds detected (“elemental composition method” in Progenesis Q), comparison of MS/MS databases available using progenesis QI (ChemSpider and Metlin), and comparisons with other MS/MS databases including the Massbank of North America, along with published literature and purchased standards (Sidda et al., 2020). The annotation confidence is labeled according to the recommendations of the Metabolomics Standards Initiative (MSI) (Sumner et al., 2007). Of the metabolites considered, the 31 that had significance (p < 0.05) in more than one family were annotated, as well as salidroside, which was higher in HHK than LHK progeny in Family Y (32 metabolites total) (Figure 3 and Supplementary Table 3).
A total of 952 eggs were applied in this experiment, only thirteen of which (1.4%) produced larvae that were killed by means other than the host response (Table 1 and Supplementary Table 4). Least square family means and mean standard error for the proportion of host-killed larvae were 0.152 ± 0.022 (Family C), 0.442 ± 0.026 (Family Y), and 0.441 ± 0.027 (Family Z) (Supplementary Figure 1). Differences in family least squares means were highly significant for the comparison of Family Y vs. Family C (adjusted p < 0.001) and the comparison Family Z vs. Family C (adjusted p < 0.001). Least square family means for Family Y and Family Z were not significantly different from each other (adjusted p = 0.488). Models were well fitted as assessed by plots of studentized residuals (Supplementary Figure 1). Progeny in all three families were distributed over a range of values for the response variable proportion of host-killed larva (Figure 2). The differences among family least squares means for the response variable larval weight (Lwt) were not significant for any comparison: Family Y vs. Family C (adjusted p = 0.99), Family Z vs. Family C (adjusted p = 0.403), Family Y and Family Z (adjusted p = 0.439).
Table 1. Results of the emerald ash borer (EAB) infestation bioassay consisting of a uniform egg density on each individual in family C, family Y, and family Z, and evaluated 3 and 8 weeks after egg application.
Untargeted metabolite profiling using UHPLC-MS revealed complex chemotypes with low deviation. The levels of metabolites were normalized to a constant internal standard, with a constant mass of tissue extracted. After deconvolution, 1,278 metabolite features were observed, which was reduced to 147 metabolite features for downstream analysis, as detailed above (Figure 3). The median relative standard deviation (RSD) of these metabolite features was 29.8% in pooled controls (Supplementary Figure 3).
The relationship of metabolites to infestation status [uninfested (Uni)] and phenotypic class [low host-killed larvae (LHK), high host-killed larvae (HHK)] within each family was assessed by ANOVA followed by post hoc contrasts for 147 metabolite features (Figure 3 and Supplementary Table 1). All 147 metabolite features were significant (p < 0.05) in at least one contrast, with 9 to 49 metabolite features significant for different contrasts (Figure 3 and Supplementary Table 1). The greatest number of significant metabolite features were identified in Family Y, and more metabolite features were identified in infested compared to uninfested progeny. There were fewer significant differences in the number of metabolite features between infested progeny with low host-killed larvae vs. high host-killed larvae within each family.
Following the identification of significant metabolite features, we used orthogonal partial least squares-discriminant analyses (OPLS-DAs) (Lee et al., 2018) to examine the metabolite features’ ability to accurately classify the host-killed larvae phenotype (high or low proportion of host-killed larvae) in each pairwise comparison (Figure 4 and Supplementary Table 1). To prevent overfitting, we performed a three-fold cross-validation on our data and reported the average prediction accuracies as the performance of our classification model. More than 70% of individuals were assigned correctly to the host-killed larvae phenotype class, with the majority of other individuals being unclassified but not incorrectly assigned (Figure 4A and Supplementary Table 1). The OPLS-DAs for the entire data set showed R2Y and Q2 values above 50% and within 20% of each other, indicating good classification performance (Bevilacqua and Bro, 2020). A specific example is the OPLS-DA for Family Y for the classes HHK and LHK, showing R2Y and Q2 values of 70 and 59%, respectively (Figure 4B).
Figure 4. Summary of model results classifying individual host trees by infestation status (UNI) and phenotypic class (high host-killed larvae HHK, or low-host killed larvae LLK). (A) Orthogonal partial least squares projection to latent square discriminate analysis (OPLS-DA) model performance, averaged across triplicate prediction models. The graph indicates the percentage of individuals classified correctly, incorrectly, or unable to be classified by each model. (B) OPLS-DA model utilizing all test samples in a comparison of high vs. low host-killed larvae. R2Y is the explained variation. Q2 is the model predictive ability. Approximate groupings are visualized by color circles.
We annotated 32 metabolite features, including the 31 that had a significant p-value in more than one family’s comparisons and an additional metabolite feature (the phenylethanoid glycoside salidroside) previously identified as important for EAB defense (Chakraborty et al., 2014). Our annotations included compounds from a wide variety of chemical families, including the first detection of specialized alkaloid metabolites in green ash tissue: secoiridoid glycosides, secoiridoids, and phenolic glycosides (Figures 5, 6, Supplementary Figure 6, and Supplementary Table 2).
Figure 5. Metabolite Annotations. MS/MS spectra in positive ion mode support annotations of metabolite structures: (A) product ions of m/z 642.24 ([M + NH4]+) for verbascoside, (B) product ions of m/z 271.06 ([M + H]+) for apigenin, (C) product ions of m/z 584.21 ([M + NH4]+) for Excelside A.
Figure 6. Chemical families of annotated compounds. (A) Proportions of the chemical families in the annotated metabolites. (B) Summary of pairwise comparisons for specific compounds. “Number” is metabolite number (Supplementary Table 2). LHK v UNI, HHK v UNI, HHK v LHK indicates pairwise comparisons between high (HHK) or low host-killed larvae (LHK) phenotypes or uninfested (UNI) individuals. C, Y, Z refer to full-sibling families Family C (susceptible parents), Families Y and Z (lingering ash parents) (Figure 1). Box with letter indicates the phenotypic category that had significantly higher concentration of the indicated metabolite (p < 0.05, L in red LHK, H in gray HHK, U in blue UNI). Annotated metabolites 1–6 are alkaloids, 9–17 are secoiridoid glycosides, 18–20 are secoiridoids, 24 and 25 are the phenylethanoid glycosides salidroside and verbascoside, respectively.
The alkaloids noted appeared to belong to three main subgroups. Alkaloids 1 (C12H17NO4) and 2 and 3 (both C14H19NO5) shared key MS/MS product ions of m/z 196.09, 146.06, and 118.07 (Supplementary Figures 4, 6 and Supplementary Table 3), which had even masses typical of an odd (non-zero) number of nitrogen atoms, and were elevated in HHK compared to LHK individuals. Based on inspection of extracted ion chromatograms, we cannot rule out the possibility that alkaloids 2 and 3 are a single compound exhibiting split chromatographic peaks. The low hydrogen/carbon ratios for these suggest they may be aromatic, perhaps indole-derived alkaloids, but searches of MS/MS databases available yielded no matches to known substances. These nitrogen-containing metabolites had low relative abundances and were not purified for NMR characterization during this experiment. Putative Alkaloid 4 (C25H30N6O11) had a significant fragment at m/z 232.07, did not share similar peaks with other alkaloids, and was at lowest abundance in HHK individuals. Putative alkaloids 5 (C25H34N4O8) and 6 (C25H32N4O7) also appear to share a similar base structure, both yielding MS/MS product ions at m/z 114.10 and 97.07 (Supplementary Figure 6) and were at higher abundances in UNI individuals compared to HHK individuals.
Among the 31 multi-family features, we annotated 16 secoiridoids and secoiridoid glycosides with structures similar to previously observed compounds (Supplementary Figures 7, 8 and Supplementary Table 2), as compared to the 28 seen in leaves of Fraxinus excelsior (Sidda et al., 2020). We found that among these 16 annotated compounds, six were elevated in both LHK and HHK individuals compared to UNI individuals, an additional five were elevated in LHK compared to UNI individuals, and five of the 16 were elevated in LHK compared to HHK individuals (Figure 5B and Supplementary Figures 3, 6, 7). An additional three secoiridoid glycosides were noted in our study without any multi-family patterns (Supplementary Figure 7).
Secoiridoid 20 (m/z 569.23) was elevated in all infested comparisons (LHK v UNI, HHK v UNI) across all families. Another two secoiridoid glycosides, Nueznehide isomer 2 (m/z 704.2781) and GL5 (m/z 928.3429), previously found in higher abundances in trees that are highly susceptible to ash dieback in previous investigations (Sollars et al., 2017; Sidda et al., 2020), were significantly higher in LHK compared to UNI individuals across families (Supplementary Figure 4). Specific isomers had different patterns and were present at different abundances. For example, Nuzhenide isomer 2 was a more reliable predictor of infestation than Nuzhenide isomers 1 & 3. The phenylethanoid glycosides verbascoside and salidroside were not consistently associated with phenotype class. Verbascoside was highest in LHK individuals while having no detectable increase in HHK individuals compared to UNI individuals. Conversely, Salidroside was observed at a higher abundance in HHK compared to LHK individuals in family Y. The only compounds that were higher in HHK individuals compared to LHK individuals were three compounds annotated as aromatic alkaloids 1-3 and the phenylethanoid glycoside salidroside [Figure 6B (numbers 1–3 and 24), Supplementary Figure 4, and Supplementary Table 2].
We investigated the response of select green ash individuals to EAB infestation using segregating F1 families, a reproducible phenotyping method, and an untargeted metabolomics approach. We minimized the environmental variance by growing the F1 families from seed at the same time and testing them at the same age, in the same greenhouse, and in the same growing season. The results of the infestation bioassays show that the progeny of the lingering ash parent crosses killed significantly more larvae on average, but the average larval weight of the survivors was not different between the two lingering ash progeny families (Family Y and Family Z) and the susceptible Pe-97 x ‘Summit’ family (Family C). The ability of the host to kill a high proportion of early instar larvae may contribute to the survival of the trees, even though some surviving larvae reach the last instar. In similar EAB infestation bioassays conducted on different ash species, Kelly et al. (2020) found that some species had no L4 larvae; however, none of the species had 100% host-killed larvae, indicating that none of the hosts were immune to EAB. The results presented here are also consistent with observations in China, where Asian Fraxinus species are not entirely immune to EAB but can survive attack and are rarely killed by EAB unless stressed or weakened (Liu et al., 2003; Wei et al., 2004). We focused on the response variables host-killed larvae and larval weights because the former was the sole criterion used for the phenotypic contrast HLK vs. LLK and the latter, essentially the combined weights of large larvae, showed that the response we investigated was not immunity in the sense of the capacity to prevent biological attack. The other connotation of immunity, the capacity to withstand a biological attack, usually implies a set of operational responses that result in categorial phenotypes, which we certainly did not observe in this investigation (Miller et al., 2017).
In both green ash and Manchurian ash, healthy trees marshal sufficient defensive responses to survive the presence of native stem borers. Green ash are infested by the native stem borers Podosesia syringae Harris and P. aureocincta Möschler (Lepidoptera: Sesiidae), damaging the value of the wood but rarely killing the tree (Solomon, 1993). While these clearwing moths belong to a different order of insects than EAB (Coleoptera: Buprestidae), the larvae of all three attack phloem tissues. Manchurian ash are infested by EAB, with only a few insects managing to mature and reproduce (Dang et al., 2022). Only when a Manchurian ash tree is severely damaged or drought-stressed can EAB infestation kill the tree, similar to the mortality seen from native borers on green ash in North America. The shared common ancestry of the Asian ash Fraxinus section Fraxinus which includes the North American black ash and the North American Fraxinus section Meliodies may have resulted in similar regulatory networks and structural compounds useful for generalized defense against phloem feeders. Genetic diversity for defensive responses against native insects and pathogens could translate to genetic diversity for a measure of defensive responses against non-native insects and pathogens. The existence of native individuals with effective defensive responses to a pathogen with which the host has not recently evolved is well illustrated in the literature. Breeding programs based on native resistance to non-native invaders have produced American beech (Fagus grandifolia Ehrh.) resistant to beech bark disease (disease agent Neonectria spp. transmitted by the scale insect Cryptococcus fagisuga Lindinger, 1936 (Koch and Heyd, 2013). In the gymnosperms, recent examples include eastern white pine (Pinus strobus L.) resistant to white pine blister rust (disease agent Cronartium ribicola J.C. Fisch.) (Pike et al., 2018; Sniezko and Liu, 2022) and Port Orford cedar [Chamaecyparis lawsoniana (A.Murray bis) Parl.] resistant to a root rot oomycete pathogen [disease agent Phytophthora lateralis Tucker & Milbrath, (1942)] (Sniezko et al., 2012). The success of these and other programs demonstrate that heritable resistance to invasive pests and pathogens can exist in native populations.
The distribution of the response variable proportion of host-killed larvae is expected when the parents are heterozygous for multiple loci with additive effects (Hill and Mackay, 2004). Although the results of our investigation are consistent with a multigenic model, a rigorous test of this hypothesis will require additional families and multiple generations. The action of multiple genes is also suggested by the 53 candidate genes identified in comparative genomics studies of Fraxinus species with differing responses to EAB (Villari et al., 2016; Kelly et al., 2020).
The chemotypes of infested HHK and LHK individuals were distinguishable based on the relative abundances of select metabolites (Figure 6 and Supplementary Tables 1–3), not their presence or absence. We provide an initial annotation of metabolites for further study, including secoiridoids and three putative aromatic alkaloids. This first detection of these alkaloids in green ash suggests a potential role for specific alkaloids in defense against herbivory in the Meliodes. Comparisons of the chemotypes of infested vs. uninfested individuals across families revealed that secoiridoids are associated with a response to infestation that does not predict effective defensive responses. Our data suggest that these specific secoiridoids and verbascoside may indicate a general defensive response. A review of constitutive metabolic differences among Fraxinus species suggested that secoiridoid compounds have a role in response to EAB, but these studies did not compare infested and uninfested individuals within the same species (Villari et al., 2016). This association of secoiridoids with a response to infestation is consistent with previous studies that suggested that infested trees or trees artificially stressed with methyl jasmonate produced higher amounts of these metabolites than uninfested trees (Whitehill et al., 2014). While high abundances of specific secoiridoids in F. excelsior are proposed to be indicative of tolerance (Nemesio-Gorriz et al., 2020) or susceptibility to ash dieback (Sollars et al., 2017) and were predicted to provide a future robust reservoir of anti-feeding deterrents to EAB (Sidda et al., 2020), our data does not support the hypothesis that secoiridoids play a significant role in defense against EAB larval infestation in green ash, but their broad scale induction under infestation may be effective against other threats.
The four metabolites, annotated as three aromatic alkaloids and salidroside that were significantly elevated in HHK individuals compared to LHK or uninfested individuals, require evaluation in other studies to confirm whether these metabolites are part of an effective induced response to EAB in lingering ash.
Identification of secoiridoids and alkaloids at different quantities in different phenotypic classes within family provides the first evidence that the lingering ash phenotype may result from a rare but partially effective shift in the regulation of a number of genes. These compounds are potential candidates for biomarkers of infestation or effective responses to EAB, in addition to those identified using comparative genomics (Kelly et al., 2020) and transcriptomics approaches (Chiu et al., 2023). The results of this investigation support the continuance of breeding programs that, in concert with biocontrol and management, could provide trees that are resilient to EAB and help conserve the Fraxinus gene pool while ongoing work on host-insect interactions will contribute to our understanding of how forest trees recognize and defend themselves against stem-boring insects.
The original contributions presented in this study are publicly available. The data can be found in the MetaboLites data repository, accession number MTBLS7999.
JR-S, JK, and RS conceived and designed the work. AJ made the substantial contributions to the conception and the design of the work. JK, TP, DC, MM, and RS coordinated the activities between Delaware facility, the AJ lab, the TP lab, and the JR-S lab. JK, DC, and MM selected the lingering ash and designed and carried out the breeding plan. MM performed the statistical GLMM statistical analyses. The TP lab provided the EAB eggs for the bioassay. TP with JK, DC, and MM developed and refined the EAB infestation bioassay protocols. DC, AD, JW, and MM provided the plant care and executed the infestation bioassay. DC, MM, and RS performed the stem dissections. RS performed the metabolomic analyses and analyzed the data with support from AJ. RS and JR-S wrote the manuscript. AJ, TP, MM, KO, and JK assisted with the manuscript revision and final editing. All authors have seen and approved the final submission.
JR-S acknowledges support from USDA-USFS APHIS grants 18-IA-11242316-105 and 20-JV-11242303-050 and the Tree Fund Foundation, Tree Fund grant 18-JD-01. RS acknowledges support from NIH training grant T32GM075762. JK acknowledges support from USDA APHIS 18-IA-11242316-105, Michigan Invasive Species Grant Program grant IS18-119, the Commonwealth of Pennsylvania Department of Conservation and Natural Resources Bureau of Forestry 18-CO-11242316-014, and the U.S. Forest Service Special Technology Development Program grant NA-2017-01. AJ acknowledges also support from Michigan AgBioResearch through the USDA National Institute of Food and Agriculture, Hatch project number MICL02474, and USDA-USFS grant 20-JV-11242303-050.
We thank Warren Chatwin, Benjamin Gombash, and Christina Murray for their helpful comments on the manuscript and Gavin Nupp, Miranda McKibben, and Jarod Sanchez for their work propagating and maintaining the study trees and assistance in conducting the ash resistance EAB infestation bioassays. We also thank Patrick Cunniff, Brandon Chou, Declan Thanner, and Julie Huston for assistance in collecting and organizing tissue samples and managing logistics.
The authors declare that the research was conducted in the absence of any commercial or financial relationships that could be construed as a potential conflict of interest.
All claims expressed in this article are solely those of the authors and do not necessarily represent those of their affiliated organizations, or those of the publisher, the editors and the reviewers. Any product that may be evaluated in this article, or claim that may be made by its manufacturer, is not guaranteed or endorsed by the publisher.
The Supplementary Material for this article can be found online at: https://www.frontiersin.org/articles/10.3389/ffgc.2023.1166421/full#supplementary-material
Anderegg, W. R. L., Trugman, A. T., Badgley, G., Anderson, C. M., Bartuska, A., Ciais, P., et al. (2020). Climate-driven risks to the climate mitigation potential of forests. Science 368:eaaz7005. doi: 10.1126/science.aaz7005
Aubin, I., Cardou, F., Ryall, K., Kreutzweiser, D., and Scarr, T. (2015). Ash regeneration capacity after emerald ash borer (Eab) outbreaks: Some early results. For. Chronicle 91, 291–298. doi: 10.5558/tfc2015-050
Aukema, J. E., Leung, B., Kovacs, K., Chivers, C., Britton, K. O., Englin, J., et al. (2011). Economic impacts of non-native forest insects in the continental United States. PLoS One 6:e24587. doi: 10.1371/journal.pone.0024587
Baral, H.-O., Queloz, V., and Hosoya, T. (2014). Hymenoscyphus fraxineus, the correct scientific name for the fungus causing ash dieback in Europe. IMA Fungus 5, 79–80. doi: 10.5598/imafungus.2014.05.01.09
Bevilacqua, M., and Bro, R. (2020). Can We Trust Score Plots? Metabolites 10:278. doi: 10.3390/metabo10070278
Bradshaw, C. J. A., Leroy, B., Bellard, C., Roiz, D., Albert, C., Fournier, A., et al. (2016). Massive yet grossly underestimated global costs of invasive insects. Nat. Commun. 7:12986. doi: 10.1038/ncomms12986
Chakraborty, S., Whitehill, J. G., Hill, A. L., Opiyo, S. O., Cipollini, D., Herms, D. A., et al. (2014). Effects of water availability on emerald ash borer larval performance and phloem phenolics of Manchurian and black ash. Plant Cell Environ. 37, 1009–1021.
Chamorro, M. L., Volkovitsh, M. G., Poland, T. M., Haack, R. A., and Lingafelter, S. W. (2012). Preimaginal stages of the emerald ash borer, Agrilus planipennis fairmaire (Coleoptera: Buprestidae): An invasive pest on ash trees (Fraxinus). PLoS One 7:e33185. doi: 10.1371/journal.pone.0033185
Chen, J., Xu, Y., Wei, G., Liao, S., Zhang, Y., Huang, W., et al. (2015). Chemotypic and genetic diversity in Epimedium sagittatum from different geographical regions of China. Phytochemistry 116, 180–187. doi: 10.1016/j.phytochem.2015.04.005
Chiu, C. C., Pelletier, G., Stival Sena, J., Roux-Dalvai, F., Prunier, J., Droit, A., et al. (2023). Integrative analysis of green ash phloem transcripts and proteins during an emerald ash borer infestation. BMC Plant Biol. 23:123. doi: 10.1186/s12870-023-04108-y
Dang, Y., Wei, K., Wang, X., Duan, J. J., Jennings, D. E., and Poland, T. M. (2022). Introduced plants induce outbreaks of a native pest and facilitate invasion in the plants’ native range: Evidence from the emerald ash borer. J. Ecol. 110, 593–604. doi: 10.1111/1365-2745.13822
Duan, J. J., Bauer, L. S., Abell, K. J., Ulyshen, M. D., and Van Driesche, R. G. (2015). Population dynamics of an invasive forest insect and associated natural enemies in the aftermath of invasion: Implications for biological control. J. Appl. Ecol. 52, 1246–1254. doi: 10.1111/1365-2664.12485
Duan, J. J., Bauer, L. S., and Van Driesche, R. G. (2017). Emerald ash borer biocontrol in ash saplings: The potential for early stage recovery of North American ash trees. For. Ecol. Manage. 394, 64–72. doi: 10.1016/j.foreco.2017.03.024
Gloss, A. D., Vergnol, A., Morton, T. C., Laurin, P. J., Roux, F., and Bergelson, J. (2022). Genome-wide association mapping within a local Arabidopsis thaliana population more fully reveals the genetic architecture for defensive metabolite diversity. Philos. Trans. R. Soc. B Biol. Sci. 377:20200512. doi: 10.1098/rstb.2020.0512
Hanberry, B. B. (2014). Rise of Fraxinus in the United States between 1968 and 2013. J. Torrey Bot. Soc. 141, 242–249. doi: 10.3159/TORREY-D-13-00072.1
Herms, D. A., and McCullough, D. G. (2014). Emerald ash borer invasion of North America: History, biology, ecology, impacts, and management. Annu. Rev. Entomol. 59, 13–30. doi: 10.1146/annurev-ento-011613-162051
Hicke, J. A., Allen, C. D., Desai, A. R., Dietze, M. C., Hall, R. J., Ted Hogg, E. H., et al. (2012). Effects of biotic disturbances on forest carbon cycling in the United States and Canada. Glob. Change Biol. 18, 7–34. doi: 10.1111/j.1365-2486.2011.02543.x
Hill, W. G., and Mackay, T. F. C. (2004). D. S. Falconer and Introduction to Quantitative Genetics. Genetics 167, 1529–1536. doi: 10.1093/genetics/167.4.1529
Hoover, K., and Riddle, A. A. (2020). Forest carbon primer. Washington, DC: Congressional Research Service.
Kelly, L. J., Plumb, W. J., Carey, D. W., Mason, M. E., Cooper, E. D., Crowther, W., et al. (2020). Convergent molecular evolution among ash species resistant to the emerald ash borer. Nat. Ecol. Evol. 4, 1116–1128. doi: 10.1038/s41559-020-1209-3
Knight, K. S., Brown, J. P., and Long, R. P. (2013). Factors affecting the survival of ash (Fraxinus spp.) trees infested by emerald ash borer (Agrilus planipennis). Biol. Invasions 15, 371–383. doi: 10.1007/s10530-012-0292-z
Knight, K. S., Herms, D., Plumb, R., Sawyer, E., Spalink, D., Pisarczyk, E., et al. (2012). “Dynamics of surviving ash (Fraxinus spp.) populations in areas long infested by emerald ash borer (Agrilus planipennis),” in Proceedings of the fourth international workshop on the genetics of host-parasite interactions in forestry: Disease and insect resistance in forest trees. Gen. Tech. Rep. PSW-GTR-240, eds R. Sniezko, A. Yanchuk, J. Kliejunas, K. Palmieri, J. Alexander, and S. Frankel (Albany, CA: Pacific Southwest Research Station, Forest Service, US Department of Agriculture), 143–152.
Koch, J., Carey, D., Mason, M., Poland, T., and Knight, K. (2015). Intraspecific variation in Fraxinus pennsylvanica responses to emerald ash borer (Agrilus planipennis). New For. 46, 995–1011. doi: 10.1007/s11056-015-9494-4
Koch, J. L., Carey, D. W., Knight, K. S., Poland, T., Herms, D. A., and Mason, M. E. (2012). “Breeding strategies for the development of emerald ash borer-resistant North American ash,” in Proceedings of the fourth international workshop on the genetics of host-parasite interactions in forestry: Disease and insect resistance in forest trees. Gen. Tech. Rep. PSW-GTR-240, eds R. Sniezko, A. Yanchuk, J. Kliejunas, K. Palmieri, J. Alexander, and S. Frankel (Albany, CA: Pacific Southwest Research Station, Forest Service, US Department of Agriculture), 235–239.
Koch, J. L., and Heyd, R. L. (2013). Battling beech bark disease: Establishment of beech seed orchards in Michigan. Newslett. Michigan Entomol. Soc. 58, 11–14.
Kovacs, K. F., Haight, R. G., Mccullough, D. G., Mercader, R. J., Siegert, N. W., and Liebhold, A. M. (2010). Cost of potential emerald ash borer damage in U.S. communities, 2009–2019. Ecol. Econ. 69, 569–578. doi: 10.1016/j.ecolecon.2009.09.004
Krist, F. Jr., Ellenwood, J., Woods, M., Mcmahan, A., Cowardin, J., Ryerson, D., et al. (2014). National insect and disease forest risk assessment: 2013-2027. Washington, DC: US Department of Agriculture.
Lee, L. C., Liong, C.-Y., and Jemain, A. A. (2018). Partial least squares-discriminant analysis (PLS-DA) for classification of high-dimensional (HD) data: A review of contemporary practice strategies and knowledge gaps. Analyst 143, 3526–3539. doi: 10.1039/C8AN00599K
Liu, H., Bauer, L. S., Gao, R., Zhao, T., Petrice, T. R., and Haack, R. A. (2003). Exploratory survey for the emerald ash borer, Agrilus planipennis (Coleoptera: Buprestidae), and its natural enemies in China. Great Lakes Entomol. 36:11.
López-Goldar, X., Villari, C., Bonello, P., Borg-Karlson, A. K., Grivet, D., Zas, R., et al. (2018). Inducibility of plant secondary metabolites in the stem predicts genetic variation in resistance against a key insect herbivore in maritime pine. Front. Plant Sci. 9:1651. doi: 10.3389/fpls.2018.01651
López-Goldar, X., Villari, C., Bonello, P., Borg-Karlson, A. K., Grivet, D., Sampedro, L., et al. (2019). Genetic variation in the constitutive defensive metabolome and its inducibility are geographically structured and largely determined by demographic processes in maritime pine. J. Ecol. 107, 2464–2477. doi: 10.1111/1365-2745.13159
Lovett, G. M., Weiss, M., Liebhold, A. M., Holmes, T. P., Leung, B., Lambert, K. F., et al. (2016). Nonnative forest insects and pathogens in the United States: Impacts and policy options. Ecol. Appl. 26, 1437–1455. doi: 10.1890/15-1176
Lybrand, D. B., Anthony, T. M., Jones, A. D., and Last, R. L. (2020). An integrated analytical approach reveals trichome acylsugar metabolite diversity in the wild tomato Solanum pennellii. Metabolites 10:401. doi: 10.3390/metabo10100401
Mason, M., Carey, D., Romero-Severson, J., Knight, K., Poland, T., and Koch, J. (2023). Select genotypes of white and green ash exhibit elevated resistance to emerald ash borer and estimates of genetic heritability. New For. (Under review).
Miller, R. N., Costa Alves, G. S., and Van Sluys, M. A. (2017). Plant immunity: Unravelling the complexity of plant responses to biotic stresses. Ann. Bot. 119, 681–687. doi: 10.1093/aob/mcw284
Miniat, C. F., Fraterrigo, J. M., Brantley, S. T., Callaham, M. A., Cordell, S., Dukes, J. S., et al. (2021). “Impacts of invasive species on forest and grassland ecosystem processes in the United States,” in Invasive species in forests and rangelands of the United States, eds T. M. Poland, T. Patel-Weynand, D. M. Finch, C. F. Miniat, D. C. Hayes, and V. M. Lopez (Cham: Springer International Publishing). doi: 10.1007/978-3-030-45367-1_3
Mithöfer, A., and Boland, W. (2012). Plant defense against herbivores: Chemical aspects. Annu. Rev. Plant Biol. 63, 431–450. doi: 10.1146/annurev-arplant-042110-103854
Nemesio-Gorriz, M., Menezes, R. C., Paetz, C., Hammerbacher, A., Steenackers, M., Schamp, K., et al. (2020). Canditate metabolites for ash dieback tolerance in Fraxinus excelsior. J. Exp. Bot. 71, 6074–6083. doi: 10.1093/jxb/eraa306
Pike, C. C., Berrang, P., Rogers, S., David, A., Sweeney, C., and Hendrickson, J. (2018). Improving the resistance of eastern white pine to white pine blister rust disease. For. Ecol. Manag. 423, 114–119. doi: 10.1016/j.foreco.2018.03.001
Poland, T. M., and McCullough, D. G. (2006). Emerald ash borer: Invasion of the urban forest and the threat to North America’s ash resource. J. For. 104, 118–124.
Potter, K., Escanferla, M., Jetton, R., and Man, G. (2019). Important insect and disease threats to United States tree species and geographic patterns of their potential impacts. Forests 10:304. doi: 10.3390/f10040304
Raffa, K. F., and Smalley, E. B. (1995). Interaction of pre-attack and induced monoterpene concentrations in host conifer defense against bark beetle-fungal complexes. Oecologia 102, 285–295. doi: 10.1007/BF00329795
Sadre, R., Magallanes-Lundback, M., Pradhan, S., Salim, V., Mesberg, A., Jones, A. D., et al. (2016). Metabolite diversity in alkaloid biosynthesis: A multilane (diastereomer) highway for camptothecin synthesis in Camptotheca acuminata. Plant Cell 28, 1926–1944. doi: 10.1105/tpc.16.00193
Sidda, J. D., Song, L., Parker, J. L., Studholme, D. J., Sambles, C., and Grant, M. (2020). Diversity of secoiridoid glycosides in leaves of UK and Danish ash provide new insight for ash dieback management. Sci. Rep. 10:19566. doi: 10.1038/s41598-020-76140-z
Sniezko, R., Yanchuk, A., Kliejunas, J., Palmieri, K., Alexander, J., and Frankel, S. (2012). Fourth international workshop on the genetics of host-parasite interactions in forestry: Disease and insect resistance in forest trees, Eugene, Oregon, USA, 31 July-5 August 2011. Eugene, OR: Pacific Southwest Research Station, USDA Forest Service. doi: 10.2737/PSW-GTR-240
Sniezko, R. A., and Liu, J.-J. (2022). Genetic resistance to white pine blister rust, restoration options, and potential use of biotechnology. For. Ecol. Manage. 520:120168. doi: 10.1016/j.foreco.2022.120168
Sollars, E. S., Harper, A. L., Kelly, L. J., Sambles, C. M., Ramirez-Gonzalez, R. H., Swarbreck, D., et al. (2017). Genome sequence and genetic diversity of European ash trees. Nature 541:212. doi: 10.1038/nature20786
Solomon, J. D. (1993). Ash pests: A guide to major insects, diseases, air pollution injury, and chemical injury. New Orleans, LA: US Department of Agriculture, Forest Service, Southern Forest Experiment Station. doi: 10.2737/SO-GTR-96
Steiner, K. C., Graboski, L. E., Knight, K. S., Koch, J. L., and Mason, M. E. (2019). Genetic, spatial, and temporal aspects of decline and mortality in a Fraxinus provenance test following invasion by the emerald ash borer. Biol. Invasions 21, 3439–3450. doi: 10.1007/s10530-019-02059-w
Sumner, L. W., Amberg, A., Barrett, D., Beale, M. H., Beger, R., Daykin, C. A., et al. (2007). Proposed minimum reporting standards for chemical analysis. Metabolomics 3, 211–221. doi: 10.1007/s11306-007-0082-2
Villari, C., Herms, D. A., Whitehill, J. G., Cipollini, D., and Bonello, P. (2016). Progress and gaps in understanding mechanisms of ash tree resistance to emerald ash borer, a model for wood-boring insects that kill angiosperms. New Phytol. 209, 63–79.
Volf, M., Hrcek, J., Julkunen-Tiitto, R., and Novotny, V. (2015). To each its own: Differential response of specialist and generalist herbivores to plant defence in willows. J. Anim. Ecol. 84, 1123–1132. doi: 10.1111/1365-2656.12349
Walters, R., and Yawney, H. (2004). Silvics manual: Volume 2: Hardwoods. Agricultural Handbook 654. Washington, DC: United States Department of Agriculture, Forest Service.
Wei, X., Reardon, D., Wu, Y., and Sun, J.-H. (2004). Emerald ash borer, Agrilus planipennis Fairmaire (Coleoptera: Buprestidae), in China: A review and distribution survey. Acta Entomol. Sin. 47, 679–685.
Whitehill, J. G., Opiyo, S. O., Koch, J. L., Herms, D. A., Cipollini, D. F., and Bonello, P. (2012). Interspecific comparison of constitutive ash phloem phenolic chemistry reveals compounds unique to Manchurian ash, a species resistant to emerald ash borer. J. Chem. Ecol. 38, 499–511. doi: 10.1007/s10886-012-0125-7
Whitehill, J. G., Rigsby, C., Cipollini, D., Herms, D. A., and Bonello, P. (2014). Decreased emergence of emerald ash borer from ash treated with methyl jasmonate is associated with induction of general defense traits and the toxic phenolic compound verbascoside. Oecologia 176, 1047–1059. doi: 10.1007/s00442-014-3082-8
Keywords: secoiridoid, emerald ash borer (Agrilus planipennis Fairmaire), green ash (Fraxinus pennsylvanica), liquid chromatography-mass spectrometry (LCMS), emerald ash borer infestation bioassay, induced host defensive response, Fraxinus pennsylvanica metabolic profiles, host-insect interaction
Citation: Stanley RK, Carey DW, Mason ME, Doran A, Wolf J, Otoo KO, Poland TM, Koch JL, Jones AD and Romero-Severson J (2023) Emerald ash borer (Agrilus planipennis) infestation bioassays and metabolic profiles of green ash (Fraxinus pennsylvanica) provide evidence for an induced host defensive response to larval infestation. Front. For. Glob. Change 6:1166421. doi: 10.3389/ffgc.2023.1166421
Received: 15 February 2023; Accepted: 01 June 2023;
Published: 24 July 2023.
Edited by:
Amit Roy, Czech University of Life Sciences Prague, CzechiaReviewed by:
Jennifer Gene Klutsch, University of Alberta, CanadaCopyright © 2023 Stanley, Carey, Mason, Doran, Wolf, Otoo, Poland, Koch, Jones and Romero-Severson. This is an open-access article distributed under the terms of the Creative Commons Attribution License (CC BY). The use, distribution or reproduction in other forums is permitted, provided the original author(s) and the copyright owner(s) are credited and that the original publication in this journal is cited, in accordance with accepted academic practice. No use, distribution or reproduction is permitted which does not comply with these terms.
*Correspondence: Jeanne Romero-Severson, anJvbWVyb3NAbmQuZWR1
Disclaimer: All claims expressed in this article are solely those of the authors and do not necessarily represent those of their affiliated organizations, or those of the publisher, the editors and the reviewers. Any product that may be evaluated in this article or claim that may be made by its manufacturer is not guaranteed or endorsed by the publisher.
Research integrity at Frontiers
Learn more about the work of our research integrity team to safeguard the quality of each article we publish.