- 1Co-Innovation Center for Sustainable Forestry in Southern China, Nanjing Forestry University, Nanjing, Jiangsu, China
- 2College of Biology and the Environment, Nanjing Forestry University, Nanjing, Jiangsu, China
- 3Advanced Analysis and Testing Center, Nanjing Forestry University, Nanjing, Jiangsu, China
- 4Dongtai Forest Farm, Yancheng, Jiangsu, China
Although patterns in the vertical distribution of soil organic carbon (SOC) are key to assessing soil C sequestration potential, they remain poorly understood. We sampled 18 soil profiles at one meter depth at the Dongtai Forest Farm to investigate the vertical distribution of SOC, the stoichiometric relationship between SOC and total nitrogen (TN) and the controlling factors of SOC at different soil depths. We found that SOC content decreased significantly with increasing soil depth. Approximately 67% of SOC was stored in the top 30 cm. The N-C scaling slope (i.e., the slope of the relationship between log-transformed N and C not significantly different from 1.0) revealed significant differences between top- and subsoil with N and C scaled isometrically in topsoil (0–30 cm), but not in subsoil (30–100 cm). SOC content was co-regulated by soil physiochemical and microbial properties at the site level with soil chemical and microbial properties dominant in the top- and subsoil, respectively. Topsoil SOC increased with soil TN, available phosphorus (AP) and fungal abundance. Subsoil SOC increased with the fungal-to-bacterial ratio, fungal abundance and soil dissoluble organic carbon (DOC). Our study highlights the dominance of microbial community in regulating SOC in the subsoil and advances our understanding of the variation in mechanisms regulating SOC along the soil profile.
1. Introduction
Soil carbon (C) sequestration is primarily achieved through microbial turnover, partial decomposition and transformation of dead organic matter (Lefèvre et al., 2017). The soil C pool plays a key role in maintaining soil health through increasing organic matter as an energy and nutrition source for biota (Janzen, 2006) and by providing ecosystem services such as regulating climate, water supplies and biodiversity (Milne et al., 2015). Soil is a major sink for atmospheric CO2 (Lal, 2004; Lal et al., 2015) and is the largest C pool in terrestrial ecosystems (Batjes, 1996). Soil is estimated to contain more C than is stored in the atmosphere and vegetation combined (Lehmann and Kleber, 2015). Any small changes in soil C pool can have substantial impacts on atmospheric CO2 concentration as well as a potential positive feedback effect on climate change (Ren et al., 2022). For this reason, an increasing number of studies are focusing on soil organic C (SOC) sequestration. In the coming decades, increased C sequestration in soil may be an effective means to slow the rise of atmospheric CO2 and mitigate climate change (Jackson et al., 2017). To accurately predict the global C cycle, it may be necessary to have an in-depth understanding of the dynamics and influencing factors of the SOC pool.
The main source of SOC is vegetative photosynthetic production (Wang et al., 2021). Concentration of SOC is generally inversely related to soil depth (Jobbágy and Jackson, 2000; Gross and Harrison, 2019) with an immense amount of SOC storage and turnover occurring in the top 30 centimeters (Wesemael et al., 2005; Meersmans et al., 2009; Luo et al., 2019). This pattern results largely from terrestrial plant litter distribution and concentration of roots along the soil profile (Rubino et al., 2010; Sayer et al., 2011). Perhaps because of this, few studies to date have focused on the subsoil organic C pool. Research has shown that in subsoil layers (below 30cm), there are large stocks of SOC with slow turnover that may respond differently to disturbances compared with topsoil stores (e.g., Batjes, 1996; Jobbágy and Jackson, 2000; Luo et al., 2019). Nevertheless, our current understanding of the SOC stocks and their dynamics in the subsoil is hampered by the lack of field data (e.g., Batjes, 1996; Jobbágy and Jackson, 2000; Meersmans et al., 2009; Gross and Harrison, 2019; Luo et al., 2019), making it difficult to validate the global C model and accurately predict future climate change (Luo et al., 2019; Xia et al., 2022). It is thus important to consider the potential for all soil layers to sequester C sand mitigate climate change (Heitkötter et al., 2017).
Element C and N are the most basic elements in soil organisms, playing crucial roles in their activities and the circulation of soil nutrients (Zhang et al., 2017). Due to the interaction between C and N in ecological processes such as photosynthesis, respiration and microbial decomposition, C-N coupling is widespread in terrestrial ecosystems and has received warranted attention (Reich et al., 2006; Vitousek et al., 2010; Peñuelas et al., 2012). Importantly, the C-N coupling relationship in terrestrial ecosystems reflects potential SOC sequestration capacity that contributes to terrestrial C cycling (Xu et al., 2016). Previous studies have reported an isometric relationship between N and C in the topsoil (i.e., the slope of the relationship between log N and log C is not significantly different from 1.0). However, few studies have focused on the coupled pattern of N and C in soil environments compared to plant parts, which suggests that our understanding of changes in C:N stoichiometric patterns in the soil is not well developed (Cleveland and Liptzin, 2007; Fanin et al., 2014; Zhao et al., 2017; Liu et al., 2019), especially along soil layers. Studies of whether the C-N isometric relationship observed in topsoil also occurs in subsoil and the link to soil C sequestration potential are needed (Yang et al., 2010).
Biological and abiotic factors in the soil environment can significantly alter SOC distribution and C sequestration potential (Zeng et al., 2022). Previous studies have suggested that SOC storage is driven by soil physicochemical properties (pH, moisture, total N, total P, available C, available N, and available P), microbes (fungal and bacterial decomposition groups), and plants (plant community and plant litter quality). Among the dozens of biotic and abiotic factors affecting SOC storage (Averill et al., 2014; Wu et al., 2017; Luo et al., 2020; Chen et al., 2021; Wang et al., 2021; Zhang et al., 2021), soil properties have received much attention. For example, N limits C storage in terrestrial ecosystems, even in N-rich tropical forests (Lu et al., 2021). Cai et al. (2022) described the limiting effects of N accumulation on C storage in terrestrial ecosystems using the sequestrated organic C:N as a criterion. Additionally, microbes are an indispensable factor affecting SOC stabilization, regulating the functional properties in SOC turnover (Magnani et al., 2007; Salome et al., 2010). The formation and mineralization of SOC are mediated by soil microbes and may also be the precursors of SOC formation (Lynch and Bragg, 1985; Kohl et al., 2015). Feedback responses of soil microbial communities are often excluded from terrestrial C cycle models due to the complexity of microbial physiological processes and their poorly understood mechanisms. To fill this gap, previous studies have used the fungal-to-bacteria ratio (F:B) as an indicator given that soil microbes are classified into two dominant decomposer groups, fungi and bacteria (e.g., Six et al., 2006; Koranda et al., 2014; Chen et al., 2021). The ratio has been extensively used in soil C cycling studies, finding that SOC produced by fungi is more stable, and a higher F:B may be more conducive to soil C sequestration (Malik et al., 2016). Fungal biomass has a larger C:N ratio, thereby improving C use efficiency (Bhattacharya et al., 2016). Hence, changes in soil microbial dominance (represented by the F:B) and physicochemical properties largely affect the structure and function of soil ecosystems, and thus can be a reliable indicators of ecosystem C sequestration and sustainability (Zhang et al., 2016; Kumar and Ghoshal, 2017; Ni et al., 2020).
Poplar is one of the most important economic tree species in China (Zhang et al., 2016; Li et al., 2018). There are approximately 8.54 million hectares of poplar plantations in China, accounting for 18.14% of total plantation area (Tun et al., 2018). Jiangsu Province is responsible for 7% of the national forestry output value with 0.7% of the national forest land area, of which poplar plantation contributed 66.7%. Poplar plantations have provided many ecological benefits, such as erosion control, soil quality improvement, and wildlife habitat (Sartori et al., 2007). Less attention has been paid to their capacity for soil C sequestration, particularly in deep soil layers (Block et al., 2006; Garten et al., 2011). Here, we report an experiment where we measured SOC storage patterns along the soil profile and explored factors regulating the distribution of SOC at Dongtai Forest Farm on China’s east coast. We predicted that: (1) SOC storage would decrease with increasing soil depth and a high proportion of SOC would be stored in top soil layers as widely found; (2) N would scale isometrically with C along the soil profile, and (3) the dominant factors regulating SOC distribution would vary among soil depths and subsoil SOC would be strongly affected by soil microbial properties.
2. Materials and methods
2.1. Experimental site description
Our experimental site is located at the Dongtai Forest Farm on the coast of the Yellow Sea in Yancheng, Jiangsu Province, China (120o49’E, 32o52’N). The forest farm was established in 1965 and covers an area of approximately 3000 ha–1. The climate of this region is classified as oceanic and monsoon. The mean annual air temperature is 14.9°C and mean annual precipitation is 1,056.6 mm (1960 to 2020 records) (Geng et al., 2021). The plantation soil is desalinated meadow and sandy with alkaline soil pH (Bian et al., 2019). The vegetation is mainly artificial deciduous and evergreen broad-leaf forest, with tree coverage approximately 85% of the ground surface (Yang et al., 2017). Poplar (Populus deltoids cv. ‘I – 35’) and dawn redwood (Metasequoia glyptostroboides) are the dominant tree species planted on the farm.
2.2. Soil sampling and physicochemical analyses
In July 2019, 18 plots of 50 × 50 m were randomly located and surveyed on the plantation. Soil samples in each plot were collected with a 5 cm-diameter soil corer and separated into 0–10, 10–20, 20–30, 30–40, 40–50, 50–60, 60–70, 70–80, 80–90, and 90–100 cm depth intervals. We collected soil samples from the same soil depth using a six-point “S” shape sampling method. Soil samples were combined and homogenized into a composite sample. Soil samples were immediately sieved (< 2 mm) to remove soil fauna, rocks and fine roots, sealed in plastic bags and transported in coolers to our laboratory at the Nanjing Forestry University. We disinfected the soil corer and sieves with 75% ethanol between samples and sieving. Soil samples were air dried or refrigerated at 4 and –80°C for subsequent analyses.
Soil moisture was measured by drying 10 g of fresh soil at 105°C for 48 h. Soil pH was measured at a soil-to-water ratio of 1: 2.5 (v:v) with a pH meter (Model PHS – 25, INESA Instrument, Shanghai, China). We determined SOC and TN with an elemental analyzer (Vario EL III, Elementar Analysen Systeme, Germany) after the addition of 1 mol⋅L –1 HCL to remove inorganic C components. Total phosphorus (TP) was determined using acid-soluble colorimetry (Wang et al., 2010). Briefly described, a 0.25 g sample of air-dried soil was placed in Erlenmeyer flask, 3 ml concentrated sulfuric acid and 10 drops of perchloric acid were added and heated at 400°C to a white transparent state. Samples were then transferred to a 100 ml volumetric flask and brought up to volume. After solutions clarified, we mixed supernatant with a color developer and added them to 96 -well plates. We measured the absorbance of the colored mixture at 700 nm using a microplate reader (Infinite M Nano +, Tecan, Switzerland). For DOC measurement, extracted solutions with the soil-to-water ratio of 1: 4 (v:v) were filtered by 0.45 μm filter and their C was measured using a total organic N/C analyzer (Multi N/C 2100S, analytikjena, Germany). Soil NH4+ and NO3– contents were determined using spectrophotometry (Miranda et al., 2001), with the sum calculated as the soil AN content (Dai et al., 2018). The amount of available phosphorus (AP) was extracted using a NaHCO3 solution and measured using a Mo-blue method (Watanabe and Olsen, 1965).
2.3. Quantitative real-time PCR (qPCR)
To determine the abundance of fungi and bacteria and their relative abundance, total bacterial and fungal DNA were extracted from 0.25 g samples of frozen soil using the TIANamp Soil DNA kit (TIANGEN, DP336), following manufacturer protocol. We used a microplate reader (Infinite M Nano +, Tecan, Switzerland) to measure the nucleic acid concentration of the processed samples at 260 nm, and then stored at –20°C for later use. Each 20 μl qPCR reaction system contained 0.5 μl of template DNA, 10 μL SuperReal PreMix Plus (SYBR Green, TIANGEN, FP205), 8.5 μl double distilled water, and 0.5 μl of each primer. The abundance of total bacteria and total fungi was assessed by quantitative real-time PCR (qPCR) based on increasing fluorescence intensity of the SYBR Green dye during amplification. Target specific primers are 5.8s/ITS1f (5′-CGCTGCGTTCTTCATCG-3′/5′-TCCGTAGGTGAACCTGCGG-3′) for fungi and Eub338/Eub518 (5′-ACTCCTACGGGAGGCAGCAG-3′/5′-ATTACCGCGGCTGCTGG-3′) for bacterial (Fierer et al., 2005). For each sample, three independent qPCR assay were performed for each gene. The qPCR reactions were performed under thermal cycling conditions including 3 min at 95°C, 40 cycles of 10 s at 95°C, and 30 s at 60 °C for DNA amplification in qTOWER3G Real-Time PCR Thermocycler (Analytik Jena, Germany). qPCR Ct values were reported as gene copy number g–1 of dry soil, including bacterial and fungal gene copy number (BCN and FCN). The F:B was calculated as the ratio of FCN and BCN (Zhou et al., 2018), which represents the relative abundance of fungi and bacteria of specific soil samples.
2.4. Statistical analyses
All statistical analyses were carried out with R software, version 4.1.1 (R Core Team, 2021). We used Shapiro-Wilk’s test to examine the normality and Bartlett test to examine homogeneity of variance of the dataset. All data were log-transformed except soil pH and soil moisture to enforce a normal distribution. However, for ease of comparison with other studies, data in Figures 1–4 depict the untransformed original data. One-way ANOVA was used to examine soil layer effects.
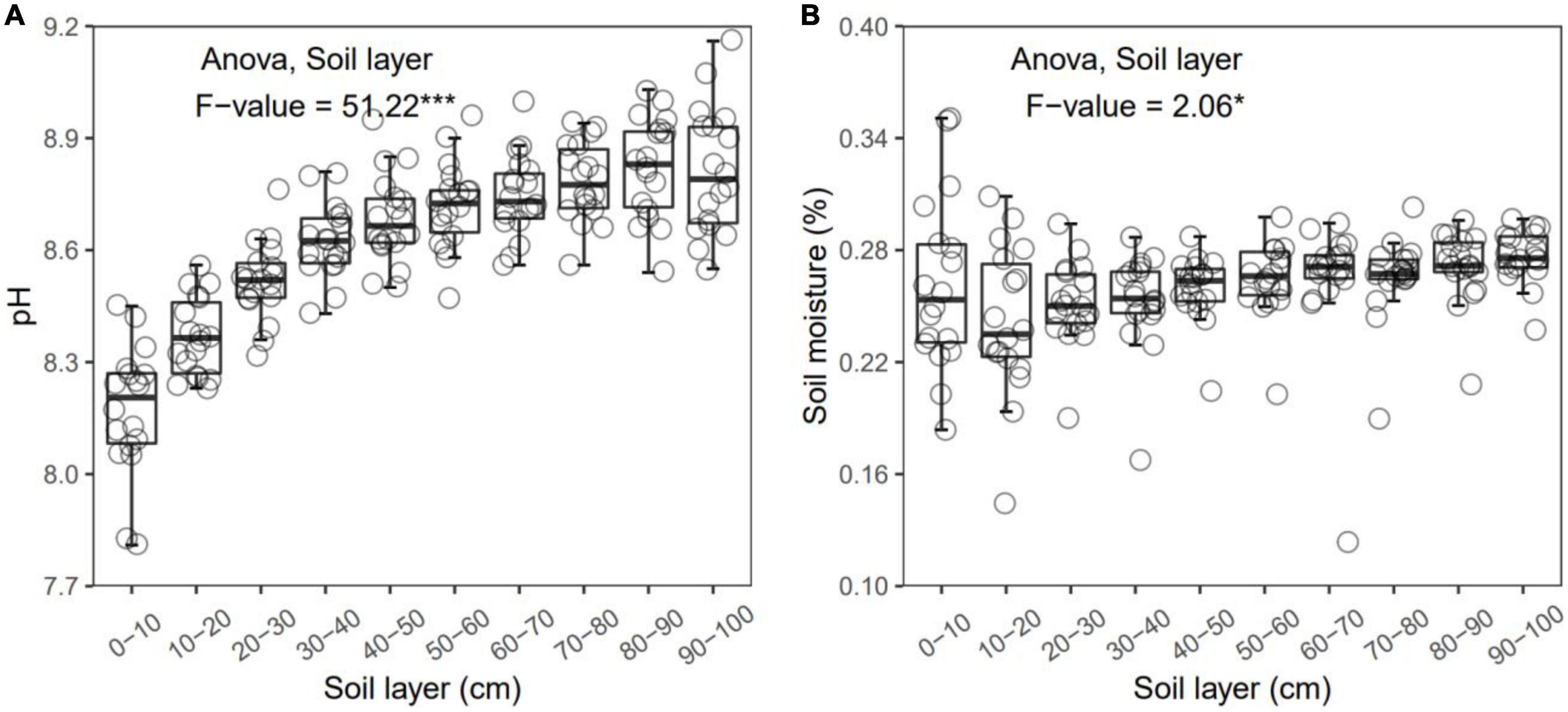
Figure 1. Soil pH (A) and moisture (B) in each layer along the soil profile. *p < 0.05; ***p < 0.001.
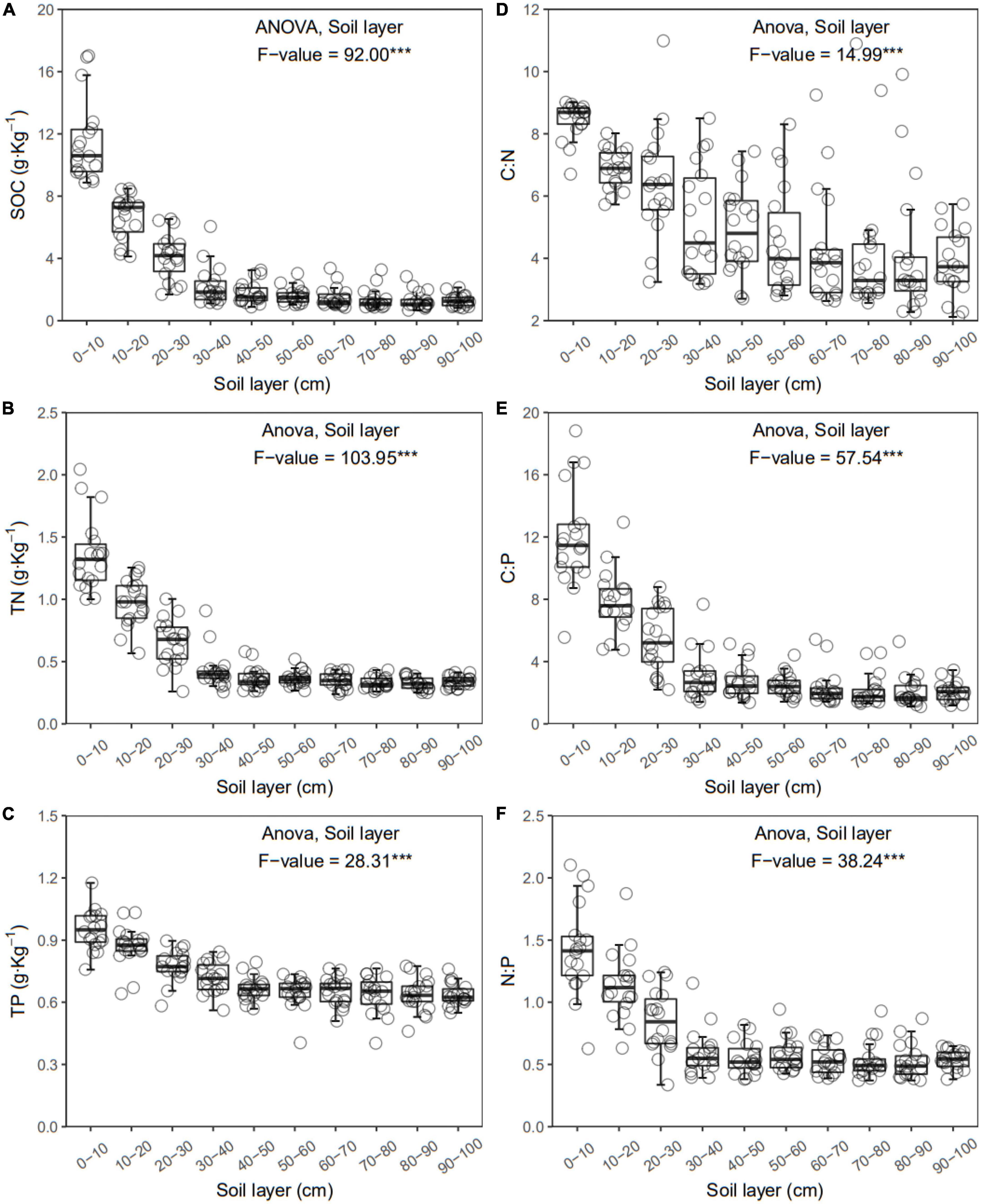
Figure 2. Soil organic carbon (SOC) (A), total nitrogen (TN) (B), total phosphorus (TP) (C), C:N (D), C:P (E), and N:P (F) of each layer along the soil profile. ***p < 0.001.
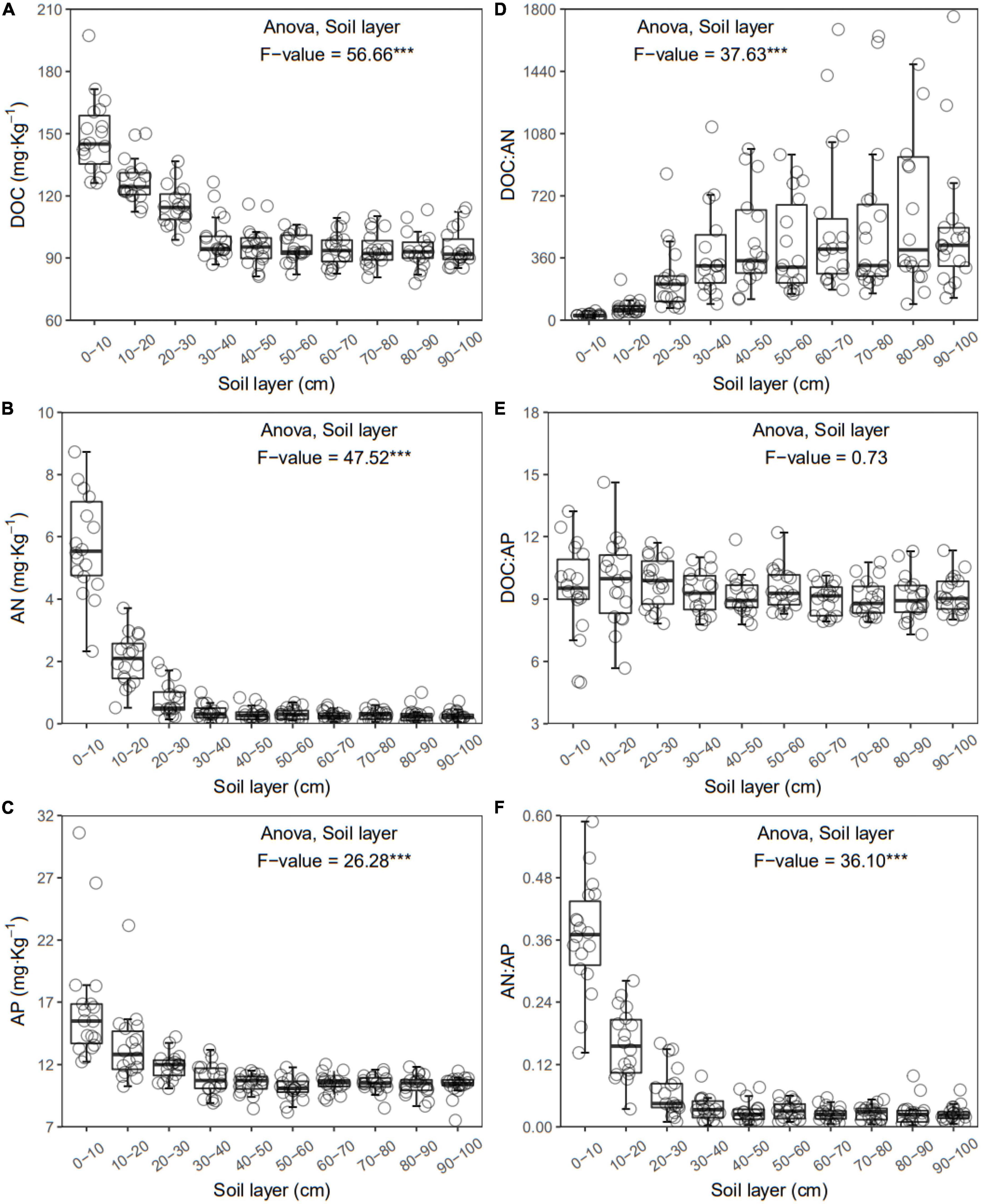
Figure 3. Dissolved organic carbon (DOC) (A), available nitrogen (AN) (B), available phosphorus (AP) (C), DOC:AN (D), DOC:AP (E), and AN:AP (F) in each layer along the soil profile. ***p < 0.001.
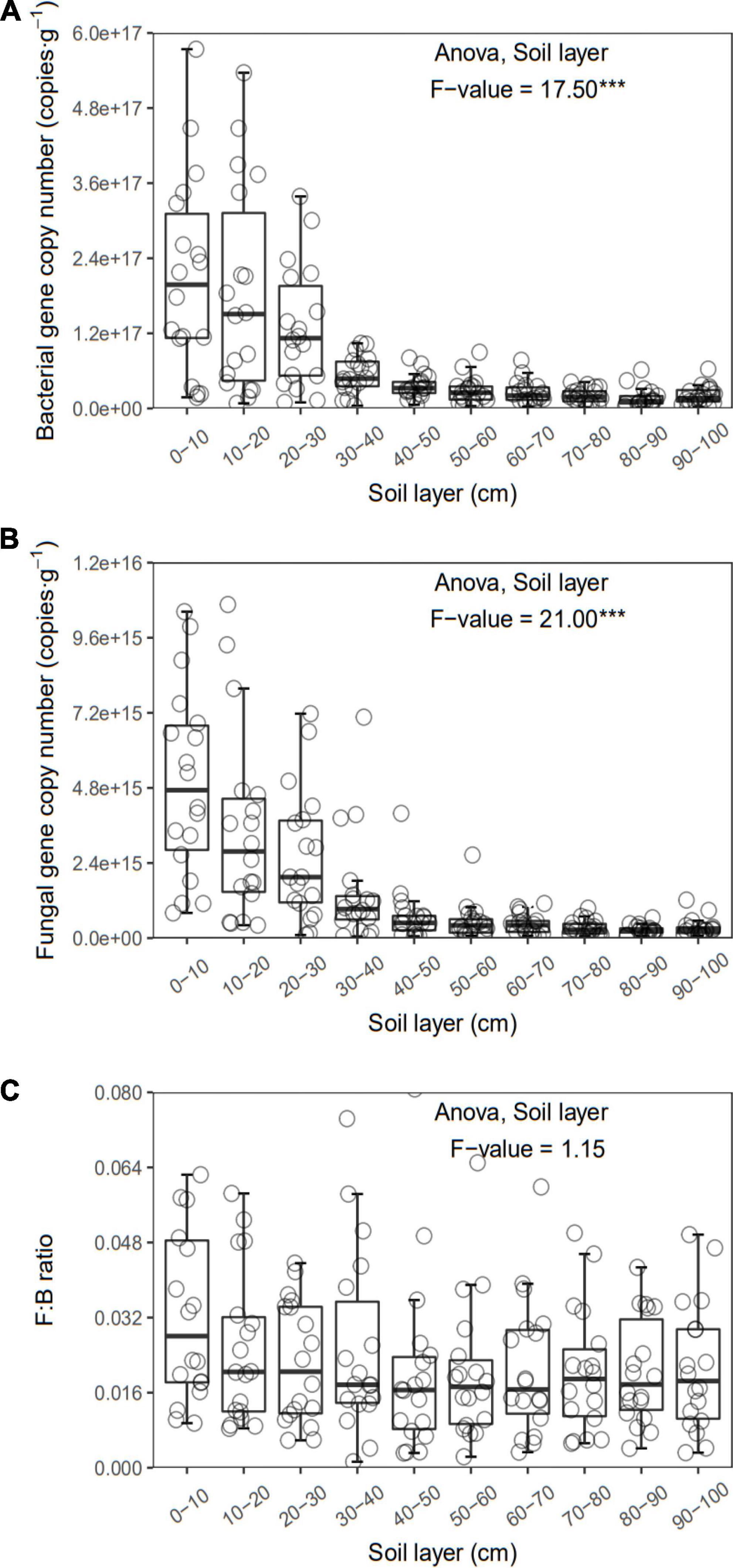
Figure 4. Bacterial (A) and fungal (B) gene copy number and F:B (C) of each layer along the soil profile. ***p < 0.001.
We determined whether there was an isometric relationship between N and C (i.e., deviation of the slope of the relationship between log N and log C from 1.0). The isometric hypothesis was confirmed at different soil depths if the scaling slope of N-C stoichiometric relationship was not significantly different from 1.0 within a 95% confidence interval. We used a log–log function to quantify the N-C stoichiometric relationship:
log y = a + b (log x) (1)
where x is C stock (g kg–1), y is N stock (g kg–1), a is the intercept, b is the scaling slope. To test the C-N stoichiometric relationship, we performed type II reduced major axis (RMA) regression with the software package “Standardized Major Axis Tests and Routines” (Yang and Luo, 2011).
Best subset (BSS) regression analyses were used to assess the common effect of soil environment, chemical properties and microbial properties on SOC storage with the ‘leaps’ package in R (version 4.1.1) (Doetterl et al., 2015). Regression of BSS tests all possible combinations of input predictors and finds a subset of size k that gives the highest predictive power with the lowest prediction error. We selected the best predictors based on adjusted R2 and corrected Bayesian information criterion (BICc). Model residuals were inspected for normality and variance homoscedasticity. The following predictors were taken into account in our analyses: (1) soil environment including soil pH and soil moisture, (2) soil chemical properties (TN, TP, DOC, AN, and AP) and (3) soil microbial properties (BCN, FCN, and F:B). We tested the variance inflation factor (VIF) of each predictor to avoid multicollinearity issues, all of which were lower than 10, indicating no multicollinearity among predictors. To assess the relative importance of BSS-determined predictors as drivers of SOC storage, we calculated the relative proportions of parameter estimates for each predictor to all parameter estimates in the model and grouped them into the above three groups. The higher coefficient values represent higher relative importance of predictors on SOC storage.
3. Results
3.1. Soil physiochemical and microbial properties
In general, all the soil physiochemical properties we measured varied greatly along the soil profile. In particular, soil pH and gravimetric moisture increased with increasing soil depth (all p < 0.05, Figure 1). Other soil properties, including SOC, TN, TP, C:N, C:P, N:P, DOC, AN, AP, and AN:AP, all decreased significantly along the soil profile to 1 m (all p < 0.05, Figures 2, 3). DOC:AN increased with increasing soil depth (p < 0.05, Figure 3) but DOC:AP did not change with increasing soil depth (p > 0.05, Figure 3). For example, SOC of the 0 – 10 cm sample was 11.48 g kg–1, 8.85 times higher than the 1.30 g kg–1 of the 90–100 cm sample. In terms of microbial properties, BCN and FCN decreased significantly with increasing soil depth (all p < 0.001, Figures 4A, B). We found that the F:B ranged from 0.0013 to 0.1006, with no significant differences among soil layers to 1 m depth (p > 0.05, Figure 4C).
3.2. C-N stoichiometric relationships
Scaling slopes of the C–N stoichiometric relationship between log N and log C significantly decreased with increasing soil depth (p < 0.05, Figure 5A). The scaling slopes of the 0–30 cm soil layers did not significantly differ from 1.0 (p < 0.05, Figure 5A), the scaling slopes of the 30–100 cm soil layers were much lower than 1.0 (all p > 0.05, Figure 5A). The stoichiometric relationships between C and N of the top- (0–30 cm) and subsoil (30–100 cm) layers were statistically different from each other (p < 0.05, Figure 5A), and C and N did not exhibit isometric relationships in the subsoil layer (Figure 5B).
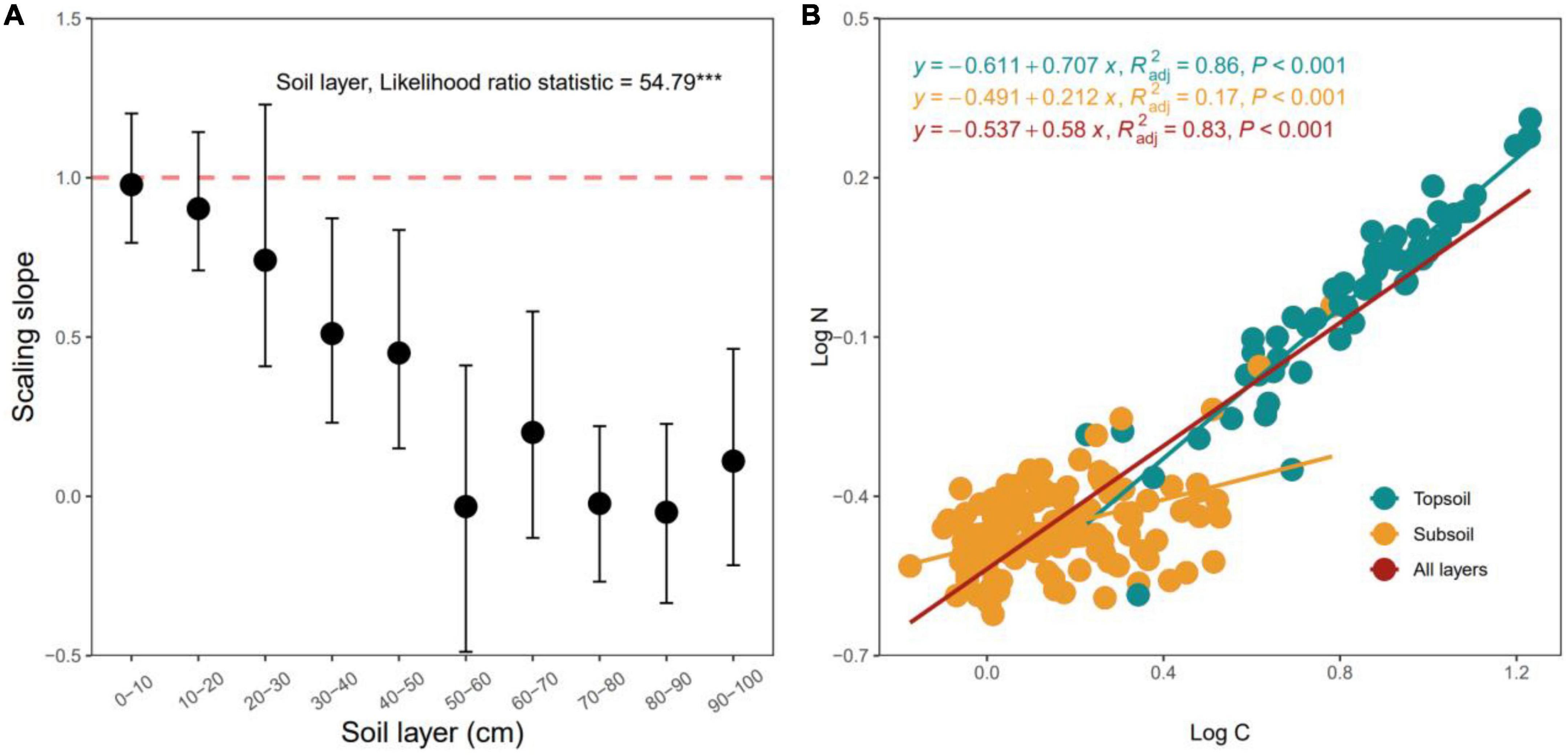
Figure 5. Variation in the scaling slopes of the N-C stoichiometric relationships along the soil profile (A) and C-N stoichiometric relationships for the topsoil, subsoil and all layers (B). The scaling slope indicates the slope of the type II (i.e., reduced major axis, RMA) relationship between log-transformed N and C. The error bar is the 95% confidence interval of the scaling slope of the relationship between log N and log C. The dashed line denotes the scaling slope is equal to 1.0, indicating the isometric scaling between N and C along the soil layer gradient. ***p < 0.001.
3.3. Factors regulating SOC variation
The best models explained 91.57, 88.27, and 82.95% of the variation in SOC content along all soil layers and for the top- and subsoil layers, respectively. Specifically, soil chemical properties were responsible for 55.62% of the explained variation in SOC across all soil layers (Figure 6A). Content of SOC significantly increased with TN and DOC (all p < 0.05, Figure 6A). Microbial properties including F:B and FCN accounted for 32.00% positive effects of the explained variation in SOC (Figure 6A). Content of SOC was negatively affected by soil environment and decreased significantly with soil pH (p < 0.05, Figure 6A).
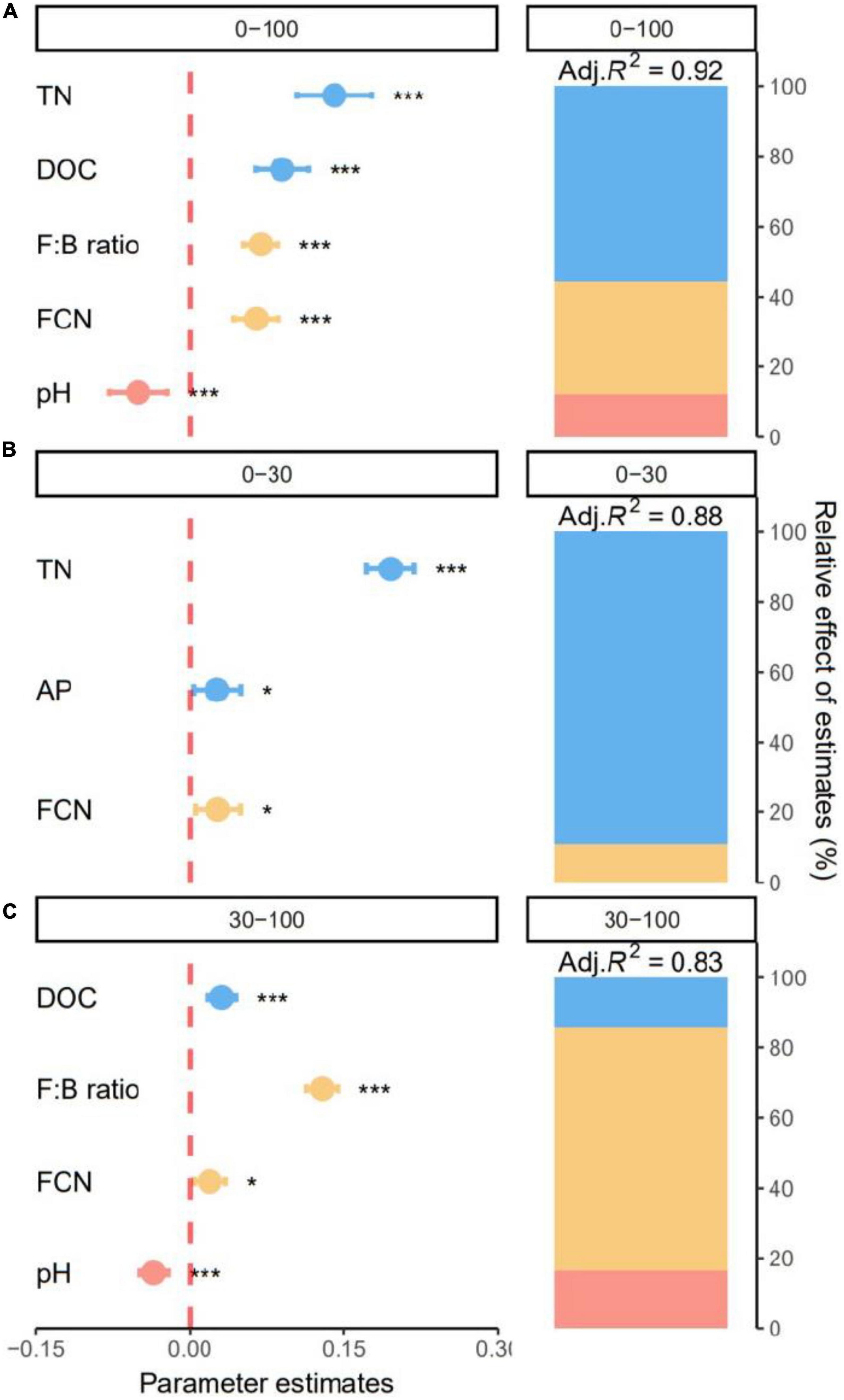
Figure 6. Effect of soil properties on soil organic carbon (SOC). Relative effects of soil chemical properties, soil environments, and microbial properties on soil organic carbon (SOC) across all soil layers (0–100 cm), (A) and for the topsoil (0–30 cm), (B) and subsoil (30–100 cm), (C) layers. Average parameter estimates (standardized regression coefficients) of model predictors, associated 95% confidence intervals and relative importance of each factor, expressed as the percentage of explained variation. The conditional R2 of the model and the p value of each predictor are given as: *p < 0.05; ***p < 0.001. TN, total nitrogen; DOC, dissolved organic C; FCN, fungal gene copy number; AP, available phosphorus.
In the topsoil, soil chemical properties dominated SOC variation. Soil TN was the major driver of SOC content, accounting for 89.08% of the explained variation (Figure 6B). Content of SOC increased significantly with TN and AP (all p < 0.05, Figure 6B). Additionally, SOC increased significantly with FCN (p < 0.05) and microbial properties accounted for 10.92% of the explained variation (Figure 6B). For the subsoil, the best model explained 82.95% of variation in SOC (Figure 6C). Soil environment, soil chemical properties, and microbial properties were responsible for 16.42, 14.51, and 69.07% of explained variation in SOC (Figure 6C). Specifically, subsoil SOC significantly increased with DOC, F:B and FCN and decreased with soil pH (all p < 0.05, Figure 6C).
4. Discussion
4.1. SOC distribution along the soil profile
In terrestrial ecosystems, SOC represents the largest organic C storage and is unevenly distributed among soil layers (Jobbágy and Jackson, 2000). Consistent with many previous studies (e.g., Wu et al., 2017; Wang et al., 2022; Xia et al., 2022), our results showed that the SOC content first decreased and then stabilized with the increase of soil depth. 66.92% of the SOC were stored in the 0 – 30 cm soil layer, which is larger than the average value of 54.00% across China (Wang et al., 2004). Theoretically, the vertical distribution pattern of SOC is mainly attributable to the vertical decrease in C input quantity and the C transformation through soil microbial decomposition (Antony et al., 2022). In the aboveground pathway, plant litter is processed by microbes on the soil surface to form SOC input to the topsoil SOC pool (Wynn et al., 2006). Most SOC is enriched in the topsoil, and only a small portion is transported to deeper soil layers (Wang et al., 2015). In the underground pathway, plant C enters the soil through root litter and exudates (Brady et al., 2008), which is processed by microbes and finally stored in the SOC pool (Prescott, 2010). Different from the above-ground pathway, that can only receive C inputs from the soil surface (Prescott and Vesterdal, 2021), plant C in the underground pathway is distributed vertically through the plant root system and can be directly input into the subsoil (Jobbágy and Jackson, 2000). However, its input capacity is strongly influenced by the vertical distribution pattern of plant roots (Yang et al., 2007). Limited by the uneven distribution of underground nutrients, approximately 60% of the global plant root biomass is concentrated in the top 30 cm of soil (Jackson et al., 1996). At the same experimental site, 85.95% of the fine root biomass is found in the top 30 cm soil layer (Geng et al., 2021). Topsoil with greater root biomass usually has higher input of root litter and exudates and thus accumulates more SOC (Yang et al., 2007).
The soil microbial community is also a transit pathway for SOC formation (Prescott and Vesterdal, 2021). Although the amount of plant input determines how much plant C initially enters the soil environment (Schimel and Schaeffer, 2012; Janzen, 2015), SOC formation requires microbial decomposition and transformation of plant C (Eswaran et al., 1993). Microbes increase SOC storage by forming microbial biomass and mineral-related residues in the process of decomposing plant-derived materials (Cotrufo and Lavallee, 2022). Higher soil microbial populations translates to a greater ability of soil microbes converting plant-derived materials to MBC pools (Liang et al., 2019), and increasing C storage. Our results showed that the vast majority of soil microbes, as indicated by BCN and FCN, were in the topsoil, rapidly decreasing with soil depth. Therefore, more SOC is integrated, and stored, in the topsoil through microbial processing (Miltner et al., 2012). In the subsoil, there is a relatively small number of soil microbes (Ekelund et al., 2001) that, together with the low input of plant-derived substances (Mikutta et al., 2006), results in a low level of SOC conversion (Gill et al., 1999). Stored SOC content is significantly lower in the sub- than the topsoil, even if SOC in the subsoil is more stable and can be stored for longer periods of time (Liang and Balser, 2008; Rumpel and Kögel-Knabner, 2011). Additionally, leaching may play an important role determining the vertical distribution of SOC since the relatively high precipitation in our study area. Plant litter combines microbial decomposition and transformation of C to jointly dictate the vertical distribution of SOC storage, which is significantly higher in the top- than subsoil (Prescott, 2010).
4.2. Isometric C-N relationships at different soil depths
We found a decrease in soil C:N along the soil profile, which has also been observed elsewhere (e.g., Jenkinson et al., 2008; Rumpel and Kögel-Knabner, 2011). This appears to be due to the highly processed subsoil C and the fact that the subsoil C:N is similar to that of the microbes themselves (Wallander et al., 2003). N was isometrically scaled with respect to C in the topsoil as has been frequently observed (e.g., Cleveland and Liptzin, 2007; Yang et al., 2010). This may be closely associated with the origin of SOC. Topsoil C and N predominantly come from above- and below-ground plant inputs that typically has an isometric relationship (Cleveland and Liptzin, 2007). Through decomposition and transformation, these plant-derived materials are stored in large quantities in soil, which, in turn, strongly affects the soil C-N relationship, resulting in a statistically strong log-log relationship between C and N in topsoil (Zhang et al., 2017). However, the relationship between C and N in the subsoil was not isometric— the slope was significantly lower than 1.0. This indicates that the C and N in the subsoil can become decoupled. One possible reason for this is that with increasing soil depth, the relationship between soil C and N gradually shifts from plant- to microbial-origin (Cotrufo et al., 2019). Indeed, the input of plant-derived materials decreased with increasing soil depth, and its contribution to the subsoil C-N relationship continued to decrease (Jobbágy and Jackson, 2000). Along the soil profile, soil C and N gradually changed to microbial-dominated, processed and transformed by microbes (Fang et al., 2005; Rumpel and Kögel-Knabner, 2011), and became more similar to the relationship of microbial C and N through microbial turnover (Dal Ferro et al., 2020). In the transition from plant- to microbial-dominated, the soil C and N in the subsoil decoupled. Indeed, the decoupling suggests that the subsoil N content did not decrease simultaneously with SOC. Underutilized N accumulating in the subsoil may stimulate C sequestration (Cotrufo et al., 2019). As a result, subsoil at our study site may have high potential for C storage, something that should be considered when predicting future C sequestration for poplar plantations in China.
4.3. Regulation of SOC at different soil depths
In general, we found that SOC storage was largely regulated by soil chemical properties and the microbial community across soil depths with a differentiation between the top- and subsoil layers. In the topsoil layer, SOC storage is mainly controlled by soil chemical properties. SOC storage increased with increasing TN content, which was consistent with the synchronous increase of soil SOC and N reported in many previous studies (e.g., Christopher and Lal, 2007; Khan et al., 2007; Liang et al., 2018). This may result from the contribution of plants to SOC storage and the regulation and preservation mechanisms of TN to SOC. First, plant matter is the largest source of SOC and its stoichiometric ratio leads to the accumulation of SOC (Cai et al., 2022). High soil TN ensures the supply of N to plants. The mechanisms of plant root metabolism (Luo et al., 2017), mean that high N content can stimulate plant root growth and increase plant productivity (Quinn et al., 2010), thereby increasing C inputs to soil. Second, high N content effectively reduces SOC mineralization (Lu et al., 2021), probably due to N altering microbial nutrient requirements and delaying SOC decomposition (Rousk et al., 2010; Chen et al., 2015). At the same time, high N content can acidify soils (Guo et al., 2010), altering the microbial community structure and decreasing microbial biomass and respiration intensity (Nottingham et al., 2015). Soil N can also regulate soil C accumulation in mineral-associated components through the formation of SOC complexes by polyvalent Fe and Al release in the N cycle (Lal et al., 1997; Mueller et al., 2012). This increases SOC stability and shields C from microbial decomposition. Therefore, SOC is generally higher in topsoil layers with higher soil TN content.
Soil AP and FCN are also positive drivers of topsoil SOC storage. This may result from the stimulating effect of soil AP on the diversity and abundance of fungal communities, which indirectly promotes SOC storage (Bradford et al., 2008; Li et al., 2016). P is required for microbial physiological processes, including synthesis of enzymes and phospholipids, as well as fungal mycorrhiza growth (Averill and Waring, 2018; Johnston et al., 2019). The accumulation of microbial necromass was significantly correlated with the turnover of microbial metabolic by-products, regulated by soil P content (Yuan et al., 2021). Increasing soil AP leads to increasing fungi-derived microbial necromass, thereby enhancing their contribution to SOC (Luo et al., 2022).
Unlike topsoil, we found soil fungal abundance and F:B positively dominated SOC storage. Microbes are drivers of SOC turnover processes, and their unique physiological predispositions have significant effects on organic C storage (De Vries et al., 2006). Our results are consistent with previous findings that SOC content was positively influenced by fungal abundance as well as by F:B (e.g., Bailey et al., 2002; Six et al., 2006; Ananyeva et al., 2015; Bhattacharya et al., 2016). Although soil microbial communities are typically dominated by bacteria, fungi play a disproportionately important role in SOC storage (Nemergut et al., 2008; Khan et al., 2016). This may result from fungal physiology that allows it to retain organic C (Peng et al., 2013; Kumar and Ghoshal, 2017). Compared to bacteria, fungi have higher microbial growth efficiency and produce more microbial biomass and by-products (Liu et al., 2022). As fungal proportion increases, microbial communities will retain more C and release less CO2 per unit of substrate consumed (Bailey et al., 2002). Moreover, where there is a higher proportion of fungi in the microbial community, microbial-derived organics degrade more slowly because fungal products are more chemically stable and are protected from decomposition by binding to minerals and soil aggregates (Simpson et al., 2004; Bhattacharya et al., 2016; Liang et al., 2017). These phenomena result in the high positive correlations observed between microbial properties and SOC storage in subsoil.
In the subsoil, DOC is also an important source of the stabilized C. We found that subsoil organic C sequestration increased with DOC content. According to the DOC transport mechanism (Guggenberger and Kaiser, 2003; Kaiser and Kalbitz, 2012), DOC is affected by rainfall and water flow (Schrumpf et al., 2013), and is released from saturated soil mineral adsorption sites in surface soil layers (Sanderman et al., 2008). It is further absorbed and processed by microbes and eventually transported to deeper soil layers where it supports microbial community activity and the formation of recalcitrant organic C (e.g., microbial necromass) (Neff and Asner, 2001; Cotrufo et al., 2013), especially in subsoil where fungi dominate SOC storage (Sae-Tun et al., 2022). Interestingly, we observed that soil environmental factors were relatively minor controls of SOC in either top- or subsoil, possibly because our study was located in a plantation with little environmental heterogeneity.
5. Conclusion
We investigated the vertical distribution pattern of SOC storage and soil C-N coupling relationships using data from 18 soil profiles to 1 m depth in a coastal poplar plantation. We applied comprehensive analyses, accounting for of soil environment, soil physiochemical and microbial properties to better understand the regulating factors of SOC storage. We found SOC storage was two times higher in the topsoil (0–30 cm) than the subsoil (30–100 cm), mainly attributable to the enrichment of aboveground litter and roots of plants in the topsoil. Contrary to the strong log-log C-N relationship in topsoil, C and N in subsoil was not isometric with a slope significantly lower than 1.0, suggesting a higher C sequestration potential. The distribution of SOC along the soil profile was generally regulated by soil chemical and microbial properties with TN and AP dominant in the topsoil and FCN and F:B dominant in the subsoil. Fungi, and its ratio to bacteria, played positive roles in SOC storage, especially at deep soil layers. Our results suggest that to accurately assess soil C potential and the reliability of applying climate variables to simulate soil C storage, the influence of microbial community structure must be considered and more attention should be paid to subsoils.
Data availability statement
The original contributions presented in this study are included in the article/supplementary material, further inquiries can be directed to the corresponding author.
Author contributions
XX conceived the idea and designed the study. BL collected and analyzed the data with help from CX, LG, NL, ZZ, and QL. BL and XX prepared the manuscript with input from all authors. All authors contributed to the project conceptualization, assisted in the study and/or manuscript preparation.
Funding
This study was financially supported by the National Key Research and Development Program of China (2022YFD210020002), the Jiangsu Agriculture Science and Technology Innovation Fund (CX(20)3113), the National Natural Science Foundation of China (31700376), the Natural Science Key Fund for Colleges and Universities of Jiangsu, China (17KJA180006), the “5151” Talent Program of Nanjing Forestry University, and the Priority Academic Program Development of Jiangsu Higher Education Institutions (PAPD).
Acknowledgments
We thank our many colleagues for their help with field and lab work.
Conflict of interest
The authors declare that the research was conducted in the absence of any commercial or financial relationships that could be construed as a potential conflict of interest.
Publisher’s note
All claims expressed in this article are solely those of the authors and do not necessarily represent those of their affiliated organizations, or those of the publisher, the editors and the reviewers. Any product that may be evaluated in this article, or claim that may be made by its manufacturer, is not guaranteed or endorsed by the publisher.
References
Ananyeva, N. D., Castaldi, S., Stolnikova, E. V., Kudeyarov, V. N., and Valentini, R. (2015). Fungi-to-bacteria ratio in soils of European Russia. Arch. Agron. Soil Sci. 61, 427–446. doi: 10.1080/03650340.2014.940916
Antony, D., Collins, C. D., Clark, J. M., and Sizmur, T. (2022). Soil organic matter storage in temperate lowland arable, grassland and woodland topsoil and subsoil. Soil Use Manag. 38, 1532–1546. doi: 10.1111/sum.12801
Averill, C., and Waring, B. (2018). Nitrogen limitation of decomposition and decay: How can it occur? Glob. Change Biol. 24, 1417–1427. doi: 10.1111/gcb.13980
Averill, C., Turner, B. L., and Finzi, A. C. (2014). Mycorrhiza-mediated competition between plants and decomposers drives soil carbon storage. Nature 505, 543–545. doi: 10.1038/nature12901
Bailey, V. L., Smith, J. L., and Bolton, H. (2002). Fungal-to-bacterial ratios in soils investigated for enhanced C sequestration. Soil Biol. Biochem. 34, 997–1007. doi: 10.1016/S0038-0717(02)00033-0
Batjes, N. H. (1996). Total carbon and nitrogen in the soils of the world. Eur. J. Soil Sci. 47, 151–163. doi: 10.1111/j.1365-2389.1996.tb01386.x
Bhattacharya, S. S., Kim, K. H., Das, S., Uchimiya, M., Jeon, B. H., Kwon, E., et al. (2016). A review on the role of organic inputs in maintaining the soil carbon pool of the terrestrial ecosystem. J. Environ. Manag. 167, 214–227. doi: 10.1016/j.jenvman.2015.09.042
Bian, H., Geng, Q., Xiao, H., Shen, C., Li, Q., Cheng, X., et al. (2019). Fine root biomass mediates soil fauna community in response to nitrogen addition in poplar plantations (Populus deltoids) on the east coast of China. Forests 10:122. doi: 10.3390/f10020122
Block, R. M. A., Van Rees, K. C. J., and Knight, J. D. (2006). A review of fine root dynamics in Populus plantations. Agrofor. Syst. 67, 73–84. doi: 10.1007/s10457-005-2002-7
Bradford, M. A., Fierer, N., Jackson, R. B., Maddox, T. R., and Reynolds, J. F. (2008). Nonlinear root-derived carbon sequestration across a gradient of nitrogen and phosphorous deposition in experimental mesocosms. Glob. Change Biol. 14, 1113–1124. doi: 10.1111/j.1365-2486.2008.01564.x
Brady, N. C., Weil, R. R., and Weil, R. R. (2008). The nature and properties of soils. Upper Saddle River, NJ: Prentice Hall.
Cai, Z., Yan, X., and Gu, B. (2022). Applying C:N ratio to assess the rationality of estimates of carbon sequestration in terrestrial ecosystems and nitrogen budgets. Carbon Res. 1:2. doi: 10.1007/s44246-022-00004-6
Chen, D., Li, J., Lan, Z., Hu, S., Bai, Y., and Niu, S. (2015). Soil acidification exerts a greater control on soil respiration than soil nitrogen availability in grasslands subjected to long-term nitrogen enrichment. Funct. Ecol. 30, 658–669. doi: 10.1111/1365-2435.12525
Chen, J., Wang, H., Hu, G., Li, X., Dong, Y., Zhuge, Y., et al. (2021). Distinct accumulation of bacterial and fungal residues along a salinity gradient in coastal salt-affected soils. Soil Biol. Biochem. 158:108266. doi: 10.1016/j.soilbio.2021.108266
Christopher, S. F., and Lal, R. (2007). Nitrogen management affects carbon sequestration in North American cropland soils. Crit. Rev. Plant Sci. 26, 45–64. doi: 10.1080/07352680601174830
Cleveland, C. C., and Liptzin, D. (2007). C:N:P stoichiometry in soil: Is there a “Redfield ratio” for the microbial biomass? Biogeochemistry 85, 235–252. doi: 10.1007/s10533-007-9132-0
Cotrufo, M. F., and Lavallee, J. M. (2022). Soil organic matter formation, persistence, and functioning: A synthesis of current understanding to inform its conservation and regeneration. Adv. Agron. 172, 1–66.
Cotrufo, M. F., Ranalli, M. G., Haddix, M. L., Six, J., and Lugato, E. (2019). Soil carbon storage informed by particulate and mineral-associated organic matter. Nat. Geosci. 12, 989–994. doi: 10.1038/s41561-019-0484-6
Cotrufo, M. F., Wallenstein, M. D., Boot, C. M., Denef, K., and Paul, E. (2013). The microbial efficiency-matrix stabilization (MEMS) framework integrates plant litter decomposition with soil organic matter stabilization: Do labile plant inputs form stable soil organic matter? Glob. Change Biol. 19, 988–995. doi: 10.1111/gcb.12113
Dai, Z., Su, W., Chen, H., Barberan, A., Zhao, H., Yu, M., et al. (2018). Long-term nitrogen fertilization decreases bacterial diversity and favors the growth of Actinobacteria and Proteobacteria in agro-ecosystems across the globe. Glob. Change Biol. 24, 3452–3461. doi: 10.1111/gcb.14163
Dal Ferro, N., Piccoli, I., Berti, A., Polese, R., and Morari, F. (2020). Organic carbon storage potential in deep agricultural soil layers: Evidence from long-term experiments in northeast Italy. Agric. Ecosyst. Environ. 300:106967. doi: 10.1016/j.agee.2020.106967
De Vries, F. T., Hoffland, E., Van Eekeren, N., Brussaard, L., and Bloem, J. (2006). Fungal/bacterial ratios in grasslands with contrasting nitrogen management. Soil Biol. Biochem. 38, 2092–2103. doi: 10.1016/j.soilbio.2006.01.008
Doetterl, S., Stevens, A., Six, J., Merckx, R., Van Oost, K., Casanova Pinto, M., et al. (2015). Soil carbon storage controlled by interactions between geochemistry and climate. Nat. Geosci. 8, 780–783. doi: 10.1038/ngeo2516
Ekelund, F., Rønn, R., and Christensen, S. (2001). Distribution with depth of protozoa, bacteria and fungi in soil profiles from three Danish forest sites. Soil Biol. Biochem. 33, 475–481. doi: 10.1016/S0038-0717(00)00188-7
Eswaran, H., Van Den Berg, E., and Reich, P. (1993). Organic carbon in soils of the world. Soil Sci. Soc. Am. J. 57, 192–194. doi: 10.2136/sssaj1993.03615995005700010034x
Fang, C., Smith, P., Moncrieff, J. B., and Smith, J. U. (2005). Similar response of labile and resistant soil organic matter pools to changes in temperature. Nature 433, 57–59. doi: 10.1038/nature03138
Fanin, N., Hättenschwiler, S., Schimann, H., Fromin, N., and Bailey, J. K. (2014). Interactive effects of C, N and P fertilization on soil microbial community structure and function in an Amazonian rain forest. Funct. Ecol. 29, 140–150. doi: 10.1111/1365-2435.12329
Fierer, N., Jackson, J. A., Vilgalys, R., and Jackson, R. B. (2005). Assessment of soil microbial community structure by use of taxon-specific quantitative PCR assays. Appl. Environ. Microbiol. 71, 4117–4120. doi: 10.1128/Aem.71.7.4117-4120.2005
Garten, C. T., Wullschleger, S. D., and Classen, A. T. (2011). Review and model-based analysis of factors influencing soil carbon sequestration under hybrid poplar. Biomass Bioenerg. 35, 214–226. doi: 10.1016/j.biombioe.2010.08.013
Geng, Q., Ma, X., Liao, J., Wu, W., Niu, S., Luo, Y., et al. (2021). Contrasting nutrient-mediated responses between surface and deep fine root biomass to N addition in poplar plantations on the east coast of China. For. Ecol.Manag. 490:119152. doi: 10.1016/j.foreco.2021.119152
Gill, R., Burke, I. C., Milchunas, D. G., and Lauenroth, W. K. (1999). Relationship between root biomass and soil organic matter pools in the shortgrass steppe of Eastern Colorado. Ecosystems 2, 226–236. doi: 10.1007/s100219900070
Gross, C. D., and Harrison, R. B. (2019). The case for digging deeper: Soil organic carbon storage, dynamics, and controls in our changing world. Soil Syst. 3:28. doi: 10.3390/soilsystems3020028
Guggenberger, G., and Kaiser, K. (2003). Dissolved organic matter in soil: Challenging the paradigm of sorptive preservation. Geoderma 113, 293–310. doi: 10.1016/s0016-7061(02)00366-x
Guo, J. H., Liu, X. J., Zhang, Y., Shen, J. L., Han, W. X., Zhang, W. F., et al. (2010). Significant acidification in major Chinese croplands. Science 327, 1008–1010. doi: 10.1126/science.1182570
Heitkötter, J., Heinze, S., and Marschner, B. (2017). Relevance of substrate quality and nutrients for microbial C-turnover in top and subsoil of a Dystric Cambisol. Geoderma 302, 89–99. doi: 10.1016/j.geoderma.2017.04.029
Jackson, R. B., Canadell, J., Ehleringer, J. R., Mooney, H. A., Sala, O. E., and Schulze, E. D. (1996). A global analysis of root distributions for terrestrial biomes. Oecologia 108, 389–411. doi: 10.1007/Bf00333714
Jackson, R. B., Lajtha, K., Crow, S. E., Hugelius, G., Kramer, M. G., and Piñeiro, G. (2017). The ecology of soil carbon: Pools, vulnerabilities, and biotic and abiotic controls. Annu. Rev. Ecol. Evol. Syst. 48, 419–445. doi: 10.1146/annurev-ecolsys-112414-054234
Janzen, H. (2015). Beyond carbon sequestration: Soil as conduit of solar energy. Eur. J. Soil Sci. 66, 19–32. doi: 10.1111/ejss.12194
Janzen, H. H. (2006). The soil carbon dilemma: Shall we hoard it or use it? Soil Biol. Biochem. 38, 419–424. doi: 10.1016/j.soilbio.2005.10.008
Jenkinson, D. S., Poulton, P. R., and Bryant, C. (2008). The turnover of organic carbon in subsoils. Part 1. Natural and bomb radiocarbon in soil profiles from the Rothamsted long-term field experiments. Eur. J. Soil Sci. 59, 391–399. doi: 10.1111/j.1365-2389.2008.01025.x
Jobbágy, E. G., and Jackson, R. B. (2000). The vertical distribution of soil organic carbon and its relation to climate and vegeation. Ecol. Appl. 10, 423–436.
Johnston, E. R., Kim, M., Hatt, J. K., Phillips, J. R., Yao, Q., Song, Y., et al. (2019). Phosphate addition increases tropical forest soil respiration primarily by deconstraining microbial population growth. Soil Biol. Biochem. 130, 43–54. doi: 10.1016/j.soilbio.2018.11.026
Kaiser, K., and Kalbitz, K. (2012). Cycling downwards–dissolved organic matter in soils. Soil Biol. Biochem. 52, 29–32. doi: 10.1016/j.soilbio.2012.04.002
Khan, K. S., Mack, R., Castillo, X., Kaiser, M., and Joergensen, R. G. (2016). Microbial biomass, fungal and bacterial residues, and their relationships to the soil organic matter C/N/P/S ratios. Geoderma 271, 115–123. doi: 10.1016/j.geoderma.2016.02.019
Khan, S. A., Mulvaney, R. L., Ellsworth, T. R., and Boast, C. W. (2007). The myth of nitrogen fertilization for soil carbon sequestration. J. Environ. Qual. 36, 1821–1832. doi: 10.2134/jeq2007.0099
Kohl, L., Laganière, J., Edwards, K. A., Billings, S. A., Morrill, P. L., Van Biesen, G., et al. (2015). Distinct fungal and bacterial δ13C signatures as potential drivers of increasing δ13C of soil organic matter with depth. Biogeochemistry 124, 13–26. doi: 10.1007/s10533-015-0107-2
Koranda, M., Kaiser, C., Fuchslueger, L., Kitzler, B., Sessitsch, A., Zechmeister-Boltenstern, S., et al. (2014). Fungal and bacterial utilization of organic substrates depends on substrate complexity and N availability. FEMS Microbiol. Ecol. 87, 142–152. doi: 10.1111/1574-6941.12214
Kumar, C. M., and Ghoshal, N. (2017). Impact of land-use change on soil microbial community composition and organic carbon content in the dry tropics. Pedosphere 27, 974–977. doi: 10.1016/s1002-0160(17)60404-1
Lal, R. (2004). Soil carbon sequestration impacts on global climate change and food security. Science 304, 1623–1627. doi: 10.1126/science.1097396
Lal, R., Kimble, J. M., Follett, R. F., and Stewart, B. A. (1997). Soil processes and the carbon cycle. Boca Raton, FL: CRC Press.
Lal, R., Negassa, W., and Lorenz, K. (2015). Carbon sequestration in soil. Curr. Opin. Environ. Sustain. 15, 79–86. doi: 10.1016/j.cosust.2015.09.002
Lefèvre, C., Rekik, F., Alcantara, V., and Wiese, L. (2017). Soil organic carbon: The hidden potential. Rome: Food and Agriculture Organization of the United Nations (FAO).
Lehmann, J., and Kleber, M. (2015). The contentious nature of soil organic matter. Nature 528, 60–68. doi: 10.1038/nature16069
Li, Y., Chen, H., Song, Q., Liao, J., Xu, Z., Huang, S., et al. (2018). Changes in soil arthropod abundance and community structure across a poplar plantation chronosequence in reclaimed coastal saline soil. Forests 9:644. doi: 10.3390/f9100644
Li, Y., Niu, S., and Yu, G. (2016). Aggravated phosphorus limitation on biomass production under increasing nitrogen loading: A meta-analysis. Glob. Change Biol. 22, 934–943. doi: 10.1111/gcb.13125
Liang, C., Amelung, W., Lehmann, J., and Kästner, M. (2019). Quantitative assessment of microbial necromass contribution to soil organic matter. Glob. Change Biol. 25, 3578–3590. doi: 10.1111/gcb.14781
Liang, C., and Balser, T. C. (2008). Preferential sequestration of microbial carbon in subsoils of a glacial-landscape toposequence, Dane County, WI, USA. Geoderma 148, 113–119. doi: 10.1016/j.geoderma.2008.09.012
Liang, C., Schimel, J. P., and Jastrow, J. D. (2017). The importance of anabolism in microbial control over soil carbon storage. Nat. Microbiol. 2:17105. doi: 10.1038/nmicrobiol.2017.105
Liang, X., Liu, S., Wang, H., and Wang, J. (2018). Variation of carbon and nitrogen stoichiometry along a chronosequence of natural temperate forest in northeastern China. J. Plant Ecol. 11, 339–350. doi: 10.1093/jpe/rtx008
Liu, X., Peng, C., Zhang, W., Li, S., An, T., Xu, Y., et al. (2022). Subsoiling tillage with straw incorporation improves soil microbial community characteristics in the whole cultivated layers: A one-year study. Soil Tillage Res. 215:105188. doi: 10.1016/j.still.2021.105188
Liu, Y., Ma, W., Kou, D., Niu, X., Wang, T., Chen, Y., et al. (2019). How deep do we dig for surface soil? A comparison of patterns of microbial C:N:P stoichiometry between topsoil and subsoil along an aridity gradient. Biogeosci. Discuss. 17, 2009–2019. doi: 10.5194/bg-2019-351
Lu, X., Vitousek, P. M., Mao, Q., Gilliam, F. S., Luo, Y., Turner, B. L., et al. (2021). Nitrogen deposition accelerates soil carbon sequestration in tropical forests. Proc. Natl. Acad. Sci. U.S.A. 118:e2020790118. doi: 10.1073/pnas.2020790118
Luo, C., Zhang, B., Liu, J., Wang, X., Han, F., and Zhou, J. (2020). Effects of different ages of Robinia pseudoacacia plantations on soil physiochemical properties and microbial communities. Sustainability 12:9161. doi: 10.3390/su12219161
Luo, R., Kuzyakov, Y., Zhu, B., Qiang, W., Zhang, Y., and Pang, X. (2022). Phosphorus addition decreases plant lignin but increases microbial necromass contribution to soil organic carbon in a subalpine forest. Glob. Change Biol. 28, 4194–4210. doi: 10.1111/gcb.16205
Luo, Z., Feng, W., Luo, Y., Baldock, J., and Wang, E. (2017). Soil organic carbon dynamics jointly controlled by climate, carbon inputs, soil properties and soil carbon fractions. Glob. Change Biol. 23, 4430–4439. doi: 10.1111/gcb.13767
Luo, Z., Wang, G., and Wang, E. (2019). Global subsoil organic carbon turnover times dominantly controlled by soil properties rather than climate. Nat. Commun. 10:3688. doi: 10.1038/s41467-019-11597-9
Lynch, J. M., and Bragg, E. (1985). Microoranisms and soil aggregate stability. Adv. Soil Sci. 2, 133–171. doi: 10.1007/978-1-4612-5088-3_3
Magnani, F., Mencuccini, M., Borghetti, M., Berbigier, P., Berninger, F., Delzon, S., et al. (2007). The human footprint in the carbon cycle of temperate and boreal forests. Nature 447, 849–851. doi: 10.1038/nature05847
Malik, A. A., Chowdhury, S., Schlager, V., Oliver, A., Puissant, J., Vazquez, P. G., et al. (2016). Soil fungal: Bacterial ratios are linked to altered carbon cycling. Front. Microbiol. 7:1247. doi: 10.3389/fmicb.2016.01247
Meersmans, J., Van Wesemael, B., De Ridder, F., Fallas Dotti, M., De Baets, S., and Van Molle, M. (2009). Changes in organic carbon distribution with depth in agricultural soils in northern Belgium, 1960-2006. Glob. Change Biol. 15, 2739–2750. doi: 10.1111/j.1365-2486.2009.01855.x
Mikutta, R., Kleber, M., Torn, M. S., and Jahn, R. (2006). Stabilization of soil organic matter: Association with minerals or chemical recalcitrance? Biogeochemistry 77, 25–56. doi: 10.1007/s10533-005-0712-6
Milne, E., Banwart, S. A., Noellemeyer, E., Abson, D. J., Ballabio, C., Bampa, F., et al. (2015). Soil carbon, multiple benefits. Environ. Dev. 13, 33–38. doi: 10.1016/j.envdev.2014.11.005
Miltner, A., Bombach, P., Schmidt-Brücken, B., and Kästner, M. (2012). SOM genesis: Microbial biomass as a significant source. Biogeochemistry 111, 41–55. doi: 10.1007/s10533-011-9658-z
Miranda, K. M., Espey, M. G., and Wink, D. A. (2001). A rapid, simple spectrophotometric method for simultaneous detection of nitrate and nitrite. Nitric Oxide 5, 62–71. doi: 10.1006/niox.2000.0319
Mueller, K. E., Eissenstat, D. M., and Hobbie, S. E. (2012). Tree species effects on coupled cycles of carbon, nitrogen, and acidity in mineral soils at a common garden experiment. Biogeochemistry 111, 601–614. doi: 10.1007/sl0533-011-9695-7
Neff, J. C., and Asner, G. P. (2001). Dissolved organic carbon in terrestrial ecosystems: Synthesis and a model. Ecosystems 4, 29–48. doi: 10.1007/s100210000058
Nemergut, D. R., Townsend, A. R., Sattin, S. R., Freeman, K. R., Fierer, N., Neff, J. C., et al. (2008). The effects of chronic nitrogen fertilization on alpine tundra soil microbial communities: Implications for carbon and nitrogen cycling. Environ. Microbiol. 10, 3093–3105. doi: 10.1111/j.1462-2920.2008.01735.x
Ni, X., Liao, S., Tan, S., Peng, Y., Wang, D., Yue, K., et al. (2020). The vertical distribution and control of microbial necromass carbon in forest soils. Glob. Ecol. Biogeogr. 29, 1829–1839. doi: 10.1111/geb.13159
Nottingham, A. T., Turner, B. L., Stott, A. W., and Tanner, E. V. (2015). Nitrogen and phosphorus constrain labile and stable carbon turnover in lowland tropical forest soils. Soil Biol. Biochem. 80, 26–33. doi: 10.1016/j.soilbio.2014.09.012
Peng, S., Guo, T., and Liu, G. (2013). The effects of arbuscular mycorrhizal hyphal networks on soil aggregations of purple soil in southwest China. Soil Biol. Biochem. 57, 411–417. doi: 10.1016/j.soilbio.2012.10.026
Peñuelas, J., Sardans, J., Rivas-Ubach, A., and Janssens, I. A. (2012). The human-induced imbalance between C, N and P in earth’s life system. Glob. Change Biol. 18, 3–6. doi: 10.1111/j.1365-2486.2011.02568.x
Prescott, C. E. (2010). Litter decomposition: What controls it and how can we alter it to sequester more carbon in forest soils? Biogeochemistry 101, 133–149. doi: 10.1007/s10533-010-9439-0
Prescott, C. E., and Vesterdal, L. (2021). Decomposition and transformations along the continuum from litter to soil organic matter in forest soils. For. Ecol. Manag. 498:119522. doi: 10.1016/j.foreco.2021.119522
Quinn, T. R., Canham, C. D., Weathers, K. C., and Goodale, C. L. (2010). Increased tree carbon storage in response to nitrogen deposition in the US. Nat. Geosci. 3, 13–17. doi: 10.1038/ngeo721
R Core Team (2021). R: A language and environment for statistical computing (4.0.4). Vienna, Austria: R Foundation for Statistical Computing.
Reich, P. B., Hobbie, S. E., Lee, T., Ellsworth, D. S., West, J. B., Tilman, D., et al. (2006). Nitrogen limitation constrains sustainability of ecosystem response to CO2. Nature 440, 922–925. doi: 10.1038/nature04486
Ren, C., Wang, J., Bastida, F., Delgado-Baquerizo, M., Yang, Y., Wang, J., et al. (2022). Microbial traits determine soil C emission in response to fresh carbon inputs in forests across biomes. Glob. Change Biol. 28, 1516–1528. doi: 10.1111/gcb.16004
Rousk, J., Brookes, P. C., and Bååth, E. (2010). Investigating the mechanisms for the opposing pH relationships of fungal and bacterial growth in soil. Soil Biol. Biochem. 42, 926–934. doi: 10.1016/j.soilbio.2010.02.009
Rubino, M., Dungait, J. A. J., Evershed, R. P., Bertolini, T., De Angelis, P., D’onofrio, A., et al. (2010). Carbon input belowground is the major C flux contributing to leaf litter mass loss: Evidences from a 13 C labelled-leaf litter experiment. Soil Biol. Biochem. 42, 1009–1016. doi: 10.1016/j.soilbio.2010.02.018
Rumpel, C., and Kögel-Knabner, I. (2011). Deep soil organic matter—a key but poorly understood component of terrestrial C cycle. Plant Soil 338, 143–158. doi: 10.1007/s11104-010-0391-5
Sae-Tun, O., Bodner, G., Rosinger, C., Zechmeister-Boltenstern, S., Mentler, A., and Keiblinger, K. (2022). Fungal biomass and microbial necromass facilitate soil carbon sequestration and aggregate stability under different soil tillage intensities. Appl. Soil Ecol. 179:104599. doi: 10.1016/j.apsoil.2022.104599
Salome, C., Nunan, N., Pouteau, V., Lerch, T. Z., and Chenu, C. (2010). Carbon dynamics in topsoil and in subsoil may be controlled by different regulatory mechanisms. Glob. Change Biol. 16, 416–426.
Sanderman, J., Baldock, J. A., and Amundson, R. (2008). Dissolved organic carbon chemistry and dynamics in contrasting forest and grassland soils. Biogeochemistry 89, 181–198. doi: 10.1007/s10533-008-9211-x
Sartori, F., Lal, R., Ebinger, M. H., and Eaton, J. A. (2007). Changes in soil carbon and nutrient pools along a chronosequence of poplar plantations in the Columbia Plateau, Oregon, USA. Agric. Ecosyst. Environ. 122, 325–339. doi: 10.1016/j.agee.2007.01.026
Sayer, E. J., Heard, M. S., Grant, H. K., Marthews, T. R., and Tanner, E. V. J. (2011). Soil carbon release enhanced by increased tropical forest litterfall. Nat. Clim. Change 1, 304–307. doi: 10.1038/nclimate1190
Schimel, J. P., and Schaeffer, S. M. (2012). Microbial control over carbon cycling in soil. Front. Microbiol. 3:348. doi: 10.3389/fmicb.2012.00348
Schrumpf, M., Kaiser, K., Guggenberger, G., Persson, T., Kögel-Knabner, I., and Schulze, E. D. (2013). Storage and stability of organic carbon in soils as related to depth, occlusion within aggregates, and attachment to minerals. Biogeosciences 10, 1675–1691. doi: 10.5194/bg-10-1675-2013
Simpson, R. T., Frey, S. D., Six, J., and Thiet, R. K. (2004). Preferential accumulation of microbial carbon in aggregate structures of notillage soils. Soil Sci. Soc. Am. J. 68, 1249–1255. doi: 10.2136/sssaj2004.1249
Six, J., Frey, S. D., Thiet, R. K., and Batten, K. M. (2006). Bacterial and fungal contributions to carbon sequestration in agroecosystems. Soil Sci. Soc. Am. J. 70, 555–569. doi: 10.2136/sssaj2004.0347
Tun, T. N., Guo, J., Fang, S., and Tian, Y. (2018). Planting spacing affects canopy structure, biomass production and stem roundness in poplar plantations. Scand. J. For. Res. 33, 464–474. doi: 10.1080/02827581.2018.1457711
Vitousek, P. M., Porder, S., Houlton, B. Z., and Chadwick, O. A. (2010). Terrestrial phosphorus limitation: Mechanisms, implications, and nitrogen–phosphorus interactions. Ecol. Appl. 20, 5–15. doi: 10.1890/08-0127.1
Wallander, H., Nilsson, L. O., Hagerberg, D., and Rosengren, U. (2003). Direct estimates of C:N ratios of ectomycorrhizal mycelia collected from Norway spruce forest soils. Soil Biol. Biochem. 35, 997–999. doi: 10.1016/S0038-0717(03)00121-4
Wang, C., Qu, L., Yang, L., Liu, D., Morrissey, E., Miao, R., et al. (2021). Large-scale importance of microbial carbon use efficiency and necromass to soil organic carbon. Glob. Change Biol. 27, 2039–2048. doi: 10.1111/gcb.15550
Wang, J. B., Chen, Z. H., Chen, L. J., Zhu, A. N., and Wu, Z. J. (2010). Surface soil phosphorus and phosphatase activities affected by tillage and crop residue input amounts. Plant Soil Environ. 57, 251–257. doi: 10.17221/437/2010-PSE
Wang, S., Huang, M., Shao, X., Mickler, R. A., Li, K., and Ji, J. (2004). Vertical distribution of soil organic carbon in China. Environ. Manag. 33, S200–S209. doi: 10.1007/s00267-003-9130-5
Wang, S., Lu, W., and Zhang, F. (2022). Vertical distribution and controlling factors of soil inorganic carbon in poplar plantations of coastal eastern China. Forests 13:81.
Wang, Y., Shao, M. A., Zhang, C., Liu, Z., Zou, J., and Xiao, J. (2015). Soil organic carbon in deep profiles under Chinese continental monsoon climate and its relations with land uses. Ecol. Eng. 82, 361–367. doi: 10.1016/j.ecoleng.2015.05.004
Watanabe, F. S., and Olsen, S. R. (1965). Test of an ascorbic acid method for determining phosphorus in water and NaHCO3 Extracts from Soil. Soil Sci. Soc. Am. J. 29, 677–678. doi: 10.2136/sssaj1965.03615995002900060025x
Wesemael, B. V., Caroline Roelandt, S. L., and Orshoven, J. V. (2005). Modelling the evolution of regional carbon stocks in Belgian cropland soils. Can. J. Soil Sci. 85, 511–521. doi: 10.4141/S04-085
Wu, L., Li, L., Yao, Y., Qin, F., Guo, Y., Gao, Y., et al. (2017). Spatial distribution of soil organic carbon and its influencing factors at different soil depths in a semiarid region of China. Environ. Earth Sci. 76:654. doi: 10.1007/s12665-017-6982-1
Wynn, J. G., Bird, M. I, Vellen, L., Grand-Clement, E., Carter, J., and Berry, S. L. (2006). Continental-scale measurement of the soil organic carbon pool with climatic, edaphic, and biotic controls. Glob. Biogeochem. Cycles 20:GB1007. doi: 10.1029/2005gb002576
Xia, S., Song, Z., Van Zwieten, L., Guo, L., Yu, C., Wang, W., et al. (2022). Storage, patterns and influencing factors for soil organic carbon in coastal Wetlands of China. Glob. Change Biol. 28, 6065–6085. doi: 10.1111/gcb.16325
Xu, X., Li, D., Cheng, X., Ruan, H., and Luo, Y. (2016). Carbon: Nitrogen stoichiometry following afforestation: A global synthesis. Sci. Rep. 6:19117. doi: 10.1038/srep19117
Yang, B., Zhang, W., Xu, H., Wang, S., Xu, X., Fan, H., et al. (2017). Effects of soil fauna on leaf litter decomposition under different land uses in eastern coast of China. J. For. Res. 29, 973–982. doi: 10.1007/s11676-017-0521-5
Yang, Y. H., Fang, J. Y., Guo, D. L., Ji, C. J., and Ma, W. H. (2010). Vertical patterns of soil carbon, nitrogen and carbon: Nitrogen stoichiometry in Tibetan grasslands. Biogeosci. Discuss. 7, 1–24. doi: 10.5194/bgd-7-1-2010
Yang, Y., and Luo, Y. (2011). Carbon: Nitrogen stoichiometry in forest ecosystems during stand development. Glob. Ecol. Biogeogr. 20, 354–361. doi: 10.1111/j.1466-8238.2010.00602.x
Yang, Y., Mohammat, A., Feng, J., Zhou, R., and Fang, J. (2007). Storage, patterns and environmental controls of soil organic carbon in China. Biogeochemistry 84, 131–141. doi: 10.1007/s10533-007-9109-z
Yuan, Y., Li, Y., Mou, Z., Kuang, L., Wu, W., Zhang, J., et al. (2021). Phosphorus addition decreases microbial residual contribution to soil organic carbon pool in a tropical coastal forest. Glob. Change Biol. 27, 454–466. doi: 10.1111/gcb.15407
Zeng, X.-M., Feng, J., Yu, D.-L., Wen, S.-H., Zhang, Q., Huang, Q., et al. (2022). Local temperature increases reduce soil microbial residues and carbon stocks. Glob. Change Biol. 28, 6433–6445. doi: 10.1111/gcb.16347
Zhang, W., Cui, Y., Lu, X., Bai, E., He, H., Xie, H., et al. (2016). High nitrogen deposition decreases the contribution of fungal residues to soil carbon pools in a tropical forest ecosystem. Soil Biol. Biochem. 97, 211–214. doi: 10.1016/j.soilbio.2016.03.019
Zhang, G., Zhang, P., Peng, S., Chen, Y., and Cao, Y. (2017). The coupling of leaf, litter, and soil nutrients in warm temperate forests in northwestern China. Sci. Rep. 7:11754. doi: 10.1038/s41598-017-12199-5
Zhang, S., Deng, Q., Wang, Y.-P., Chen, J., Yu, M., Fang, X., et al. (2021). Linkage of microbial living communities and residues to soil organic carbon accumulation along a forest restoration gradient in southern China. For. Ecosyst. 8:57. doi: 10.1186/s40663-021-00334-8
Zhao, F., Zhang, L., Sun, J., Ren, C., Han, X., Yang, G., et al. (2017). Effect of Soil C, N and P stoichiometry on soil organic C fractions after afforestation. Pedosphere 27, 705–713. doi: 10.1016/s1002-0160(17)60479-x
Keywords: soil organic carbon, poplar plantation, surface and deep soil layers, isometric scaling, soil microbial community, fungi:bacteria ratio
Citation: Liu B, Xu X, Xu C, Ju C, Guo L, Li N, Zhu Z, Li Q, Shen C and Cao G (2023) Soil organic carbon regulation from chemistry in top- but microbial community in subsoil in eastern coastal China poplar plantations. Front. For. Glob. Change 6:1154934. doi: 10.3389/ffgc.2023.1154934
Received: 31 January 2023; Accepted: 03 March 2023;
Published: 21 March 2023.
Edited by:
Jianping Wu, Yunnan University, ChinaReviewed by:
Zhenghu Zhou, Northeast Forestry University, ChinaRong Mao, Jiangxi Agricultural University, China
Copyright © 2023 Liu, Xu, Xu, Ju, Guo, Li, Zhu, Li, Shen and Cao. This is an open-access article distributed under the terms of the Creative Commons Attribution License (CC BY). The use, distribution or reproduction in other forums is permitted, provided the original author(s) and the copyright owner(s) are credited and that the original publication in this journal is cited, in accordance with accepted academic practice. No use, distribution or reproduction is permitted which does not comply with these terms.
*Correspondence: Xia Xu, eHV4aWEuMTk4MkBvdXRsb29rLmNvbQ==