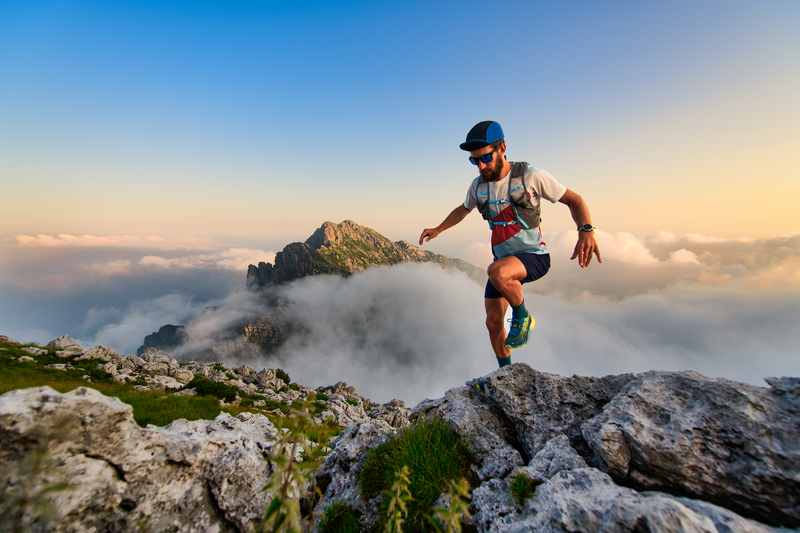
95% of researchers rate our articles as excellent or good
Learn more about the work of our research integrity team to safeguard the quality of each article we publish.
Find out more
REVIEW article
Front. For. Glob. Change , 21 April 2023
Sec. Forest Disturbance
Volume 6 - 2023 | https://doi.org/10.3389/ffgc.2023.1110431
This article is part of the Research Topic Impacts of Climate Change on Long Term Viability of Trees Species with Recalcitrant Seeds View all 5 articles
Recalcitrant seeds are characterized by desiccation and freezing sensitivity, and short storage longevity. These physiological attributes obviate their ex situ conservation in conventional seed banks, where seeds are stored dry at sub-zero temperatures (typically, 15% relative humidity and –20°C) for extended periods of time. Propagation of plants for field collections (e.g., botanical gardens, nurseries, and arboretums) is a valuable ex situ conservation option. However, these collections are relatively costly, require high maintenance, preserve limited genetic diversity and/or are directly exposed to biotic (e.g., pests) and abiotic (e.g., climatic) threats. Therefore, recalcitrant-seeded (RS) species are dependent on cryopreservation for their safe and long-term ex situ conservation. Different explant sources such as whole seeds, zygotic embryos, dormant buds, shoot tips, and pollen, can be used for plant propagation of RS species in field collections as well as for their cryopreservation. The success of the propagation or the cryopreservation of these explants often depends on their developmental status, vigor, and/or tolerance to desiccation and chilling/freezing. These attributes are modulated by the environment where the donor plant grows and we hypothesize that climate change, by affecting these biological attributes, would impact the success of explant propagation and cryopreservation. To support this hypothesis, we have reviewed how temperature changes and drought, the two main climate change scenarios, affect the main biological attributes that are directly involved in the success of ex situ conservation of tropical and temperate RS species. In general, increases in temperature and drought will negatively affect plant development in field collections and the quality of the explants used in cryopreservation. Consequently, field collections of RS species may need to be moved to more suitable places (e.g., higher latitudes/altitudes). Additionally, we may find a reduction in the success of cryopreservation of RS species germplasm directly harvested from field collections. However, we cannot always generalize these effects for all species since they often depend on the origin of the species (e.g., tropical and temperate species tend to respond to climate change differently), the genotype, the adaptive genetic potential of each population, and the severity of the environmental change. On the other hand, the increase in temperatures and water stress in donor plants at high-latitude areas and also some tropical environments may favor the production of seeds and seedlings better adapted to drying, and hence, increase the success of plant propagation and zygotic embryo cryopreservation.
The ex situ conservation of plant genetic diversity is mainly achieved by the storage of seeds in conventional seed banks, where seeds are stored dry at sub-zero temperatures (e.g., ∼15% relative humidity and –20°C) for decades (FAO, 2014; Breman et al., 2021; Walters and Pence, 2021). Despite the low number of species that have been characterized in terms of seed storage behavior to date [<5% of seed-bearing plant species; 18,147 species (Wyse and Dickie, 2017) over 376,366 species of the world vascular flora (Qian et al., 2022)], nearly 92% of seeded plants globally are predicted to be tolerant to desiccation (Wyse and Dickie, 2017). This explains why conventional seed banking is a widespread methodology, with potentially over 2,100 seed banks established globally (Breman et al., 2021). However, conventional seed banking is not possible for recalcitrant seeds (Roberts, 1973), as they are characterized by physiological attributes that obviate their conventional storage in seed banks (Walters et al., 2013; Pence et al., 2020). In a broad sense, recalcitrant seeds are sensitive to drying below a certain threshold (ca. 0.2–0.3 g H2O/g DW or relative humidity <90%; FAO, 2014; Walters, 2015). If stored at –20°C at such high moisture contents, lethal intracellular ice crystals are formed (FAO, 2014; Walters, 2015). In addition, recalcitrant seeds tend to be chilling sensitive and lose viability relatively fast (days to months) when stored hydrated (FAO, 2014). Other “non-orthodox” species with “intermediate” storage behaviors in terms of drying and freezing sensitivity, or very short longevity (e.g., Coffea, Citrus, etc.) also present challenges during their conventional storage in seed banks (Walters et al., 2013; Pence et al., 2020) and are collectively considered as exceptional plant species (Pence et al., 2022).
To overcome the limitation imposed by seed recalcitrance and other non-orthodox storage behaviors, ex situ conservation has mainly been achieved through the propagation and cultivation of a small number of plants in field collections in botanical gardens, nurseries, arboretums, or GenBank field collections (Engelmann and Engels, 2002; Breman et al., 2021). Here, the correct propagation and horticultural management of plants are indispensable (Rae, 2011; Cibrian-Jaramillo et al., 2013; Ensslin and Godefroid, 2019). However, this is a relatively costly option that requires high maintenance and a considerable amount of space, where minimal genetic diversity is preserved and is directly exposed to biotic (e.g., pests) and abiotic (e.g., climatic) threats (Li and Pritchard, 2009; Pence, 2011). In addition to field collections, other valuable resources for the ex situ conservation of RS species are in vitro collections. Here, whole plants or explants, represented as shoot tips or embryonic lines, are cultured under aseptic conditions and preserved under slow (minimal) growth conditions, with sub-culturing when needed (Cha-um and Kirdmanee, 2007). While this option is still costly and requires high maintenance, the genetic diversity conserved in terms of the number of genotypes per species can be increased compared with field collections (Pence, 2011). Nonetheless, in this option, the preserved species and genotypes are potentially exposed to somaclonal variation that could affect the genetic identity of the species/genotypes preserved. To overcome the limits of field and in vitro collections in terms of costs and long-term maintenance, cryopreservation of diverse plant explants is proposed for the safe and long-term ex situ conservation of RS species, especially those that are endangered (Pence, 2011; Walters et al., 2013; Pence et al., 2020; Breman et al., 2021; Philpott et al., 2022).
The main effects of climate change on plants have been extensively investigated and reviewed (e.g., Parmesan and Hanley, 2015, and articles within the same special issue on “Plants and Climate Change”; Fahad et al., 2021; Baskin and Baskin, 2022), and include exposure to increased temperatures, increased drought, catastrophic climate events, and/or increased sea levels. The average global temperature rise is expected to reach or exceed 1.5°C within the next few decades, affecting all regions of Earth (IPCC, 2021). The effects of climate change on RS species have often been studied from an ecological perspective (Ramírez-Valiente et al., 2009; Joët et al., 2013; Amimi et al., 2020; Badano and Sánchez-Montes de Oca, 2022; Magni et al., 2022) and there are recent reports on how climate change may affect their propagation from seeds as well (Pritchard et al., 2022). However, the effect of climate change on the available ex situ conservation options for RS species has been largely overlooked.
Sexual, vegetative and in vitro propagation of RS species for field and in vitro collections, as well as for cryopreservation, can be achieved using different explants such as zygotic embryos, embryonic axes, dormant buds, shoot tips, somatic embryos, embryogenic cell lines, undifferentiated calli, and pollen (Reed, 2008; Engelmann, 2011; Pence et al., 2020). The success of these propagation options and the cryopreservation of plant explants, often depends on their developmental status (Goveia et al., 2004), vigor, and/or tolerance to desiccation (Pammenter and Berjak, 2014), and chilling/freezing (Popova et al., 2012; Xia et al., 2014; Ballesteros and Pence, 2017; Bharuth and Naidoo, 2020). These attributes are typically modulated by the environment where the donor plants grow (Gutterman, 2000). We hypothesize that climate change, by affecting these biological attributes, will impact the success of explant cryopreservation limiting the success of ex situ conservation methods such as cryopreservation for RS species.
To support this hypothesis, we have reviewed and discussed the current knowledge on how the expected climatic drivers induced by climate change (mainly changes in temperature and rainfall) can impact the successful propagation-based ex situ conservation of terrestrial tropical and temperate RS species by modulating the main biological attributes of propagules. Two case studies on plants of significant conservation interest, namely, Cycads (tropical) and Quercus spp. (temperate) are included to illustrate these findings. Finally, a set of recommendations to guide future strategies for the ex situ conservation of RS species in the face of climate change are provided.
Plants propagated in botanical gardens, arboretums, field GeneBanks, or plant nurseries represent a valuable option for ex situ conservation of RS species (Li and Pritchard, 2009; Primack and Miller-Rushing, 2009; Breman et al., 2021; Primack et al., 2021). However, climate change projections introduce large uncertainties around the sustainable use of field collections due to the increase in the frequency of extreme climate events (flooding, late frosts, intensive summer droughts, heat waves, and amongst other events). Severe weather events in the past decade have already impacted global coconut (the RS Cocos nucifera) production, with crop changes to different genotypes and a shift in cultivation areas predicted for India, the world’s third biggest producer of coconut (Hebbar et al., 2022). A shift in production regions is also predicted for cacao (Theobroma indica), another RS species, in West Africa and Colombia (Schroth et al., 2016; González-Orozco et al., 2022). Similarly, Asare-Nuamah et al. (2022) showed that climate events resulted in stunted growth, poor germination, and increased attack by pests and diseases on Mango (Mangifera indica) in Ghana. Extreme climate events can affect germination strategies, initial seedling establishment, mature plant growth rate (Primack and Miller-Rushing, 2009; Pritchard et al., 2022), and/or the timing of key phenological processes for the development of fruits and seeds (Santos et al., 2017; Ma et al., 2022). For example, in both temperate and tropical tree species, early flowering induced by warmer winters may result in low fruit set due to the lack of pollinators or the negative effect of low night temperatures on fruit development (Dinesh and Reddy, 2012; Ma et al., 2022). On the other hand, rising temperatures also appear to reduce fruit set by inducing flower drops, affecting the synchronization of flowering or inducing sex changes in hermaphrodite and male plants, promoting stigma and stamen sterility, or reducing the functionality of pollen grains and causing poor pollinator activity (Dinesh and Reddy, 2012; Nepi et al., 2001). All these alterations in the reproductive biology of RS species could ultimately limit their conservation value (Lobdell and Thompson, 2017).
From a perspective of water-stress physiology, some RS species like Quercus ilex and Q. faginea are anisohydric, displaying less strict stomatal control and more negative water potential during drought and heat waves. It has been hypothesized that these plants would be more likely to suffer extensive embolism and, ultimately, hydraulic failure during an intense drought (McDowell et al., 2008; Resco de Dios et al., 2020). Therefore, the geographical location of field collections housing RS species should consider the potential impact of climate change, especially in terms of the increased frequency of heatwaves and hot droughts (Williams and Dumroese, 2013).
For short-term (from months up to 2 years) applications such as plant production for ecological restoration or distribution, technological solutions can be used to overcome the impacts of climate change. For example, a slow-growth method in a nursery where seedlings are maintained at 16°C, 90% relative humidity and under 4 h of low light intensity (i.e., 400 lux; Krishnapillay et al., 1999) can be employed.
Long term horticultural practices, specifically breeding, can contribute to the production of phenotypes with desirable morphological and physiological attributes that improve plant responses to stressors associated with climate change (Andivia et al., 2021). Other horticultural practices that could be of use include preconditioning and enhancement of root and stem growth by nutrient supplementation (Davis and Jacobs, 2005; Oliet et al., 2009; Ovalle et al., 2016a; Acevedo et al., 2020). Root and stem architecture play a critical role in plant hydraulics and in turn drought response (McDowell et al., 2008). Furthermore, an adequate volume container increases root mass and improves the root absorptive ability conferring a greater seedling drought avoidance capability (Villar-Salvador et al., 2004; Ovalle et al., 2016b). Fall nutrient loading can also enhance stress resistance (Timmer, 1997). Another effective horticultural practice for RS species growing in field collections is nutrient loading that consists of applying a nutritional concentration during the fall season. This will increase the availability of nutrient reserves that are rapidly remobilized to support the nutrient demand of new growth once the seedlings are under stress (Oliet et al., 2013; Andivia et al., 2014).
Finally, for fully grown trees for which correct flowering and fruit set are dependent on a certain degree of chilling (which may not be adequate in temperate tree species due to climate change; see section “5. Climate change and ex situ conservation of RS species through dormant buds”) to induce and release dormancy, the use of agricultural/horticultural practices have been suggested (Luedeling, 2012). These treatments or practices include tree defoliation to induce dormancy (Salama et al., 2021), microclimate manipulation by shading and special irrigation practices to increase chill requirements of plants and break dormancy, and the application of dormancy-breaking chemicals to promote budbreak when chill accumulation is not sufficient due to climate change (Luedeling et al., 2009; Luedeling, 2012). However, for some RS species in field collections, these procedures may not be effective, and field collections may have to be established/moved to other locations at higher altitudes or latitudes where temperature regimes are more suitable for growth (Luedeling et al., 2009; Luedeling, 2012).
Temperature and water availability are critical drivers during the different stages of seed development, including maturation (Gutterman, 2000), dormancy (initiation, break), and germination (Probert, 2000). Global climate change, in altering environmental cues related to temperature and rainfall, could therefore preclude, delay, or enhance regeneration from seeds (Walck et al., 2011; Sentinella et al., 2020; Pritchard et al., 2022). There is also ample direct evidence to suggest that changes in temperature and rainfall will affect the yield and quality of seeds in orthodox crops (Hatzig et al., 2018; Abdul Rahman and Ellis, 2019; Wijewardana et al., 2019; Ben Mariem et al., 2021; Poggi et al., 2022) and native plant species (Buechling et al., 2016; Delgado-Vargas et al., 2018). More recent evidence suggests that these effects may extend to the regulation of dormancy and germination (Bernareggi et al., 2016; Klupczyńska and Pawłowski, 2021) as well viability retention (Ooi et al., 2009; Ooi, 2012) and seed longevity (White et al., 2022).
In orthodox seeds, quality attributes such as germ inability, desiccation tolerance, and longevity are acquired during the three phases of their development: (i) histodifferentiation and cell expansion, (ii) reserve accumulation, and (iii) maturation drying (Bewley et al., 2013). Acquisition of desiccation tolerance is associated with events that mostly occur during the reserve accumulation phase (Bewley et al., 2013). In the case of recalcitrant seeds, although they have a similar pattern of development regarding the first two phases, they do not fully develop desiccation tolerance, are dispersed relatively wet (usually >40% water content, fresh weight), and do not present the maturation drying phase (Finch-Savage and Blake, 1994; Kermode and Finch-Savage, 2002). However, there are differences in the level of desiccation tolerated across RS species (Sun, 1999), within genera (Xia et al., 2012, 2014; Ganatsas and Tsakaldimi, 2013; León-Lobos and Ellis, 2018; de Almeida Garcia Rodrigues et al., 2022), genotypes of the same species (Lamarca and Barbedo, 2015; Ganatsas et al., 2016), and even among seeds from the same genotype (Finch-Savage and Blake, 1994). Differences in the level of desiccation tolerance have also been detected in intermediate type (i.e., sub-orthodox) seeded species at genera (Dussert et al., 2000) and species level (Ellis et al., 1991). In recalcitrant seeds, the level of desiccation tolerance can also vary according to the desiccation rate (Farrant et al., 1985; Ntuli et al., 2011), equilibrium dehydration method, and temperature (Sun and Liang, 2001). Because seeds increase their tolerance to desiccation as they advance in their development and lose water, the degree of maturity that they reach at the time of harvest or dispersal is one of the factors that affect their tolerance to desiccation (Berjak and Pammenter, 1997; Kermode and Finch-Savage, 2002).
In general, a stress condition during anthesis and seed development, such as drought or extreme temperatures, will affect the number of seeds that are formed, the quality and seed size in orthodox seeds (Delouche, 1980; Singh et al., 2013). It can be assumed that similar effects would be seen in recalcitrant seeds (see Llanderal-Mendoza et al., 2017 for evidence of this). However, the effects are less evident on seed quality. Although higher temperatures and lower water availability commonly result in the production of smaller seeds in orthodox-seeded species, seed quality is not necessarily affected (Pieta Filho and Ellis, 1991; Sinniah et al., 1998; Contreras et al., 2009).
In the case of recalcitrant seeds, there is limited information on the effects of the maternal environment on seed quality, which directly influences storability, with practically no direct or experimental evidence on the effect of temperatures and water availability on seed development and germinative potential. Additionally, specific attributes of recalcitrant seed post-harvest physiology such as desiccation tolerance and sensitivity to low temperatures, which in general have been under-studied, become very important for predicting the potential impacts of climate on the storability, and hence, germplasm conservation of RS species.
Recalcitrant-seeded species are naturally in greatest abundance in sub-tropical and tropical regions (Berjak and Pammenter, 2008; Wyse and Dickie, 2017), and to a lesser extent in temperate and Mediterranean ecosystems (Wyse and Dickie, 2017), which do, however, host some highly representative genera, with unique and endangered genera, such as Quercus (Denk et al., 2017). The regions that harbor RS species have already experienced a significant rise in atmospheric temperature (IPCC, 2021) and alterations in rainfall patterns (Tabari, 2020). As discussed earlier, environmental conditions (temperature and water deficit) have a direct consequence on seed quality and subsequent offspring performance (Fenner, 1991; Gutterman, 2000). Following this logic, and based on data now emerging on the potential responses of recalcitrant seeds to abiotic stress [water stress (Varghese et al., 2011), warming (Sershen et al., 2014), and acid rain (Ramlall et al., 2015)], climate change will likely affect the viability of stored RS seeds as well as the response of explants derived from these seeds to biotechnologies.
For example, Sershen et al. (2014) showed that even though exposure of Trichilia emetica seeds to elevated temperatures (∼ 5 to 6°C above ambient) did not disrupt metabolic and ultrastructural integrity in embryonic axes, it hastened germinative development possibly due to an earlier burst of reactive oxygen species (ROS) that triggers germination (based on the findings in orthodox seeds by El-Maarouf-Bouteau and Bailly (2008) and Varghese et al. (2011) for recalcitrant seeds). This has implications for the short- to medium-term storage of recalcitrant seed germplasm that involves maintenance at water contents close to that at shedding and at ambient or slightly lower temperatures (termed “hydrated-storage”; Berjak et al., 1989). The duration of storage under these conditions is curtailed by in-storage germination-associated events, culminating in seed death (Berjak et al., 1989; Pammenter et al., 1994). If seeds were to enter this storage at an advanced stage of germinative development and/or heightened levels of ROS production, storage lifespan could be further reduced through germination or loss of viability. This rationale is supported by the fact that storage at temperatures lower than ambient has been shown to post-pone the onset of germination in recalcitrant seeds by slowing down the metabolic rate (e.g., Pritchard et al., 1995), provided the seeds are not chilling-sensitive. Additionally, ROS have also been implicated in cell wall loosening and radical protrusion during germinative development (Bailly, 2004; Müller et al., 2009), and if climate change were to result in water stress in recalcitrant seeds during or shortly after shedding, then these seeds could experience desiccation induced oxidative stress (Varghese et al., 2011), compromising their viability even before they enter storage.
Furthermore, exposing recalcitrant seeds to elevated temperatures (above ambient) promotes the proliferation of a spectrum of endogenous microbes that can start immediately after shedding and can curtail the lifespan of recalcitrant seeds in hydrated storage (Mycock and Berjak, 1990; Sutherland et al., 2002). Even when seeds are treated with fungicidal agents and stored at low temperatures these microbes are almost impossible to suppress (Mycock and Berjak, 1995; Moothoo-Padayachie et al., 2018).
As alluded to above, there is evidence that exposure of recalcitrant seeds to abiotic stress such as elevated temperature (Pukacka and Ratajczak, 2005; Sershen et al., 2014) and water stress (Varghese et al., 2011) can lead to the enhanced production of potentially harmful ROS [reviewed by Pritchard et al. (2022)]. This implies that if the recalcitrant seeds to be used as a source of the embryonic axes/zygotic embryos (explants) for cryopreservation have been exposed to climate-induced temperature or water stress during development and/or after shedding, then the explants from them may already possess heightened levels of ROS production upon harvest. This will predispose them to oxidative stress induced viability loss during the excision and partial dehydration steps which in themselves lead to increased ROS production during cryopreservation (Berjak and Pammenter, 2014).
The Quercus case study (subsection “8. Case study–Quercus spp”) details the potential impacts of climate change on the long-term storability of typical recalcitrant seeds. These impacts could emerge as a consequence of the effects of climate change on the morpho-physiological characteristics of the seeds of this genus in various parts of the world. Further, Daws et al. (2004, 2006) found that recalcitrant seeds of Aesculus hippocastanum and Acer pseudoplatanus (Sapindaceae) from the northern limit of this species in Europe (Scotland) were less developed when shed because of cooler temperatures during development. These seeds were smaller (fresh mass), presented embryonic axes with higher water content, and had lower tolerance to desiccation than seeds produced in southern locations (Greece in the case of A. hippocastanum; France and Italy in the case of A. pseudoplatanus). These studies suggest that for certain RS temperate trees, an increase in air temperature during seed development should favor the production of more developed and desiccation tolerant seeds, which could benefit their germplasm conservation.
Despite the importance of temperature in seed production and quality, their effects would depend on the species and other environmental factors during plant growth and seed development. For instance, Xia et al. (2014) found that the embryo of Quercus gambelii, a species adapted to the desert environment of North America, displayed intermediate desiccation tolerance, and embryos from temperate desert populations were more tolerant than those from the warm desert. In the case of species from tropical environments, different studies have reported that seed maturation and quality would be more affected by precipitation during the growing season than by temperatures. For instance, in Inga vera (Lamarca and Barbedo, 2015), Eugenia pyriformis (Lamarca et al., 2016), and Araucaria angustifolia (Shibata et al., 2021) seeds produced under low precipitation matured in less time, had lower water content at shedding and were more tolerant to desiccation than seeds produced under higher precipitation.
Sensitivity to chilling injury is another important attribute for recalcitrant seed conservation that may be affected by the environment. Bharuth et al. (2020), in evaluating the effect of chilling on Ekebergia capensis (Meliaceae) from southern Africa, found that when stored at 3°C seeds from the temperate environment [Port Elizabeth, South Africa (SA)] were able to retain full viability (100%) for 8 weeks, while seeds from sub-tropical (St. Lucia, SA) and tropical (Tanzania) environments were susceptible to chilling and lost all viability after 38 and 9 days, respectively. These differences in viability status were reflected at the ultrastructural level in embryonic axes, in the form of increased vacuolation, and mitochondrial and nuclear degeneration (Bharuth et al., 2020). Furthermore, embryonic axes of E. capensis seeds from the temperate environment (Port Elizabeth, SA, USA) exhibited 30% survival after cryopreservation, while those from the warmer environment (St. Lucia, SA, USA) did not survive cryopreservation (Bharuth and Naidoo, 2020).
Chilling sensitivity appears to be intimately linked to cryo-survival and if climate change does bring about changes in localized populations of RS species, this could influence the success achieved in conserving the germplasm of these seeds via cryopreservation.
Ex situ pollen conservation is a valuable alternative to overcoming the critical limitation of seed banking for safeguarding the germplasm of RS species (Pence and Bruns, 2022). By containing all the information of the haploid genome of the species, it is a way of conserving genes more than genotypes, and in this sense, its use should be thought of as a complementary way to the storage of other plant propagules (Grout and Roberts, 1995; Berthaud, 1997; Ren et al., 2019). The most used ex situ pollen conservation method involves the grains’ dehydration before storage in a fridge (4°C), freezer (–20°C), or in liquid nitrogen (Dinato et al., 2020). However, not all pollen types survive the initial desiccation needed for successful cryopreservation. As in seeds, we can find “orthodox” or “recalcitrant” pollen, depending on their tolerance or sensitivity to dehydration (Pacini et al., 2006). Nevertheless, most species studied to date produce orthodox pollen (Franchi et al., 2011). Although more commonly applied to crop species, hand-pollination and pollen storage have been used as part of an integrated conservation strategy for a few endangered RS tree species. For example, pollen conservation of Castanea dentata (American chestnut) provides options to restore genetic diversity of lines that are resistant to chestnut blight (Fernando et al., 2006; Walters and Pence, 2021). Similarly, the long-term storage of pollen has been found as part of the integrated conservation strategy for Metrosideros bartlettii, a critically endangered tree from New Zealand (van der Walt et al., 2022). Interestingly, there are several examples of RS species with desiccation tolerant (i.e., orthodox) pollen that allows the relatively easy ex situ storage of dry pollen in species with recalcitrant seeds. Examples include Acer pseudoplatanus, Aesculus hippocastanum, Camellia sinensis, Castanea sativa, Eriobotrya japonica, Fagus sylvatica, Litchi chinensis, Quercus spp., Salix caprea, Cocos nucifera, among others (Franchi et al., 2011; Ballesteros and Pritchard, 2020).
Pollen can be stored in liquid nitrogen for many years without losing its ability to pollinate, fertilize, or bring about fruit production (Xu et al., 2014; Ren et al., 2019). However, certain factors have been recognized as responsible for the success of pollen cryopreservation: its initial hydration state, the type of pollen or morphology (bi or trinucleate), the maturity of the pollen grain, the genotype and taxonomy, the physiological state of the donor plant, ecological adaptations, pollen grain size, and protocol/methodology used (Xu et al., 2014; Ren et al., 2019; Vishwakarma et al., 2021). Some of these factors are modulated by climate change.
Various studies show that the proportion of RS species decreases as the habitat becomes drier (Tweddle et al., 2003; Probert et al., 2009). Unfortunately, all projected climate change scenarios predict with likely/high confidence that extreme events associated with droughts, either in intensity or frequency, will increase in the future in more regions of the world (IPCC, 2021). For example, in Chile, the decline of precipitation has been accentuated since 2010, with annual rainfall deficits ranging between 25 and 45% (i.e., “Central Chile Mega Drought”; CR2, 2015) due to natural and anthropogenic forces (Garreaud et al., 2020).
The reproductive stage of plants is the phase most sensitive to drought, and although this stage involves many processes, anther and pollen development are the most sensitive among them (Saini and Lalonde, 1997; Guo et al., 2016). Drought generates changes in internal cellular homeostasis, altering intracellular sugar content, hormonal balance, and the presence of ROS (Yu et al., 2019). We, therefore, hypothesize that drought stress in plants could indirectly affect the use of pollen in cryopreservation by decreasing the quantity and quality of pollen, reducing its tolerance to desiccation and/or storage longevity in terms of germination ability (Figure 1).
Figure 1. Potential effects of drought-induced phenotypes on ex situ pollen conservation stages. Failures in the metabolism and delivery of assimilates and carbohydrates, hormonal imbalance, and collapse in maintaining homeostatic balance to control ROS, result in (i) increased pollen abortion rates, affecting the amount available for collections, (ii) pollen with low sucrose and starch content, which has been related to lower longevity and tolerance to desiccation, directly affecting the suitability of pollen to resist the drying process and storage, (iii) defects in the exine and Ubisch bodies, which is hypothesized could affect the resistance of the pollen grain to desiccation and changes in internal pressure, as well decreasing its aptitude as an explant to be cryopreserved, and (iv) increased pollen sterility, impacting its germination ability and ultimately, being unable to recover for fertilization. In a separate case within an elliptical figure, drought stress within tolerable ranges for the plant, which it is capable of coping with, could be beneficial by increasing the sucrose content of the pollen, increasing its longevity, and working as a precondition for its desiccation. (–) Decrease suitability; (+), increase suitability.
Sucrose confers desiccation tolerance in ways reminiscent of orthodox seeds, that is, by forming a glassy state that limits cell mobility and protects membranes by replacing water in chemical bonds (Buitink and Leprince, 2004). Although the amount of soluble sugars is higher in anthers during a drought, the transfer of these sugars to the pollen grains is blocked due to a dysfunction of the tapetum and the suppression of enzymes of sugar metabolism and starch biosynthesis (Sheoran and Saini, 1996; Yu et al., 2019), which ultimately causes sterility and pollen abortion (Jin et al., 2013). An explanation of the above is the hormonal imbalance derived from drought stress, which affects not only the functionality of the tapetum and sugar metabolism (De Storme and Geelen, 2014), but also ROS detoxification system (Hu et al., 2011; Jin et al., 2013; Dong et al., 2017; Zhang et al., 2021), anther dehiscence, pollen maturation, induction of pollen germination (Kinoshita-Tsujimura and Kakimoto, 2011), and defects in the exine and Ubisch bodies (Aya et al., 2009). Since the latter provides resistance to the internal pressure of pollen, environmental injury, and desiccation damage (Edlund et al., 2004; Mach, 2012; Hu et al., 2022), such defects could also affect the ability of pollen to tolerate the decrease in water contents necessary for cryopreservation.
An illustrative example of these potential effects on pollen can be found in studies on pollen flows of the RS species Theobroma cacao in Ecuador, which shows a significant correlation between pollen production and climatic factors, mainly precipitation. Interestingly, the months with the highest pollen production and rainfall also have the highest air temperatures. In this sense, it is important to understand the interactions between air temperature and soil moisture since an adequate water supply becomes critical to ensure adequate pollen production when temperatures rise (García-Cruzatty et al., 2020; Mena-Montoya et al., 2020).
An increase in temperature also has a significant impact on the performance of male gametophytes, and thus, has a qualitative and quantitative impact on seed production (Hedhly, 2011). Poor fertilization and fruit production in coconut, for example, have been linked to above average temperatures impacting the germination of pollen on the stigma as well as pollen tube growth through the style (Hebbar et al., 2018, 2022). In fact, Zinn et al. (2010) found that even a single day of extreme weather conditions had a detrimental impact on the reproductive success of several plant species. Early stages of pollen development, including anther wall development, microsporogenesis and microgametogenesis are particularly vulnerable, and the application of heat stress during this developmental window can lead to pollen abortion (Raja et al., 2019; Chaturvedi et al., 2021).
Environmental conditions during another development also influence the chemical composition of pollen (see Delph et al., 1997 and references within). Investigations into the impact of heat stress on crop species showed that an accumulation of ROS in the anthers resulted in an imbalance in ROS and ROS-quenching enzymes (Zhao et al., 2018), which, as with drought, leads to sterile phenotypes. It should also be noted that heat stress often coincides with drought and higher light intensities, and the combined effect of these stresses on pollen are not well studied (Raja et al., 2019). However, there is evidence that mature pollen grains can respond to high-temperature stress through acclimatization of their physiological and biochemical mechanisms and by synthesizing heat shock proteins (Rosbakh et al., 2018; Zhang et al., 2021).
Pollen from the highly recalcitrant tropical species Mangifera indica has been successfully cryopreserved (Chaudhury et al., 2010). However, recent studies indicate that its flowering is extremely sensitive to temperature changes, which would decrease the rate of pollen germination and the length of the pollen tube. Moreover, in all the genotypes evaluated, pollen tubes were more tolerant to low-temperature than high-temperature stress (Liu et al., 2023). Similarly, in another highly RS fruit species, Litchi chinensis, it has been shown that high temperatures and reduced relative humidity affect pollen by increasing dehiscence and decreasing the germination rate; however, the severity of the affectation is strongly influenced by the genotype (Matsuda and Higuchi, 2017). This species also has an ex situ conservation strategy that includes pollen cryopreservation (Chaudhury et al., 2010).
Therefore, climate change, particularly heat stress, reduced relative humidity, and drought is likely to modulate pollen development, morphology and biochemistry in both recalcitrant-seeded and recalcitrant-pollen species adversely affecting pollen conservation. An increase in the frequency of intense and severe tropical cyclones is also expected, with extreme winds, rainfalls, and floods (Walsh et al., 2016; Wu et al., 2020; Chand et al., 2022). Although there are no specific studies regarding the effect of these phenomena on pollen of RS species, research on pollen from orthodox species suggests that microsporogenesis could be more sensitive to flooding than to drought, affecting its quantity, viability, germination and size (Yamburov et al., 2014). The effect on size is of particular importance since some studies suggest that the size of the pollen grain is a variable that could affect the tolerance to cryopreservation (Ren et al., 2019).
In the autumn, vegetative and floral buds of some species from temperate climates move through a set of natural adaptations permitting their dormancy and subsequent cold acclimation (i.e., cold hardiness) (Hänninen and Tanino, 2011; Cooke et al., 2012; Wisniewski et al., 2018; Fadón et al., 2020; Chang et al., 2021). The entry into this dormant and cold-acclimated stage allows vegetative and floral buds to survive the chilling and freezing cold conditions of winter. This natural adaptation to cold/freezing environments was ingeniously used in the 1960s to develop a particular set of cryopreservation technologies (Sakai, 1960) that are currently the base for the long-term preservation of genetic resources of many clonal temperate fruit and nut trees and shrubs (Tanner et al., 2021). These cryobiotechnologies are important for the long-term ex situ preservation of species with orthodox seeds that present extremely short longevity when banked, such as those from the genera Salix and Populus (Sakai, 1960; Bonnart et al., 2014; Ballesteros and Pence, 2017; Tanner et al., 2021). In addition, dormant bud cryopreservation has a great potential for the ex situ conservation of RS species such as oaks (Quercus spp.), chestnuts (Castanea spp.), and horse-chestnuts (Aesculus spp.) (Ballesteros and Pritchard, 2020).
The effects of climate change on dormant buds’ cryopreservation could be significant. Buds used for cryopreservation are collected during mid-winter, when they have the high levels of dormancy needed to achieve the greatest freezing tolerance (Tanner et al., 2021). If collected earlier, at the onset of cold acclimation during the fall season, buds have lower freezing tolerance (Tanner et al., 2021). If collected after buds’ de-acclimation in spring, buds become cold tender and are susceptible to freeze damage (Arora and Taulavuori, 2016). Hence, twigs collected outside of mid-winter dormancy can be expected to have very low survival using the standard cryopreservation method (Sakai, 1960; Tanner et al., 2021). It is furthermore possible that the time range for sample collection will be reduced if species and populations experience late autumns and early springs due to climate change (Arora and Taulavuori, 2016; Vyse et al., 2019). In addition, due to the shorter time in the dormancy stage, they may acquire lower cold -acclimation (Cooke et al., 2012; Wisniewski et al., 2018; Fadón et al., 2020; Chang et al., 2021), producing buds in the mid-winter with lower cold-hardiness than desired. In this sense, it is important to note that the geographical origin of source trees seems to have a strong effect on cryopreservation success, with species from colder locations or years (so with higher cold hardiness) showing higher recovery after cryopreservation (Stushnoff and Junttila, 1986; Towill et al., 2004; Toldam-Andersen et al., 2007; Jenderek et al., 2011).
Despite the challenges encountered for dormant bud cryopreservation in the context of climate change, there are some mitigatory measures. In terms of winter dormant bud cryopreservation, twigs collected outside of mid-winter dormancy may present better cryopreservation success using shoot tip vitrification-based protocols (Tanner et al., 2021). Alternatively, in some cases, dormant buds could be artificially acclimated by exposure to gradually cooler temperature under controlled laboratory conditions after twig harvest (Tanner et al., 2021).
Dormancy and cold acclimation of buds are also very important for healthy tree growth, pollen development and fruit set. The level of chill accumulated during this stage is critical to coordinate the subsequent floral development and vegetative growth at the climatically favorable time of the year (Cooke et al., 2012; Fadón et al., 2020; Chang et al., 2021). In recent years, regulation and release of bud dormancy and cold acclimation have received a lot of interest from the perspective of global climate change. For instance, high autumn and/or winter temperatures, as well as early spring induced by climate change have been shown to have profound effects on phenology (Cooke et al., 2012; Fadón et al., 2020; Chang et al., 2021), causing dramatic and unpredictable effects on deciduous fruit tree orchards by advancing or delaying dormancy and leaf senescence, flowering, or fruit ripening (Luedeling, 2012; Fadón et al., 2020). In addition, the ability of some species to tolerate low temperature or freezing is being modified (Chang et al., 2021), while warmer autumn temperatures have been related to late dormancy induction and late leaf senescence (Beil et al., 2021). Late dormancy induction can have a negative impact on plants, particularly when early autumn frosts co-occur (Cooper et al., 2019; Vyse et al., 2019). In addition, it has been shown that warmer autumn temperatures result in a shortening of winter, with a delayed spring leaf-out (Beil et al., 2021). Warmer winters do not appear to affect the spring phenology (Beil et al., 2021), but seem to have unpredictable effects. For example, mild temperatures during winter may lead to an erratic bud burst and blooming due to the lack of accumulated cold temperatures during winter dormancy (Fadón and Rodrigo, 2018). Finally, an advance in the arrival of the warm temperatures of late winter and spring is causing early de-acclimation of buds (Arora and Taulavuori, 2016; Vyse et al., 2019). Since de-acclimated buds are no longer tolerant to freezing, early de-acclimation is exposing buds of many trees to late frost events, leading to devastating effects on whole ecosystems and significantly reducing the yield of crop plants (Arora and Taulavuori, 2016; Vyse et al., 2019).
Due to this variety of effects of climate change on the phenology of woody perennials, the suitability of orchard locations for some species and cultivars has considerably shifted, leading growers to modify the array of fruit tree species and varieties (Luedeling et al., 2009; Fadón et al., 2020; Dinu et al., 2021). This is particularly relevant for field collections of RS species with temperate origins (see section “2. Ex situ conservation of RS species as field collections and the effects of climate change on their production and culture”), although the impact will vary across locations. For example, temperate trees grown in warmer regions of the Mediterranean or in tropical and subtropical regions may face insufficient winter chill, which has a crucial role in dormancy and productivity (Luedeling, 2012; Salama et al., 2021). Temperate regions will experience relatively little change in relation to chill but may suffer stronger de-acclimation and late frost effects (Vyse et al., 2019). On the other hand, cold regions, as winters warm up, may even see chill increases, which may favor the migration of species toward northern regions where certain fast-growing deciduous species can outcompete the slow-growth cold acclimated deciduous trees or conifers (Sykes and Prentice, 1996; Luedeling, 2012; Chang et al., 2021). In addition, as bud dormancy and tolerance to cold winter temperatures are required for proper flowering and fruit set (Fadón et al., 2020), shifts in the suitability of orchard locations for some species will affect pollen and seed production and quality, affecting not only breeding but also seed collections for ecological restoration (detailed in the pollen and seed subsections).
Shoot tips are one of the most used explants for cryopreservation of plants, particularly clonal crop species or genotypes of interest, but also exceptional wild plants, including many RS species (Nadarajan et al., 2008; Varghese et al., 2009; Normah et al., 2019; Streczynski et al., 2019; Kulus, 2020; Pence et al., 2020; Pence and Chaiken, 2021). Generally, shoot tips are excised from plants cultured in vitro, and then processed for their cryopreservation. Sometimes, shoot tips are directly excised from winter dormant buds (Tanner et al., 2021), from seedlings of seeds germinated in vitro or from young shoots developed in spring (Pence and Chaiken, 2021). Most shoot tips also go through a process of in vitro multiplication prior to their cryopreservation (Benson et al., 2007; Uchendu et al., 2013; Normah et al., 2019; Pence and Chaiken, 2021).
The developmental and physiological status of the shoot tips is critical for their cryopreservation, and as with seeds and dormant buds, differences in response to cryopreservation are found across species and genotypes adapted to different environments. The response of shoot tips to cryopreservation can be significantly different between temperate and tropical species (Normah et al., 2019). For example, temperate genotypes, due to their natural adaptation to winter low temperatures (see section “5. Climate change and ex situ conservation of RS species through dormant buds”), present positive responses to some degree of desiccation, cold hardiness, acclimation, and dormancy, and these are important factors used to cryoprotect the shoot tips in cryopreservation protocols (Benson et al., 2007; Normah et al., 2019). In contrast, tropical and warm temperate species are more sensitive to chilling and desiccation stresses, and their cryopreservation protocols must be developed accordingly (Benson et al., 2007; Normah et al., 2019). Despite this general trend related to the potential of a species to cold acclimate, there are not many publications clarifying why shoot tip cryopreservation differs across species, genotypes, or geographical regions. Some authors indicate a better performance of cryopreservation in genotypes that present average to high field cold hardiness in contrast to those with low field cold hardiness (Kushnarenko et al., 2009), but this trend is not always clear (Reed, 1990).
Cold acclimation is commonly stimulated in naturally acclimating species or genotypes to increase the cold hardiness of the shoot tips and achieve enhanced shoot tip survival after cryopreservation (Scottez et al., 1992; Vandenbussche et al., 1999; Benson et al., 2007; Uchendu et al., 2013; Folgado et al., 2015; Funnekotter et al., 2017; Mathew et al., 2018; Normah et al., 2019; Roque-Borda et al., 2021). Additionally, it can also be used for some chill-sensitive species (Folgado and Panis, 2019; Normah et al., 2019). Cold acclimation of shoot tips is typically applied to full plants growing in vitro before shoot tips are excised, to in vitro rejuvenated buds, or to in vitro shoot cultures. Cold- hardened dormant buds, which have undergone natural seasonal acclimation (see section “5. Climate change and ex situ conservation of RS species through dormant buds” of this article), may also be used as a source of cold-acclimated shoot tips (Uchendu et al., 2013; Tanner et al., 2021).
Tropical species generally do not possess natural adaptations to low temperatures, and cryopreservation protocols developed in these species rely on the addition of sucrose and other compounds to overcome the lack of natural cold hardiness present in temperate species (Benson et al., 2007; Folgado et al., 2015; Normah et al., 2019). This suggests that climate change alterations should not affect the current cryopreservation protocols developed for tropical species. On the other hand, temperate species and genotypes, as well as chilling tolerant tropical species, tend to rely on their natural adaptation to low temperatures for the successful development of cryopreservation protocols. Therefore, cryopreservation protocols currently used for these RS species may be less successful as they will potentially suffer the physiological changes against natural cold hardiness induced by climate change (see section “5. Climate change and ex situ conservation of RS species through dormant buds”). These effects will be considerable for shoot tips directly excised from winter dormant buds (Uchendu et al., 2013; Tanner et al., 2021) or could be significant for shoot tips obtained directly from spring sprouts. However, most shoot tips used for cryopreservation are obtained after a step of in vitro shoot multiplication with multiple passes. These shoots tips cultured in vitro do not retain the direct cold hardiness achieved outdoors as is the case in winter dormant buds, but they have the potential to adapt to cold, as this is genetically controlled (Volk, 2010; Zeng et al., 2014).
However, will warmer scenarios induced by climate change modify the genetic composition of temperate and chilling tolerant tropical RS species making them less adapted to low temperatures? (Ramantha Rao and Sthapit, 2012). The study of the evolution of temperate species through climate changes in the past has shown that some species that adapted well to warmer climates lost their genetic and physiological ability to tolerate cold and increased their susceptibility to freezing (Chang et al., 2021). These changes, though, occurred slowly over evolutionary time scales, which differ from the fast rate of changes induced by the current climate change. However, certain genetic plasticity has been observed in some tree species to adapt to new climate change scenarios, mainly through epigenetic modifications (Alberto et al., 2013; Chang et al., 2021). The extent of these adaptations and their reversibility during cold acclimation in vitro are not fully understood (Chang et al., 2021), hence, it is difficult to predict how potential genetic changes in temperate species when adapting to new climate change scenarios will affect their potential to adapt to cold in vitro. There have been no published attempts to clarify this in the case of tropical tree species (Ramantha Rao and Sthapit, 2012).
In addition to temperature changes, climate change is affecting water availability and increasing drought in many environments (see introduction). In this regard, Pence and Chaiken (2021) observed in their experiments that the successful cryopreservation of Quercus spp. was achieved in species from wet environments with poor success associated with species from dry environments. If this pattern can be generalized for Quercus or other RS genera from both temperate and tropical areas, drier environments due to climate change could potentially have a negative effect on cryopreservation of the species living and adapting to these drier environments. But more comparative studies are needed to confirm this suggestion.
Cycads are distributed in over 60 countries across five continents; Central and Latin America, Australia, Asia, and Africa (Okubamichael et al., 2016). Cycads are also the most threatened group of plants on earth with more than 62% of species threatened with extinction and urgent integrated conservation efforts are required to prevent extinction (Wade et al., 2016). Globally botanical gardens hold significant cycad conservation collections, which due to their recalcitrant-seeded nature is almost exclusively represented as field collections (Griffith et al., 2014; Cousins and Witkowski, 2017). For example, the Lowveld National Botanical Garden in South Africa has been collecting seed from native Encephalartos species since the early 1980’s and now has extensive field gene banks from which thousands of seedlings are generated each year (van der Walt, 2010). Similarly, the Montgomery Botanical Center cycad collection spans over 5 ha and represents cycads from Asia, Africa, America, and Australia (Griffith et al., 2014). Plants held in field collections, seed orchards or field GeneBanks, are however, vulnerable to abiotic and biotic impacts and the physical proximity of plants can increase the risk of infestation by plant pathogens (Volis, 2017).
Evidence shows that climate change will aggravate the impacts of invasive species into novel communities and ecosystems (Wei et al., 2018). One of these pathogens is the Cycad Aulacaspis Scale (CAS) (Aulacaspis yasumatsui) Takagi, which was first discovered in 1972 in Thailand on Cycas revoluta (Takagi, 1977). Since its discovery, CAS has spread to 39 countries and is now considered the single most important threat to natural cycad populations by the International Union for the Conservation of Nature Cycad Specialist Group (IUCN) (IUCN, 2005; Marler et al., 2021). Although CAS naturally occurs on Cycas revoluta, it has been recorded on 21 different cycad species from eight genera (Bowenia, Ceratozamia, Dioon, Encephalartos, Macrozamia, Microcycas, Stangeria, and Zamia). At least two species, Cycas micronesica and C. taitungensis now face imminent extinction due to CAS (Muniappan et al., 2012) and the Cycad Specialist Group has been working on ways to mitigate the impact of CAS on both wild and cultivated cycad collections since 2006 (IUCN, 2005). By Nesamari et al. (2015) CAS was found to be widespread in three provinces in South Africa, namely, Gauteng, KwaZulu-Natal and Limpopo, where it established in botanical gardens on Cycas thouarsii, C. revoluta and seven native Encephalartos species. It is furthermore predicted that favorable conditions for CAS establishment exist under current and future climate scenarios in an additional five provinces of South Africa (Satishchandra and Geerts, 2020). These areas include localities of botanical gardens with significant Encephalartos conservation collections (i.e., Lowveld National Botanical Garden in Mpumalanga and Kirstenbosch National Botanical Garden in Cape Town). Cycads infected with CAS have shown a drastic decline in non-structural carbohydrates and the ongoing depletion of carbohydrates eventually kills the host plant (Marler et al., 2021). In addition to the impact on adult plants, the gametophyte starch pool of CAS infected seed has been found to be 63% smaller compared to healthy seed resulting in a six-fold decrease in germination of seed infected with CAS (Marler et al., 2021). An increase of CAS, as predicted for various climate change scenarios, will likely impact adult plants, the production of new leaf flushes, and development of gametophytes. This may result in low quality shoots, seed and pollen which are the main propagules used for cryopreservation of cycads.
Thermogenesis functions as an attractant or reward for insect pollinators in basal angiosperms and cycads, and pollination periods in cycad cones have been found to have daily patterns of heat production (Suinyuy and Johnson, 2018). The impact of climate change on cycad pollinators is unknown but ambient temperatures are crucial in the initiation, magnitude, and timing of cone temperature in Encephalartos and Macrozamia species (Laidlaw and Forster, 2012; Suinyuy and Johnson, 2018). An increase in temperatures may therefore result in earlier flowering, which will require pollinators to adapt, thereby impacting reproduction (Laidlaw and Forster, 2012).
Cycads produce recalcitrant seeds and while tissue culture methods via somatic embryogenesis have been developed for some species, establishing plantlets ex vitro has been mostly unsuccessful (Litz et al., 2004; Griffith et al., 2014; Nadarajan et al., 2018) although research is ongoing (Berjak and Pammenter, 2014). Cycads are deciduous and the storage of pollen has been identified as an essential conservation tool for many species (Nadarajan et al., 2018). Although pollen cryopreservation has been successful in some Cycas and Encephalartos species (Osborne et al., 1992; Mostert et al., 2017), limited research and baseline data on pollen biology and storage is hampering effective application for ex situ conservation (Nadarajan et al., 2018). Although there are no studies on the predicted impacts of climate change on cycad pollen quality and quantity specifically, the impact of increased temperatures on the development of male gametophytes has been extensively described, especially in crop species (see pollen section above).
Cycads, as a flagship group for ex situ conservation, clearly illustrate the detrimental impact of climate change on the quality of explants (vegetative buds, pollen, seed, and zygotic embryos) obtained from adult plants in ex situ collections.
Quercus (Oak) is a large genus within the family Fagaceae comprising of around 500 taxa (Carrero et al., 2020), predominantly distributed in the temperate regions of the Northern Hemisphere, ranging from the Mediterranean in Europe to North America and the subtropical forests in Asia and Central-South America (Nixon, 2006). Oak forests are facing a sustained decline due to various driving factors including climate change, pests, pathogens and anthropogenic forest degradation (Thomas et al., 2003; Haavik et al., 2015; Tiberi et al., 2016; Carrero et al., 2020). As a consequence, thirty-one percent of oaks are considered threatened by extinction (Carrero et al., 2020).
There is an urgent need for conservation of oak genetic diversity, which is at present mainly conducted ex situ in field collections due to the recalcitrant nature of the seeds (that impedes its dry storage in seed banks). There are 296 oak species reported in ex situ collections globally, while nearly one-third, including 58 threatened species, of oak species (134) not reported in any ex situ collection (Carrero et al., 2020). This means that more than half of the Critically Endangered or Endangered taxa face imminent extinction if current threats are not addressed (Carrero et al., 2020) and the ex situ conservation of oak genetic resources is not expanded into more efficient germplasm that can be long-term stored and preserved (Ballesteros and Pritchard, 2020).
Quercus species are predominantly monoecism, wind pollinated and highly self-incompatible (Ducousso et al., 1993; references included). As in most flowering plants, reproductive biology in oaks, in particular flowering phenology, is strongly affected by climatic conditions (Gómez-Casero et al., 2007; Gerst et al., 2017). This is particularly relevant for deciduous oak species producing winter dormant buds, as flowering phenology is directly related to environmental cues occurring mainly during winter and spring and detected by the dormant buds (see dormant buds’ subsection; Fadón et al., 2020; Chang et al., 2021). For example, winter precipitation and temperature have been found to be the main climate drivers of vegetative growth and reproductive potential for native California oaks, with high winter and spring temperatures advancing bud burst onsets (Armstrong-Herniman and Greenwood, 2021). Similarly, shorter Quercus phenological ranges were obtained in years with warmer springs, and longer ranges in colder years in the Iberian Peninsula (Gómez-Casero et al., 2007). All these phenological changes are due to buds’ de-acclimation processes, which are increasingly being detected due to climate change in oaks and many other temperate tree species (Arora and Taulavuori, 2016; Vyse et al., 2019; Vogel, 2022). Recent climatic change is leading to changes in the timing and intensity of the pollen season in members of this genus (López-Orozco et al., 2021; Adams-Groom et al., 2022). Other effects on flowering strongly related to climate change, but not necessarily negative are, for example, the triggering of a secondary Quercus flowering in autumn, as found in some species in the Iberian Peninsula after dry summers and warm autumns (García-Mozo et al., 2022). This seems to be an unusual event, but it has been related to a relict capacity of some Mediterranean Quercus spp. to adapt to climatic changes, as it has occurred frequently during the evolutionary history of this area (García-Mozo et al., 2022).
In addition to flowering phenology, there is some evidence that climate change could affect pollen quality and production in Quercus species, as in Q. ilex (Bykova et al., 2018). Hence, smaller quantities of lower quality pollen produced due to climate change, could reduce the reproductive potential of the species (Bykova et al., 2018; Schermer et al., 2019).
Climatic conditions also drive mast seeding and interannual variation in seed production in Quercus species, as found for most long-lived and wind-pollinated plants (Abrahamson and Layne, 2003; Koenig et al., 2016). Here, we can find indirect and direct evidence that the change in environmental conditions due to climate change is altering seed production in oaks globally. For example, Pérez-Ramos et al. (2015) detected that mast seeding in Mediterranean oaks was strongly and positively correlated with water availability and air temperature, mainly in the spring and summer. Warmer springs increased seed production in deciduous species, but this effect was variable for the evergreen species (Pérez-Ramos et al., 2015). In this regard, a marked increase in seed production due to increased spring temperature had been detected in diverse deciduous oaks (Caignard et al., 2017; Shibata et al., 2020). On the other side, Shibata et al. (2020) found evidence of decadal changes in the masting behavior of the Japanese oak Q. crispula showing that the shortening of the masting interval was associated with rising temperature. In addition, Sánchez-Humanes and Espelta (2011) detected (experimentally) that increased drought reduced seed production on Q. ilex in Spain. The negative effects of increased water deficit during summer have also been detected on female flowers and total fruit biomass production (Liu et al., 2015; Gavinet et al., 2019) and fruit abortion rate in Q. ilex (Le Roncé et al., 2021).
Climate variables affect the seed morphophysiological traits in Quercus species suggesting a potential impact of climate change on seed quality. Evidence of this includes the fact that acorn length increased significantly with mean temperature of the warmest month, decreased with precipitation, and displayed negative linear relationships with equivalent latitude in Q. variabilis from China, (Zhou et al., 2013; Gao et al, 2021). A similar finding was detected by Llanderal-Mendoza et al. (2017) for Q. rugosa from Mexico. Moreover, larger acorns, in general, have higher germination (Bonito et al., 2011; Llanderal-Mendoza et al., 2017; Sánchez-Montes de Oca et al., 2018; Gavranović Markić et al., 2022) and result in larger seedlings (Lloret et al., 1999; Alfonso-Corrado et al., 2014; Shi et al., 2019), and also seedling survival under greenhouse conditions (Alfonso-Corrado et al., 2014) and in situ (Aizen and Woodcock, 1996; Ramírez-Valiente et al., 2009). Ramírez-Valiente et al. (2009) found that Q. suber populations originating from the site with the driest summer in Spain produced bigger acorns that also showed the higher seedling survival rate under dry conditions. A similar finding was obtained by Zhang et al. (2017) for Q. acutissima from China while Badano and Sánchez-Montes de Oca (2022) illustrated it in the Mexican species Q. viminea and Q. eduardii by placing seeds under a simulated increase of 2°C with a 17–18% reduction in rainfall.
In terms of seed storage, all oaks studied to date have recalcitrant seeds (Xia et al., 2012, 2022; Royal Botanic Gardens Kew, 2022); consequently, traditional long-term ex situ conservation in seed banks is not possible and thus require cryobiotechnological approaches (Walters et al., 2013; Ballesteros and Pritchard, 2020). Seed embryonic axes (with shoot and root meristems) are the preferred explants for oak cryopreservation as, analogously to conventional seed banking, high genetic diversity can be captured and axes can be grown into full plants with relatively simple micropropagation procedures (Ballesteros and Pritchard, 2020). However, the successful cryopreservation of embryonic axes is often species-specific and appears to be influenced by the environmental conditions at which the seed producing trees grow (Xia et al., 2012). According to these authors, the embryonic axes from two North American species adapted to cold, wet winters, were more tolerant to desiccation and freezing (the stresses inferred by cryopreservation) than the embryonic axes from Chinese subtropical species from a semi-humid habitat. Similarly, Amimi et al. (2020) found that Q. ilex and Q. canariensis seeds from Tunisia originating from high elevation, where frost events are frequent, showed the lowest freezing sensitivity compared with Q. coccifera and Q. suber. These results suggest that the warmer and drier conditions expected by climate change could negatively impact the cryopreservation output of oak embryonic axis cryopreservation. However, this environmentally-modulated response to cryopreservation of the oak seeds may not be shared among all species. For example, another study indicated that seed of subalpine oak species from China were most desiccation sensitive and died faster when dried than subtropical and temperate oak from the same country (Xia et al., 2022), suggesting the opposite trend found for North American and Mediterranean species (Xia et al., 2012; Amimi et al., 2020).
Pollen cryopreservation could also be used as a complementary technology to support conservation and breeding programs in oaks. Interestingly, whilst the seeds of oak are recalcitrant it appears that the pollen has much greater desiccation tolerance (Ballesteros and Pritchard, 2020). However, climate change could impact the potential longevity of pollen stored in germplasm banks (Volk, 2011), as this tends to be produced in smaller quantities and with lower quality when trees experience long-term drought stress during the summer (Bykova et al., 2018).
As an alternative to seeds (embryonic axes) and pollen, cryopreservation of clonal tissues cultured in vitro has been feasible for some Quercus species using somatic embryos and shoot tips (Valladares et al, 2004; Vidal et al., 2010; Pence and Chaiken, 2021; Martínez et al., 2022), so the establishment of long-term cryopreserved collections of these species is feasible through these clonal tissues (Vidal et al., 2010). In addition, cryopreservation of winter dormant buds is suggested as a promising cryobiotechnology method (Ballesteros and Pritchard, 2020). While the effects of climate change on the cryopreservation of in vitro cultured somatic embryos and shoot tips are not clear (see, e.g., section “6. Climate change and ex situ conservation of RS species through shoot tips”), oak winter dormant bud cryopreservation options may be reduced due to the negative effects of climate change on their cold hardiness (see section “5. Climate change and ex situ conservation of RS species through dormant buds”).
One of our main conclusions is that climate change will have significant effects on the cryopreservation of germplasm (e.g., pollen, seed/seed embryos, dormant buds) directly harvested from field collections of RS species. Germplasm quality and physiology are fundamental for a successful cryopreservation and these attributes will likely be affected by climate change in diverse ways and intensities depending on species and locations. Climate change may not affect the genotypes conserved in vitro for many generations, but may affect new genotypes introduced in such collections. However, we cannot always generalize whether these effects are negative or positive for all species since, in most cases, they depend on the origin of the species (often tropical and temperate species are predicted to respond to climate change differently), the genotype, the adaptive genetic potential of each population, the severity of the environmental change, and the ex situ conservation option and/or explant in question (Figure 2).
Figure 2. Summary of the effects of climate change on the ex situ conservation options of recalcitrant seeded species.
In terms of field collections, RS species grown in tropical and subtropical habitats may encounter serious developmental problems related to high temperatures and water deficit (Figure 2). These problems can also affect temperate RS species growing in warm climates for which a certain level of chilling is required, but may favor the growth of these plants in northern locations where it is currently too cold for their proper development (Figure 2). Literature suggests that some of the limitations of field collections, i.e., the nursery techniques that become a maladaptive selective force on critical traits to respond to abiotic stress, can be intensified by climate change. However, we can also use these selective forces in our favor. For instance, some innovative nursery and horticultural techniques are being developed to improve desirable quality attributes concerning the response of plants to different types of stress imposed by a changing environment, which would allow us to grow these species in their current locations without the need to move field collections to higher latitudes/altitudes.
When focusing on seeds and seed tissues, increases in temperature or decreases in rainfall could cause effects on seeds related to their ex situ conservation in the short or long (hydrated storage or cryopreservation, respectively) term (Figure 2). For example, recalcitrant seeds can increase their pathogenic load, present an early germination triggered by an earlier burst of ROS, and/or low viability/longevity in storage due to the increased oxidative damage accumulated. Similarly, when embryonic axes/zygotic embryos are excised for cryopreservation, these explants may be more predisposed to contamination, or more prone to oxidative damage during excision, desiccation and recovery, which has been related to a low success of cryopreservation protocols.
Finally, we must consider the environmental effects on the mother plant regarding the seed tolerance to the stresses inferred by desiccation and low temperatures, which are key to predict the response of the embryonic axes to cryopreservation. There are two current hypotheses, one suggesting that a drier/warmer climate could favor the acquisition of desiccation tolerance and hence a better response to cryopreservation (Figure 2), while the second suggests that this tolerance is determined by the influence of cold environments. However, more data is needed to support these hypotheses and understand the potential effects of climate change on the cryopreservation of the embryonic axes of recalcitrant seeds from both tropical and temperate environments.
In terms of pollen, there is strong evidence that drought events and high temperatures affect pollen development by altering hormonal and ROS balance and sugar metabolism, ultimately limiting the amount of pollen produced and fertility attributes (Figure 1). However, there is also the possibility that better-adapted genotypes or cases of less severe water stress may generate certain acclimatization or preconditioning of the pollen as an explant for cryopreservation by making it more tolerant to desiccation due to its higher sucrose content. Nevertheless, these specific hypotheses require more evidence to ensure a beneficial effect for cryopreservation protocols due to decreased rainfall as a result of climate change.
Regarding dormant buds, significant research has emerged from a climate change perspective to assess effects on phenology in terms of regulation and release of dormancy and cold acclimation. These traits are important not only for the maintenance of field collections but also for the cryopreservation of these explants and shoot tips. Generally, dormant buds used for cryopreservation are collected during mid-winter, when they have the required levels of dormancy and cold acclimation. However, climate change could disturb these attributes concerning the season’s progress and cause the collection of dormant buds that are not acclimatized or less suitable for successfully tolerating cryopreservation protocols (Figure 2). Similarly, this could apply to shoot tips of temperate or cold-tolerant tropical species in future climate change scenarios in terms of a potential loss of cold acclimatization that would impair their performance with cryopreservation protocols.
This review is mainly based on analysis of successes and failures in applying ex situ conservation and cryopreservation protocols of RS species from contrasting environments and inferences from evidence of stress physiology and ecophysiology of orthodox-seeded species, for which much more information is available. These approaches allow us to highlight hypotheses that require more stringent testing than possible here, given the challenges and urgencies that seed recalcitrance imposes on ex situ conservation. In this sense, it is suggested to focus research efforts on the following general lines: (i) ecophysiological considerations that determine the boundary between a (beneficial) plastic acclimatization response and potentially irreversible fitness damage on propagules, mainly for species prioritized by their vulnerability in the face of climate change, (ii) possibilities of adaptive management in artificial acclimatization protocols (new or updated) in plant biotechnology, particularly in vitro culture and cryopreservation, if in situ conditions affect such suitability. Likewise, possibilities for innovation in field collections and nursery practices could yield a source of better artificially acclimatized plants, seedlings, and propagules, as long as genetic diversity is conserved.
PL-L and DB conceived the initial idea for this manuscript, which was further developed by the AF, PL-L, SC, JO, KvdW, S, and DB. All authors contributed to write the manuscript and revision, read, and approved the submitted version.
Funding was provided to JO by the ANID/FONDECYT grant 11191147 and ANID PIA/BASAL FB0002; PL-L by the FONDEQUIP Program from the Chilean National Agency for Research and Development (ANID) grant: EQM200205; and AF by the ANID, Advanced Human Capital Training Program, National Doctoral Scholarship: 21211942.
The authors declare that the research was conducted in the absence of any commercial or financial relationships that could be construed as a potential conflict of interest.
All claims expressed in this article are solely those of the authors and do not necessarily represent those of their affiliated organizations, or those of the publisher, the editors and the reviewers. Any product that may be evaluated in this article, or claim that may be made by its manufacturer, is not guaranteed or endorsed by the publisher.
Abdul Rahman, S. M., and Ellis, R. H. (2019). Seed quality in rice is most sensitive to drought and high temperature in early seed development. Seed Sci. Res. 29, 238–249. doi: 10.1017/S0960258519000217
Abrahamson, W. G., and Layne, J. N. (2003). Long-term patterns of acorn production for five oak species in xeric Florida uplands. Ecology 84, 2476–2492. doi: 10.1890/01-0707
Acevedo, M., Álvarez, C., Cartes, E., Dumroese, R. K., and González, M. (2020). Production and establishment techniques for the restoration of Nothofagus alessandrii, an endangered keystone species in a Mediterranean forest. New For. 51, 159–174. doi: 10.1007/s11056-019-09724-x
Adams-Groom, B., Selby, K., Derrett, S., Frisk, C. A., Pashley, C. H., Satchwell, J., et al. (2022). Pollen season trends as markers of climate change impact: Betula Quercus and Poaceae. Sci. Total Env. 831:154882. doi: 10.1016/j.scitotenv.2022.154882
Aizen, M. A., and Woodcock, H. (1996). Effects of acorn size on seedling survival and growth in Quercus rubra following simulated spring freeze. Canad. J. Bot. 74, 308–314. doi: 10.1139/b96-037
Alberto, F. J., Aitken, S. N., Alía, R., González-Martínez, S. C., Hänninen, H., Kremer, A., et al. (2013). Potential for evolutionary responses to climate change–evidence from tree populations. Glob. Change Biol. 19, 1645–1661. doi: 10.1111/gcb.12181
Alfonso-Corrado, C., Campos, J. E., Mendoza, A., Aguirre-Hidalgo, V., Valencia-Davalos, S., González-Adame, G., et al. (2014). Restoration-focused germination and development of five central Mexican oak species. Open J. For. 4, 171–180. doi: 10.4236/ojf.2014.43023
Amimi, N., Dussert, S., Vaissayre, V., Ghouil, H., Doulbeau, S., Costantini, C., et al. (2020). Variation in seed traits among Mediterranean oaks in Tunisia and their ecological significance. Ann. Bot. 125, 891–904. doi: 10.1093/aob/mcz211
Andivia, E., Fernández, M., and Vázquez-Piqué, J. (2014). Assessing the effect of late-season fertilization on Holm oak plant quality: Insights from morpho-nutritional characterizations and water relations parameters. New For. 45, 149–163. doi: 10.1007/s11056-013-9397-1
Andivia, E., Villar-Salvador, P., Oliet, J. A., Puértolas, J., Dumroese, R. K., Ivetić, V., et al. (2021). Climate and species stress resistance modulate the higher survival of large seedlings in forest restorations worldwide. Ecol. Appl. 31, 1–11. doi: 10.1002/eap.2394
Armstrong-Herniman, W., and Greenwood, S. (2021). The role of winter precipitation as a climatic driver of the spring phenology of five California Quercus species (Fagaceae). Madroño 68, 450–460. doi: 10.3120/0024-9637-68.4.450
Arora, R., and Taulavuori, K. (2016). Increased risk of freeze damage in woody perennials VIS-À-VIS climate change: Importance of deacclimation and dormancy response. Front. Envir. Sci. 4:44. doi: 10.3389/fenvs.2016.00044
Asare-Nuamah, P., Antwi-Agyei, P., and Dick-Sagoe, C. (2022). Mitigating the risks of climate variability and change on mango seedlings in Ghana: Evidence from mango seedlings producers in the Yilo Krobo Municipality. Environ. Chall. 8:100594. doi: 10.1016/j.envc.2022.100594
Aya, K., Ueguchi-Tanaka, M., Kondo, M., Hamada, K., Yano, K., Nishimura, M., et al. (2009). Gibberellin modulates anther development in rice via the transcriptional regulation of GAMYB W. Plant Cell 21, 1453–1472. doi: 10.1105/tpc.108.062935
Badano, E. I., and Sánchez-Montes de Oca, E. J. (2022). Seed fate, seedling establishment and the role of propagule size in forest regeneration under climate change conditions. For. Ecol. Manag. 503:119776. doi: 10.1016/j.foreco.2021.119776
Bailly, C. (2004). Active oxygen species and antioxidants in seed biology. Seed Sci. Res. 14, 93–107. doi: 10.1079/SSR2004159
Ballesteros, D., and Pence, V. C. (2017). Survival and death of seeds during liquid nitrogen storage: A case study on seeds with short lifespans. CryoLetters 38, 278–289.
Ballesteros, D., and Pritchard, H. W. (2020). The cryobiotechnology of oaks: An integration of approaches for the long-term ex situ conservation of Quercus species. Forests 11:1281. doi: 10.3390/f11121281
Baskin, C. C., and Baskin, J. M. (eds) (2022). Plant regeneration from seeds: a global warming perspective. Oxford: Academic Press.
Beil, I., Kreyling, J., Meyer, C., Lemcke, N., and Malyshev, A. V. (2021). Late to bed, late to rise—warmer autumn temperatures delay spring phenology by delaying dormancy. Glob. Change Biol. 27, 5806–5817. doi: 10.1111/gcb.15858
Ben Mariem, S., Soba, D., Zhou, B., Loladze, I., Morales, F., and Aranjuelo, I. (2021). Climate Change, crop yields, and grain quality of C3 cereals: A meta-analysis of [CO2], temperature, and drought effects. Plants 10:1052. doi: 10.3390/plants10061052
Benson, E. E., Harding, K., and Johnston, J. W. (2007). “Cryopreservation of shoot tips and meristems,” in Cryopreservation and freeze-drying protocols, eds J. G. Day and G. N. Stacey (New York, NY: Humana Press), 163–183. doi: 10.1007/978-1-59745-362-2_12
Berjak, P., and Pammenter, N. W. (1997). “Progress in the understanding and manipulation of desiccation-sensitive (recalcitrant) seeds,” in Basic and applied aspects of seed biology, eds R. H. Ellis, M. Black, A. J. Murdoch, and T. D. Hong (Dordrecht: Springer), 689–703.
Berjak, P., and Pammenter, N. W. (2008). From avicennia to zizania: Seed recalcitrance in perspective. Ann. Bot. 101, 213–228. doi: 10.1093/aob/mcm168
Berjak, P., and Pammenter, N. W. (2014). Cryostorage of germplasm of tropical recalcitrant-seeded species: Approaches and problems. Int. J. Plant Sci. 175, 29–39. doi: 10.1086/673303
Berjak, P., Farrant, J. M., and Pammenter, N. W. (1989). “The basis of recalcitrant seed behaviour,” in Recent advances in the development and germination of seeds, ed. R. B. Taylorson (New York, NY: Plenum Press), 89–105. doi: 10.1007/978-1-4613-0617-7_8
Bernareggi, G., Carbognani, M., Mondoni, A., and Petraglia, A. (2016). Seed dormancy and germination changes of snowbed species under climate warming: The role of pre-and post-dispersal temperatures. Ann. Bot. 118, 529–539. doi: 10.1093/aob/mcw125
Berthaud, J. (1997). Strategies for conservation of genetic resources in relation with their utilization. Euphytica 96, 1–12. doi: 10.1023/A:1002922220521
Bewley, J. D., Bradford, K. J., Hilhorst, H. W., and Nonogaki, H. (eds) (2013). Seeds: Physiology of development, germination and dormancy. New York, NY: Springer. doi: 10.1007/978-1-4614-4693-4
Bharuth, V., and Naidoo, C. (2020). Responses to cryopreservation of recalcitrant seeds of Ekebergia capensis from different provenances. S. Afr. J. Bot. 132, 1–14. doi: 10.1016/j.sajb.2020.04.007
Bharuth, V., Naidoo, C., Pammenter, N. W., Lamb, J. M., and Moodley, T. (2020). Responses to chilling of recalcitrant seeds of Ekebergia capensis from different provenances. S. Afr. J. Bot. 130, 8–24. doi: 10.1016/j.sajb.2019.12.001
Bonito, A., Varone, L., and Gratani, L. (2011). Relationship between acorn size and seedling morphological and physiological traits of Quercus ilex L. from different climates. Photosynthetica 49, 75–86. doi: 10.1007/s11099-011-0014-2
Bonnart, R., Waddell, J., Haiby, K., Widrlechner, M. P., and Volk, G. M. (2014). Cryopreservation of Populus trichocarpa and Salix dormant buds with recovery by grafting or direct rooting. CryoLetters 35, 507–515.
Breman, E., Ballesteros, D., Castillo-Lorenzo, E., Cockel, C., Dickie, J., Faruk, A., et al. (2021). Plant diversity conservation challenges and prospects—the perspective of botanic gardens and the Millennium Seed Bank. Plants 10:2371. doi: 10.3390/plants10112371
Buechling, A., Martin, P. H., Canham, C. D., Shepperd, W. D., and Battaglia, M. A. (2016). Climate drivers of seed production in Picea engelmannii and response to warming temperatures in the southern Rocky Mountains. J. Ecol. 104, 1051–1062. doi: 10.1111/1365-2745.12572
Buitink, J., and Leprince, O. (2004). Glass formation in plant anhydrobiotes: survival in the dry state. Cryobiology 48, 215–228. doi: 10.1016/j.cryobiol.2004.02.011
Bykova, O., Limousin, J.-M., Ourcival, J.-M., and Chuine, I. (2018). Water deficit disrupts male gametophyte development in Quercus ilex. Plant Biol. 20, 450–455. doi: 10.1111/plb.12692
Caignard, T., Kremer, A., Firmat, C., Nicolas, M., Venner, S., and Delzon, S. (2017). Increasing spring temperatures favor oak seed production in temperate areas. Sci. Rep. 7:8555. doi: 10.1038/s41598-017-09172-7
Carrero, C., Jerome, D., Beckman, E., Byrne, A., Coombes, A. J., Deng, M., et al. (2020). The red list of oaks 2020. Lisle: The Morton Arboretum.
Chand, S. S., Walsh, K. J. E., Camargo, S. J., Kossin, J. P., Tory, K. J., Wehner, M. F., et al. (2022). Declining tropical cyclone frequency under global warming. Nat. Clim. Change 12, 655–661. doi: 10.1038/s41558-022-01388-4
Chang, C. Y. Y., Bräutigam, K., Hüner, N. P., and Ensminger, I. (2021). Champions of winter survival: Cold acclimation and molecular regulation of cold hardiness in evergreen conifers. New Phytol. 229, 675–691. doi: 10.1111/nph.16904
Chaturvedi, P., Wiese, A. J., Ghatak, A., Záveská Drábková, L., Weckwerth, W., and Honys, D. (2021). Heat stress response mechanisms in pollen development. New Phytol. 231, 571–585. doi: 10.1111/nph.17380
Chaudhury, R., Malik, S. K., and Rajan, S. (2010). An improved pollen collection and cryopreservation method for highly recalcitrant tropical fruit species of Mango (Mangifera indica L.) and Litchi (Litchi chinensis Sonn.). CryoLetters 31, 268–278.
Cha-um, S., and Kirdmanee, C. (2007). Minimal growth in vitro culture for preservation of plant species. Fruit Veg. Cereal Sci. Biotechnol. 1, 13–25.
Cibrian-Jaramillo, A., Hird, A., Oleas, N., Ma, H., Meerow, A. W., Francisco-Ortega, J., et al. (2013). What is the conservation value of a plant in a botanic garden? Using indicators to improve management of ex situ collections. Bot. Rev. 79, 559–577. doi: 10.1007/s12229-013-9120-0
Contreras, S., Bennett, M. A., and Tay, D. (2009). Temperature during seed development affects weight, germinability, and storability of lettuce seeds. Seed Sci. Technol. 37, 398–412. doi: 10.15258/sst.2009.37.2.13
Cooke, J. E., Eriksson, M. E., and Junttila, O. (2012). The dynamic nature of bud dormancy in trees: Environmental control and molecular mechanisms. Plant Cell Env. 35, 1707–1728. doi: 10.1111/j.1365-3040.2012.02552.x
Cooper, H. F., Grady, K. C., Cowan, J. A., Best, R. J., Allan, G. J., and Whitham, T. G. (2019). Genotypic variation in phenological plasticity: Reciprocal common gardens reveal adaptive responses to warmer springs but not to fall frost. Glob. Change Biol. 25, 187–200. doi: 10.1111/gcb.14494
Cousins, S. R., and Witkowski, E. T. F. (2017). African cycad ecology, ethnobotany and conservation: A synthesis. Bot. Rev. 83, 152–194. doi: 10.1007/s12229-017-9183-4
Davis, A. S., and Jacobs, D. F. (2005). Quantifying root system quality of nursery seedlings and relationship to outplanting performance. New For. 30, 295–311. doi: 10.1007/s11056-005-7480-y
Daws, M. I., Cleland, H., Chmielarz, P., Gorian, F., Leprince, O., Mullins, C. E., et al. (2006). Variable desiccation tolerance in Acer pseudoplatanus seeds in relation to developmental conditions: A case of phenotypic recalcitrance? Funct. Plant Biol. 33, 59–66. doi: 10.1071/FP04206
Daws, M. I., Lydall, E., Chmielarz, P., Leprince, O., Matthews, S., Thanos, C. A., et al. (2004). Developmental heat sum influences recalcitrant seed traits in Aesculus hippocastanum across Europe. New Phytol. 162, 157–166. doi: 10.1111/j.1469-8137.2004.01012.x
de Almeida Garcia Rodrigues, G., da Silva, D., Ribeiro, M. I., Loaiza-Loaiza, O. A., Alcantara, S., Komatsu, R. A., et al. (2022). What affects the desiccation tolerance threshold of Brazilian Eugenia (Myrtaceae) seeds? J. Plant Res. 135, 579–591. doi: 10.1007/s10265-022-01396-7
De Storme, N., and Geelen, D. (2014). The impact of environmental stress on male reproductive development in plants: Biological processes and molecular mechanisms. Plant Cell Envir. 37, 1–18. doi: 10.1111/pce.12142
Delgado-Vargas, V. A., Magdaleno-Villar, J. J., Ayala-Garay, ÓJ., and Garfias-Sánchez, D. (2018). Seed quality of three native tomato varieties and a commercial one produced under high temperatures. Rev. Chapingo Serie Hort. 24, 215–227. doi: 10.5154/r.rchsh.2018.04.009
Delouche, J. C. (1980). Environmental effects on seed development and seed quality. HortScience 15, 13–18.
Delph, L. F., Johannsson, M. H., and Stephenson, A. G. (1997). How environmental factors affect pollen performance: Ecological and evolutionary perspectives. Ecology 78, 1632–1639.
Denk, T., Grimm, G. W., Manos, P. S., Deng, M., and Hipp, A. L. (2017). “An updated infrageneric classification of the oaks: Review of previous taxonomic schemes and synthesis of evolutionary patterns,” in Oaks physiological ecology. Exploring the functional diversity of genus L, Quercus eds E. Gil-Pelegrín, J. Peguero-Pina, and D. Sancho-Knapik (Cham: Springer), 13–38. doi: 10.1007/978-3-319-69099-5_2
Dinato, N. B., Santos, I. R. I., Vigna, B. B. Z., de Paula, A. F., and Fávero, A. P. (2020). Pollen cryopreservation for plant breeding and genetic resources conservation. CryoLetters 41, 115–127.
Dinesh, M. R., and Reddy, B. M. C. (2012). “Physiological basis of growth and fruit yield characteristics of tropical and sub-tropical fruits to temperature,” in Tropical fruit tree species and climate change, eds B. R. Sthapit, V. Ramanatha Rao, and S. R. Sthapit (New Delhi: Bioversity International), 45–70.
Dinu, D. G., Ricciardi, V., Demarco, C., Zingarofalo, G., De Lorenzis, G., Buccolieri, R., et al. (2021). Climate change impacts on plant phenology: Grapevine (Vitis vinifera) bud break in wintertime in Southern Italy. Foods 10:2769. doi: 10.3390/foods10112769
Dong, B., Zheng, X., Liu, H., Able, J. A., Yang, H., Zhao, H., et al. (2017). Effects of drought stress on pollen sterility, grain yield, abscisic acid and protective enzymes in two winter wheat cultivars. Front. Plant Sci. 8:1008. doi: 10.3389/fpls.2017.01008
Ducousso, A., Michaud, H., and Lumaret, R. (1993). Reproduction and gene flow in the genus Quercus L. Ann. Sci. For. 50(Suppl. 1), 91S–106S. doi: 10.1051/forest:19930708
Dussert, S., Chabrillange, N., Engelmann, F., Anthony, F., Louarn, J., and Hamon, S. (2000). Relationship between seed desiccation sensitivity, seed water content at maturity and climatic characteristics of native environments of nine Coffea L. species. Seed Sci. Res. 10, 293–300. doi: 10.1017/S096025859900015X
Edlund, A. F., Swanson, R., and Preuss, D. (2004). Pollen and stigma structure and function: The role of diversity in pollination. Plant Cell 16, S84–S97. doi: 10.1105/tpc.015800
Ellis, R. H., Hong, T. D., and Roberts, E. H. (1991). An intermediate category of seed storage behaviour? II. Effects of provenance, immaturity, and imbibition on desiccation-tolerance In Coffee. J. Exp. Bot. 42, 653–657. doi: 10.1093/jxb/42.5.653
El-Maarouf-Bouteau, H., and Bailly, C. (2008). Oxidative signalling in seed germination and dormancy. Plant Sign. Behav. 3, 175–182. doi: 10.4161/psb.3.3.5539
Engelmann, F. (2011). Use of biotechnologies for the conservation of plant biodiversity. In Vitro Cell. Dev. Biol. Plant 47, 5–16. doi: 10.1007/s11627-010-9327-2
Engelmann, F., and Engels, J. M. M. (2002). “Technologies and strategies for ex situ conservation,” in Managing plant genetic diversity, eds J. M. M. Engels, V. Ramanatha, A. H. D. Brown, and M. T. Jackson (Rome: International Plant Genetic Resources Institute), 89–103.
Ensslin, A., and Godefroid, S. (2019). How the cultivation of wild plants in botanic gardens can change their genetic and phenotypic status and what this means for their conservation value. Sibbaldia 17, 51–69. doi: 10.7892/boris.127313
Fadón, E., and Rodrigo, J. (2018). Unveiling winter dormancy through empirical experiments. Env. Exp. Bot. 152, 28–36. doi: 10.1016/j.envexpbot.2017.11.006
Fadón, E., Fernandez, E., Behn, H., and Luedeling, E. (2020). A conceptual framework for winter dormancy in deciduous trees. Agronomy 10:241. doi: 10.3390/agronomy10020241
Fahad, S., Sonmez, O., Saud, S., Wang, D., Wu, C., Adnan, M., et al. (eds) (2021). Climate change and plants: Biodiversity, growth and interactions. Boca Raton, FL: CRC Press.
Farrant, J. M., Berjak, P., and Pammenter, N. W. (1985). The effect of drying rate on viability retention of recalcitrant propagules of Avicennia marina. S. Afr. J. Bot. 51, 432–438. doi: 10.1016/S0254-6299(16)31621-0
Fenner, M. (1991). The effects of the parent environment on seed germinability. Seed Sci. Res. 1, 75–84. doi: 10.1017/S0960258500000696
Fernando, D. D., Richards, J. L., and Kikkert, J. R. (2006). In vitro germination and transient GFP expression of American chestnut (Castanea dentata) pollen. Plant Cell Rep. 25, 450–456. doi: 10.1007/s00299-005-0088-z
Finch-Savage, W. E., and Blake, P. S. (1994). Indeterminate development in desiccation-sensitive seeds of Quercus robur L. Seed Sci. Res. 4, 127–133. doi: 10.1017/S0960258500002129
Folgado, R., and Panis, B. (2019). “Cryopreservation of ashe magnolia shoot-tips by droplet vitrification” in III international symposium on plant cryopreservation. Acta Hortic. 1234, 233–240. doi: 10.17660/ActaHortic.2019.1234.31
Folgado, R., Panis, B., Sergeant, K., Renaut, J., Swennen, R., and Hausman, J. F. (2015). Unravelling the effect of sucrose and cold pretreatment on cryopreservation of potato through sugar analysis and proteomics. Cryobiology 71, 432–441. doi: 10.1016/j.cryobiol.2015.09.006
Franchi, G. G., Piotto, B., Nepi, M., Baskin, C. C., Baskin, J. M., and Pacini, E. (2011). Pollen and seed desiccation tolerance in relation to degree of developmental arrest, dispersal, and survival. J. Exp. Bot. 62, 5267–5281. doi: 10.1093/jxb/err154
Funnekotter, B., Colville, L., Kaczmarczyk, A., Turner, S. R., Bunn, E., and Mancera, R. L. (2017). Monitoring of oxidative status in three native Australian species during cold acclimation and cryopreservation. Plant Cell Rep. 36, 1903–1916. doi: 10.1007/s00299-017-2204-2
Ganatsas, P., and Tsakaldimi, M. (2013). A comparative study of desiccation responses of seeds of three drought-resistant Mediterranean oaks. For. Ecol. Manag. 305, 189–194. doi: 10.1016/j.foreco.2013.05.042
Ganatsas, P., Tsakaldimi, M., Zarkadi, P., and Stergiou, D. (2016). Intraspecific differences in the response to drying of Quercus ithaburensis acorns. Plant Biosyst. 151, 1–9. doi: 10.80/11263504.2016.1219415
Gao, S., Ren, Y., Masabni, J., Zou, F., Xiong, H., and Zhu, J. (2021). Influence of geographical and Climatic factors on Quercus variabilis blume fruit phenotypic diversity. Diversity 13:329. doi: 10.3390/d13070329
García-Cruzatty, L. C., Vera-Pinargote, L., Zambrano-Gavilanes, F., Zamora-Macías, A., and Cedeño-Ortega, J. (2020). Pollen production in Theobroma cacao L. Genotypes national type and CCN-51 and Its relationship with climatic factors on the ecuadorian coast. Acta Agrobot. 73:7323. doi: 10.5586/aa.7323
García-Mozo, H., López-Orozco, R., Oteros, J., and Galán, C. (2022). Factors driving autumn Quercus flowering in a thermo-Mediterranean area. Agronomy 12:2596. doi: 10.3390/agronomy12112596
Garreaud, R. D., Boisier, J. P., Rondanelli, R., Montecinos, A., Sepúlveda, H. H., and Veloso-Aguila, D. (2020). The central chile mega drought (2010–2018): A climate dynamics perspective. Int. J. Climatol. 40, 421–439. doi: 10.1002/joc.6219
Gavinet, J., Ourcival, J. M., and Limousin, J. M. (2019). Rainfall exclusion and thinning can alter the relationships between forest functioning and drought. New Phytol. 223, 1267–1279. doi: 10.1111/nph.15860
Gavranović Markić, A., Bogdan, S., Gradečki Poštenjak, M., Lanšćak, M., Vujnović, Z., Bogunović, S., et al. (2022). Acorn yields and seed viability of pedunculate oak in a 10-year period in forest seed objects across Croatia. South East Eur. For. 13, 27–36. doi: 10.15177/seefor.22-01
Gerst, K. L., Rossington, N. L., and Mazer, S. J. (2017). Phenological responsiveness to climate differs among four species of Quercus in North America. J. Ecol. 105, 1610–1622. doi: 10.1111/1365-2745.12774
Gómez-Casero, M. T., Galán, C., and Vilches, E. D. (2007). Flowering phenology of Mediterranean “Quercus” species in different locations (Córdoba, SW Iberian Peninsula). Acta Bot. Malacit. 32, 127–146.
González-Orozco, C. E., Porcel, M., Rodriguez-Medina, C., and Yockteng, R. (2022). Extreme climate refugia: a case study of wild relatives of cacao (Theobroma cacao) in Colombia. Biodivers. Conserv. 31, 161–182. doi: 10.1007/s10531-021-02327-z
Goveia, M., Kioko, J. I., and Berjak, P. (2004). Developmental status is a critical factor in the selection of excised recalcitrant axes as explants for cryopreservation: A study on Trichilia dregeana Sond. Seed Sci. Res. 14, 241–248. doi: 10.1079/SSR2004173
Griffith, M. P., Calonje, M., Meerow, A. W., Tut, F., Kramer, A. T., Hird, A., et al. (2014). Can a botanic garden cycad collection capture the genetic diversity in a wild population? Int. J. Plant Sci. 176, 1–10. doi: 10.1086/678466
Grout, B. W. W., and Roberts, A. V. (1995). “Storage of free pollen, pollen embryos and the zygotic embryos of seed by cryopreservation and freeze drying,” in Genetic preservation of plant cells in vitro, ed. B. Grout (Berlin: Springer), 63–74. doi: 10.1007/978-3-642-78661-7_5
Guo, C., Yao, L., You, C., Wang, S., Cui, J., Ge, X., et al. (2016). MID1 plays an important role in response to drought stress during reproductive development. Plant J. 88, 280–293. doi: 10.1111/tpj.13250
Gutterman, Y. (2000). “Maternal effects on seeds during development,” in Seeds: The ecology of regeneration in plant communities, ed. M. Fenner (Wallingford: CAB International), 59–84.
Haavik, L. J., Billings, S. A., Guldin, J. M., and Stephen, F. M. (2015). Emergent insects, pathogens and drought shape changing patterns in oak decline in North America and Europe. For. Ecol. Manag. 354, 190–205. doi: 10.1016/j.foreco.2015.06.019
Hänninen, H., and Tanino, K. (2011). Tree seasonality in a warming climate. Trends Plant Sci 16, 412–416. doi: 10.1016/j.tplants.2011.05.001
Hatzig, S. V., Nuppenau, J., Snowdon, R. J., and Schießl, S. V. (2018). Drought stress has transgenerational effects on seeds and seedlings in winter oilseed rape (Brassica napus L.). BMC Plant Biol. 18:297. doi: 10.1186/s12870-018-1531-y
Hebbar, K. B., Abhin, P. S., Sanjo Jose, V., Neethu, P., Santhosh, A., Shil, S., et al. (2022). Predicting the potential suitable climate for coconut (Cocos nucifera L.) Cultivation in india under climate change scenarios using the MaxEnt model. Plants 11:731. doi: 10.3390/plants11060731
Hebbar, K., Rose, H., Nair, A., Kannan, S., Niral, V., Arivalagan, M., et al. (2018). Differences in in vitro pollen germination and pollen tube growth of coconut (Cocos nucifera L.) cultivars in response to high temperature stress. Environ. Exp. Bot. 153, 35–44. doi: 10.1016/j.envexpbot.2018.04.014
Hedhly, A. (2011). Sensitivity of flowering plant gametophytes to temperature fluctuations. Environ. Exp. Bot. 74, 9–16. doi: 10.1016/j.envexpbot.2011.03.016
Hu, L., Liang, W., Yin, C., Cui, X., Zong, J., Wang, X., et al. (2011). Rice MADS3 Regulates ROS Homeostasis during Late Anther Development. Plant Cell 23, 515–533. doi: 10.1105/tpc.110.074369
Hu, M., Li, Y., Zhang, X., Song, W., Jin, W., Huang, W., et al. (2022). DRP1 encodes a desiccation-related protein that is critical for Ubisch bodies and pollen exine development in maize. J. Exp. Bot. 73, 6800–6815. doi: 10.1093/jxb/erac331
IPCC (2021). “Climate change 2021: The physical science basis,” in Contribution of working group I to the sixth assessment report of the intergovernmental panel on climate change, eds V. Masson-Delmotte, P. Zhai, A. Pirani, S. L. Connors, C. Péan, S. Berger, et al. (Cambridge: Cambridge University Press). doi: 10.1017/9781009157896
IUCN (2005). Report and recommendations on cycad aulacaspis scale, aulacaspis yasumatsui takagi (Hemiptera: Diaspididae). Available online at: https://www.fs.usda.gov/Internet/FSE_DOCUMENTS/stelprdb5437896.pdf (Accessed November 26, 2022).
Jenderek, M. M., Forsline, P., Postman, J., Stover, E., and Ellis, D. (2011). Effect of geographical location, year, and cultivar on survival of Malus sp. dormant buds stored in vapors of liquid nitrogen. HortScience 46, 1230–1234. doi: 10.21273/HORTSCI.46.9.1230
Jin, Y., Yang, H., Wei, Z., Ma, H., and Ge, X. (2013). Rice male development under drought stress: Phenotypic changes and stage-dependent transcriptomic reprogramming. Mol. Plant 6, 1630–1645. doi: 10.1093/mp/sst067
Joët, T., Ourcival, J. M., and Dussert, S. (2013). Ecological significance of seed desiccation sensitivity in Quercus ilex. Ann. Bot. 111, 693–701. doi: 10.1093/aob/mct025
Kermode, A. R., and Finch-Savage, W. E. (2002). “Desiccation sensitivity in orthodox and recalcitrant seeds in relation to development,” in Desiccation and survival in plants: Drying without dying, eds M. Black and H. W. Pritchard (Wallingford: CABI Publishing), 149–184.
Kinoshita-Tsujimura, K., and Kakimoto, T. (2011). Cytokinin receptors in sporophytes are essential for male and female functions in Arabidopsis thaliana. Plant Sign. Behav. 6, 66–71. doi: 10.4161/psb.6.1.13999
Klupczyńska, E. A., and Pawłowski, T. A. (2021). Regulation of seed dormancy and germination mechanisms in a changing environment. Int. J. Mol. Sci. 22:1357. doi: 10.3390/ijms22031357
Koenig, W. D., Alejano, R., Carbonero, M. D., Fernández-Rebollo, P., Knops, J. M. H., Marañón, T., et al. (2016). Is the relationship between mast- seeding and weather in oaks related to their life- history or phylogeny? Ecology 97, 2603–2615. doi: 10.1002/ecy.1490
Krishnapillay, B., Tsan, F. Y., Marzalina, M., Jayanthi, N., and Nashatul Zaimah, N. A. (1999). “Slow growth as a method to ensure continuous supply of planting materials for recalcitrant seeded species,” in Proceedings of the IUFRO seed symposium “recalcitrant seeds” at kuala lumpur, malaysia, 12-15 october 1998, eds M. Marzalina, K. C. Khoo, N. Jayanthi, F. Y. Tsan, and B. Krishnapillay (Kuala Lumpur: Forest Research Institute Malaysia), 280–285.
Kulus, D. (2020). Cryopreservation of bleeding heart (Lamprocapnos spectabilis (L.) Fukuhara) shoot tips using encapsulation-dehydration. CryoLetters 41, 75–85.
Kushnarenko, S. V., Romadanova, N. V., and Reed, B. M. (2009). Cold acclimation improves regrowth of cryopreserved apple shoot tips. CryoLetters 30, 47–54.
Laidlaw, M. J., and Forster, P. I. (2012). Climate predictions accelerate decline for threatened Macrozamia cycads from Queensland, Australia. Biology 1, 880–894. doi: 10.3390/biology1030880
Lamarca, E. V., and Barbedo, C. J. (2015). Sensibilidade à dessecação de embriões de inga vera willd. Formados sob diferentes condições ambientais. Rev. Árvore 39, 1083–1092. doi: 10.1590/0100-67622015000600011
Lamarca, E. V., Camargo, M., Teixeira, S., Silva, E., Faria, J., and Barbedo, C. J. (2016). Variations in desiccation tolerance in seeds of Eugenia pyriformis: dispersal at different stages of maturation. Rev. Cienc. Agron. 47, 118–126. doi: 10.5935/1806-6690.20160014
Le Roncé, I., Gavinet, J., Ourcival, J.-M., Mouillot, F., Chuine, I., and Limousin, J.-M. (2021). Holm oak fecundity does not acclimate to a drier world. New Phytol. 231, 631–645. doi: 10.1111/nph.17412
León-Lobos, P., and Ellis, R. (2018). Comparison of seed desiccation sensitivity amongst Castanea sativa, Quercus ilex and Q. cerris. Seed Sci. Technol. 46, 233–237. doi: 10.15258/sst.2018.46.2.05
Li, D. Z., and Pritchard, H. W. (2009). The science and economics of ex situ plant conservation. Trends Plant Sci. 14, 614–621. doi: 10.1016/j.tplants.2009.09.005
Litz, R. E., Moon, P. A., Benson, E. M., Stewart, J., and Chávez, V. M. (2004). A biotechnology strategy for medium- and long-term conservation of Cycads. Bot. Rev. 70, 39–46.
Liu, D., Ogaya, R., Barbeta, A., Yang, X., and Peñuelas, J. (2015). Contrasting impacts of continuous moderate drought and episodic severe droughts on the aboveground-biomass increment and litterfall of three coexisting Mediterranean woody species. Glob. Change Biol. 21, 4196–4209. doi: 10.1111/gcb.13029
Liu, X., Xiao, Y., Zi, J., Yan, J., Li, C., Du, C., et al. (2023). Differential effects of low and high temperature stress on pollen germination and tube length of mango (Mangifera indica L.) genotypes. Sci. Rep. 13:611. doi: 10.1038/s41598-023-27917-5
Llanderal-Mendoza, J., Gugger, P. F., Oyama, K., Uribe-Salas, D., and González-Rodríguez, A. (2017). Climatic determinant of acorn size and germination percentage of Quercus rugosa (Fagaceae) along a latitudinal gradient in Mexico. Bot. Sci. 95, 37–47. doi: 10.17129/botsci.640
Lloret, F. C., Casanovas, C., and Peñuelas, J. (1999). Seedling survival of Mediterranean shrubland species in relation to root: shoot ration, seed size and water and nitrogen use. Funct. Ecol. 13, 210–216. doi: 10.1046/j.1365-2435.1999.00309.x
Lobdell, M. S., and Thompson, P. G. (2017). “Ex-situ conservation of Quercus oglethorpensis in living collections of Arboreta and Botanical Gardens,” in Gene conservation of tree species -banking on the future. Proceedings of a workshop, eds R. A. Sniezko, G. Man, V. Hipkins, K. Woeste, D. Gwaze, J. T. Kliejunas, et al. (Portland, OR: US Department of Agriculture, Forest Service), 144–153.
López-Orozco, R., García-Mozo, H., Oteros, J., and Galán, C. (2021). Long-term trends in atmospheric Quercus pollen related to climate change in southern Spain: A 25-year perspective. Atmos. Environ. 262:118637. doi: 10.1016/j.atmosenv.2021.118637
Luedeling, E. (2012). Climate change impacts on winter chill for temperate fruit and nut production: A review. Sci. Hortic. 144, 218–229. doi: 10.1016/j.scienta.2012.07.011
Luedeling, E., Zhang, M., Luedeling, V., and Girvetz, E. H. (2009). Sensitivity of winter chill models for fruit and nut trees to climatic changes expected in California’s Central Valley. Agric. Ecosyst. Environ. 133, 23–31. doi: 10.1016/j.agee.2009.04.016
Ma, Q., Hänninen, H., Berninger, F., Li, X., and Huang, J. G. (2022). Climate warming leads to advanced fruit development period of temperate woody species but divergent changes in its length. Glob. Chang. Biol. 28, 6021–6032. doi: 10.1111/gcb.16357
Mach, J. (2012). Open wide! Exine patterning and aperture formation in Arabidopsis pollen. Plant Cell 24:4311. doi: 10.1105/tpc.112.241110
Magni, C. R., Saavedra, N., Espinoza, S. E., Yañez, M., Quiroz, I. A., Faúndez, A., et al. (2022). The recruitment of the recalcitrant-seeded Cryptocarya alba (Mol.) looser, established via direct seeding is mainly affected by the seed source and forest cover. Plants 11:2918. doi: 10.3390/plants11212918
Marler, T., Lindstrom, A., and Watson, G. (2021). Aulacaspis yasumatsui delivers a blow to international cycad horticulture. Horticulturae 7:147. doi: 10.3390/horticulturae7060147
Martínez, M. T., Suárez, S., Moncaleán, P., and Corredoira, E. (2022). Cryopreservation of holm oak embryogenic cultures for long-term conservation and assessment of polyploid stability. Plants 11:1266. doi: 10.3390/plants11091266
Mathew, L., McLachlan, A., Jibran, R., Burritt, D. J., and Pathirana, R. (2018). Cold, antioxidant and osmotic pre-treatments maintain the structural integrity of meristematic cells and improve plant regeneration in cryopreserved kiwifruit shoot tips. Protoplasma 255, 1065–1077. doi: 10.1007/s00709-018-1215-3
Matsuda, H., and Higuchi, H. (2017). Effects of temperature and humidity on lychee (Litchi chinensis Sonn.) Pollen germination during anther dehiscence. Trop.l Agric. Dev. 61, 62–69.
McDowell, N., Pockman, W. T., Allen, C. D., Breshears, D. D., Cobb, N., Kolb, T., et al. (2008). Mechanisms of plant survival and mortality during drought: Why do some plants survive while others succumb to drought? New Phytol. 178, 719–739. doi: 10.1111/j.1469-8137.2008.02436.x
Mena-Montoya, M., García-Cruzatty, L. C., Cuenca-Cuenca, E., Pinargote, L. D. V., Villamar-Torres, R., and Jazayeri, S. M. (2020). Pollen flow of Theobroma cacao and its relationship with climatic factors in the central zone of the Ecuadorian littoral. Bioagro 32, 39–48.
Moothoo-Padayachie, A., Varghese, B., Pammenter, N. W., Govender, P., and Sershen (2018). A comparison of partial dehydration and hydrated storage-induced changes in viability, reactive oxygen species production, and glutathione metabolism in two contrasting recalcitrant-seeded species. Acta Physiol. Plant. 40, 1–10. doi: 10.1007/s11738-017-2589-0
Mostert, E., Gaertner, M., Holmes, P. M., Rebelo, A. G., and Richardson, D. M. (2017). Impacts of invasive alien trees on threatened lowland vegetation types in the Cape Floristic Region, South Africa. S. Afr. J. Bot. 108, 209–222. doi: 10.1016/j.sajb.2016.10.014
Müller, K., Linkies, A., Vreeburg, R., Fry, S., Krieger-Liszkay, A., and Leubner-Metzger, G. (2009). In vivo cell wall loosening by hydroxyl radicals during cress seed germination and elongation growth. Plant Physiol. 150, 1855–1865. doi: 10.1104/pp.109.139204
Muniappan, R., Watson, G. W., Evans, G. A., Rauf, A., and Natalia Von, E. (2012). Cycad aulacaspis scale, a newly introduced insect pest in indonesia. HAYATI J. Biosci. 19, 110–114. doi: 10.4308/hjb.19.3.110
Mycock, D. J., and Berjak, P. (1990). Fungal contaminants associated with several homoiohydrous (recalcitrant) seed species. Phytophylactica 22, 413–418.
Mycock, D. J., and Berjak, P. (1995). “The implications of seed-associated mycoflora during storage,” in Seed development and germination, eds J. Kigel and G. Galili (New York, NY: Marcel Dekker), 747–766.
Nadarajan, J., Benson, E. E., Xaba, P., Harding, K., Lindstrom, A., Donaldson, J., et al. (2018). Comparative biology of cycad pollen, seed and tissue – A plant conservation perspective. Bot. Rev. 84, 295–314. doi: 10.1007/s12229-018-9203-z
Nadarajan, J., Mansor, M., Krishnapillay, B., Staines, H. J., Benson, E. E., and Harding, K. (2008). Applications of differential scanning calorimetry in developing cryopreservation strategies for Parkia speciosa, a tropical tree producing recalcitrant seeds. CryoLetters 29, 95–110.
Nepi, M., Franchi, G. G., and Padni, E. (2001). Pollen hydration status at dispersal: Cytophysiological features and strategies. Protoplasma 216, 171–180. doi: 10.1007/BF02673869
Nesamari, R., Millar, I., Coutinho, T. A., and Roux, J. (2015). South African cycads at risk: Aulacaspis yasumatsui (Hemiptera: Coccoidea: Diaspididae) in South Africa. Afr. Entomol. 23, 196–206.
Nixon, K. C. (2006). “Global and neotropical distribution and diversity of oak (genus Quercus) and oak forests,” in Ecology and conservation of neotropical montane oak forests, ed. M. Kappelle (Berlin: Springer), 3–13. doi: 10.1007/3-540-28909-7_1
Normah, M. N., Sulong, N., and Reed, B. M. (2019). Cryopreservation of shoot tips of recalcitrant and tropical species: Advances and strategies. Cryobiology 87, 1–14. doi: 10.1016/j.cryobiol.2019.01.008
Ntuli, T. M., Finch-Savage, W. E., Berjak, P., and Pammenter, N. W. (2011). Increased drying rate lowers the critical water content for survival in embryonic axes. of English oak (Quercus robur L.) seeds. J. Integr. Plant Biol. 53, 270–280. doi: 10.1111/j.1744-7909.2010.01016.x
Okubamichael, D. Y., Jack, S., De Wet Bösenberg, J., Timm Hoffman, M., and Donaldson, J. S. (2016). Repeat photography confirms alarming decline in South African cycads. Biodivers. Conserv. 25, 2153–2170. doi: 10.1007/s10531-016-1183-x
Oliet, J., Planelles, R., Artero, F., Valverde, R., Jacobs, D. F., and Segura, M. L. (2009). Field performance of Pinus halepensis planted in Mediterranean arid conditions: relative influence of seedling morphology and mineral nutrition. New For. 37, 313–331. doi: 10.1007/s11056-008-9126-3
Oliet, J., Puértolas, J., Planelles, R., and Jacobs, D. F. (2013). Nutrient loading of forest tree seedlings to promote stress resistance and field performance: A Mediterranean perspective. New For. 44, 649–669. doi: 10.1007/s11056-013-9382-8
Ooi, M. K. (2012). Seed bank persistence and climate change. Seed Sci. Res. 22, S53–S60. doi: 10.1017/S0960258511000407
Ooi, M. K. J., Auld, T. D., and Denham, A. J. (2009). Climate change and bet-hedging: Interactions between increased soil temperatures and seed bank persistence. Glob Chang Biol. 15, 2375–2386. doi: 10.1111/j.1365-2486.2009.01887.x
Osborne, R., Robbertse, P. J., and Claassen, M. I. (1992). The longevity of cycad pollen in storage. S. Afr. J. Bot. 58, 250–254. doi: 10.1016/S0254-6299(16)30842-0
Ovalle, J. F., Arellano, E. C., Ginocchio, R., and Becerra, P. (2016a). Fertilizer location modifies root zone salinity, root morphology, and water-stress resistance of tree seedlings according to the watering regime in a dryland reforestation. J. Plant Nutr. Soil Sci. 179, 223–233. doi: 10.1002/jpln.201500181
Ovalle, J. F., Arellano, E. C., Oliet, J. A., Becerra, P., and Ginocchio, R. (2016b). Linking nursery nutritional status and water availability post-planting under intense summer drought: The case of a South American mediterranean tree species. IFOREST 9, 758–765. doi: 10.3832/ifor1905-009
Pacini, E., Guarnieri, M., and Nepi, M. (2006). Pollen carbohydrates and water content during development, presentation, and dispersal: A short review. Protoplasma 228, 73–77. doi: 10.1007/S00709-006-0169-Z
Pammenter, N. W., and Berjak, P. (2014). Physiology of desiccation-sensitive (recalcitrant) seeds and the implications for cryopreservation. Int. J. Plant Sci. 175, 21–28. doi: 10.1086/673302
Pammenter, N. W., Berjak, P., Farrant, J. M., Smith, M. T., and Ross, G. (1994). Why do stored hydrated recalcitrant seeds die? Seed Sci. Res. 4, 187–191. doi: 10.1017/S0960258500002178
Parmesan, C., and Hanley, M. E. (2015). Plants and climate change: Complexities and surprises. Ann. Bot. 116, 849–864. doi: 10.1093/aob/mcv169
Pence, V. C. (2011). Evaluating costs for the in vitro propagation and preservation of endangered plants. In Vitro Cell. Dev. Biol. 47, 176–187. doi: 10.1007/s11627-010-9323-6
Pence, V. C., and Bruns, E. B. (2022). The tip of the iceberg: Cryopreservation needs for meeting the challenge of exceptional plant conservation. Plants 11, 1–15. doi: 10.3390/plants11121528
Pence, V. C., and Chaiken, M. F. (2021). Shoot tip cryopreservation as a conservation tool for species of Quercus: Effects of species and environment on recovery. CryoLetters 42, 159–167.
Pence, V. C., Ballesteros, D., Walters, C., Reed, B. M., Philpott, M., Dixon, K. W., et al. (2020). Cryobiotechnologies: Tools for expanding long-term ex situ conservation to all plant species. Biol. Conserv. 250, 108736. doi: 10.1016/j.biocon.2020.108736
Pence, V. C., Meyer, A., Linsky, J., Gratzfeld, J., Pritchard, H. W., Westwood, M., et al. (2022). Defining exceptional species—A conceptual framework to expand and advance ex situ conservation of plant diversity beyond conventional seed banking. Biol. Conserv. 266:109440.
Pérez-Ramos, I. M., Padilla-Díaz, C. M., Koenig, W. D., and Marañón, T. (2015). Environmental drivers of mast-seeding in Mediterranean oak species: does leaf habit matter? J. Ecol. 103, 691–700. doi: 10.1111/1365-2745.12400
Philpott, M., Pence, V. C., and Coffey, E. E. D. (2022). Building capacity in the conservation of exceptional plant species. Appl. Plant Sci. 10:e11498. doi: 10.1002/aps3.11498
Pieta Filho, C. P., and Ellis, R. H. (1991). The development of seed quality in spring barley in four environments. I. Germination and longevity. Seed Sci. Res. 1, 163–177. doi: 10.1017/S096025850000083
Poggi, G. M., Aloisi, I., Corneti, S., Esposito, E., Naldi, M., Fiori, J., et al. (2022). Climate change effects on bread wheat phenology and grain quality: A case study in the north of Italy. Front. Plant Sci. 13:936991. doi: 10.3389/fpls.2022.936991
Popova, E. V., Kim, D. H., Han, S. H., Pritchard, H. W., and Lee, J. C. (2012). Narrowing of the critical hydration window for cryopreservation of salix caprea seeds following ageing and a reduction in vigour. CryoLetters 33, 219–230.
Primack, R. B., and Miller-Rushing, A. (2009). The role of botanical gardens in climate change research. New Phytol. 182, 303–313. doi: 10.1111/j.1469-8137.2009.02800.x
Primack, R. B., Ellwood, E. R., Gallinat, A. S., and Miller-Rushing, A. J. (2021). The growing and vital role of botanical gardens in climate change research. New Phytol. 231, 917–932. doi: 10.1111/nph.17410
Pritchard, H. W., Haye, A. J., Wright, W. J., and Steadman, K. J. (1995). A comparative study of seed viability in Inga species: Desiccation tolerance in relation to the physical characteristics and chemical composition of the embryo. Seed Sci. Technol. 23, 85–100.
Pritchard, H. W., Sershen Tsan, F. Y., Wen, B., Jaganathan, G. K., Calvi, G., et al. (2022). “Regeneration in recalcitrant-seeded species and risks from climate change,” in Plant regeneration from seeds: A global warming perspective, eds C. C. Baskin and J. M. Baskin (Oxford: Academic Press), 259–273. doi: 10.1016/B978-0-12-823731-1.00014-7
Probert, R. J. (2000). “The role of temperature in the regulation of seed dormancy and germination,” in Seed, the ecology of regeneration in plant communities, ed. M. Fenner (Wallingford: CABI Publishing), 261–292. doi: 10.1079/9780851994321.0261
Probert, R. J., Daws, M. I., and Hay, F. R. (2009). Ecological correlates of ex situ seed longevity: A comparative study on 195 species. Ann. Bot. 104, 57–69. doi: 10.1093/aob/mcp082
Pukacka, S., and Ratajczak, E. (2005). Production and scavenging of reactive oxygen species in Fagus sylvatica seeds during storage at varied temperature and humidity. J. Plant Physiol. 162, 873–885. doi: 10.1016/j.jplph.2004.10.012
Qian, H., Zhang, J., and Zhao, J. (2022). How many known vascular plant species are there in the world? An integration of multiple global plant databases. Biodiv. Sci. 30:22254. doi: 10.17520/biods.2022254
Rae, D. (2011). Fit for purpose: The importance of quality standards in the cultivation and use of live plant collections for conservation. Biodivers. Conserv. 20, 241–258. doi: 10.1007/s10531-010-9932-8
Raja, M. M., Vijayalakshmi, G., Naik, M. L., Basha, P. O., Sergeant, K., Hausman, J. F., et al. (2019). Pollen development and function under heat stress: From effects to responses. Acta Physiol. Plant 41, 1–20. doi: 10.1007/s11738-019-2835-8
Ramantha Rao, V., and Sthapit, B. R. (2012). “Tropical fruit tree genetic resources: Status and effect of climate change,” in Tropical fruit tree species and climate change, eds B. R. Sthapit, V. R. Rao, and S. R. Sthapit (New Delhi: Bioversity International), 97–128.
Ramírez-Valiente, J. A., Aranda, I., Sanchéz-Gómez, D., Rodríguez-Calcerrada, J., Valladares, F., and Robson, T. M. (2009). Increased root investment can explain the higher survival of seedlings of ‘mesic’ Quercus suber than ‘xeric’ Quercus ilex in sandy soils during a summer drought. Tree Physiol. 39, 64–75. doi: 10.1093/treephys/tpy084
Ramlall, C., Varghese, B., Ramdhani, S., Pammenter, N. W., Bhatt, A., Berjak, P., et al. (2015). Effects of simulated acid rain on germination, seedling growth and oxidative metabolism of recalcitrant-seeded Trichilia dregeana grown in its natural seed bank. Physiol. Plant. 153, 149–160. doi: 10.1111/ppl.12230
Reed, B. M. (1990). Survival of in vitro-grown apical meristems of Pyrus following cryopreservation. HortScience 25, 111–113. doi: 10.21273/HORTSCI.25.1.111
Ren, R., Li, Z., Li, B., Xu, J., Jiang, X., Liu, Y., et al. (2019). Changes of pollen viability of ornamental plants after long-term preservation in a cryopreservation pollen bank. Cryobiology 89, 14–20. doi: 10.1016/j.cryobiol.2019.07.001
Resco de Dios, V., Arteaga, C., Peguero-Pina, J. J., Sancho-Knapik, D., Qin, H., Zveushe, O. K., et al. (2020). Hydraulic and photosynthetic limitations prevail over root non-structural carbohydrate reserves as drivers of resprouting in two Mediterranean oaks. Plant Cell Environ. 43, 1944–1957. doi: 10.1111/pce.13781
Roque-Borda, C. A., Kulus, D., Vacaro de Souza, A., Kaviani, B., and Vicente, E. F. (2021). Cryopreservation of agronomic plant germplasm using vitrification-based methods: An overview of selected case studies. Int. J. Mol. Sci. 22:6157. doi: 10.3390/ijms22116157
Rosbakh, S., Pacini, E., Nepi, M., and Poschlod, P. (2018). An unexplored side of regeneration niche: Seed quantity and quality are determined by the effect of temperature on pollen performance. Front. Plant Sci. 9:1036. doi: 10.3389/fpls.2018.01036
Royal Botanic Gardens Kew (2022). Seed information database (SID). Version 7.1. Available online at: http://data.kew.org/sid/ (Accessed November 11, 2022).
Saini, H. S., and Lalonde, S. (1997). Injuries to reproductive development under water stress, and their consequences for crop productivity. J. Crop Prod. 1, 223–248. doi: 10.1300/J144v01n01_10
Sakai, A. (1960). Survival of the twig of woody plants at - 196° C. Nature 185, 393–394. doi: 10.1038/185393a0
Salama, A. M., Ezzat, A., El-Ramady, H., Alam-Eldein, S. M., Okba, S. K., Elmenofy, H. M., et al. (2021). Temperate fruit trees under climate change: Challenges for dormancy and chilling requirements in warm winter regions. Horticulturae 7:86. doi: 10.3390/horticulturae7040086
Sánchez-Humanes, B., and Espelta, J. M. (2011). Increased drought reduces acorn production in Quercus ilex coppices: Thinning mitigates this effect but only in the short term. Forestry 84, 73–82. doi: 10.1093/forestry/cpq045
Sánchez-Montes de Oca, E. J., Badano, E. I., Silva-Alvarado, L. E., Flores, J., Barragan-Torres, F., and Flores-Cano, J. A. (2018). Acorn weight as determinant of germination in red and white oaks: Evidences from a common-garden greenhouse experiment. Ann. For. Sci. 75:12. doi: 10.1007/s13595-018-0693-y
Santos, J. A., Costa, R., and Fraga, H. (2017). Climate change impacts on thermal growing conditions of main fruit species in Portugal. Clim. Change 140, 273–286. doi: 10.1007/s10584-016-1835-6
Satishchandra, N. K., and Geerts, S. (2020). Modeling the distribution of the invasive alien cycad aulacaspis scale in Africa under current and future climate scenarios. J. Econ. Entomol. 113, 2276–2284. doi: 10.1093/jee/toaa156
Schermer, E., Bel-Venner, M.-C., Fouchet, D., Siberchicot, A., Boulanger, V., Caignard, T., et al. (2019). Pollen limitation as a main driver of fruiting dynamics in oak populations. Ecol. Lett. 22, 98–107. doi: 10.1111/ele.13171
Schroth, G., Läderach, P., Martinez-Valle, A. I., Bunn, C., and Jassogne, L. (2016). Vulnerability to climate change of cocoa in West Africa: Patterns, opportunities and limits to adaptation. Sci. Total Environ. 556, 231–241. doi: 10.1016/j.scitotenv.2016.03.024
Scottez, C., Chevreau, E., Godard, N., Arnaud, Y., Duron, M., and Dereuddre, J. (1992). Cryopreservation of cold-acclimated shoot tips of pear in vitro cultures after encapsulation- dehydration. Cryobiology 29, 691–700. doi: 10.1016/0011-2240(92)90073-B
Sentinella, A. T., Warton, D. I., Sherwin, W. B., Offord, C. A., and Moles, A. T. (2020). Tropical plants do not have narrower temperature tolerances, but are more at risk from warming because they are close to their upper thermal limits. Glob. Ecol. Biogeogr. 29, 1387–1398. doi: 10.1111/geb.13117
Sershen, Perumal, A., Varghese, B., Govender, P., Ramdhani, S., and Berjak, P. (2014). Effects of elevated temperatures on germination and subsequent seedling vigour in recalcitrant Trichilia emetica seeds. S. Afr. J. Bot. 90, 153–162. doi: 10.1016/j.sajb.2013.11.005
Sheoran, I. S., and Saini, H. S. (1996). Drought-induced male sterility in rice: Changes in carbohydrate levels and enzyme activities associated with the inhibition of starch accumulation in pollen. Sex. Plant Reprod. 9, 161–169. doi: 10.1007/BF02221396
Shi, W., Villar-Salvador, P., Li, G., and Jiang, X. (2019). Acorn size is more important than nursery fertilization for outplanting performance of Quercus variabilis container seedlings. Ann. For. Sci. 76:22. doi: 10.1007/s13595-018-0785-8
Shibata, M., Coelho, C. M. M., de Garighan, J. A., dos Santos, H. P., Araldi, C. G., and Maraschin, M. (2021). Seed development of Araucaria angustifolia: Plant hormones and germinability in 2 years of seed production. New For. 52, 759–775. doi: 10.1007/s11056-020-09821-2
Shibata, M., Masaki, T., Yagihashi, T., Shimada, T., and Saitoh, T. (2020). Decadal changes in masting behaviour of oak trees with rising temperature. J. Ecol. 108, 1088–1100. doi: 10.1111/1365-2745.13337
Singh, R. P., Prasad, P. V., and Reddy, K. R. (2013). Impacts of changing climate and climate variability on seed production and seed industry. Adv. Agron. 118, 49–110. doi: 10.1016/B978-0-12-405942-9.00002-5
Sinniah, U. R., Ellis, R. H., and John, P. (1998). Irrigation and seed quality development in rapid-cycling brassica: Seed germination and longevity. Ann. Bot. 82, 309–314. doi: 10.1006/anbo.1998.0748
Streczynski, R., Clark, H., Whelehan, L. M., Ang, S. T., Hardstaff, L. K., Funnekotter, B., et al. (2019). Current issues in plant cryopreservation and importance for ex situ conservation of threatened Australian native species. Aust. J. Bot. 67, 1–15. doi: 10.1071/BT18147
Stushnoff, C., and Junttila, O. (1986). Seasonal development of cold stress resistance in several plant species at a coastal and a continental location in North Norway. Polar Biol. 5, 129–133. doi: 10.1007/BF00441691
Suinyuy, T. N., and Johnson, S. D. (2018). Geographic variation in cone volatiles and pollinators in the thermogenic African cycad Encephalartos ghellinckii Lem. Plant Biol. (Stuttg) 20, 579–590. doi: 10.1111/plb.12685
Sun, W. Q. (1999). Desiccation sensitivity of sixty-four tropical, subtropical and temperate recalcitrant seeds. A. J. Trop. Biol. 3, 9–13.
Sun, W. Q., and Liang, Y. (2001). Discrete levels of desiccation sensitivity in various seeds as determined by the equilibrium dehydration method. Seed Sci. Res. 11, 317–323. doi: 10.1079/SSR200188
Sutherland, J. R., Diekmann, M., and Berjak, P. (eds) (2002). Forest tree seed health. IPGRI Technical Bulletin NO: 6. Rome: International Plant Genetic Resources Institute (IPGRI).
Sykes, M. T., and Prentice, I. C. (1996). Climate change, tree species distributions and forest dynamics: A case study in the mixed conifer/northern hardwoods zone of northern Europe. Clim. Change 34, 161–177. doi: 10.1007/BF00224628
Tabari, H. (2020). Climate change impact on flood and extreme precipitation increases with water availability. Sci. Rep. 10:13768. doi: 10.1038/s41598-020-70816-2
Takagi, S. (1977). A new species of Aulacaspis associated with a cycad in Thailand (Homoptera: Cocoidea). Insecta Matsumurana New Ser. 11, 63–72.
Tanner, J. D., Chen, K. Y., Bonnart, R. M., Minas, I. S., and Volk, G. M. (2021). Considerations for large-scale implementation of dormant budwood cryopreservation. Plant Cell Tissue Organ. Cult. 144, 35–48. doi: 10.1007/s11240-020-01884-5
Thomas, F. M., Blank, R., and Hartmann, G. (2003). Abiotic and biotic factors and their interactions as causes of oak decline in Central Europe. For. Pathol. 32, 277–307. doi: 10.1046/j.1439-0329.2002.00291.x
Tiberi, R., Branco, M., Bracalini, M., Croci, F., and Panzavolta, T. (2016). Cork oak past: A review of insect damage and management. Anna. For. Sci. 73, 219–232. doi: 10.1007/s13595-015-0534-1
Timmer, V. R. (1997). Exponential nutrient loading: A new fertilization technique to improve seedling performance on competitive sites. New For. 13, 275–295. doi: 10.1023/A:1006502830067
Toldam-Andersen, T. B., Krogholm, K. S., and Nygaard, T. B. (2007). Cryopreservation of dormant buds of apple cultivars in a mild maritime winter climate. Adv. Hortic. Sci. 21, 193–197. doi: 10.1400/85557
Towill, L. E., Forsline, P. L., Walters, C., Waddell, J. W., and Laufmann, J. (2004). Cryopreservation of Malus germplasm using a winter vegetative bud method: Results from 1915 accessions. CryoLetters 25, 323–334.
Tweddle, J. C., Dickie, J. B., Baskin, C. C., and Baskin, J. M. (2003). Ecological aspects of seed desiccation sensitivity. J. Ecol. 91, 294–304. doi: 10.1046/j.1365-2745.2003.00760.x
Uchendu, E. E., Shukla, M. R., Reed, B. M., and Saxena, P. K. (2013). Melatonin enhances the recovery of cryopreserved shoot tips of American elm (Ulmus americana L.). J. Pineal Res. 55, 435–442. doi: 10.1111/jpi.12094
Valladares, S., Toribio, M., Celestino, C., and Vieitez, A. M. (2004). Cryopreservation of embryogenic cultures from mature Quercus suber trees using vitrification. CryoLetters 25, 177–186.
van der Walt, K. (2010). “The critical difference between extinction and survival: Ex situ conservation of Encephalartos species in the Lowveld National Botanical Garden, South Africa,” in Proceedings of the 4th global botanic gardens conference, Dublin.
van der Walt, K., Alderton-Moss, J., and Lehnebach, C. A. (2022). Cross-pollination and pollen storage to assist conservation of Metrosideros bartlettii (Myrtaceae), a critically endangered tree from Aotearoa New Zealand. Pac. Conserv. Biol. 29, 141–152. doi: 10.1071/PC21054
Vandenbussche, B., Leurdian, S., Verdoodt, V., Gysemberg, M., and De Proft, M. (1999). Changes in sugar content and fatty acid composition of in vitro sugar beet shoots after cold acclimation: Influence on survival after cryopreservation. Plant Growth Regul. 28, 157–163. doi: 10.1023/A:1006262827160
Varghese, B., Sershen, Berjak, P., Varghese, D., and Pammenter, N. W. (2011). Differential drying rates of recalcitrant Trichilia dregeana embryonic axes: A study of survival and oxidative stress metabolism. Physiol. Plant. 142, 326–338. doi: 10.1111/j.1399-3054.2011.01469.x
Varghese, D., Berjak, P., and Pammenter, N. W. (2009). Cryopreservation of shoot tips of Trichilia emetica, a tropical recalcitrant-seeded species. CryoLetters 30, 280–290.
Vidal, N., Vieitez, A. M., Fernández, M. R., Cuenca, B., and Ballester, A. (2010). Establishment of cryopreserved gene banks of European chestnut and cork oak. Eur. J. For. Res. 129, 635–643. doi: 10.1007/s10342-010-0364-5
Villar-Salvador, P., Planelles, R., Oliet, J., Peñuelas-Rubira, J. L., Jacobs, D. F., and González, M. (2004). Drought tolerance and transplanting performance of holm oak (Quercus ilex) seedlings after drought hardening in the nursery. Tree Physiol. 24, 1147–1155. doi: 10.1093/treephys/24.10.1147
Vishwakarma, P. K., Vincent, L., Vasugi, C., and Rajasekharan, P. E. (2021). Effect of cryopreservation on pollen viability, fertility and morphology of different Psidium species. Cryobiology 98, 112–118. doi: 10.1016/j.cryobiol.2020.11.017
Vogel, J. (2022). Drivers of phenological changes in southern Europe. Int. J. Biometeorol. 66, 1903–1914. doi: 10.1007/s00484-022-02331-0
Volis, S. (2017). Conservation utility of botanic garden living collections: Setting a strategy and appropriate methodology. Plant Divers. 39, 365–372. doi: 10.1016/j.pld.2017.11.006
Volk, G. M. (2010). Application of functional genomics and proteomics to plant cryopreservation. Curr. Genomics 11, 24–29. doi: 10.2174/138920210790217945
Volk, G. M. (2011). “Collecting pollen for genetic resources conservation,” in Collecting plant genetic diversity: Technical guidelines, eds L. Guarino, V. Ramanatha Rao, and E. Goldberg (Nairobi: CGIAR), 1–10.
Vyse, K., Pagter, M., Zuther, E., and Hincha, D. K. (2019). Deacclimation after cold acclimation—a crucial, but widely neglected part of plant winter survival. J. Exp. Bot. 70, 4595–4604. doi: 10.1093/jxb/erz229
Wade, E. M., Nadarajan, J., Yang, X., Ballesteros, D., Sun, W., and Pritchard, H. W. (2016). Plant species with extremely small populations (PSESP) in China: A seed and spore biology perspective. Plant Divers. 38, 209–220. doi: 10.1016/j.pld.2016.09.002
Walck, J. L., Hidayati, S. N., Dixon, K. W., Thompson, K., and And Poschlod, P. (2011). Climate change and plant regeneration from seed. Glob Chang Biol. 17, 2145–2161. doi: 10.1111/j.1365-2486.2010.02368.x
Walsh, K. J. E., Mcbride, J. L., Klotzbach, P. J., Balachandran, S., Camargo, S. J., Holland, G., et al. (2016). Tropical cyclones and climate change. Wiley Interdiscip. Rev. Clim. Change. 7, 65–89. doi: 10.1002/wcc.371
Walters, C. (2015). Orthodoxy, recalcitrance and in-between: Describing variation in seed storage characteristics using threshold responses to water loss. Planta 242, 397–406. doi: 10.1007/s00425-015-2312-6
Walters, C., and Pence, V. C. (2021). The unique role of seed banking and cryobiotechnologies in plant conservation. Plants People Planet 3, 83–91. doi: 10.1002/ppp3.10121
Walters, C., Berjak, P., Pammenter, N., Kennedy, K., and Raven, P. (2013). Preservation of recalcitrant seeds. Science 339, 915–916. doi: 10.1126/science.1230935
Wei, J., Zhao, Q., Zhao, W., and Zhang, H. (2018). Predicting the potential distributions of the invasive cycad scale Aulacaspis yasumatsui (Hemiptera: Diaspididae) under different climate change scenarios and the implications for management. PeerJ 6:e4832. doi: 10.7717/peerj.4832
White, F. J., Hay, F. R., Abeli, T., and Mondoni, A. (2022). Two decades of climate change alters seed longevity in an alpine herb: Implications for ex situ seed conservation. Alp. Bot. 133, 11–20. doi: 10.1007/s00035-022-00289-8
Wijewardana, C., Reddy, K. R., Krutz, L. J., Gao, W., and Bellaloui, N. (2019). Drought stress has transgenerational effects on soybean seed germination and seedling vigor. PLoS One 14:e0214977. doi: 10.1371/journal.pone.0214977
Williams, M. I., and Dumroese, R. K. (2013). Preparing for climate change: Forestry and assisted migration. J. For. 111, 287–297. doi: 10.5849/jof.13-016
Wisniewski, M., Nassuth, A., and Arora, R. (2018). Cold hardiness in trees: A mini-review. Front. Plant Sci. 9:1394. doi: 10.3389/fpls.2018.01394
Wu, L., Wen, Z., and Huang, R. (2020). Tropical cyclones in a warming climate. Sci. China Earth Sci. 63, 456–458. doi: 10.1007/s11430-019-9574-4
Wyse, S. V., and Dickie, J. B. (2017). Predicting the global incidence of seed desiccation sensitivity. J. Ecol. 105, 1082–1093. doi: 10.1111/1365-2745.12725
Xia, K., Daws, M. I., and Peng, L. (2022). Climate drives patterns of seed traits in Quercus species across China. New Phytol. 234, 1629–1638. doi: 10.1111/nph.18103
Xia, K., Daws, M. I., Hay, F. R., Chen, W.-Y., Zhou, Z.-K., and Pritchard, H. W. (2012). A comparative study of desiccation responses of seeds of Asian Evergreen Oaks, Quercus subgenus Cyclobalanopsis and Quercus subgenus Quercus. S. Afr. J. Bot. 78, 47–54. doi: 10.1016/j.sajb.2011.05.001
Xia, K., Hill, L. M., Li, D., and Walters, C. (2014). Factors affecting stress tolerance in recalcitrant embryonic axes from seeds of four Quercus (Fagaceae) species native to the USA or China. Ann. Bot. 114, 1747–1759. doi: 10.1093/aob/mcu193
Xu, J., Li, B., Liu, Q., Shi, Y., Peng, J., Jia, M., et al. (2014). Wide-scale pollen banking of ornamental plants through cryopreservation. CryoLetters 35, 312–319.
Yamburov, M. S., Astafurova, T. P., Zhuk, K. V., Romanova, S. B., and Smolina, V. M. (2014). The effects of drought and flood stress on pollen quality and quantity in clivia miniata (lindl.) Bosse (amaryllidaceae). Biomed. Pharmacol. J. 7, 575–580. doi: 10.13005/bpj/526
Yu, J., Jiang, M., and Guo, C. (2019). Molecular sciences crop pollen development under drought: From the phenotype to the mechanism. Int. J. Mol. Sci. 20:1550. doi: 10.3390/ijms20071550
Zeng, C., Chen, Z., Xia, J., Zhang, K., Chen, X., Zhou, Y., et al. (2014). Chilling acclimation provides immunity to stress by altering regulatory networks and inducing genes with protective functions in cassava. BMC Plant Biol. 14:207. doi: 10.1186/s12870-014-0207-5
Zhang, H., Yang, X., Yu, M., Han, T., and Wu, T. (2017). Variations in seed size and seed mass related to tree growth over 5 years for 23 provenances of Quercus acutissima from across China. J. For. Res. 28, 917–923. doi: 10.1007/s11676-017-0375-x
Zhang, Z., Hu, M., Xu, W., Wang, Y., Huang, K., Zhang, C., et al. (2021). Understanding the molecular mechanism of anther development under abiotic stresses. Plant Mol. Biol. 105, 1–10. doi: 10.1007/s11103-020-01074-z
Zhao, Q., Zhou, L., Liu, J., Cao, Z., Du, X., Huang, F., et al. (2018). Involvement of CAT in the detoxification of HT-induced ROS burst in rice anther and its relation to pollen fertility. Plant Cell Rep. 37, 741–757. doi: 10.1007/s00299-018-2264-y
Zhou, X., He, Z.-B., Kang, H.-Z., Sun, X., and Liu, C.-J. (2013). Variations of seed morphology related to climate for Quercus variabilis across temperate subtropical China. Chin. J. Plant Ecol. 37, 481–491. doi: 10.3724/SP.J.1258.2013.00050
Keywords: cryopreservation, living collections, seeds, zygotic embryos, pollen, dormant buds, temperature, drought
Citation: Fernández A, León-Lobos P, Contreras S, Ovalle JF, Sershen, van der Walt K and Ballesteros D (2023) The potential impacts of climate change on ex situ conservation options for recalcitrant-seeded species. Front. For. Glob. Change 6:1110431. doi: 10.3389/ffgc.2023.1110431
Received: 28 November 2022; Accepted: 04 April 2023;
Published: 21 April 2023.
Edited by:
Dylan Craven, Major University, ChileReviewed by:
Rainer Vollmer, International Potato Center, PeruCopyright © 2023 Fernández, León-Lobos, Contreras, Ovalle, Sershen, van der Walt and Ballesteros. This is an open-access article distributed under the terms of the Creative Commons Attribution License (CC BY). The use, distribution or reproduction in other forums is permitted, provided the original author(s) and the copyright owner(s) are credited and that the original publication in this journal is cited, in accordance with accepted academic practice. No use, distribution or reproduction is permitted which does not comply with these terms.
*Correspondence: Daniel Ballesteros, ZGFuaWVsLmJhbGxlc3Rlcm9zQHV2LmVz; Pedro León-Lobos, cGxlb25AaW5pYS5jbA==
†ORCID: Pedro León-Lobos, orcid.org/0000-0003-0946-2896; Karin van der Walt, orcid.org/0000-0002-4957-7017
Disclaimer: All claims expressed in this article are solely those of the authors and do not necessarily represent those of their affiliated organizations, or those of the publisher, the editors and the reviewers. Any product that may be evaluated in this article or claim that may be made by its manufacturer is not guaranteed or endorsed by the publisher.
Research integrity at Frontiers
Learn more about the work of our research integrity team to safeguard the quality of each article we publish.