- 1Department of Biology, Wake Forest University, Winston-Salem, NC, United States
- 2Center for Global Discovery and Conservation Science, Arizona State University, Tempe, AZ, United States
- 3Department of Biology, Center for Energy, Environment, and Sustainability, Wake Forest University, Winston-Salem, NC, United States
Landslides are a central component of tropical montane forest disturbance regimes, including in the tropical Andes biodiversity hotspot, one of the most biodiverse ecosystems in the world. Technological developments in remote sensing have made landscape-scale landslide studies possible, unlocking new avenues for understanding montane biodiversity, ecosystem functioning, and the future effects of climate change. Here, we outline three axes of inquiry for future landslide ecology research in Andean tropical montane forest. We focus exclusively on the Andes due to the vast floral diversity and high endemicity of the tropical Andes biodiversity hotspot, and its importance for global biodiversity and regional ecosystem service provisioning; the broad elevational, latitudinal, and topographic gradients across which landslide dynamics play out; and the existence of long-term plot networks that provide the necessary baseline data on mature forest structure, composition, and functioning to contextualize disturbance impacts. The three lines of study we outline, which draw heavily on remote sensing data and techniques, will deepen scientific understanding of tropical montane forest biodiversity and ecosystem functioning, and the potential impacts of climate change on both. They are: (1) tracking landslide biodiversity dynamics across time and space with high spatial and temporal resolution satellite and unoccupied aerial vehicle imagery; (2) assessing the ecological influence of landslides through the lens of plant functional diversity with imaging spectroscopy; and (3) understanding current and predicting future landslide regimes at scale by building a living landslide inventory spanning the tropical Andes. The research findings from these three axes of inquiry will shed light on the role of landslides and the process of forest recovery from them in both the Andes and worldwide.
Introduction
Despite their importance to global biodiversity and ecosystem services, tropical montane forest (TMF) disturbance regimes are understudied (Crausbay and Martin, 2016; Martin and Bellingham, 2016). Landslides, a central component of many TMF disturbance regimes, leave long-lasting legacies on montane landscapes (Restrepo et al., 2009; Walker and Shiels, 2013). They have helped shape the highly diverse TMF of the tropical Andes biodiversity hotspot (Kessler and Kluge, 2008; Richter et al., 2009), which extends from Venezuela, through Colombia, Ecuador, Peru, and Bolivia, to northeastern Chile and northwestern Argentina (Myers et al., 2000; Critical Ecosystem Partnership Fund, 2021). The hotspot is home to at least 30,000 plant species, including an estimated 15,000 endemic species, making the Andean flora the most diverse in the world (Mittermeier et al., 2011). Andean TMF is also one of Earth’s most threatened habitats, with climate change predicted to significantly alter the region’s hydrological and temperature regimes, thus changing forest compositions, disrupting ecosystem processes, and altering natural disturbance regimes (Still et al., 1999; Crausbay and Martin, 2016). Increasing scientific understanding of landslides in the tropical Andes is integral to the long-term protection and management of Andean TMF.
Landslides are widespread in the tropical Andes and contribute to large-scale ecosystem processes. For example, on average, 0.08% of Peru’s Kosñipata Valley is affected by landslides each year (Clark et al., 2016), a rate equal to the per century rate estimated for Puerto Rico’s Upper Luquillo Mountains (Guariguata, 1990) and seven times greater than estimated for eastern Puerto Rico (Larsen and Torres-Sanchez, 1992). This translates into an average hillslope turnover time of 1,320 years, more rapid than observed rates from 13 catchments in New Zealand’s western Southern Alps (Hilton et al., 2011), and 24 times faster than in the mountains of Mexico and Central America (Restrepo and Alvarez, 2006). Landsliding rates may be even higher in other parts of the Andes; a study in an area of very steep relief in the Bolivian Eastern Cordillera demonstrated landslides affect 4–6% of the landscape over 10–35 years (Blodgett and Isacks, 2007), a rate of 0.11–0.6% per year, or 11.4–17.1% per century. Landslides play a key role in landscape evolution (Korup et al., 2010) and the mobilization of geological material (Muenchow et al., 2012), including the export of sediment, rock and non-rock derived nutrients, and forest carbon to rivers (Hilton et al., 2008, 2011; Restrepo et al., 2009; Ramos Scharrón et al., 2012; Clark et al., 2016; Croissant et al., 2019; Hilton and West, 2020). By exposing underlying bedrock, landslides also make nutrients available to plants (Guariguata, 1990; Zarin and Johnson, 1995; Vitousek et al., 2003; Porder et al., 2005). Finally, populations of landslides contribute to longer-term carbon cycling in mountain landscapes (Ramos Scharrón et al., 2012; Frith et al., 2018; Hilton and West, 2020).
Here, we outline a vision for three axes of study for landslide research in Andean TMF that apply remotely-sensed data to understand how landslides contribute to TMF biodiversity, ecosystem functioning, and the potential effects of climate change on landslide regimes (Figure 1). We focus exclusively on the Andes for three reasons: (1) the vast floral diversity and high endemicity of the tropical Andes biodiversity hotspot, and its importance for global biodiversity and regional ecosystem service provisioning (Myers et al., 2000; Breuer et al., 2013; Spracklen and Righelato, 2014); (2) the broad elevational, latitudinal, and topographic gradients, longer than all other tropical mountain ranges in the world (Young et al., 2007), across which landslide dynamics play out; and (3) the existence of several long-term and thoroughly characterized plot networks across the region that provide the baseline data on mature forest structure, composition, and functioning necessary to contextualize disturbance impacts (Malhi et al., 2010; Malizia et al., 2020). Though we focus on the Andes, the themes, and research avenues we discuss are broadly applicable to TMF globally.
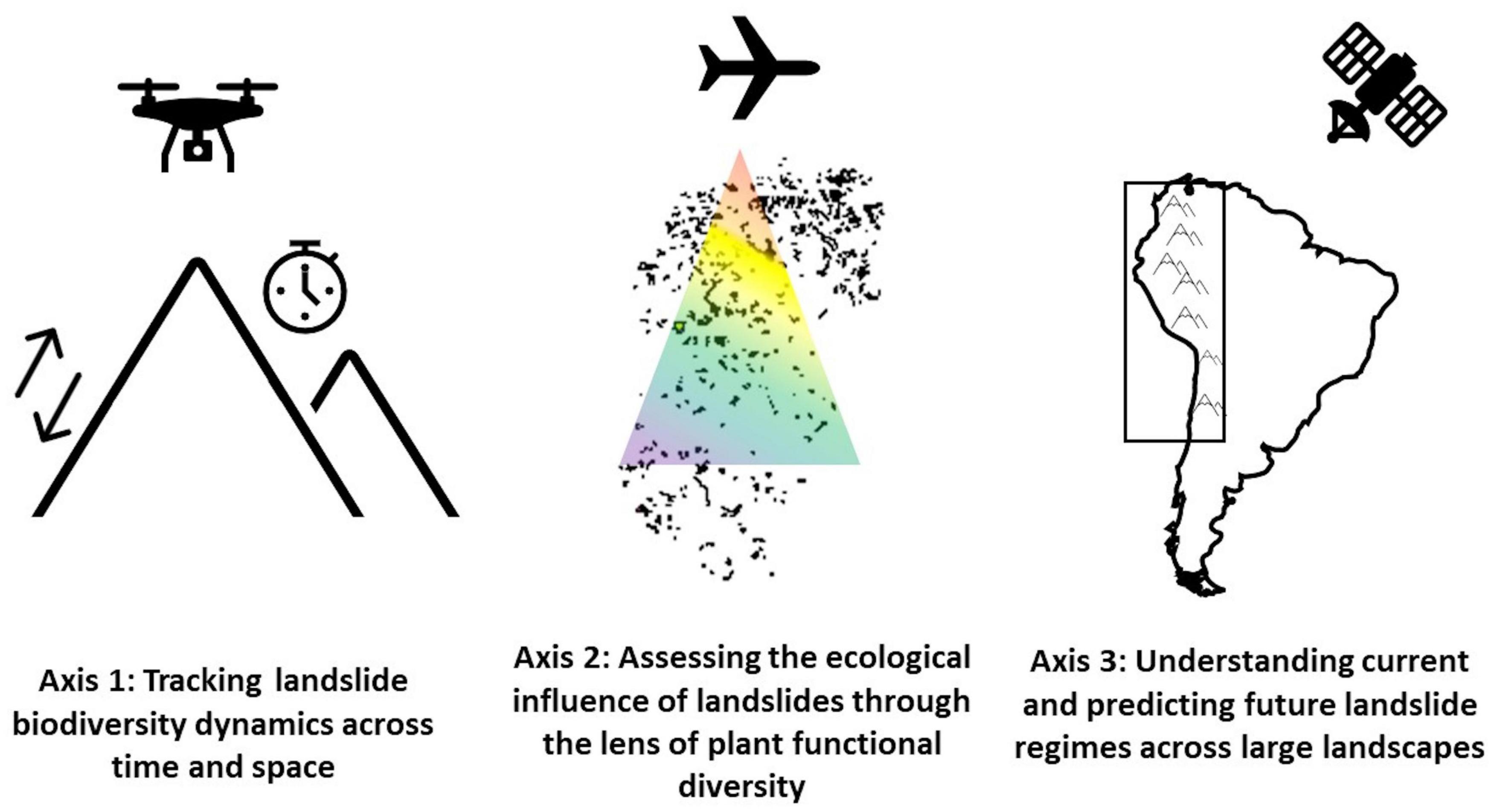
Figure 1. Conceptual representation of the three axes of landslide ecology research outlined here. We propose (1) tracking landslide biodiversity dynamics across time and space (e.g., elevational gradients) using high-resolution satellite and UAV imagery; (2) assessing plant functional diversity on and around regenerating landslides with air and/or space-borne imaging spectroscopy; and (3) understanding current and predicting future landslide regimes across large landscapes using a combination of deep learning and high-resolution satellite imagery to build a living landslide inventory spanning the tropical Andes. The spatial scale of inquiry changes with each axis, starting with data collected from collections of individual landslides for Axis 1, examining populations of landslides within discrete regions (e.g., catchments) for Axis 2, and finally mapping landslides across the entire tropical Andes biodiversity hotspot for Axis 3.
Technological developments in remote sensing over the past few decades have increased the efficiency and accuracy of landslide mapping, analysis, and monitoring (Lin et al., 2004; Petley, 2012; Amatya et al., 2021; Casagli et al., 2023). The relatively recent availability of medium- to high spatial and temporal resolution satellite imagery (e.g., Sentinel-2 visible bands at 10 m every 5–10 days, PlanetScope at 3 m daily), now allows scientists to observe the planet in near real-time and high detail (Finer et al., 2018). The high spatial resolution is important because previously, many freely available images (e.g., Landsat at 30 m) were not at sufficient spatial resolution to capture small landslides, an important component of landslide regimes (Clark et al., 2016; Fayne et al., 2019). Satellites with high temporal resolution largely solve the challenge of procuring cloud-free imagery from a given time period (Roy et al., 2021), which can be difficult in tropical montane locations. However, satellite imagery is just one tool of many useful for landslide research (Casagli et al., 2023). There are myriad other existing and in-development remote sensing tools, some of which are integrated in the vision outlined here, that could also be applied to these endeavors, each with their unique combination of spatial resolution, revisit time, scene extent, and accuracy (Casagli et al., 2023).
Axis 1: Tracking landslide biodiversity dynamics across time and space
Studies from TMF in Puerto Rico, Hawaii, Jamaica and elsewhere have identified general types of plants present during landslide succession (Guariguata, 1990; Dalling, 1994; Restrepo and Vitousek, 2001; Walker et al., 2010). However, Andean TMF are home to hyperdiverse and unique floral assemblages, with ∼50% of plant species endemic to the region (Myers et al., 2000). There remains a gap in our understanding of the types and diversity of landslide-establishing plants in the Andes. For example, the families of Asteraceae, Melastomataceae, Poaceae, and Solanaceae are both species-rich (Pérez-Escobar et al., 2022) and establish on Andean landslides during succession (Kessler, 1999; Ohl and Bussmann, 2004; Restrepo et al., 2009; Meier, 2013; Freund, 2022), meaning there may be groups of plants never or rarely observed on landslides in other regions that play important roles in Andean landslide succession. Fully cataloguing the diversity of plants found on landslides will shed light on the role of these disturbances, which provide habitat for species that do not establish, survive or grow in closed-canopy forests (Kessler, 1999), in maintaining TMF biodiversity at regional scales (Ohl and Bussmann, 2004; Richter et al., 2009).
This knowledge gap is compounded by the fact that existing information comes from piecemeal, rather than systematic, samples of a relatively small number of Andean landslides. Plant diversity on landslides is known to vary with age (a proxy for successional stage), elevation (Ohl and Bussmann, 2004; Bussmann et al., 2008), and the presence of residual forest soils (Walker et al., 1996). But in the Andes many landslides occur in steep, inaccessible parts of the landscape, making systematic field collection of vegetation data difficult or precluding it entirely. This biases the understanding of landslide vegetation to only the most readily accessible landslides (e.g., those near research stations or roads), complicating efforts to understand how biodiversity on regenerating landslides changes with time and/or across elevational or other environmental gradients. Field studies of Andean landslides have produced information from a relatively small sample of landslides of disparate (and sometimes unknown) ages between 1,400–2,800 m a.s.l. (Stern, 1995; Kessler, 1999; Ohl and Bussmann, 2004; Meier, 2013), just a portion of the expansive TMF elevational gradient found across much of the Andes. We still do not know how the types and diversity of plants occupying regenerating landslides varies with elevation, particularly below 1,400 m and above 2,800 m. Given the pool of colonizing tree species and the community of animal seed dispersers (and presumably pollinators) is known to continuously turn over across TMF elevational gradients (Patterson et al., 1998; Jankowski et al., 2013; Lough, 2017; Mena and Pacheco, 2020), the suite of species that establish on landslides, and therefore the successional process itself, likely also differs across low- and high-elevation landslides.
High resolution satellite and unoccupied aerial vehicle (UAV) imagery (Figure 2), as well as other remote sensing techniques, can help mitigate these biases by expanding the extent of TMF in which tree biodiversity can be assessed. Previous work has demonstrated that high resolution satellite imagery (Quickbird at 2.4 m and RapidEye at 5 m, respectively) alone or in combination with LiDAR can be used to measure tropical tree diversity (Fricker et al., 2015; George-Chacon et al., 2019). Very high resolution WorldView-2 satellite (Wagner et al., 2018) and UAV (Peck et al., 2012) imagery have also been used to delineate and identify tree species. On landslides specifically, high- resolution satellite and UAV imagery (at resolutions <1 m) have been used to map land cover and manually identify tree species (Furukawa et al., 2021; Freund, 2022; Saito et al., 2022). Deep learning algorithms can automate the identification of both canopy and shorter-statured species (such as those present during early stages of landslide succession), including in regenerating forests, from UAV-acquired images (Zhang et al., 2020; Moura et al., 2021; Veras et al., 2022). Using these tools, landslide researchers can now investigate ecologically relevant questions such as how plant species richness and diversity vary on landslides of similar ages across expansive elevational gradients (400–3,800 m in much of the Andes), as well as how these metrics vary across landslides of different ages within elevational bands, to better characterize the process of TMF regeneration after landslides.
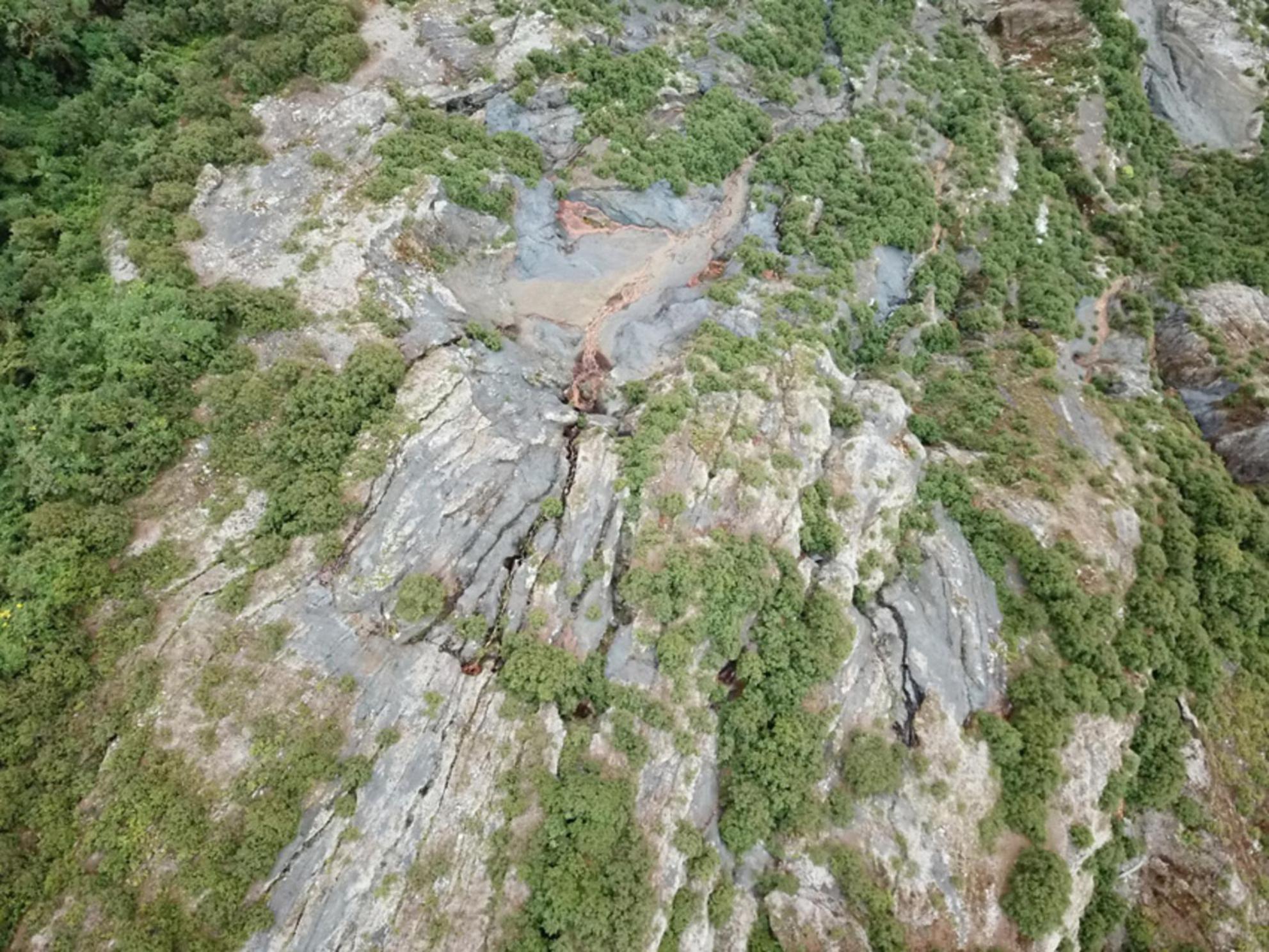
Figure 2. Example of high-resolution UAV imagery of a landslide in Manu National Park, Kosñipata Valley, Peru (13°06′31″ S, 71°36′22″ W), demonstrating the utility of UAVs for surveying TMF vegetation on landslides. This image was taken at 3 cm resolution with a DJI Mavic Pro UAV equipped with a 12.35-megapixel camera and a 1/2.3 CMOS sensor.
Landslides as potential tree migration corridors
Andean tree communities are currently shifting their distributions upslope as temperatures warm, and communities are undergoing thermophilization (Duque et al., 2015; Fadrique et al., 2018). But in all except a few locations, this appears to be occurring too slowly to track rising temperatures (Feeley et al., 2011; Fadrique et al., 2018; Farfan Rios, 2019). Our current understanding of plant migration (e.g., Ibáñez et al., 2009; Jump et al., 2012; Feeley et al., 2013) has come from studies in mature and undisturbed habitats, highly competitive environments that may stymie the movement of migrating species (Caplat et al., 2008, 2013). However, recent evidence from a variety of forest and disturbance types suggests that disturbances may facilitate plant migration in response to climate change by creating opportunities for trees to establish above their current latitudinal or elevational ranges (Landhäusser et al., 2010; Leithead et al., 2010, 2012; Duque et al., 2015; Guo et al., 2018; Tanner et al., 2022). It is very likely that migration through disturbed areas is also occurring in Andean TMF (Lutz et al., 2013). High-resolution satellite and/or UAV imagery should be applied to examine the role of landslides in this process.
Landslides are suitable pathways for rapid movement as they open competition-free space across vertical gradients spanning tens-to-hundreds of meters. Given an adiabatic lapse rate in the Andes of ∼5.5°C km–1 (Bush et al., 2004), mean temperatures on a landslide with 100-m elevational range could differ by >0.5°C from top to bottom. The slow recovery rates characteristic of Andean TMF on landslides can maintain competition-free space for years (Blodgett and Isacks, 2007; Dislich and Huth, 2012; Freund et al., 2021), providing opportunities for trees to establish above their current elevational ranges. There is some evidence for species migration through landslides from the Peruvian Andes. A comparison of the abundances of small and large (<5 cm and >10 cm diameter at breast height, respectively) trees in permanent vegetation plots identified 21 species abundant as large trees but rarely present as smaller size-classes in mature TMF (Garcia Cabrera, 2011), suggesting they may have originally established after a landslide or other disturbance and persisted in the canopy through succession. We have observed at least 12 of them (e.g., Alzatea verticillata, Axinaea pennellii, Weinmannia spp.) on landslides in the same study site (Freund, unpublished data1). Further research is needed to compare the elevational ranges of these species in mature and disturbed sites. The combination of canopy species identification from high-resolution UAV and satellite imagery with machine learning now puts this within reach (Brodrick et al., 2019).
Answering this question of whether TMF trees use landslides to shift their elevational distributions would fundamentally change the way we think about their responses to climate change. Current understanding of tree migration paints a bleak picture for the future of Andean tree communities as at most study sites trees are seemingly migrating at a fraction of the rate needed to maintain equilibrium with climate (e.g., Fadrique et al., 2018; Farfan Rios, 2019), and much of the population shift attributed to migration is simply due to mortality of species at the warm margin of their range, with little or no expansion at the cool margin (Feeley et al., 2013; Farfan Rios, 2019). Migration must be examined in a landscape context as the permeability for movement will vary both with disturbance regimes and the traits and regeneration niches of the species themselves; in the tropical Andes, landslides are an ideal part of the landscape for such an examination.
Axis 2: Assessing the ecological influence of landslides through the lens of plant functional diversity
Understanding how populations of landslides influence carbon uptake and storage, primary productivity, and related ecosystem processes at the landscape scale will help elucidate the resilience of montane landscapes to global change (Restrepo et al., 2009). Quantifying the ecological influence of landslides can be achieved by using air- and/or satellite-borne hyperspectral imaging, or imaging spectroscopy, to measure plant functional diversity (Jetz et al., 2016; Asner et al., 2017) of landslide-affected and undisturbed areas. This approach views plant biodiversity through the lens of species’ structural and biochemical functional traits, which correspond to their roles in ecosystem processes and services (Díaz et al., 2007). Field studies of TMF plant functional diversity have demonstrated elevational and topographic trends in plant functional diversity (Duivenvoorden and Cuello, 2012; Homeier et al., 2021; Báez et al., 2022b; Pierick et al., 2023) though these have largely focused on adult trees in mature forest (Báez et al., 2022a) covering a relatively small proportion of the wider landscape. An exception to these small-scale studies is Asner et al.’s (2014a) airborne imaging spectroscopy and LiDAR-based study, which revealed high landscape diversity and elevational turnover in functional traits and forest structure across the Amazon-Andes gradient and demonstrated the clear signature of landslides on the landscape (Asner et al., 2014a). There remains much to learn about the plant functional diversity of disturbed and regenerating Andean TMF, and how it contributes to ecosystem processes.
Hyperspectral imaging measures electromagnetic reflectance from surfaces, in this case forest canopies, in narrow spectral bands (typically 5–10 nm) in the electromagnetic spectrum ranging from ultraviolet through medium-wave infrared (400–2,500 nm). The technique can be used to detect the chemical properties of trees, among them functional traits linked to photosynthesis, primary production, defense, biogeochemical processes, and evolutionary history. Unique combinations of these values can be used to accurately assign functional traits to canopy assemblages and even describe individual species (Clark et al., 2005), especially when paired with remote sensing techniques to measure forest structure, such as LiDAR (Lucas et al., 2008; Asner et al., 2015; Shi et al., 2018). While many studies have applied imaging spectroscopy to the detection, monitoring, and characterization of landslides (e.g., Vellico et al., 2010; Ye et al., 2019), to our knowledge this method has not yet been applied to studies of landslide regeneration in highly diverse tropical forests. However, given its power for measuring tropical plant functional diversity and linking plant communities to ecosystem-level processes (see review in Asner et al., 2017), it is a powerful option for understanding the role of landslides in Andean TMF.
Community and ecosystem dynamics on regenerating landslides
One major outstanding question about Andean landslides that analyses of satellite and LiDAR data have not yet been able to clearly answer is how long it takes tree species composition, diversity, and aboveground biomass to recover to mature forest levels (Freund et al., 2021). Field studies of landslides in Puerto Rico and Jamaica estimate it can take between ∼52 to 500 years, respectively, for aboveground biomass to recover (Guariguata, 1990; Dalling, 1994). However, current best estimates of forest recovery post-landslides come from a modeling study of the Ecuadorian Andes, which estimated that species composition can recover in 100–200 years and aboveground biomass within 300 years (Dislich and Huth, 2012). These wide-ranging estimates must be constrained if we are to quantify TMF carbon balances and understand the lasting effects of landslides on Andean forests it is important to refine these estimates (Duque et al., 2021).
Applying hyperspectral imaging to large sample sizes of regenerating landslides, particularly decades-old landslides where forests have regained the stature and surface-level appearance of undisturbed/mature forests, could achieve this goal in a way that previous attempts field and other remotely-sensed data have not (Asner, 2008). Recovery of forest structure and volume generally happens relatively quickly after disturbances (Letcher and Chazdon, 2009; Pan et al., 2011), but changes in species composition as light-wooded pioneer species by successively heavier-wooded mid- and late-successional species take much longer (Denslow, 2000; ter Steege and Hammond, 2001; Slik, 2005). While LiDAR has been useful in refining estimates of forest structure recovery times on regenerating landslides (Freund et al., 2021), applying imaging spectroscopy will unlock the ability of researchers to examine changes in landslide species composition during regeneration, characterize community composition and functional diversity (Kalacska et al., 2007), and compare the characteristics of regrown landslides to surrounding mature forest across environmental gradients (Asner et al., 2014a). Historical aerial and/or satellite imagery will be important in this line of inquiry, specifically in identifying decades-old landslides not easily identifiable in the field (Ohl and Bussmann, 2004) or from contemporary imagery.
Effects of landslides on adjacent intact forest
In addition to directly stripping slopes of vegetation and soil, landslides may affect adjacent intact forest through above- and belowground edge effects. Edges are boundaries between two habitat types that delineate areas with different environmental characteristics (Ries et al., 2004), in this case, intact TMF and landslide scars. Abiotic factors such as temperature, light, and relative humidity vary across edges, altering growing conditions and tree mortality in adjacent forests (Cadenasso et al., 1997; Gehlhausen et al., 2000; Harper et al., 2005), which in turn can affect forest structure and composition (Young, 1993; Murcia, 1995; Harper et al., 2005; Marchand and Houle, 2006). Studies have found mixed effects of edges on tree functional diversity (Apaza-Quevedo et al., 2015; Razafindratsima et al., 2018), and there are many unknowns about the relevance of edges to larger ecosystem processes. However, given the high edge-to-interior ratio of many Andean landslides (Bussmann et al., 2008; Freund et al., 2021), the existence of detectable edge effects of landslides on functional diversity of adjacent intact forest could substantially increase the total footprint of landslides on TMF. Finally, while nearly all discussion of edge effects is focused on aboveground processes, landslides fundamentally change the local hydrology of hillslopes (Mirus et al., 2017), and the importance of this on the surrounding vegetation remains unknown.
The importance of a landscape perspective
A full accounting of landslide effects on TMF ecosystem functioning requires putting landslides in the context of the wider landscape. Landslides create hot and cold spots of forest productivity (sensu Dislich and Huth, 2012), which contribute to substantial observed variation in above and belowground TMF biomass and carbon density (Girardin et al., 2014; Spracklen and Righelato, 2016; Malhi et al., 2017). However, given the diverse evolutionary histories of Andean TMF tree lineages (Griffiths et al., 2021) and the region’s complex terrain and biophysical characteristics, landslides are likely not the only source of this heterogeneity (Spasojevic et al., 2014; Pierick et al., 2021). Using imaging spectroscopy to survey plant functional diversity across large spatial scales, and therefore a range of topographic and environmental conditions, will reveal the contribution of cycles of landsliding and subsequent forest regeneration to landscape-level variability in TMF processes. This work would build on existing large scale surveys of leaf optical traits and canopy chemistry across an Andes-to-Amazon elevation gradient, which identified clear patterns in canopy chemical traits with elevation and a strong influence of phylogeny (Asner et al., 2014b,c). There may be similar trends in functional traits with slope inclination, aspect, or other topographic variables that interact with disturbance histories in previously unforeseen ways.
Axis 3: Understanding current and predicting future landslide regimes across large landscapes
Technological advances in remote sensing technology continue to expand scientific understanding of current and future landslide regimes, particularly by opening new possibilities for mapping, monitoring, and studying these natural phenomena at very large spatial scales (Guzzetti et al., 2012; Casagli et al., 2023). One additional challenge for large-scale landslide mapping not readily solved by improvements to satellite imagery alone is that the creation of landslide inventory maps has traditionally required researchers to manually examine aerial and satellite imagery to delineate affected areas (e.g., Guns and Vanacker, 2014; Clark et al., 2016; Delgado et al., 2022). However, high-resolution satellite data paired with computer vision for object and change detection (Zhong et al., 2020; Amatya et al., 2021; Lu et al., 2022) unlocks the ability to identify past landslide activity and monitor landslides in near real-time at regional and even continental scales (Yang et al., 2022). For example, this approach has recently been used to detect and map landslides in Nepal (Prakash et al., 2021; Meena et al., 2022), Taiwan, China, Japan (Ghorbanzadeh et al., 2021, 2022), and the Patagonian Andes (Morales et al., 2022). In the Andes, Morales et al. (2022) applied a convolutional neural network to Sentinel-2 images to develop a 10,000-landslide inventory covering approximately 20,000 km2. Their model, the first automated landslide detection model in the region, was most accurate in areas with vegetation cover (Morales et al., 2022), suggesting this approach will work well across forested regions of the Andes.
Building on the success of Morales et al. (2022), we propose that a combination of deep learning and high-resolution satellite imagery be used to develop and maintain an automated “living landslide inventory” spanning all Andean TMF. By maximizing the spatial extent of study, and therefore range (and possible combinations) of abiotic variables represented, this effort would increase scientific understanding of how the environment and human activity (e.g., Guns and Vanacker, 2014) shapes landslide occurrences, extents, and frequencies without the limitations and biases inherent to studies at smaller spatial scales (Lobo and Dalling, 2014; Marvin et al., 2014). It will also lay the groundwork for studies of how South American landslide regimes shift in response to global climate change, a critical knowledge gap (Gariano and Guzzetti, 2016) and fill an important environmental planning and policymaking need for local, regional, and national governments in Andean countries (Hermanns et al., 2012). Here, we detail several specific scientific contributions that would be made possible by this pan-Andean landslide inventory.
Characterizing factors shaping current landslide regimes in Andean TMF
Smaller-scale studies of Andean TMF, generally at the catchment scale, have characterized landslide regimes with landslide inventory mapping (Clark et al., 2016; Vanacker et al., 2020) and hazard/susceptibility modeling (Brenning, 2005; Roa Lobo, 2007; Muenchow et al., 2012; Younes Cárdenas and Erazo Mera, 2016; Palacio Cordoba et al., 2020), among other methods. Such studies have found that landsliding rates and risks vary with elevation, geology and geomorphology, rainfall and soil permeability, and slope (Ließ et al., 2011; Clark et al., 2016; Aristizábal et al., 2022). They are also influenced by anthropogenic disturbance (Guns and Vanacker, 2014; Brenning et al., 2015). However, these variables are often correlated with each other. For example, in Peru’s Kosñipata Valley elevation and slope co-vary, with slopes >40° more common below 2,000 m (Clark et al., 2016). Aligning the large-scale living landslide inventory with other available environmental data (e.g., high-resolution digital terrain models, soil maps) could uncover the relative influences of abiotic variables on landslide rates.
The limited spatial scale of landslide investigations to date has potentially also biased estimates of landslide size-frequency distributions by underestimating the occurrence of large landsliding events. Landslide populations follow power law distributed size-frequency distributions (Pelletier et al., 1997; Stark and Hovius, 2001; Brown et al., 2002; Larsen and Montgomery, 2012; Clark et al., 2016), with frequent small landslides and relatively few large landslides. For example, landslides >50,000 m2 made up approximately 1% of events in a 25-year inventory from southeastern Peru (Freund, 2022). However, studies of gap size frequency distributions in TMF have been limited in spatial scale due to their reliance on ground-based (field) sampling or airborne LiDAR. Because size-frequency distribution estimates vary with the spatial scale at which they are measured (Lobo and Dalling, 2014; Marvin et al., 2014), with larger sample areas yielding more accurate estimates due to the capture of larger, more rare events, it is possible current understanding of Andean landslide regimes underestimates the sizes and frequencies of events at the heavy tail of the power law distribution. Filling this gap is important because large landslides, while rare, play fundamental roles in hillslope evolution (Densmore et al., 1997; Korup et al., 2007), sediment export (Vanacker et al., 2007; Townsend-Small et al., 2008), and the movement of organic carbon through TMF ecosystems (Ramos Scharrón et al., 2012).
In addition to very large landslides, an automated pan-Andes landslide inventory would help identify the frequency and spatial distribution of clustered landsliding events triggered by extreme precipitation or seismic activity. These events create populations of similar-aged landslides concentrated in small geographic areas (Garwood et al., 1979; Restrepo et al., 2009; Clark et al., 2016), leaving visually striking patterns on the landscape that may persist for decades (Freund et al., 2021). The prevalence of these events across time and space in the Andes (and South America, generally) is virtually unknown (Benz and Blum, 2019), though it is clear they are a substantial component of at least some landslide regimes. For example, a 2010 extreme rainfall event in Peru’s Kosñipata Valley triggered at least 185 landslides below 2,600 m within a 185 km2 area, comprising 27% of the total observed landslide footprint in the catchment over a 25-year period (Clark et al., 2016). As a result, Clark et al. (2016) found high landslide susceptibility at low elevations, a pattern not evident when the landslide cluster was excluded from the dataset. Obtaining more information on the frequency and spatial organization of clustered landslide events on the landscape (e.g., do they recur on the same slopes, are they constrained to certain orographic exposures or geologies?) will reveal the role of clustered landsliding events on shaping TMF ecosystems.
Applying current knowledge to future landslides
Landslide regimes will be altered by climate change through changes in temperature and hydrological patterns (Gariano and Guzzetti, 2016). Exactly how and where they will be most affected is a critical outstanding question for predicting the future of Andean TMF. While some factors that contribute to a location’s landslide susceptibility, such as slope steepness and lithology, are unchanged by warming temperatures (Guzzetti et al., 1999), climate change is projected to alter the timing, frequency, volume, and intensity of rainfall in the Andes (Urrutia and Vuille, 2009; Magrin et al., 2014; Eghdami and Barros, 2019; Sarmiento and Kooperman, 2019). It will also likely affect cloud regimes, which are highly complex due in part to the rugged topography of the Andes (Halladay et al., 2012a,b). In general, Andean cloud bases are predicted to move upslope, shrinking the amount of TMF subject to persistent cloud immersion (Still et al., 1999; Helmer et al., 2019), though it is difficult to downscale regional-scale climate models to predict future moisture/precipitation regimes in specific locations (Buytaert et al., 2010). If the elevational distribution of rainfall events (particularly extreme rainfall events, e.g., Clark et al., 2016) and moisture input from persistent cloud cover is altered (Bruijnzeel et al., 2011), the elevational distributions of landslides in Andean TMF will also change, though the direction and exact magnitude of those changes will likely vary across the region.
An automated landslide inventory spanning the tropical Andes region, with its extreme environmental heterogeneity and long climate gradients, will be a valuable tool for exploring how climate change will alter TMF landslide regimes (Crausbay and Martin, 2016), especially when coupled with additional remotely-sensed data. Moisture regimes in the Andes are spatio-temporally complex. Precipitation varies across the region due to large-scale geographic and orographic effects (Garreaud, 2009; Hierro et al., 2020), and also varies seasonally and on interannual time scales (Segura et al., 2019; Sierra et al., 2022). Comparing landslide sizes, frequencies, and spatial distributions in historically dry and wet valleys with similar topography and geology, as well as comparing across valleys with different local cloud and humidity dynamics (Muenchow et al., 2012), are two ways among many a pan-Andes landslide inventory would help to answer questions about how climate change will affect landslide rates. Results can then be integrated into models of forest change and other ecosystem processes to forecast the future of Andean TMF (e.g., Caplat et al., 2008). A living landslide inventory and associated environmental data, all remote-sensing derived, would make this possible.
Conclusion
Although landslides are a large and severe example of natural forest disturbances, their study is rooted in fundamental principles of ecosystem succession, plant physiology and demography, and landscape ecology. The three axes of inquiry we pose here advance important and timely questions about landslide ecology in the tropical Andes biodiversity hotspot. Answering them will require a variety of advanced remote sensing methods, including but not limited to high-resolution UAV and satellite imaging, airborne LiDAR, and satellite-borne and airborne imaging spectroscopy. This work will improve scientific understanding of the region’s biodiversity, natural disturbance dynamics, ecosystem functioning, and responses to climate change. Achieving an automated pan-Andes landslide inventory will have additional benefits for environmental policy and planning in the region, thus increasing the resilience of human and ecological communities in this critical biodiversity hotspot.
Author contributions
CF was responsible for the conceptualization and writing of the manuscript. MS assisted with the conceptualization and gave input on the manuscript. Both authors contributed to the article and approved the submitted version.
Funding
Support for the research came from the Andes Biodiversity and Ecosystem Research Group, the WFU Pilot Research Fund, the Gordon and Betty Moore Foundation Andes-Amazon Program, the American Philosophical Society Lewis and Clark Fund for Exploration and Field Research, and WFU Vecellio and Richter grants to CF and NSF DEB LTREB 1754647 to MS.
Acknowledgments
We thank the administration and staff of Manu National Park, the Peruvian Protected Areas Service (SERNANP), and the Peruvian National Forest and Wild Fauna Service (SERFOR) for permission to carry out fieldwork that led to the ideas in this manuscript. CF would also like to thank Greg Asner, Milenka Montoya Pillco, Flor Perez, Lucero Alfaro Curitumay, Rachel Kelly Jordan, Stephen Bechtel, and William Farfan Rios for their assistance in shaping her understanding of landslides in the Peruvian Andes.
Conflict of interest
The authors declare that the research was conducted in the absence of any commercial or financial relationships that could be construed as a potential conflict of interest.
Publisher’s note
All claims expressed in this article are solely those of the authors and do not necessarily represent those of their affiliated organizations, or those of the publisher, the editors and the reviewers. Any product that may be evaluated in this article, or claim that may be made by its manufacturer, is not guaranteed or endorsed by the publisher.
Footnotes
- ^ Freund, CA (2017–2018). Investigating the effects of landslides in Andean tropical forests. (Unpublished data).
References
Amatya, P., Kirschbaum, D., Stanley, T., and Tanyas, H. (2021). Landslide mapping using object-based image analysis and open source tools. Eng. Geol. 282:106000. doi: 10.1016/j.enggeo.2021.106000
Apaza-Quevedo, A., Lippok, D., Hensen, I., Schleuning, M., and Both, S. (2015). Elevation, topography, and edge effects drive functional composition of woody plant species in tropical montane forests. Biotropica 47, 449–458. doi: 10.1111/btp.12232
Aristizábal, E., Garcia, E. F., Marin, R. J., Gómez, F., Guzmán-Martínez, J., Aristizábal, E., et al. (2022). Rainfall-intensity effect on landslide hazard assessment due to climate change in north-western Colombian Andes. Rev. Fac. Ing. Univ. Antioquia 103, 51–66. doi: 10.17533/udea.redin.20201215
Asner, G. P. (2008). “Hyperspectral remote sensing of canopy chemistry, physiology, and biodiversity in tropical rainforests,” in Hyperspectral remote sensing of tropical and sub-tropical forests, eds M. Kalacska and G. A. Sanchez-Azofeifa (Boca Raton, FL: CRC Press), 261–296. doi: 10.1201/9781420053432.ch12
Asner, G. P., Anderson, C. B., Martin, R. E., Knapp, D. E., Tupayachi, R., Sinca, F., et al. (2014a). Landscape-scale changes in forest structure and functional traits along an Andes-to-Amazon elevation gradient. Biogeosciences 11, 843–856. doi: 10.5194/bg-11-843-2014
Asner, G. P., Martin, R. E., Carranza-Jiménez, L., Sinca, F., Tupayachi, R., Anderson, C. B., et al. (2014b). Functional and biological diversity of foliar spectra in tree canopies throughout the Andes to Amazon region. New Phytol. 204, 127–139. doi: 10.1111/nph.12895
Asner, G. P., Martin, R. E., Tupayachi, R., Anderson, C. B., Sinca, F., Carranza-Jiménez, L., et al. (2014c). Amazonian functional diversity from forest canopy chemical assembly. Proc. Natl. Acad. Sci. U.S.A. 111, 5604–5609. doi: 10.1073/pnas.1401181111
Asner, G. P., Martin, R. E., Anderson, C. B., and Knapp, D. E. (2015). Quantifying forest canopy traits: Imaging spectroscopy versus field survey. Remote Sens. Environ. 158, 15–27. doi: 10.1016/j.rse.2014.11.011
Asner, G. P., Martin, R. E., Knapp, D. E., Tupayachi, R., Anderson, C. B., Sinca, F., et al. (2017). Airborne laser-guided imaging spectroscopy to map forest trait diversity and guide conservation. Science 355, 385–389. doi: 10.1126/science.aaj1987
Báez, S., Fadrique, B., Feeley, K., and Homeier, J. (2022b). Changes in tree functional composition across topographic gradients and through time in a tropical montane forest. PLoS One 17:e0263508. doi: 10.1371/journal.pone.0263508
Báez, S., Cayuela, L., Macía, M. J., Álvarez-Dávila, E., Apaza-Quevedo, A., Arnelas, I., et al. (2022a). FunAndes – A functional trait database of Andean plants. Sci. Data 9:511. doi: 10.1038/s41597-022-01626-6
Benz, S. A., and Blum, P. (2019). Global detection of rainfall-triggered landslide clusters. Nat. Hazards Earth Syst. Sci. 19, 1433–1444. doi: 10.5194/nhess-19-1433-2019
Blodgett, T. A., and Isacks, B. L. (2007). Landslide erosion rate in the Eastern Cordillera of northern Bolivia. Earth Interact. 11, 1–30. doi: 10.1175/2007EI222.1
Brenning, A. (2005). Spatial prediction models for landslide hazards: Review, comparison and evaluation. Natural Hazards Earth Syst. Sci. 5, 853–862. doi: 10.5194/nhess-5-853-2005
Brenning, A., Schwinn, M., Ruiz-Páez, A. P., and Muenchow, J. (2015). Landslide susceptibility near highways is increased by 1 order of magnitude in the Andes of southern Ecuador, Loja province. Natural Hazards Earth Syst. Sci. 15, 45–57. doi: 10.5194/nhess-15-45-2015
Breuer, L., Windhorst, D., Fries, A., and Wilcke, W. (2013). “Supporting, regulating, and provisioning hydrological services,” in Ecosystem services, biodiversity and environmental change in a tropical mountain ecosystem of south ecuador ecological studies, eds J. Bendix, E. Beck, A. Bräuning, F. Makeschin, R. Mosandl, S. Scheu, et al. (Berlin: Springer), 107–116. doi: 10.1007/978-3-642-38137-9_9
Brodrick, P. G., Davies, A. B., and Asner, G. P. (2019). Uncovering ecological patterns with convolutional neural networks. Trends Ecol. Evol. 34, 734–745. doi: 10.1016/j.tree.2019.03.006
Brown, J. H., Gupta, V. K., Li, B. L., Milne, B. T., Restrepo, C., and West, G. B. (2002). The fractal nature of nature: Power laws, ecological complexity and biodiversity. Philos. Trans. R. Soc. Biol. Sci. 357, 619–626. doi: 10.1098/rstb.2001.0993
Bruijnzeel, L. A., Mulligan, M., and Scatena, F. N. (2011). Hydrometeorology of tropical montane cloud forests: Emerging patterns. Hydrol. Process. 25, 465–498. doi: 10.1002/hyp.7974
Bush, M. B., Silman, M. R., and Urrego, D. H. (2004). 48,000 years of climate and forest change in a biodiversity hot spot. Science 303, 827–829. doi: 10.1126/science.1090795
Bussmann, R. W., Wilcke, W., and Richter, M. (2008). “Landslides as important disturbance regimes - Causes and regeneration,” in Gradients in a tropical mountain ecosystem of ecuador, eds E. Beck, J. Bendix, I. Kottke, F. Makeschin, and R. Mosandl (Berlin: Springer), 319–330.
Buytaert, W., Vuille, M., Dewulf, A., Urrutia, R., Karmalkar, A., and Célleri, R. (2010). Uncertainties in climate change projections and regional downscaling in the tropical Andes: Implications for water resources management. Hydrology Earth Syst. Sci. 14, 1247–1258. doi: 10.5194/hess-14-1247-2010
Cadenasso, M. L., Traynor, M. M., and Pickett, S. T. A. (1997). Functional location of forest edges: Gradients of multiple physical factors. Can. J. For. Res. 27, 774–782. doi: 10.1139/cjfr-27-5-774
Caplat, P., Anand, M., and Bauch, C. (2008). Interactions between climate change, competition, dispersal, and disturbances in a tree migration model. Theor. Ecol. 1, 209–220. doi: 10.1007/s12080-008-0021-5
Caplat, P., Cheptou, P.-O., Diez, J., Guisan, A., Larson, B. M. H., Macdougall, A. S., et al. (2013). Movement, impacts and management of plant distributions in response to climate change: Insights from invasions. Oikos 122, 1265–1274. doi: 10.1111/j.1600-0706.2013.00430.x
Casagli, N., Intrieri, E., Tofani, V., Gigli, G., and Raspini, F. (2023). Landslide detection, monitoring and prediction with remote-sensing techniques. Nat. Rev. Earth Environ. 4, 51–64. doi: 10.1038/s43017-022-00373-x
Clark, K. E., West, A. J., Hilton, R. G., Asner, G. P., Quesada, C. A., Silman, M. R., et al. (2016). Storm-triggered landslides in the Peruvian Andes and implications for topography, carbon cycles, and biodiversity. Earth Surf. Dyn. 4, 47–70.
Clark, M. L., Roberts, D. A., and Clark, D. B. (2005). Hyperspectral discrimination of tropical rain forest tree species at leaf to crown scales. Remote Sens. Environ. 96, 375–398. doi: 10.1016/j.rse.2005.03.009
Crausbay, S. D., and Martin, P. H. (2016). Natural disturbance, vegetation patterns and ecological dynamics in tropical montane forests. J. Trop. Ecol. 32, 384–403. doi: 10.1017/S0266467416000328
Critical Ecosystem Partnership Fund (2021). Technical summary of the ecosystem profile - Tropical andes biodiversity hotspot. Arlington, VA: Critical Ecosystem Partnership Fund.
Croissant, T., Steer, P., Lague, D., Davy, P., Jeandet, L., and Hilton, R. G. (2019). Seismic cycles, earthquakes, landslides and sediment fluxes: Linking tectonics to surface processes using a reduced-complexity model. Geomorphology 339, 87–103. doi: 10.1016/j.geomorph.2019.04.017
Dalling, J. W. (1994). Vegetation colonization of landslides in the Blue Mountains, Jamaica. Biotropica 26, 392–399.
Delgado, F., Zerathe, S., Schwartz, S., Mathieux, B., and Benavente, C. (2022). Inventory of large landslides along the Central Western Andes (ca. 15°–20° S): Landslide distribution patterns and insights on controlling factors. J. South Am. Earth Sci. 116:103824. doi: 10.1016/j.jsames.2022.103824
Denslow, J. (2000). “Patterns of structure and diversity across a tropical moist forest chronosequence,” in Proceedings of the Vegetation Science in Retrospect and Perspective. IAVS Symposium, (Uppsala: Opulus Press), 237–241.
Densmore, A. L., Anderson, R. S., McAdoo, B. G., and Ellis, M. A. (1997). Hillslope evolution by bedrock landslides. Science 275, 369–372. doi: 10.1126/science.275.5298.369
Díaz, S., Lavorel, S., De Bello, F., Quétier, F., Grigulis, K., and Robson, T. M. (2007). Incorporating plant functional diversity effects in ecosystem service assessments. Proc. Natl. Acad. Sci. U.S.A. 104, 20684–20689. doi: 10.1073/pnas.0704716104
Dislich, C., and Huth, A. (2012). Modelling the impact of shallow landslides on forest structure in tropical montane forests. Ecol. Modell. 239, 40–53. doi: 10.1016/j.ecolmodel.2012.04.016
Duivenvoorden, J. F., and Cuello, A. N. L. (2012). Functional trait state diversity of Andean forests in Venezuela changes with altitude. J. Veg. Sci. 23, 1105–1113. doi: 10.1111/j.1654-1103.2012.01428.x
Duque, A., Peña, M. A., Cuesta, F., González-Caro, S., Kennedy, P., Phillips, O. L., et al. (2021). Mature Andean forests as globally important carbon sinks and future carbon refuges. Nat. Commun. 12:2138. doi: 10.1038/s41467-021-22459-8
Duque, A., Stevenson, P. R., and Feeley, K. J. (2015). Thermophilization of adult and juvenile tree communities in the northern tropical Andes. Proc. Natl. Acad. Sci. U.S.A. 112, 10744–10749. doi: 10.1073/pnas.1506570112
Eghdami, M., and Barros, A. P. (2019). Extreme orographic rainfall in the eastern Andes tied to cold air intrusions. Front. Environ. Sci. 7:101. doi: 10.3389/fenvs.2019.00101
Fadrique, B., Báez, S., Duque, Á, Malizia, A., Blundo, C., Carilla, J., et al. (2018). Widespread but heterogeneous responses of Andean forests to climate change. Nature 564, 207–212. doi: 10.1038/s41586-018-0715-9
Farfan Rios, W. (2019). Forest responses to climate change along an Andes-to-Amazon elevational gradient. Dissertations. Winston-Salem, NC: Wake Forest University. Available online at: http://hdl.handle.net/10339/94320
Fayne, J. V., Ahamed, A., Roberts-Pierel, J., Rumsey, A. C., and Kirschbaum, D. (2019). Automated satellite-based landslide identification product for Nepal. Earth Interact. 23, 1–24. doi: 10.1175/EI-D-17-0022.1
Feeley, K. J., Hurtado, J., Saatchi, S., Silman, M. R., and Clark, D. B. (2013). Compositional shifts in Costa Rican forests due to climate-driven species migrations. Glob. Change Biol. 19, 3472–3480. doi: 10.1111/gcb.12300
Feeley, K. J., Silman, M. R., Bush, M. B., Farfan, W., Cabrera, K. G., Malhi, Y., et al. (2011). Upslope migration of Andean trees. J. Biogeogr. 38, 783–791. doi: 10.1111/j.1365-2699.2010.02444.x
Finer, M., Novoa, S., Weisse, M. J., Petersen, R., Mascaro, J., Souto, T., et al. (2018). Combating deforestation: From satellite to intervention. Science 360, 1303–1305. doi: 10.1126/science.aat1203
Freund, C. A. (2022). Landslide distributions and succession across a 2.5-km Andes-to-Amazon elevational gradient. Available online at: https://wakespace.lib.wfu.edu/bitstream/handle/10339/100781/Freund_wfu_0248D_11737.pdf (accessed October 9, 2022).
Freund, C. A., Clark, K. E., Curran, J. F., Asner, G. P., and Silman, M. R. (2021). Landslide age, elevation and residual vegetation determine tropical montane forest canopy recovery and biomass accumulation after landslide disturbances in the Peruvian Andes. J. Ecol. 109, 3555–3571. doi: 10.1111/1365-2745.13737
Fricker, G. A., Wolf, J. A., Saatchi, S. S., and Gillespie, T. W. (2015). Predicting spatial variations of tree species richness in tropical forests from high-resolution remote sensing. Ecol. Appl. 25, 1776–1789. doi: 10.1890/14-1593.1
Frith, N. V., Hilton, R. G., Howarth, J. D., Gröcke, D. R., Fitzsimons, S. J., Croissant, T., et al. (2018). Carbon export from mountain forests enhanced by earthquake-triggered landslides over millennia. Nat. Geosci. 11, 772–776. doi: 10.1038/s41561-018-0216-3
Furukawa, F., Laneng, L. A., Ando, H., Yoshimura, N., Kaneko, M., and Morimoto, J. (2021). Comparison of RGB and multispectral unmanned aerial vehicle for monitoring vegetation coverage changes on a landslide area. Drones 5:97. doi: 10.3390/drones5030097
Garcia Cabrera, K. (2011). Spatial variability in species composition in Neotropical montane tree communities. Winston-Salem, NC: Wake Forest University.
Gariano, S. L., and Guzzetti, F. (2016). Landslides in a changing climate. Earth Sci. Rev. 162, 227–252. doi: 10.1016/j.earscirev.2016.08.011
Garreaud, R. D. (2009). The Andes climate and weather. Adv. Geosci. 22, 3–11. doi: 10.5194/adgeo-22-3-2009
Garwood, N. C., Janos, D. P., and Brokaw, N. (1979). Earthquake-caused landslides: A major disturbance to tropical forests. Science 205, 997–999. doi: 10.1126/science.205.4410.997
Gehlhausen, S. M., Schwartz, M. W., and Augspurger, C. K. (2000). Vegetation and microclimatic edge effects in two mixed-mesophytic forest fragments. Plant Ecol. 147, 21–35. doi: 10.1023/A:1009846507652
George-Chacon, S. P., Dupuy, J. M., Peduzzi, A., and Hernandez-Stefanoni, J. L. (2019). Combining high resolution satellite imagery and lidar data to model woody species diversity of tropical dry forests. Ecol. Indic. 101, 975–984. doi: 10.1016/j.ecolind.2019.02.015
Ghorbanzadeh, O., Crivellari, A., Ghamisi, P., Shahabi, H., and Blaschke, T. (2021). A comprehensive transferability evaluation of U-Net and ResU-Net for landslide detection from Sentinel-2 data (case study areas from Taiwan, China, and Japan). Sci. Rep. 11:14629. doi: 10.1038/s41598-021-94190-9
Ghorbanzadeh, O., Shahabi, H., Crivellari, A., Homayouni, S., Blaschke, T., and Ghamisi, P. (2022). Landslide detection using deep learning and object-based image analysis. Landslides 19, 929–939. doi: 10.1007/s10346-021-01843-x
Girardin, C. A. J., Farfan-Rios, W., Garcia, K., Feeley, K. J., Jørgensen, P. M., Murakami, A. A., et al. (2014). Spatial patterns of above-ground structure, biomass and composition in a network of six Andean elevation transects. Plant Ecol. Divers. 7, 161–171. doi: 10.1080/17550874.2013.820806
Griffiths, A. R., Silman, M. R., Farfán Rios, W., Feeley, K. J., García Cabrera, K., Meir, P., et al. (2021). Evolutionary heritage shapes tree distributions along an Amazon-to-Andes elevation gradient. Biotropica 53, 38–50. doi: 10.1111/btp.12843
Guariguata, M. R. (1990). Landslide disturbance and forest regeneration in the upper Luquillo mountains of Puero Rico. J. Ecol. 78, 814–832.
Guns, M., and Vanacker, V. (2014). Shifts in landslide frequency–area distribution after forest conversion in the tropical Andes. Anthropocene 6, 75–85. doi: 10.1016/j.ancene.2014.08.001
Guo, F., Lenoir, J., and Bonebrake, T. C. (2018). Land-use change interacts with climate to determine elevational species redistribution. Nat. Commun. 9:1315. doi: 10.1038/s41467-018-03786-9
Guzzetti, F., Carrara, A., Cardinali, M., and Reichenbach, P. (1999). Landslide hazard evaluation: A review of current techniques and their application in a multi-scale study, Central Italy. Geomorphology 31, 181–216. doi: 10.1016/S0169-555X(99)00078-1
Guzzetti, F., Mondini, A. C., Cardinali, M., Fiorucci, F., Santangelo, M., and Chang, K.-T. (2012). Landslide inventory maps: New tools for an old problem. Earth Sci. Rev. 112, 42–66. doi: 10.1016/j.earscirev.2012.02.001
Halladay, K., Malhi, Y., and New, M. (2012a). Cloud frequency climatology at the Andes/Amazon transition: 1. Seasonal and diurnal cycles. J. Geophys. Res. Atmos. 117:D23. doi: 10.1029/2012JD017770
Halladay, K., Malhi, Y., and New, M. (2012b). Cloud frequency climatology at the Andes/Amazon transition: 2. Trends and variability. J. Geophys. Res. Atmos. 117:D23103. doi: 10.1029/2012JD017789
Harper, K. A., Macdonald, S. E., Burton, P. J., Chen, J., Brosofske, K. D., Saunders, S. C., et al. (2005). Edge influence on forest structure and composition in fragmented landscapes. Conserv. Biol. 19, 768–782.
Helmer, E. H., Gerson, E. A., Baggett, L. S., Bird, B. J., Ruzycki, T. S., and Voggesser, S. M. (2019). Neotropical cloud forests and páramo to contract and dry from declines in cloud immersion and frost. PLoS One 14:e0213155. doi: 10.1371/journal.pone.0213155
Hermanns, R. L., Valderamma, P., Faqué, L., Penna, I. M., Sepúlveda, S., Moreiras, S., et al. (2012). Landslides in the Andes and the need to communicate on an interandean level on landslide mapping and research. Rev. Asoc. Geol. Argentina 69, 321–327.
Hierro, R., Burgos Fonseca, Y., Ramezani Ziarani, M., Llamedo, P., Schmidt, T., de la Torre, A., et al. (2020). On the behavior of rainfall maxima at the eastern Andes. Atmos. Res. 234:104792. doi: 10.1016/j.atmosres.2019.104792
Hilton, R. G., and West, A. J. (2020). Mountains, erosion and the carbon cycle. Nat. Rev. Earth Environ. 1, 284–299. doi: 10.1038/s43017-020-0058-6
Hilton, R. G., Galy, A., and Hovius, N. (2008). Riverine particulate organic carbon from an active mountain belt: Importance of landslides. Glob. Biogeochem. Cycles 22:GB1017. doi: 10.1029/2006GB002905
Hilton, R. G., Meunier, P., Hovius, N., Bellingham, P. J., and Galy, A. (2011). Landslide impact on organic carbon cycling in a temperate montane forest. Earth Surf. Process. Landf. 36, 1670–1679. doi: 10.1002/esp.2191
Homeier, J., Seeler, T., Pierick, K., and Leuschner, C. (2021). Leaf trait variation in species-rich tropical Andean forests. Sci. Rep. 11:9993. doi: 10.1038/s41598-021-89190-8
Ibáñez, I., Clark, J. S., and Dietze, M. C. (2009). Estimating colonization potential of migrant tree species. Glob. Change Biol. 15, 1173–1188. doi: 10.1111/j.1365-2486.2008.01777.x
Jankowski, J. E., Merkord, C. L., Rios, W. F., Cabrera, K. G., Revilla, N. S., and Silman, M. R. (2013). The relationship of tropical bird communities to tree species composition and vegetation structure along an Andean elevational gradient. J. Biogeogr. 40, 950–962. doi: 10.1111/jbi.12041
Jetz, W., Cavender-Bares, J., Pavlick, R., Schimel, D., Davis, F. W., Asner, G. P., et al. (2016). Monitoring plant functional diversity from space. Nat. Plants 2, 1–5. doi: 10.1038/nplants.2016.24
Jump, A. S., Huang, T.-J., and Chou, C.-H. (2012). Rapid altitudinal migration of mountain plants in Taiwan and its implications for high altitude biodiversity. Ecography 35, 204–210. doi: 10.1111/j.1600-0587.2011.06984.x
Kalacska, M., Sanchez-Azofeifa, G. A., Rivard, B., Caelli, T., White, H. P., and Calvo-Alvarado, J. C. (2007). Ecological fingerprinting of ecosystem succession: Estimating secondary tropical dry forest structure and diversity using imaging spectroscopy. Remote Sens. Environ. 108, 82–96. doi: 10.1016/j.rse.2006.11.007
Kessler, M. (1999). Plant species richness and endemism during natural landslide succession in a prehumid montane forest in the Bolivian Andes. Ecotropica 5, 123–136.
Kessler, M., and Kluge, J. (2008). Diversity and endemism in tropical montane forests: From patterns to processes. Biodivers. Ecol. Series 2, 35–50.
Korup, O., Clague, J. J., Hermanns, R. L., Hewitt, K., Strom, A. L., and Weidinger, J. T. (2007). Giant landslides, topography, and erosion. Earth Planet. Sci. Lett. 261, 578–589. doi: 10.1016/j.epsl.2007.07.025
Korup, O., Densmore, A. L., and Schlunegger, F. (2010). The role of landslides in mountain range evolution. Geomorphology 120, 77–90. doi: 10.1016/j.geomorph.2009.09.017
Landhäusser, S. M., Deshaies, D., and Lieffers, V. J. (2010). Disturbance facilitates rapid range expansion of aspen into higher elevations of the Rocky Mountains under a warming climate. J. Biogeogr. 37, 68–76. doi: 10.1111/j.1365-2699.2009.02182.x
Larsen, I. J., and Montgomery, D. R. (2012). Landslide erosion coupled to tectonics and river incision. Nat. Geosci. 5, 468–473. doi: 10.1038/ngeo1479
Larsen, M. C., and Torres-Sanchez, A. J. (1992). Landslides triggered by hurricane hugo in Eastern Puerto Rico, September 1989. Caribb. J. Sci. 28, 113–125.
Leithead, M. D., Anand, M., and Silva, L. C. R. (2010). Northward migrating trees establish in treefall gaps at the northern limit of the temperate-boreal ecotone, Ontario, Canada. Oecologia 164, 1095–1106. doi: 10.1007/s00442-010-1769-z
Leithead, M. D., Silva, L. C. R., and Anand, M. (2012). Recruitment patterns and northward tree migration through gap dynamics in an old-growth white pine forest in northern Ontario. Plant Ecol. 213, 1699–1714. doi: 10.1007/sl
Letcher, S. G., and Chazdon, R. L. (2009). Rapid recovery of biomass, species richness, and species composition in a forest chronosequence in Northeastern Costa Rica. Biotropica 41, 608–617. doi: 10.1111/j.1744-7429.2009.00517.x
Ließ, M., Glaser, B., and Huwe, B. (2011). Functional soil-landscape modelling to estimate slope stability in a steep Andean mountain forest region. Geomorphology 132, 287–299. doi: 10.1016/j.geomorph.2011.05.015
Lin, C. Y., Lo, H. M., Chou, W. C., and Lin, W. T. (2004). Vegetation recovery assessment at the Jou-Jou Mountain landslide area caused by the 921 earthquake in Central Taiwan. Ecol. Modell. 176, 75–81. doi: 10.1016/j.ecolmodel.2003.12.037
Lobo, E., and Dalling, J. W. (2014). Spatial scale and sampling resolution affect measures of gap disturbance in a lowland tropical forest: Implications for understanding forest regeneration and carbon storage. Proc. R. Soc. Biol. Sci. 281:20133218. doi: 10.1098/rspb.2013.3218
Lough, D. A. (2017). Vertebrate community changes across a 3200 m Amazon-to-Andes gradient: Composition, structure, and occupancy. Winston-Salem, NC: Wake Forest University.
Lu, P., Shi, W., and Li, Z. (2022). Landslide mapping from planetscope images using improved region-based level set evolution. IEEE Geosci. Remote Sens. Lett. 19, 1–5. doi: 10.1109/LGRS.2021.3122964
Lucas, R., Mitchell, A., and Bunting, P. (2008). “Hyperspectral data for assessing carbon dynamics and biodiversity of forests,” in Hyperspectral remote sensing of tropical and sub-tropical forests, eds M. Kalacska and G. A. Sanchez-Azofeifa (Boca Raton, FL: CRC Press), 47–86.
Lutz, D. A., Powell, R. L., and Silman, M. R. (2013). Four decades of Andean timberline migration and implications for biodiversity loss with climate change. PLoS One 8:e74496. doi: 10.1371/journal.pone.0074496
Magrin, G. O., Marengo, J. A., Boulanger, J.-P., Buckeridege, M. S., Castellanos, E. J., Poveda, G., et al. (2014). “Central and south america,” in Climate Change 2014: Impacts, Adaptation, and Vulnerability. Part B: Regional Aspects. Contribution of Working Group II to the Fifth Assessment Report of the Intergovernmental Panel on Climate Change, eds V. R. Barros, C. B. Field, D. J. Dokken, M. D. Mastrandrea, K. J. Mach, and T. E. Bilir (Cambridge: Cambridge University Press), 1499–1566.
Malhi, Y., Girardin, C. A. J., Goldsmith, G. R., Doughty, C. E., Salinas, N., Metcalfe, D. B., et al. (2017). The variation of productivity and its allocation along a tropical elevation gradient: A whole carbon budget perspective. New Phytol. 214, 1019–1032. doi: 10.1111/nph.14189
Malhi, Y., Silman, M., Salinas, N., Bush, M., Meir, P., and Saatchi, S. (2010). Elevation gradients in the tropics: Laboratories for ecosystem ecology and global change research. Glob. Change Biol. 16, 3171–3175. doi: 10.1111/j.1365-2486.2010.02323.x
Malizia, A., Blundo, C., Carilla, J., Osinaga Acosta, O., Cuesta, F., Duque, A., et al. (2020). Elevation and latitude drives structure and tree species composition in Andean forests: Results from a large-scale plot network. PLoS One 15:e0231553. doi: 10.1371/journal.pone.0231553
Marchand, P., and Houle, G. (2006). Spatial patterns of plant species richness along a forest edge: What are their determinants? For. Ecol. Manag. 223, 113–124. doi: 10.1016/j.foreco.2005.10.064
Martin, P. H., and Bellingham, P. J. (2016). Towards integrated ecological research in tropical montane cloud forests. J. Trop. Ecol. 32, 345–354. doi: 10.1017/S0266467416000432
Marvin, D. C., Asner, G. P., Knapp, D. E., Anderson, C. B., Martin, R. E., Sinca, F., et al. (2014). Amazonian landscapes and the bias in field studies of forest structure and biomass. Proc. Natl. Acad. Sci. U.S.A. 111, E5224–E5232. doi: 10.1073/pnas.1412999111
Meena, S. R., Soares, L. P., Grohmann, C. H., van Westen, C., Bhuyan, K., Singh, R. P., et al. (2022). Landslide detection in the Himalayas using machine learning algorithms and U-Net. Landslides 19, 1209–1229. doi: 10.1007/s10346-022-01861-3
Meier, W. (2013). “Recuperación natural de la vegetación después de derrumbes en la cordillera de la Costa, estado Vargas, Venezuela,” in Recorriendo el paisaje vegetal de Venezuela, eds M. Ernesto, H. Otto, and M. Jafet (Caracas: Instituto Venezolano de Investigaciones Científicas), 211–229.
Mena, J. L., and Pacheco, V. (2020). Mountains and traits: Environmental heterogeneity and mammal assemblages along an elevational gradient in the Northern Andes. Stud. Neotrop. Fauna Environ. 57, 1–13. doi: 10.1080/01650521.2020.1851345
Mirus, B. B., Smith, J. B., and Baum, R. L. (2017). Hydrologic impacts of landslide disturbances: Implications for remobilization and hazard persistence. Water Resour. Res. 53, 8250–8265. doi: 10.1002/2017WR020842
Mittermeier, R. A., Turner, W. R., Larsen, F. W., Brooks, T. M., and Gascon, C. (2011). Global biodiversity conservation: The critical role of hotspots. Biodivers. Hotspots 49, 3–22. doi: 10.5860/choice.49-4434
Morales, B., Garcia-Pedrero, A., Lizama, E., Lillo-Saavedra, M., Gonzalo-Martín, C., Chen, N., et al. (2022). Patagonian andes landslides inventory: The deep learning’s way to their automatic detection. Remote Sens. 14:4622. doi: 10.3390/rs14184622
Moura, M. M., de Oliveira, L. E. S., Sanquetta, C. R., Bastos, A., Mohan, M., and Corte, A. P. D. (2021). Towards Amazon forest restoration: Automatic detection of species from UAV imagery. Remote Sens. 13:2627. doi: 10.3390/rs13132627
Muenchow, J., Brenning, A., and Richter, M. (2012). Geomorphic process rates of landslides along a humidity gradient in the tropical Andes. Geomorphology 13, 271–284. doi: 10.1016/j.geomorph.2011.10.029
Murcia, C. (1995). Edge effects in fragmented forests: Implications for conservation. Trends Ecol. Evol. 10, 58–62.
Myers, N., Mittermeier, R. A., Mittermeier, C. G., da Fonseca, G. A. B., and Kent, J. (2000). Biodiversity hotspots for conservation priorities. Nature 403:5. doi: 10.1038/35002501
Ohl, C., and Bussmann, R. W. (2004). Recolonisation of natural landslides in tropical mountain forests of Southern Ecuador. Feddes Repert. 115, 248–264. doi: 10.1002/fedr.200311041
Palacio Cordoba, J., Mergili, M., and Aristizábal, E. (2020). Probabilistic landslide susceptibility analysis in tropical mountainous terrain using the physically based r.slope.stability model. Nat. Hazards Earth Syst. Sci. 20, 815–829. doi: 10.5194/nhess-20-815-2020
Pan, Y., Birdsey, R. A., Fang, J., Houghton, R., Kauppi, P. E., Kurz, W. A., et al. (2011). A large and persistent carbon sink in the world’s forests. Science 333, 988–993.
Patterson, B. D., Stotz, D. F., Solari, S., Fitzpatrick, J. W., and Pacheco, V. (1998). Contrasting patterns of elevational zonation for birds and mammals in the Andes of southeastern Peru. J. Biogeogr. 25, 593–607. doi: 10.1046/j.1365-2699.1998.2530593.x
Peck, M., Mariscal, A., Padbury, M., Cane, T., Kniveton, D., and Chinchero, M. A. (2012). Identifying tropical Ecuadorian Andean trees from inter-crown pixel distributions in hyperspatial aerial imagery. Appl. Veg. Sci. 15, 548–559. doi: 10.1111/j.1654-109X.2012.01196.x
Pelletier, J. D., Malamud, B. D., Blodgett, T., and Turcotte, D. L. (1997). Scale-invariance of soil moisture variability and its implications for the frequency-size distribution of landslides. Eng. Geol. 48, 255–268. doi: 10.1016/S0013-7952(97)00041-0
Pérez-Escobar, O. A., Zizka, A., Bermúdez, M. A., Meseguer, A. S., Condamine, F. L., Hoorn, C., et al. (2022). The Andes through time: Evolution and distribution of Andean floras. Trends Plant Sci. 27, 364–378. doi: 10.1016/j.tplants.2021.09.010
Petley, D. (2012). “Remote sensing techniques and landslides,” in Landslides: Types, mechanisms and modeling, eds J. J. Clague and D. Stead (Cambridge: Cambridge University Press).
Pierick, K., Leuschner, C., and Homeier, J. (2021). Topography as a factor driving small-scale variation in tree fine root traits and root functional diversity in a species-rich tropical montane forest. New Phytol. 230, 129–138. doi: 10.1111/nph.17136
Pierick, K., Link, R. M., Leuschner, C., and Homeier, J. (2023). Elevational trends of tree fine root traits in species-rich tropical Andean forests. Oikos 2023:e08975. doi: 10.1111/oik.08975
Porder, S., Paytan, A., and Vitousek, P. M. (2005). Erosion and landscape development affect plant nutrient status in the Hawaiian Islands. Oecologia 142, 440–449. doi: 10.1007/s00442-004-1743-8
Prakash, N., Manconi, A., and Loew, S. (2021). A new strategy to map landslides with a generalized convolutional neural network. Sci. Rep. 11:9722. doi: 10.1038/s41598-021-89015-8
Ramos Scharrón, C. E., Castellanos, E. J., and Restrepo, C. (2012). The transfer of modern organic carbon by landslide activity in tropical montane ecosystems. J. Geophys. Res. Biogeosci. 117:G3. doi: 10.1029/2011JG001838
Razafindratsima, O. H., Brown, K. A., Carvalho, F., Johnson, S. E., Wright, P. C., and Dunham, A. E. (2018). Edge effects on components of diversity and above-ground biomass in a tropical rainforest. J. Appl. Ecol. 55, 977–985. doi: 10.1111/1365-2664.12985
Restrepo, C., and Alvarez, N. (2006). Landslides and their contribution to land-cover change in the mountains of Mexico and Central America. Biotropica 38, 446–457.
Restrepo, C., and Vitousek, P. (2001). Landslides, alien species, and the diversity of a hawaiian montane mesic ecosystem. Biotropica 33, 409–420. doi: 10.1111/j.1744-7429.2001.tb00195.x
Restrepo, C., Walker, L. R., Shiels, A. B., Bussmann, R., Claessens, L., Fisch, S., et al. (2009). Landsliding and its multiscale influence on mountainscapes. BioScience 59, 685–698. doi: 10.1525/bio.2009.59.8.10
Richter, M., Diertl, K. H., Emck, P., Peters, T., and Beck, E. (2009). Reasons for an outstanding plant diversity in the tropical Andes of Southern Ecuador. Landsc. Online 12, 1–35. doi: 10.3097/LO.200912
Ries, L., Fletch, R. J. Jr., Battin, J., Sisk, T. D., and Fletcher, R. J. (2004). Ecological responses to habitat edges: Mechanisms, models, and variability explained. Annu. Rev. Ecol. Evol. Syst. 35, 491–522.
Roa Lobo, J. G. (2007). Identifying landslide hazards in a tropical mountain environment, using geomorphologic and probabilistic approaches. Available online at: https://www.proquest.com/docview/304854586/abstract/4E06822645764730PQ/1 (accessed March 18, 2023).
Roy, D. P., Huang, H., Houborg, R., and Martins, V. S. (2021). A global analysis of the temporal availability of PlanetScope high spatial resolution multi-spectral imagery. Remote Sens. Environ. 264:112586. doi: 10.1016/j.rse.2021.112586
Saito, H., Uchiyama, S., and Teshirogi, K. (2022). Rapid vegetation recovery at landslide scars detected by multitemporal high-resolution satellite imagery at Aso volcano, Japan. Geomorphology 398:107989. doi: 10.1016/j.geomorph.2021.107989
Sarmiento, F. O., and Kooperman, G. J. (2019). A socio-hydrological perspective on recent and future precipitation changes over tropical montane cloud forests in the Andes. Front. Earth Sci. 7:324. doi: 10.3389/feart.2019.00324
Segura, H., Junquas, C., Espinoza, J. C., Vuille, M., Jauregui, Y. R., Rabatel, A., et al. (2019). New insights into the rainfall variability in the tropical Andes on seasonal and interannual time scales. Clim. Dyn. 53, 405–426. doi: 10.1007/s00382-018-4590-8
Shi, Y., Skidmore, A. K., Wang, T., Holzwarth, S., Heiden, U., Pinnel, N., et al. (2018). Tree species classification using plant functional traits from LiDAR and hyperspectral data. Int. J. Appl. Earth Observ. Geoinf. 73, 207–219. doi: 10.1016/j.jag.2018.06.018
Sierra, J. P., Junquas, C., Espinoza, J. C., Segura, H., Condom, T., Andrade, M., et al. (2022). Deforestation impacts on Amazon-Andes hydroclimatic connectivity. Clim. Dyn. 58, 2609–2636. doi: 10.1007/s00382-021-06025-y
Slik, J. W. F. (2005). Assessing tropical lowland forest disturbance using plant morphological and ecological attributes. For. Ecol. Manag. 205, 241–250. doi: 10.1016/j.foreco.2004.10.011
Spasojevic, M. J., Grace, J. B., Harrison, S., and Damschen, E. I. (2014). Functional diversity supports the physiological tolerance hypothesis for plant species richness along climatic gradients. J. Ecol. 102, 447–455. doi: 10.1111/1365-2745.12204
Spracklen, D. V., and Righelato, R. (2014). Tropical montane forests are a larger than expected global carbon store. Biogeosciences 11, 2741–2754. doi: 10.5194/bg-11-2741-2014
Spracklen, D. V., and Righelato, R. (2016). Carbon storage and sequestration of re-growing montane forests in southern Ecuador. For. Ecol. Manag. 364, 139–144. doi: 10.1016/j.foreco.2016.01.001
Stark, C. P., and Hovius, N. (2001). The characterization of landslide size distributions. Geophys. Res. Lett. 28, 1091–1094.
Stern, M. J. (1995). “Vegetation recovery on earthquake-triggered landslide sites in the Ecuadorian Andes,” in Biodiversity and conservation of neotropical montane forests: Proceedings of the neotropical montane forest biodiversity and conservation symposium, eds S. P. Churchill, H. Balslev, E. Forero, and J. L. Luteyn (Bronx, NY: The New York Botanical Garden), 207–220.
Still, C. J., Foster, P. N., and Schneider, S. H. (1999). Simulating the effects of climate change on tropical montane cloud forests. Nature 398, 608–610. doi: 10.1038/19293
Tanner, E. V. J., Bellingham, P. J., Healey, J. R., and Feeley, K. J. (2022). Hurricane disturbance accelerated the thermophilization of a Jamaican montane forest. Ecography 2022:e06100. doi: 10.1111/ecog.06100
ter Steege, H., and Hammond, D. S. (2001). Character convergence, diversity, and disturbance in tropical rain forest in Guyana. Ecology 82, 3197–3212.
Townsend-Small, A., McClain, M. E., Hall, B., Noguera, J. L., Llerena, C. A., and Brandes, J. A. (2008). Suspended sediments and organic matter in mountain headwaters of the Amazon River: Results from a 1-year time series study in the central Peruvian Andes. Geochim. Cosmochim. Acta 72, 732–740. doi: 10.1016/j.gca.2007.11.020
Urrutia, R., and Vuille, M. (2009). Climate change projections for the tropical Andes using a regional climate model: Temperature and precipitation simulations for the end of the 21st century. J. Geophys. Res. 114:D02108. doi: 10.1029/2008JD011021
Vanacker, V., Guns, M., Clapuyt, F., Balthazar, V., Tenorio, G., and Molina, A. (2020). Distribución espacio-temporal de los deslizamientos y erosión hídrica en una cuenca Andina tropical. Pirineos 175:051. doi: 10.3989/pirineos.2020.175001
Vanacker, V., Molina, A., Govers, G., Poesen, J., and Deckers, J. (2007). Spatial variation of suspended sediment concentrations in a tropical Andean river system: The Paute River, southern Ecuador. Geomorphology 87, 53–67. doi: 10.1016/j.geomorph.2006.06.042
Vellico, M., Sterzai, P., Pietrapertosa, C., Mora, P., Berti, M., Corsini, A., et al. (2010). Hyperspectral and thermal methodologies applied to landslide monitoring. Vienna: EGU General Assembly.
Veras, H. F. P., Ferreira, M. P., da Cunha Neto, E. M., Figueiredo, E. O., Corte, A. P. D., and Sanquetta, C. R. (2022). Fusing multi-season UAS images with convolutional neural networks to map tree species in Amazonian forests. Ecol. Inform. 71:101815. doi: 10.1016/j.ecoinf.2022.101815
Vitousek, P., Chadwick, O., Matson, P., Allison, S., Derry, L., Kettley, L., et al. (2003). Erosion and the rejuvenation of weathering-derived nutrient supply in an old tropical landscape. Ecosystems 6, 762–772. doi: 10.1007/s10021-003-0199-8
Wagner, F. H., Ferreira, M. P., Sanchez, A., Hirye, M. C. M., Zortea, M., Gloor, E., et al. (2018). Individual tree crown delineation in a highly diverse tropical forest using very high resolution satellite images. ISPRS J. Photogramm. Remote Sens. 145, 362–377. doi: 10.1016/j.isprsjprs.2018.09.013
Walker, L. R., Landau, F. H., Velázquez, E., Shiels, A. B., and Sparrow, A. D. (2010). Early successional woody plants facilitate and ferns inhibit forest development on Puerto Rican landslides. J. Ecol. 98, 625–635. doi: 10.1111/j.1365-2745.2010.01641.x
Walker, L. R., Zarin, D. J., Fetcher, N., Myster, R. W., and Johnson, A. H. (1996). Ecosystem development and plant succession on landslides in the Caribbean. Biotropica 28, 566–576.
Yang, S., Wang, Y., Wang, P., Mu, J., Jiao, S., Zhao, X., et al. (2022). Automatic identification of landslides based on deep learning. Appl. Sci. 12:8153. doi: 10.3390/app12168153
Ye, C., Li, Y., Cui, P., Liang, L., Pirasteh, S., Marcato, J., et al. (2019). Landslide detection of hyperspectral remote sensing data based on deep learning with constrains. IEEE J. Sel. Top. Appl. Earth Observ. Remote Sens. 12, 5047–5060. doi: 10.1109/JSTARS.2019.2951725
Younes Cárdenas, N., and Erazo Mera, E. (2016). Landslide susceptibility analysis using remote sensing and GIS in the western Ecuadorian Andes. Nat. Hazards 81, 1829–1859. doi: 10.1007/s11069-016-2157-8
Young, K. R. (1993). Tropical timberlines: Changes in forest structure and regeneration between two peruvian timberline margins. Arctic Alpine Res. 25, 167–174. doi: 10.1080/00040851.1993.12003000
Young, K. R., León, B., Jørgensen, P. M., and Ulloa Ulloa, C. (2007). “Tropical and subtropical landscapes of the andes,” in The physical geography of South America, eds T. T. Veblen, K. R. Young, and A. R. Orme (Oxford: Oxford University Press), 200–216.
Zarin, D. J., and Johnson, A. H. (1995). Nutrient accumulation during primary succession in a montane tropical forest, Puerto Rico. Soil Sci. Soc. Am. 59, 1444–1452.
Zhang, C., Atkinson, P. M., George, C., Wen, Z., Diazgranados, M., and Gerard, F. (2020). Identifying and mapping individual plants in a highly diverse high-elevation ecosystem using UAV imagery and deep learning. ISPRS J. Photogramm. Remote Sens. 169, 280–291. doi: 10.1016/j.isprsjprs.2020.09.025
Keywords: Andes, natural disturbance, landslide, forest regeneration, tropical montane forest
Citation: Freund CA and Silman MR (2023) Developing a more complete understanding of tropical montane forest disturbance ecology through landslide research. Front. For. Glob. Change 6:1091387. doi: 10.3389/ffgc.2023.1091387
Received: 07 November 2022; Accepted: 02 May 2023;
Published: 18 May 2023.
Edited by:
Alexandra C. Morel, University of Dundee, United KingdomReviewed by:
Carla Restrepo, University of Puerto Rico at Río Piedras, Puerto RicoNathan Brooks English, Central Queensland University, Australia
Copyright © 2023 Freund and Silman. This is an open-access article distributed under the terms of the Creative Commons Attribution License (CC BY). The use, distribution or reproduction in other forums is permitted, provided the original author(s) and the copyright owner(s) are credited and that the original publication in this journal is cited, in accordance with accepted academic practice. No use, distribution or reproduction is permitted which does not comply with these terms.
*Correspondence: Miles R. Silman, c2lsbWFubXJAd2Z1LmVkdQ==