- 1Department of Physical Geography and Ecosystem Science, Lund University, Lund, Sweden
- 2Department of Ecology and Environmental Science, Umeå University, Umeå, Sweden
- 3Environmental Change Institute, School of Geography and the Environment, University of Oxford, Oxford, United Kingdom
The boreal landscape stores an estimated 40% of the earth's carbon (C) found in terrestrial vegetation and soils, with a large portion collected in thick organic soil layers. These ground stores are subject to substantial removals due to the centurial return of wildfire, which has strong impacts on the soil microbial community and nutrient cycling, which in turn can control ecosystem recovery patterns and process rates, such as C turnover. Currently, predictive knowledge used in assessing fire impacts is largely focused on ecosystems that experience only superficial burning and few robust observations exist regarding the effect that smoldering combustion in deeper active soil layers has on post-fire soil activity. This study provided a highly replicated and regionally extensive survey of wildfire impact on microbial community structure (using fatty acid biomarkers) and nutrient cycling (using in situ ionic resin capsules) across broad gradients of climate, forest properties and fire conditions within 50 separate burn scars and 50 additional matched unburnt boreal forest soils. The results suggest a strong metabolic shift in burnt soils due to heat impact on their structure and a decoupling from aboveground processes, releasing ecosystem N limitation and increasing mobilization of N, P, K, and S as excess in conjunction with an altered, C-starved microbial community structure and reduced root uptake due to vegetation mortality. An additional observed climatic control over burnt soil properties has implications for altered boreal forest function in future climate and fire regimes deserving of further attention.
1. Introduction
The boreal landscape stores an estimated 40% of the 2,500 Pg carbon (C) found in the earth's terrestrial vegetation and soils (Bradshaw and Warkentin, 2015; Friedlingstein et al., 2020). The size of this storage pool is largely attributed to temperature and moisture limitations on microbial decomposition of organic matter inputs to the soil, which have been observed to be controlled by variation in climate and soil drainage (Rapalee et al., 1998; Malhi et al., 1999; Brovkin et al., 2012; Clemmensen et al., 2013, 2015; Eckdahl et al., 2022a). These soil inputs tend to be collected in thick, C-rich, organic layers on the forest floor, where most soil C and nutrient turnover occurs (Deluca and Boisvenue, 2012). Growth of boreal vegetation is typically limited by mobilization of nitrogen (N) to the rooting zone, while growth of soil microbes is often hindered by the acquisition of C for its structural and energetic needs (Hobbie and Hobbie, 2013; Högberg et al., 2017). This complementary resource limitation promotes a strong mutualism where excess C in vegetation is delivered as labile root exudates to the soil environment stimulating N mobilizing microbial activity which allows for its uptake within the rhizosphere (Chen et al., 2014; Högberg et al., 2017; Frey, 2019; Hicks et al., 2021, 2022). Feeding of labile C from vegetation to root-associated microbes establishes an aboveground-belowground feedback mechanism where N is mobilized upon demand and therefore sequestered (i.e., immobilized) rather than leached from the ecosystem, promoting a closure of the nutrient cycle and long-term resource conservation (Högberg et al., 2017; Frey, 2019). An additional plant-microbe feedback is established via inputs of plant litter to the soil surface, where processing of its more labile C fractions promotes microbial biomass incorporation of relatively limited soil N (Schimel and Bennett, 2004; Hensgens et al., 2020). This copiotrophic microbial metabolizing of plant-provided labile C is contrasted by an often competing oligotrophic C metabolism where saprotrophic microbes invest increased amounts of energy into the processing of less labile C compounds in soil organic matter (Högberg et al., 2017; Fanin et al., 2019; Frey, 2019). A high energy demand triggers decomposition of excess N containing compounds for energy extraction which can result in the mineralization of organic N and its net release into the soil solution, thereby opening the cycling between immobilized states in living and non-living material (Schimel and Bennett, 2004). If this N waste is not opportunistically sequestered by other organisms which demand it, these openings can contribute to N leaching and eventual loss from the ecosystem (Schimel and Bennett, 2004). In undisturbed ecosystems, losses via nutrient cycle openings that outpace nutrient input and altered nutrient turnover rates may together or separately contribute to ecosystem retrogression (Wardle et al., 2004; Peltzer et al., 2010). In contrast, disturbed ecosystems can often experience surges in primary production due to temporary pulses of nutrients which reduce resource limitations (Neff et al., 2005; Shenoy et al., 2013; Ludwig et al., 2018; Mekonnen et al., 2019; Johnstone et al., 2020). However, even short-term imbalances in disturbed soil metabolisms and lack of resource-preserving feedback mechanisms can result in loss of excess nutrients through openings providing for negative impacts on ecosystem function and consequential eutrophication of surrounding ecosystems (Wan et al., 2001; Charette and Prepas, 2003; Smithwick et al., 2005; Betts and Jones, 2009; Granath et al., 2021). For these reasons, quantitative knowledge of nutrient cycling in relation to balances of metabolic strategies in soils spanning disturbed and undisturbed states is crucial for understanding ecosystem resilience, especially in an era of changing climate and disturbance regimes.
The major natural disturbance in boreal forests is the centurial recurrence of wildfire. Wildfire can have substantial influence on ecosystems by combusting large portions of vegetation and surface litter thereby severing many aboveground-belowground relationships and transferring significant quantities of heat-altered residual organic material (i.e., pyrogenic C) and ash to the soil below (Kardol and Wardle, 2010; McLauchlan et al., 2020; Köster et al., 2021; Pellegrini et al., 2021; Coppola et al., 2022; Eckdahl et al., 2022a,b; Jones et al., 2022). These changes can result in metabolic shifts and microbial community restructuring due to significant alterations in pH as well as the adjusted lability of C inputs and their ratio to nutrient elements such as N (C:N) (Sun et al., 2015, 2016; Whitman et al., 2019; Köster et al., 2021; Nelson et al., 2022). Notably, wildfire heating tends to produce pulses of available C and nutrients thereby promoting copiotrophic soil metabolism and rapid vegetation regrowth (Shenoy et al., 2013; Köster et al., 2021). Although, in boreal systems, these pulses tend to last not more than one growing season before their abiotically synthesized pools of labile resources are exhausted, concentrating a more refractory portion of heat-altered C in the soil (Wan et al., 2001; Köster et al., 2021). In addition to being an altered substrate for decomposition, these pyrogenic C fractions tend to have increased specific surface area and greater count of negatively charged binding sites when compared to unburnt soil material, which serve to increase soil cation-exchange capacity and adjust nutrient mobilization rates over extended periods (Makoto et al., 2012; Makoto and Koike, 2021). Despite these major potential impacts on soil function, soils of high mineral content typically receive lower heating compared to the more combustible materials above and resist direct fire impact, keeping its morphological and mineralogical structure relatively intact (McLauchlan et al., 2020). As a result, fire research within sub-boreal latitudes has focused on developing predictive models based on aboveground and surface ecosystem structure which serve to only indirectly explain the altered soil processes below (Rabin et al., 2017; Jones et al., 2019; McLauchlan et al., 2020; Hanan et al., 2021). However, boreal forests tend to have thick, flammable organic soil layers, which enable wildfire to have immediate impacts on residual soil structure while removing large portions of accessible C and nutrient resource pools from its most active layers (Boby et al., 2010; Walker et al., 2018; Eckdahl et al., 2022a). Once initiated, smoldering combustion in organic layers can last for weeks or more under fluctuating oxygen and heat conditions and have dramatic impacts on soil pore connectivity and hydrology, as well as strongly alter C substrate quality in situ (Miyanishi and Johnson, 2002; Watts and Kobziar, 2013; Wiggins et al., 2020; Scholten et al., 2021; Nelson et al., 2022) and selectively remove elements significantly altering their ratios to one another (Santín et al., 2016; Dymov et al., 2021; Eckdahl et al., 2022a). Boreal wildfire can also have direct impacts on the microbial community by selecting for heat-resistant groups and converting those that are heat-susceptible into pools of soil necromass (Köster et al., 2021). Therefore, to be accurately applied globally, fire models and indices must account for biome-specific variation in burn dynamics which consider the depth and distribution of direct heat impact on all of an ecosystem's potentially combustible material.
Several simple metrics of microbial community structure have proven useful for assessing metabolic processes in soils and have the potential to respond to fire-induced shifts in ecosystem structure and the direct impact of heat. For example, the fungal to bacterial ratio (F:B) has been associated with soil material turnover rates, resource acquisition and usage strategies and shown to be strongly controlled by soil properties such as pH, moisture and temperature (Rousk and Bååth, 2011; Malik et al., 2016; Soares and Rousk, 2019). Although, the generality of some aspects of this relationship has been brought into question due to recent evidence of strong functional overlap between the two groups as well as a great diversity of capabilities within them (Rousk and Frey, 2015). In particular, further separation of the bacterial group has shown that high ratios of gram-positive to gram-negative (GP:GN) bacterial phospholipid-derived fatty acid (PLFA) markers are linked to oligotrophic soil environments and energy-intensive decomposition of complex C compounds while low ratios are associated with copiotrophy and processing of abundant, relatively labile C compounds (Fanin et al., 2019). Actinobacteria are often studied separately from the other gram positive bacteria (i.e., Firmicutes) (Joergensen, 2022) and are associated with tolerance to extreme conditions and processing of charred soil material (Nelson et al., 2022) thereby providing a potentially strong metric for fire disturbance impact on microbial community structure. Actinobacteria have also been observed to form filaments and exhibit resource acquisition and processing strategies similar to saprotrophic fungi (Mercer et al., 1996; Lacombe-Harvey et al., 2018; Nelson et al., 2022), whose niche they may fill if fungal biomass is reduced in early succession, as signified by shifts in the PLFA ratios of these two groups (Act:F). Variation in these 3 microbial ratios are expected to relate strongly to the net mobilization of nutrients, especially in recently burnt soils with enhanced openings in biogeochemical cycling due to fire-altered elemental ratios (which can leave nutrients in greater excess relative to C) and reduced uptake via mortality of nutrient limited vegetation (Schimel and Bennett, 2004). These openings may result in total nutrient reductions which serve to rebalance the ecosystem resource availability in response to the concurrent metabolic strategies associated with fire-disturbed soils (Figure 1). This in turn may impact the reestablishment pathways of a more diverse set of C and nutrient demands in recovering forests (Shenoy et al., 2013; Mekonnen et al., 2019; Johnstone et al., 2020). Therefore, quantitative understanding of early-post-fire metabolic trends is of crucial importance for assessing the impacts of climate change altered fire regimes across boreal forests.
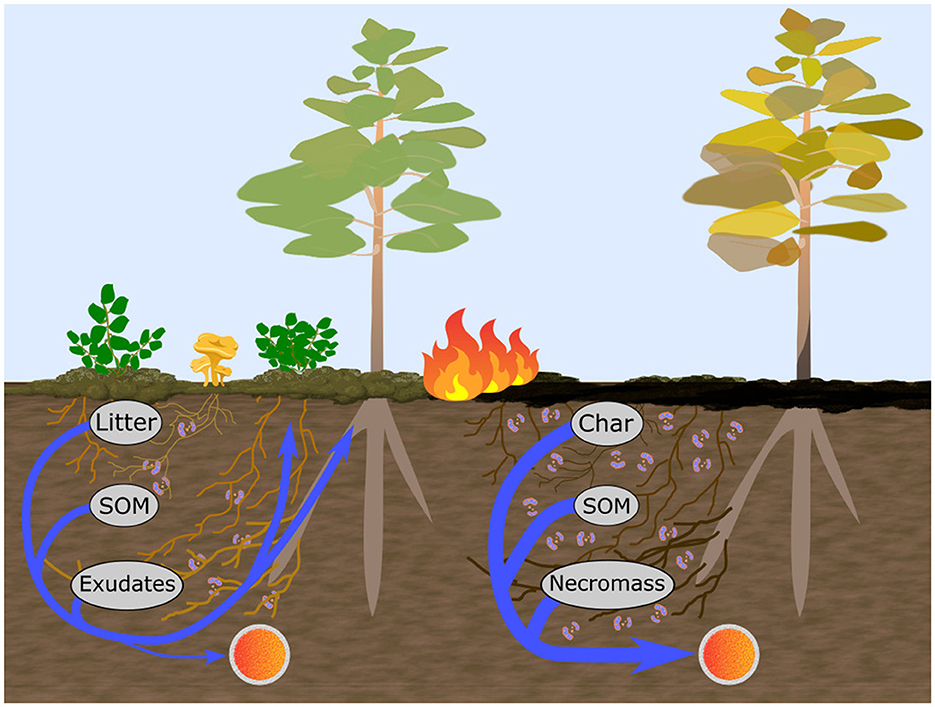
Figure 1. Conceptual diagram motivating the study hypotheses. Unburnt soils (left) receive inputs of plant-derived litter and root exudates containing labile C. Microbial C copiotrophy and plant nutrient demand promotes retention and/or immediate reuptake of relatively limited N within microbial and plant biomass during processing of organic matter, hindering its net mobilization as measured by ionic-resin capsules (orange spheres) installed at the bottom of the organic soil layer. Burning (right) typically completely removes understory vegetation and increases stand overstory mortality, removing a large portion of fresh C inputs and leaving behind charred material, residual heat-altered soil organic matter (SOM) and necromass (darkened root and mycorrizhal webs to the right). This restriction to a more refractory C pool at 1 year post-fire was expected to induce a wider spread C limitation in the soil thereby increasing dominance of oligotrophic microbial C metabolism (e.g., via increased gram-positive bacteria, right image) which enhances net mobilization of excess N and other nutrients (arrows) found in processed organic matter.
Currently, investigations of fire severity in boreal forests have been provided over narrow gradients of only one or two metrics, often within single or closely grouped burn scars, with little differentiation of the impact of individual metrics on specific aspects of microbial community structure or nutrient turnover (Vega et al., 2013; Whitman et al., 2019; Köster et al., 2021). Furthermore, knowledge regarding the influence of climate variation on fire impact is lacking, despite its recognized major role in more generally affecting ecosystem structure and functioning. Combined with fire, increasing mean annual temperature (MAT) in boreal forests is expected to enhance nutrient cycling rates contributing to substantial shifts in ecosystem structure (Mekonnen et al., 2019). Building regional to global knowledge of fire influence on soil metabolism is currently limited by the resulting difficulty in comparing research outputs produced across varied fire seasons and research methodologies. Therefore, highly replicated, methodologically consistent and simultaneously recorded surveys of metrics regarding forest structure, fire severity and climate over large gradients, which geographically span important boreal sub-regions, combined with quantitative assessment of their impacts on soil microbial community and nutrient cycling are warranted.
This study aimed to provide a detailed, quantitative survey of fire-induced shifts in soil metabolism using variation of microbial community ratios and nutrient mobilization levels across broad gradients of six readily measurable, fire-susceptible forest properties and climate in boreal Fennoscandia. The approach allowed for rapid, low cost and repeatable quantification of a broader range of regional burn conditions than might be observed through study of a single burn scar, providing the results with increased potential for future application and comparability. Specifically, it was hypothesized that:
1. Fire significantly shifted microbial community structure and increased the mobilization of nutrients. These shifts, along with non-shifted control and burnt plot values were best predicted by usage of several ecosystem properties, particularly organic layer pH, bulk density (ρ), C:N, and total C as well as plot-wide tree mortality and the amount of char added to burnt soils.
2. Climate variation, indicated by MAT and mean annual precipitation (MAP), can predict microbial and nutrient structure through additional uniquely explained variation and through shared variation with measured ecosystem properties.
3. Fire shifted the sampled boreal forest soils to an oligotrophic C metabolism by 1 year post fire, characterized by increased GP:GN and Act:F, due to soil charring and decreased soil C:N along with increased tree mortality and near-complete understory removal, which severed flow of labile C to the soil. These metabolic shifts related to mobilization of excess soil N in burnt forests with these openings in the N cycle relating to tree mortality (reduced uptake) and MAT (increased rates of biotic soil activity).
4. Soil charring increased soil cation-exchange capacity serving to buffer excess N mobilization by adsorption of NH4 but repel negative ions such as NO3 from the soil system.
5. Fire-labile nutrients such as P show similar patterns of increased mobilization as N due to C-limited decomposition of heat-induced necromass and remaining organic matter in burnt soils, with additional element-specific effects (e.g., pH).
2. Materials and methods
2.1. Plot selection
This study utilized 50 separate wildfires occurring during summer 2018 in Sweden, which were part of a previously published study linking climate variation to fire severity (Eckdahl et al., 2022a). The 50 burnt plots were selected to maximize spread across climate gradients within Sweden from a pool of 325 fires identified from the summer 2018 period that had perimeters manually mapped by the Swedish Forest Agency. Perimeters were drawn around burn scars delineated using Normalized Burn Ratio (NBR) values derived from Sentinel-2 bottom-of-atmosphere corrected bands 8 and 12. Close to the highest NBR pixel values in each separate burn scar, one 20 × 20 m2 plot was established along with an equally sized, paired control plot located in unburnt forest between 15 and 150 m (average 58 m) outside the burn scar. The control plots served as estimates of pre-fire properties for each of their corresponding burnt plots. Using geodatasets prior to the field campaign, plot pairs were best matched to resemble each other in terms of overstory biomass, basal area, tree species dominance, and stand age, with these properties otherwise allowed to vary across the climatic and geographical span of the region. Topo-edaphically evaluated soil moisture potential (TEM), which was considered a metric of soil drainage, was also used to match plots and avoid wetland areas (Naturvårdsverket, 2018). TEM was provided at 10 m resolution and given as integer values ranging from 0 to 240 (in order of increasing moisture potential) and was based on the Soil Topographic Wetness Index (Buchanan et al., 2014) in areas where soil type information was available and on the two topographic indices Depth to Water (Murphy et al., 2007) and the Topographic Wetness Index (Beven and Kirkby, 1979) where soil information was unavailable. Elevation data was provided by the Swedish Mapping, Cadastral and Land Registration Authority from a 50 m resolution digital elevation model (Lantmäteriet, 2021). Slope was calculated using the “slope” function within the ArcGIS software environment (Esri Inc., 2019). All stands were located on minimally sloping land (<15° slope), and elevation change between plot pairs was minimized. The plot pairs were spread across broad gradients of MAT and MAP, ranging from 0.43–7.77°C to 539–772 mm, respectively, during the years 1961–2017 (Figure 2). Further details and data sources are provided in Eckdahl et al. (2022a).
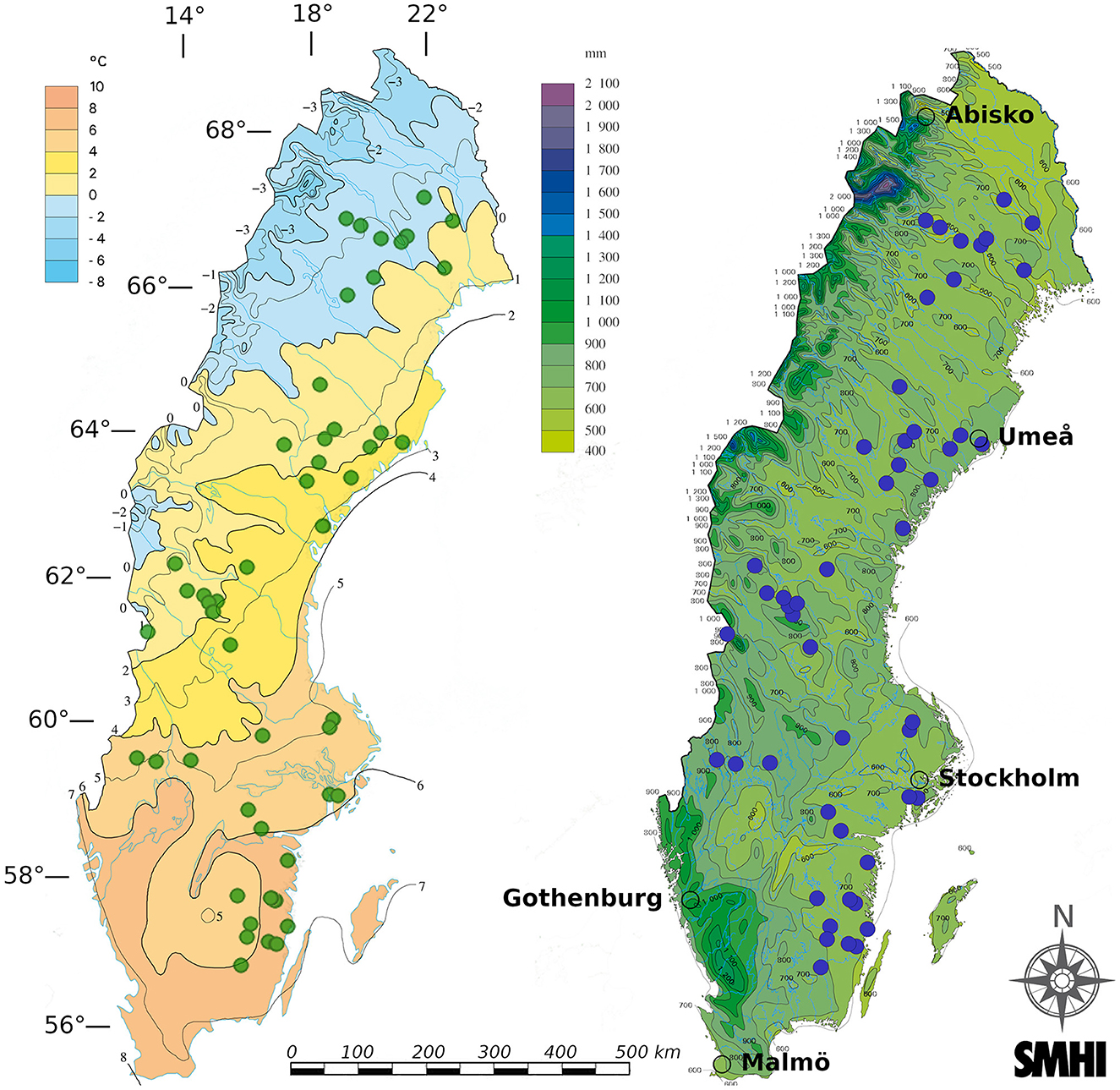
Figure 2. The 50 plot pairs spanned the approximately 57–67 latitudinal range within Sweden. They are seen as colored points on figures provided by the Swedish Meteorological and Hydrological Institute (SMHI) of mean annual temperature (left) and mean annual precipitation (right) over the last normal period 1961-1990. Major cities/research centers are marked with empty circle perimeters and WGS 84 (EPSG:4979) latitudes (horizontal bars) and longitudes (vertical bars) are given in degrees.
2.2. Vegetation mortality and fire impact survey
Once on site, overstory and understory plants were surveyed to assess the vegetation impact of fire. Plot-wide tree mortality was measured as the percentage of stems with no green needles in their respective canopies (Sidoroff et al., 2007) for all trees with their base inside the plot boundaries and diameter at 130 cm from their base >5 cm, and abbreviated as “TMort” in tables and figures. Tree species were also recorded, finding the 50 burnt plots to be largely dominated by Scots pine (Pinus sylvestris) with the percentage of Norway spruce (Picea abies) stems between 25 and 50% in five plots, between 50%-75% in three plots, and >75% in two plots. Birch stems (Betula pendula and Betula pubescens) were <25% in 44 plots and between 25 and 50% in six plots, of which only one was spruce dominant. All plots showed some visible charring of tree boles, though only three plots had >1% plot-wide canopy blackening (i.e., evidence of time-of-fire canopy burning). The observed lack of charring in the canopy led to the assumption that the majority of the direct heating impact of fire was restricted to only the lower tree boles and flaming and smoldering combustion in the soil layers, as discussed further in Eckdahl et al. (2022a). While overstory biomass density likely has a measurable effect on belowground processes, representative variables were not included in statistical analysis due to the observed low canopy damage in order to retain focus on the more immediately fire-susceptible ecosystem properties. Aboveground understory vegetation was completely consumed in most burnt plots (causing a paucity of variation for use in correlative analysis) and its pre-fire properties were therefore estimated by the paired control plots. There, understory was typically below 1 m in height and composed <2% of the C found in the soil when averaged across the 50 control plots (Eckdahl et al., 2022a).
2.3. Soil sampling and measurement
Soil sampling and measurements were performed approximately 1 year post fire over the dates August 5 to August 20 in 2019. This delay was intended to allow ecosystem properties to stabilize, in particular to avoid short-lived spikes in heat-mobilized nutrients and better isolate the effects of early post-fire biotic processes. Samples were taken from duff and moss/litter soil layers from all plots (as described below). Char layer samples were additionally collected from the pyrogenic material which formed a distinct soil layer across the surfaces of all burnt plots. The organic layer was considered the grouping of the duff, moss/litter and char layers.
The thickness of each layer was measured in millimeters at 20 separate points equally spaced across each plot diagonal (Kristensen et al., 2015). The duff layer was considered the conglomerate of the F (partially decomposed material) and H (humic material) layers in accordance with the Canadian system of soil classification (Canadian Agricultural Services Coordinating Committee, 1998). The moss/litter layer was all unburnt material on top of the duff layer, including visually identifiable detritus and living moss. In all burnt sites, a surface layer of conglomerated char formed a clear boundary on top of the residual soil below, allowing for distinct measurement. Here, char is defined as fully blackened, brittle material with apparent high heat exposure due to fire.
Duff samples were collected near the four plot corners by excavating four soil volumes of approximately 25 × 25 cm−2 area and at least the full depth of the organic layer. This volume was trimmed to discard the mineral and moss/litter layers off the bottom and top of the volumes, respectively. Right angles were then gently cut with sharp scissors, and the three dimensions were measured in millimeters (collected samples were at least 400 cm3 each and aimed to sample the entire in situ depth). Moss/litter samples were collected by cutting squares, with attention to preservation of the natural in situ volume, until filling a 553 cm3 steel container. Char layer samples were similarly collected in a 112 cm3 container. At least one sample each of moss/litter and char were acquired from each plot quadrant, though more were taken at equal spacing along a transect to fill the containers if the layer was thin. On the upper surface of the char layer were small portions of dry, unburnt material, which were likely post-fire additions of litter to the forest floor. This material was discarded from the char collection and was not included in C stock estimates due to the study design's dual purpose of estimating immediate fire-induced shifts in soil C.
All soils were podzols, except 6 which had no mineral soil across the measured transects and had organic layers directly on top of bedrock. The podzols had an organic (average 45.5% C by weight in all control plots, 11.5 cm average depth) layer clearly separated from a coarse grained low C (average 5.3% in control plots, 6.26 cm average depth) mineral layer below (Eckdahl et al., 2022a). Lacking differentiation by soil class, the variation in soil properties important to this study was expected to be best captured within the organic layer variables ρ, C:N, char, pH, and total C. Additional details on sampling and soil measurements are provided in Eckdahl et al. (2022a).
2.4. Site temperature and moisture assessment
Under a given set of macroclimatic conditions, ecosystem structure can alter temperature in the subcanopy and soil which together may have a more direct effect than regional MAT on this study's sampled microbial, nutrient and forest properties. Three recently produced maps of yearly average temperature in the subcanopy at 15 cm above the forest floor (Haesen et al., 2021) and in the soil between 0 and 5 cm depth and 5–15 cm (Lembrechts et al., 2022) had strong multicollinearity with MAT in both control and burnt plots that was considered too great for these variables to be used together in statistical analysis (r > 0.94 for all). Soil and subcanopy temperatures were not found to be significantly different between burnt and control plots pairs due to soil maps having too low resolution (1 km) and subcanopy maps (25 m resolution) not incorporating the effects of the 2018 wildfires. Organic layer temperature and moisture (using a Delta-T HH2 meter and Thetaprobe type ML2x probe) were recorded at each plot center and four of its corners during the 2019 field campaign and return visits over the period July 29 to August 11 in 2020 (plot pairs measured within at most 2 h, northernmost plot-pair unmeasured due to salvage logging). No significant difference in moisture was found between burnt and control pairs in either year, though burnt plot soil temperatures were significantly elevated relative to their paired controls in 2019 (1.34 ± 0.28°C) and 2020 (1.95 ± 0.33°C) at 95% confidence (two-tailed Z-test). These one-off measurements of moisture and temperature were not included in statistical analysis due to the highly variable nature of these soil properties over the year. Temperature logging ibuttons installed at 2 cm above the bottom of the organic layer found a temperature increase (averaged over time between the two site visits) of 0.39 ± 0.16°C in burnt plots relative to control in 23 plot pairs (two-tailed t-test at 95% confidence), though otherwise had too high a failure rate to provide adequate sample size for incorporation into analysis. Soil temperature and moisture conditions were therefore left to be approximated by variability in their larger-scale drivers including TEM (soil drainage), MAP (precipitation input), MAT (evapotranspiration) and TMort (albedo, soil solar insolation, evapotranspiration).
2.5. Initial soil sample processing and carbon, nitrogen, bulk density, and pH analysis
The duff layer was sieved to 4 mm to include most of its organic material while reliably removing visibly distinguishable roots in order to reduce their potential interference in PLFA analysis (Frostegård et al., 2011). A portion of sieved soil was freeze dried, after having been kept frozen in the field, for PLFA and pH analyses (see below). The remaining fine and coarse fractions of the duff layer, along with the moss/litter and char layers in their entireties, were dried at 40°C for 3 days and then pulverized for total C and N analysis. The pulverized samples were packed in tin capsules and combusted in a Costech ECS 4010 elemental analyzer, equipped with a 2 m packed chromatographic column for gas separation, to produce values of the C and N fractions per sample weight. After every 10 samples, standardized acetanilide (provided by the company Elemental Microanalysis, Okehampton, United Kingdom) was run to calibrate the machine within 1%. Duff layer elemental weight ratios were recalculated by the sum of C or N in its fine and coarse fractions and divided by total sample weight. For each plot, C and N weight fractions were multiplied by area-normalized mass of each soil layer, which itself was calculated by multiplying ρ (i.e., bulk density as measured by total soil layer sample weight divided by its volume) by the average measured thickness of that layer, providing C and N values in units of kg m−2. Organic layer area-normalized C and N was calculated by summing those values in each of its included sub-layers and its ρ value was calculated by summing the area-normalized mass of the sub-layers and dividing by their combined depth in meters (producing units of kg m−3). Organic layer C:N was calculated by dividing total organic layer kg C m−2 by its kg N m−2. To retain focus on readily measurable forest properties, the effects of soil charring throughout the soil profile were tested as proxied by the kg m−2 (i.e., area-normalized total mass) of the sampled pyrogenic char layer alone.
pH was measured on the <4 mm freeze dried duff samples using an HI pH-211 meter (Hanna Instruments). 1.0 g of soil was mixed with 20.0 mL of deionized water (resulting in an approximated 1:2.5 volume:volume dilution) and shaken vigorously for at least 1 min. The electrode was immersed in this solution for an additional 1 min, giving stable readings to two decimal places.
2.6. PLFA processing and data handling
Freeze dried soil samples were sent to the Swedish University of Agricultural Sciences location in Umeå, Sweden for processing of PLFA markers. The extraction and methanolysis of the soil PLFA followed the method in Bligh and Dryer (Bligh and Dyer, 1959) as modified by White et al. (1979). Resulting fatty acid methyl esters (FAME) were injected into a Trace GC Ultra gas chromatograph (Thermo Fisher Scientific, Bremen, Germany) by means of splitless injection and separated by a 30 m × 0.25 mm × 0.25 μm DB-5 column (Agilent Technologies, Santa Clara, CA). The chromatographic conditions were as follows. Injection volume: 1 μL; injection split ratio: 10; injector temperature: 280°C; carrier flow: 1 mL min−1; temperature gradient: starting temperature 80°C, hold for 1 min, increase to 155°C at 20°C min−1, hold for 0 min, increase to 300°C at 20°C min−1, hold 15 min. Samples were subsequently analyzed by an ISQ LT single quadropole mass spectrometer (Thermo Fisher Scientific, Bremen, Germany). This gave quantitative measures of 24 individual FAME compounds as nmol per g soil sample, as calibrated by an internal standard of methyl nonadeconoate (19:0) provided by Sigma-Aldrich (product number 74208). Instrument stability and retention times were verified using bacterial acid methyl ester (BAME) and Supelco 37 Component FAME mixtures both provided by Sigma-Aldrich (respective product numbers 47080-U and CRM47885).
Total measured PLFA can be suitably used as a metric of living soil microbial biomass due to its tendency not to persist as soil organic matter (Frostegård et al., 2011; Zhang et al., 2019; Joergensen, 2022). The spectroscopically measured FAME concentrations were utilized as an estimate of relative microbial biomass per mass soil (nmol g−1) across the sampled plots and abbreviated as “MicConc.” Total microbial biomass per unit area was calculated by multiplying MicConc by soil organic layer mass (producing units of nmol cm−2) and abbreviated as “MicMass.”
Fungal PLFA markers for each plot were considered as the sum of the measured methylated fatty acids 16:1ω5, 18:2ω6,9, 18:1ω9 (Willers et al., 2015; Joergensen, 2022). Actinobacteria consisted of 10Me16:0, 10Me17:0, 10Me18:0 markers (Willers et al., 2015; Joergensen, 2022). GN bacteria were the sum of cy17:0, cy19:0, 16:1ω7, 16:1ω9, 17:1ω8, and 18:1ω7 (Willers et al., 2015; Fanin et al., 2019; Joergensen, 2022). The GP bacteria were the Actinobacteria marker concentrations plus i14:0, i15:0, a15:0, i16:0, i17:0, and a17:0 (Fanin et al., 2019). General bacteria were the sum of the markers for GP and GN (Willers et al., 2015). Because the remaining markers 14:0, 15:0, 16:0, 17:0, 18:0, and 20:0 are not unique to any of these groups (Zelles, 1999; Joergensen, 2022), they were only used in the estimates of total microbial biomass (i.e., MicConc and MicMass).
The F:B ratio was constructed for each plot by dividing the fungal PLFA concentration by the general bacteria. GP:GN was calculated by dividing GP markers by GN. Act:F was formed by dividing Actinobacteria markers by fungal markers, though excluding 16:1ω5 which is representative of arbuscular mycorrhizal fungi (Joergensen, 2022), in order to better isolate the relationship between Actinobacteria and saprotrophic fungi [though these two markers do not distinguish free-living saprotrophs from ectomycorrhizae (Joergensen, 2022)].
2.7. Resin capsule installation, processing, and data handling
300 count PST-1 ion-exchange resin capsules were purchased from Unibest (Walla Walla, Washington). During the 2019 field campaign, 3 resin capsules per plot were buried equally spaced radially at about 5 m from the plot center in the organic layer consistently at approximately 2 cm above its interface with the mineral layer below. There they acted to adsorb solvated ions effectively irreversibly, providing a time-integrated view of nutrient mobilization into the soil solution. 277 viable resin capsules were retrieved during a second field campaign approximately 1 year later, with the remaining missing or damaged. The northernmost burnt plot was salvage logged between installation and retrieval, causing major disturbance, and resin capsules from its control pair were not found. Therefore, sample size for analyses involving resin capsules was reduced to n = 49 for burnt and control plot analysis each, and their differences (n = 50 for all analyses excluding resin capsule data). While in the field, the capsules were cleaned by shaking vigorously in a sealed vial with several applications of clean deionized water until applied water was clear and free from debris. Capsules were kept refrigerated each in individual plastic bags until sent back to Unibest for processing.
Lab work was managed by Unibest and involved further cleaning with deionized water and then extraction of resin capsule adsorbates individually by rinsing with 2 N HCl at a rate of 1 mL min−1 for 50 min, resulting in volumetric 50 mL solutions of leachate. Each solution was separately analyzed for concentration of chemical species and given in ppm (mg L−1 for mass of target element alone). NH4 and NO3 concentrations were determined from aliquots of the extract solutions using flow injection analysis (FIA) and UV/VIS spectroscopy with an FIAlab-2500 (FIAlab Instruments, Seattle, Washington). Concentration of 12 elements (P, K, S, Ca, Na, Fe, Mg, Cu, Zn, Mn, Al, and B) were determined using inductively coupled plasma optical emission spectroscopy (ICP-AES) using an Agilent 5110 ICP-OES (Agilent Technologies, Santa Clara, California). This technique is destructive, involving injecting of the extract solution into a high energy plasma, and provided only the concentration of the elements based on their unique spectroscopic signature, not the identity of their resin-adsorbed ionic forms. Resulting ppm values for both characterization methods were matrix matched by identical processing of separate resin capsules which were soaked in third-party-manufactured standard solutions of common soil ions. This procedure was performed before every run of 25 samples and provided an analytical repeatability within ± 0.5%. The resulting 277 sets of ppm values were averaged per plot providing 49 burnt and 49 paired control values for each chemical species. Three additional constructed variables were used in analysis. These were iN (inorganic nitrogen), the sum of the NH4 and NO3 variables, NH4:NO3, the NH4 variable divided by NO3 and iN:P, iN divided by the P variable.
2.8. Data analysis
2.8.1. Statistical distributions, confidence intervals, and percentage change
The climate and forest properties that were used as predictor variables are summarized in Table 1. In this study, “fire severity” refers to the shifts of these forest properties from control to paired burnt plots, i.e., their Δ values. Microbial community variables used in analysis included F:B, GP:GN, Act:F, MicMass and MicConc. Nutrient levels in soils were assessed via the variables NH4, NO3, P, K, S, Ca, Na, Fe, Mg, Cu, Zn, Mn, Al, B, iN, NH4:NO3, and iN:P. Three statistical distributions were formed for each variable, one for their control plot values, one for their burnt plot values and another for the values produced by subtracting control plot values individually from those in their paired burnt plot. The values of these differenced variables will be referred to as “plot-pair differences” or prefixed with the Δ symbol. MAT and MAP (given at 2 km resolution) were identical for each control-burnt plot pair, and so the same values were used within control, burnt and Δ variable analysis. Pyrogenic layers were not present in control plots, and therefore not included in control plot analysis and its Δ variable was set equal to that in burnt plots. All variable distributions (except MAT and MAP which were only analyzed as a predictor variable) were approximated as normal with all statistically significant (p < 0.05) bivariate correlations measured demonstrating linear relationships, with no obvious deviation or need for variable transformation upon individual inspection. Confidence intervals were marked with the ± sign and constructed at the 95% level using the formula
Where is the sample mean, z is always 1.96 (the critical value for a two-tailed Z-test at α = 0.05), σ is the sample standard deviation and n the sample size. Significance of differences between control and burnt plots was deemed to be when their interval did not include zero. Coefficients of variation (CV) were calculated as the standard deviation of a variable distribution divided by its mean. Percentage change of variables from burnt to control plots were calculated from the two averaged values derived from the burnt and control plot variable distributions.
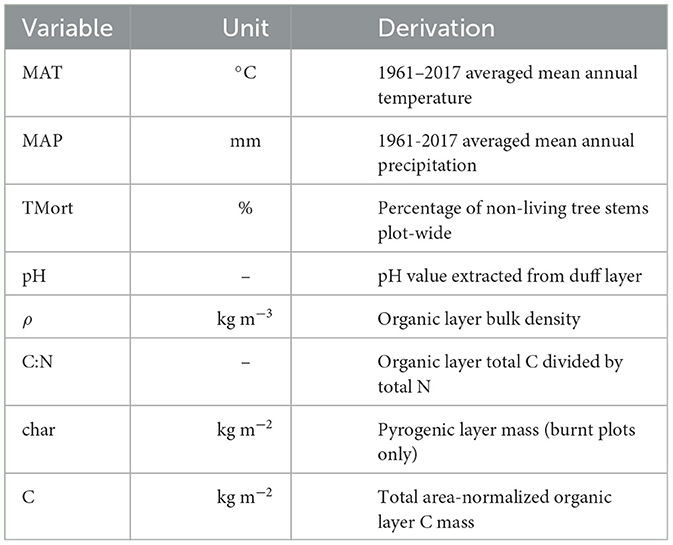
Table 1. All climate and forest property predictor variables used in analysis with units and description of their derivation.
2.8.2. Redundancy analysis
In order to quantify the ability of climate, forest properties and fire severity to predict microbial and nutrient variables, redundancy analysis (RDA) was performed for control, burnt and Δ normalized (i.e., converted to Z-scores) datasets using the rda class in the R package vegan (Oksanen et al., 2022), outputting R2 and for each model. Model selection was performed to drop explanatory variables that did not contribute to increasing through the usage of the function ordiR2step within the vegan R package. Overall model and per-axis significance was calculated using 1000 permutations in the anova function in the R vegan package and deemed significant when the produced p value was <0.05. The RDA results were displayed as triplots across the two axes of largest explanatory power using the fitted y values (so-called “lc scores”). Canonical coefficients for the explanatory variables were extracted directly from the rda object.
To estimate the proportional effects of climate and forest properties/fire severity on microbial community and nutrient mobilization, variance partitioning was executed by performing two additional RDA analyses per the three datasets, one with MAT and MAP as explanatory variables alone and another with only the remaining explanatory variables. Shared explained variance was calculated as the magnitude of the difference of the total model and the sum of the two segmented model values using the varpart function in the R package vegan.
2.8.3. Simple and multiple regression
To analyze the impact of climate, forest properties and fire severity on the individual response variables, multiple regression was used. To increase model parsimony, isolating only the most significant explanatory variables, each normalized (i.e., converted to Z-scores) explanatory variable matrix used in RDA was submitted to backwards step-wise regression by removing the explanatory variable with the highest p value until all variables and the total model had p values below 0.05 or only one explanatory variable remained. This was performed using the outputs from the OLS class in the statsmodels (Seabold and Perktold, 2010) package using the Python 3 interpreter. The OLS class used the ordinary least squares regression approach to predict a single response variable based on linear combinations of predictor variables and gave as an output R2 values for the model fit and standardized regression coefficients (β) for the explanatory variables. Bivariate correlation strength between each variable pair was extracted as the Pearson correlation coefficient (r) from the scipy.linregress method from the SciPy package (Virtanen et al., 2020) in Python 3.
2.8.4. Canonical correlation analysis
Canonical correlation analysis was performed to produce biplots of the multivariate relationships between nutrient and microbial datasets using the CCorA class from vegan. Significance was extracted from the model object using a permutational (n = 1,000) F-test of Pillai's trace. Canonical correlation coefficients were extracted from the model object for the first axis of each dataset and values were derived from the object's internal calls to the vegan rda class.
3. Results
3.1. Fire-induced differences of all variables
3.1.1. Forest properties and fire severity
All forest properties had significant shifts from control to burnt plots (Table 2). Burnt plots had a reduction of organic layer C compared to controls of 0.75 ± 0.63 kg C m2. This was accompanied by the formation of 2.06 ± 0.46 kg m2 of char on burnt plots. ρ increased by an average of 45.0% in burnt plots relative to control while pH shifted up by 0.27 ± 0.14. Organic layer C:N ratio dropped from an average of 47.68 (CV = 0.22) in control plots to 38.22 (CV = 0.25) in burnt plots, with an average plot-pair difference of -9.46 ± 2.97. Finally, tree mortality increased due to fire, jumping from an average of 4.21% (CV = 1.40) in control plots to 44.96% (CV = 0.70) in burnt plots. Bivariate covariation patterns of these variables are given in Supplementary Table S1 and visualized in Figure 3.
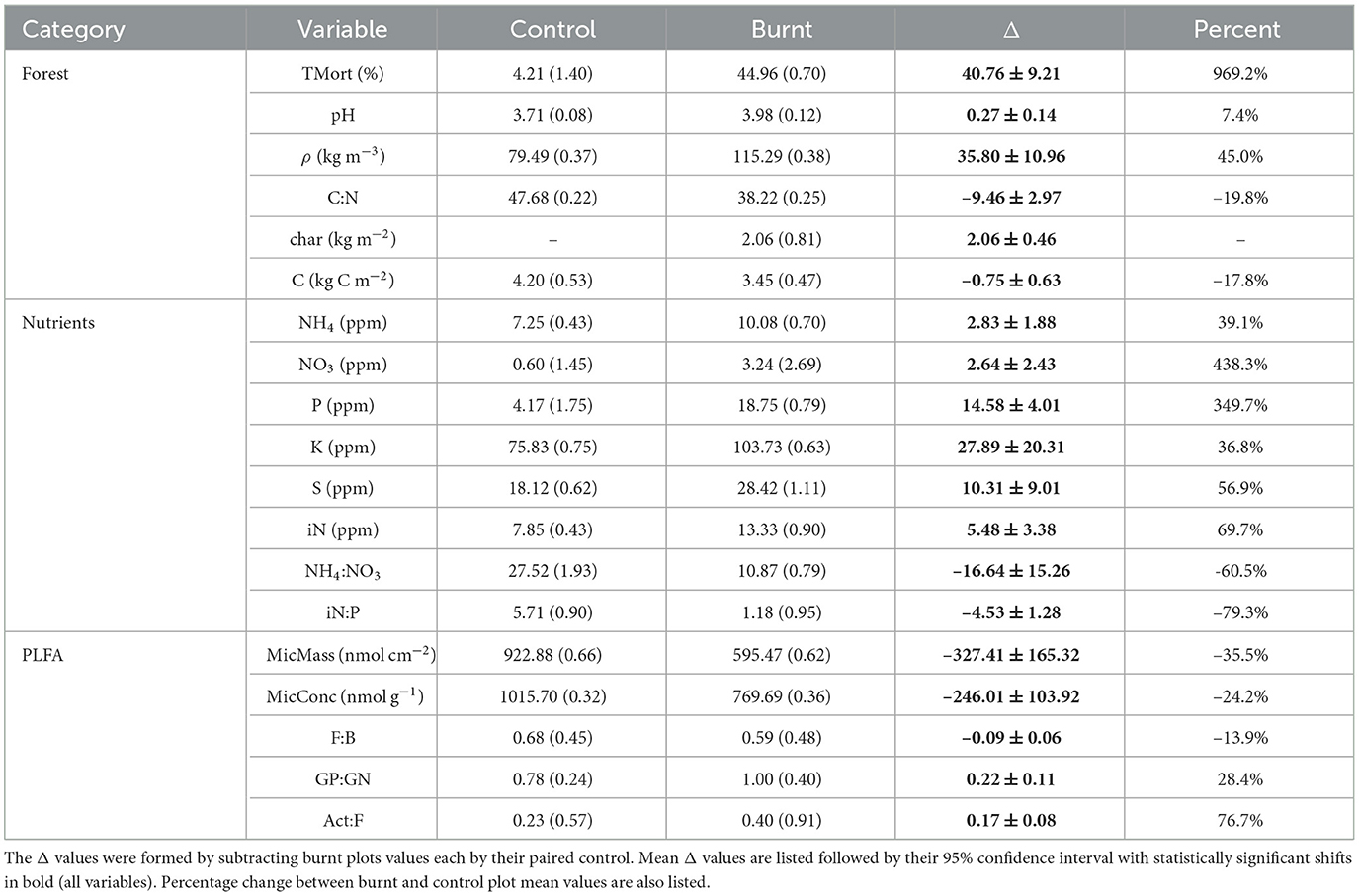
Table 2. Mean values for control and burnt plot sampled variables are listed followed by their coefficient of variation in parentheses.
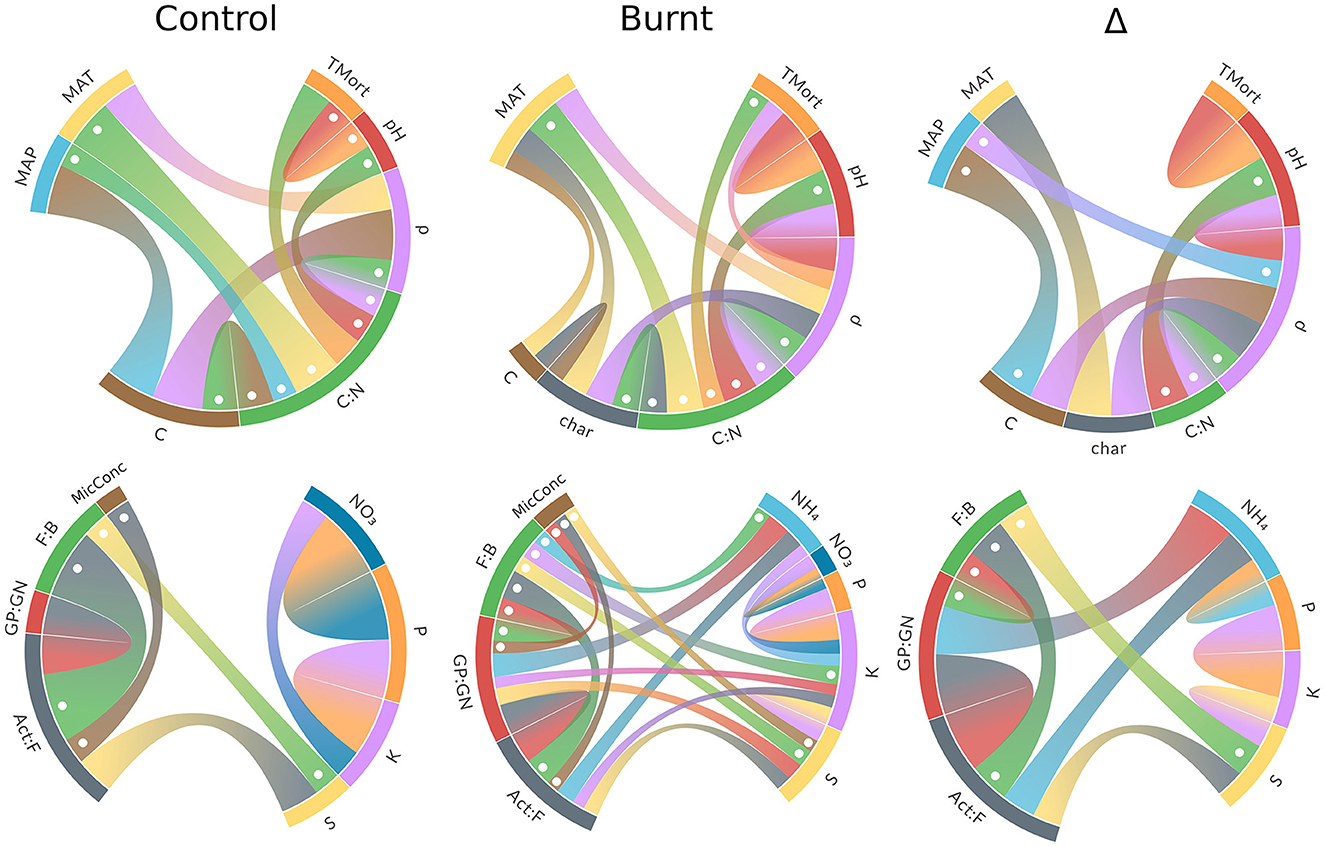
Figure 3. Chord diagrams of significant (p < 0.05) bivariate correlations between climate and forest properties (top row) and microbe and nutrient variables (bottom row). Ribbon thickness is scaled within each diagram (but not across diagrams) to Pearson's r with negative values dotted. Numerical results for climate and forest property diagrams are found in Supplementary Table S1 and microbe and nutrient variable diagrams in Supplementary Table S4.
3.1.2. Nutrient mobilization
Burnt plots experienced large, significant increases in resin capsule-adsorbed inorganic N (iN, 69.7%) relative to their paired controls. NO3 (438.3%) had a greater fire-induced percentage increase than NH4 (39.1%) resulting in a decrease in NH4:NO3 (-60.5%). P (349.7%), K (36.8%), S (56.9%) also had significant increases due to fire with a large drop in iN:P (-79.3%). The remaining nutrients showed no significant differences across the paired burnt and control plots, and therefore were not considered in the rest of the analysis in order to retain focus on fire-labile nutrients. Quantitative data on significant nutrient shifts are shown in Table 2.
3.1.3. Microbial community
Total soil PLFA concentration per soil mass (MicConc) dropped by 24.2% from an average of 1015.70 nmol g−1(CV = 0.32) in control plots to 769.69 nmol g−1 (CV = 0.36) in burnt plots (Table 2). PLFA estimated per unit area (MicMass) dropped by –35.5% due to fire.
GP:GN (28.4%) and Act:F (76.7%) significantly increased in burnt plots relative to controls with F:B (-13.9%) having a smaller, significant decrease (Table 2). Changes in these ratios (Δ values) were each not significantly directly correlated to ΔMicConc.
3.2. Grouped comparison of soil microbes and nutrients to climate, forest properties, and fire severity
Climate, forest property and fire severity variables were used in RDA to explain the 4 PLFA variables (MicConc, GP:GN, F:B, and Act:F) and the 5 nutrient variables (NH4, NO3, P, K, and S) separately for control, burnt and Δ variable distributions. Because they were calculated from these utilized variables, MicMass, iN, NH4:NO3, and iN:P were not included in RDA. The metric for soil drainage, TEM, did not contribute to improving in any RDA and was therefore excluded from all analysis. The first two axes and overall results of the three models were significant. The remaining axes were insignificant, indicating two axes were sufficient for capturing the most dominant relationships for each model.
Model selection was performed, finding that dropping control plot tree mortality increased the of its respective model, though only by 0.001. Burnt plot model was improved by 0.009 by dropping pH. Because these differences were considered small, all variables were therefore retained in RDA models to match those entered into multiple regression analysis to simplify comparison of results. The RDA triplots (Figure 4) and canonical coefficients (Supplementary Table S2) demonstrate the relationships between variables. In each RDA, no explanatory variables were concerningly correlated (r < 0.65 for all) and variable inflation factors were all below 5, and shown in Supplementary Table S3.
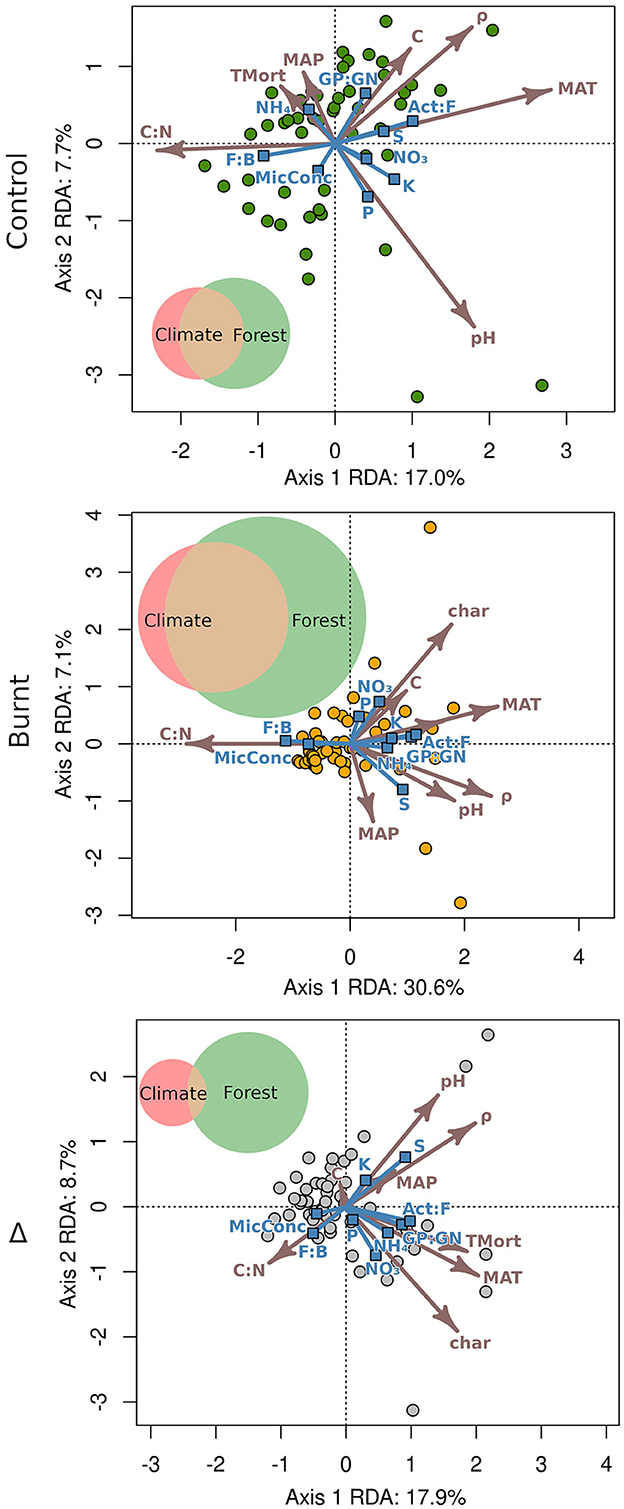
Figure 4. Redundancy analysis triplots with explanatory vectors (arrows) scaled by 3 for legibility. Predicted variables are marked by vectors ending in blue squares and plot scores by circles. Variance partitioning is visualized for each chart with relative area of climate (MAT, MAP), forest (all other explanatory variables), and their overlap proportioned across plots to their magnitude of for control (climate: 0.041, forest: 0.098, shared: 0.082, total: 0.221), burnt (climate: 0.033, forest: 0.181, shared: 0.153, total: 368), and Δ (climate: 0.053, forest: 0.201, shared: 0.013, total: 267) values.
Climate and fire severity had an impact on fire-induced differences in nutrients and microbial community with = 0.267. The Δ triplot and its canonical coefficients reveal that ΔC had a proportionally low influence on model explanation. Variance partitioning (Figure 4) found a stronger direct contribution from fire severity ( = 0.201) than from climate ( = 0.053). Fire had the effect of tightening the overall relationship between explanatory and dependent variables when comparing burnt plots ( = 0.368) to control ( = 0.221). Since climate explained an approximately equal amount of partitioned variance in burnt and control plots, the increased overall in burnt plots relative to control was due to an approximate doubling of values of both the forest property variables and the shared variance component.
Predictor variables better explained the 4 microbial variables when compared to the 5 nutrient variables in separate RDA analysis of control and burnt plot values but not Δ values, with model fits given in Table 3.
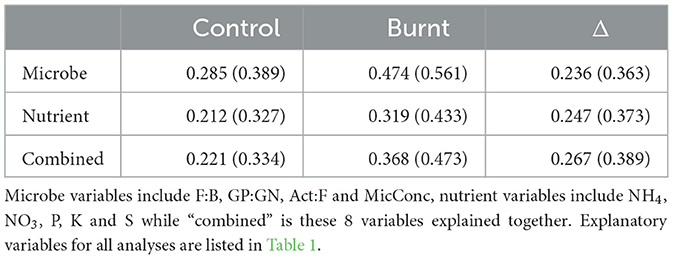
Table 3. (R2 in parentheses) for RDA analysis on dependent variable categories (left column) for the 3 variable sets (header row).
3.3. Individual comparison of soil microbe and nutrient variables to climate, forest properties, and fire severity variables
Results of regressing individual microbe and nutrient variables on climate, forest properties and fire severity after backwards step-wise model selection are shown in Table 4 and visualized in Figure 5. The results indicate varied predictive power of climate and fire severity on the individual Δ microbe and nutrient variables. The strongest effects were found from MAT, Δρ, ΔC:N, and Δchar with no impact from ΔpH and ΔC. Fire-induced increases in tree mortality had more frequent effects on the shifts of nutrients and microbial community than its variation did on these non-shifted variables in burnt or control plots. The highest Δ model R2 values were for S, Act:F, NH4, and NO3 while GP:GN, F:B and MicConc were significantly, though poorly explained (R2 < 0.3) and P and K had no significant regression models.
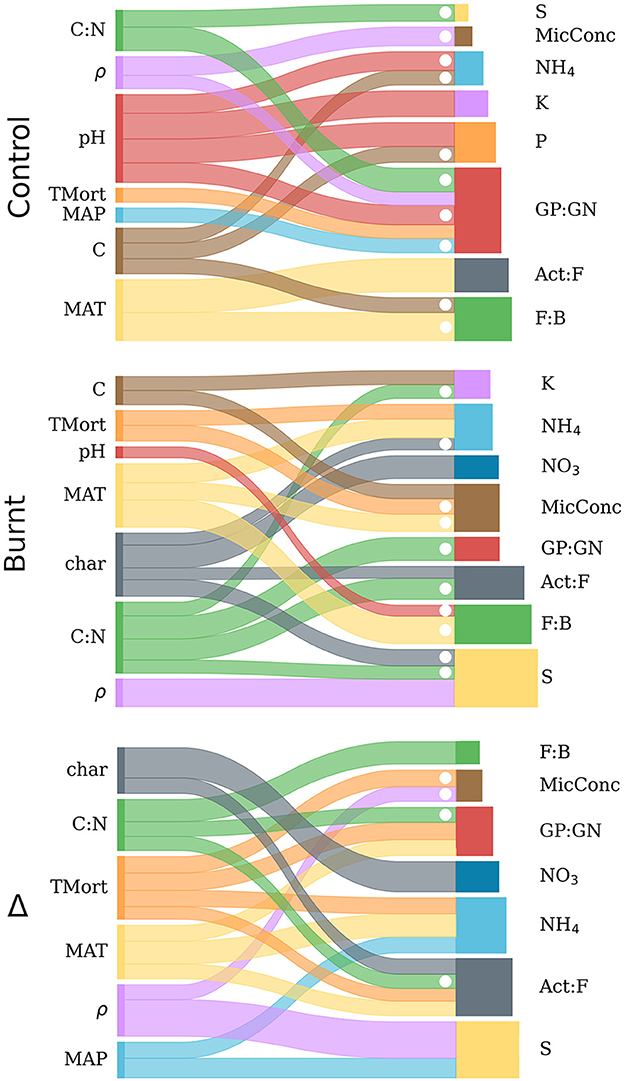
Figure 5. Sankey diagrams representing the backwards step-wise model selection results from control, burnt and Δ variable distributions. For each of the three graphs, explanatory variables are listed on the left and connected by ribbons to the dependent variables they predict (right). Ribbon width is scaled to the magnitude of the standardized regression coefficient from its corresponding independent variable (within each graph, but not across graphs), and dotted when this value was negative. The bars on the right sides of the graphs are scaled horizontally to the R2 values derived from multiple regression for each independent variable and are proportional across all graphs. Numerical results for the diagrammed regressions are found in Table 4.
Burnt plot regressions had more frequent strong explanations (R2 > 0.5) of dependent variables than in control plots due mostly to improved prediction of microbial variables. The rearrangement of explanatory variable influence on the regressands when compared to control plots, as seen in Table 4, show fire induced a clear shift of the climate and forest property covariation patterns with soil processes. Notably, a reduced influence of pH on nutrient mobilization, an influence of soil charring, and an added control of MAT on NH4 mobilization was observed in burnt plots.
In additional multiple regression analysis, MicMass was significantly explained in control plots (R2 = 0.738) by ρ (β = –0.293) and C (β = 0.997), burnt plots (R2 = 0.711) by C (β = 0.843), and Δ values (R2 = 0.640) by ΔC:N (β = –0.262) and ΔC (β = 0.727).
3.4. Relationships between microbial community and nutrient mobilization
Pearson r values for significant bivariate correlations among the microbe and nutrient variables are given in Supplementary Table S4 and visualized in Figure 3. These results reveal that NH4 and S were the nutrients with the most connections to microbes in their Δ values, and more connection points between microbes and nutrients were formed overall in burnt plots relative to control. Nutrients have more frequent correlations with themselves in burnt plots than in control plots, though control plots have stronger, if fewer, of these correlations.
Canonical correlation models between nutrient and microbial variables were significant for burnt (p < 0.001) and Δ (p = 0.010) dataset pairs but not for control (p = 0.064). The first canonical vectors were correlated for control (r = 0.570), burnt (r = 0.736) and Δ (r = 0.645) values (Table 5), with charts of first and second axes found in Supplementary Figure S1. RDA analysis found that for control, burnt and Δ dataset pairs the grouped microbial variables were better explained by the nutrient dataset, than vice versa, as judged by increased (Table 5).
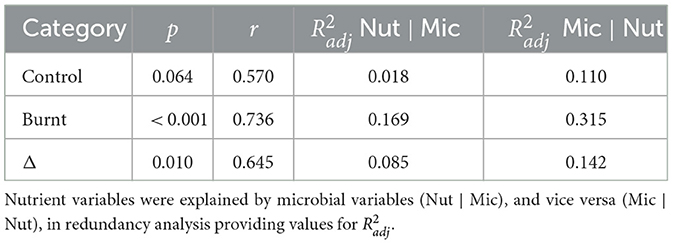
Table 5. Canonical correlation analysis was performed to compare nutrient and microbial datasets with p values for the entire model derived from the F-test of Pillai's trace and Pearson's r extracted as the correlation between the first pair of canonical variables.
4. Discussion
The results of this study provide a highly-replicated survey of important boreal forest properties and their response to fire which were previously only available from cross-study comparison. Additionally, the geographic expanse of field sampling allowed for substantial coverage of the extent of forest conditions found within Fennoscandia and provided novel analysis of the modified effect that altered temperature and precipitation can have on recently burnt ecosystems. The following discussion serves as an answer to the original hypotheses of this study while lending support to previous observations of fire processes in the boreal region and suggesting additional avenues of further research effort.
4.1. Microbial and nutrient response to fire can be predicted by climate and forest properties
4.1.1. Forest structural shifts explain altered microbial community and enhanced nutrient mobilization across broad ranges of climatic influence
Fire had a significant effect on restructuring the microbial community and enhancing openings in the nutrient cycle. This was demonstrated by strong fire-induced shifts in Act:F (76.7%), GP:GN (28.4%) and F:B (–13.9%) as well as a significant drop in overall microbial biomass per soil mass (MicConc, –24.2%). These results are consistent with studies finding fire to increase dominance of Actinobacteria and reduce fungal biomass in boreal forests (Whitman et al., 2019; Nelson et al., 2022), though limited comparison is available for fire's influence on shifting GP:GN. Reduction in MicConc was approximately half that found in North American boreal forests (52% reduction) which tend to experience higher fire intensity and therefore potentially higher heat-induced mortality (Holden et al., 2016). Resin capsules detected significant increases in NH4 (39.1%), NO3 (438.3%), P (349.7%), K (36.8%), and S (56.9%) in the soil solution, all of which have previously been found to be elevated in early post-fire runoff in Swedish boreal forests (Granath et al., 2021).
These combined shifts were significantly predicted by fire severity and climate metrics in RDA ( = 0.267), though fire-induced reduction of organic layer C (ΔC) only had minimal contribution to RDA prediction and no significant influence when predicting the same variables individually using multiple regression. ΔpH did not significantly contribute to multiple regression for any dependent Δ variables despite high canonical coefficients on the two RDA axes and strong effects of fire-shifted pH on microbial communities documented in previous study (Day et al., 2019; Whitman et al., 2019). Considering it also had the highest variance inflation factors of any variables (suggesting issues with multicollinearity and possible confounding), assessing the impact of fire induced shifts of pH on soils should be performed with caution and consideration of the broader fire impact as well as the expected effect across the specific extent of pH variation analyzed. These combined results demonstrate that accurate assessment of fire severity on the soil microbial community and nutrient cycling in boreal forest soils requires simultaneous assessment of multiple aspects of ecosystem restructuring and examination of their robustness between statistical methods, with careful consideration of the expected impact across their sampled variation. Furthermore, ΔC appeared to be only suitable as a metric for fire-induced shifts in total area-normalized microbial biomass (MicMass), which it significantly predicted in multiple regression along with ΔC:N (R2 = 0.640), rather than shifts in microbial community structure and rates of nutrient mobilization.
4.1.2. Influences of forest structure and climate on soil activity are differentiated by time-since-fire
Climate and forest properties were also predictive of the microbial community and nutrient levels in control plots ( = 0.221) with greater explanatory power in burnt plots ( = 0.368), indicating microbial and nutrient variation is more strongly related to the sampled structure of burnt forests than unburnt. Control plot was only slightly improved (0.222) by dropping the variable for tree mortality (TMort). Control plot tree mortality was only 4.21% on average, and was better used as an indicator of disturbance impact in burnt soils where its variation appeared to have strong effects on the microbial community and nutrient turnover. Burnt plots had an improved (0.377) through the dropping of pH, which had the highest variable inflation factor of the explanatory variables in the model, again suggesting explanatory power of its variation in burnt plots should be carefully assessed among covarying ecosystem properties when making causal assumptions about its effects. Multiple regression results otherwise appeared to have no obvious strong deviations from that represented by RDA axis loading.
This study aimed to explain microbial-nutrient processes with general and readily measurable metrics for application at broad scales, however, finer scale sampling may lead to increased explanatory power of some models. For example, fire impact on tree mortality and understory removal can alter sub-canopy microclimate and evapotranspiration rates as well as increase solar insolation on soil surfaces with a decreased albedo due to charring, creating a patchwork of microsites across the forest floor (Certini, 2005; Chambers et al., 2005; Bond-Lamberty et al., 2009; Pimentel and Arheimer, 2021). Measurement of these influences on soil conditions (e.g., altered temperature and moisture) may enhance the link between the measured climate and forest properties and microbial and nutrient variables. Furthermore, a more detailed characterization of the microbial community may allow for building of more specified explanatory models, for example, regarding the influence of pH (Day et al., 2019; Whitman et al., 2019) or tree mortality (Pérez-Izquierdo et al., 2021) on proportions of fungal functional groups.
4.1.3. Observable expression of microbial and nutrient interrelation is heightened in burnt soil
The number of significant bivariate correlations between the microbial and nutrient variables as well as canonical correlation (first axes) were greater in burnt plots (r = 0.749, overall model significant) compared to control (r = 0.546, overall model insignificant). This demonstrates a stronger coupling of microbial community structure to the openness of the nutrient cycle in burnt soils. While microbial group variation may contribute strongly to nutrient turnover rates in unburnt soils (e.g., gross mineralization, uptake into biomass), their lesser connection to nutrient mobilization compared to burnt soils is suggestive of increased presence of preservative feedback mechanisms that promote nutrient cycle closure, such as resource exchange with plants (Högberg et al., 2017; Frey, 2019). Additionally, microbial variables were more strongly predicted in both control and burnt plots by the environmental variables (climate and forest properties, Table 3) and by nutrients (Table 5) than nutrients were by the environmental variables and microbes. This shows that the microbial community was much more responsive to the sampled environmental factors with a substantial portion of their variation tending to be expressed through the mobilization of nutrients, especially in burnt plots, where nutrient variables were overall less predictable. The result may be in part due to a wider array of possible, though here unmeasured, influences (including residual impact of the chaotic heating patterns during fire) which drive mobilization of the large pool of nutrients already present in soil matter (Wan et al., 2001; Granath et al., 2021). Patterns which explain early stage recovery of the living microbial community may generally only be able to be explained by a more restricted set of quantifiable variables, which are constrained by their relation to microbial requirements for growth and observable metabolic waste products (Schimel and Bennett, 2004). While this study robustly demonstrates a quantitative response of the microbial community and nutrient cycling over broad gradients of climate and readily measurable forest structure, it also forms a basis for further research questions regarding more detailed investigation that can decipher the mechanisms by which these two ecosystem components covary and feed back on one another and what additional factors may predict their variation.
4.2. Fire enhances climatic control on microbial community structure and nutrient cycling
The incorporation of MAT and MAP into RDA and multiple regression analysis exemplified their ability to improve explanation of microbial structure and nutrient cycling in burnt and unburnt soils and the differences between them. Variance partitioning (Figure 4) of RDA models demonstrated the strongest proportional direct influence of climate on the dependent variables was across control plots, with forest properties and fire severity becoming relatively more important in burnt and Δ variable distributions, respectively. Shared explained variance of climate and forest properties was substantial in both burnt and control plots though constituted a greater proportion of the total explained variance within burnt plots. Although, shared explained variance in plot-pair differences was approximately 10 times lower than in burnt plots. These patterns show that fire tended to enhance coupling of climate and forest properties when explaining soil microbial community structure and nutrient mobilization in burnt plots, while climate and fire severity more separately affected the fire-induced shifts in these factors (i.e., Δ values). Assuming a one-way causal influence of climate on forest properties, the consequence of these observations is that climatic control on the forest properties that affect soil microbial community and nutrient mobilization can increase in recently burnt plots, further emphasizing the need to better incorporate the cascading influence of climate factors into research regarding wildfire impact on ecosystem restructuring.
The results also indicate that climate, most strongly MAT, independently increased microbial and nutrient dissimilarity (i.e., Δ variables) between burnt and unburnt stands. Studies that occur within a single climate zone may overlook this effect due to its lack of variation, while the broad climatic gradient encompassed within the current study highlights climate variation as an important factor that influences fire impacts on soil processes and can enhance comparison of studies across larger geographic areas.
4.3. Ecosystem restructuring through burning is responsible for major shifts in important microbial indicators
4.3.1. Warmer post-fire conditions favor bacteria over fungi
The large increase in tree mortality, from 4.21% in control plots to 44.96% in burnt plots on average, likely reduced rhizosphere based metabolism and living biomass of its dependent organisms (e.g., mycorrhizal fungi) as has been observed in a previous Swedish boreal wildfire study (Pérez-Izquierdo et al., 2021). This, paired with the demise of typically more heat sensitive and slow growing fungal species (relative to bacteria) (Treseder et al., 2004; Köster et al., 2021) were expected to be responsible for the observed significant drop in F:B in burnt plots relative to control. However, shifts in this ratio (ΔF:B) were not related to tree mortality or soil charring, but to ΔC:N. F:B across burnt plots was negatively and strongly controlled by pH and MAT which demonstrates that over natural gradients of these variables, experimentally-based observations that fungi are generally more cold and low-pH adapted are well supported (Pietikäinen et al., 2005; Rousk et al., 2009). Though, contrary to expectation, pH did not covary with F:B in control plots but had a strong influence from MAT and total organic layer C, which may be due in part to the low variability of pH across these plots (mean = 3.71, CV = 0.08). Therefore, the proportional adjustments in fungal and bacterial PLFA markers appear to be less dependent on the more immediate, mortal effects of fire (e.g., tree mortality, charring) and more dependent on longer-term post-fire shifts in abiotic soil conditions (MAT, pH) and substrate quality (C:N).
Recent knowledge regarding overlap of functional capabilities of fungi and bacteria has made it difficult to generalize their ratio when explaining processes in the soil (Rousk and Frey, 2015). However, the two categories still retain many general differences as highlighted by F:B being the first and second best explained dependent variable in multiple regression analysis within control (R2 = 0.446) and burnt (R2 = 0.600) plots, respectively. These strong covariations with important ecosystem variables across recently burnt and unburnt forests merit further paired study of fungi and bacteria in flammable ecosystems.
4.3.2. Gram-positive bacteria dominate when soil carbon inputs decrease
Averaged across the 50 burnt plots, fire increased mortality to nearly half the overstory and removed almost the entire understory C pool [understory represents a major fraction of C litter input (Nilsson and Wardle, 2005; Hensgens et al., 2020)], suggesting substantial reductions of labile C input to the soil from root exudates and fresh litter (Pérez-Izquierdo et al., 2021). Along with the typical exhausting of heat-labilized C by 1-year post fire, this leaves a greater proportion of microbially accessible C to be found in soil stores (Ludwig et al., 2018). Therefore, microbial substrate in the sampled burnt plots was expected to be limited to the more refractory root and microbial necromass along with heat-exposed residual soil material. The shifting toward this resource pool and the strong increase in GP:GN in burnt plots (28.2%) reflects recent research linking higher GP:GN ratios to feeding on less bioavailable C sources (Fanin et al., 2019). Indeed, GP:GN was negatively related to C:N within multiple regression in control plots [reduced C:N associated with more decomposed material (Callesen et al., 2007)] as well as in burnt plots and plot-pair differences [reduced C:N associated with extent of soil charring (Eckdahl et al., 2022a)]. ΔGP:GN was additionally explained by increased tree mortality and MAT, indicating the heightened advantage of GP bacteria via the severing of labile C from rhizosphere-based metabolisms. These trends support the two ideas that fire shifts soil material toward greater recalcitrance (Nelson et al., 2022) and that GP bacteria dominate over GN bacteria when feeding on these recalcitrant pools (Fanin et al., 2019). A more detailed and simultaneous observation of these two trends is merited to better understand the processing capabilities of these bacterial groups regarding recalcitrant material of both more direct biogenic as well as pyrogenic origin.
4.3.3. Actinobacteria outperform fungi in both warmer and heat-altered soils
A particular subgroup of GP bacteria, Actinobacteria, increased strongly in relation to the PLFA markers for fungi in response to burning (i.e., 76.7% increased Act:F). The observed linkage of Actinobacteria to fire resistance and rapid regrowth in post-fire soil and feeding on residual, charred C substrate (Nelson et al., 2022) along with the capability of forming filaments and performing similar decomposition functions as saprotrophic fungi (Mercer et al., 1996; Lacombe-Harvey et al., 2018; Nelson et al., 2022), suggests they are able to fill fungal niches in fire-disturbed soil environments. The current study found burnt plot Act:F to be strongly influenced by soil C:N and charring, with additional effects of MAT and tree mortality on ΔAct:F. While GP:GN strongly relates to C:N, which itself correlates with amount of char in burnt plots (r = –0.457), the additional direct effect of char on Act:F aligns with the above-mentioned observations that Actinobacteria are particularly responsive (survival and regrowth rates) to the extent of soil heating. The only control on Act:F in control plots was MAT (R2 = 0.422), where this strong relationship is further suggestive of the potential niche overlap of the two groups with fungi outperforming Actinobacteria in cooler soils. Therefore, Actinobacteria are proposed to take on generalist decomposition strategies similar to filamentous saprotrophic fungi, but which they can outperform in both fire-disturbed and warmer conditions.
4.3.4. Selective mortal and generative effects of high heat may regulate the relative influence of aboveground and belowground processes on ecosystem function in response to climate warming
Soil microbial biomass concentrations (MicConc) were negatively influenced by tree mortality (TMort) in burnt plot multiple regression, demonstrating synchronized reduction of aboveground and belowground biomass in burnt forests across a wide range of fire conditions. However, when controlling for tree mortality, an additional negative influence of MAT on MicConc was observed, suggesting belowground biomass concentration is proportionally elevated within burnt forests in cooler regions. This adjustment in ratios of surviving biomass density across the forest floor interface, paired with associated microbial community functional shifts, may alter the relative dominance of aboveground-belowground feedback mechanisms (e.g., complementary C and N limitation) in regulating soil processing, potentially leaving a larger portion of nutrient turnover to be controlled by the metabolic demands of soil organisms alone in more northern forests (Pérez-Izquierdo et al., 2021). Predicted climate warming and increased fire frequency may provide for this trend of burn impact to extend over time in addition to space, continuously driving regional change of soil turnover processes and rates. Further, time-sensitive study is needed to distinguish the competing mortal and generative influences of fire on ecological community structure, which are difficult to separate through observations taken only over discrete time periods. Particularly, methods providing for the differentiation of fungal groups beyond what is currently possible using PLFA markers may give greater insight into soil process rates under both the shifted roles of saprotrophic and mycorrhizal fungal groups relative to Actinobacteria (Zak et al., 2011) as well as the mechanisms and time-scales by which these fungal groups and associated vegetation can reestablish dominance during recovery from wildfire (Treseder et al., 2004).
4.4. Wildfire can rebalance relative availability of carbon which promotes nutrient mobilizing forest functioning
The typically high intensity wildfire in North American boreal forests has been observed to both remove C at similar rates as N from the organic soil as a whole (Boby et al., 2010) and leave behind pyrogenic material of enhanced C:N (Santín et al., 2016). While in contrast, recent observations have shown that the low-intensity fire regimes of Eurasia can selectively remove C from the organic layer, leaving behind residual pyrogenic material of greatly reduced C:N (Dymov et al., 2021; Eckdahl et al., 2022a). These contrasting patterns of immediate wildfire elemental rebalancing may be responsible for fundamental regional differences in early post-fire nutrient cycling and microbial community functioning, making comparison of knowledge regarding these topics difficult when early post-fire ecosystem conditions are not considered holistically. For example, post-fire experimental N fertilization in North American boreal soil has been shown to shift microbial strategy toward an overall greater seeking of C over N from soil organic matter, significantly increasing soil decomposition rates (Allison et al., 2010). The N preservation of Eurasian wildfire burning may allow for a general soil N excess that mimics this N fertilization and stimulates enhanced C removal, closing the large gaps regarding total fire-induced C loss which are initially produced by the higher emission rates during burning in boreal North America (Eckdahl et al., 2022a). This N excess can additionally be stimulated by fire-induced mortality and incineration of nutrient demanding plants and reduced provisioning of labile C inputs to the soil, leaving C to be sourced to a greater extent from residual heat-altered soil organic material (Figure 1). The current study observed a near 10-fold increase in tree mortality (44.96% on average in burnt plots) accompanied by an average 90% removal of understory biomass along with transformation of about 25% of residual organic soil into a conglomerated layer of highly nitrogenous char (reducing overall organic layer C:N from 47.68 to 38.22). These factors are considered to be important for priming soils for expulsion of excess nutrient in active soil layers during microbial acquisition of C through the decomposing of soil organic matter during early post-fire recovery. This section analyzes the observed nutrient mobilization patterns and discusses their connection to climate, forest properties and the microbial community.
4.4.1. Nitrogen mobilization is driven by microbial community structure after wildfire nutrient pulses are exhausted
It is proposed that in the sampled burnt plots specifically, N mobilization was more controlled by the microbial community than vice versa. This is supported by previous reports that the pulses of heat-labilized C and N tend to be largely exhausted by 1 year post fire (Wan et al., 2001; Ludwig et al., 2018; Granath et al., 2021) and the demonstrated association of increased GP:GN, with more complex C substrate processing (Fanin et al., 2019). This metabolic shift toward C oligotrophy entails greater energy requirements to incorporate C into biomass and more useful forms of chemical energy (e.g., ATP), therefore driving processing of N-containing compounds which expels excess mineralized N as waste (Schimel and Bennett, 2004). Indeed, GP:GN was strongly positively correlated to NH4 mobilization in burnt soils (r = 0.630). ΔGP:GN was additionally positively explained by tree mortality and MAT, indicating the increased advantage of GP bacteria via the severing of labile C from rhizosphere-based metabolisms. Alternatively, if GP:GN was more driven by intake of residual heat-synthesized NH4 that was left unincorporated due to root mortality, it would be expected that this N either would be occluded from resin adsorption due to biotic sequestration, as is in control plots, or resemble NO3 in burnt plots where its exact relative excess appeared to be unrelated, rather than steadily outpacing any microbial indicators. Therefore, it is most likely that GP:GN was a genuine indicator of N-mobilizing post-fire oligotrophy.
4.4.2. N saturation may be a dominance strategy of Actinobacteria which inhibits soil turnover
Elevating mineral N in the soil solution has the potential to increase relative proportions of highly competitive, though N-limited copiotrophy-oriented microbes. However, this can only be true when enough C is available to match N uptake. With the reduced abiotic and plant sourced C along with elevated available N, C from soil organic matter is likely to be largely conserved by the oligotrophs that extract it, stifling N utilization by competing microbes. Although, elevated dissolved N can still have reciprocal effects on the microbial community that do manage to receive enough C.
Considering the suggestion of saprotrophic niche overlap of Actinobacteria and fungi, a surprisingly strong relationship was measured between Act:F and NH4 in burnt plots (r = -0.499), which may be produced by lack of differentiation between mycorrhizal and free-living saprotrophic fungi by PLFA markers or confounding by unmeasured ecosystem shifts that affect both NH4 mobilization and Act:F variables themselves. For example, the covariation of F:B with NH4 found in the current study's burnt plots (r = -0.397), may be simply due to a confounding relationship with MAT, rather than suggesting a differentiated N utilization strategy of fungi and bacteria. However, experiments in unburnt boreal forests in northern Sweden found a threshold of high N fertilization that reduced overall microbial biomass with no effect on F:B, though Actinobacteria concentrations had strong absolute increases (Maaroufi et al., 2019). Also, relieved N-deprivation in remaining living trees after fire has the potential to reduce exudate support to mycorrhizae, limiting their biomass contribution to the fungal community (Högberg et al., 2010; Meier et al., 2020). Additionally, increased inorganic N concentrations in the soil have been observed to enhance the ability of Actinobacteria to process soil material over saprotrophic fungi (potentially via exoenzyme downregulation), consequentially reducing soil turnover rates (Zak et al., 2011). Therefore, elevated NH4 may be both a waste product of actinobacterial metabolism of low C:N charred material and a promoter of its dominance relative to fungi. Increasing inorganic N in the soil solution may serve to enhance decomposition of relatively limited C (Allison et al., 2010), though eventually inhibit it at higher levels via the shifting of functional roles among saprotrophic microbe groups. The extent of this effect deserves further study, especially in highly nitrogenous post-fire soils.
4.4.3. Climate enhanced nitrogen mobilization is permitted by tree mortality and buffered by increased soil cation-exchange capacity
NH4 mobilization in burnt plots, as measured by the installed resin capsules, was significantly explained in multiple regression by MAT (β = 0.486), tree mortality (β = 0.383) and amount of char (β = –0.308). The positive relationship to MAT is likely due to its effect of elevating temperature dependent soil process rates (Mekonnen et al., 2019) while the positive relationship to tree mortality can be explained by the reduction of NH4 uptake via a decrease of living roots, leaving a greater proportion of gross mineralized N to be adsorbed by the installed resin capsules. An additional effect of tree mortality may be through a proportional shifting of microbial C substrate from labile root exudates to residual heat-altered soil stores, including root necromass, causing a relative deficit in C availability and enhanced N mineralization (Schimel and Bennett, 2004; Högberg et al., 2017; Köster et al., 2021).
The negative relationship of NH4 to char can be due to immobilization of positively charged NH4 by the increased specific surface area and negative charge typical of charred material (Makoto and Koike, 2021). This observed negative influence suggests NH4 is continuing to be immobilized by charred material after the 1 year post-fire period due to soil storage capacity for NH4 not yet being reached. The only significant correlations from climate, forest properties and fire severity to NO3 and ΔNO3 were positive ones to char layer mass. This suggests the opposite effect to NH4, with NO3 ions being repelled from soil-bound states due to its negative charge, with the charge reversal and observed strong overall fire-induced drop in NH4:NO3 mobilization (-60.5%) pointing to this electrostatic effect. Previous observation of reduced nitrification rates in burnt Swedish boreal forest soils (Ibáñez et al., 2022) and the lack of explanatory variables for NO3 mobilization in the current study also suggest it is largely a residual, more direct consequence of abiotic fire-induced mineralization, while the restricted association of biotic factors to NH4 alone suggests they directly control its mobilization at the 1 year post-fire stage rather than their relationship being a product of confounding abiotic heating influences (i.e., that fire increases both NH4 and NO3 though affects the microbial community in ways that covary only with NH4). Soil charring may act to buffer mobilization of excess NH4, while potentially enhancing eutrophication of surrounding ecosystems through expelling of abiotically synthesized NO3.
4.4.4. Macronutrients are generally expelled from organic matter under soil carbon deprivation
Biomass derived nutrients were expected to share mobilization patterns with N due to similarly being expelled as waste during decomposition of soil organic matter under C limitation. Indeed, the significantly increased K and S mobilization in burnt plots relative to control correlated to burnt plot F:B, GP:GN and Act:F as did NH4. Control plot F:B and Act:F also correlated to S [found largely in organic matter, along with N (Houle and Carignan, 1992)], but no microbial metrics had significant bivariate correlations to NH4 in control plots. This is again suggestive of fire driven release of general ecosystem N limitation and a shift toward widespread limitation of C, and hence the increased observable nutrient mobilization. Additionally, the emerging influence of GP:GN on nutrient mobilization in burnt plots also reflects its role in shifts toward an oligotrophic C-limited soil metabolism and expelling of nutrient excess.
S and K mobilization were additionally explained by the negative multiple regression influence of C:N in burnt plots, with low C:N indicative of C limitation in soils (Callesen et al., 2007), further highlighting the potential influence of reduced C availability in enhancing the mineralization of these nutrients during decomposition. Burnt plot S was additionally negatively explained by char and also positively by ρ, which had a large, here unexplained, contribution to the overall high R2 (0.664), suggesting its influence over fire-related processes in soils is deserving of further investigation.
Although none of the remaining nutrient elements experienced significant fire-induced elevation of resin capsule sequestration, their ratios to more fire-labile elements and drivers of their mobilization patterns may have shifted across burnt and unburnt soils. Further usage of this study's large, highly-replicated and freely-available dataset is encouraged to directly address or support further research questions.
4.4.5. Wildfire mobilized phosphorous can form large soil standing pools vulnerable to leaching
Resin capsule-adsorbed P had no bivariate correlations with the microbial community in control, burnt or Δ variable sets and had no significant relationships to measured environmental variables except in control plots where it was explained by pH (similar to K) and total organic layer C. Interestingly, multiple regression revealed a release of observed control plot pH influences from the mobilization of both burnt plot and Δ values for P and K. The asymmetry of RDA analysis demonstrated that in both burnt and control plots, microbial community variation is to a greater extent explained by the measured openings in the nutrient cycle than vice versa (Table 5). A greater proportion of unexplained nutrient variation in burnt soils may be explained by additional observable factors. However, the lack of measured control over P and K, in particular, from the included burnt plot variables may be in part due to a statistical drowning of their otherwise measurable influences due to the residual chaotic, time-of-fire heating effects on nutrient mineralization and/or ecosystem changes that released bound ionic forms. This may be especially true for P (and for similar reasons, NO3) with a measured drastic increase (349.7%) in burnt plots relative to control, which likely allowed fire-mobilized P to remain elevated in the soil solution despite leaching and immobilization processes over the 1 year post-fire period.
While N is most commonly the limiting nutrient in boreal systems, periods of fire absence much greater than typical boreal fire return intervals have been observed to induce P limitations on ecosystem structure and process rates (Lagerström et al., 2009). Currently, not enough quantitative data is available regarding fire-related P mobilization and associated removal in boreal forest soils to predict whether the large fire-enhanced P mobilization observed in the current study, or those that might be observed in future fire regimes of increasing frequency and severity, could cause P limitation within a given fire return interval. It is also unknown whether this potential for retrogressive characteristics such as reductions in peak ecosystem biomass may be prevented by altered P demands of concurrent climate change induced shifts in vegetation and microbial communities. Although, the persistence of drastically increased reservoirs of solvated P in burnt soils measured in the current study (especially in relation to more typically limited inorganic N, ΔiN:P = –79.3%) imply a potential for significant removal via leaching from the burnt forest systems (Granath et al., 2021) and resulting eutrophication of surrounding ecosystems that should be considered for further quantitative analysis in future boreal wildfire study.
4.4.6. Alternate ecosystem trajectories in boreal Eurasia may be possible under current and future climate and fire regimes
One key emerging question is whether the suggested rebalancing of the soil C:N ratio (e.g., through mobilization and subsequent leaching of N) to match early post-fire metabolic demands will have notable, lasting impacts on the reestablishment of typically N-limited organisms, such as plants. Recent observation and modeling efforts suggest more frequent fire and enhanced N cycling rates associated with climate warming in boreal forests can lead to major shifts in vegetation cover (Shenoy et al., 2013; Mekonnen et al., 2019; Johnstone et al., 2020; Baltzer et al., 2021). Observations in the current study linking increased MAT to reduced C:N, N-mineralizing microbial community structure and enhanced NH4 mobilization in burnt soils closely resemble the proposed drivers of these vegetation cover shifts. Furthermore, separate wildfire research in Sweden under climate conditions of the past decade has shown that elevated gross mineralization of N (per unit C) can persist at 4 years post fire, even when soil C:N and net N mobilization is equivalent to nearby unburnt stands (Ibáñez et al., 2022). This large 2014 fire was observed to have widespread conifer regeneration failure rates with replacement of overstory species that are expected to grow into the largest deciduous dominated forest in boreal Fennoscandia (Gustafsson et al., 2019). With the complicated land-use history of Sweden, it is difficult to determine where an ever-shifting “natural” boreal ecotone could currently lie (Axelsson et al., 2002; Barnekow et al., 2008; Goldblum and Rigg, 2010) and whether overstory dominance shifts are due mostly to fire's release of management influence or previous climatic constraints. Further time-extended research of nutrient cycling and vegetation regrowth in response to current fire conditions is needed to predict future forest trajectories across characteristic boreal regions, however the patterns found in the current study suggest that increasing MAT and burn frequency in similar forest fire regimes have the potential to alter the proportional cycling of elements across the broad expanse of the Eurasian continent (Mekonnen et al., 2019; Baltzer et al., 2021), thereby significantly influencing continental-scale land cover and global biogeochemical cycles.
4.5. Summary and outlook
The results of this study suggest wildfire shifted the sampled forest soils toward oligotrophic processing of C in an excess of N, P, K and S, providing for releases of these four macronutrients from soil organic matter to remain mobilized as waste in the soil solution. This process was strongly related to the GP:GN ratio, which may be responsible for continued expelling of excess nutrients from the forest system until nutrient-conserving aboveground-belowground feedback mechanisms are reestablished via sufficient plant regrowth. Climate appears to have an altered and overall enhanced control over ecosystem function and structure in recently burnt forests, and the effects of the previous decades of global warming carry the potential to emerge as modified ecosystem recovery trajectories, such as shifts toward more rapid nutrient cycling rates and widespread increases in deciduous dominance.
Gradient studies allow for the quantitative observation of the influences of multiple interacting factors on measurable process rates that emerge through the amplifying or mitigating feedback mechanisms present in all natural ecosystems, which by design are removed in more strict experimental approaches (Dunne et al., 2004; Fukami and Wardle, 2005; De Frenne et al., 2013; Currie, 2019). This study explained its observations through knowledge of fundamental biological properties of organisms, ecological theories of species interaction and principles of mass balance that were developed over many years and utilizing a variety of approaches. Similarly, the addressing of the hypotheses of this study requires further varied research strategies to confirm and extrapolate upon. However, the high replication, extensive variation of measured properties and relative freedom from pseudoreplication (Bataineh et al., 2006) allow this study to serve as both context and inspiration for more controlled investigation into fire processes while also being geographically expansive enough to allow its results to be suitable for regional comparison of the varied circumpolar impacts of boreal wildfire. Furthermore, the novel analysis of climate demonstrates the range of its importance in assessing microbial and nutrient function in burnt soils and opens an avenue for development of increased understanding of the impacts of climate change on flammable ecosystems.
Data availability statement
All original data collected and prepared for this manuscript is fully available at the following repository: https://doi.org/10.5281/zenodo.7406599. All additional data used for analysis in this paper was taken directly from the following publication: https://doi.org/10.5194/bg-19-2487-2022.
Author contributions
JE, JK, and DM: conceptualization, investigation, methodology, project administration, supervision, and writing—review and editing. JE: data curation, formal analysis, software, validation, visualization, and writing—original draft preparation. JK and DM: funding acquisition and resources. All authors contributed to the article and approved the submitted version.
Funding
JE was supported by the strategic research area Biodiversity and Ecosystems in a Changing Climate, BECC, at Lund University. JK was supported by the Carlsberg Foundation (grant CF20-0238). DM was supported by funding from the European Research Council under the European Union's Horizon 2020 research and innovation programme (ECOHERB; grant no. 682707).
Acknowledgments
Thanks to Michael Gundale and representatives from SLU Umeå and Unibest for advice, explanation and lab work regarding sample collection and processing. Ryan Sponseller contributed helpful insight to results interpretation. Attentive lab management and assistance by Marcin Jackowicz-Korczynski at Lund University is recognized and valued. Field assistance from Femke Pijcke, Caroline Hall, Mathias Welp, Rieke Madsen, Neija Elvekjær, Geerte Fälthammar-de Jong, Lotte Wendt, Julia Iwan, and Henni Ylänne was greatly appreciated. Assistance with figure production was provided by Tamara van Steijn. Thanks to the editor and two reviewers for their time investment toward the processing and improvement of this manuscript. Gratitude is given to friends and colleagues for support with ideas and inspiration for this research.
Conflict of interest
The authors declare that the research was conducted in the absence of any commercial or financial relationships that could be construed as a potential conflict of interest.
Publisher's note
All claims expressed in this article are solely those of the authors and do not necessarily represent those of their affiliated organizations, or those of the publisher, the editors and the reviewers. Any product that may be evaluated in this article, or claim that may be made by its manufacturer, is not guaranteed or endorsed by the publisher.
Supplementary material
The Supplementary Material for this article can be found online at: https://www.frontiersin.org/articles/10.3389/ffgc.2023.1136354/full#supplementary-material
References
Allison, S. D., Gartner, T. B., Mack, M. C., McGuire, K., and Treseder, K. (2010). Nitrogen alters carbon dynamics during early succession in boreal forest. Soil Biol. Bioch. 42, 1157–1164. doi: 10.1016/j.soilbio.2010.03.026
Axelsson, A.-L., Östlund, L., and Hellberg, E. (2002). Changes in mixed deciduous forests of boreal sweden 1866-1999 based on interpretation of historical records. Landsc. Ecol. 17, 403–418. doi: 10.1023/A:1021226600159
Baltzer, J. L., Day, N. J., Walker, X. J., Greene, D., Mack, M. C., Alexander, H. D., et al. (2021). Increasing fire and the decline of fire adapted black spruce in the boreal forest. Proc. Natl. Acad. Sci. U.S.A. 118, e2024872118. doi: 10.1073/pnas.2024872118
Barnekow, L., Bragée, P., Hammarlund, D., and St. Amour, N. (2008). Boreal forest dynamics in north-eastern sweden during the last 10,000 years based on pollen analysis. Veg. Hist. Archaeobot. 17, 687–700. doi: 10.1007/s00334-008-0157-7
Bataineh, A. L., Oswald, B. P., Bataineh, M., Unger, D., Hung, I.-K., and Scognamillo, D. (2006). Spatial autocorrelation and pseudoreplication in fire ecology. Fire Ecol. 2, 107–118. doi: 10.4996/fireecology.0202107
Betts, E. F., and Jones Jr, J. B. (2009). Impact of wildfire on stream nutrient chemistry and ecosystem metabolism in boreal forest catchments of interior alaska. Arct Antarct Alp Res. 41, 407–417. doi: 10.1657/1938-4246-41.4.407
Beven, K. J., and Kirkby, M. J. (1979). A physically based, variable contributing area model of basin hydrology. Hydrol. Sci. Bull. 24, 43–69. doi: 10.1080/02626667909491834
Bligh, E. G., and Dyer, W. J. (1959). A rapid method of total lipid extraction and purification. Can. J. Biochem. Physiol. 37, 911–917. doi: 10.1139/y59-099
Boby, L. A., Schuur, E. A. G., Mack, M. C., Verbyla, D., and Johnstone, J. F. (2010). Quantifying fire severity, carbon, and nitrogen emissions in Alaska's boreal forest. Ecol. Appl. 20, 1633–1647. doi: 10.1890/08-2295.1
Bond-Lamberty, B., Peckham, S. D., Gower, S. T., and Ewers, B. E. (2009). Effects of fire on regional evapotranspiration in the central canadian boreal forest. Glob. Chang. Biol. 15, 1242–1254. doi: 10.1111/j.1365-2486.2008.01776.x
Bradshaw, C. J., and Warkentin, I. G. (2015). Global estimates of boreal forest carbon stocks and flux. Glob. Planet. Change 128, 24–30. doi: 10.1016/j.gloplacha.2015.02.004
Brovkin, V., van Bodegom, P. M., Kleinen, T., Wirth, C., Cornwell, W. K., Cornelissen, J. H. C., et al. (2012). Plant-driven variation in decomposition rates improves projections of global litter stock distribution. Biogeosciences 9, 565–576. doi: 10.5194/bg-9-565-2012
Buchanan, B. P., Fleming, M., Schneider, R. L., Richards, B. K., Archibald, J., Qiu, Z., et al. (2014). Evaluating topographic wetness indices across central new york agricultural landscapes. Hydrol. Earth Syst. Sci. 18, 3279–3299. doi: 10.5194/hess-18-3279-2014
Callesen, I., Raulund-Rasmussen, K., Westman, C., and Tau-Strand, L. (2007). Nitrogen pools and c:n ratios in well-drained nordic forest soils related to climate and soil texture. Boreal Environ. Res. 12, 681–692.
Canadian Agricultural Services Coordinating Committee (1998). The Canadian System of Soil Classification. Ottawa, ON: NRC Research Press.
Certini, G. (2005). Effects of fire on properties of forest soils: a review. Oecologia 143, 1–10. doi: 10.1007/s00442-004-1788-8
Chambers, S. D., Beringer, J., Randerson, J. T., and Chapin, III. F. S. (2005). Fire effects on net radiation and energy partitioning: contrasting responses of tundra and boreal forest ecosystems. J. Geophys. Res. Atmosph. 110, D9. doi: 10.1029/2004JD005299
Charette, T., and Prepas, E. E. (2003). Wildfire impacts on phytoplankton communities of three small lakes on the boreal plain, alberta, canada: a paleolimnological study. Can. J. Fish. Aquatic Sci. 60, 584–593. doi: 10.1139/f03-049
Chen, R., Senbayram, M., Blagodatsky, S., Myachina, O., Dittert, K., Lin, X., et al. (2014). Soil c and n availability determine the priming effect: microbial n mining and stoichiometric decomposition theories. Glob. Chang. Biol. 20, 2356–2367. doi: 10.1111/gcb.12475
Clemmensen, K. E., Bahr, A., Ovaskainen, O., Dahlberg, A., Ekblad, A., Wallander, H., et al. (2013). Roots and associated fungi drive long-term carbon sequestration in boreal forest. Science 339, 1615–1618. doi: 10.1126/science.1231923
Clemmensen, K. E., Finlay, R. D., Dahlberg, A., Stenlid, J., Wardle, D. A., and Lindahl, B. D. (2015). Carbon sequestration is related to mycorrhizal fungal community shifts during long-term succession in boreal forests. New Phytol. 205, 1525–1536. doi: 10.1111/nph.13208
Coppola, A. I., Wagner, S., Lennartz, S. T., Seidel, M., Ward, N. D., Dittmar, T., et al. (2022). The black carbon cycle and its role in the earth system. Nat. Rev. Earth Environ. 3, 516–532. doi: 10.1038/s43017-022-00316-6
Currie, D. J. (2019). Where newton might have taken ecology. Glob. Ecol. Biogeogr. 28, 18–27. doi: 10.1111/geb.12842
Day, N. J., Dunfield, K. E., Johnstone, J. F., Mack, M. C., Turetsky, M. R., Walker, X. J., et al. (2019). Wildfire severity reduces richness and alters composition of soil fungal communities in boreal forests of western canada. Glob. Chang. Biol. 25, 2310–2324. doi: 10.1111/gcb.14641
De Frenne, P., Graae, B. J., Rodríguez-Sánchez, F., Kolb, A., Chabrerie, O., Decocq, G., et al. (2013). Latitudinal gradients as natural laboratories to infer species' responses to temperature. J. Ecol. 101, 784–795. doi: 10.1111/1365-2745.12074
Deluca, T. H., and Boisvenue, C. (2012). Boreal forest soil carbon: distribution, function and modelling. Forestry 85, 161–184. doi: 10.1093/forestry/cps003
Dunne, J. A., Saleska, S. R., Fischer, M. L., and Harte, J. (2004). Integrating experimental and gradient methods in ecological climate change research. Ecology 85, 904–916. doi: 10.1890/03-8003
Dymov, A., Startsev, V., Milanovsky, E., Valdes-Korovkin, I., Farkhodov, Y., Yudina, A., et al. (2021). Soils and soil organic matter transformations during the two years after a low-intensity surface fire (subpolar ural, russia). Geoderma 404, 115278. doi: 10.1016/j.geoderma.2021.115278
Eckdahl, J. A., Kristensen, J. A., and Metcalfe, D. B. (2022a). Climatic variation drives loss and restructuring of carbon and nitrogen in boreal forest wildfire. Biogeosciences 19, 2487–2506. doi: 10.5194/bg-19-2487-2022
Eckdahl, J. A., Rodriguez, P. C., Kristensen, J. A., Metcalfe, D. B., and Ljung, K. (2022b). Mineral soils are an important intermediate storage pool of black carbon in fennoscandian boreal forests. Glob. Biogeochem. Cycles 36, e2022GB007489. doi: 10.1029/2022GB007489
Fanin, N., Kardol, P., Farrell, M., Nilsson, M.-C., Gundale, M. J., and Wardle, D. A. (2019). The ratio of gram-positive to gram-negative bacterial plfa markers as an indicator of carbon availability in organic soils. Soil Biol. Biochem. 128, 111–114. doi: 10.1016/j.soilbio.2018.10.010
Frey, S. D. (2019). Mycorrhizal fungi as mediators of soil organic matter dynamics. Annu. Rev. Ecol. Evol. Syst. 50, 237–259. doi: 10.1146/annurev-ecolsys-110617-062331
Friedlingstein, P., O'sullivan, M., Jones, M. W., Andrew, R. M., Hauck, J., Olsen, A., et al. (2020). Global carbon budget 2020. Earth Syst. Sci. Data 12, 3269–3340. doi: 10.5194/essd-12-3269-2020
Frostegård, Å., Tunlid, A., and Bååth, E. (2011). Use and misuse of plfa measurements in soils. Soil Biol. Biochem. 43, 1621–1625. doi: 10.1016/j.soilbio.2010.11.021
Fukami, T., and Wardle, D. A. (2005). Long-term ecological dynamics: reciprocal insights from natural and anthropogenic gradients. Proc. R. Soc. B Biol. Sci. 272, 2105–2115. doi: 10.1098/rspb.2005.3277
Goldblum, D., and Rigg, L. S. (2010). The deciduous forest - boreal forest ecotone. Geography Compass 4, 701–717. doi: 10.1111/j.1749-8198.2010.00342.x
Granath, G., Evans, C. D., Strengbom, J., Fölster, J., Grelle, A., Strömqvist, J., et al. (2021). The impact of wildfire on biogeochemical fluxes and water quality in boreal catchments. Biogeosciences 18, 3243–3261. doi: 10.5194/bg-18-3243-2021
Gustafsson, L., Berglind, M., Granström, A., Grelle, A., Isacsson, G., Kjellander, P., et al. (2019). Rapid ecological response and intensified knowledge accumulation following a north european mega-fire. Scand. J. Forest Res. 34, 234–253. doi: 10.1080/02827581.2019.1603323
Haesen, S., Lembrechts, J. J., De Frenne, P., Lenoir, J., Aalto, J., Ashcroft, M. B., et al. (2021). Foresttemp - sub-canopy microclimate temperatures of european forests. Glob. Chang. Biol. 27, 6307–6319. doi: 10.1111/gcb.15892
Hanan, E., Kennedy, M. C., Ren, J., Johnson, M. C., and Smith, A. M. S. (2021). Missing climate feedbacks in fire models: limitations and uncertainties in fuel loadings and the role of decomposition in fine fuel succession. Earth Space Sci. Open Arch, 14, e2021MS002818. doi: 10.1002/essoar.10508012.1
Hensgens, G., Laudon, H., Peichl, M., Gil, I. A., Zhou, Q., and Berggren, M. (2020). The role of the understory in litter doc and nutrient leaching in boreal forests. Biogeochemistry 149, 87–103. doi: 10.1007/s10533-020-00668-5
Hicks, L. C., Lajtha, K., and Rousk, J. (2021). Nutrient limitation may induce microbial mining for resources from persistent soil organic matter. Ecology 102, e03328. doi: 10.1002/ecy.3328
Hicks, L. C., Yuan, M., Brangarí, A., Rousk, K., and Rousk, J. (2022). Increased above-and belowground plant input can both trigger microbial nitrogen mining in subarctic tundra soils. Ecosystems 25, 105–121. doi: 10.1007/s10021-021-00642-8
Hobbie, J., and Hobbie, E. (2013). Microbes in nature are limited by carbon and energy: the starving-survival lifestyle in soil and consequences for estimating microbial rates. Front. Microbiol. 4, 324. doi: 10.3389/fmicb.2013.00324
Högberg, M. N., Briones, M. J. I., Keel, S. G., Metcalfe, D. B., Campbell, C., Midwood, A. J., et al. (2010). Quantification of effects of season and nitrogen supply on tree below-ground carbon transfer to ectomycorrhizal fungi and other soil organisms in a boreal pine forest. New Phytol. 187, 485–493. doi: 10.1111/j.1469-8137.2010.03274.x
Högberg, P., Näsholm, T., Franklin, O., and Högberg, M. N. (2017). Tamm review: On the nature of the nitrogen limitation to plant growth in fennoscandian boreal forests. For. Ecol Manag. 403, 161–185. doi: 10.1016/j.foreco.2017.04.045
Holden, S. R., Rogers, B. M., Treseder, K. K., and Randerson, J. T. (2016). Fire severity influences the response of soil microbes to a boreal forest fire. Environ. Res. Lett. 11, 035004. doi: 10.1088/1748-9326/11/3/035004
Houle, D., and Carignan, R. (1992). Sulfur speciation and distribution in soils and aboveground biomass of a boreal coniferous forest. Biogeochemistry 16, 63–82. doi: 10.1007/BF02402263
Ibáñez, T., Rütting, T., Nilsson, M.-C., Wardle, D., and Gundale, M. (2022). Mid-term effects of wildfire and salvage logging on gross and net soil nitrogen transformation rates in a swedish boreal forest. For. Ecol. Manag. 517, 120240. doi: 10.1016/j.foreco.2022.120240
Joergensen, R. G. (2022). Phospholipid fatty acids in soil–drawbacks and future prospects. Biol. Fertil. Soils 58, 1–6. doi: 10.1007/s00374-021-01613-w
Johnstone, J., Celis, G., Chapin, I. I. I. F, Hollingsworth, T., Jean, M., et al. (2020). Factors shaping alternate successional trajectories in burned black spruce forests of alaska. Ecosphere 11, e03129. doi: 10.1002/ecs2.3129
Jones, M., Santín, C., Werf, G., and Doerr, S. (2019). Global fire emissions buffered by the production of pyrogenic carbon. Nat. Geosci. 12, 742–747. doi: 10.1038/s41561-019-0403-x
Jones, M. W., Abatzoglou, J. T., Veraverbeke, S., Andela, N., Lasslop, G., Forkel, M., et al. (2022). Global and regional trends and drivers of fire under climate change. Rev. Geophys. 60, e2020RG000726. doi: 10.1029/2020RG000726
Kardol, P., and Wardle, D. A. (2010). How understanding aboveground-belowground linkages can assist restoration ecology. Trends Ecol. Evol. 25, 670–679. doi: 10.1016/j.tree.2010.09.001
Köster, K., Aaltonen, H., Berninger, F., Heinonsalo, J., Köster, E., Ribeiro-Kumara, C., et al. (2021). Impacts of wildfire on soil microbiome in boreal environments. Curr. Opin. Environ. Sci. Health 22, 100258. doi: 10.1016/j.coesh.2021.100258
Kristensen, T., Ohlson, M., Bolstad, P., and Nagy, Z. (2015). Spatial variability of organic layer thickness and carbon stocks in mature boreal forest stands–implications and suggestions for sampling designs. Environ. Monit. Assess. 187, 1–19. doi: 10.1007/s10661-015-4741-x
Lacombe-Harvey, M. -,È., Brzezinski, R., and Beaulieu, C. (2018). Chitinolytic functions in actinobacteria: ecology, enzymes, and evolution. Appl. Microbiol. Biotechnol. 102, 7219–7230. doi: 10.1007/s00253-018-9149-4
Lagerström, A., Esberg, C., Wardle, D. A., and Giesler, R. (2009). Soil phosphorus and microbial response to a long-term wildfire chronosequence in northern sweden. Biogeochemistry 95, 199–213. doi: 10.1007/s10533-009-9331-y
Lantmäteriet (2021). Markhöjdmodell nedladdning, grid 50+. Available online at: https://www.lantmateriet.se/sv/Kartor-och-geografisk-information/geodataprodukter/produktlista/markhojdmodell-nedladdning-grid-50/#steg=1
Lembrechts, J. J., van den Hoogen, J., Aalto, J., Ashcroft, M. B., De Frenne, P., Kemppinen, J., et al. (2022). Global maps of soil temperature. Glob. Chang. Biol. 28, 3110–3144. doi: 10.1111/gcb.16060
Ludwig, S. M., Alexander, H. D., Kielland, K., Mann, P. J., Natali, S. M., and Ruess, R. W. (2018). Fire severity effects on soil carbon and nutrients and microbial processes in a siberian larch forest. Glob. Chang. Biol. 24, 5841–5852. doi: 10.1111/gcb.14455
Maaroufi, N. I., Nordin, A., Palmqvist, K., Hasselquist, N. J., Forsmark, B., Rosenstock, N. P., et al. (2019). Anthropogenic nitrogen enrichment enhances soil carbon accumulation by impacting saprotrophs rather than ectomycorrhizal fungal activity. Glob. Chang. Biol. 25, 2900–2914. doi: 10.1111/gcb.14722
Makoto, K., and Koike, T. (2021). Charcoal ecology: Its function as a hub for plant succession and soil nutrient cycling in boreal forests. Ecol. Res. 36, 4–12. doi: 10.1111/1440-1703.12179
Makoto, K., Shibata, H., Kim, Y., Satomura, T., Takagi, K., Nomura, M., et al. (2012). Contribution of charcoal to short-term nutrient dynamics after surface fire in the humus layer of a dwarf bamboo-dominated forest. Biol. Fertil Soils 48, 569–577. doi: 10.1007/s00374-011-0657-y
Malhi, Y., Baldocchi, D., and Jarvis, P. (1999). The carbon balance of tropical, temperate and boreal forests. Plant Cell Environ. 22, 715–740. doi: 10.1046/j.1365-3040.1999.00453.x
Malik, A. A., Chowdhury, S., Schlager, V., Oliver, A., Puissant, J., Vazquez, P. G. M., et al. (2016). Soil fungal:bacterial ratios are linked to altered carbon cycling. Front. Microbiol. 7, 1247. doi: 10.3389/fmicb.2016.01247
McLauchlan, K. K., Higuera, P. E., Miesel, J., Rogers, B. M., Schweitzer, J., Shuman, J. K., et al. (2020). Fire as a fundamental ecological process: research advances and frontiers. J. Ecol. 108, 2047–2069. doi: 10.1111/1365-2745.13403
Meier, I. C., Tückmantel, T., Heitkötter, J., Müller, K., Preusser, S., Wrobel, T. J., et al. (2020). Root exudation of mature beech forests across a nutrient availability gradient: the role of root morphology and fungal activity. New Phytol. 226, 583–594. doi: 10.1111/nph.16389
Mekonnen, Z. A., Riley, W. J., Randerson, J. T., Grant, R. F., and Rogers, B. M. (2019). Expansion of high-latitude deciduous forests driven by interactions between climate warming and fire. Nat. Plants 5, 952–958. doi: 10.1038/s41477-019-0495-8
Mercer, D. K., Iqbal, M., Miller, P., and McCarthy, A. J. (1996). Screening actinomycetes for extracellular peroxidase activity. Appl. Environ. Microbiol. 62, 2186–2190. doi: 10.1128/aem.62.6.2186-2190.1996
Miyanishi, K., and Johnson, E. A. (2002). Process and patterns of duff consumption in the mixedwood boreal forest. Can. J. Forest Res. 32, 1285–1295. doi: 10.1139/x02-051
Murphy, P. N., Ogilvie, J., Connor, K., and Arp, P. A. (2007). Mapping wetlands: a comparison of two different approaches for new brunswick, canada. Wetlands 27, 846–854. doi: 10.1672/0277-5212(2007)27846:MWACOT2.0.CO;2
Naturvårdsverket (2018). Markfuktighetsindex Producerat Som del av Nationella Marktäckedata, nmd. Available online at: https://metadatakatalogen.naturvardsverket.se/metadatakatalogen/GetMetaDataById?id=cae71f45-b463-447f-804f-2847869b19b0
Neff, J., Harden, J., and Gleixner, G. (2005). Fire effects on soil organic matter content, composition, and nutrients in boreal interior alaska. Can. J. For. Res. 35, 2178–2187. doi: 10.1139/x05-154
Nelson, A. R., Narrowe, A. B., Rhoades, C. C., Fegel, T. S., Daly, R. A., Roth, H. K., et al. (2022). Wildfire-dependent changes in soil microbiome diversity and function. Nat. Microbiol. 7, 1419–1430. doi: 10.1038/s41564-022-01203-y
Nilsson, M.-C., and Wardle, D. A. (2005). Understory vegetation as a forest ecosystem driver: evidence from the northern swedish boreal forest. Front. Ecol. Environ. 3, 421–428. doi: 10.1890/1540-9295(2005)0030421:UVAAFE2.0.CO;2
Oksanen, J., Simpson, G. L., Blanchet, F. G., Kindt, R., Legendre, P., Minchin, P. R., et al. (2022). vegan: Community Ecology Package. R package version 2.6–4.
Pellegrini, A. F., Harden, J., Georgiou, K., Hemes, K. S., Malhotra, A., Nolan, C. J., et al. (2021). Fire effects on the persistence of soil organic matter and long-term carbon storage. Nat. Geosci. 15, 5–13. doi: 10.1038/s41561-021-00867-1
Peltzer, D. A., Wardle, D. A., Allison, V. J., Baisden, W. T., Bardgett, R. D., Chadwick, O. A., et al. (2010). Understanding ecosystem retrogression. Ecol. Monog. 80, 509–529. doi: 10.1890/09-1552.1
Pérez-Izquierdo, L., Clemmensen, K. E., Strengbom, J., Granath, G., Wardle, D. A., Nilsson, M.-C., et al. (2021). Crown-fire severity is more important than ground-fire severity in determining soil fungal community development in the boreal forest. J. Ecol. 109, 504–518. doi: 10.1111/1365-2745.13529
Pietikäinen, J., Pettersson, M., and Bååth, E. (2005). Comparison of temperature effects on soil respiration and bacterial and fungal growth rates. FEMS Microbiol. Ecol. 52, 49–58. doi: 10.1016/j.femsec.2004.10.002
Pimentel, R., and Arheimer, B. (2021). Hydrological impacts of a wildfire in a boreal region: the västmanland fire 2014 (sweden). Sci. Total Environ. 756, 143519. doi: 10.1016/j.scitotenv.2020.143519
Rabin, S. S., Melton, J. R., Lasslop, G., Bachelet, D., Forrest, M., Hantson, S., et al. (2017). The fire modeling intercomparison project (firemip), phase 1: experimental and analytical protocols with detailed model descriptions. Geosci. Model Dev. 10, 1175–1197. doi: 10.5194/gmd-10-1175-2017
Rapalee, G., Trumbore, S. E., Davidson, E. A., Harden, J. W., and Veldhuis, H. (1998). Soil carbon stocks and their rates of accumulation and loss in a boreal forest landscape. Glob. Biogeochem. Cycles 12, 687–701. doi: 10.1029/98GB02336
Rousk, J., and Bååth, E. (2011). Growth of saprotrophic fungi and bacteria in soil. FEMS Microbiol. Ecol. 78, 17–30. doi: 10.1111/j.1574-6941.2011.01106.x
Rousk, J., Brookes, P. C., and Bååth, E. (2009). Contrasting soil ph effects on fungal and bacterial growth suggest functional redundancy in carbon mineralization. Appl. Environ. Microbiol. 75, 1589–1596. doi: 10.1128/AEM.02775-08
Rousk, J., and Frey, S. D. (2015). Revisiting the hypothesis that fungal-to-bacterial dominance characterizes turnover of soil organic matter and nutrients. Ecol. Monog. 85, 457–472. doi: 10.1890/14-1796.1
Santín, C., Doerr, S. H., Merino, A., Bryant, R., and Loader, N. J. (2016). Forest floor chemical transformations in a boreal forest fire and their correlations with temperature and heating duration. Geoderma 264, 71–80. doi: 10.1016/j.geoderma.2015.09.021
Schimel, J. P., and Bennett, J. (2004). Nitrogen mineralization: Challenges of a changing paradigm. Ecology 85, 591–602. doi: 10.1890/03-8002
Scholten, R. C., Jandt, R., Miller, E. A., Rogers, B. M., and Veraverbeke, S. (2021). Overwintering fires in boreal forests. Nature 593, 399–404. doi: 10.1038/s41586-021-03437-y
Seabold, S., and Perktold, J. (2010). “Statsmodels: econometric and statistical modeling with python,” in 9th Python in Science Conference Austin, TX.
Shenoy, A., Kielland, K., and Johnstone, J. F. (2013). Effects of fire severity on plant nutrient uptake reinforce alternate pathways of succession in boreal forests. Plant Ecol. 214, 587–596. doi: 10.1007/s11258-013-0191-0
Sidoroff, K., Kuuluvainen, T., Tanskanen, H., and Vanha-Majamaa, I. (2007). Tree mortality after low-intensity prescribed fires in managed pinus sylvestris stands in southern finland. Scand. J. For. Res. 22, 2–12. doi: 10.1080/02827580500365935
Smithwick, E. A., Turner, M. G., Mack, M. C., and Chapin, F. S. (2005). Postfire soil n cycling in northern conifer forests affected by severe, stand-replacing wildfires. Ecosystems 8, 163–181. doi: 10.1007/s10021-004-0097-8
Soares, M., and Rousk, J. (2019). Microbial growth and carbon use efficiency in soil: links to fungal-bacterial dominance, soc-quality and stoichiometry. Soil Biol. Biochem. 131, 195–205. doi: 10.1016/j.soilbio.2019.01.010
Sun, H., Santalahti, M., Pumpanen, J., Köster, K., Berninger, F., Raffaello, T., et al. (2015). Fungal community shifts in structure and function across a boreal forest fire chronosequence. Appl. Environ. Microbiol. 81, 7869–7880. doi: 10.1128/AEM.02063-15
Sun, H., Santalahti, M., Pumpanen, J., Köster, K., Berninger, F., Raffaello, T., et al. (2016). Bacterial community structure and function shift across a northern boreal forest fire chronosequence. Sci. Rep. 6, 1–12. doi: 10.1038/srep32411
Treseder, K. K., Mack, M. C., and Cross, A. (2004). Relationships among fires, fungi, and soil dynamics in alaskan boreal forests. Ecol. Appl. 14, 1826–1838. doi: 10.1890/03-5133
Vega, J. A., Fontúrbel, T., Merino, A., Fernández, C., Ferreiro, A., and Jiménez, E. (2013). Testing the ability of visual indicators of soil burn severity to reflect changes in soil chemical and microbial properties in pine forests and shrubland. Plant Soil. 369, 73–91. doi: 10.1007/s11104-012-1532-9
Virtanen, P., Gommers, R., Oliphant, T. E., Haberland, M., Reddy, T., Cournapeau, D., et al. (2020). SciPy 1.0: fundamental algorithms for scientific computing in python. Nat. Methods 17, 261–272. doi: 10.1038/s41592-020-0772-5
Walker, X. J., Rogers, B. M., Baltzer, J. L., Cumming, S. G., Day, N. J., Goetz, S. J., et al. (2018). Cross-scale controls on carbon emissions from boreal forest megafires. Glob. Chang. Biol. 24, 4251–4265. doi: 10.1111/gcb.14287
Wan, S., Hui, D., and Luo, Y. (2001). Fire effects on nitrogen pools and dynamics in terrestrial ecosystems: a meta-analysis. Ecol. Appl. 11, 1349–1365. doi: 10.1890/1051-0761(2001)0111349:FEONPA2.0.CO;2
Wardle, D. A., Walker, L. R., and Bardgett, R. D. (2004). Ecosystem properties and forest decline in contrasting long-term chronosequences. Science 305, 509–513. doi: 10.1126/science.1098778
Watts, A. C., and Kobziar, L. N. (2013). Smoldering combustion and ground fires: ecological effects and multi-scale significance. Fire Ecol. 9, 124–132. doi: 10.4996/fireecology.0901124
White, D., Davis, W., Nickels, J., King, J., and Bobbie, R. (1979). Determination of the sedimentary microbial biomass by extractable lipid phosphate. Oecologia 40, 51–62. doi: 10.1007/BF00388810
Whitman, T., Whitman, E., Woolet, J., Flannigan, M. D., Thompson, D. K., and Parisien, M.-A. (2019). Soil bacterial and fungal response to wildfires in the canadian boreal forest across a burn severity gradient. Soil Biol. Biochem. 138, 107571. doi: 10.1016/j.soilbio.2019.107571
Wiggins, E. B., Andrews, A., Sweeney, C., Miller, J. B., Miller, C. E., Veraverbeke, S., et al. (2020). Evidence for a larger contribution of smoldering combustion to boreal forest fire emissions from tower observations in alaska. Atmosph. Chem. Phys. Discuss. 2020, 1–26. doi: 10.5194/acp-2019-1067
Willers, C., Jansen van Rensburg, P., and Claassens, S. (2015). Phospholipid fatty acid profiling of microbial communities-a review of interpretations and recent applications. J. Appl. Microbiol. 119, 1207–1218. doi: 10.1111/jam.12902
Zak, D. R., Pregitzer, K. S., Burton, A. J., Edwards, I. P., and Kellner, H. (2011). Microbial responses to a changing environment: implications for the future functioning of terrestrial ecosystems. Fungal Ecol. 4, 386–395. doi: 10.1016/j.funeco.2011.04.001
Zelles, L. (1999). Fatty acid patterns of phospholipids and lipopolysaccharides in the characterisation of microbial communities in soil: a review. Biol. Fertil Soils 29, 111–129. doi: 10.1007/s003740050533
Keywords: boreal forest wildfire, climate change, microbial community, nitrogen, nutrient cycling, smoldering combustion, Sweden, vegetation
Citation: Eckdahl JA, Kristensen JA and Metcalfe DB (2023) Climate and forest properties explain wildfire impact on microbial community and nutrient mobilization in boreal soil. Front. For. Glob. Change 6:1136354. doi: 10.3389/ffgc.2023.1136354
Received: 03 January 2023; Accepted: 27 February 2023;
Published: 31 March 2023.
Edited by:
Nicasio T. Jiménez-Morillo, University of Evora, PortugalReviewed by:
Barbara Kitzler, Austrian Research Centre for Forests (BFW), AustriaMarco Antonio Jiménez-González, Autonomous University of Madrid, Spain
Copyright © 2023 Eckdahl, Kristensen and Metcalfe. This is an open-access article distributed under the terms of the Creative Commons Attribution License (CC BY). The use, distribution or reproduction in other forums is permitted, provided the original author(s) and the copyright owner(s) are credited and that the original publication in this journal is cited, in accordance with accepted academic practice. No use, distribution or reproduction is permitted which does not comply with these terms.
*Correspondence: Johan A. Eckdahl, am9oYW4uZWNrZGFobCYjeDAwMDQwO25hdGVrby5sdS5zZQ==