- Department of Forest and Conservation Sciences, University of British Columbia, Forestry, Vancouver, BC, Canada
Every year, salmon return to their natal streams to spawn. Their return represents an ecosystem subsidy of nutrients and energy from the sea to the land; these materials feed into terrestrial food webs, plant communities, and forest soils. Here we test the long term effects of salmon inputs on soil fertility by sampling soils from Haíɫzaqv (Heiltsuk) Nation territory on the Central Coast of British Columbia, Canada. A total of 20 soil chemical properties were assessed via two sampling methodologies: first, across 23 watersheds representing a regional gradient of salmon density (kg of salmon per meter of stream reach per year); and second, at four sites above and below waterfalls that blocked salmon migration. At each sampling point, soil material at two depths was collected. Multivariate analysis regional gradient showed salmon density, the moss community, and the shrub community to be significant factors related to soil chemistry. Similarly, being above or below the waterfall, the moss community, and the tree community were significant in the waterfall comparison. Generalized linear mixed models along regional salmon density gradient showed an increase in nitrate (NO3−) correlated with salmon inputs (p < 0.05), and moderately significant (p < 0.1) increases in ammonium (NH4+), phosphorus (P), aluminum (Al), and copper (Cu). Net cation exchange capacity (CEC) did not change; however, magnesium (Mg) significantly decreased along these gradients (p < 0.05), while sodium (Na) had a declining tendency and calcium (Ca) had an increasing tendency. Being below salmon-blocking waterfalls or the salmon density below falls was a factor in higher total nitrogen, nitrate, ammonium, phosphorus, total sulfur (S), magnesium, and sodium concentrations; below falls sites also had lower pH and aluminum. Exploratory analysis of the regional gradient data using a random forest model apportioned high importance to soil depth, the moss community, the shrub community, salmon density and distance from the stream. These results show that salmon inputs are correlated with a number of changes in recipient soils, and these are consistent with an interpretation of improved fertility at these sites.
1 Introduction
Every autumn, migratory Pacific salmon (Oncorhynchus sp.) return to their native streams to spawn and die. They carry with them a lifetime’s accumulation of nutrients stored in their bodies; this high-protein, high-fat material represents an ecosystem subsidy of marine-derived nutrients (MDN) from the ocean to riparian zones. These nutrients permeate stream environments, increasing aquatic nutrient loads (Levi et al., 2013); biofilm and plankton biomass (Collins et al., 2015); and change fish abundance, individual fish biomass, behavior, and spawning success (Swain and Reynolds, 2015; Collins et al., 2016). Salmon presence also affects nearby terrestrial sites by changing plant communities and increasing the abundance of nitrophyllic plant species (Mathewson et al., 2003; Hocking and Reynolds, 2011); increasing plant growth, fruit growth, and other plant traits (Sayer, 2008; Quinn et al., 2018; Siemens et al., 2020; Dennert et al., 2023); and increasing bird diversity and abundance (Christie and Reimchen, 2008; Wagner and Reynolds, 2019). In addition, their carcasses provide food for numerous large mammals such as bears and wolves (Hocking et al., 2009; Reimchen, 2017), small mammals such as mink and river otter (Dolloff, 1993; Ben-David et al., 1997), and insects (Hocking and Reimchen, 2006; Hocking et al., 2009; Reimchen, 2017; Rammell et al., 2021). These myriad effects have contributed to salmon being identified as a keystone species in structuring the ecology of riparian “salmon forests” (Helfield and Naiman, 2006; Walsh et al., 2020).
Nutrients from salmon reach terrestrial environments either via uptake by plants or by displacement by animals. Plant uptake can come from the stream itself or via diffusion through the hyporheic zone (Keefe and Edwards, 2003; Pinay et al., 2009). Insects represent the largest animal vector by mass (Reimchen, 2017), followed by bear consumption and distribution of carcass remnants (Cederholm et al., 1989; Reimchen, 2017). Urine, feces, and partially consumed carcasses represent three chemically distinct vectors of nutrient transfer to terrestrial environments (Cederholm et al., 1999). Urine contributes mostly nitrogen in ureic form, while salmon carcasses contain large amounts of N, P, K, Ca, and S, as well as smaller amounts of Mg, Fe, Mn, and other substances (Drake et al., 2005).
Ultimately, these nutrients either pass through or accumulate in the soil. The effects of MDN on soil have been examined using a variety of approaches, including assessing soil nutrients across regional salmon gradients (Reimchen et al., 2003; Hocking and Reynolds, 2011); above and below barriers to salmon migration (waterfalls or dams; Helfield and Naiman, 2001; Bartz and Naiman, 2005; Gende et al., 2007; Perry et al., 2017); and carcass addition or displacement experiments (Drake et al., 2005; Hocking and Reynolds, 2012; Wheeler et al., 2014; Wheeler and Kavanagh, 2017; Feddern et al., 2019; D’Amore et al., 2020). In other studies, soils have been sampled perpendicular to streams (Scott et al., 2008); across chronosequences of alluvial deposits (D’Amore et al., 2011, 2020; Morris and Stanford, 2011); and inside and outside bear exclosures (Holtgrieve et al., 2009). Salmon flesh is isotopically enriched in 15N due to its marine origin and because salmon are high-trophic level predators. Thus, the methodologies described above have often used nitrogen isotopic signatures as a primary response variable. This has shown increased δ15N (Reimchen et al., 2003; Bartz and Naiman, 2005; Morris et al., 2005; Gende et al., 2007; Morris and Stanford, 2011; Wheeler and Kavanagh, 2017; Feddern et al., 2019), decreased δ15N (Scott et al., 2008), or no change (Perry et al., 2017) along gradients of salmon nutrient inputs. In addition, MDN have been shown to be associated with increased total soil N (Bartz and Naiman, 2005; Gende et al., 2007; Holtgrieve et al., 2009; Morris and Stanford, 2011; Wheeler et al., 2014; Perry et al., 2017; Wheeler and Kavanagh, 2017), nitrate (Drake et al., 2005; Gende et al., 2007; Holtgrieve et al., 2009), ammonium (Drake et al., 2005; Gende et al., 2007; Holtgrieve et al., 2009), and NO2 (gas; Holtgrieve et al., 2009) concentrations. Despite some studies finding no effect of MDN on total N (Scott et al., 2008), and no effect on nitrate and ammonium (Feddern et al., 2019), the preponderance of evidence points to an increase in soil 15N, soil nitrogen, and soil nitrogen compounds in response to salmon inputs.
Soil characteristics other than nitrogen have been more rarely studied in salmon forest soil assessments. Phosphorus, critical for the health of plant proteins, nucleic acids, and photosynthesis (Maathuis, 2009), has been found to have variable responses to MDN addition, and studies have found it to increase (Drake et al., 2005), not change (Bartz and Naiman, 2005) or decrease (Perry et al., 2017) in response to increased salmon inputs. Calcium, potassium, and other exchangeable cations (sodium, magnesium, and aggregate cation exchange capacity) are also important for plant cell signaling, photosynthesis, and the structuring of cell walls (Maathuis, 2009). Two studies that examined soil cations reported conflicting results in cation concentrations, one increasing and one decreasing, in response to salmon inputs (Drake et al., 2005; Perry et al., 2017). These same two studies reported that salmon inputs were correlated with increases in soil sulfur, iron, and manganese.
pH has rarely been reported in the salmon forest literature, despite being a major driver of both soil chemistry (Strawn et al., 2020) and microbial community composition (Delgado-Baquerizo et al., 2018). Its response to salmon inputs has only been reported in a single study where it was found to be higher below than above salmon-blocking dams (Perry et al., 2017). Carbon is increasingly being recognized as another soil “master variable” that mediates all other soil functions (Schmidt et al., 2011; Solly et al., 2020). Carbon has received considerable attention in recent years for its role in climate change, as well as a mediator of soil redox potential that positively correlates with soil fertility (Diacono and Montemurro, 2011; Schmidt et al., 2011; Jiang et al., 2018). Three studies have examined soil carbon with regards to salmon inputs, and two found that soil carbon stocks increased and CO2 flux decreased with salmon inputs (Wheeler et al., 2014; Wheeler and Kavanagh, 2017), and one showed mixed results of both increases and decreases of soil carbon along a series of salmon-blocking dams (Perry et al., 2017).
Soil fertility is a integrative concept that accounts for nutrient availability, water conditions, soil aeration and physical stability, and the absence of substances that inhibit plant growth (Patzel et al., 2000; Fisher et al., 2012; Nicolodi and Gianello, 2014). Temperate rain forests are considered nitrogen-limited (Edmonds et al., 1989; LeBauer and Treseder, 2008), and for this reason studies focused on marine-derived nitrogen dynamics have been thought to be most salient on north Pacific rainforest sites (Oliver et al., 2017). However, phosphorus (Richardson et al., 2004; Kranabetter et al., 2005), calcium (Perakis et al., 2013; Trant et al., 2016), and co-limitations of calcium with nitrogen (Perakis et al., 2006; Prescott et al., 2013; Hynicka et al., 2016; Kranabetter et al., 2020), and phosphorus with nitrogen (Blevins et al., 2006), have also been implicated in limiting productivity in temperate rainforests. Assessments of soil fertility should treat soil as a complex system (Nicolodi and Gianello, 2014), and consider a suite of soil nutrients and physical characteristics that support plant growth. Soil fertility can be thought of as a set of correlated conditions that represent a “fertile state,” and is associated with high nitrogen, phosphorous, and exchangeable cation concentrations (primarily Ca2+, K+, and Mg+); moderate pH; high soil organic matter; and low iron and aluminum concentrations (Chatterjee and Clay, 2016). Salmon carcasses contain many of the elements of this set, including nitrogen and nitrogenous substances (proteins, urea, ammonium, and nitrate); organic elements such as phosphorus, sulfur and potassium (proteins); and other exchangeable cations (particularly calcium in their bones). Thus, it may be expected that salmon inputs are contributing to the transition to, and maintenance of, fertile soils.
The goal of this study was to assess the long-term effects of salmon inputs on soil in the Central Coast of British Columbia, Canada. Our objectives were to: (O1) assess whether salmon inputs are associated with changes in soil chemical properties, with particular attention given to expected changes with respect to soil fertility; and (O2) establish baselines of soil chemical properties for riparian salmon forests in the BC Central Coast region. We hypothesized that salmon would increase soil fertility, and thus predict that salmon inputs would be correlated with higher soil nitrogen and nitrogenous substances (% total N, NH4+, NO3−); higher exchangeable phosphorus (P); and higher exchangeable cations (K+, Mg2+, Na+, CEC, and Ca2+). In addition, the hypothesis of increased fertility predicts that changes in soil acidity (pH) will be moderate; ions that negatively affect plant growth such as Al3+ and Fe will decrease with salmon inputs; and soil carbon will increase. Since sulfur, manganese, iron, and zinc are also components of salmon carcasses, we predict that these will also increase with salmon inputs.
To test these predictions, two natural-experiment study designs were used to observe the effects of variation in salmon inputs. The first design used a “between watershed” approach on 23 well-studied watersheds representing a “regional gradient” of salmon density, measured as kg of salmon per meter of stream reach per year [kgsalmon/(mstream yr)] (Hocking and Reynolds, 2011). The second used a “within-watershed” approach by making “above and below” waterfall comparisons at four watersheds where waterfalls block salmon migration, and which thus representing a sharp discontinuity in MDN inputs within individual watersheds. At each site, we made 20 chemical measurements, including nitrogenous substances (total soil N, ammonium-N, and nitrate-N); phosphorus (P), and pH. We also measured carbon (%C and loss-on-ignition), sulfur (%S and extractible), and extractible manganese (Mn). Extractible cations were measured, including calcium (Ca), potassium (K), magnesium (Mg), sodium (Na), and total CEC; and metals, including extractible aluminum (Al), iron (Fe), copper (Cu), zinc (Zn), and boron (B). In order to measure changes with depth in the soil profile, samples were taken at two soil depths: 0–5 cm, representative of the forest floor and shallow soil; and 10–15 cm, representative of organic deeper layers, while staying within the zone of metabolically important fine root density. Finally, to control for the effects of litter and root exudates on soil properties, four layers of riparian vegetation were measured as covariates, including moss, herb, shrub, and tree layers, with additional covariates examined using a machine-learning approach. This study thus assesses a complex of soil chemical properties across two datasets and two soil depths to evaluate the contribution of marine-derived salmon inputs to soil fertility in the Pacific Northwest.
2 Materials and methods
2.1 Study sites and timing
The study was conducted in Haíɫzaqv (Heiltsuk) Nation territory in the Central Coast Regional District of British Columbia, Canada, near the community of Bella Bella (Supplementary Figure S1-1; Supplementary Table S1-2). These study sites are in the Central variant of the Very Wet Hypermaritime subzone of the Coastal Western Hemlock biogeoclimatic zone (CWHvh2). Dominated by a cool maritime climate with high rainfall, the landscape is a mosaic of highland bogs where water is stagnant, and forested slopes where water sheds (Lamb and Megill, 2003). The forested riparian zones are a mix of western hemlock (Tsuga heterophylla), western red cedar (Thuja plicata), Amabilis fir (Abies amabilis), and Sitka spruce (Picea sitchensis), with red alder (Alnus rubra) appearing in disturbed sites. The understory includes huckleberry and blueberry species (Vaccinium parvifolium, V. alaskensae, and V. ovalifolium), false azalea (Rhododendron menziesii), salal (Gaultheria shallon), salmonberry (Rubus spectabilis), and devil’s club (Oploplanax horridus).
Regional geology is primarily alkaline and peralkaline geochemistry (Souther, 1986), and recent glacial dynamics imply that soil formation has occurred only in the last ~14,000 years (Eamer et al., 2017). Ferro-Humic Podzols (using the Canadian classification system; World Reference Base for Soil Resources – Podsols; USDA soil taxonomy—Spodosols) dominate the broader coastal region, extending from northern Vancouver Island to the Alaskan border. The Central Coast, however, is characterized by a narrow coastal band of Folisols (WRB—Folic Histosols; USDA—Folists) that encompass the study sites (Carpenter et al., 2014). The soils found here matched these soil maps and were primarily Humic and Lignic Folisols (Organic order, Folisol great group; n = 54), with the occasional Ferro-Humic Podzol (Podzolic order, Ferro-Humic great group; n = 4; Fox et al., 1987). These Folisols were largely of homogenous organic composition down to gravel or bedrock.
A total of 23 streams were included in the study, representing a gradient of salmon density (0–67 kg salmon per meter of stream reach per year). These streams have been well studied previously (Hocking and Reynolds, 2011) and have ongoing long term (>16 years) observational time series of salmon density. Four of the 23 streams had waterfalls, which were utilized for above/below waterfall comparisons (Supplementary Figure S1-1). This study was conducted in July and August to be as distant annually from the salmon run as possible, and in order to emphasize long-term and legacy effects of salmon deposition.
2.2 Soil sampling
Two soil cores were collected from each stream, with an additional two cores above waterfalls in the four watersheds where they occurred. Samples were taken from microsites of flat, deep soil (>50 cm), within 100 m of the stream mouth, within 30 m perpendicular distance to the stream. Twenty meters from the stream mouth we entered the forest and used an opportunistic sampling protocol that attempted to keep the species of the nearest large tree balanced across the study while maintaining proximity to the stream and suitable soil depth for our sampling protocol. We sampled the first location that matched these characteristics, and then repeated the process on the other side of the stream. The average sampling location was 12.8 m from the stream. At each microsite, a small soil pit was dug to 50 cm depth and the soil profile described (Supplementary Table S2-1). GPS coordinates, distance from the stream mouth, and distance to the closest bank were recorded. Forest floors were generally dominated by decomposing moss and conifer needles. These moss and coarse litter layers were gently removed and the top 5 cm collected as a single sample (hereafter called “shallow soil”). The underlying 10–15 cm of organic soil was collected as a second sample (hereafter referred to as “deep” or “deeper soil”).
2.3 Plant community
To account for the effect of the plant community on soil chemistry, vegetation surveys were conducted at each plot. First, percent cover of each moss and herb species was visually estimated by AL in a 1 m2 plot centered on the soil core. Juvenile shrubs (<5 cm) were included in the herb layer. This resulted in an estimate for the abundance of each moss and herb species j in each plot. Second, the effect of shrubs and trees on the soil core was estimated by characterizing a larger 5 m radius circular plot around the soil core. Shrubs with height > 1 m were identified to species and their aboveground volume estimated by measuring their cubic volume (). Their distance and bearing to the core were measured, and these figures summed to estimate the effect of each individual shrub on the soil core as a “shrub influence index,” calculated as:
This measurement thus attributes more “influence” on the soil core to a shrub that is larger and closer than a shrub that is smaller and further away.
Third, every tree with circumference > 2 cm in the 5 m radius plot was measured. Tree species identity, circumference, distance and bearing from the core were measured. Similar to shrubs, a “tree influence index” was calculated as:
with larger influence thus being attributed to trees of larger circumference closer to the soil core. Finally, for each tree and shrub species j the influence indices of all individuals in each plot were summed, giving an estimate of the aggregate effect of the influence of species j on the sampled soil core.
Principal component analysis (PCA) was used to reduce the dimensionality of these four vegetation indices (moss and herb percent cover, and shrub and tree influence indices) to use as explanatory variables in downstream multivariate ordinations, GLMs, and machine learning models. In each vegetation PCA, the variation explained and major loading for the first principal axis was: moss (36% of variation explained; positive direction along the principal axis—Rhytidiadelphus loreus); herbs (35%; positive direction—Gaultheria shallon and Blechnum spicant; negative direction—Maianthemum dilatatum and Dryopteris expansa); shrubs (34%; positive direction—Gaultheria shallon; negative direction—Rhododendron menziesii and Vaccinium sp.); trees (39% of variation; negative direction—Thuja plicata).
2.4 Soil analyses
Soil chemical analyses were carried out by the British Columbia Ministry of Environment & Climate Change Strategy Analytical Laboratory in Victoria, British Columbia, Canada. Total percent C, N, and S were volatilized via combustion and analyzed using a Thermo Flash 2000 elemental analyzer. Nitrate and ammonium were extracted with a 2 N KCl extraction (Carter and Gregorich, 2007) and analyzed using an Astoria Pacific A2 auto-analyzer. Loss on ignition (LOI) was calculated via complete combustion in an ashing furnace and measured using an analytical balance, and pH was measured with an ion meter diluted 1:1 with distilled water. All other elements were extracted using a 0.1 N Barium Chloride Extraction (Hendershot and Duquette, 1986) and/or a Mehlich III extraction (Mehlich, 1984) and analyzed with a Teledyne Leeman Prodigy ICP-OES. Soil tests, units, and methods are summarized in Supplementary Table S3-1.
2.5 Salmon density data
Annual fish count data (Supplementary material 4) were contributed by Dr. John Reynolds’ research team at Simon Fraser University, the Canadian Department of Fisheries and Oceans, and the Heiltsuk Integrated Resource Management Department. Salmon biomass for year t at site j was calculated by multiplying annual salmon escapement estimates of site j by the average biomass of the corresponding salmon species i at spawning time Four salmon species are found at these study sites: Chum (Oncorhynchus keta), Pink (O. gorbusha), Coho (O. kisutch), and Sockeye (O. nerka); biomass estimates at spawning for Chum and Pink were taken from previous values at these sites (Hocking and Reynolds, 2011), and Coho and Sockeye were taken from an earlier study (Shaul et al., 2007). Chum and Pink accounted for the vast majority of salmon at the sites (>95% of total biomass). Annual biomass measures for each stream j were averaged over the 3 years prior to sampling (2014–2016) in order to be consistent with previous studies and because initial assessments showed this time horizon to be of higher predictive value than either averaging over the entire time series (16 years) or taking only the previous year’s abundance (2016). From these biomass estimates, density indices were calculated by dividing average biomass by the length of spawning reach of each watershed , and this metric is henceforth referred to as “salmon density.” Its units are thus .
2.6 Data analysis
Two interconnected data sets were analyzed in this study: a regional gradient of salmon density at 23 sites (“regional salmon gradient data”), and an above and below waterfalls comparison at four of these sites (“above/below waterfall data”). All statistical analyses were performed in R (R Core Team, 2019).
Given we had strong a-priori hypotheses and most of the response variables had linear responses, partial redundancy analysis (pRDA; Legendre and Legendre, 2012) was appropriate to test the relationships between explanatory variables and aggregate soil chemistry (Figure 1). This multivariate approach weighted all chemical response variables equally. For the gradient data analyses, all chemical variables were used (k = 20) and the ordination was constrained by salmon density (continuous), soil layer (2 levels: shallow soil and deeper soil) and the first axis of each vegetation PCA (continuous). For the above/below waterfall data, analysis was constrained by position relative to the waterfall (2 levels: above or below), salmon density of the stream below falls, soil layer, and the first axis of each vegetation PCA. To account for sampling structure, both site and soil core were “partialled out” as conditioning variables. Euclidian distances were used and all variables were scaled to zero with unit variance. p-values of each term represent the marginal effects of each term after all other effects are included in the model and calculated over 1,000 permutations within sampling structure constraints, and used pseudo-F statistics for comparison (Legendre et al., 2011). Non-significant response variables were then dropped in stepwise fashion using the R function ordistep, and only the reduced models are reported here (Supplementary Tables S5-1, S5-2). For all tests, the function rda was used as implemented in the R package vegan (Oksanen et al., 2022).
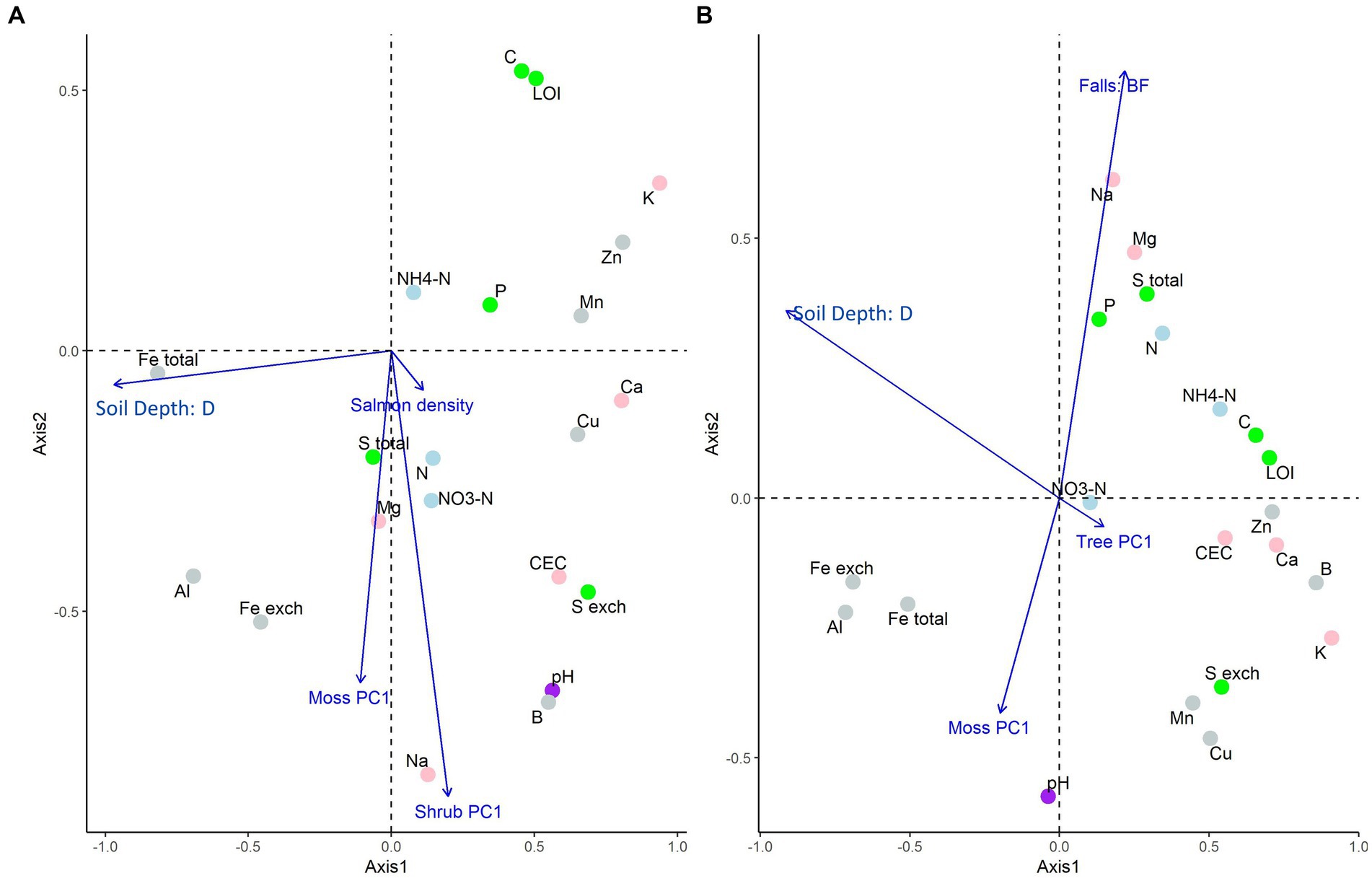
Figure 1. Partial redundancy analysis ordinations for aggregate soil chemistry of A. the “regional salmon density gradient” dataset and B. the “above and below waterfall” dataset, both on the Central Coast of British Columbia, Canada. In plot (A), significant constraining response variables were salmon density, soil depth, and the first vegetation PCA axis of moss and shrubs; in plot (B), significant constraining response variables were waterfall positionality, soil depth, and the first vegetation PCA axis of moss and trees (Supplementary Tables S4-1, S4-2). This ordination uses “type II” (or “species”) scaling, and so perpendicular distances from response-variable point to the explanatory variable arrow is proportional to the variance explained by that factor.
Second, a generalized linear mixed-effects model approach was used for hypothesis testing. For the regional gradient dataset, the full model equation was Response vaiablek ~ salmon density + soil type + moss PCA1 + herb PCA1 + shrub PCA1 + tree PCA1 with soil core within site modeled as random effects. A similar model was used for the above/below waterfall data, using the full model Response vaiablek ~ Fall position (above or below) + salmon density + soil type + Fall position: salmon density, again modeling soil core within site as random effects. We modeled salmon density and the interaction term because if salmon inputs are driving changes in soil chemistry, then these changes should be higher in watersheds with more salmon, and this difference should matter below waterfalls but not above waterfalls. In all models, explanatory variables were standardized to zero mean with unit variance before fitting. Both Gaussian distributions (identity- and log-link functions), and Gamma (log-link) distributions were tested, and the model with best fit selected for use. Model assumptions, fit, and multicollinearity among explanatory variables were visually assessed via diagnostic plots generated by the performance R package. The model that best fit assumptions was manually selected (Supplementary Table S6-1) and standardized coefficients were visualized using marginal effects curves and forest plots (Figures 2–5). To help with model fit in the regional gradient dataset, 38 outliers values were removed out of 1,080 measurements, representing values from eight soil cores (Supplementary Table S7-1); analysis was repeated with these outliers left in with no qualitative differences in results. ANOVA tables are presented in Supplementary material S8 (regional gradient analysis) and Supplementary material S9 (above and below waterfall analysis); in order to visualize nonlinear relationships loess curves are also presented for the raw regional data (Supplementary Figure S10-1).
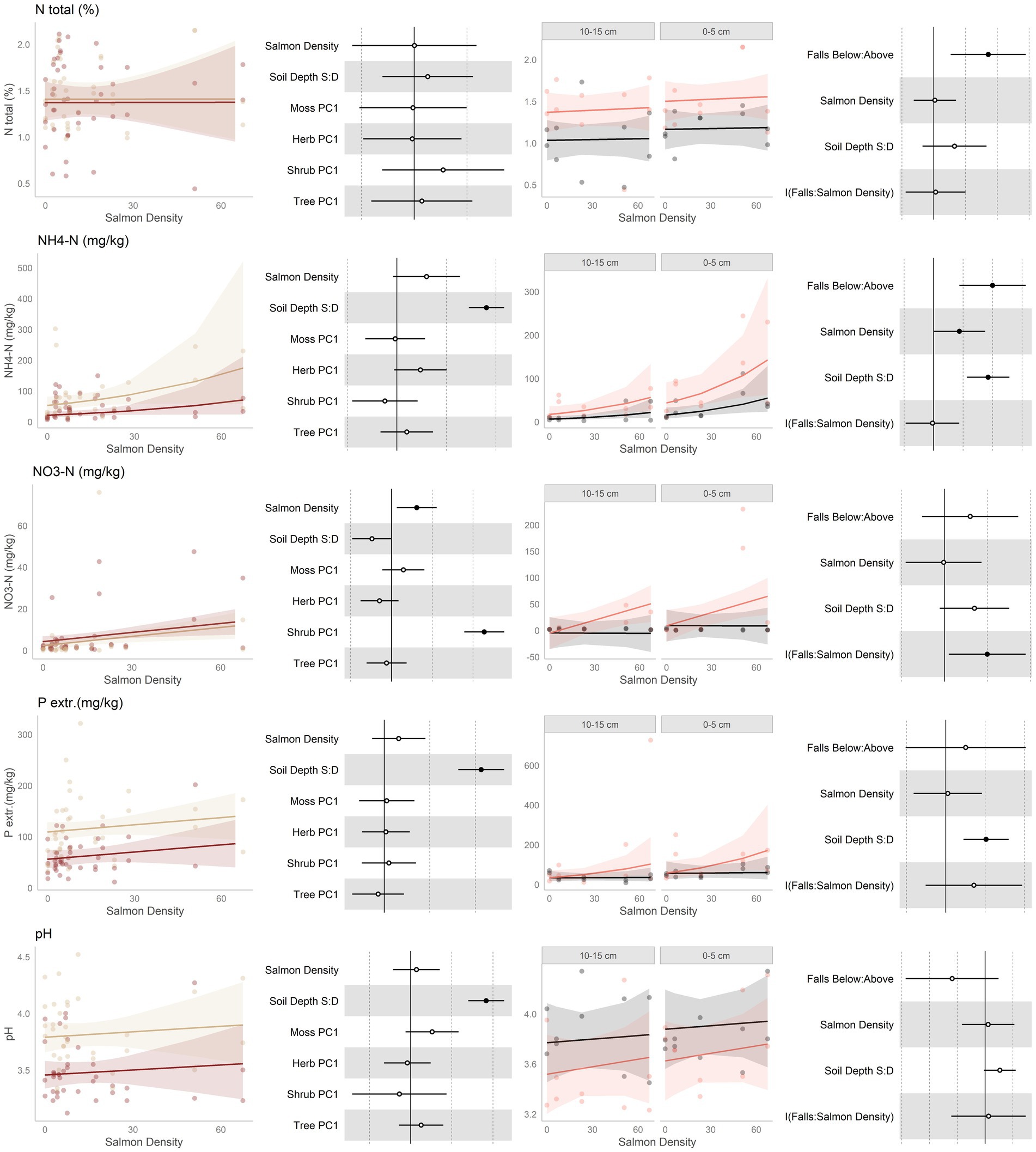
Figure 2. Data and modeling results for percent total nitrogen, ammonium, nitrate, extractible phosphorus, and pH (rows, top to bottom). Included is the “regional gradient” dataset (columns 1 and 2) and the “above/below” waterfall data (columns 3 and 4), from the Central Coast of British Columbia, Canada. Column 1 is a scatterplot of raw data of the response variable as a function of salmon density across regional salmon density gradient. Two lines of best fit are displayed, one for each soil depth: tan represents 0-5 cm (“shallow soil”), and brown 10–15 (“deeper soil”). The lines of best fit are marginal modeling results that keeps the values of all other response variables at their mean values; hence they should be viewed as an approximation of the coefficients displayed in column 2. Column 2 are forest plots displaying standardized coefficient values from generalized linear mixed models, with significant (p < 0.05) values indicated by filled dots and 95% confidence intervals displayed. Contrast for the soil depth column uses deeper soil as a reference. Column 3 displays above and below waterfall data, with the lines of best fit similarly representing marginal fits that keep the values of all other response variables at their mean values. “Above waterfalls” are represented as black lines, and “below waterfalls” as salmon-colored lines. Plot facets are soil depth (0–5 cm = shallow soil, 10–15 cm = deeper soil), displayed with deeper soil first since shallow soil is expected to show a stronger relationship with salmon addition and to follow the same left-to-right relationship with soil density established in the first column. Contrasts are shallow soil relative to deeper soil (S:D), and below waterfalls relative to above waterfalls.
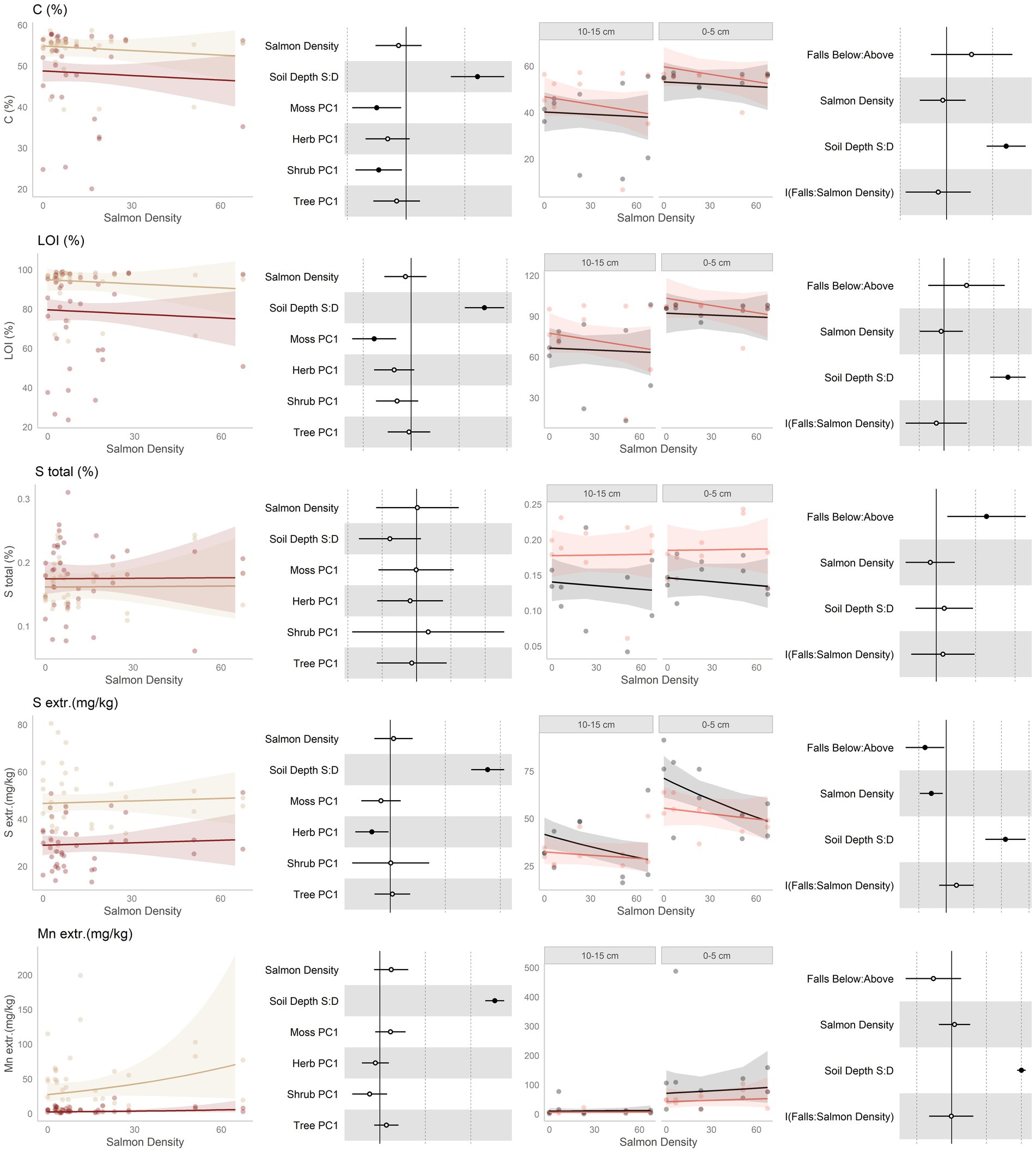
Figure 3. Data and modeling results for percent total carbon, loss-on-ignition (LOI), total sulfur (S), extractible sulfur (S), and extractable manganese (Mn; rows, top to bottom). Included is the “regional gradient” dataset (columns 1 and 2) and the “above/below” waterfall data (columns 3 and 4), from the Central Coast of British Columbia, Canada. Column 1 is a scatterplot of raw data of the response variable as a function of salmon density across regional salmon density gradient. Two lines of best fit are displayed, one for each soil depth: tan represents 0–5 cm (“shallow soil”), and brown 10–15 (“deeper soil”). The lines of best fit are marginal modeling results that keeps the values of all other response variables at their mean values; hence they should be viewed as an approximation of the coefficients displayed in column 2. Column 2 are forest plots displaying standardized coefficient values from generalized linear mixed models, with significant (p < 0.05) values indicated by filled dots and 95% confidence intervals displayed. Contrast for the soil depth column uses deeper soil as a reference. Column 3 displays above and below waterfall data, with the lines of best fit similarly representing marginal fits that keep the values of all other response variables at their mean values. “Above waterfalls” are represented as black lines, and “below waterfalls” as salmon-colored lines. Plot facets are soil depth (0–5 cm = shallow soil, 10–15 cm = deeper soil), displayed with deeper soil first since shallow soil is expected to show a stronger relationship with salmon addition and to follow the same left-to-right relationship with soil density established in the first column. Contrasts are shallow soil relative to deeper soil (S:D), and below waterfalls relative to above waterfalls.
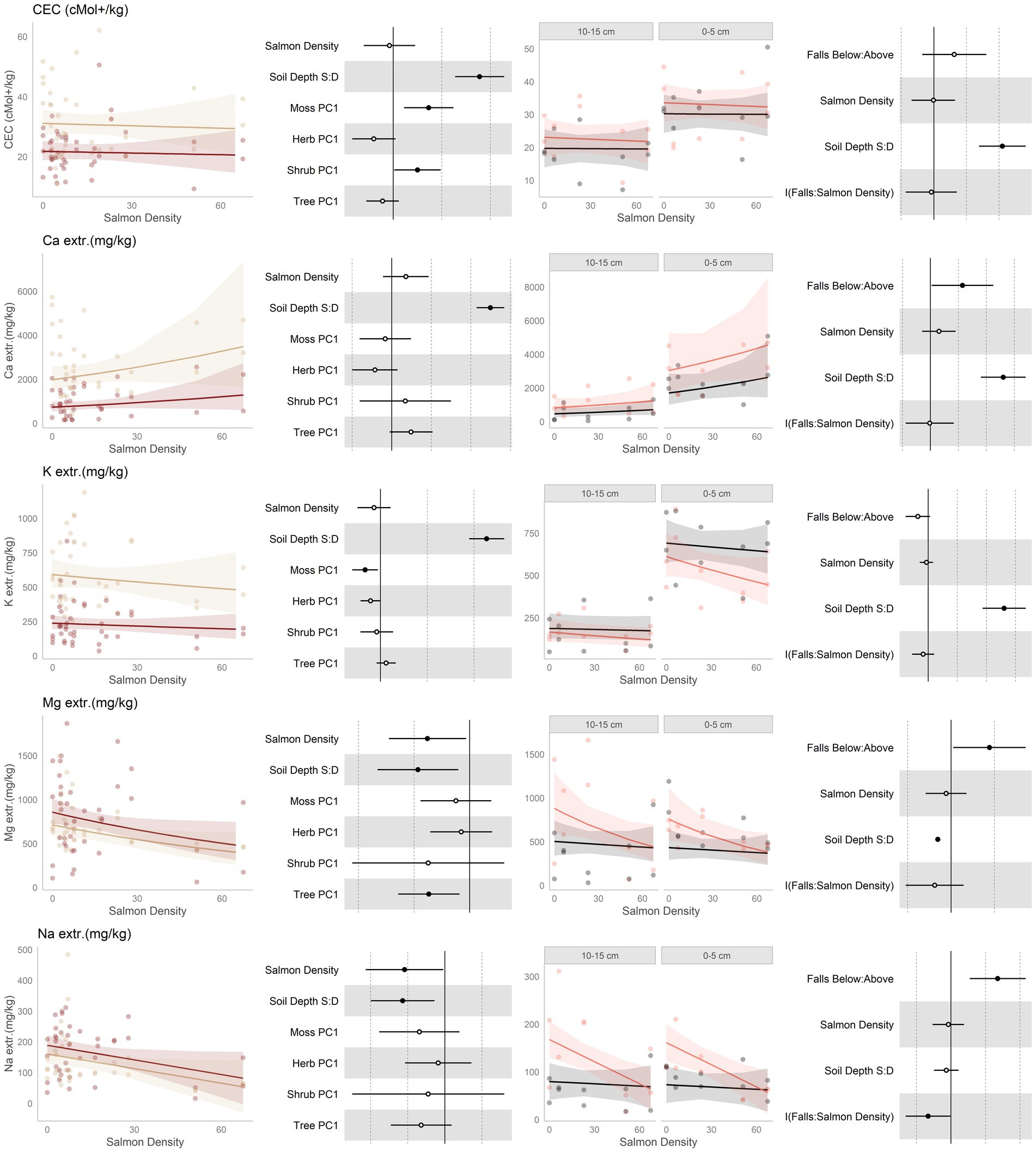
Figure 4. Data and modeling results for total cation exchange capacity (CEC), and extractible cations calcium (Ca2+), potassium (K+), magnesium (Mg2+), and sodium (Na2+; rows, top to bottom). Included is the “regional gradient” dataset (columns 1 and 2) and the “above/below” waterfall data (columns 3 and 4), from the Central Coast of British Columbia, Canada. Column 1 is a scatterplot of raw data of the response variable as a function of salmon density across regional salmon density gradient. Two lines of best fit are displayed, one for each soil depth: tan represents 0-5 cm (“shallow soil”), and brown 10–15 (“deeper soil”). The lines of best fit are marginal modeling results that keeps the values of all other response variables at their mean values; hence they should be viewed as an approximation of the coefficients displayed in column 2. Column 2 are forest plots displaying standardized coefficient values from generalized linear mixed models, with significant (p < 0.05) values indicated by filled dots and 95% confidence intervals displayed. Contrast for the soil depth column uses deeper soil as a reference. Column 3 displays above and below waterfall data, with the lines of best fit similarly representing marginal fits that keep the values of all other response variables at their mean values. “Above waterfalls” are represented as black lines, and “below waterfalls” as salmon-colored lines. Plot facets are soil depth (0–5 cm = shallow soil, 10–15 cm = deeper soil), displayed with deeper soil first since shallow soil is expected to show a stronger relationship with salmon addition and to follow the same left-to-right relationship with soil density established in the first column. Contrasts are shallow soil relative to deeper soil (S:D), and below waterfalls relative to above waterfalls.
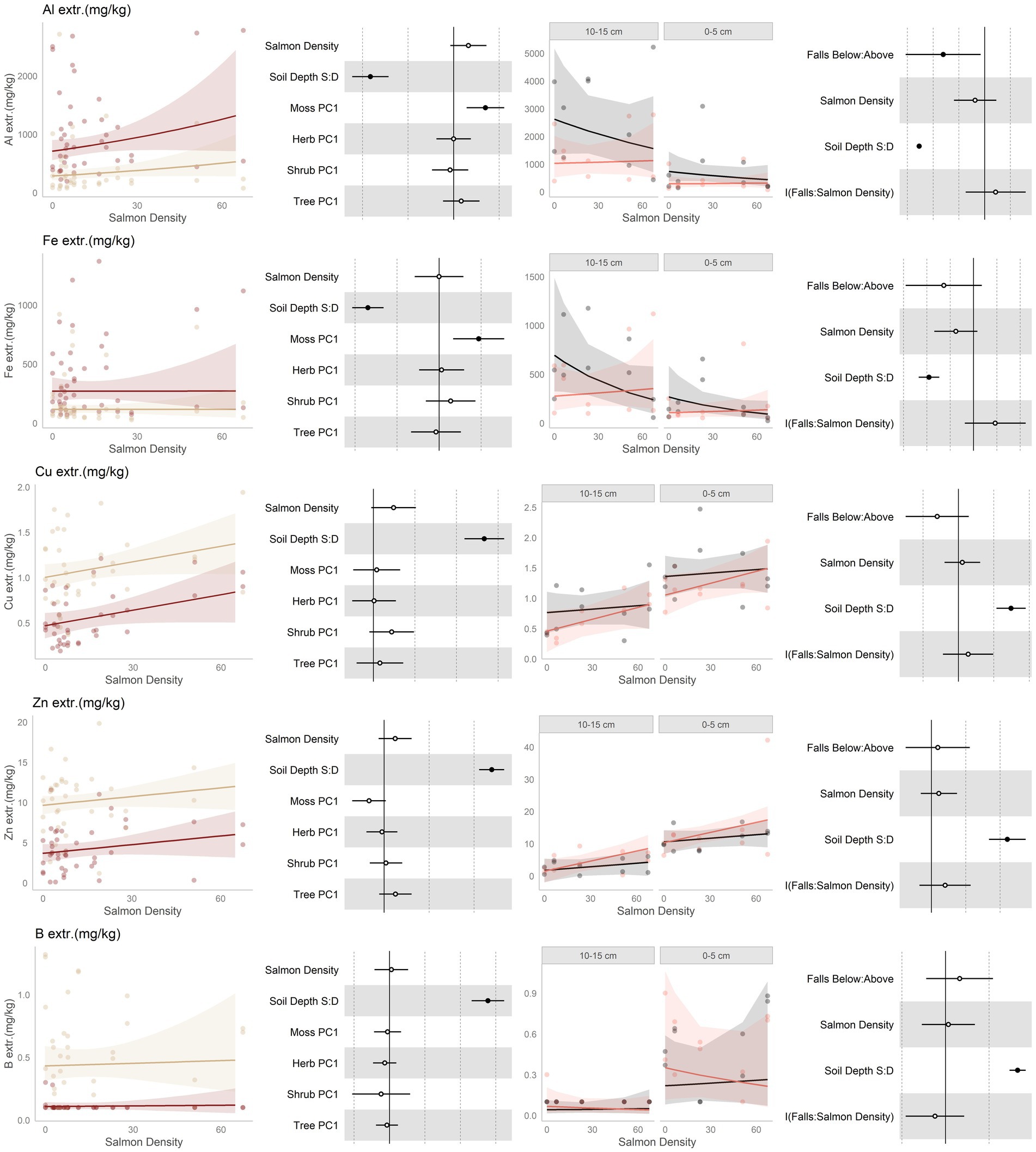
Figure 5. Data and modeling results for extractible aluminum (Al3+), extractible iron (Fe), extractible copper (Cu), extractible zinc (Zn), and extractible boron (B; rows, top to bottom). Included is the “regional gradient” dataset (columns 1 and 2) and the “above/below” waterfall data (columns 3 and 4), from the Central Coast of British Columbia, Canada. Column 1 is a scatterplot of raw data of the response variable as a function of salmon density across regional salmon density gradient. Two lines of best fit are displayed, one for each soil depth: tan represents 0–5 cm (“shallow soil”), and brown 10–15 (“deeper soil”). The lines of best fit are marginal modeling results that keeps the values of all other response variables at their mean values; hence they should be viewed as an approximation of the coefficients displayed in column 2. Column 2 are forest plots displaying standardized coefficient values from generalized linear mixed models, with significant (p < 0.05) values indicated by filled dots and 95% confidence intervals displayed. Contrast for the soil depth column uses deeper soil as a reference. Column 3 displays above and below waterfall data, with the lines of best fit similarly representing marginal fits that keep the values of all other response variables at their mean values. “Above waterfalls” are represented as black lines, and “below waterfalls” as salmon-colored lines. Plot facets are soil depth (0–5 cm = shallow soil, 10–15 cm = deeper soil), displayed with deeper soil first since shallow soil is expected to show a stronger relationship with salmon addition and to follow the same left-to-right relationship with soil density established in the first column. Contrasts are shallow soil relative to deeper soil (S:D), and below waterfalls relative to above waterfalls.
Next, an exploratory approach was used to examine a broader set of explanatory variables in the gradient dataset. A random forest model was built for each response variable using the caret and randomForest R packages (Liaw and Wiener, 2002; Kuhn, 2008). 19 explanatory variables were used, including: salmon density, distance of the sampling point from the stream, soil depth, percent cover of coarse woody debris in a 5 m radius (CWD), species of the nearest large tree (FT), and the first two PCA axes of mosses, herbs, shrubs, and trees. Standardized importance coefficients for each explanatory variable (columns) from each model (rows) were then visualized using a heatmap (Figure 6). These coefficients estimate the importance of the explanatory variables in predicting response variables, but cannot estimate the direction of effects.
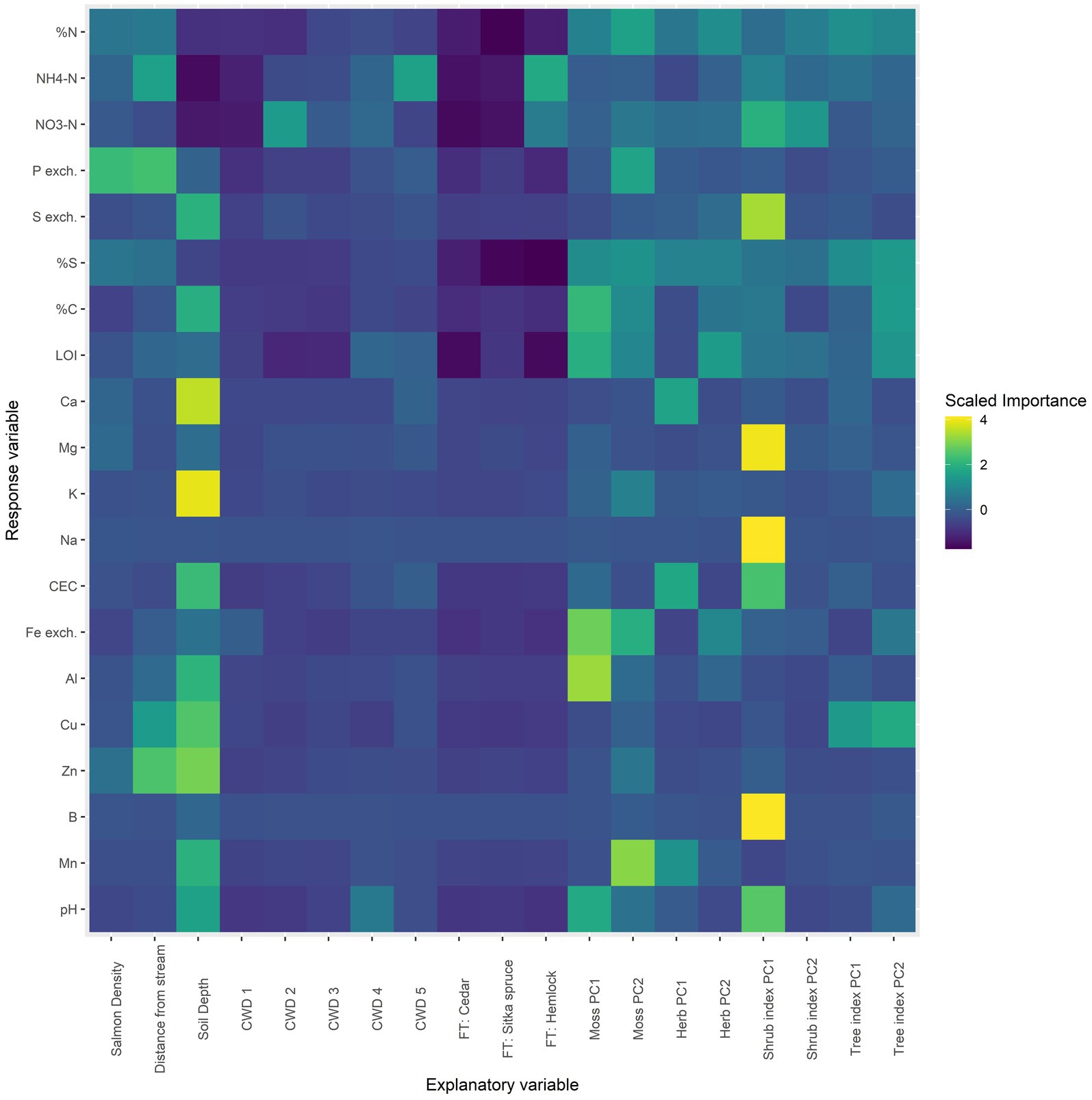
Figure 6. Random forest model for all chemical response variables as a function of an extended set of explanatory variables. For each row, yellow denotes a high importance attributed to that response variable and dark blue a low importance. “Salmon density” is the salmon density index of the site; “distance from stream” is the perpendicular distance of each soil core from the stream; “soil depth” is a categorical variable denoting soil depth with two levels: 0–5 cm (shallow) and 10–15 cm (deep).
3 Results
3.1 Aggregate soil chemistry
In the regional gradient data set (Figure 1A), salmon density, soil depth, and the first moss and shrub principal component (PC) axes were found to affect aggregate soil chemistry (p = 0.001, Supplementary Table S5-1). The first herb and tree PC axes were found to not be significant and hence were dropped from the model. 28% of the variance was accounted for by the reduced model, and variance inflation factors (VIFs) among explanatory variables was low (<1.5). In this ordination, salmon density had a smaller effect than the other explanatory variables (length of arrows in biplot), but was associated with nitrogenous substances, P and S (perpendicular distance of each point from arrows indicate the associated effect size on that response variable).
Similarly, in the above/below waterfall data (Figure 1B), position relative to the waterfall; soil depth; the first moss PC axis; and the first tree PC axis had significant effects on aggregate soil chemistry (p = 0.001; Supplementary Table S5-2). Salmon density, and the first herb and shrub PC axes were not significant and hence dropped from the model. 39% of the variance was accounted for by the reduced model and VIFs among explanatory variables was low (<1.3). In this ordination, plot location relative to falls had a large effect relative to the other explanatory variables, and was related to sodium, magnesium, sulfur, phosphorus, nitrogenous substances, and pH.
3.2 Individual GLMs
For this discussion, we refer to effects with p-values less than 0.05 simply as “changes,” effects with p-values between 0.1 and 0.05 as “trends,” and changes with p-values that are greater than 0.1 as “tendencies.” While we make a large number of comparisons, we also have strong a-priori predictions and multiple experimental designs; to aid interpretation, we present confidence intervals alongside raw data (Amrhein et al., 2019; Halsey, 2019).
3.2.1 Nitrogenous compounds
Nitrogenous substances were predicted to increase with salmon inputs (Figure 2, rows 1–3). Along the regional gradient of salmon density, total N did not change, ammonium tended to increase, and nitrate increased. Similarly, in the above/below waterfall comparisons, total N was higher below than above waterfalls, although this was independent of the magnitude of salmon density below falls. Ammonium was higher below than above waterfalls, and this was higher when there was more salmon in the watershed. Finally, nitrate was higher above falls when salmon were present, but there was no difference when salmon were not. In general, there was no change in total N or nitrate with soil depth, but ammonium tended to be higher in shallower soil.
3.2.2 Phosphorus and pH
It was predicted that extractible phosphorus would increase with salmon inputs (Figure 2, rows 4). P had an increasing tendency with salmon inputs along the regional gradient, but was not higher below than above waterfalls. There was, however, a positive tendency with salmon density below waterfalls but not above waterfalls. Phosphorus tended to be higher in shallow soil than deeper soil.
It was predicted that an increase in pH (less acidic) would be associated with higher soil fertility. pH showed no consistent response to salmon inputs along the regional gradient (Figure 2, rows 5). There was, however, a tendency for pH to be lower below waterfalls than above waterfalls. In both datasets, shallower soil had higher pH than deeper soils.
3.2.3 Carbon, sulfur, and manganese
No predictions were made for changes in soil carbon due to salmon inputs, aside from the expectation that higher soil carbon tends to be associated with higher soil fertility. Soil carbon, as measured by both %C and loss-on-ignition (LOI), was neither affected by salmon density nor by position relative to waterfalls (Figure 3, rows 1 and 2). Carbon content was both higher and less variable in shallower soils than deeper soil, and was impacted by both the moss and shrub communities.
Sulfur (Figure 3, rows 3 and 4) was predicted to increase with salmon inputs due to direct deposition from carcasses. Both total and extractable sulfur showed no trend along the regional salmon density gradient. The above and below waterfall comparison is more complex: total sulfur was found to be higher below waterfalls, but this was unrelated to salmon density. Extractible sulfur showed the opposite trend, and was lower below than above waterfalls, and in addition was affected negatively by salmon density. This density effect to be driven by above-falls sites where there were no salmon, and declines in extractible sulfur were lower in below-falls sites. In both datasets, total S was unaffected by soil depth while extractible sulfur was higher in shallow soil than deeper soil.
Due to being present in salmon carcasses, manganese (Figure 3, row 5) was predicted to increase with salmon inputs. This element had an increasing trend with salmon density across the regional salmon density gradient, and this was driven mostly by changes in shallow soils. However, it had a tendency to be lower below waterfalls than above waterfalls. It was higher in shallow soil than deeper soil.
3.2.4 Exchangeable cations
Cation exchange capacity (CEC; Figure 4, row 1) was predicted to increase with salmon inputs. It showed, however, no change along either the salmon density gradient and only a small tendency to be higher below falls. CEC was found to be higher in shallow soil than deeper soils, and in the regional dataset was shown to be affected by the moss and shrub communities.
The preponderance (about half) of the extractible cations measured was calcium, which was predicted to increase with salmon inputs due to direct deposition from Ca in salmon bones and cartilage. Indeed, calcium had an increasing tendency with salmon inputs along the regional gradient, and was higher below than above waterfalls (Figure 4, row 2). In both cases, calcium was higher in shallow than deeper soil. Interestingly, in shallow soil a U-shaped response was seen with respect to salmon density where Ca declined with salmon density at low to medium densities, and increased at higher input levels (Supplementary Figure S10-1). Calcium also tended to be affected with the tree community, and had a tendency to be affected by the herb community.
Similar to calcium, potassium (Figure 4, row 3) was predicted to increase with salmon inputs. Contrary to this expectation, potassium had a declining tendency with salmon inputs along the regional gradient, and tended to be lower below than above waterfalls. There was also some tendency for more negative slopes with respect to salmon density below than above waterfalls, particularly in shallow soil. Potassium was higher in shallow than deeper soil, and in the regional data it was affected by the moss community. Magnesium (Figure 4, row 4) was predicted to increase with salmon inputs. However, magnesium decreased along the regional salmon density gradient; and while higher below than above waterfalls, it had a tendency to decrease with salmon density below waterfalls but showed no change above. Since sodium is not an appreciable part of salmon carcasses, we made no strong predictions regarding its correlation with salmon inputs. Sodium (Figure 4, row 5) was similar to magnesium and decreased with increasing salmon inputs along the regional density gradient and, while it was higher overall below than above waterfalls, it had a negative slope associated with salmon density below falls but a constant slope above.
3.2.5 Metals
High levels of exchangeable aluminum are toxic to plants, and so consistent with our hypothesis of increased fertility Al was predicted to be lower in more salmon-amended soils. Our findings for aluminum are mixed; it had a tendency to increase with salmon density along the regional gradient, but was lower below than above waterfalls (Figure 5, row 1). Among the waterfall sites, there was a tendency for Al to be negatively correlated with salmon density; however, this was driven mainly by the above-falls samples where there were no salmon. Finally, Aluminum was consistently lower in shallower soil, and was affected by the moss community.
Since iron has similar soil chemistry to aluminum, it was predicted to act similarly to salmon inputs. Unlike aluminum, however, iron is present in salmon carcasses so was additionally predicted to increase due to direct deposition. Exchangeable iron did not change in response to salmon inputs along the regional gradient (Figure 5, row 2), but our waterfall data are more complex: it tended to be higher above than below waterfalls over most of the salmon density range, but due to having a negative slope above falls this relationship flips at high levels of salmon input.
No predictions were made for copper, but it was found to have a positive trend with salmon inputs along the regional gradient (Figure 5, row 3). However, while it had a tendency to be lower below than above waterfalls, it had tendency to increase with watershed salmon density below, but not above, waterfalls. Copper was higher in shallow than deeper soil. Zinc was predicted to be associated with salmon density due to direct deposition from carcasses. It had a positive tendency with salmon inputs along the regional gradient (Figure 5, row 4), and a positive tendency with salmon inputs below more than above waterfalls. Zinc was higher in shallow than deeper soil, and tended to be affected by the moss and tree communities. Finally, no predictions were made for boron (Figure 5, row 5), and boron did not respond to salmon inputs or position relative to waterfalls. It was higher in shallower than deeper soils.
3.3 Exploratory analysis
Random forest models are useful for providing a broad overview of associations between response and explanatory variables, however, they do not allow for interpretation of the direction of effects but only their “importance” as a classifier in the regression tree (Cutler et al., 2007; Boulesteix et al., 2012). In this context, the most important factors overall were soil depth, the two axes of the moss PCA, and the first axis of the shrub PCA (Figure 6). Focusing on salmon density, it was of highest importance in phosphorus, the nitrogen compounds, total sulfur, calcium, and magnesium—all elements found in salmon carcasses. In addition, distance from the stream was associated with these elements as well as with zinc and copper. These results corroborate the multivariate results that soil depth is the most important factor associated with soil chemistry, followed by the moss and shrub communities, and then salmon density and distance from the stream.
4 Discussion
The first goal of this study was to assess the long-term effects of salmon inputs on soil chemistry. In order to assess this, we employed two experimental designs: first, a between-watershed comparison along a regional gradient of salmon density, and second, a within-watershed comparison at sites below and above waterfalls that block salmon migration. There are advantages and disadvantages to each approach. The regional approach is spatially broad but may confound effects from salmon inputs with watershed-level effects that correlate with salmon density (for example, larger streams have more salmon but also gather water and nutrients from larger watersheds), or with spatial correlations across the landscape (here we treated all watersheds as independent). In addition, since this was a natural experiment and we were confined to using streams with long-term salmon counts, our final dataset had many “low salmon density” sites, but only two “very high” density sites. This left a gap in salmon density between 30 and 60 kg/m of stream per year, which results in high leverage attributed to high-density sites in the models used here. On the other hand, the above- and below-waterfall design experimentally controls for these regional and watershed-level effects since comparisons are made within watersheds. However, this methodology confounds salmon inputs with topographical, geological, and edaphic factors that coincide with waterfall presence. Given these caveats, in the discussion below we ascribe highest confidence to findings where the two methodologies agree.
From a multivariate perspective, it was found that salmon inputs along a gradient of salmon density were correlated to changes in aggregate soil chemical profiles (Figure 1A; Supplementary Table S5-1), and that sites below waterfalls were different than sites above waterfalls (Figure 1B; Supplementary Table S5-2). Taken together, these findings are consistent with salmon inputs changing the aggregate soil chemistry at these sites.
It was further predicted that these changes would be consistent with increased site soil fertility, and that salmon inputs would result in increased nitrogen loading. This was confirmed for ammonium-N and nitrate-N, which both increased with salmon density along the regional gradient and were higher below than above waterfalls. Percent soil nitrogen was also higher below than above waterfalls, however this was not sensitive to the density of salmon. This may imply that higher below-waterfall total N is the result of edaphic factors other than salmon inputs, such as being flatter and lower nutrient-receiving sites. Interpretation of total %N is complicated by the fact that the data previous findings on these streams (Hocking and Reynolds, 2011) demonstrate a shift in the plant community to more nitrophyllic assemblages with increased salmon inputs. Together with evidence for greater plant production and biomass associated with salmon (Sayer, 2008; Quinn et al., 2018; Siemens et al., 2020), it is probable that deposited nitrogen accumulates in the above-ground rather than below-ground compartments, and thus %N in the soil does not change; to resolve these questions a more comprehensive approach to measuring whole-ecosystem N stocks is needed. Given that salmon-derived nitrogen is primarily delivered in soluble ammonium and nitrate form (Drake et al., 2005), the stronger response in these chemicals over %N is consistent with expectations from salmon inputs. Assuming that nitrogen is a limiting nutrient in this system (LeBauer and Treseder, 2008), these results are consistent with our expectation that there is an increase in soil fertility with salmon input.
Phosphorus by mass is the second most important plant nutrient next to nitrogen (Strawn et al., 2020) and is important in plant enzymatic, nucleic acid, and photosynthetic health (Maathuis, 2009). Phosphorus was predicted to increase with salmon inputs, as P has been positively associated with salmon inputs in previous studies (Drake et al., 2005; Hood et al., 2019; D’Amore et al., 2020). Approximately half the phosphorus in salmon carcasses is found in the skeleton, and half in soft tissue (Drake et al., 2005). Hence it could be expected to enter the soil in both inorganic (skeletal) and organic (soft tissue) forms, and this has been found in the water column (Hood et al., 2019). We found only limited evidence for exchangeable-P increasing with salmon inputs. This lack of strong evidence may be because phosphorus is less mobile in the soil profile than nitrogen, and is thus more spatially variable than other elements given the “patchy” underlying distribution of salmon carcasses. Alternatively, this lack of response may also be because inorganic extractible phosphorus, as measured here, may not be the most relevant phosphorus fraction to measure in these soils where organic-P is likely the dominant form of phosphorus due to their extremely low pH (Turner and Blackwell, 2013; Penn and Camberato, 2019). With regards to soil fertility, organic-P amendments are more effective in increasing plant-available P in acidic soil environments than inorganic-P amendments (Chen et al., 2018), and organic-P may be the main driver of tree fertility elsewhere in Pacific Northwest forests (Collins et al., 2015; Kranabetter et al., 2020). Future studies focusing on phosphorus and salmon inputs should measure a more comprehensive suite of phosphorus forms in order to resolve these questions.
On a large geographic scale, pH is perhaps the most important single soil character for determining soil fertility (Strawn et al., 2020). Moderate pH around 7 is associated with more fertile soils, with extremes of acidity or alkalinity leading to nutrient assimilation problems and/or plant toxicity. Soils undergoing high levels of nitrogen fertilization can become acidified through the release of hydrogen ions from the oxidation of ammonium (NH4+) to nitrate (NO3−; Gao et al., 2015; Tian and Niu, 2015). This nitrogen-driven acidification can reduce soil fertility, as low pH (in the range found here) is associated with aluminum toxicity in agricultural soils (Fenn et al., 2006; Zhao and Shen, 2018), and decreased stocks of exchangeable cations such as calcium due to nitrate-assisted co-leaching in regions of high rainfall such as the Central Coast (Perakis et al., 2013; Brady and Weil, 2016). In agricultural and forest settings, these negative effects can be caused by nitrogen deposition at similar or lower deposition rates than those estimated for MDN inputs (Tian and Niu, 2015). Here we found no change in pH attributable to increased salmon inputs along the regional salmon density gradient, and the tendency for pH to be lower below falls was found to be independent of the degree of salmon presence in the watershed. The lack of soil acidification despite high levels of N input may be because salmon nitrogen arrives in a diversity of organic forms, such as the proteins and amino acids found in salmon carcasses, which are more complex than inorganic additions and less likely to induce soil acidification (Chen and Xu, 2008). For this reason, the addition of nitrogen from salmon carcasses proper may be qualitatively different than the addition of inorganic nitrogen in forest fertilization strategies, or in “carcass analog” that have been used in some systems to offset salmon declines in the Pacific Northwest (Pearsons et al., 2007; Kohler et al., 2012). Inorganic N-amendment analogu may thus be poor stand-ins for salmon carcasses, at least with regards to forest soil, and investigations into compensatory approaches should consider the use of organic-N amendments, such as fish silage (Prescott, 1997), instead of inorganic additions.
High organic matter content in agricultural soils is thought to be closely related to soil fertility, and is important in influencing almost all other soil processes (Dhaliwal et al., 2019). Soil carbon is of considerable importance in climate change mitigation strategies, and understanding factors affecting soil carbon sequestration can maximize value emerging from carbon credit projects. In the short term, nitrogen fertilization is thought to increase ecosystem metabolism and reduce soil C (Gao et al., 2014), but in the medium to long term the opposite occurs and N addition is associated with increased soil C (Zang et al., 2016; Huang et al., 2020); this response however is complex and depends on context (Allison et al., 2009). Total %C and loss-on-ignition (LOI) in this study was largely unaffected by salmon inputs. We found some evidence for a higher %C below falls, but this had a decreasing, not increasing, tendency with salmon inputs. Given this, the most likely interpretation is that salmon inputs either have no effect or a small negative effect on percent soil carbon. Similar to nitrogen however, carbon stocks are influenced by aboveground biomass quality and quantity, and so total ecosystem carbon stocks and not simply soil %C should be measured in a comprehensive assessment to clarify carbon processes. Given higher aboveground biomass associated with salmon inputs shown in other studies, it is probable that despite our finding of stable soil %C there are nevertheless ecosystem-level carbon sequestration benefits arising from salmon inputs. This is consistent with the high %C found here relative to regional soils (Carpenter et al., 2014), which underscores the importance of these riparian rainforests as high-priority carbon sinks in a global context (Post et al., 1985; Buotte et al., 2020).
Sulfur is another essential macronutrient for plant function, and is necessary for protein synthesis and important in the production of phytochelators which detoxify metals and metalloids (Maathuis, 2009), activities that are particularly important in buffering plant function in acidic soil (Zhao et al., 2014, p. 201). Our findings on the correlation of salmon inputs and sulfur were mixed: %S and extractible sulfur were not correlated with salmon density across the regional gradient; and %S was found to be higher but extractible sulfur lower below waterfalls. This lack of consistency in our results is surprising since sulfur is directly deposited by salmon carcasses (Drake et al., 2005). Similar to phosphorus, this sulfur is primarily of organic nature, which is less mobile than inorganic sulfates (Wilhelm Scherer, 2009), and thus less likely to be displaced in the soil profile or leached from the system. Regardless, given that sulfate is the stable oxidation state and reservoir of exchangeable S in aerobic soils, and both retention and plant availability of sulfate anions is generally high in acidic soil environments (Strawn et al., 2020), sulfur deficiency is unlikely at these sites and any possible inputs are thus of limited importance to site fertility.
Manganese is involved in redox biochemistry in both soil and plants, particularly in the oxidative decomposition of carbon by Mn-peroxidase, and temperate rainforest fungal communities and soil C-cycling are sensitive to manganese amendments (Kranabetter, 2019; Kranabetter et al., 2021). Soil extractible manganese levels found here are largely unaffected by salmon inputs. Despite this, manganese is a component of salmon carcasses, and manganese in these samples is indeed related to changes in the fungal saprophytic community (Larocque, 2022). It is thus possible that manganese from salmon inputs is simply be taken up by the plant and fungal community and not present in the soil profile.
Cation exchange capacity (CEC) is a measure of the number of exchangeable cations that a soil can adsorb, and hence is a measure of the soil’s net capacity to retain positively charged particles strongly enough to prevent leaching, but loosely enough to be available for plants. Since many cations are important for biological functioning (NH4+, Ca2+, Mg2+, K+), high CEC is generally associated with soil fertility. While CEC overall did not change with increased salmon inputs, loess plots indicate compensatory responses between soil layers (Supplementary Figure S10-1), pointing to increased eluviation from shallow to deeper soil layers at low to medium levels of salmon input which is consistent with nitrogen-associated co-leaching. At very high levels of salmon input this relationship reverses, which is consistent with accumulation via direct deposition from carcasses. Since CEC is an aggregate measure, the apparent stability of CEC may disguise underlying dynamics in the individual cation species (Ca2+, K+, Mg2+, and Na+).
Calcium is important in maintaining plant structural integrity and cellular messaging, and has been suggested to be a limiting nutrient in coastal systems (Trant et al., 2016), in particular for western redcedar and associated plants. Ca2+ is intimately associated with N cycling (Perakis et al., 2006; Hynicka et al., 2016) and generally shows an inverse relationship with NO3− addition, with co-leaching of NO3− anions and Ca2+ cations the most commonly suggested mechanism (Strawn et al., 2020). Calcium had an increasing tendency along the regional salmon density gradient, and was higher below than above waterfalls. The unimodal response in shallow soil loess curves (Supplementary Figure S10-1) is consistent with the occurrence of two simultaneous mechanisms—increased nitrogen-associated leaching, which dominates shallow soil at low to medium salmon densities, and direct deposition of Ca from salmon bones and cartilage, which dominates at high levels of salmon input.
Potassium is important in maintaining plant turgor pressure and osmotic potential, cellular signaling, and the activation of numerous enzymes (Maathuis, 2009), and is considered the third most important macronutrient in plants after nitrogen and phosphorus (Pandey et al., 2020). Magnesium, meanwhile, has a central role in the chlorophyll molecule, and is also important as a enzymatic cofactor in both transcription and translation (Maathuis, 2009). Sodium has a variety of roles in plant metabolism, including acting to maintain turgor pressure and as a transport cation (Subbarao et al., 2003). Here the preponderance of evidence is that potassium, magnesium, and sodium declined with salmon inputs. This is consistent with the model of simultaneous leaching and deposition suggested above, since salmon carcasses deposit potassium and magnesium (K+, Mg2+), but do so in lower concentrations than calcium (Drake et al., 2005). As a group, K+, Mg2+, and Na2+ tended to decrease with salmon inputs, while Ca2+ overall increased; this is consistent with nitrogen-assisted cation leaching in these soils, with the caveat that calcium losses are replaced via carcass deposition. This process is also consistent with the relatively steady CEC observations in this and other studies, and may thus represent a long-term “calcium pump” where potassium, magnesium, and other cations are slowly displaced by calcium in the soil profile over time. This could have mixed effects on site fertility depending on context, but here is likely positively correlated with fertility since high calcium is associated with greater tree biomass in nearby areas due to anthropological soil amendments from shellfish harvesting (Trant et al., 2016; Cox et al., 2020), particularly large old-growth redcedar trees (D’Amore et al., 2009).
Turning to the metals, higher aluminum and iron concentrations are associated with poor soil fertility. Extractible aluminum had a positive trend along the regional gradient, but this was driven mainly by extreme values in the high-density sites. It was lower below than above waterfalls, and we found some evidence of a declining trend with salmon density differences above, but not below, waterfalls. Iron showed no relationship with salmon density across the regional gradient, and similar relationships as aluminum above and below waterfalls (Figure 5, row 2). Given full consideration, this is poor evidence for a strong relationship between aluminum and iron and salmon inputs, and it is unlikely that any fertility differences due to salmon inputs are associated with these metals.
Zinc plays several roles in plant physiology, including phospholipid structure, photosynthesis, protein synthesis, and tolerance to drought and disease (Noulas et al., 2018). Zinc had an increasing tendency across the regional salmon density gradient, and had a tendency to be higher below than above waterfalls when salmon were present but not when they were absent. Copper behaved similarly in response to salmon inputs, and also has a number of physiological roles in plants, including as a cofactor in protein synthesis and transportation pathways (Yruela, 2005), and has also been shown to be a predictor of soil microbiome community structure in maize soils (Peng et al., 2022) and in the samples examined here (Larocque, 2022). Copper is stabilized by soil organic matter (Dhaliwal et al., 2019), and has been shown in agroforestry systems to accumulate in response to organic fertilizer application (Roussos et al., 2017) such as fish. Boron, meanwhile, showed no response to salmon inputs (Figure 5, row 5). Taken together, these findings demonstrate that salmon have impacts on soil micronutrient as well as macronutrient concentrations, and this should be considered in future literature.
The primary goal of this study was to measure the effect of salmon inputs on soil chemistry. We hypothesized that salmon would increase soil fertility, and thus predicted that salmon would affect the suite of measured soil chemical properties to a more “fertile state.” These predictions were largely corroborated, with salmon increasing nitrate and ammonium, but not total soil %N; limited evidence for increases in exchangeable phosphorus; and no strong evidence for changes in pH. In addition, there was no strong relationship between Al and Fe and salmon inputs; no changes carbon; and some increases in micronutrient concentrations. We also found CEC to remain stable in response to salmon inputs, but found there were changes in underlying soil cation balance as calcium increased and other ions decreased with salmon inputs. In general, this increase in fertility-associated metrics with salmon density found here are compatible with fish silage fertilization experiments in cedar-hemlock forests from Northern Vancouver Island (Weetman and Prescott, 2000; Blevins et al., 2006), where additions of fish silage resulted in positive growth responses in western redcedar, western hemlock and Amabilis fir; these responses were similar or superior to inorganic fertilizer applications (Prescott, 1997). They are also consistent with findings that show increased plant and fruit growth in response to salmon inputs (Sayer, 2008; Quinn et al., 2018; Siemens et al., 2020). Taken together, this supports the hypothesis of salmon inputs increasing the fertility at these sites.
Overall, it is likely that the organic amendments brought by salmon are processed differently than inorganic amendments in forest soils (Chen et al., 2018), and so agricultural models of soil health may be inappropriate in acidic and organic-rich forest soils such as those found in temperate rainforests. Our understanding of these processes may be enhanced by improved general understanding of how acidic organic soils work, better understanding of microbiome nutrient transformations, and by directly measuring deposition and uptake of salmon inputs, including amino acids (Metcalfe et al., 2011), organic phosphates, and lipids (Dinel et al., 1990). Effects of salmon fertilization will be mediated by local edaphic conditions and soil communities, and different soils and soil ecologies may respond differently to these inputs (D’Amore et al., 2011). This may account for differences and resolve controversy found between studies that span a large geographic and edaphic range.
This study was conducted in the late summer in order to be as distant in the annual round from the salmon run as possible in order to focus on the medium- and long-term legacy effects of salmon inputs. A tradeoff of this approach is the potential influence of seasonal effects, particularly late-summer drought, to confound these results. At the time of sampling, many of the more soluble nutrients deposited by salmon may already have been taken up by plants or leached from the system. These findings should thus be regarded as a lower bound for “salmon effects” on soil conditions, and greater impacts should be expected to be found in times more proximate to the salmon run. Similarly, it is likely that the ephemeral nutrient hotspots directly under carcasses represent different processes than those measured here, and this should be investigated using an appropriate experimental design.
5 Conclusion
Overall, this study represents the most comprehensive investigation of the effect of salmon inputs on riparian soil to date. It adds a suite of soil characteristics to the growing list of “salmon-sensitive” ecological features (Walsh et al., 2020), supports the hypothesis that salmon inputs improve the fertility of recipient sites, and may help explain mechanisms behind changes in plant community composition (Hocking and Reynolds, 2011) and physiology (van den Top et al., 2018; Siemens et al., 2020). These findings provide further evidence for increased nitrate and ammonium inputs from salmon and are consistent with an interpretation of fertility gain at these sites.
Data availability statement
The datasets presented in this study can be found in online repositories. The names of the repository/repositories and accession number(s) can be found at: https://github.com/AllenLarocque/Salmon_Chemistry_Frontiers_2023.
Author contributions
This study was conceptualized, designed, implemented, analyzed, and written by AL. SS helped throughout production, particularly with study conceptualization, funding, and manuscript preparation. All authors contributed to the article and approved the submitted version.
Funding
This work was funded by MITACS Accelerate Internship IT04265, in partnership with Brinkman Associates Reforestation; Donner Canadian Foundation, Grant No. E-47-19; NSERC Strategic Project Grant to SS; and NSERC Discovery Grant to SS.
Acknowledgments
This manuscript is a modified analysis and presentation of the second chapter of AL’s PhD dissertation (Larocque, 2022). Special thanks to John Reynolds and the Hieltsuk Integrated Resource Management Department who have provided salmon time series data. John Reynolds also provided important scientific and editorial comments. Thanks to Bianca Eskelson for advice on statistical analysis, and Rob Guy and Les Lavkulich for input on data interpretation. Field work was facilitated by Allison Dennert, Howard Humchitt and Walter Campbell. Special thanks to Gabriel Orrego, Huamani Orrego, Robyn Simard, Jessica Walsh, Lindsey Ogston and Ernest Wu for field assistance. Thanks to Shannon Guichon, Zac de Moor, and Joey Timmermans for laboratory assistance. Most importantly, thanks to the Heiltsuk Nation for being gracious hosts and for permission to pursue this work in their territory.
Conflict of interest
The authors declare that the research was conducted in the absence of any commercial or financial relationships that could be construed as a potential conflict of interest.
Publisher’s note
All claims expressed in this article are solely those of the authors and do not necessarily represent those of their affiliated organizations, or those of the publisher, the editors and the reviewers. Any product that may be evaluated in this article, or claim that may be made by its manufacturer, is not guaranteed or endorsed by the publisher.
Supplementary material
The Supplementary material for this article can be found online at: https://www.frontiersin.org/articles/10.3389/ffgc.2023.1010294/full#supplementary-material
References
Allison, S. D., LeBauer, D. S., Ofrecio, M. R., Reyes, R., Ta, A.-M., and Tran, T. M. (2009). Low levels of nitrogen addition stimulate decomposition by boreal forest fungi. Soil Biol. Biochem. 41, 293–302. doi: 10.1016/j.soilbio.2008.10.032
Amrhein, V., Trafimow, D., and Greenland, S. (2019). Inferential statistics as descriptive statistics: there is no replication crisis if we Don’t expect replication. Am. Stat. 73, 262–270. doi: 10.1080/00031305.2018.1543137
Bartz, K. K., and Naiman, R. J. (2005). Effects of salmon-borne nutrients on riparian soils and vegetation in Southwest Alaska. Ecosystems 8, 529–545. doi: 10.1007/s10021-005-0064-z
Ben-David, M., Hanley, T. A., Klein, D. R., and Schell, D. M. (1997). Seasonal changes in diets of coastal and riverine mink: the role of spawning Pacific salmon. Can. J. Zool. 75, 803–811. doi: 10.1139/z97-102
Blevins, L. L., Prescott, C. E., and Van Niejenhuis, A. (2006). The roles of nitrogen and phosphorus in increasing productivity of western hemlock and western redcedar plantations on northern Vancouver Island. For. Ecol. Manage. 234, 116–122. doi: 10.1016/j.foreco.2006.06.029
Boulesteix, A.-L., Janitza, S., Kruppa, J., and König, I. R. (2012). Overview of random forest methodology and practical guidance with emphasis on computational biology and bioinformatics. WIREs Data Mining Knowl Discov 2, 493–507. doi: 10.1002/widm.1072
Brady, N. C., and Weil, R. R. (2016). The nature and properties of soils. Upper Saddle River, NJ, USA: Pearson.
Buotte, P. C., Law, B. E., Ripple, W. J., and Berner, L. T. (2020). Carbon sequestration and biodiversity co-benefits of preserving forests in the western United States. Ecol. Appl. 30:e02039. doi: 10.1002/eap.2039
Carpenter, D. N., Bockheim, J. G., and Reich, P. F. (2014). Soils of temperate rainforests of the north American Pacific coast. Geoderma 230-231, 250–264. doi: 10.1016/j.geoderma.2014.04.023
Carter, M. R., and Gregorich, E. G. (2007). Soil sampling and methods of analysis. 2nd Edn Boca Raton, FL, USA: CRC Press.
Cederholm, C. J., Houston, D. B., Cole, D. L., and Scarlett, W. J. (1989). Fate of Coho Salmon (Oncorhynchus kisutch) carcasses in spawning streams. Can. J. Fish. Aquat. Sci. 46, 1347–1355. doi: 10.1139/f89-173
Cederholm, C. J., Kunze, M. D., Murota, T., and Sibatani, A. (1999). Pacific Salmon carcasses: essential contributions of nutrients and energy for aquatic and terrestrial ecosystems. Fisheries 24, 6–15. doi: 10.1577/1548-8446(1999)024<0006:PSC>2.0.CO;2
Chatterjee, A., and Clay, D. (2016). Soil Fertility Management in Agroecosystems. American Society of Agronomy and Soil Science Society of America. Madison, WI, USA
Chen, Y., Camps-Arbestain, M., Shen, Q., Singh, B., and Cayuela, M. L. (2018). The long-term role of organic amendments in building soil nutrient fertility: a meta-analysis and review. Nutr. Cycl. Agroecosyst. 111, 103–125. doi: 10.1007/s10705-017-9903-5
Chen, C. R., and Xu, Z. H. (2008). Analysis and behavior of soluble organic nitrogen in forest soils. J. Soil. Sediment. 8, 363–378. doi: 10.1007/s11368-008-0044-y
Christie, K. S., and Reimchen, T. E. (2008). Presence of salmon increases passerine density on Pacific northwest streams. Auk 125, 51–59. doi: 10.1525/auk.2008.125.1.51
Collins, S. F., Baxter, C. V., Marcarelli, A. M., and Wipfli, M. S. (2016). Effects of experimentally added salmon subsidies on resident fishes via direct and indirect pathways. Ecosphere 7:1248. doi: 10.1002/ecs2.1248
Collins, S. F., Marcarelli, A. M., Baxter, C. V., and Wipfli, M. S. (2015). A critical assessment of the ecological assumptions underpinning compensatory mitigation of salmon-derived nutrients. Environ. Manag. 56, 571–586. doi: 10.1007/s00267-015-0538-5
Cox, K. D., Davies, H. L., Davidson, K. H., Gerwing, T. G., Dudas, S. E., and Juanes, F. (2020). Shellfish subsidies along the Pacific coast of North America. Ecography 43, 668–681. doi: 10.1111/ecog.04476
Cutler, D. R., Edwards, T. C. Jr., Beard, K. H., Cutler, A., Hess, K. T., Gibson, J., et al. (2007). Random forests for classification in ecology. Ecology 88, 2783–2792. doi: 10.1890/07-0539.1
D’Amore, D. V., Bonzey, N. S., Berkowitz, J., Rüegg, J., and Bridgham, S. (2011). Holocene soil-geomorphic surfaces influence the role of salmon-derived nutrients in the coastal temperate rainforest of Southeast Alaska. Geomorphology 126, 377–386. doi: 10.1016/j.geomorph.2010.04.014
D’Amore, D. V., Chaloner, D. T., Gerig, B. S., Berkowitz, J. F., and Bridgham, S. D. (2020). The nutrient legacy left by salmon tissue on riparian soils in Southeast Alaska. Soil Sci. Soc. Am. J. 84, 877–887. doi: 10.1002/saj2.20043
D’Amore, D. V., Hennon, P. E., Schaberg, P. G., and Hawley, G. J. (2009). Adaptation to exploit nitrate in surface soils predisposes yellow-cedar to climate-induced decline while enhancing the survival of western redcedar: a new hypothesis. For. Ecol. Manage. 258, 2261–2268. doi: 10.1016/j.foreco.2009.03.006
Delgado-Baquerizo, M., Oliverio, A. M., Brewer, T. E., Benavent-González, A., Eldridge, D. J., Bardgett, R. D., et al. (2018). A global atlas of the dominant bacteria found in soil. Science 359, 320–325. doi: 10.1126/science.aap9516
Dennert, A. M., Elle, E., and Reynolds, J. D. (2023). Experimental addition of marine-derived nutrients affects wildflower traits in a coastal meta-ecosystem. R. Soc. Open Sci. 10:221008. doi: 10.1098/rsos.221008
Dhaliwal, S. S., Naresh, R. K., Mandal, A., Singh, R., and Dhaliwal, M. K. (2019). Dynamics and transformations of micronutrients in agricultural soils as influenced by organic matter build-up: a review. Environ Sustain Indic 1-2:100007. doi: 10.1016/j.indic.2019.100007
Diacono, M., and Montemurro, F. (2011). “Long-term effects of organic amendments on soil fertility” in Sustainable Agriculture Volume 2. eds. E. Lichtfouse, M. Hamelin, M. Navarrete, and P. Debaeke (Netherlands: Springer), 761–786.
Dinel, H., Schnitzer, M., and Mehuys, G. R. (1990). “Soil lipids: origin, nature, content, decomposition, and effect on soil physical properties” in J.-M. Bollag, Soil Biochemistry (Abingdon, Oxfordshire, United Kingdom: Routledge), 397–430.
Dolloff, C. A. (1993). Predation by river otters (Lutra canadensis) on juvenile coho salmon (Oncorhynchus kisutch) and Dolly Varden (Salvelinus malma) in Southeast Alaska. Can. J. Fish. Aquat. Sci. 50, 312–315. doi: 10.1139/f93-036
Drake, D. C., Smith, J. V., and Naiman, R. J. (2005). Salmon decay and nutrient contributions to riparian forest soils. Northwest Sci 79, 61–71.
Eamer, J. B., Shugar, D. H., Walker, I. J., Lian, O. B., Neudorf, C. M., and Telka, A. M. (2017). A glacial readvance during retreat of the cordilleran ice sheet, British Columbia central coast. Quatern. Res. 87, 468–481. doi: 10.1017/qua.2017.16
Edmonds, R. L., Binkley, D., Feller, M. C., Sollins, P., Abee, A., and Myrold, D. D. (1989). “Nutrient cycling: Effects on productivity of northwest forests” in Maintaining the long-term productivity of Pacific northwest Forest ecosystems. eds. D. Perry, R. Meurisse, B. Thomas, R. Miller, J. Boyle, and C. Perry, et al. (Portland, Ore: Timber Press), 17–35.
Feddern, M. L., Holtgrieve, G. W., Perakis, S. S., Hart, J., Ro, H., and Quinn, T. P. (2019). Riparian soil nitrogen cycling and isotopic enrichment in response to a long-term salmon carcass manipulation experiment. Ecosphere 10:e02958. doi: 10.1002/ecs2.2958
Fenn, M. E., Huntington, T. G., McLaughlin, S. B., Eagar, C., Gomez, A., and Cook, R. B. (2006). Status of soil acidification in North America. J. For. Sci. 52, S3–S13. doi: 10.17221/10152-JFS
Fisher, J. B., Badgley, G., and Blyth, E. (2012). Global nutrient limitation in terrestrial vegetation. Global Biogeochem. Cycles 26:4252. doi: 10.1029/2011GB004252
Fox, C. A., Tarnocai, C., and Trowbridge, R. (1987). Classification, macromorphology and chemical characteristics of Folisols from British Columbia. Can. J. Soil Sci. 67, 765–778. doi: 10.4141/cjss87-074
Gao, Q., Hasselquist, N. J., Palmroth, S., Zheng, Z., and You, W. (2014). Short-term response of soil respiration to nitrogen fertilization in a subtropical evergreen forest. Soil Biol. Biochem. 76, 297–300. doi: 10.1016/j.soilbio.2014.04.020
Gao, W., Yang, H., Kou, L., and Li, S. (2015). Effects of nitrogen deposition and fertilization on N transformations in forest soils: a review. J. Soil. Sediment. 15, 863–879. doi: 10.1007/s11368-015-1064-z
Gende, S. M., Miller, A. E., and Hood, E. (2007). The effects of salmon carcasses on soil nitrogen pools in a riparian forest of southeastern Alaska. Can. J. For. Res. 37, 1194–1202. doi: 10.1139/X06-318
Halsey, L. G. (2019). The reign of the p-value is over: what alternative analyses could we employ to fill the power vacuum? Biol. Lett. 15:20190174. doi: 10.1098/rsbl.2019.0174
Helfield, J. M., and Naiman, R. J. (2001). Effects of salmon-derived nitrogen on riparian forest growth and implications for stream productivity. Ecology 82, 2403–2409. doi: 10.1890/0012-9658(2001)082[2403:EOSDNO]2.0.CO;2
Helfield, J. M., and Naiman, R. J. (2006). Keystone interactions: Salmon and Bear in riparian forests of Alaska. Ecosystems 9, 167–180. doi: 10.1007/s10021-004-0063-5
Hendershot, W. H., and Duquette, M. (1986). A simple barium chloride method for determining cation exchange capacity and exchangeable cations 1. Soil Sci. Soc. Am. J. 50, 605–608. doi: 10.2136/sssaj1986.03615995005000030013x
Hocking, M. D., and Reimchen, T. E. (2006). Consumption and distribution of salmon (Oncorhynchus spp.) nutrients and energy by terrestrial flies. Can. J. Fish. Aquat. Sci. 63, 2076–2086. doi: 10.1139/f06-110
Hocking, M. D., and Reynolds, J. D. (2011). Impacts of Salmon on riparian plant diversity. Science 331, 1609–1612. doi: 10.1126/science.1201079
Hocking, M. D., and Reynolds, J. D. (2012). Nitrogen uptake by plants subsidized by Pacific salmon carcasses: a hierarchical experiment. Can. J. For. Res. 42, 908–917. doi: 10.1139/x2012-045
Hocking, M. D., Ring, R. A., and Reimchen, T. E. (2009). The ecology of terrestrial invertebrates on Pacific salmon carcasses. Ecol. Res. 24, 1091–1100. doi: 10.1007/s11284-009-0586-5
Holtgrieve, G. W., Schindler, D. E., and Jewett, P. K. (2009). Large predators and biogeochemical hotspots: Brown bear (Ursus arctos) predation on salmon alters nitrogen cycling in riparian soils. Ecol. Res. 24, 1125–1135. doi: 10.1007/s11284-009-0591-8
Hood, E., Fellman, J. B., Edwards, R. T., D’Amore, D. V., and Scott, D. (2019). Salmon-derived nutrient and organic matter fluxes from a coastal catchment in Southeast Alaska. Freshw. Biol. 64, 1157–1168. doi: 10.1111/fwb.13292
Huang, X., Terrer, C., Dijkstra, F. A., Hungate, B. A., Zhang, W., and van Groenigen, K. J. (2020). New soil carbon sequestration with nitrogen enrichment: a meta-analysis. Plant and Soil 454, 299–310. doi: 10.1007/s11104-020-04617-x
Hynicka, J. D., Pett-Ridge, J. C., and Perakis, S. S. (2016). Nitrogen enrichment regulates calcium sources in forests. Glob. Chang. Biol. 22, 4067–4079. doi: 10.1111/gcb.13335
Jiang, J., Wang, Y.-P., Yu, M., Cao, N., and Yan, J. (2018). Soil organic matter is important for acid buffering and reducing aluminum leaching from acidic forest soils. Chem. Geol. 501, 86–94. doi: 10.1016/j.chemgeo.2018.10.009
Keefe, O., and Edwards, R. T. (2003). Evidence for hyporheic transfer and removal of marine-derived nutrients in a sockeye stream in Southwest Alaska. In: American Fisheries Society Symposium, 99–110. Available at: http://riversandcreeks.com/research/publications/okeefe_edwards_2002.pdf
Kohler, A. E., Pearsons, T. N., Zendt, J. S., Mesa, M. G., Johnson, C. L., and Connolly, P. J. (2012). Nutrient enrichment with Salmon carcass analogs in the Columbia River basin, USA: a stream food web analysis. Trans. Am. Fish. Soc. 141, 802–824. doi: 10.1080/00028487.2012.676380
Kranabetter, J. M. (2019). Increasing soil carbon content with declining soil manganese in temperate rainforests: is there a link to fungal Mn? Soil Biol. Biochem. 128, 179–181. doi: 10.1016/j.soilbio.2018.11.001
Kranabetter, J. M., Banner, A., and Groot, A.De. (2005). An assessment of phosphorus limitations to soil nitrogen availability across forest ecosystems of north coastal British Columbia. Can. J. For. Res., 35, 530–540. doi: 10.1139/x04-192
Kranabetter, J. M., Philpott, T. J., and Dunn, D. E. (2021). Manganese limitations and the enhanced soil carbon sequestration of temperate rainforests. Biogeochemistry 156, 195–209. doi: 10.1007/s10533-021-00840-5
Kranabetter, J. M., Sholinder, A., and De Montigny, L. (2020). Contrasting conifer species productivity in relation to soil carbon, nitrogen and phosphorus stoichiometry of British Columbia perhumid rainforests. (Göttingen, Germany: Copernicus Publications) 17, 1247–1260.
Kuhn, M. (2008). Building predictive models in R using the caret package. J. Stat. Softw. 28:i05. doi: 10.18637/jss.v028.i05
Lamb, E. G., and Megill, W. (2003). The shoreline fringe Forest and adjacent peatlands of the southern Central British Columbia coast. Can Field Nat 117, 209–217. doi: 10.22621/cfn.v117i2.684
Larocque, A. T. (2022). Fish, forests, fungi: Soils in the ‘salmon forests’ of British Columbia Vancouver, BC, Canada: University of British Columbia.
LeBauer, D. S., and Treseder, K. K. (2008). Nitrogen limitation of net primary productivity in terrestrial ecosystems is globally distributed. Ecology 89, 371–379. doi: 10.1890/06-2057.1
Legendre, P., Oksanen, J., and Braak, C. J. F.Ter. (2011). Testing the significance of canonical axes in redundancy analysis. Methods Ecol. Evol., 2, 269–277. doi: 10.1111/j.2041-210X.2010.00078.x
Levi, P. S., Tank, J. L., Rüegg, J., Janetski, D. J., Tiegs, S. D., Chaloner, D. T., et al. (2013). Whole-stream metabolism responds to spawning Pacific salmon in their native and introduced ranges. Ecosystems 16, 269–283. doi: 10.1007/s10021-012-9613-4
Maathuis, F. J. (2009). Physiological functions of mineral macronutrients. Curr. Opin. Plant Biol. 12, 250–258. doi: 10.1016/j.pbi.2009.04.003
Mathewson, D. D., Hocking, M. D., and Reimchen, T. E. (2003). Nitrogen uptake in riparian plant communities across a sharp ecological boundary of salmon density. BMC Ecol. 3, 1–11. doi: 10.1186/1472-6785-3-4
Mehlich, A. (1984). Mehlich 3 soil test extractant: a modification of Mehlich 2 extractant. Commun. Soil Sci. Plant Anal. 15, 1409–1416. doi: 10.1080/00103628409367568
Metcalfe, R. J., Nault, J., and Hawkins, B. J. (2011). Adaptations to nitrogen form: comparing inorganic nitrogen and amino acid availability and uptake by four temperate forest plants. Can. J. For. Res. 41, 1626–1637. doi: 10.1139/x11-090
Morris, M. R., and Stanford, J. A. (2011). Floodplain succession and soil nitrogen accumulation on a salmon river in southwestern Kamchatka. Ecological monographs 81, 43–61. doi: 10.1890/08-2296.1
Morris, A. E., Stark, J. M., and Gilbert, B. K. (2005). Evaluation of isotopic fractionation error on calculations of marine-derived nitrogen in terrestrial ecosystems. Can. J. For. Res. 35, 1604–1616. doi: 10.1139/x05-094
Nicolodi, M., and Gianello, C. (2014). Understanding soil as an open system and fertility as an emergent property of the soil system (526-2016–37873). Sustain Agricult Res. 4:94. doi: 10.22004/ag.econ.230408
Noulas, C., Tziouvalekas, M., and Karyotis, T. (2018). Zinc in soils, water and food crops. J. Trace Elem. Med. Biol. 49, 252–260. doi: 10.1016/j.jtemb.2018.02.009
Oksanen, J., Simpson, G., Blanchet, F., Kindt, R., Legendre, P., and Minchin, P. (2022). vegan Community Ecology Package, R package version 2, 6–4.
Oliver, A. A., Tank, S. E., Giesbrecht, I., Korver, M. C., Floyd, W. C., Sanborn, P., et al. (2017). A global hotspot for dissolved organic carbon in hypermaritime watersheds of coastal British Columbia. Biogeosciences 14, 3743–3762. doi: 10.5194/bg-14-3743-2017
Pandey, D., Kehri, H. K., Zoomi, I., Singh, U., Chaudhri, K. L., and Akhtar, O. (2020). “Potassium solubilizing microbes: diversity, ecological significances and biotechnological applications” in Plant microbiomes for sustainable agriculture. eds. A. N. Yadav, J. Singh, A. A. Rastegari, and N. Yadav (Cham: Springer International Publishing), 263–286.
Patzel, N., Sticher, H., and Karlen, D. L. (2000). Soil fertility—phenomenon and concept. J. Plant Nutr. Soil Sci. 163, 129–142. doi: 10.1002/(SICI)1522-2624(200004)163:2<129::AID-JPLN129>3.0.CO;2-D
Pearsons, T. N., Roley, D. D., and Johnson, C. L. (2007). Development of a carcass analog for nutrient restoration in streams. Fisheries 32, 114–124. doi: 10.1577/1548-8446(2007)32[114:DOACAF]2.0.CO;2
Peng, Z., Liang, C., Gao, M., Qiu, Y., Pan, Y., Gao, H., et al. (2022). The neglected role of micronutrients in predicting soil microbial structure. NPJ Biofilms Microbiomes 8:103. doi: 10.1038/s41522-022-00363-3
Penn, C. J., and Camberato, J. J. (2019). A Critical Review on Soil Chemical Processes that Control How Soil pH Affects Phosphorus Availability to Plants. Agriculture, 9, doi: 10.3390/agriculture9060120
Perakis, S. S., Maguire, D. A., Bullen, T. D., Cromack, K., Waring, R. H., and Boyle, J. R. (2006). Coupled nitrogen and calcium cycles in forests of the Oregon coast range. Ecosystems 9, 63–74. doi: 10.1007/s10021-004-0039-5
Perakis, S. S., Sinkhorn, E. R., Catricala, C. E., Bullen, T. D., Fitzpatrick, J. A., Hynicka, J. D., et al. (2013). Forest calcium depletion and biotic retention along a soil nitrogen gradient. Ecol. Appl. 23, 1947–1961. doi: 10.1890/12-2204.1
Perry, L. G., Shafroth, P. B., and Perakis, S. S. (2017). Riparian soil development linked to Forest succession above and below dams along the Elwha River, Washington, USA. Ecosystems 20, 104–129. doi: 10.1007/s10021-016-0080-1
Pinay, G., O’Keefe, T. C., Edwards, R. T., and Naiman, R. J. (2009). Nitrate removal in the hyporheic zone of a salmon river in Alaska. River Res. Appl. 25, 367–375. doi: 10.1002/rra.1164
Post, W. M., Pastor, J., Zinke, P. J., and Stangenberger, A. G. (1985). Global patterns of soil nitrogen storage. Nature 317, 613–616. doi: 10.1038/317613a0
Prescott, C. (1997). SCHIRP—Salal cedar hemlock integrated research program. Research update #1: December 1996. Available at: https://cfs.nrcan.gc.ca/publications?id=4717
Prescott, C., Nery, V., Niejenhuis, A.Van, Sajedi, T., and Marshall, P. (2013). Nutrition management of cedar and hemlock plantations in coastal British Columbia. New For., 44, 769–784. doi: 10.1007/s11056-013-9380-x
Quinn, T. P., Helfield, J. M., Austin, C. S., Hovel, R. A., and Bunn, A. G. (2018). A multidecade experiment shows that fertilization by salmon carcasses enhanced tree growth in the riparian zone. Ecology 99, 2433–2441. doi: 10.1002/ecy.2453
R Core Team. (2019). R: a language and environment for statistical computing [computer software]. R foundation for statistical Computing Available at: https://www.R-project.org/
Rammell, N. F., Dennert, A. M., Ernst, C. M., and Reynolds, J. D. (2021). Effects of spawning Pacific salmon on terrestrial invertebrates: insects near spawning habitat are isotopically enriched with nitrogen-15 but display no differences in body size. Ecol. Evol. 11, 12728–12738. doi: 10.1002/ece3.8017
Reimchen, T. E. (2017). Diverse ecological pathways of Salmon nutrients through an intact marine-terrestrial Interface. Can Field Nat. 131:1965. doi: 10.22621/cfn.v131i4.1965
Reimchen, T. E., Mathewson, D. D., Hocking, M. D., Moran, J., and Harris, D. (2003). Isotopic evidence for enrichment of salmon-derived nutrients in vegetation, soil, and insects in riparian zones in coastal British Columbia. In: American Fisheries Society Symposium, 59–70.
Richardson, S. J., Peltzer, D. A., Allen, R. B., McGlone, M. S., and Parfitt, R. L. (2004). Rapid development of phosphorus limitation in temperate rainforest along the Franz Josef soil chronosequence. Oecologia 139, 267–276. doi: 10.1007/s00442-004-1501-y
Roussos, P. A., Gasparatos, D., Kechrologou, K., Katsenos, P., and Bouchagier, P. (2017). Impact of organic fertilization on soil properties, plant physiology and yield in two newly planted olive (Olea europaea L.) cultivars under Mediterranean conditions. Sci. Hortic. 220, 11–19. doi: 10.1016/j.scienta.2017.03.019
Sayer, A. M. (2008). Marine derived nitrogen use by Kenai peninsula riparian plants: effects on mineral nutrition and growth [M.S., University of Alaska Anchorage]. Available at: http://search.proquest.com/docview/304337010/abstract/C7CCAEBBB7F540B2PQ/1
Schmidt, M. W. I., Torn, M. S., Abiven, S., Dittmar, T., Guggenberger, G., Janssens, I. A., et al. (2011). Persistence of soil organic matter as an ecosystem property. Nature 478, 49–56. doi: 10.1038/nature10386
Scott, E. E., Perakis, S. S., and Hibbs, D. E. (2008). δ15N patterns of Douglas-fir and red Alder riparian forests in the Oregon coast range. For. Sci. 54, 140–147. doi: 10.1093/forestscience/54.2.140
Shaul, L., Weitkamp, L., Simpson, K., and Sawada, J. (2007). Trends in abundance and size of coho salmon in the Pacific rim. North Pac Anadrom Fish Commission Bull 4, 93–104.
Siemens, L. D., Dennert, A. M., Obrist, D. S., and Reynolds, J. D. (2020). Spawning salmon density influences fruit production of salmonberry (Rubus spectabilis). Ecosphere 11:e03282. doi: 10.1002/ecs2.3282
Solly, E. F., Weber, V., Zimmermann, S., Walthert, L., Hagedorn, F., and Schmidt, M. W. I. (2020). A critical evaluation of the relationship between the effective cation exchange capacity and soil organic carbon content in Swiss Forest soils. Front For Glob Change 3:98. doi: 10.3389/ffgc.2020.00098
Souther, J. G. (1986). The western Anahim Belt: root zone of a peralkaline magma system. Can. J. Earth Sci. 23, 895–908. doi: 10.1139/e86-091
Strawn, D. G., Bohn, H. L., and O’Connor, G. A. (2020). Soil chemistry. 5th Edn Hoboken, NJ, USA: Wiley-Blackwell.
Subbarao, G. V., Ito, O., Berry, W. L., and Wheeler, R. M. (2003). Sodium—A functional plant nutrient. Crit. Rev. Plant Sci. 22, 391–416. doi: 10.1080/07352680390243495
Swain, N. R., and Reynolds, J. D. (2015). Effects of Salmon-derived nutrients and habitat characteristics on population densities of stream-resident sculpins. PloS One 10:e0116090. doi: 10.1371/journal.pone.0116090
Tian, D., and Niu, S. (2015). A global analysis of soil acidification caused by nitrogen addition. Environ. Res. Lett. 10:024019. doi: 10.1088/1748-9326/10/2/024019
Trant, A. J., Nijland, W., Hoffman, K. M., Mathews, D. L., McLaren, D., Nelson, T. A., et al. (2016). Intertidal resource use over millennia enhances forest productivity. Nat. Commun. 7:12491. doi: 10.1038/ncomms12491
Turner, B. L., and Blackwell, M. S. A. (2013). Isolating the influence of pH on the amounts and forms of soil organic phosphorus. European Journal of Soil Science, 64, 249–259. doi: 10.1111/ejss.12026
van den Top, G. G., Reynolds, J. D., Prins, H. H., Mattsson, J., Green, D. J., and Ydenberg, R. C. (2018). From salmon to salmonberry: the effects of salmon-derived nutrients on the stomatal density of leaves of the nitriphilic shrub Rubus spectabilis. Funct. Ecol. 32, 2625–2633. doi: 10.1111/1365-2435.13202
Wagner, M. A., and Reynolds, J. D. (2019). Salmon increase forest bird abundance and diversity. PloS One 14:e0210031. doi: 10.1371/journal.pone.0210031
Walsh, J. C., Pendray, J. E., Godwin, S. C., Artelle, K. A., Kindsvater, H. K., Field, R. D., et al. (2020). Relationships between Pacific salmon and aquatic and terrestrial ecosystems: implications for ecosystem-based management. Ecology 101:e03060. doi: 10.1002/ecy.3060
Weetman, G. F., and Prescott, C. (2000). The structure, functioning and management of old-growth cedar-hemlock-fir forests on Vancouver Island, British Columbia. For Handbook 2, 275–287.
Wheeler, T. A., and Kavanagh, K. L. (2017). Soil biogeochemical responses to the deposition of anadromous fish carcasses in inland riparian forests of the Pacific northwest, USA. Can. J. For. Res. 47, 1506–1516. doi: 10.1139/cjfr-2017-0194
Wheeler, T. A., Kavanagh, K. L., and Daanen, S. A. (2014). Terrestrial Salmon carcass decomposition: nutrient and isotopic dynamics in Central Idaho. Northwest Sci 88, 106–119. doi: 10.3955/046.088.0206
Wilhelm Scherer, H. (2009). Sulfur in soils. J. Plant Nutr. Soil Sci. 172, 326–335. doi: 10.1002/jpln.200900037
Yruela, I. (2005). Copper in plants. Braz. J. Plant Physiol. 17, 145–156. doi: 10.1590/S1677-04202005000100012
Zang, H., Wang, J., and Kuzyakov, Y. (2016). N fertilization decreases soil organic matter decomposition in the rhizosphere. Appl. Soil Ecol. 108, 47–53. doi: 10.1016/j.apsoil.2016.07.021
Zhao, X. Q., Chen, R. F., and Shen, R. F. (2014). Coadaptation of plants to multiple stresses in acidic soils. Soil Sci. 179, 503–513. doi: 10.1097/SS.0000000000000086
Keywords: marine-derived nutrients, soils, salmon, ecosystem subsidies, legacies
Citation: Larocque A and Simard SW (2023) Legacy of salmon-derived nutrients on riparian soil chemistry and soil fertility on the Central Coast of British Columbia, Canada. Front. For. Glob. Change. 6:1010294. doi: 10.3389/ffgc.2023.1010294
Edited by:
Frank Hagedorn, Snow and Landscape Research (WSL), SwitzerlandReviewed by:
David Damore, Forest Service (USDA), United StatesThomas Henry DeLuca, Oregon State University, United States
Copyright © 2023 Larocque and Simard. This is an open-access article distributed under the terms of the Creative Commons Attribution License (CC BY). The use, distribution or reproduction in other forums is permitted, provided the original author(s) and the copyright owner(s) are credited and that the original publication in this journal is cited, in accordance with accepted academic practice. No use, distribution or reproduction is permitted which does not comply with these terms.
*Correspondence: Allen Larocque, YWxsZW4ubGFyb2NxdWVAZ21haWwuY29t