- USDA Forest Service, Northern Research Station, Durham, NH, United States
The present study compared seasonal changes in the concentrations of calcium oxalate (CaOx) crystals and total calcium (Ca) in the foliage of red spruce (Picea rubens Sarg.), white pine (Pinus strobus L.), black oak (Quercus velutina L.), and sugar maple (Acer saccharum Marshall) trees. Samples were collected from the same four replicate trees of each species starting in June 2014 through September 2015 for a total of six times for conifers and four times for hardwoods. Calcium oxalate was extracted from tissues using a method developed in our laboratory in 2015. The purity of the extracted CaOx was indicated by an r2 of 0.98 between Ca and oxalate (Ox) for the data pooled across all species and all sampling times. As expected, the concentrations of CaOx varied between species. We hypothesized that the only role of CaOx crystals is to bind excess Ca, so based on this hypothesis the concentrations of CaOx would increase over the growing season both in conifer and hardwood trees, and in conifers, its quantities would be higher in the older relative to the younger needles. However, we found, that for most species, CaOx concentrations were not significantly different from each other for all collection times. In addition, relative to total Ca, the percent of Ca that existed in the form of CaOx varied widely with species, time of collection within a species, and needle age. Thus, no specific trend was observed for CaOx accumulations with changes in seasons. Concentrations of CaOx were indeed higher in older spruce and pine needles. Based on the available literature on this topic and our data, this could mean that CaOx amounts are dynamic and are continuously being adjusted according to the metabolic needs of cells for either Ca or Ox while still performing the function of shedding off excess Ca.
Introduction
Calcium oxalate (CaOx) crystals have been observed in all photosynthetic organisms including angiosperms and gymnosperms (Franceschi and Nakata, 2005). These crystals are found as either extracellular or intracellular deposits in different types of plant tissues. In some species, CaOx crystals are known to comprise up to 90% of the plant’s dry mass. Genetics and environment both control the amount, shape, and size of CaOx crystals (Raman et al., 2014 and references therein). In angiosperm leaves, CaOx crystals are primarily present in the vacuoles of special crystal idioblasts. Excess Ca taken up by cells is actively transported into the vacuoles from the cytoplasm where it combines with Ox to make CaOx crystal idioblasts (Franceschi and Horner, 1980). In contrast to angiosperms, conifers often prevent the entrance of excess Ca into the cells and precipitate it outside in the apoplast as CaOx. Although in the leaves of conifers, apoplastic crystallization dominates, at times, CaOx crystals have also been observed in the intracellular spaces in the phloem or xylem parenchyma (Franceschi and Horner, 1980; Fink, 1991 and references therein). Hudgins et al. (2003) observed different patterns of CaOx crystal deposition in the secondary phloem of stem tissues from 46 conifer species belonging to Pinaceae and non-Pinaceae families. Whereas in Pinaceae, crystals were located intracellularly in crystalliferous parenchyma, in non-Pinaceae, crystals were found mainly in extracellular spaces. In red spruce foliage, inferred calcium oxalate crystals were found adhering to the outer walls of collapsed chlorenchyma cells. They were also found embedded in the cuticle of the epidermal cell wall and beneath the epicuticular layer of wax in red spruce (Smith et al., 2009). At present, literature regarding the exact location of CaOx crystals in the leaves of many tree species is scarce.
Only the hydrated forms of crystals are present in living organisms (the anhydrous form only exists as a synthetic compound). Presently there is not much information available on the mechanisms of interconversions of hydration states and the role played by water in the formation of calcium oxalate crystal structures within living plant cells. However, according to Izatulina et al. (2018 and references therein), CaOx crystals isolated from kidney stones existed in three hydrated forms: whewellite (CaC2O4⋅H2O; COM), weddellite (CaC2O4⋅2 H2O; COD and caoxite (CaC2O4⋅3 H2O; COT). Under ambient laboratory conditions, whewellite (CaOx monohydrate) is considered to be the most stable crystalline form. It is the dehydration that occurs either from heating at higher than ambient temperatures and/or changes in temperature over time that causes the transformation of di and tri-hydrate forms to the monohydrate form of CaOx crystals (Izatulina et al., 2018).
Although both Ca carbonate and CaOx are present in plants, CaOx seems to be the more common form for Ca storage due to the multifunctionality of Ox (Karabourniotis et al., 2020). Despite the abundance of CaOx, its role in plants is debatable. Literature suggests that these crystals serve as a bioavailable reserve for both calcium (Ca) and oxalate (Ox) that perform many different metabolic functions within cells (Franceschi and Nakata, 2005; Gaberščik et al., 2020; Gómez-Espinoza et al., 2020; Karabourniotis et al., 2020). Some of these functions include heavy metal detoxification, defense from herbivory, and protection against abiotic and biotic stress. Karabourniotis et al. (2020) found that in response to abscisic acid treatment, CaOx crystals degrade and CO2 is released during the metabolism of Ox. This process supports a low rate of photosynthesis under conditions of drought and carbon starvation that limits carbon losses, lowers the risk of photoinhibition, and conserves water. However, others suggest that although CaOx is a bioavailable source in a few specialized cases, its major function is to shed off excess Ca in the form of CaOx (Paiva, 2019 and references therein). To add to this complexity, the functions and concentrations of CaOx crystals may vary with the species, season, and age of plants (Paiva, 2019 and references therein). Therefore, before more in-depth research can be done on the cellular functions of CaOx in tree species, one needs to gather background information to determine whether their cellular concentrations change through the growing season.
Literature is scarce on the available methods for the fractionation of total Ca into various forms present within cells and their role(s) in metabolism. Our group recently developed a method of sequential extractions to quantitate CaOx from tree foliage extracted in the third fraction with 2N HCl or 5% perchloric acid (PCA) following first extractions with water and second with 2N acetic acid (Minocha et al., 2015a). The objective of the present study was to evaluate the changes in concentrations of CaOx through changing seasons (June 2014–September 2015) and in the percent of total Ca present in the form of CaOx in the foliage of conifer and hardwood trees using this method. We hypothesized that the only role of CaOx crystals is to bind excess Ca in conifer and hardwood trees. Based on this hypothesis, one would predict that as concentrations of foliar Ca increase over the duration of the growing season so would CaOx and that its quantities should be higher in the older foliage of conifers.
Materials and methods
Site description
Leaf samples were collected from two conifers [red spruce (Picea rubens Sarg.) and white pine (Pinus strobus L.)] and two hardwood species [black oak (Quercus velutina L.) and sugar maple (Acer saccharum Marshall)] growing on land owned and managed by the University of New Hampshire, Durham, NH. Trees in this study varied in age, height, and diameter at breast height (dbh) (Table 1).
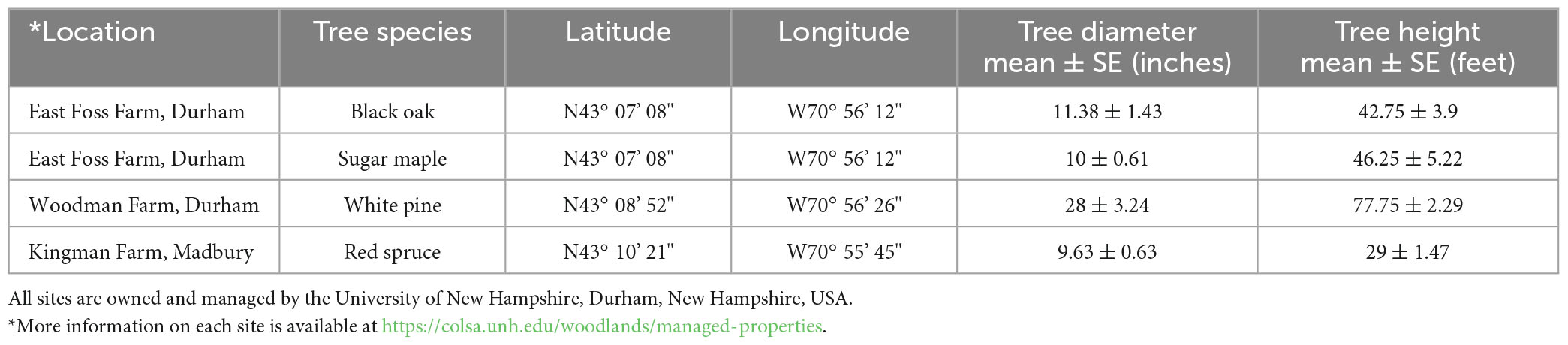
Table 1. Site locations, tree species, GPS coordinates for sampling sites, diameter at breast height, and tree heights.
Small branches were abscised from sun-lit positions in mid-canopy using a pole pruner. Foliage remained intact on the woody stems and was placed in a cooler for transport back to the US Forest Service, Louis C. Wyman Forestry Sciences Laboratory (Durham, NH) for processing. All samples were processed within 4 h of collection. Samples were collected four times for hardwoods (June and September 2014, and July and September 2015) and six times for conifers (June and September 2014, February, April, July, and September 2015). The same four replicate trees per species were used for each collection throughout the study. For spruce and pine, both current-year (CY) and previous-year (PY) foliage was collected, though due to defoliation caused by needle cast fungal disease, PY pine foliage was not available from September 2014 through April 2015.
For each tree, visually healthy leaves from several branchlets were chosen. From those leaves, a pool of approximately 4 g of leaf disks was collected from the maple and oak using a paper punch (6.4 mm in diameter, main veins excluded) and from spruce and pine, foliage was finely chopped (1–2 mm) using scissors. Each sample was mixed to create a homogeneous pool from which four sub-samples of 300 mg each were weighed; duplicate samples were taken for both sequential and direct extractions in 5% PCA. The remainder of the tissue was used for extraction of total calcium and moisture content determinations.
Sequential extractions of Ca and Ox
Sequential extractions of Ca and oxalic acid were conducted in solvents of increasing strength using pooled tissue according to the procedure described in Minocha et al. (2015a). Briefly, 10 ml of double-distilled water (referred to as water from this point on) was added to 300 mg of fresh foliage for nutrient extractions by three cycles of freezing and thawing (Minocha et al., 1994). The tissue was allowed to settle and the extract was then carefully transferred to a clean scintillation vial using a pipette. This fraction contained a small amount of water-soluble calcium (possibly Ca acetate, Ca citrate, Ca gluconate, Ca lactate) and oxalate (possibly sodium hydrogen oxalate, potassium hydrogen oxalate, and some free oxalate) as described in Supplementary Table 1. This water-soluble fraction is readily available for required physiological processes within cells. The resulting pellets were rinsed with 40 ml of water and then dried overnight at 50°C. The dried tissue was reconstituted in 10 ml of 2N acetic acid, and the above-described steps of freeze-thawing, washing, and drying were repeated. This second extracted fraction included Ca as Ca pectate and Ox as potassium oxalate and sodium oxalate. In the final step, the pellets were reconstituted in 10 ml of 5% PCA, frozen and thawed three times, and then kept frozen at –20°C until analyses. This step extracts the remaining Ca and Ox in the form of CaOx crystals (Minocha et al., 2015a).
Direct extraction of Ca and total Ox in 5% PCA
Direct extraction in 5% PCA simultaneously elicits all three portions of Ca and Ox that were extracted in each of the three sequential steps in water, acetate, and PCA. Three hundred mg of fresh foliar tissue was extracted in 10 ml of 5% PCA by three freeze-thaw cycles according to Minocha et al. (2015a).
Extraction of total foliar calcium
A sub-sample of approximately one gram was taken from each pool of tissue and oven-dried at 70°C for 7 days. Samples were ground for 1 min to produce a fine homogeneous powder with a shatterbox (SPEX SamplePrep, Metuchen, NJ, USA). One hundred mg of dry tissue was digested in 5 ml of concentrated nitric acid (15.8NHNO3) in Teflon vessels using a microwave (MARS Xpress, CEM Corporation, Matthews, NC, USA) following EPA compendium SW-846, Test Method 3052 (Microwave assisted acid digestion of siliceous and organically based metrics, 1996). The temperature was ramped to 200°C for 15 min and then held at 200°C for 15 min.
Quantification of calcium ions
Samples were quantified for Ca according to the procedure detailed in Minocha et al. (2015a). Briefly, total digests were brought to a final volume of 25 ml with water, and sequential and direct extracts were diluted 5 times with the appropriate matrix before Ca analysis and quantitation using a simultaneous axial inductively coupled plasma emission spectrophotometer (ICP-OES, Vista CCD, Varian, Palo Alto, CA, USA) and Vista Pro software (Version 4.0). Analysis was conducted according to EPA compendium SW-846, Test Method 6010D. Concentrations of Ca in National Institute of Standards and Technology (NIST, Gaithersburg, MD, USA) standards for Eastern white pine (SRM 1575A), apple (SRM 1515), and peach (SRM 1547) foliage were within acceptable limits. For all samples, a standard curve was repeated after every 20 samples, and check standards were run after every recalibration and after every 10 samples.
HPLC method for quantification of oxalic acid
Oxalic acid content was determined using a fast and easy high-performance liquid chromatographic (HPLC) method optimized in our laboratory for tree foliage extracted in 5% PCA (Minocha et al., 2015a). Samples were analyzed and quantified using a Series 200 Perkin-Elmer HPLC system including a pump, auto-sampler fitted with a 200 μl sample injection loop (20 μl injection volume), and UV/VIS detector set at 0.2 (0.0–1.35AU) for analog to digital conversion range and 0.02 (0.02–5.0 s) for response or peak width (Perkin-Elmer Corporation, Norwalk, CT, USA). Absorbance was measured at 215 nm. Oxalic acid (as well as several other organic acids) was separated on a Phenomenex Synergi-Hydro-RP 4 μm C18 column, 100 mm × 4.6 mm id fitted with a Phenomenex Security Guard, 5 μm, 4 mm × 3 mm id C18 guard column, all heated to 25°C (Phenomenex, Torrance, CA, USA), using an isocratic method running 25 mM phosphate buffer ((KH2PO4 + H3PO4) + 0.5 mM TBA + 1% MeOH, pH 2.5) at a flow rate of 1.0 ml/minute. Oxalic acid typically elutes in ≤ 1.5 minutes. Perkin-Elmer TotalChrom software (Version 6.2.1) was used to integrate data. A calibration curve based on the elution profile of Ox was used to estimate concentrations in sample extracts.
Statistical analysis
Although modified Thompson Tau Test was conducted to remove outliers from the data before conducting statistical tests (Thompson, 1985), no outliers were found. For each species, the effect of time of collection on foliar Ca concentrations in various fractions were tested individually using a one-way ANOVA. Separate ANOVAs were conducted for Ca and Ox concentrations as dependent variables. For all ANOVAs, the solvent type was the independent variable (P = 0.05). Means from significant ANOVAs were compared using Tukey’s Least Significant Difference (LSD) test (P = 0.05). A two-tailed Student’s T-test was used to determine the significant difference between a group of two means. (**P = 0.05 and *P = 0.1). All analyses were done using SYSTAT Version 10.2 for Windows (SYSTAT, Richmond, CA, USA) and Microsoft Excel (Version 2010).
Results and discussion
Intraspecific tree-to-tree variability in CaOx
As expected, and previously observed in other studies (Minocha et al., 2010, 2015b,2019), in this study also, regardless of species and time of collection, there was a significant amount of intraspecific tree-to-tree variation in the concentrations of CaOx quantified from the third sequential extraction in 5% PCA (Supplementary Figures 1, 2). The variability coefficient for diameter at breast height (dbh) ranged between 12–25% and 6–18% for tree heights. Besides the physical differences of individual trees, several other factors including the genetic composition of the tree (Westerband et al., 2021 and references therein), microsite soil chemistry, temperature, relative humidity, and unique microbiomes present on and inside the leaves affect the accumulation of Ca and Ox in foliage. These factors add dimensions of variability not only to Ca and Ox but also to other foliar nutrients and other highly responsive metabolites. Although presented in the Supplementary material, the details on the constituents of the first (water) and second (acetate) sequential extractions (Supplementary Tables 2–5) will not be discussed in this study because they are described in Minocha et al. (2015a). The extractants from these two fractions were not the focus of this study but they had to be removed to get to CaOx crystals. The Supplementary material included here may be useful to others.
Purity of CaOx crystals
When the data of all collection times and all species were pooled, quantities of Ca and Ox (extracted as CaOx) in the third sequential fraction with 5% PCA, correlated significantly with each other (r2 = 0.98) (Figure 1A). As described in the below paragraph, this correlation became even stronger (r2 = 0.99) when July 2015, and September 2014 and 2015 data were excluded (Figure 1B). The weakest, yet still significant relationship was for data pooled for July and September 2015 (r2 = 0.97, Figure 1C). When the pooled data were further separated by species, we found that along with the major effect of season/collection time, needle age also contributed to this relationship (PY foliage had a slightly lower correlation coefficient compared to CY foliage); this was true for both conifer species (Supplementary Figure 3).
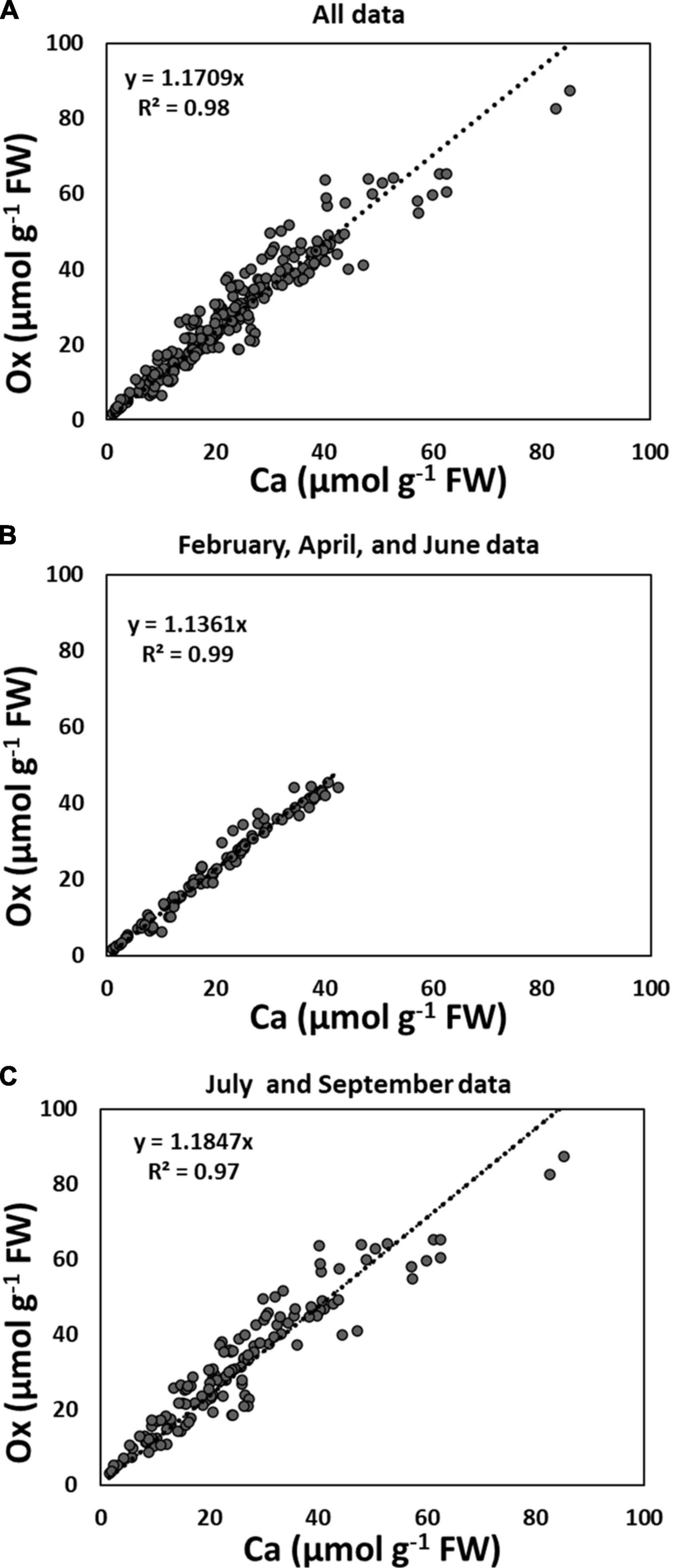
Figure 1. Correlation between Ca and Ox (extracted as CaOx crystals with 5% perchloric acid) in the third sequential fraction following water and 2N Acetic acid: (A) all species collected in June and September 2014, and February, April, July, and September 2015, n size = 230; (B) all species collected in June 2014, and February and April 2015, n = 94; (C) all species collected in July and September 2014 and 2015, n = 136. Each data point is the average of two analytical replicates.
Although not significant, there was a trend toward higher amounts of Ox than Ca in CaOx crystals for all species. Closer observation of Figure 2 further revealed that these differences in Ca and Ox concentrations within the CaOx fraction were even larger for July and September of 2015 as compared to other times. This was true for all species. The reason for this might lie in the differences in the hydration state of CaOx crystals during warmer months. An examination of the monthly average temperature records at the Hubbard Brook Experimental Forest, NH revealed that overall September 2015 with a mean monthly temperature of 15°C was warmer than September 2014 with a mean monthly temperature of 12°C (https://www.fs.usda.gov/rds/archive/catalog/LTER-ext-HBEF-2020-0012; USDA Forest Service, 2020). Temperature has been shown to affect the hydration states of CaOx crystals (Izatulina et al., 2018). At present, the extent and types of hydration of CaOx crystals are unknown for the four species under study. The weight of one mole of anhydrous CaOx (CaC2O4, formula weight 128.1) consists of 31% (Ca) and 69% (Ox) by weight. Our calculations were done based on the assumption that all crystals were anhydrous (though these crystals probably are never present in living beings as anhydrous) and this assumption may be even more erroneous for the summer months. Supposing in nature these crystals existed as monohydrate, the additional weight of one mole of water (18 g) would have proportionality affected the molar mass of Ox by approximately two-fold (69% of 18 g) relative to Ca (31% of 18 g) leading to higher concentrations of Ox relative to Ca though, in reality, they were equal. This would also explain why the correlations between Ca and Ox were weaker for all species in July and September (Figure 1).
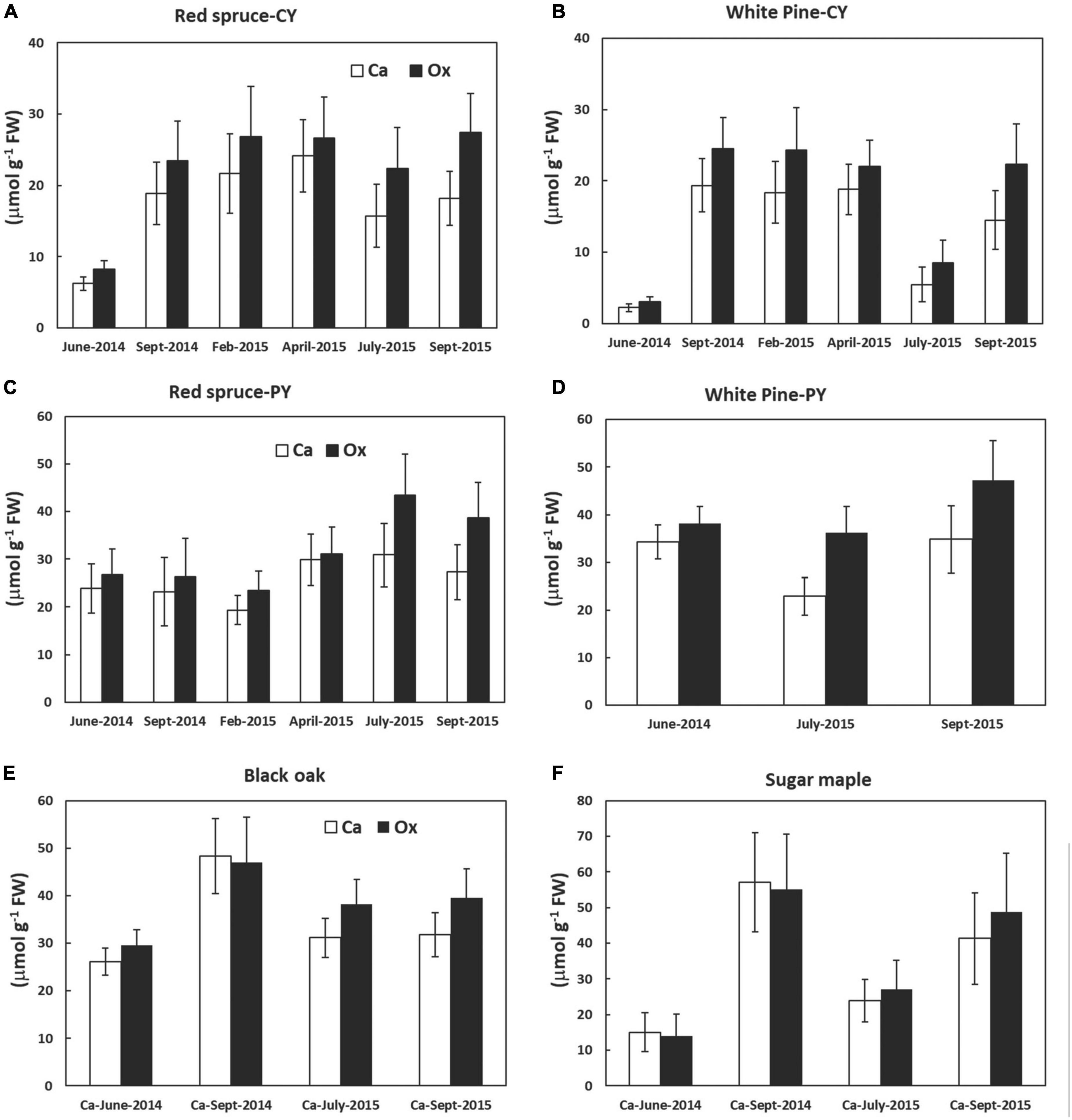
Figure 2. Calcium and Ox concentrations (extracted as CaOx crystals in the third sequential fraction in 5% perchloric acid) in various tree foliage collected from June 2014 through September 2015; (A) CY foliage of red spruce, (B) CY foliage of white pine, (C) PY foliage of red spruce, (D) PY foliage of white pine, (E) black oak, and (F) sugar maple. P = 0.05. Data are mean ± SE, n = 4 where each data point is the average of two analytical replicates. No significant differences were observed between the amounts of Ca and oxalate at any time for all species tested.
Changes in concentrations of CaOx crystals over seasons and across species
The focus of this study was to evaluate changes in the concentrations of CaOx and in the percentage of Ca present in the form of CaOx relative to total Ca over time for four different tree species. In general, although the differences were not statistically significant overtime, CaOx concentrations were lowest in June, particularly in CY foliage of both conifers and in sugar maple leaves (Figure 2) rejecting the hypothesis stating that the only role of CaOx crystals is to bind excess Ca. If the hypothesis were to be accepted, then the concentrations of CaOx would have increased throughout the growing season, and it would be higher in older foliage of conifers at all times. The discovery of no clear pattern for the accumulation of CaOx over the growing season for any of these four species points to the possibility of other roles for these crystals as suggested in the literature and briefly discussed below.
Changes in the concentrations of foliar Ca extracted as CaOx and total Ca concentrations in foliar tissue were species-specific and varied with needle age in the conifers (Figure 3). However, differences in the quantities of Ca extracted as CaOx over time were not significant in most cases. The few exceptions were the presence of significantly higher amounts of Ca from September 2014 through April 2015 for CY-white pine and in September 2014 for black oak (Figure 3). Although in several cases a trend toward the lowest amount of Ca extracted as CaOx could be seen for June 2014, it was not significant. As expected, except for PY foliage, there were significant seasonal differences in total Ca (Figure 3).
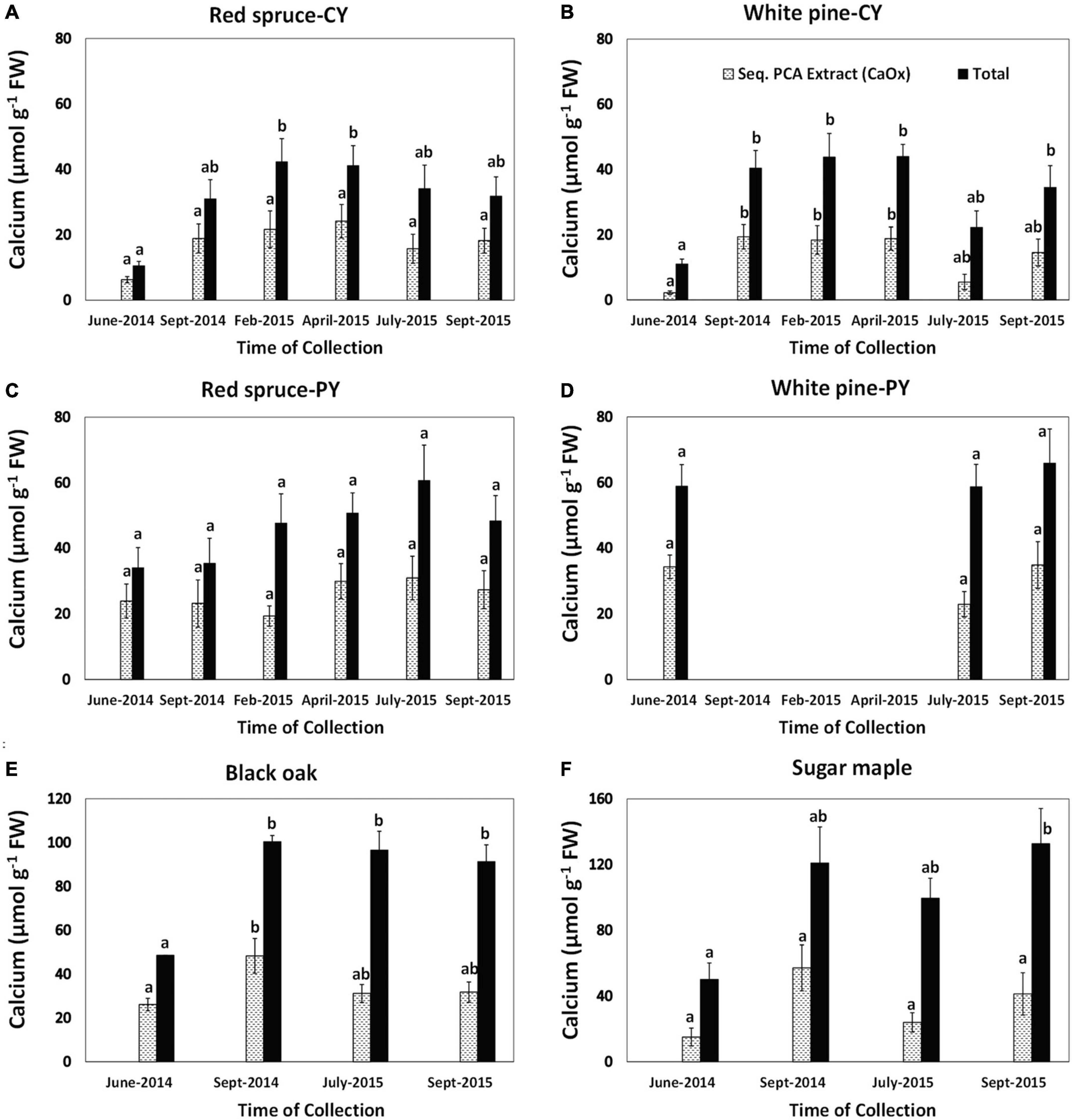
Figure 3. Concentrations of calcium extracted as CaOx in the third sequential extraction in 5% PCA and total Ca in foliage: (A) CY foliage of red spruce, (B) CY foliage of white pine, (C) PY foliage of red spruce, (D) PY foliage of white pine, (E) black oak, and (F) sugar maple. Letters above the bars show differences over time within each fraction. P = 0.05. Data are mean ± SE, n = 4 where each data point is the average of two analytical replicates. White pines suffered defoliation in the fall of 2014 so PY needle samples were not available from the winter of 2014 to 2015.
Changes in the percent of total Ca present as CaOx (Table 2) were also species-specific and varied with needle age in the conifers. Although significant changes with time were observed for this Ca fraction in all cases except for CY-red spruce (Table 2), there was no pattern to these changes in any of the species for the duration of the study indicating no relationship between the accumulation of CaOx and total Ca with time in the growing season. Similarly, significant changes in the percent of total Ox present in CaOx were observed for all species except for black oak (Table 3). In general, depending upon the species, 70–90% of total Ox was tied up as CaOx in the winter months. However, in the summer, especially in the case of conifers, less than one-third of Ox was present as CaOx assumingly leaving more than two-thirds of total cellular Ox to be used for other purposes, possibly including metabolic activities. Under conditions of Al toxicity, it is known that in cell cultures of red spruce, oxalate concentrations increased to bind with Al to reduce Al toxicity (Minocha and Long, 2004). These data further point to the possible need-based synthesis of CaOx from available Ca and Ox (Ox can also be synthesized as needed) within the cells.
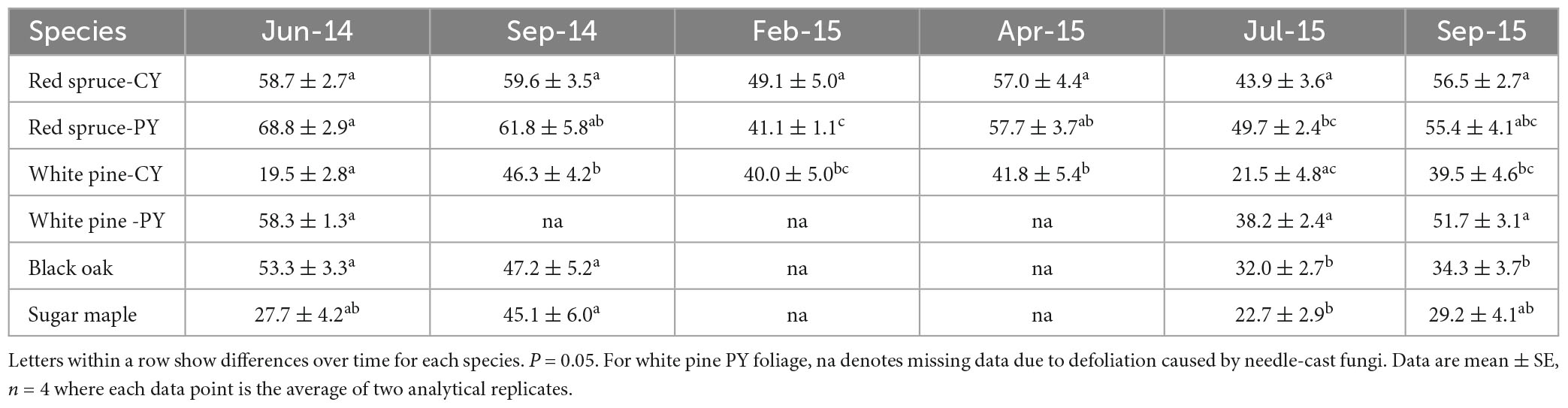
Table 2. Calcium in CaOx (extracted in the third sequential extraction in 5% PCA following water and 2N Acetic acid) as a percent of total cellular Ca.
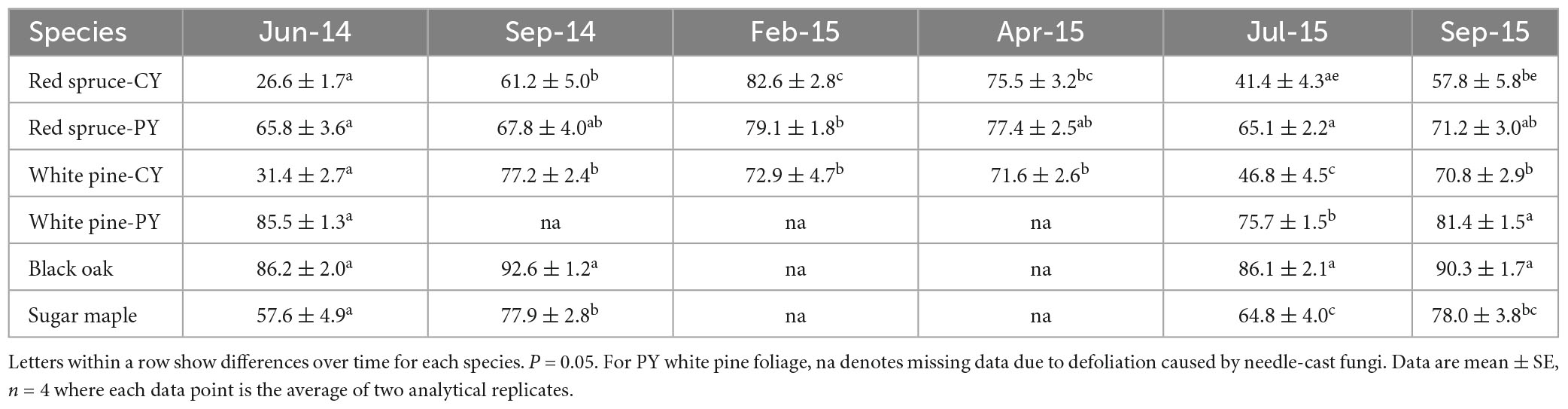
Table 3. Oxalate in CaOx (extracted in the third sequential extraction in 5% PCA following water and 2N Acetic acid) as a percent of total cellular Ox.
Calcium crystals are present in plant cells either in the form of CaOx or Ca carbonate (CaCO3), though the concentration of CaOx is much higher. Calcium oxalate crystals provide an adaptive advantage to plants under many conditions including drought, metal toxicity, and limited CO2 availability (Karabourniotis et al., 2020). Under drought conditions, CaOx crystal decomposition led to the release of CO2 to assist with photosynthesis in C4 plants (Tooulakou et al., 2016a). This newly discovered photosynthetic pathway was named “alarm photosynthesis,” by the authors because of the involvement of abscisic acid which causes stomatal closure. This photosynthetic process helps plants conserve water, limit carbon losses to the atmosphere, and lower the risk of photoinhibition, suggesting that CaOx crystals seem to play a crucial role in the survival of plants (Tooulakou et al., 2016b). Gómez-Espinoza et al. (2020) also found the decomposition of CaOx crystals to be a complementary mechanism for CO2 supply in Colobanthus quitensis growing in the harsh habitat of Antarctica. This may be one of the reasons for the vast presence of this mysterious pathway in some plants. Both diurnal and seasonal changes in the size of CaOx crystals have recently been reported in plants (Tooulakou et al., 2016a,b; Gómez-Espinoza et al., 2020) indicating some sort of functional role for these crystals. Although it has long been speculated that CaOx crystals are mainly present in the cells to serve as a reserve of Ca in plants, recent findings (some cited here) shed light on the possibility that CaOx crystals also play a role in providing Ox for critical cellular functions.
While not much is available in the published literature regarding the mechanisms for the in vivo or in vitro dissolution of CaOx crystals in plants, a few studies conducted with human kidneys might shed some light on this process in plants. Structural and chemical evolution studies of calcium oxalates are of particular importance in this regard as CaOx phase transitions occur at temperatures typical of the human body and can therefore have a significant effect on health. Chaiyarit et al. (2016) demonstrated that CaOx monohydrate crystals could be degraded/dissolved by endolysosomes inside renal tubular cells. Thus enzymatic actions within plant cells could also be able to degrade these crystals to release needed Ca or Ox. Recently, various parts of four medicinal plants (Herniaria hirsuta L., Opuntia ficus-indica flowers, Zea mays styles, and Ammi visnaga L. seeds), that have been traditionally used in Morocco for the treatment of kidney stones, were used to demonstrate in vitro dissolution of CaOx monohydrate (whewellite) crystals (El Habbani et al., 2021). Further evidence for the function of these crystals in Ca regulation is indicated by their disappearance under various conditions such as Ca deficiency (Franceschi, 1989) and developmental maturation (Ilarslan et al., 1997, 2001; Storey et al., 2003) in plants.
Gal et al. (2012) reported similarities in the anatomical locations and optical functions of cystoliths (CaCO3 crystals) and certain calcium oxalate druses in the leaves of Ficus microcarpa, Ficus elastica, and Carya illinoinensis trees. These authors suggested that light scattering by biominerals like CaOx in leaves may be a widespread phenomenon that helps redistribute light patterns and enables the leaf to use the incoming light flux more efficiently. In light of this information, the findings regarding the accumulation of calcium oxalate crystals and changes in light scattering patterns in the leaves of six species of deciduous tree in response to changes in environmental conditions (Dineva, 2019) point to a functional role of these crystals under stressful conditions.
Conclusions
This study not only revalidated the work published earlier by Minocha et al. (2015a) on the extraction and quantitation of CaOx crystals but also advanced our knowledge regarding seasonal changes in CaOx concentrations in the foliage of several tree species. In most cases, there were no significant differences in the amount of CaOx present during the growing season thus rejecting the hypothesis that the only role of CaOx crystals is to bind excess Ca in conifer and hardwood trees. However, the percent of total Ca present as CaOx varied widely with species, time of collection within a species, and needle age in the case of conifers. There was no discernable pattern(s) in the concentrations CaOx in the foliage of any species. This indirectly indicates that CaOx accumulation did not occur over time through the growing season only to shed off excess CaOx as a waste product, but rather other factors might be involved in controlling the need-dependent production of these crystals.
Data availability statement
The datasets presented in this study can be found in online repositories. The name of the repository and accession number can be found below: Minocha, Rakesh; Long, Stephanie. 2022. Calcium oxalate concentration, soluble elements, free polyamines, and amino acid data were extracted seasonally from the foliage of conifer and hardwood trees in New Hampshire. Fort Collins, CO: Forest Service Research Data Archive. https://doi.org/10.2737/RDS-2022-0066.
Author contributions
Both authors contributed equally to this manuscript.
Funding
Funding for this work was provided solely by the USDA Forest Service.
Acknowledgments
The authors are grateful to Gloria Quigley and Kenneth Dudzik for their help with the collection and analysis of samples.
Conflict of interest
The authors declare that the research was conducted in the absence of any commercial or financial relationships that could be construed as a potential conflict of interest.
Publisher’s note
All claims expressed in this article are solely those of the authors and do not necessarily represent those of their affiliated organizations, or those of the publisher, the editors and the reviewers. Any product that may be evaluated in this article, or claim that may be made by its manufacturer, is not guaranteed or endorsed by the publisher.
Supplementary material
The Supplementary Material for this article can be found online at: https://www.frontiersin.org/articles/10.3389/ffgc.2023.1161088/full#supplementary-material
Abbreviations
CaOx, calcium oxalate; PCA, perchloric acid.
References
Chaiyarit, S., Singhto, N., and Thongboonkerd, V. (2016). Calcium oxalate monohydrate crystals internalized into renal tubular cells are degraded and dissolved by endolysosomes. Chem. Biol. Interact. 246, 30–35. doi: 10.1016/j.cbi.2015.12.018
Dineva, S. (2019). Deposition of calcium oxalate crystals and tolerance of deciduous trees to pollution. J. Appl. Forest Ecol. 7, 8–13.
El Habbani, R., Lahrichi, A., Sqalli Houssaini, T., Kachkoul, R., Mohim, M., Chouhani, B., et al. (2021). In vitro mass reduction of calcium oxalate urinary calculi by some medicinal plants. Afr. J. Urol. 27, 28. doi: 10.1186/s12301-021-00132-2
Fink, S. (1991). The micromorphological distribution of bound calcium in needles of Norway spruce [Picea abies (L.) Karst.]. New Phytol. 119, 33–40.
Franceschi, V. (1989). Calcium oxalate formation is a rapid and reversible process in Lemna minor L. Protoplasma. 148, 130–137. doi: 10.1007/BF02079332
Franceschi, V., and Horner, J. (1980). Calcium oxalate crystals in plants. Bot. Rev. 46, 361–427. doi: 10.1007/BF02860532
Franceschi, V., and Nakata, P. (2005). Calcium oxalate in plants: Formation and function. Annu. Rev. Plant Biol. 56, 41–71. doi: 10.1146/annurev.arplant.56.032604.144106
Gaberščik, A., Grašič, M., Vogel-Mikuš, K., Germ, M., and Golob, A. (2020). Water shortage strongly alters formation of calcium oxalate druse crystals and leaf traits in Fagopyrum esculentum. Plants 9:917. doi: 10.3390/plants9070917
Gal, A., Brumfeld, V., Weiner, S., Addadi, L., and Oron, D. (2012). Certain biominerals in leaves function as light scatterers. J. Adv. Mater. 24, O77–O83. doi: 10.1002/adma.201104548
Gómez-Espinoza, O., González-Ramírez, D., Bresta, P., Karabourniotis, G., and Bravo, L. (2020). Decomposition of calcium oxalate crystals in Colobanthus quitensis under CO2 limiting conditions. Plants 9, 1307. doi: 10.3390/plants9101307
Hudgins, J., Krekling, T., and Franceschi, V. (2003). Distribution of calcium oxalate crystals in the secondary phloem of conifers: a constitutive defense mechanism? New Phytol. 159, 677–690. doi: 10.1046/j.1469-8137.2003.00839.x
Ilarslan, H., Palmer, R., and Horner, H. (2001). Calcium oxalate crystals in developing seeds of soybean. Ann. Bot. 88, 243–257. doi: 10.1006/anbo.2001.1453
Ilarslan, H., Palmer, R., Imsande, J., and Horner, H. (1997). Quantitative determination of calcium oxalate and oxalate in developing seeds of soybean (Leguminosae). Am. J. Bot. 84:1042. doi: 10.2307/2446147
Izatulina, A., Gurzhiy, V., Krzhizhanovskaya, M., Kuz’mina, M., Leoni, M., and Frank-Kamenetskaya, O. (2018). Hydrated calcium oxalates: Crystal structures, thermal stability, and phase evolution. Cryst. Growth Des. 18, 5465–5478. doi: 10.1021/acs.cgd.8b00826
Karabourniotis, G., Horner, H., Bresta, P., Nikolopoulos, D., and Liakopoulos, G. (2020). New insights into the functions of carbon-calcium inclusions in plants. New Phytol. 228, 845–854. doi: 10.1111/nph.16763
Minocha, R., Chamberlain, B., Long, S., Turplapati, S., and Quigley, G. (2015a). Extraction and estimation of the quantity of calcium oxalate crystals in the foliage of conifer and hardwood trees. Tree Physiol. 35, 574–580. doi: 10.1093/treephys/tpv031
Minocha, R., Turlapati, S., Long, S., McDowell, W., and Minocha, S. (2015b). Long-term trends of changes in pine and oak foliar nitrogen metabolism in response to chronic nitrogen amendments at the Harvard Forest, MA. Tree Physiol. 35, 894–909. doi: 10.1093/treephys/tpv044
Minocha, R., and Long, S. (2004). Effects of aluminum on organic acid metabolism and secretion by red spruce cell suspension cultures and the reversal of Al effects on growth and polyamine metabolism by exogenous organic acids. Tree Physiol. 24, 55–64. doi: 10.1093/treephys/24.1.55
Minocha, R., Long, S., Thangavel, P., Minocha, S., Eagar, C., and Driscoll, C. (2010). Elevation dependent sensitivity of northern hardwoods to Ca addition at hubbard brook experimental forest NH, USA. For. Ecol. Manage. 260, 2115–2125. doi: 10.1016/j.foreco.2010.09.002
Minocha, R., Long, S., Turlapati, S., and Fernandez, I. (2019). Dynamic species-specific metabolic changes in the trees exposed to chronic N+S additions at the Bear Brook Watershed in Maine, USA. Ann. For. Sci. 76, 25. doi: 10.1007/s13595-019-0808-0
Minocha, R., Shortle, W., Long, S., and Minocha, S. (1994). A rapid and reliable procedure for extraction of cellular polyamines and inorganic ions from plant tissues. J. Plant Growth Regul. 13, 187–193. doi: 10.1007/BF00226036
Paiva, E. (2019). Are calcium oxalate crystals a dynamic calcium store in plants? New Phytol. 223, 1707–1711. doi: 10.1111/nph.15912
Raman, V., Horner, H., and Khan, I. (2014). New and unusual forms of calcium oxalate raphide crystals in the plant kingdom. J Plant Res. 127, 721–730. doi: 10.1007/s10265-014-0654-y
Smith, K., Shortle, W., Connolly, J., Minocha, R., and Jellison, J. (2009). Calcium fertilization increases the concentration of calcium in sapwood and calcium oxalate in foliage of red spruce. Environ. Exp. Bot. 67, 277–283. doi: 10.1016/j.envexpbot.2009.07.007
Storey, R., Wyn Jones, R., Schachtman, D., and Treeby, M. (2003). Calcium-accumulating cells in the meristematic region of grapevine root apices. Funct Plant Biol. 30, 719–727. doi: 10.1071/FP02212
Thompson, R. (1985). A note on restricted maximum likelihood estimation with an alternative outlier model. J. R. Stat. Soc. Series B Stat. Method. 47, 53–55. doi: 10.1111/j.2517-6161.1985.tb01329.x
Tooulakou, G., Giannopoulos, A., Nikolopoulos, D., Bresta, P., Dotsika, E., Orkoula, M., et al. (2016a). Alarm photosynthesis: calcium oxalate crystals as an internal CO2 source in plants. Plant Physiol. 171, 2577–2585. doi: 10.1104/pp.16.00111
Tooulakou, G., Giannopoulos, A., Nikolopoulos, D., Bresta, P., Dotsika, E., Orkoula, M., et al. (2016b). Reevaluation of the plant “gemstones”: Calcium oxalate crystals sustain photosynthesis under drought conditions. Plant Signal. Behav. 11:e1215793. doi: 10.1080/15592324.2016.1215793
USDA Forest Service (2020). Northern Research Station. Hubbard Brook Experimental Forest: Daily Temperature Record, 1955 - present ver 10. Environmental Data Initiative. Washington, DC: USDA Forest Service.
Keywords: extraction, pine, maple, oak, spruce, HPLC, quantitation, calcium oxalate (CaOx)
Citation: Minocha R and Long S (2023) Seasonal changes in foliar calcium oxalate concentrations in conifer and hardwood trees: a potentially bioavailable source of cellular calcium and/or oxalate under stress. Front. For. Glob. Change 6:1161088. doi: 10.3389/ffgc.2023.1161088
Received: 07 February 2023; Accepted: 03 May 2023;
Published: 27 June 2023.
Edited by:
Jason Vogel, University of Florida, United StatesReviewed by:
Roque Rodríguez-Soalleiro, University of Santiago de Compostela, SpainPaula Guzmán-Delgado, University of California, Davis, United States
Copyright © 2023 Minocha and Long. This is an open-access article distributed under the terms of the Creative Commons Attribution License (CC BY). The use, distribution or reproduction in other forums is permitted, provided the original author(s) and the copyright owner(s) are credited and that the original publication in this journal is cited, in accordance with accepted academic practice. No use, distribution or reproduction is permitted which does not comply with these terms.
*Correspondence: Rakesh Minocha, cmFrZXNoLm1pbm9jaGFAdXNkYS5nb3Y=