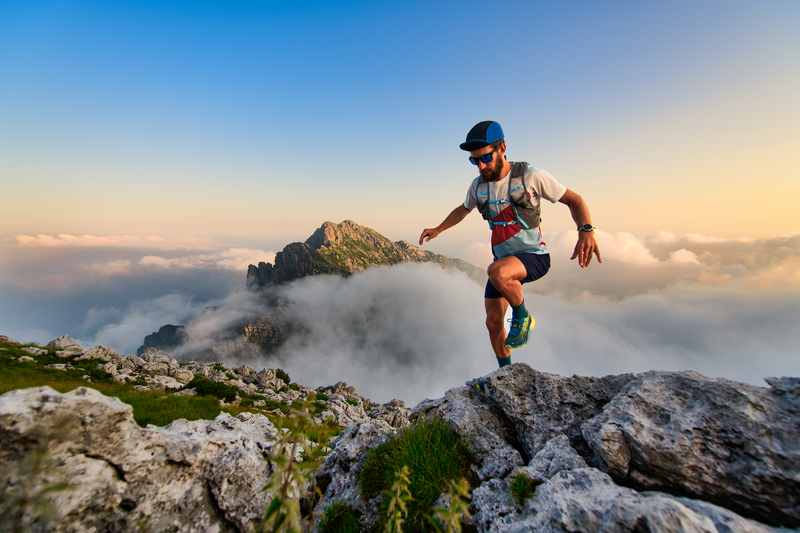
95% of researchers rate our articles as excellent or good
Learn more about the work of our research integrity team to safeguard the quality of each article we publish.
Find out more
ORIGINAL RESEARCH article
Front. For. Glob. Change , 11 April 2023
Sec. Temperate and Boreal Forests
Volume 6 - 2023 | https://doi.org/10.3389/ffgc.2023.1146758
Boreal lichen woodlands (LWs) are stable low tree-density zones of the Canadian boreal forest whose afforestation has been proposed as a way to create new C sinks and thus mitigate climate change. Planting operations in these remote areas are however costly and time-consuming, and may not be necessary when soil scarification is followed by dense natural regeneration. In the present study, we assessed the natural regeneration potential and dynamics in six boreal LWs of Québec, Canada, 11 years after soil scarification. The number, size (height and stem diameter) and age of seedlings were measured in 2-4 sampling plots per site (18 plots in total). Our data show that scarification operations produced on average 1,400 m2 ha–1 of exposed mineral soil (scarification intensity of 14%) with, however, a large within-site variability. The natural regeneration was mainly composed of black spruce seedlings (> 95%), averaged ∼12,000 seedlings ha–1 across the six sites and significantly varied among sites, mostly due to the variation in scarification intensity. Seedling density averaged ∼9 seedlings m–2 of exposed mineral soil and increased with seed tree mean diameter at breast height (DBH) (R2 = 0.51; P < 0.05) but not with the density of seed trees, revealing the importance of old and large seed trees in natural regeneration success. Together, scarification intensity and the DBH of remaining seed trees explained ∼60% of the variation in natural regeneration density across the 18 sampled plots. The rate of establishment of seedlings was generally high – with on average 60% of the carrying capacity of the substrate being reached within three years following scarification – and increased with seed tree mean DBH (R2 = 0.77; P < 0.05). However, the growth rate of seedlings was very low. Eleven years after scarification, 60% of the seedlings were < 15 cm and the height of 10-yr-old seedlings averaged 27.5 cm. Thus, even though seedling establishment was successful, the biomass accumulated by the natural regeneration was negligible in the span of a decade. Therefore, the implementation of afforestation following scarification appears to be necessary to create significant C sinks in the midterm.
Boreal lichen woodlands (LWs) are alternative and stable states of the North American boreal forest resulting from natural regeneration failures following successive natural disturbances, especially defoliating insects and wildfires (Payette et al., 2000; Jasinski and Payette, 2005; Gonzalez et al., 2013; Payette and Delwaide, 2018). The subsequent encroachment of ground-dwelling lichens of the genus Cladonia and ericaceous shrubs represent a barrier (physical and chemical) for tree seedlings’ establishment (Houle and Filion, 2003; Thiffault et al., 2004; Jasinski and Payette, 2005; Hébert et al., 2010; Kyrö et al., 2022) and causes a decrease in site fertility and tree productivity through a multitude of direct and indirect mechanisms (Sedia and Ehrenfeld, 2005; Girard et al., 2009; Hébert et al., 2010; Ouimet et al., 2018). As a consequence, LWs have low C stocks both in the vegetation (Hébert et al., 2010; Dufour et al., 2016) and in the soil (Ouimet et al., 2018) relative to previous closed-crown forests. As this ecosystem covers about 2 million km2 in Canada (Johnson and Miyanishi, 1999; Girard et al., 2008), the afforestation of LWs has been viewed as a way to create new economic opportunities as well as new C sinks for climate change mitigation (Gaboury et al., 2009; Boucher et al., 2012; Dufour et al., 2016).
The densification of LWs, however, be it natural through seeding or artificial through afforestation, requires prior soil mechanical preparation to ensure the establishment and the growth of seedlings (see Sikström et al., 2020 for a description of the most common mechanical soil preparation methods). Soil scarification is a widely used soil preparation technique that creates furrows or patches of exposed mineral soil, which has been shown to be a suitable substrate for several conifer species, including black spruce (Viereck and Johnston, 1990; Prévost, 1992; Houle and Filion, 2003; Wang and Kemball, 2005; Kemball et al., 2006; Prévost and Dumais, 2018), by increasing mineralization rate, yielding favorable humidity and temperature conditions and reducing competing vegetation (Orlander, 1987; Prévost, 1992, 1997; Karlsson et al., 2002; Hébert et al., 2006; Prévost and Dumais, 2018; Sikström et al., 2020). Soil scarification not only improves planting success after cuttings (Sikström et al., 2020) but also promotes the germination and the establishment of seedlings derived from surrounding seed trees (Prévost, 1992; Karlsson et al., 2002; Madec et al., 2012; Hébert et al., 2013; Prévost and Dumais, 2018; Laine et al., 2020; Rosenvald et al., 2020; Kyrö et al., 2022). Therefore, planting operations, which are costly and time-consuming—especially in remote areas—may not be economically justified when scarification is followed by dense natural seeding and establishment (Cyr et al., 2022). Nevertheless, several factors influence the success of natural regeneration, including seed production, dispersal and germination, as well as seedling establishment success (Gärtner et al., 2011). The remaining basal area following a fire has been shown to be a significant predictor of black spruce’s seed rain in Alaska’s boreal forest (Johnstone et al., 2009). High density of remaining seed trees in northern Finland has also been shown to increase natural Scots pine seedling density in 1 m2-scarified plots in the first decade following soil preparation (Kyrö et al., 2022). However, natural regeneration is not always limited by seed production. In this case, natural regeneration may rather depend on seedbed quality and availability (Fleming and Mossa, 1994; Peters et al., 2005; Wang and Kemball, 2005; Kemball et al., 2006; Madec et al., 2012; Kokkonen et al., 2018), or seedlings’ vigor, which is known to increase with seed size in black spruce (Skeates and Haavisto, 1995). For instance, Madec et al. (2012) have shown that the density and the distribution of seed trees were not a limiting factor for seedling establishment in scarified LWs, which was rather mostly controlled by exposed mineral soil surface area (thereafter ‘scarification intensity’ in m2 ha–1 or %).
The natural regeneration dynamics following wildfires (Filion and Morin, 1996; Wang and Kemball, 2005; Kemball et al., 2006; Johnstone et al., 2009; Brown and Johnstone, 2012) or logging (Béland et al., 2000; Downey et al., 2018; Kokkonen et al., 2018; Prévost and Dumais, 2018; Laine et al., 2020; Rosenvald et al., 2020; Sikström et al., 2020; Kyrö et al., 2022) have been well documented in boreal forests but available data are much scarcer for scarified LWs, characterized by low seed tree density, low fertility and the presence of a competing cryptogamic vegetation (lichens and bryophytes). A few studies have shown the benefits of soil scarification in LWs for both natural seeding (Madec et al., 2012) and the growth of planted seedlings (Hébert et al., 2006, 2013), but as afforestation experiments in LWs started only a few decades ago, our long-term knowledge of natural regeneration dynamics in this habitat is limited. In addition, the growth of black spruce seedlings has been shown to be slow during the first five years following soil scarification in boreal forests and more research is needed to determine long-term survival and growth rates of seeded black spruce (Fleming and Mossa, 1994; Prévost and Dumais, 2018). It is not clear whether their growth and survival is affected in the long-term by seedlings density and the colonization of exposed mineral soil areas by lichen, moss or ericaceous species. A comparison of biomass production by naturally regenerated vs. planted seedlings is also needed to decide whether planting operations are necessary and to account for the C sequestration potential that natural seeding-derived seedlings represent in plantations.
The main goal of this study was to assess the potential and the dynamics of natural regeneration in boreal LWs of Québec, Canada, following soil scarification as a unique intervention. We addressed the following specific research questions: (1) Does the high seedling establishment reported by Madec et al. (2012) within five years after soil scarification persist in time?; (2) To what extent does substrate and seed availability limit natural regeneration in this habitat?; (3) Does seedling establishment rate decrease with time since scarification?; and (4) Does natural regeneration a decade after scarification represent a significant biomass stock? For this purpose, we assessed the density, the species, the size and the age structure of the established seedlings in six LWs that had been subjected to scarification 11 years earlier. We also assessed the relationship between the dynamics of seedling establishment and a set of variables measured in a total of 18 plots spread out across the six sites to identify the main drivers of natural regeneration success in scarified LWs. We hypothesized that seedling establishment rate and density would increase with the density of remaining seed trees on the study plots. Another goal was to report on the scarification intensity (i.e. the surface area of exposed mineral soil produced by the scarification) and its variation among sites as this type of data is rarely reported in the literature (Sikström et al., 2020). In agreement with Madec et al. (2012), we hypothesized that this factor would be a major driver of natural regeneration in the study plots. These results will provide precious information on the dynamics of natural regeneration and its rate of biomass and carbon accumulation in boreal LWs, and will thus help forest managers to make decisions regarding the need for implementing afforestation in this habitat.
The study sites are located in the black spruce-feather moss boreal forest domain (Saucier et al., 2009), about 75 km north-east of Chibougamau and about 200 km north-west of the Lac Saint-Jean, Québec (50°13′15″N; 73°23′27″W; Figure 1). The climate is considered as sub-arctic or continental, with typically cold and long winters and warm summers. Mean annual air temperature was −1.12°C and mean annual precipitation 923 mm (1970-1980 period) at the closest weather station located about 40 km away from the study area (Mistassini post; 50°25′00,000″ N; 73°53′00,000″ W). January and February temperatures averaged −20°C and July temperatures +16°C. Forested soils in the region are typical podzols which have developed from Precambrian charnockitic gneiss covered by thick undifferentiated till and are generally well drained. Successive wildfires (the latter of which happened in 1967) have turned the original forests into lichen woodlands (LWs) with a typical thin organic soil layer (typically < 15 cm) and short vegetation mainly composed of ericaceous species including Rhododendron groenlandicum L., Kalmia angustifolia L. and Vaccinium angustifolium L., and various terricolous lichens of the Cladonia genus (Payette and Delwaide, 2018). Pictures of an unscarified and a scarified LWs are provided in the Supplementary Figure 1. The surface area of the study LWs ranged from 2.1 to 4.7 ha (Table 1), and were surrounded by the original black spruce forest (Supplementary Figure 2). The level of closure of the LWs (the density and basal area of remaining mature trees) varied among sites, but there was also a strong within-site variation in both seed tree density, basal area and diameter at breast height (DBH) (Table 1 and Supplementary Figure 3). The experimental sites were scarified in the summer of 2010 with no further intervention since. Operators were warned not to affect remaining seed trees as much as possible.
Figure 1. Location of the six study sites including a total of 18 sampling plots (green dots) in Québec, Canada (50°13′15″N; 73°23’27″W).
The study was conducted in late June 2021 in six boreal LWs that had been subjected to conventional soil scarification using a disk trencher in 2010. Disk scarification is the most widely used soil mechanical preparation prior to planting in Québec and has been shown to be the most effective method for afforestation of LWs (Prévost, 1992; Hébert et al., 2013). Soil scarification creates an alternance of furrows or patches of exposed mineral soil resulting from the removal of lichens, shrubs and the organic soil (thereafter referred to as exposed mineral soil) and mounds of vegetation and displaced organic matter [see Figure 1 in Sikström et al. (2020)]. The exposed mineral soil patches are the preferential seedbed for various species, including black spruce (Prévost, 1992), and the majority of seedlings have been shown to emerge from scarification patches although they represent only a small fraction of the scarified LWs (Madec et al., 2012). The seedbeds of exposed mineral soil resulting from scarification are mineral sandy soil with a marginal proportion of organic matter. The six sites were distributed along a N-S transect of about 10 km (Figure 1). Two to four circular sampling plots (Ø = 11.3 m; surface area = 100 m2) were delimited in each site, for a total of 18 plots. All variables related to natural regeneration density and growth were measured in these sampling plots.
The density, the DBH and the location (distance from the center and orientation relative to the azimuth) of remaining trees were measured within 400 m2 circular plots (Ø = 22.6 m) centered around each 100 m2-sampling plot. The density of seed trees in scarified LWs (trees ha–1) was calculated from the number of seed trees present in these 400 m2-plots. The basal area was calculated as the sum of the section area (at 1.3 m height) of all seed trees present in the 400 m2-plots.
The vast majority of seed trees present at the sites were black spruce (390 vs. 5 jack pine). The density of seed trees varied by a factor ∼2 among sites, averaging 573 ± 200 trees ha–1 (Table 1) but there was also a large within-site variation among plots (Supplementary Figure 3).
The mean DBH of seed trees ranged from 4.7 at b2 to 8.2 cm at b5, and averaged 5.9 cm across the six sites (Table 1). The basal area of seed trees was higher at b4, b5, and b6 than at b1, b2, and b3 due to higher mean DBH or/and seed tree density (Table 1).
Natural regeneration density was assessed by counting the number of seedlings within all the patches of exposed mineral soil present in each 100 m2-plot. Based on field observations and Madec et al. (2012), we considered the seedling regeneration on non-scarified areas as negligible. The number of seedlings in each plot was reported (1) per hectare of scarified LW (the number of seedlings per plot × 100) : this value is referred to as “natural regeneration (seedlings ha–1)” thereafter; and (2) per surface unit of exposed mineral soil surface area (the number of seedlings per plot / total surface area of exposed mineral soil per plot): this value is referred to as “seedling density (seedlings m–2)” thereafter. Natural regeneration was therefore the product of seedling density and scarification intensity. We measured the diameter at stump height (DSH; i.e., diameter of the main stem at 0.15 m above the ground) and the height of all seedlings taller than 0.15 m with a tree caliper and a measuring tape, respectively. In most plots, soil scarification resulted in small patches of exposed mineral soil rather than regular furrows due to the presence of seed trees or rocks. All the patches included in a sampling plot were photographed with a high-resolution digital camera at approximately 2.2 m above ground. A frame of known dimensions (30 × 30 cm) was used as a reference scale on each picture. The contours of the patches were later delineated and their surface area measured from the pictures by using the image processing and analyzing software ImageJ (Schneider et al., 2012).
To study the age structure of the natural regeneration, three ∼1 m2-patches of exposed mineral soil were selected on a NW-SE transect just outside each of the 18 sampling plots (for a total of 3 × 18 = 54 subplots). All the seedlings within the subplots were gently uprooted, put in bags and brought back to the university campus. Seedlings’ age was then inferred from the number of apical bud scars between the collar and the tip of the main stem. The bud scars at the base of each annual shoot were generally visible to the naked eyes. When it was not the case, the main stem was cut off just above the collar for tree ring counting. White chalk was gently applied to the transverse section of the stem to facilitate the visualization of tree rings. The age of the seedlings was then determined by counting the number of annual tree rings under a binocular magnifier. To assess the potential difference between both methods, the age of seedlings was determined by both methods on a subsample of 256 randomly chosen seedlings. In total, the age was determined for 860 seedlings.
An establishment rate index (I50; in years) – corresponding to the time after scarification necessary to reach 50% of the seedling density 11 years after scarification (at sampling time) – was computed for each plot. The I50 was determined graphically from a graph with time from scarification (years) on the x-axis, and the percentage of the maximum seedling density (ranging from 0 to 100%) on the y-axis. It corresponds to the x-value for a y-value of 50% (see Figure 4B). This index is a proxy of the rate at which exposed mineral soil patches were colonized by seedlings after scarification.
An analysis of variance (ANOVA) followed by a Tukey’s HSD test of multiple comparison of means was conducted to assess the difference among sites (number of sites = 6; total number of plots = 18) for the variables measured at the plot level (one value per plot), namely scarification intensity, natural regeneration density, seedling density, and the establishment index (I50). Mixed-effect models with plot as random effect were used for variables measured at the tree level, namely age, height and stem diameter, using the lmer function from the lme4 package (Bates et al., 2015) in R (R Core Team, 2015). Least-square means were then obtained for each site using the function lsmeans function from the lmerTest package (Kuznetsova et al., 2017). Pairwise differences of least-square means were then assessed using the difflsmeans function from the same package.
A Principal Component Analysis (PCA) was conducted by using the ade4 package (Dray and Dufour, 2007) to assess the relationship between plot characteristics (e.g., seed tree density and size, bare ground density) and seedlings characteristics (e.g., DSH, height, density). Linear mixed-effect models were applied on plot values (n = 18) with site as grouping variable for the random intercept to assess the relationship between (1) natural regeneration density vs. scarification intensity and seed tree density; (2) seedling density vs. seed tree density and seed tree mean DBH; and (3) seed tree mean DBH vs. mean seedling age and the establishment rate index (I50). The relationships between these variables were also assessed by fitting linear regressions to sites’ mean values (n = 6).
The scarification intensity (i.e., the surface area of exposed mineral soil resulting from scarification) averaged 1,430 ± 465 m2 ha–1 (or 14.3%) across the six sites (Table 2). The difference among sites was not statistically significant (ANOVA; F = 1.94; P = 0.16) due to a large within-site variation at most sites, especially at b4 and b5 where the coefficient of variation was 0.62 and 0.45, respectively.
Table 2. Scarification intensity, natural regeneration, seedling density, mean seedling age and seedling establishment index (I50) at the six study lichen woodlands.
A total of 2,085 seedlings were counted across the six sites. About 99% of the natural regeneration was composed of black spruce across the study sites. Eleven years after soil scarification, about 60% of the black spruce seedlings were shorter than 0.15 m and were thus not measured, and 75% were shorter than 0.2 m (Supplementary Figure 4). The tallest black spruce seedling was 0.89 m of height. Out of the 851 seedlings that were measured (seedlings with H > 0.15 m), 844 were black spruce, six were jack pine and only one was tamarack. About 50% of the measured black spruce seedlings had a DSH ≤ 0.2 cm (Supplementary Figure 4).
Natural regeneration density significantly varied among sites (ANOVA; F = 7.7; P = 0.001). It was significantly lower at b1 and b2 than at b3, b4, and b5 (Table 2). There was a strong positive linear relationship between natural regeneration density and soil scarification intensity when considering mean site values (Figure 2A), but the relationship was weaker when using linear mixed-model with site as random effect (F = 3.83; P = 0.07), reflecting the weaker relationship between both variables within each site. No relationship was found between natural regeneration and seed tree density (Figure 2B; F = 2.43; P = 0.14). Across the 18 plots, scarification intensity (m2 ha–1) and seed tree mean DBH (cm) together explained 61% of the variation in natural regeneration density (seedlings ha–1) (multiple linear regression; F = 11.9; P = 0.0008; Supplementary Table 1).
Figure 2. Top panels. Relationships between natural regeneration density (seedlings ha–1) and (A) the surface area of exposed mineral soil (m2 ha–1); and (B) seed tree density (trees ha– 1). The equation of the linear regression in panel (A) is y = 4672 + 4.8 x. Bottom panels. Relationship between seedling density (per m2 of exposed mineral soil) and (C) seed tree density (trees ha– 1); and (D) seed tree mean DBH (cm). The equation of the linear regression in panel (B) is y = 0.71 + 1.35 x.
In contrast with natural regeneration, there was no significant difference in seedling density (per m2 of exposed mineral soil) among sites (ANOVA; F = 1.18; P = 0.37). It averaged 8.9 ± 2.6 seedlings m–2 across the six sites (Table 2). There was no significant relationship between seedling density and seed tree density (F = 0.06; P = 0.81; Figure 2C). In contrast, there was a significant positive linear relationship between seedling density and seed tree mean DBH (Figure 2D) (F = 7.19; P = 0.01).
The two main components of the PCA explained 54% of the variation (Figure 3). The analysis shows (i) a close proximity of seedling density (seedling m–2 of exposed mineral soil) and mean DBH (cm) of mature trees in the LWs on the PC1; (ii) an opposition of seedlings’ size (height and DSH) and seedling density (seedling m–2 of exposed mineral soil) on the PC1; and (iii) an opposition of exposed mineral soil (m2) with the seed tree density (trees ha–1) and basal area (m2) on the PC2.
Figure 3. Projections of the variables (black arrows) and sampling plots (colored dots) on the two main axes of a principal component analysis (PCA). The two main axes explain 31 and 23% of the variation. The variables are: Exposed mineral soil (m2 ha– 1); natural regeneration density (seedlings ha– 1); seed tree DBH (cm); seedling density (seedlings m– 2 of exposed mineral soil); basal area (m2 ha– 1); seed tree density (trees ha– 1); seed tree distance (m): mean distance of mature trees from the center of the sampling plot; seedling DSH (cm); seedling height (cm). Different colours correspond to different sites (b1 to b6).
Figure 4. Left panels. (A) Age structure of the 860 seedlings analyzed the six sites. The red arrow indicates the median age value; (B) seedlings’ establishment rate during the 11 years following soil scarification. The dotted line shows the mean values across the six sites. Right panels. Relationships between seed tree mean DBH (cm) and (C) mean age of seedlings; (y = 6.1 + 0.33 x) and (D) the establishment time index [i.e., the time (years) since scarification to reach 50% of final the seedling density] (y = 7.05–0.65 x). R2 and P-values are shown for the linear regression conducted on plot values.
The method we used to determine seedlings age (by counting the number of bud scars on the main stem) was compared to tree rings counting on a subsample of 256 seedlings. Both methods yielded identical age estimation in 70% of the analyzed seedlings (i.e., Δ method = 0), whereas the bud scars method underestimated or overestimated seedlings’ age by one year in 17% and 8% of the cases, respectively (Supplementary Figure 5). In some instances, the difference between both methods was as high as –2 to + 4 years, but such differences affected only a very small proportion of seedlings population (11 out of 256 seedlings or 4% of the population) (Supplementary Figure 5). The one-year underestimation of the bud scars methods occurred in ∼20% of the 6- to 9-year-old seedlings (Supplementary Figure 5). Both methods yielded similar age estimation in 70% to 100% of seedlings of the other cohorts, although the bud scars method overestimated the age by one year of 33% of the 4-year-old seedlings.
Overall, these small and rare differences between both methods did not significantly impact the age structure of the subsample (Supplementary Figure 5). The median age was 9 years for both methods whereas the mean age was 8.84 and 8.95 years for the bud scars and the tree rings methods, respectively. As a consequence, the bud scar method was used to establish the age structure of the natural regeneration from all harvested seedlings (total of 860 seedlings).
The median seedling age varied from 7 to 10 years among sites (Supplementary Figure 6) and was 8 years across the six sites (Figure 4A). Across sites, the 7-, 8-, 9- and 10-year-old cohorts represented the same proportion (∼15%) and accounted together for > 60% of the seedling population (Figure 4A). Four-year-old or less seedlings accounted for only 6% of the population. Seedlings’ age significantly varied among sites (Supplementary Figure 6), reflecting contrasting dynamics of seedling establishment among sites.
Seedling age significantly varied among sites (F = 8.4; P = 0.001). It was the lowest at site b2 (7.0 ± 0.5) and the highest at site b4 (9.1 ± 0.4) (Table 2). The average age of seedlings increased with the average DBH of seed trees across sites (Figure 4C), although the linear mixed-model yielded an insignificant relationship (F = 2.68; P = 0.12), likely due to contrasting relationships within sites. The establishment rate index (I50; i.e., the time following scarification [in years] necessary to reach 50% of the seedling density measured 11 years after scarification) significantly varied among sites (ANOVA; F = 7.15; P = 0.002) from 2.0 years at b5 to 4.4 years at b2 (Table 2). On average, 75% of the seedling stocks were reached 5 years after scarification (Figure 4B). I50 strongly decreased with seed tree mean DBH (Figure 4D; F = 5.27; P = 0.04).
The height of 10-yr-old seedlings was not significantly different among sites (F = 0.46; P = 0.08) and averaged 27.5 ± 11.3 cm.
The soil scarification intensity averaged 14% across the study sites, which is close to the 19% reported by Madec et al. (2012) in similar LWs. The variation within sites was however large, explaining the absence of significant differences among sites despite a variation by a factor > 2 between the sites b1 and b4. This within-site variation reflected landscape heterogeneity at the site level, especially the variation in the frequency of seed trees and large rocks which make scarification operations more or less difficult. The opposition of the exposed mineral soil surface area and basal area on the PCA’s second axis (Figure 3), likely reflects the lower scarification intensity in LWs with a large number of remaining seed trees.
Theoretically, the natural regeneration density is the product of the exposed mineral soil surface area (m2 ha–1) and seedling density (seedling m–2), i.e. the number of seedlings per unit of exposed mineral soil surface area. Thus, if both terms of this equation are independent from each other, a positive relationship between natural regeneration and the surface area of exposed mineral soil (or scarification intensity) is expected. This relationship was indeed strong in our study. Natural regeneration density was significantly higher at the three sites with the highest scarification intensity (b3, b4, and b5; Table 2). Across the six sites, scarification intensity explained 66% of the variation in natural regeneration density (Figure 2A). However, when site was set as random effect in a linear mixed-effect model, the relationship between natural regeneration density and scarification intensity was not as strong (F = 3.83; P = 0.07) due to weaker or possibly negative relationships between both variables within sites. Overall, this positive relationship is in accordance with Madec et al. (2012) who found that the surface area of exposed mineral soil was the main driver of natural regeneration density. Laine et al. (2020) also found that the natural regeneration of birch seedlings in central Finland was higher in plots treated with a scarification method that exposes a larger surface area of mineral soil. Therefore, the slightly lower scarification intensity in our study (14% vs. 19%) may partly explain the observed lower natural regeneration density (12,082 seedlings ha–1) relative to the range of 15,000 to 30,000 seedlings ha–1 reported by Madec et al. (2012). In the absence of seed limitation, increasing scarification intensity would therefore likely increase natural regeneration density in a linear manner. However, the surface area of exposed mineral soil is limited by the scarification method itself and cannot increase beyond a certain level – probably close to 30% – as scarification produces three types of components in about equal proportions, namely furrows, mounds and inter-furrows (Supplementary Figure 1).
The other term of the equation, i.e. seedling density, was not related with scarification intensity (r = −0.28; P = 0.27). A decrease in seedling density with increasing scarification intensity would have revealed a seed limitation at the sites as reported by Madec et al. (2012). In contrast with our hypothesis, seedling density was also not related with seed tree density. This result contrasts with Kyrö et al. (2022) who found that Scots pine seedling density in 1 m2-scarified subplots was lower in plots containing 50 vs. 150 seed trees ha–1. A research conducted in the same region has shown that black spruce seedling density in scarified areas depended on the distance to seed tree sources and was about ∼3 times higher 10 m than 40 m away from the seed tree source (Prévost and Dumais, 2018). This suggests that the scale at which we measured seed tree features, i.e. in the vicinity of the sampling plots, was appropriate. Our data show that, in the studied LWs, seed tree density was not a relevant variable to assess seed availability, and that a qualitative aspect should also be considered. Seedling density was indeed more strongly correlated with seed trees’ DBH (Figure 2D), showing that the size and the age of seed trees in the LWs is a stronger driver of natural regeneration than their density. For instance, seed tree density was similar at b1 and b5 but basal area was about twice higher in the latter due to higher average DBH (Table 1). Therefore, the twice higher natural regeneration seedling density at b5 than at b1 may be due to the presence of a few larger and older seed trees, which is consistent with black spruce’s maximum seed production at 50–150 years of age (Viereck and Johnston, 1990). Part of the higher seedling density reported by Madec et al. (2012) likely resulted from the higher seed tree DBH at their sites (7.5-12.5 cm) relative to ours (4.7-8.2 cm). Together, scarification intensity and seed tree mean DBH explained ∼60% of natural regeneration density across the 18 plots, by increasing substrate availability and seedling density, respectively. Therefore, the ∼twice higher natural regeneration at b5 than at b1 mostly resulted from a combination of higher scarification intensity and higher seed tree size in the former than in the latter. These results suggest that forest managers should increase scarification intensity as much as possible to maximize natural regeneration density. However, it is not always doable depending on physical constraints in the field, including the presence of erratic blocks, dead and live trees, slope etc. In addition, seed availability may become limiting beyond a certain scarification intensity, especially when the number and the size of seed trees is limited. Finally, scarification operations cause soil perturbation (the displacement of the organic soil) that likely significantly reduces its C content. Increasing scarification intensity may thus increase this impact. Hence, managers must make sure that seedling density will be large enough to offset these potential additional C losses.
Our results confirm the high level of seedling establishment reported by Madec et al. (2012) in scarified LWs, and show that high seedling density is maintained beyond five years. The natural regeneration density measured in the present study is in the range of the one reported (2,000-19,000 seedlings ha–1) for Fennoscandian boreal forests following clear-cut and mechanical soil preparation (Sikström et al., 2020) and similar to conifer regeneration (9,000-12,000 seedlings ha–1) in pine shelterwoods in Estonia (Rosenvald et al., 2020). However, much higher densities of natural regeneration are also reported in shelterwoods. For instance, Scots pine natural regeneration amounted 53,000-90,000 stems ha–1 four years after soil mechanical preparation in shelterwoods with 160-200 seed trees ha–1 in southern Sweden (Béland et al., 2000), birch natural regeneration amounted ∼23,000-29,000 seedlings ha–1 in central Finland (Laine et al., 2020) and 32,000-92,000 seedlings ha–1 in northern Finland pine forests (Kyrö et al., 2022). The comparison of natural regeneration density from one study to the other is however complicated because it is influenced by various factors. As illustrated by the present study, it strongly depends on soil scarification intensity, which is not always reported in studies or measured through different methods (Sikström et al., 2020). In addition, different height thresholds are used for characterizing natural regeneration. For instance, Prévost and Dumais (2018) reported stem density for black spruce seedlings with height > 30 cm. They found a mean density of about 2,500 stems ha–1 ten years after soil disk-scarification, which is almost twice higher than what we observed (∼1,450 seedlings ha–1). However, this large difference may simply result from a difference in growth rate as a large proportion of the seedlings in the present study were smaller than 15 cm.
Other factors, including soil quality (moisture, organic matter and nutrient content) and cryptogamic vegetation cover, may also be important for black spruce’s seedling establishment and explain some of the large variability in seedling density in patches of exposed mineral soil. For instance, moss cover has been found to be a good establishment substrate for Scots pine seedlings whereas seedling mortality was up to 14 times higher on lichen beds in northern Finland’s boreal forest (Kyrö et al., 2022). More targeted research must be done in boreal LWs to clarify this point.
The size of black spruce seedlings was low, with about 60% of the population being shorter than 15 cm, 11 years after scarification. Although this result does not imply that this natural regeneration will not develop into a productive stand, it illustrates the very slow pace at which a scarified LW would turn into a closed forest in the absence of afforestation. The small average size of the natural regeneration is the consequence of two independent mechanisms, namely the individual growth rate and the establishment rate of seedlings. The individual growth rate was very low, as exemplified by the height of a 10-yr-old seedling which averaged 27.5 cm across sites. The height of 10-yr-old seedling did not significantly vary among sites. There was rather a large within-site variation in seedling height for each age-class (Supplementary Figure 8), revealing variability in both genetic potential and/or micro-site quality. Such differences (up to a factor 4 for 11-yr-old seedlings at b5) suggest an early tree social status determination (dominant vs. suppressed trees) in this specific context. On average, the height of a 7-yr-old seedling was 12.1 ± 5.3 cm and that of a 12-yr-old seedling 39.0 ± 8.0 cm across the sites, which is much lower than the height of planted seedlings of the same age reported in other studies. For instance, the height of planted 7-yr-old black spruce seedlings (i.e., five years after planting of 2-yr-old seedlings) averaged 41 cm in afforested LWs located above 50° of latitude (Marty et al., 2023). Another study has shown that the height of 12-yr-old planted black spruce seedlings (i.e., ten years after planting) averaged 66 cm and yielded a biomass of 207 g DW per seedling (Fradette et al., 2021). Although the planting density (in general 2,000–2,500 seedlings ha–1) is smaller by a factor 2 to 8 relative to the natural regeneration density reported in the present study (∼12,000 seedlings ha–1; Table 2), the growth rate of natural seedlings is so low that their biomass and C accumulation is negligible. Based on allometric equations developed by Fradette et al. (2021) and considering a mean DSH of 0.2 cm (Supplementary Figure 4), the average seedling biomass would be < 1 g DW, which would yield a biomass stock of 5–18 kg DW ha–1, whereas Fradette et al. (2021) reported biomass stocks of 400-500 kg DW ha–1 for planted seedlings after 10 years. This large difference between natural and planted seedlings may persist over time as suggested by Prévost and Dumais (2018) who found that planted black spruce seedlings had a ∼3 times greater growth than natural seedlings within the 25 years following scarification. In any case, the biomass of the planted seedlings would add up to the one of the naturally regenerated seedlings as it is unlikely that the two types of seedlings engage in a strong competition, at least in this time frame. The planted seedlings may even have a protective effect (mainly against wind) and thus increase the survival and the growth of the natural seedlings in the first years (Kokkonen et al., 2018). Therefore, afforestation seems to be necessary to accumulate enough biomass in the mid-term to compensate for soil C emissions resulting from soil scarification.
Our results show that the natural regeneration is largely monospecific (>95% black spruce). In areas with no logging purpose, implementing afforestation would make it possible to introduce other species such as jack pine (Pinus banksiana) and tamarack (Larix laricina) that may perform better in the particular biotic and abiotic conditions prevailing in LWs. Studies have for instance shown that jack pine has a higher growth rate than black spruce in the first 5 years (Marty et al., 2023) and 10 years (Fradette et al., 2021) following afforestation of LWs. Increasing the number of species may also mitigate the expected detrimental effects of climate change. It is indeed now well established that increased biodiversity improves ecosystem resilience to stress and perturbations, and sometimes their productivity, due to the emergence of complementarity mechanisms (Loreau et al., 2001; Paquette and Messier, 2011; Houle et al., 2014; Mori et al., 2021).
Along with growth rates, the establishment rate of seedlings also controls the size of the natural regeneration and potentially its future C stock. The age determination of 860 seedlings allowed us to estimate the dynamics of seedling establishment in scarified LWs. In total, only 13 seedlings (∼1.5% of the total) were 12 years old, indicating that the recruitment of seedlings the year of scarification was very low. This was likely due to the short amount of time available for seed colonization of exposed mineral patches and subsequent germination this specific year, as the scarification may have happened at the end of the summer (unavailable information). A much larger proportion of the seedling population (∼10% of the population, i.e., 88 seedlings) established the year following scarification but the recruitment was even higher the four following years. Thereafter, the recruitment of seedlings declined and was very low in the last four years, these four cohorts accounting for about 6% of the population (50 out of 256 seedlings). This decreasing rate of establishment was likely due to a saturation of the substrate and increasing competition among seedlings and with the cryptogamic vegetation. The opposition of seedlings’ size (height and DSH) and seedling density on the PC1 axis (Figure 3) may be a sign of intra-specific competition. The colonization of exposed mineral soil surfaces by the cryptogamic vegetation may also play a role in the decreasing recruitment rate of seedlings with time as well as in the variation of seedling density (pictures of scarification patches colonized by moss and lichens species are shown on Supplementary Figure 7). Terricolous lichens and mosses of the Polytrichum genus have for instance been shown to be a poor substrate for seed germination and seedling survival in Scots pine in the northern Finland boreal forest (Kyrö et al., 2022).
In the long term, this vegetation may further impede the development and growth of seedlings due to various direct and indirect effects. The encroachment of Cladonia spp. has been shown to be associated with a decrease in tree productivity and site fertility, through a multitude of competitive mechanisms (Hébert et al., 2010; Ouimet et al., 2018). Some lichen species have allelopathic effects on both mycorrhizas and tree seedlings (Brown and Mikola, 1974), which may decrease survival and growth rates of seedlings after establishment. In a field experiment conducted in spruce forest of western Québec, Canada, lichen removal increased jack pine’s fine root biomass by more than 50%, resin extractable soil potassium by 580%, and base cations by 180% (Pacé et al., 2017). Lichens also have indirect effects on tree growth, as they play a small role in nutrient cycling due to their low concentrations in major nutrients and litter production rate (Sedia and Ehrenfeld, 2005) and affect soil temperature and humidity due to their high reflectivity and low thermal conductivity (Haughian and Burton, 2018). The very low growth rate of seedlings at the study sites may therefore partly result from the presence of lichens on the substrates. Mosses, however, can have contrasting effects on seedling establishment and growth depending on species and locations. For instance, early successional mosses developing on mineral soil patches have been shown to facilitate white spruce seedling establishment at several Canadian boreal sites, whereas feather mosses had the opposite effect (Kokkonen et al., 2018). More specific research must be conducted to assess the rate of colonization by both lichen and moss species, and their specific effect on the dynamics of seedling establishment in the LWs of the study area.
Given the very low rate of establishment in the last four years, seedling density after 11 years is probably close to the maximum carrying capacity of exposed mineral soil patches. This is consistent with Prévost and Dumais (2018) who also found that black spruce density did not increase 15 years after soil scarification in the boreal forest of eastern Canada. In agreement with our hypothesis, the presence of seed trees in the vicinity of the plots was also a driver of seedling establishment rate. Their size not only increased the carrying capacity of exposed mineral soil patches (Figure 2D), but also the rate of establishment of seedlings. The time to reach 50% of the carrying capacity decreased strongly with mean seed tree DBH (Figure 4D), suggesting that the presence of large and old trees at proximity of the scarified LW not only increased seedling density but also the rate of natural regeneration. These results point towards a seed limitation at the sites, which contrasts with the conclusions of Madec et al. (2012). The presence of old and large trees would increase annual seed production and dispersal, and thus the probability of colonization of a suitable seed bed. The rate of colonization of exposed mineral soil patches may also influence their carrying capacity (i.e., long-term seedling density) as suggested by the significant negative relation between I50 and natural regeneration density (r = −0.61; P = 0.007). The gradual colonization of the soil patches by lichen and moss species following scarification may increasingly reduce substrate availability, germination success and eventually seedling density. This mechanism must however be assessed.
Our results show that natural regeneration in scarified boreal LWs is mainly controlled by the intensity of the scarification operations and the size of remaining trees, which together explained ∼60% of natural regeneration density across the sites. Natural regeneration density increased with scarification intensity by on average ∼9 seedlings m−2 of exposed mineral soil. Our data show that the average DBH of seed trees in the vicinity of the plots not only increased seedling density but also the establishment rate of seedlings, revealing a seed limitation at the sites. Our study also underlines the very slow rate of natural regeneration in scarified boreal LWs. Although the rate of establishment of the seedlings was generally high, the size and thus the biomass of the natural regeneration was very low. On average, the height of 10-yr-old seedlings averaged 27.5 cm at the study site. As soil scarification is known to strongly affect soil C stocks, it is necessary to maximize CO2 absorption by the vegetation. Given the very low growth rate of natural relative to planted seedlings reported in other studies, the present results support the implementation of afforestation following soil scarification. In any case, part of the natural regeneration will develop in the understory of the planted trees and will further increase the C sequestration potential of plantation in the short- and mid-term. As climate change mitigation is an urgent issue one cannot spare afforestation after scarification to obtain significant results before 2050 and afterwards.
The raw data supporting the conclusions of this article will be made available by the authors, without undue reservation.
CM and OF designed the study and performed field and lab work. CM analyzed the data and drafted the manuscript. CM, OF, LD, PF, RO, and CV reviewed and edited the manuscript. All authors contributed to the article and approved the submitted version.
This research was funded by Québec’s Ministère des Forêts, de la Faune et des Parcs (MFFP) and Carbone Boréal.
We thank Bastien Bestgen, Karan Singh, Laura Malage, Carl Crête, and Bastien Maurin for their assistance in the field and lab work.
The authors declare that the research was conducted in the absence of any commercial or financial relationships that could be construed as a potential conflict of interest.
All claims expressed in this article are solely those of the authors and do not necessarily represent those of their affiliated organizations, or those of the publisher, the editors and the reviewers. Any product that may be evaluated in this article, or claim that may be made by its manufacturer, is not guaranteed or endorsed by the publisher.
The Supplementary Material for this article can be found online at: https://www.frontiersin.org/articles/10.3389/ffgc.2023.1146758/full#supplementary-material
Bates, D., Mächler, M., Bolker, B. M., and Walker, S. C. (2015). Fitting linear mixed-effects models using lme4. J. Stat. Softw. 67, 1–48. doi: 10.18637/jss.v067.i01
Béland, M., Agestam, E., Ekö, P. M., Gemmel, P., and Nilsson, U. (2000). Scarification and seedfall affects natural regeneration of scots pine under two shelterwood densities and a clear-cut in southern Sweden. Scand. J. For. Res. 15, 247–255.
Boucher, J. F., Tremblay, P., Gaboury, S., and Villeneuve, C. (2012). Can boreal afforestation help offset incompressible GHG emissions from Canadian industries? Process Saf. Environ. Prot. 90, 459–466. doi: 10.1016/j.psep.2012.10.011
Brown, C. D., and Johnstone, J. F. (2012). Once burned, twice shy: Repeat fires reduce seed availability and alter substrate constraints on Picea mariana regeneration. For. Ecol. Manage. 266, 34–41. doi: 10.1016/j.foreco.2011.11.006
Brown, R., and Mikola, P. (1974). The influence of fruticose soil lichens upon the mycorrhizae and seedling growth of forest trees. Acta For. Fenn. 141, 1–23. doi: 10.14214/aff.7575
Cyr, D., Splawinski, T. B., Pascual Puigdevall, J., Valeria, O., Leduc, A., Thiffault, N., et al. (2022). Mitigating post-fire regeneration failure in boreal landscapes with reforestation and variable retention harvesting: At what cost? Can. J. For. Res. 52, 568–581. doi: 10.1139/cjfr-2021-0180
Downey, M., Valkonen, S., and Heikkinen, J. (2018). Natural tree regeneration and vegetation dynamics across harvest gaps in Norway spruce dominated forests in Southern Finland. Can. J. For. Res. 48, 524–534. doi: 10.1139/cjfr-2017-0358
Dray, S., and Dufour, A. B. (2007). The ade4 package: Implementing the duality diagram for ecologists. J. Stat. Softw. 22, 1–20.
Dufour, B., Boucher, J. F., Tremblay, P., Mailly, D., and Lord, D. (2016). Black-spruce-lichen woodlands growth and carbon drawdown potentials as revealed by mature stands. Boreal Environ. Res. 21, 71–86.
Filion, L., and Morin, H. (1996). Distribution spatiale de la régénération de l’épinette noire 8 ans après un feu en forêt boréale (Québec). Can. J. For. Res. 26, 601–610.
Fleming, R. L., and Mossa, D. S. (1994). Direct seeding of black spruce in northwestern Ontario: Seedbed relationships. For. Chron. 70, 151–158.
Fradette, O., Marty, C., Tremblay, P., Lord, D., and Boucher, J. (2021). Allometric Equations for estimating biomass and carbon stocks in afforested open woodlands with black spruce and jack pine in the eastern Canadian boreal forest. Forests 12, 1–18. doi: 10.3390/f12010059
Gaboury, S., Villeneuve, C., and Lord, D. (2009). Estimating the net carbon balance of boreal open woodland afforestation: A case-study in Quebec’s closed-crown boreal forest. For. Ecol. Manage. 257, 483–494. doi: 10.1016/j.foreco.2008.09.037
Gärtner, S. M., Lleffers, V. J., and Macdonald, S. E. (2011). Ecology and management of natural regeneration of white spruce in the boreal forest. Environ. Rev. 19, 461–478. doi: 10.1139/A11-017
Girard, F., Payette, S., and Gagnon, R. (2008). Rapid expansion of lichen woodlands within the closed-crown boreal forest zone over the last 50 years caused by stand disturbances in eastern Canada. J. Biogeogr. 35, 529–537.
Girard, F., Payette, S., and Gagnon, R. (2009). Origin of the lichen-spruce woodland in the closed-crown forest zone of eastern Canada. Glob. Ecol. Biogeogr. 18, 291–303. doi: 10.1111/j.1466-8238.2009.00449.x
Gonzalez, E., Hébert, F., Boucher, J.-F., Sirois, P., and Lord, D. (2013). Lichen-Spruce woodland early indicators of ecological resilience following silvicultural disturbances in Québec’s closed-crown forest zone. Am. J. Plant Sci. 4, 749–765. doi: 10.4236/ajps.2013.43a094
Haughian, S. R., and Burton, P. J. (2018). Microclimate differences above ground-layer vegetation in lichen-dominated pine forests of north-central British Columbia. Agric. For. Meteorol. 249, 100–106. doi: 10.1016/j.agrformet.2017.11.029
Hébert, F., Boucher, J. F., Walsh, D., Tremblay, P., Côté, D., and Lord, D. (2013). Black spruce growth and survival in boreal open woodlands 10 years following mechanical site preparation and planting. Forestry 87, 277–286. doi: 10.1093/forestry/cpt052
Hébert, F., Boucher, J.-F., Bernier, P. Y., and Lord, D. (2006). Growth response and water relations of 3-year-old planted black spruce and jack pine seedlings in site prepared lichen woodlands. For. Ecol. Manage. 223, 226–236. doi: 10.1016/j.foreco.2005.11.005
Hébert, F., Thiffault, N., Ruel, J. C., and Munson, A. D. (2010). Ericaceous shrubs affect black spruce physiology independently from inherent site fertility. For. Ecol. Manage. 260, 219–228. doi: 10.1016/j.foreco.2010.04.026
Houle, D., Moore, J. D., Ouimet, R., and Marty, C. (2014). Tree species partition N uptake by soil depth in boreal forests. Ecology 95, 1127–1133. doi: 10.1890/14-0191.1
Houle, G., and Filion, L. (2003). The effects of lichens on white spruce seedling establishment and juvenile growth in a spruce-lichen woodland of subarctic Québec. Ecoscience 10, 80–84. doi: 10.1080/11956860.2003.11682754
Jasinski, J. P., and Payette, S. (2005). The creation of alternative stable states in the southern boreal forest, Québec, Canada. Ecol. Monogr. 75, 561–583. doi: 10.1890/04-1621
Johnson, E. A., and Miyanishi, K. (1999). “Subarctic lichen woodlands,” in Savannas, barrens, and rock outcrop plant communities of north America, eds R. C. Anderson, J. Fralish, and J. M. Baskin (New York, NY: Cambridge University Press), 421–436.
Johnstone, J., Boby, L., Tissier, E., Mack, M., Verbyla, D., and Walker, X. (2009). Postfire seed rain of black spruce, a semiserotinous conifer, in forests of interior Alaska. Can. J. For. Res. 39, 1575–1588. doi: 10.1139/X09-068
Karlsson, M., Nilson, U., and Orlander, G. (2002). Natural regeneration in clear-cuts: Effects of scarification, slash removal and clear-cut age. Scand. J. For. Res. 17, 131–138.
Kemball, K. J., Wang, G. G., and Westwood, A. R. (2006). Are mineral soils exposed by severe wildfire better seedbeds for conifer regeneration? Can. J. For. Res. 36, 1943–1950. doi: 10.1139/X06-073
Kokkonen, N. A., Macdonald, S. E., Curran, I., Landhäusser, S. M., and Lieffers, V. J. (2018). Effects of substrate availability and competing vegetation on natural regeneration of white spruce on logged boreal mixedwood sites. Can. J. For. Res. 48, 324–332. doi: 10.1139/cjfr-2017-0307
Kuznetsova, A., Brockhoff, P. B., and Christensen, R. H. B. (2017). lmerTest package: Tests in linear mixed effects models. J. Stat. Softw. 82, 1–26. doi: 10.18637/jss.v082.i13
Kyrö, M. J., Hallikainen, V., Valkonen, S., Hyppönen, M., Puttonen, P., Bergsten, U., et al. (2022). Effects of overstory tree density, site preparation, and ground vegetation on natural scots pine seedling emergence and survival in northern boreal pine forests. Can. J. For. Res. 52, 860–869. doi: 10.1139/cjfr-2021-0101
Laine, T., Kankaanhuhta, V., Rantala, J., and Saksa, T. (2020). Effects of spot mounding and inverting on growth of conifers, exposed mineral soil and natural birch regeneration. Silva Fenn. 54, 1–11. doi: 10.14214/sf.10369
Loreau, M., Naeem, S., Inchausti, P., Bengtsson, J., Grime, J. P., Hector, A., et al. (2001). Biodiversity and ecosystem functioning: Current knowledge and future challenges. Science 294, 804–808. doi: 10.1126/science.1064088
Madec, C., Walsh, D., Lord, D., Tremblay, P., Boucher, J. F., and Bouchard, S. (2012). Afforestation of black spruce lichen woodlands by natural seeding. North. J. Appl. For. 29, 191–196. doi: 10.5849/njaf.11-042
Marty, C., Fradette, O., Faubert, P., Bouchard, S., and Villeneuve, C. (2023). Performance of seedlings of four coniferous species planted in two boreal lichen woodlands with contrasting soil fertility. For. Ecol. Manage. 527:120601. doi: 10.1016/j.foreco.2022.120601
Mori, A. S., Dee, L. E., Gonzalez, A., Ohashi, H., Cowles, J., Wright, A. J., et al. (2021). Biodiversity–productivity relationships are key to nature-based climate solutions. Nat. Clim. Chang. 11, 543–550. doi: 10.1038/s41558-021-01062-1
Orlander, G. (1987). “Effects of site preparation on the development of planted seedlings in northern Sweden,” in Proceedings of the IUFRO symp, Helsinky.
Ouimet, R., Boucher, J. F., Tremblay, P., and Lord, D. (2018). Comparing soil profiles of adjacent forest stands with contrasting tree densities: Lichen woodlands vs. black spruce-feathermoss stands in the continuous boreal forest. Can. J. Soil Sci. 98, 458–468. doi: 10.1139/cjss-2018-0017
Pacé, M., Fenton, N. J., Paré, D., and Bergeron, Y. (2017). Ground-layer composition affects tree fine root biomass and soil nutrient availability in jack pine and black spruce forests under extreme drainage conditions. Can. J. For. Res. 47, 433–444. doi: 10.1139/cjfr-2016-0352
Paquette, A., and Messier, C. (2011). The effect of biodiversity on tree productivity: From temperate to boreal forests. Glob. Ecol. Biogeogr. 20, 170–180. doi: 10.1111/j.1466-8238.2010.00592.x
Payette, S., and Delwaide, A. (2018). Tamm review: The north-American lichen woodland. For. Ecol. Manage. 417, 167–183. doi: 10.1016/j.foreco.2018.02.043
Payette, S., Bhiry, N., Delwaide, N., and Simard, M. (2000). Origin of the lichen woodland at its southern range limit in eastern Canada: The catastrophic impact of insect defoliators and fires on the spruce-moss forest. Can. J. For. Res. Can. Rech. For. 30, 288–305.
Peters, V. S., Macdonald, S. E., and Dale, M. R. T. (2005). The interaction between masting and fire is key to white spruce regeneration. Ecology 86, 1744–1750. doi: 10.1890/03-0656
Prévost, M. (1992). Effets du scarifiage sur les propriétés du sol, la croissance des semis et la compétition : Revue des connaissances actuelles et perspectives de recherches au Québec. Ann. Sci. For. 49, 277–296.
Prévost, M. (1997). Effects of scarification on seedbed coverage and natural regeneration after a group seed-tree cutting in a black spruce (Picea mariana) stand. For. Ecol. Manage. 94, 219–231.
Prévost, M., and Dumais, D. (2018). Long-term growth response of black spruce advance regeneration (layers), natural seedlings and planted seedlings to scarification: 25th year update. Scand. J. For. Res. 33, 583–593. doi: 10.1080/02827581.2018.1430250
R Core Team (2015). R: A language and environment for statistical computing. Vienna: R Foundation for Statistical Computing.
Rosenvald, R., Rosenvald, K., Kaart, T., and Soolmann, E. (2020). Effects of stand parameters on conifer regeneration success in pine shelterwood stands in Estonia. Eur. J. For. Res. 139, 29–40. doi: 10.1007/s10342-019-01255-6
Saucier, J.-P., Robitaille, A., and Grondin, P. (2009). Cadre bioclimatique du Québec. Man. For. 2, 186–205.
Schneider, C. A., Rasband, W. S., and Eliceiri, K. W. (2012). NIH Image to ImageJ: 25 years of image analysis. Nat. Methods 9, 671–675. doi: 10.1038/nmeth.2089
Sedia, E. G., and Ehrenfeld, J. G. (2005). Differential effects of lichens, mosses and grasses on respiration and nitrogen mineralization in soils of the New Jersey Pinelands. Oecologia 144, 137–147. doi: 10.1007/s00442-005-0037-0
Sikström, U., Hjelm, K., Hanssen, K. H., Saksa, T., and Wallertz, K. (2020). Influence of mechanical site preparation on regeneration success of planted conifers in clearcuts in fennoscandia—a review. Silva Fenn. 54. doi: 10.14214/sf.10172
Skeates, D. A., and Haavisto, V. F. (1995). Heavier black spruce seeds produce more vigorous seedlings. Technical note no.31. Sault Ste-Marie, ON: Canadian Forest Service.
Thiffault, N., Cyr, G., Prégent, G., Jobidon, R., and Charette, L. (2004). Régénération artificielle des pessières noires à éricacées: Effets du scarifiage, de la fertilisation et du type de plants après 10 ans. For. Chron. 80, 141–149.
Viereck, L., and Johnston, W. (1990). “Picea mariana (Mill.) B. S. P. -black spruce,” in Silvics of North America, Vol. 1, conifers, eds R. Burns and B. Honkala (Washington, DC: USDA Forest Service Agriculture Handbook), 654.
Keywords: boreal forest, black spruce, soil mechanical preparation, scarification intensity, natural sowing, seed bed, seedling establishment, natural regeneration
Citation: Marty C, Fradette O, Duchesne L, Faubert P, Ouimet R and Villeneuve C (2023) Natural regeneration potential and dynamics in boreal lichen woodlands of eastern Canada following soil scarification. Front. For. Glob. Change 6:1146758. doi: 10.3389/ffgc.2023.1146758
Received: 17 January 2023; Accepted: 15 March 2023;
Published: 11 April 2023.
Edited by:
Christof Bigler, ETH Zurich, SwitzerlandReviewed by:
Amy Wotherspoon, The University of British Columbia, CanadaCopyright © 2023 Marty, Fradette, Duchesne, Faubert, Ouimet and Villeneuve. This is an open-access article distributed under the terms of the Creative Commons Attribution License (CC BY). The use, distribution or reproduction in other forums is permitted, provided the original author(s) and the copyright owner(s) are credited and that the original publication in this journal is cited, in accordance with accepted academic practice. No use, distribution or reproduction is permitted which does not comply with these terms.
*Correspondence: Charles Marty, Y2hhcmxlcy5tYXJ0eTFAdXFhYy5jYQ==
Disclaimer: All claims expressed in this article are solely those of the authors and do not necessarily represent those of their affiliated organizations, or those of the publisher, the editors and the reviewers. Any product that may be evaluated in this article or claim that may be made by its manufacturer is not guaranteed or endorsed by the publisher.
Research integrity at Frontiers
Learn more about the work of our research integrity team to safeguard the quality of each article we publish.