- 1Community Ecology, Swiss Federal Institute for Forest, Snow and Landscape Research, Birmensdorf, Switzerland
- 2USDA Forest Service, Rocky Mountain Research Station, Moscow, ID, United States
- 3Remote Sensing Laboratories, Department of Geography, University of Zurich, Zurich, Switzerland
- 4Research School of Astronomy and Astrophysics, Australian National University, Canberra, ACT, Australia
- 5British Columbia Ministry of Forests, Lands and Natural Resource Operations, Victoria, BC, Canada
- 6Natural Resources Institute Finland (Luke), Joensuu, Finland
- 7College of Forest Resources and Environmental Sciences, Michigan Technological University, Houghton, MI, United States
- 8The Key Laboratory for Silviculture and Conservation of Ministry of Education, Beijing Forestry University, Beijing, China
- 9Retired, Olympia, WA, United States
- 10State Key Laboratory of Vegetation and Environmental Change, Institute of Botany, Chinese Academy of Sciences, Beijing, China
Forest ecosystems sequester approximately half of the world’s organic carbon (C), most of it in the soil. The amount of soil C stored depends on the input and decomposition rate of soil organic matter (OM), which is controlled by the abundance and composition of the microbial and invertebrate communities, soil physico-chemical properties, and (micro)-climatic conditions. Although many studies have assessed how these site-specific climatic and soil properties affect the decomposition of fresh OM, differences in the type and quality of the OM substrate used, make it difficult to compare and extrapolate results across larger scales. Here, we used standard wood stakes made from aspen (Populus tremuloides Michx.) and loblolly pine (Pinus taeda L.) to explore how climate and abiotic soil properties affect wood decomposition across 44 unharvested forest stands located across the northern hemisphere. Stakes were placed in three locations: (i) on top of the surface organic horizons (surface), (ii) at the interface between the surface organic horizons and mineral soil (interface), and (iii) into the mineral soil (mineral). Decomposition rates of both wood species was greatest for mineral stakes and lowest for stakes placed on the surface organic horizons, but aspen stakes decomposed faster than pine stakes. Our models explained 44 and 36% of the total variation in decomposition for aspen surface and interface stakes, but only 0.1% (surface), 12% (interface), 7% (mineral) for pine, and 7% for mineral aspen stakes. Generally, air temperature was positively, precipitation negatively related to wood stake decomposition. Climatic variables were stronger predictors of decomposition than soil properties (surface C:nitrogen ratio, mineral C concentration, and pH), regardless of stake location or wood species. However, climate-only models failed in explaining wood decomposition, pointing toward the importance of including local-site properties when predicting wood decomposition. The difficulties we had in explaining the variability in wood decomposition, especially for pine and mineral soil stakes, highlight the need to continue assessing drivers of decomposition across large global scales to better understand and estimate surface and belowground C cycling, and understand the drivers and mechanisms that affect C pools, CO2 emissions, and nutrient cycles.
Introduction
Forest ecosystems play an important role in the global carbon (C) cycle (Schimel, 1995; Pan et al., 2011) and sequester approximately half of the world’s organic C, most of which is stored in the soil (Dixon et al., 1994; Wei et al., 2014; Nave et al., 2019). Soil C storage represents the balance between input of fresh organic matter (OM) and turnover of labile and recalcitrant soil C through decomposition, leaching, and erosion (Mayer et al., 2020). Fresh OM, mostly dead plant litter or woody material from above- or belowground sources, is decomposed by soil fauna and microorganisms at a rate that is related to soil physical, chemical, and biological properties, vegetation composition, topography, climate, and land use, which interact to determine the fertility or productivity of a site (Jackson et al., 2017; Wiesmeier et al., 2019).
Many studies have documented the influence of climate and soil properties on fresh OM decomposition rates (e.g., Tóth et al., 2007; Goebel et al., 2011; Juhos et al., 2021), but differences in the type and quality of OM substrate used in these studies makes it challenging to compare and extrapolate results to other forest sites (Parton et al., 2007; Talbot and Treseder, 2012). By holding the quality [e.g., lignin, cellulose content, C:nitrogen (N) ratio] of the decomposing OM material constant across sites, decomposition becomes a function of soil abiotic (micro-climate, soil physical, and chemical properties) and biotic (microbial and invertebrate communities) properties (Parton et al., 2007; Zhou et al., 2007; Cornwell et al., 2009; Weedon et al., 2009; Russell et al., 2015; Zhang and Wang, 2015; Magnússon<suffix>I</suffix>, Tietema et al., 2016; Wang et al., 2018; Hu et al., 2021; Seibold et al., 2021). Therefore, using standard materials allows us to compare and better understand decomposition processes across different climatic regions, forest, and soil types (e.g., Gholz et al., 2000; Parton et al., 2007; Djukic et al., 2018; Fanin et al., 2019).
Many different types of standard materials, such as tea bags, filter paper, cotton cloth (e.g., Latter and Howson, 1977; Latter and Walton, 1988; Keuskamp et al., 2013; Djukic et al., 2018; Fanin et al., 2019), or plant material [leaf litter, fine roots (e.g., Berg, 2000; Gholz et al., 2000; Parton et al., 2007)] have been used in decomposition studies to date. However, standardized woody material, e.g., wood blocks, dowels, chop sticks, tongue depressors, or wood stakes (e.g., Jurgensen et al., 2006; Mackenzie et al., 2006; Bradford et al., 2014) are likely better suited to explore decomposition processes in forested ecosystems, as wood is a “normal” component of forest soils (surface residue, stumps, roots), important for forest soil C sequestration (e.g., Dixon et al., 1994) and its slow decomposition integrates soil abiotic and biotic conditions over longer time periods (Smyth et al., 2016; Oberle et al., 2020). Here, we use standard aspen (Populus tremuloides Michx.) and loblolly pine (Pinus taeda L.) stakes to assess decomposition. Aspen wood generally decomposes faster than pine wood as it has lower wood density, lignin content and N:lignin ratio, but higher N concentration (Cornwell et al., 2009; Weedon et al., 2009; Bani et al., 2018; Wang et al., 2018, 2020; Seibold et al., 2021).
The majority of wood decomposition studies have been conducted by placing the woody material aboveground, i.e., on top of the soil surface organic horizon (González et al., 2008; Weedon et al., 2009; Bradford et al., 2014; Liu et al., 2015; Hu et al., 2018; Lustenhouwer et al., 2020). Considerably less is known about decomposition processes of standard wood material buried belowground (e.g., Smyth et al., 2016; Erdenebileg et al., 2020; Jurgensen et al., 2020), although the volume of buried wood can contribute up to 935 m3 ha–1 in temperate forests (Weedon et al., 2009; Moroni et al., 2015). In addition, only a few studies used standardized woody material across widely different sites to allow for a direct comparison of decomposition processes across larger scales (e.g., Reed et al., 2003; Adams et al., 2021; Page-Dumroese et al., 2021). Therefore, we initiated a large-scale study using standard wood stakes to assess the effect of climate, soil properties, and ecosystem disturbance (e.g., fire, drainage, timber harvesting) on above- and belowground decomposition processes in forested ecosystem across the northern hemisphere in 1998 (Jurgensen et al., 2006).
Subsequent results from individual studies or regional sites showed that buried wood stakes generally decomposed faster than when put on the soil surface (Risch et al., 2013; Page-Dumroese et al., 2019; Wang et al., 2019; Jurgensen et al., 2020). Climatic properties, more specifically, higher soil temperatures, and to a lower degree higher precipitation, but not air temperature, were found to be the drivers of these differences in decomposition in Swiss subalpine forests (Risch et al., 2013). Similarly, higher soil temperatures explained higher decomposition rates in boreal forests in Finland (Finér et al., 2016) and after wildfires in Montana, United States (Page-Dumroese et al., 2019). However, average annual air temperature and precipitation did not affect decomposition of wood stakes placed into the mineral soil along a transect from southern British Columbia to southern Oregon (Page-Dumroese et al., 2021), while higher moisture of mineral stakes explained their faster decomposition compared to the stakes placed on the soils surface in a Chinese pine plantation (Wang et al., 2019). This latter result may suggest that actual evapotranspiration might also be a predictor for wood stake decomposition as it includes the amount of water removed from the soil surface (Meentemeyer, 1978; Aerts, 2006). Soil phosphorus was found to be positively related to decomposition in Swiss subalpine forests (Risch et al., 2013), but differences in soil N, OM, and pH did effect on decomposition in China (Wang et al., 2019). Clay type, rather than the amount of clay, in combination with temperature, also had a positive effect on wood decomposition, although this study was conducted in microcosms rather than in situ (Fissore et al., 2016).
While the results from these local and regional studies provide a general understanding on how wood decomposition of standard material is influenced by environmental and climatic properties, an integrated understanding of the long-term and large-scale processes that drive wood decomposition processes across forest ecosystems is still missing. Such knowledge is, however, needed to improve soil decomposition models and better estimate C cycling across large scales (Smyth et al., 2011). In this paper, we therefore, combined wood stake decomposition data from 44 unharvested forest sites distributed across the northern hemisphere. The sites spanned a relevant range of climatic and edaphic conditions and cover all major forest biomes, except for tropical forests (Figure 1). Standard aspen and loblolly pine stakes were placed horizontally on top of the surface organic horizons (surface), at the interface between the surface organic horizons and the mineral soil (interface), and vertically in the mineral soil (mineral) for 1–7 years, depending on local climatic and soil conditions (Supplementary Table 1).
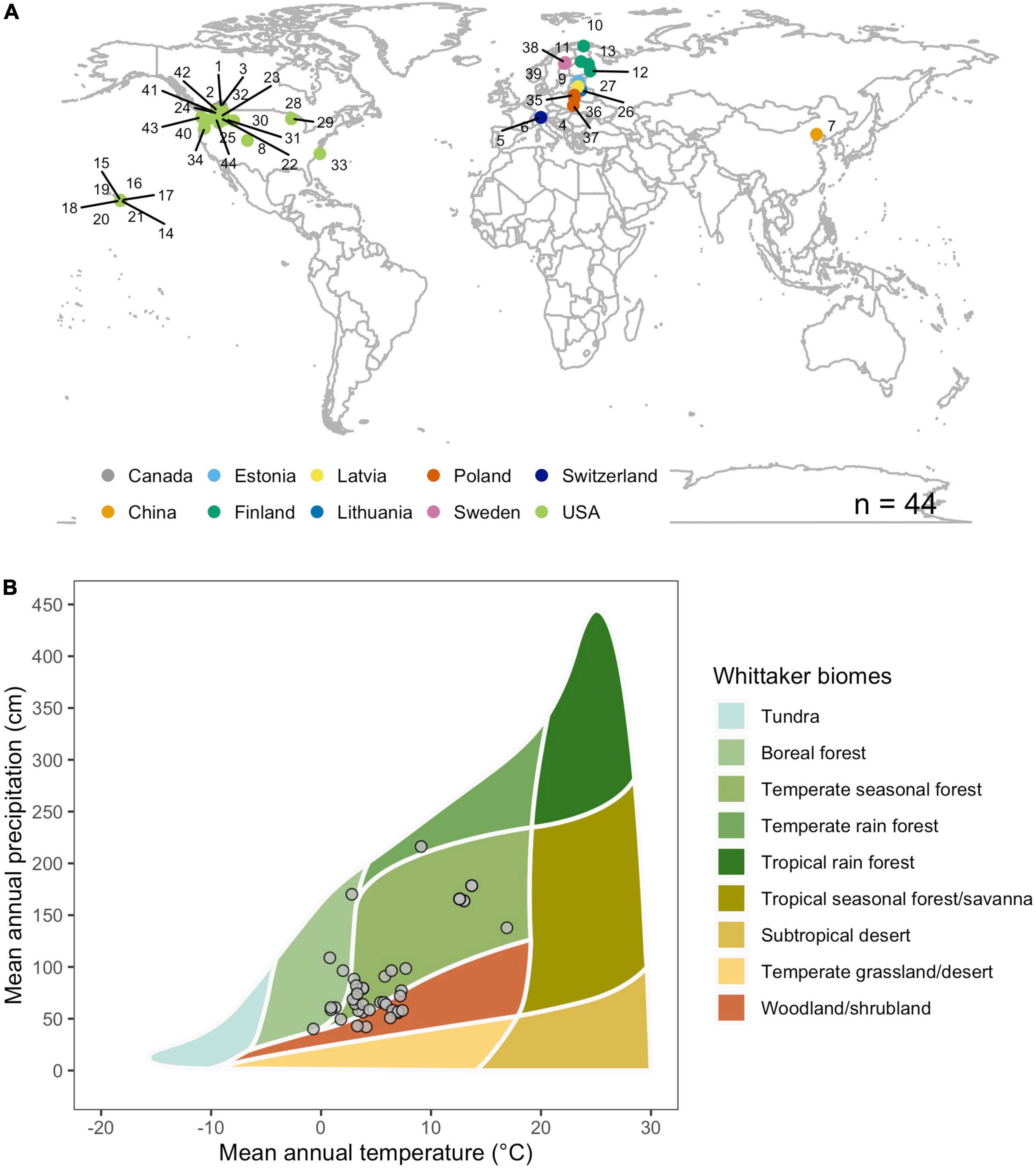
Figure 1. Geographic and climatic distribution of experimental sites. (A) Locations of the 44 sites at which our decomposition experiments were conducted. (B) The sites cover all major forest biomes with the exception of tropical rainforests and tropical seasonal forests/savannas. Mean annual temperature (MAT) and mean annual precipitation (MAP) values represent 30-year mean values between 1970 and 2020. Data was obtained from the Worldclim data base (www.worldclim.com). Additional site details can be found in Supplementary Tables 1, 2.
Our study objectives were to: (1) assess large-scale patterns in decomposition of wood stakes at different incubation locations (surface, interface, mineral) over time within unharvested forest stands, and (2) determine the impact of climate (air temperature, soil temperature, precipitation, actual evapotranspiration) and soil properties (surface organic horizon and mineral soil C and N concentrations, mineral soil pH, bulk density) on decomposition at these different incubation locations. We expected that stakes placed into the mineral soil would decompose faster than stakes placed on the soil surface or at the interface (Busse, 1994; Trofymow, 1998; Risch et al., 2013; Wang et al., 2019; Erdenebileg et al., 2020). We also anticipated that higher air temperature would generally lead to greater decomposition of stakes regardless of where the wood stakes are placed (Brischke and Rapp, 2008; Risch et al., 2013; Finér et al., 2016; Fissore et al., 2016), but that soil temperature would be an even better predictor than air temperature for the mineral stakes. We expected precipitation to interact with air temperature as a controlling factor for decomposition, in particular, for stakes placed at the soil surface where high precipitation and warm temperatures should speed up decomposition (Jurgensen et al., 2020). However, we also expected higher mineral stake decomposition at sites with lower precipitation, as water retention by the mineral soil becomes much more important for decomposition processes at drier sites. Consequently, we also expected that actual evapotranspiration is a better predictor for wood stake decomposition than temperature and precipitation, as it includes the amount of water removed from the soil surface (Meentemeyer, 1978; Aerts, 2006). Sites with higher N levels in the surface organic horizons or mineral soil (Van Der Wal et al., 2007) and, therefore, smaller C:N ratios (Scott and Binkley, 1997; Kielak et al., 2016; Hu et al., 2021), should also have higher decomposition rates at all incubation locations. In addition, we expected that changes in soil physical properties (e.g., bulk density) will affect decomposition via soil C accumulation and microbial activity (Federer et al., 1993; Ruehlmann and Körschens, 2009). Finally, we expected that local climate (Bradford et al., 2014) and soil properties (Adams et al., 2021; Page-Dumroese et al., 2021) would interact to influence decomposition rates across sites.
Materials and Methods
Study Sites
Between 1998 and 2015 we field incubated 7,277 standard wood stakes at 44 sites located in North America, Europe and Asia (Figure 1A). Site elevations ranged from 35 to 2,451 m above sea level, and the sites featured mean annual temperature (MAT) and mean annual precipitation (MAP) of −0.7 to 16.9°C and 401 to 2162 mm, respectively (Figure 1B and Supplementary Table 1). The 44 sites covered boreal and temperate forest biomes, but did not include tropical rain forests or tropical seasonal forest/savannas (Figure 1B). All sites were part of a larger study to investigate the impact of different forest ecosystems, surface topography, soil types, climatic conditions, and disturbance regimes (e.g., forest management activities, fire) on wood stake decomposition (Supplementary Table 2). However, for this study we only used data from undisturbed forest stands. For site characteristics and tree species composition see Supplementary Table 2.
Wood Stakes
We used trembling aspen and loblolly pine as standard wood materials for our study, which are commonly used as test materials in wood decomposition studies (Jurgensen et al., 2006; González et al., 2008; Woodward et al., 2011). These stakes have different wood properties (see Supplementary Table 3). Two field surface or interface (2.5 × 2.5 × 15 cm) and two mineral soil (2.5 × 2.5 × 20 cm or 2.5 × 2.5 × 30 cm) stakes were cut from a longer, sapwood stake of each tree species. Stakes were kiln-dried at 75–80°C for 24 h resulting in a stake moisture content of approximately 15% (±5%). The remaining roughly 10 cm “center section” of the same stake was used as a laboratory control (time = 0) to calculate mass loss of the field stakes. The top of each mineral soil stake was treated with a wood sealer to prevent water wicking through the stake. For additional details on wood stake properties and fabrication see Jurgensen et al. (2006).
Experimental Design and Wood Stake Placement
One to eight replicate plots (2.5 × 2.5 m to 20 × 20 m in size) were established at each of the 44 study sites (Supplementary Table 1) depending on site conditions. All stakes were installed at the beginning of the growing season when soils were moist and the stakes therefore easier to insert into the soil. Wood stakes were placed horizontally on top of the surface organic horizons (surface), and at the interface between surface organic horizons and the mineral soil (interface). Mineral soil stakes were inserted vertically into the mineral soil to a depth of 20 or 30 cm (mineral), depending on site conditions such as the depth to bedrock or the presence of an impenetrable layer (Jurgensen et al., 2020). Individual mineral stakes were placed roughly 40–50 cm apart. Note that some sites had no surface organic horizon, consequently they did not receive surface or interface stakes. When surface and interface stakes were placed, they were secured with stainless steel landscape staples (Forestry Suppliers, Inc., Jackson, MS, United States). To reduce soil compaction and damage to the mineral stakes during installation, the surface organic horizons were removed, and a hole was made with a square 2.5 × 2.5 cm coring tool. Stakes were gently inserted into the mineral soil so that the top was level with the soil surface and all sides were in contact with the mineral soil. Once the stakes were inserted, the organic layer was restored, where present. Wood stake moisture content at the time of installation was <20%.
Twenty-five stakes of each species (aspen, pine) were installed per plot for each soil available location. Five randomly selected stakes per species, soil location, and replicate were extracted at regular intervals (6, 12, or 24 months) depending on climatic and soil conditions and initial number of replicates available (Supplementary Table 1). Total stake incubation times across the 44 sites varied from 1 to 7 years (Supplementary Table 1). A total of 2,337 surface, 2,036 interface, and 2,904 mineral aspen and pine stakes were used across the 44 sites. After the stakes were extracted, they were brushed clean of adhering soil, immediately weighed in the field and air-dried before sending them to the College of Forest Resources and Environmental Science at Michigan Technological University (United States) for processing.
In the laboratory, stakes were further cleaned of any remaining soil, dried at 105°C for 48 h, and weighed. The 10 cm laboratory “control” center section from each stake was used to determine initial weight of the field-deployed surface, interface, and mineral stakes (time = 0), and to calculate wood stake mass loss during the study [for details see Jurgensen et al. (2006)]. Soil depth effects on decomposition were assessed by cutting 2.5 × 2.5 cm blocks at 3.8 and 15.2 cm from the top of the 20 cm long mineral soil stakes, and an additional block at 27.5 cm from 30 cm long stakes.
Climate and Soil Properties
Although it is desirable to collect site-specific air and soil temperature, precipitation and soil moisture data in decomposition studies (Bradford et al., 2016), this is often not feasible due to resource constraints when sites are spread across different continents. Therefore, we sourced high resolution (1 km grid) site-specific monthly air temperature and precipitation data from the CHELSEA climate model (Version 1.2, Karger et al., 2017, 2018) for the specific duration during which the wood stakes were incubated at each site. For example, at site CAN1, all wood stakes were buried in 2001 and were retrieved at yearly intervals until 2005. Hence, for wood stakes incubated from 2001 to 2002, we averaged monthly air temperature and precipitation data for 2001 and 2002. For the wood stakes incubated from 2001 to 2003, we averaged the 2001–2003 monthly air temperature and precipitation data, and so forth for the other years. We also sourced yearly actual evapotranspiration (AET) from the MODIS Global Evapotranspiration Project,1 and calculated AET for the site-specific incubation times. Note that for AET we only had data from 2000 to 2014, so we used the average of all available years (2000–2014) for the years that did not fall within this range (1998, 1999, 2015). Finally, we also sourced high-resolution (1 km grid) mean annual soil temperature (20-year average from 2000 to 2020) data (hereafter MATs) for the 0–5 cm and 5–15 cm soil layer (Lembrechts et al., 2022). We used the average of the 0–5 cm and 1–15 cm soil layer for each site.
To assess the effect of soil chemical and physical properties on decomposition, we collected three replicate samples from both the surface organic horizons (inclusive of fibric, hemic, and sapric layers) and the mineral soil to the same depth as the wood stakes were incubated (0–20 or 0–30 cm). Mineral soil samples were collected using either the soil excavation/foam core method (Page-Dumroese et al., 1999) or a slide hammer (AMS samplers, American Falls, ID, United States). All samples were sent to the USDA Forest Service, Rocky Mountain Research Station Soil Analytical Lab (Moscow, ID, United States). Organic samples were dried at 60°C for 24 h, weighed, and then ground to pass a 0.4 mm sieve. Mineral soil was dried at 105°C for 24 h and weighed to determine total bulk density (weight/unit volume). A subsample of the mineral soil was then ground to pass a 0.4 mm sieve. Both organic and mineral soil samples were analyzed for C and N concentration (Leco, Corp., St. Joseph, MI, United States). Soil pH was determined on a 2:1 water to dry soil paste. Mineral soil and organic horizon properties were averaged by site (Supplementary Table 2).
Calculations and Statistical Analyses
We used linear mixed effects model (LMM) as implemented in the R package nlme (Pinheiro et al., 2021) to assess the effects of incubation location (location), incubation time (time), and wood types (wood type) on wood stake decomposition. Location, time, wood type, and all their interactions were included as fixed effects, plots nested in sites were included as random effects. As all our fixed effects were found to be highly significant (see Section “Results”), we separated our further analyses by location and wood type [see description of structural equation models (SEM) below].
We developed a priori main and alternative causal conceptual models for surface, interface, and mineral stakes (see Supplementary Figures 1–4) and tested how climate and soil properties affect wood decomposition with structural equation modeling (SEM). To avoid collinearity in the predictor variables, we used Spearman’s correlation analysis (Supplementary Figure 5) and dropped variables with Spearman’s rho > |0.70| (Dormann et al., 2013), i.e., we dropped mineral soil N concentration. Spearman correlation coefficients for all pairs of variables were calculated with the R function “cor” and the correlogram displayed using the R library “corrplot.mixed.”
The variables included in the main surface stake models were air temperature, precipitation, and surface organic horizon C:N ratio (Supplementary Figure 1A). In addition, we calculated several alternative models. In one, we used AET instead of air temperature and precipitation (Supplementary Figure 1B), and to test whether surface organic C concentration was a better predictor than surface horizon C:N ratio, we calculated a SEM where we replaced the surface organic horizon C:N ratio with surface organic C concentration (Supplementary Figure 1A). The main and alternative models were hierarchical, with climate variables directly affecting wood decomposition and indirectly via soil properties (Supplementary Figure 1).
For the interface stakes, we increased the level of complexity and included mineral soil properties (Supplementary Figure 2). Again, we calculated a main and several alternative models for both aspen and pine wood stakes. In the two main models, air temperature and precipitation were allowed to affect decomposition directly and indirectly via organic surface C:N ratio, or mineral soil C concentration and mineral soil pH. All soil properties were allowed to directly affect decomposition (Supplementary Figure 2A). Surface horizon C:N ratio was allowed to have an indirect effect on decomposition via the mineral soil properties, and the mineral soil properties were allowed to interact with one another (Supplementary Figure 2A). The same structure was used for all alternative models. In one, we replaced the surface organic horizon C:N ratio with surface organic C concentration (Supplementary Figure 2A). As soil bulk density was not significantly correlated with soil C concentration (Supplementary Figure 5), we also calculated an alternative model where we included bulk density as an additional mineral soil property (Supplementary Figure 2B). A third alternative model used AET instead of air temperature and precipitation (Supplementary Figure 2C).
For the mineral stakes we calculated the same main and alternative models as for the interface stakes (Supplementary Figures 3A–C), but also added a fourth alternative model in which we used soil temperature (MATs) instead of air temperature (Supplementary Figure 3D), as well as a fifth alternative model in which we omitted the surface organic horizon C:N ratio for simplicity (Supplementary Figure 3E). Finally, to assess if climate properties alone could explain wood stake decomposition without including soil properties, we also calculated another series of alternative models (“climate-only models”) in which air temperature and precipitation or AET were allowed to directly affect surface, interface and mineral aspen and wood stake decomposition, respectively (Supplementary Figure 4).
We tested our conceptual models (Supplementary Figures 1–4) for aspen and pine wood stakes separately using a d-sep approach (Shipley, 2009; Lefcheck, 2016). We used the piecewiseSEM package (version 2.0.2; Lefcheck, 2016) in R 3.4.0 in which a structured set of linear models are fitted individually. This approach allowed us to account for the nested experimental design (Shipley, 2009; Lefcheck, 2016). We used the lme function of the nlme package (Pinheiro et al., 2021) to model response variables, including plots nested in sites as a random factors. For the mineral stakes we averaged the mass loss of wood blocks across soil depths (see methods described in “Wood stake incubation experiment”). However, we also calculated a model in which stake ID was included as an additional random effect. In this case all individual wood blocks per stake were included and provided a measure of decomposition at different mineral soil depth.
Results
As expected, wood stake decomposition differed considerably among the 44 sites (Supplementary Figure 6). Overall, location, time, wood type, and their two-way interactions explained 44% of the total variation in decomposition when controlling for site effects in a mixed effects model (Table 1). Stakes inserted into the mineral soil generally decomposed more (Figure 2A and Table 1) and faster (interaction between location * time; Figure 2C and Table 1) than stakes placed at the interface between the surface organic horizon and mineral soil, and stakes placed on the soil surface. As expected, aspen stakes had greater mass loss (Figure 2B and Table 1) over time than pine stakes (interaction between wood type * time) across our 44 forested sites (Figure 2D and Table 1).
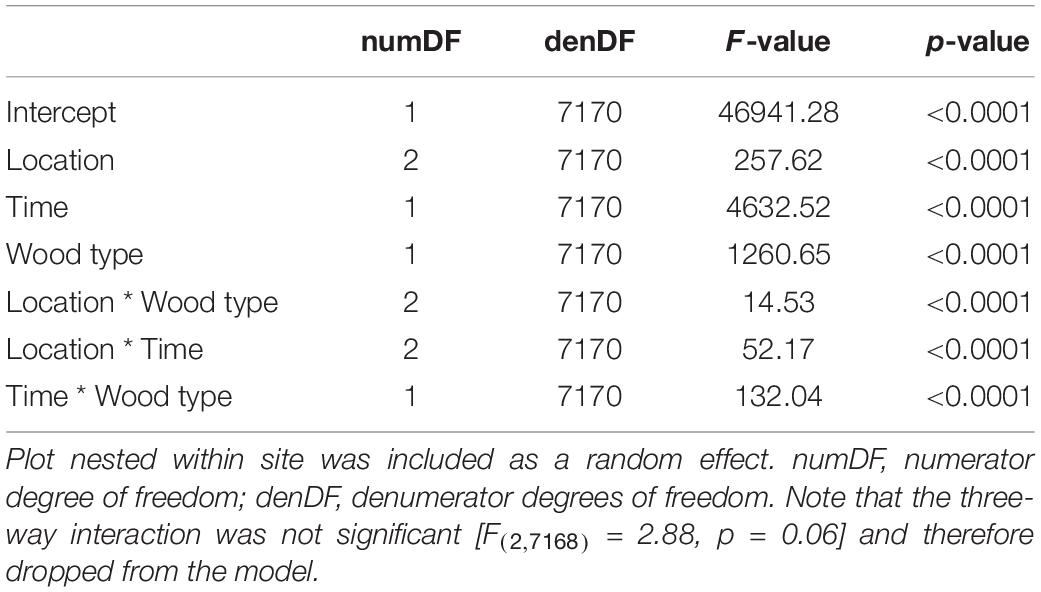
Table 1. Linear mixed effects model explaining wood decomposition based on incubation location (location), incubation time (time), wood type (wood type), and their interactions as fixed effects (R2 = 0.44, p < 0.0001).
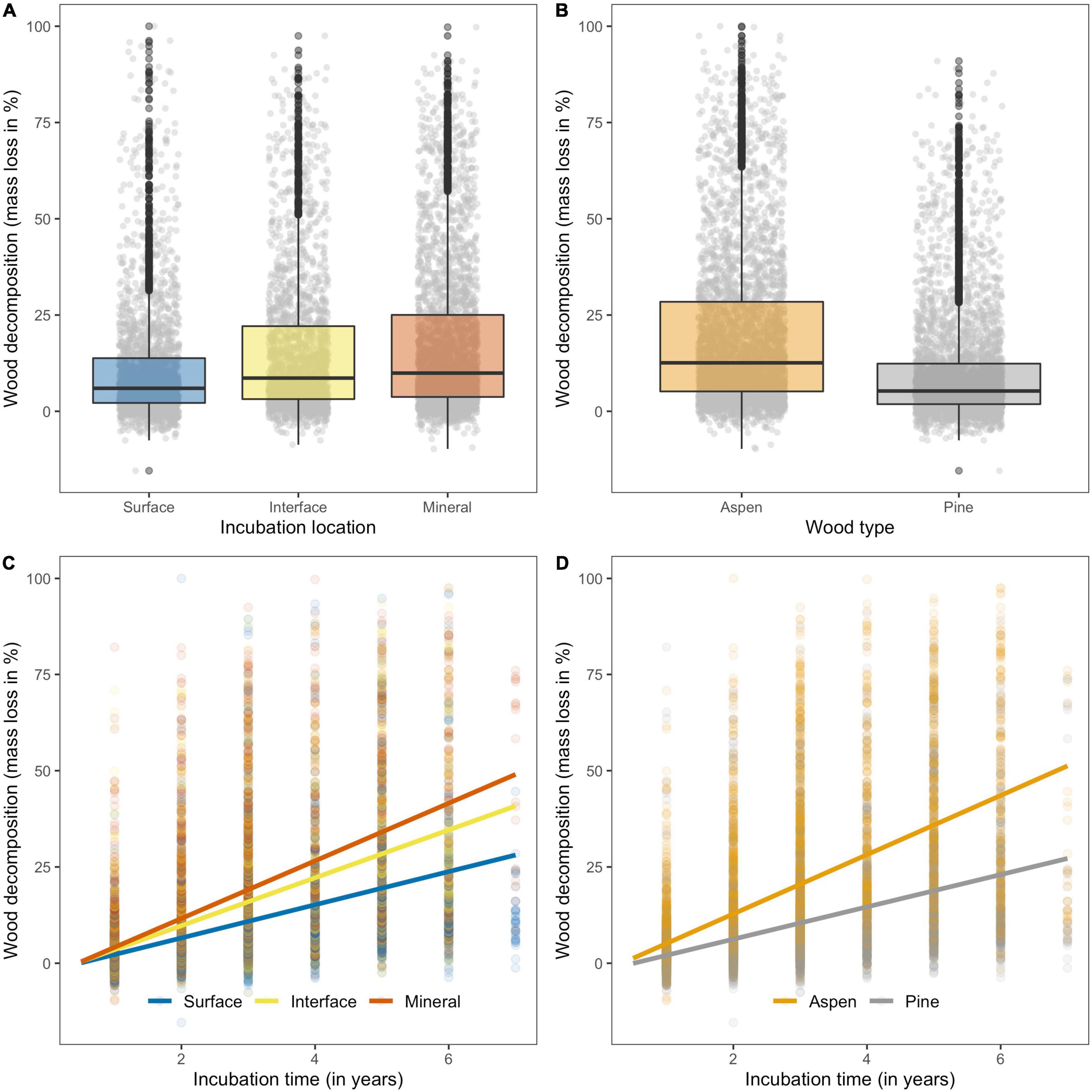
Figure 2. Effects of incubation location, wood type, and incubation time on wood decomposition (mass loss in %) across 44 forest sites located in the northern hemisphere. (A) Differences in decomposition of wood stakes placed on top of the surface organic horizon (surface; n = 2,337), the interface between the surface organic horizon and mineral soil (interface; n = 2,036) and in the mineral soil (mineral; n = 2,904). Both wood types (aspen and pine stakes) and all incubation times (1–7 years) were included. (B) Differences in decomposition between aspen (n = 3,583) and pine stakes (n = 3,694; all locations and times included). (C) Linear effects model (LMM, Table 1) predicting wood decomposition depending on incubation time for wood stakes placed on top of the surface organic horizon, the interface between the surface organic horizon and mineral soil (all locations and times included). (D) Linear effects model (LMM, Table 1) predicting wood decomposition of different wood types depending on incubation time (all locations and times included). Panels (A,B) represent boxplots of raw data showing the median (50th percentile), 25th and 75th percentile of the data across sites. Individual measures are shown in the background as dots. All statistical results can be found in Table 1.
Looking at wood stake decomposition from a processed-based perspective with our structural equation models (SEMs), our main model for surface aspen stakes explained 44% (marginal R2) of the variation in the decomposition across the 44 forest sites (Figure 3A). Air temperature (positive) and precipitation (negative) influenced the decomposition directly; hence, decomposition was higher at sites with higher air temperatures, but lower at sites with higher precipitation. Although we found no significant direct or indirect effect of the surface organic horizon C:N ratio on decomposition (Figure 3A), the C:N ratio contributed to the total explanatory power of the model. When removing the surface organic horizon C:N ratio completely (climate-only model), the total variation in decomposition explained dropped from 44 to just 1% (Supplementary Figure 7A). When replacing surface C:N ratio with surface C concentration in one of our alternative models, the total variation in decomposition explained dropped from 44 to 12% (Supplementary Figure 8A). Using AET instead of air temperature and precipitation also failed to explain decomposition differences in our surface aspen stakes (only 1 and 0.1% of variation explained; Supplementary Figures 7B, 8B). Neither our main nor alternative models explained any of the variation in surface pine stake decomposition (all marginal R2 values between 0.1 and 1%; Figure 3B and Supplementary Figures 7C,D, 8C,D).
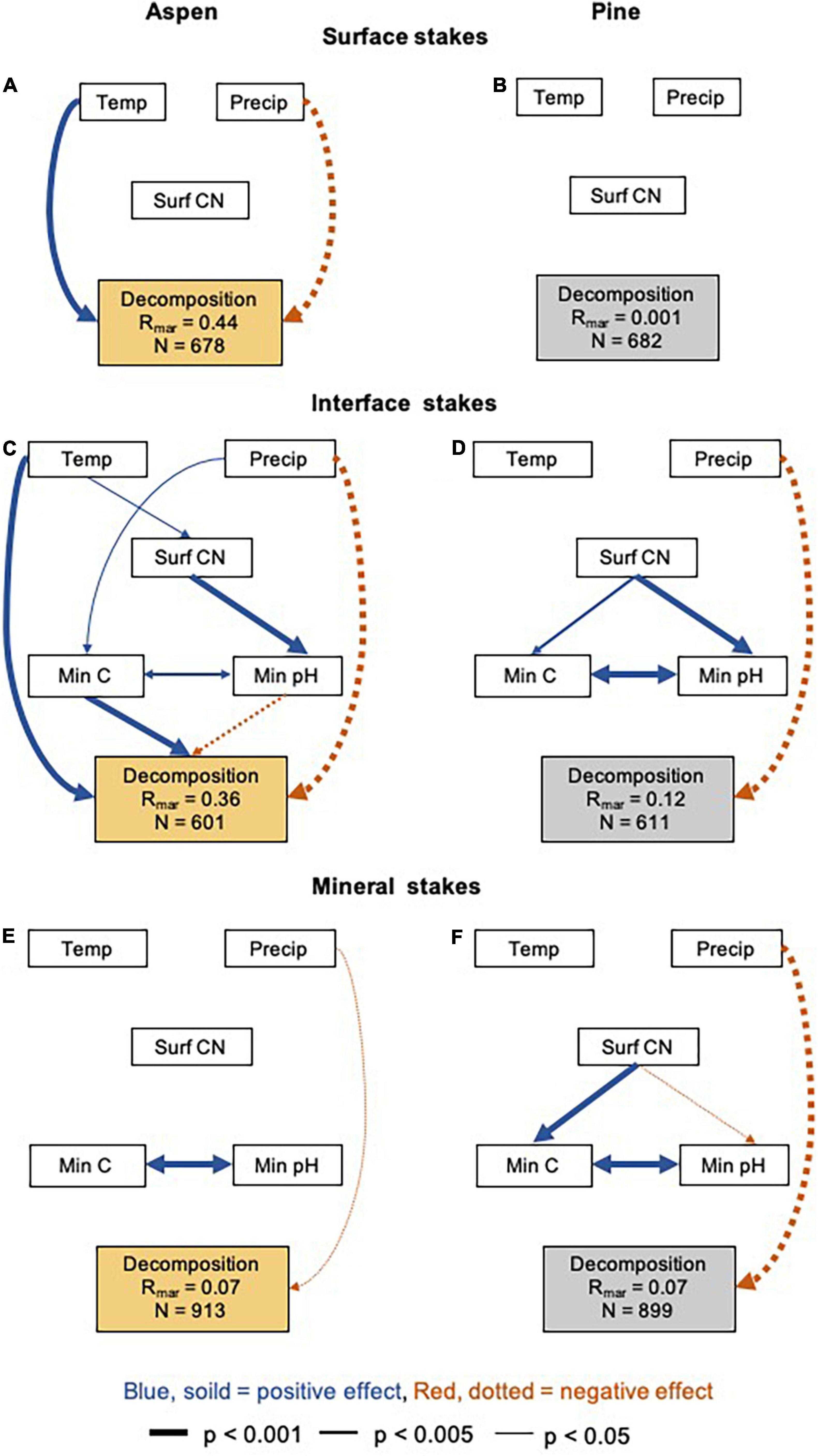
Figure 3. Influence of local environmental conditions on the wood decomposition (mass loss in %) in forested ecosystems. Structural equation model (SEM) diagrams illustrate climatic and soil properties found to affect decomposition of (A) surface aspen stakes, (B) surface pine stakes, (C) interface aspen stakes, (D) interface pine stakes, (E) mineral aspen stakes, (F) mineral pine stakes. Blue, solid lines represent positive relationships, red, dashed lines negative relationships. Line width corresponds to differences in significance levels (thick = p < 0.001, medium = p < 0.005, thin = p < 0.05). Non-significant connections were removed. Temp, average air temperature per site for the individual incubation time per wood stake; Precip, average precipitation per site for the individual incubation time per wood stake; Surf CN, surface organic horizon C:N ratio; Min C, mineral soil C concentration; Min pH, mineral soil pH. Decomposition values were log-transformed. Total number of sites included for surface aspen and pine = 19, interface aspen and pine = 10, mineral soil aspen and pine = 23. For alternative models see Supplementary Figures 7–10.
Our main SEM for aspen interface stakes explained 36% of the total variation in decomposition across our sites (Figure 3C). As with aspen surface stakes, air temperature had a positive effect on decomposition and precipitation a negative one. Air temperature was positively correlated with the surface organic horizon C:N ratio, which was positively associated with mineral soil pH. Soil pH, in turn, had a negative relationship with decomposition. Precipitation had a positive relationship with mineral soil C concentrations and a higher C concentration was positively related to decomposition of the interface aspen stakes across sites (Figure 3C). Again, replacing the surface organic horizon C:N ratio with C concentration in our alternative model resulted in less total variation in decomposition explained (31% instead of 36%; Supplementary Figure 9A). The explanatory power dropped even more for our other alternative models, i.e., including total soil bulk density (total amount of variation explained dropped from 36 to 25%; Supplementary Figure 9B) or replacing temperature and precipitation with AET (36–3%; Supplementary Figure 9C). The same was true for the climate-only models [from 36 to 3% (temperature/precipitation) and 36% to 0.1% (AET); Supplementary Figures 7E,F].
Our main SEM for interface pine stakes explained 12% of the total variation in decomposition (Figure 3D). Precipitation had a direct, negative relationship with decomposition rates. When we replaced the surface organic horizon C:N ratio with C concentration (Supplementary Figure 9D) or included BD as an additional predictor (Supplementary Figure 9E) in our alternative models, we explained a similar amount of variation (11 and 14% instead of 12%). When we replaced temperature and precipitation with AET (Supplementary Figure 9F) or when we calculated the climate-only models (Supplementary Figures 7G,H) we only explained 3, 1, and 0.1% of the variability in the decomposition of interface pine stakes, respectively.
The main SEMs for both aspen and pine mineral stakes explained only 7% of the total variation in wood stake decomposition (Figures 3E,F). Again, precipitation was directly and negatively related to decomposition. All our alternative models lead to small in- or decreases in the explanatory power compared to the main models (Supplementary Figures 10A–J): i.e., replacing surface organic horizon C:N ratio with C concentration (aspen: 7%, pine 4% of the variation explained; Supplementary Figures 10A,F), including mineral BD (aspen: 8%, pine 9%; Supplementary Figures 10B,G), replacing air temperature and precipitation with AET (aspen: 4%, pine 2%; Supplementary Figures 10C,H), replacing air temperature with soil temperature (aspen: 5%, pine 5%; Supplementary Figures 10D,I) or dropping the surface organic horizon C:N ratio from the models (aspen: 3%, pine 2%; Supplementary Figures 10E,J). Also, calculating models with only climate variables did not improve the prediction of mineral soil wood stake decomposition (aspen: 3% and 1%, pine: 2% and 0.1%; Supplementary Figures 7I–L). The same was true when we used the decomposition rates of individual wood blocks from different depths instead of averaging the individual values per stake (stake ID included as a random factor; results not shown).
Discussion
As we expected, our results showed that wood stakes incubated in the mineral soil decomposed faster than the stakes placed on the interface and the soil surface across our 44 sites. These findings confirm other results from local studies (Risch et al., 2013; Smyth et al., 2016; Page-Dumroese et al., 2019; Wang et al., 2019; Jurgensen et al., 2020). A similar result was found using tea bags across forest types in France (Fanin et al., 2020). Generally, at relatively dry sites, buried wood decomposes faster compared to wood placed on the soil surface as soil moisture conditions are more favorable for decomposition in the mineral soil compared to the soil surface (Busse, 1994; Brischke and Rapp, 2008; Smyth et al., 2016). In contrast, at cool or wet sites or at sites with compacted subsoils, buried wood can decompose slower than wood placed on the soil surface, as the buried wood might be saturated and conditions might become anaerobic (Smyth et al., 2016) or temperatures might be too cold to allow for microbial activity (Aerts, 2006). The latter most likely was the case for faster surface compared to mineral aspen and pine stake decomposition in our uncut boreal forest stands located in northern Finland (Finér et al., 2016).
As we expected, aspen stakes decomposed faster than pine stakes across all sites due to their lower wood density, lower lignin content, and higher N content as previously found for local-site studies (e.g., Risch et al., 2013; Finér et al., 2016; Page-Dumroese et al., 2019; Wang et al., 2019; Jurgensen et al., 2020). Decomposing aspen stakes also had lower fungal species richness than pine stakes (Wang et al., 2020), which also might explain their faster decomposition: general observations revealed that low fungal species richness generally is associated with rapid wood decomposition (Toljander et al., 2006; Fukami et al., 2010).
In our study, climatic conditions were stronger predictors of wood decomposition than soil properties, but climatic properties alone (climate only models) failed to explain decomposition (total amount of variation explained between 0.1 and 3% only). These findings contrast, to some extent, with Bradford et al. (2014), who suggested that local-scale soil properties or soil moisture data would be stronger predictors of soil biotic activity and wood decomposition than larger-scale climate properties, especially air temperature. This was not the case for the factors we included in our study, but the failure of our climate-only models to explain decomposition across our forest sites also points toward the strong effect of local site properties in controlling decomposition. Similar to Bradford et al. (2014), air temperature was not an important driver for surface (and interface) pine wood stake decomposition, but it was for our aspen stakes.
Even though we had similar numbers of data points for aspen and pine wood stakes, our SEMs explained decomposition of aspen surface and interface stakes better than the one of pine stakes (surface: 44 vs. 0.001% of variation explained with the model; interface: 36 vs. 12%), while explanation power was similarly low for mineral stakes of both wood types (7%). These findings indicate that we likely did not include the most important drivers of pine decomposition in our study. These missing properties could be the composition, abundance and diversity of the soil microbial community (Bani et al., 2018; Wang et al., 2020), macro-invertebrates such as termites, ants or other insects (Bradford et al., 2014; Jurgensen et al., 2020; Seibold et al., 2021) or the number of days above a threshold temperature for microbial activity (Page-Dumroese et al., 2019).
The performance for our two models for wood stake decomposition in the mineral soil was rather low, and further decreased when we replaced air with soil temperature. These findings contrast with findings in the Swiss Alps, where soil temperatures were much better predictors for decomposition than air temperature (Risch et al., 2013). However, it is worth noting that the soil temperature data we had available here originated from a global soil temperature data product (Lembrechts et al., 2022) that was highly correlated to air temperature (Supplementary Figure 11), and do not represent values obtained at each single site. If we would have had local-site soil temperature values we would still expect them to be better predictors than air temperature for decomposition rates in the mineral soil.
Despite the large number of wood stakes that decomposed under a wide range of soil and climatic conditions in our study, developing a universal model that explains wood stake decomposition remains challenging, especially for the mineral soil. However, the positive effect of air temperature and the negative effect of precipitation on decomposition remained relatively stable across our decomposition models, including the alternative models. Given the expected increases in temperature as a result of global climate change (IPCC, 2014, 2018), we would expect higher wood decomposition in all locations and, therefore, greater C fluxes from the soil to the atmosphere in the future (Davidson and Janssens, 2006; Bond-Lamberty et al., 2018). However, increased heavy precipitation events (Fischer and Knutti, 2014) might potentially counteract these C losses. If mineral soils remain saturated for longer periods of time, decomposition would likely decrease, especially at colder high-elevation or boreal sites. Our results make it clear that global models of OM decomposition need to also include local-site soil properties mentioned by Bradford et al. (2014), rather than only using readily available data of global air/soil temperature and precipitation. Ideally, such models would also include information on the composition and abundance of the soil micro- and macro-fauna as drivers of OM decomposition. The difficulties we had in explaining the variability in wood decomposition across the 44 northern hemisphere forest sites, highlight the need to continue assessing wood decomposition from local to regional and continental scales to better understand and predict C losses and gains in a changing world.
Data Availability Statement
The raw data supporting the conclusions of this article will be made available by the authors, without undue reservation. R code and data are availabe at github Doi: 10.5281/zenodo.6355363.
Author Contributions
MJ and DP-D developed the overall research idea, coordinated data collection, and laboratory analyses. AR developed and framed the research question. DP-D, MJ, AR, LF, MS, WW, TT, and MC collected the data. MH and JB assembled and calculated the climate data. AS, AR, and JB analyzed the data. AR wrote the manuscript with contributions from DP-D, MJ, AS, LF, MH, MS, WW, TT, MC, and YL. All authors contributed to the article and approved the submitted version.
Funding
Open access funding provided by Swiss Federal Institute for Forest, Snow and Landscape Research (WSL).
Conflict of Interest
The authors declare that the research was conducted in the absence of any commercial or financial relationships that could be construed as a potential conflict of interest.
Publisher’s Note
All claims expressed in this article are solely those of the authors and do not necessarily represent those of their affiliated organizations, or those of the publisher, the editors and the reviewers. Any product that may be evaluated in this article, or claim that may be made by its manufacturer, is not guaranteed or endorsed by the publisher.
Acknowledgments
We are grateful to Barbara Moser for help with climate data assembly and to Jonas Lembrechts for providing the soil temperature data. We thank Joanne Tirocke for processing and analyzing soil samples, and numerous employees at Michigan Technological University for processing the wood stakes. AS acknowledges the support of the Research Priority Program in Global Change and Biodiversity (URPP GCB) at the University of Zurich.
Supplementary Material
The Supplementary Material for this article can be found online at: https://www.frontiersin.org/articles/10.3389/ffgc.2022.829810/full#supplementary-material
Footnotes
References
Adams, M. B., Jurgensen, M., Palik, B., Miller, C., and Page-Dumroese, D. (2021). Wood stake decomposition twenty years after organic matter removal at the Lake States LTSP sites. Forest Ecol. Manag. 496:119456. doi: 10.1016/j.foreco.2021.119456
Aerts, R. (2006). The freezer defrosting: global warming and litter decomposition rates in cold biomes. J. Ecol. 94, 713–724. doi: 10.1111/j.1365-2745.2006.01142.x
Bani, A., Pioli, S., Ventura, M., Panzacchi, P., Borruso, L., Tognetti, R., et al. (2018). The role of microbial community in the decomposition of leaf litter and deadwood. Appl. Soil Ecol. 126, 75–84. doi: 10.1016/j.apsoil.2018.02.017
Berg, B. (2000). Litter decomposition and organic matter turnover in northern forest soils. Forest Ecol. Manag. 133, 13–22. doi: 10.1016/s0378-1127(99)00294-7
Bond-Lamberty, B., Bailey, V. L., Chen, M., Gough, C. M., and Vargas, R. (2018). Globally rising soil heterotrophic respiration over recent decades. Nature 560, 80–83. doi: 10.1038/s41586-018-0358-x
Bradford, M. A., Berg, B., Maynard, D. S., Wieder, W. R., and Wood, S. A. (2016). Understanding the dominant controls on litter decomposition. J. Ecol. 104, 229–238. doi: 10.1111/1365-2745.12507
Bradford, M. A., Warren Ii, R. J., Baldrian, P., Crowther, T. W., Maynard, D. S., et al. (2014). Climate fails to predict wood decomposition at regional scales. Nat. Clim. Change 4, 625–630. doi: 10.1038/nclimate2251
Brischke, C., and Rapp, A. O. (2008). Influence of wood moisture content and wood temperature on fungal decay in the field: observations in different micro-climates. Wood Sci. Tech. 42:663. doi: 10.1007/s00226-008-0190-9
Busse, M. D. (1994). Downed bole-wood decomposition in Lodgepole pine forests of Central Oregon. Soil Sci. Soc. Am. J. 58, 221–227. doi: 10.2136/sssaj1994.03615995005800010033x
Cornwell, W. K., Cornelissen, J. H. C., Allison, S. D., Bauhus, J., Eggleton, P., Preston, C. M., et al. (2009). Plant traits and wood fates across the globe: rotted, burned, or consumed? Glob. Chang. Biol. 15, 2431–2449. doi: 10.1111/j.1365-2486.2009.01916.x
Davidson, E. A., and Janssens, I. A. (2006). Temperature sensitivity of soil carbon decomposition and feedbacks to climate change. Nature 440, 165–173. doi: 10.1038/nature04514
Dixon, R. K., Brown, S., Houghton, R. A., Solomon, A. M., Trexler, M. C., and Wisniewski, J. (1994). Carbon Pools and Flux of Global Forest Ecosystems. Science 263, 185–190. doi: 10.1126/science.263.5144.185
Djukic, I., Kepfer-Rojas, S., Schmidt, I. K., Larsen, K. S., Beier, C., Berg, B., et al. (2018). Early stage litter decomposition across biomes. Sci. Tot. Env. 628-629, 1369–1394. doi: 10.1016/j.scitotenv.2018.01.012
Dormann, C. F., Elith, J., Bacher, S., Buchmann, C., Carl, G., Carré, G., et al. (2013). Collinearity: a review of methods to deal with it and a simulation study evaluating their performance. Ecography 36, 27–46. doi: 10.1111/j.1600-0587.2012.07348.x
Erdenebileg, E., Wang, C., Ye, X., Cui, Q., Du, J., Huang, Z., et al. (2020). Multiple abiotic and biotic drivers of long-term wood decomposition within and among species in the semi-arid inland dunes: a dual role for stem diameter. Funct. Ecol. 34, 1472–1484. doi: 10.1111/1365-2435.13559
Fanin, N., Bezaud, S., Sarneel, J. M., Cecchini, S., Nicolas, M., and Augusto, L. (2020). Relative Importance of Climate, Soil and Plant Functional Traits During the Early Decomposition Stage of Standardized Litter. Ecosystems 23, 1004–1018. doi: 10.1007/s10021-019-00452-z
Fanin, N., Kardol, P., Farrell, M., Kempel, A., Ciobanu, M., Nilsson, M.-C., et al. (2019). Effects of plant functional group removal on structure and function of soil communities across contrasting ecosystems. Ecol. Lett. 22, 1095–1103. doi: 10.1111/ele.13266
Federer, C. A., Turcotte, D. E., and Smith, C. T. (1993). The organic fraction–bulk density relationship and the expression of nutrient content in forest soils. Can. J. For. Res. 23, 1026–1032. doi: 10.1139/x93-131
Finér, L., Jurgensen, M., Palviainen, M., Piirainen, S., and Page-Dumroese, D. (2016). Does clear-cut harvesting accelerate initial wood decomposition? A five-year study with standard wood material. For. Ecol. Manag. 372, 10–18. doi: 10.1016/j.foreco.2016.03.060
Fischer, E. M., and Knutti, R. (2014). Detection of spatially aggregated changes in temperature and precipitation extremes. Geophys. Res. Lett. 41, 547–554. doi: 10.1002/2013gl058499
Fissore, C., Jurgensen, M. F., Pickens, J., Miller, C., Page-Dumroese, D., and Giardina, C. P. (2016). Role of soil texture, clay mineralogy, location, and temperature in coarse wood decomposition—a mesocosm experiment. Ecosphere 7:e01605.
Fukami, T., Dickie, I. A., Paula Wilkie, J., Paulus, B. C., Park, D., Roberts, A., et al. (2010). Assembly history dictates ecosystem functioning: evidence from wood decomposer communities. Ecol. Lett. 13, 675–684. doi: 10.1111/j.1461-0248.2010.01465.x
Gholz, H. L., Wedin, D. A., Smitherman, S. M., Harmon, M. E., and Parton, W. J. (2000). Long-term dynamics of pine and hardwood litter in contrasting environments: toward a global model of decomposition. Glob. Chang. Biol. 6, 751–765. doi: 10.1046/j.1365-2486.2000.00349.x
Goebel, M.-O., Bachman, J., Reichstein, M., Janssens, I. A., and Guggenberger, G. (2011). Soil water repellency and its implications for organic matter decomposition – is there a link to extreme climatic events? Glob. Chang. Biol. 17, 2640–2656. doi: 10.1111/j.1365-2486.2011.02414.x
González, G., Gould, W. A., Hudak, A. T., and Hollingsworth, T. N. (2008). Decay of aspen (Populus tremuloides Michx.) wood in moist and dry boreal, temperate, and tropical forest fragments. AMBIO 37:10. doi: 10.1579/0044-7447-37.7.588
Hu, Y., Yesilonis, I., and Szlavecz, K. (2021). Microbial and environmental controls on wood decomposition in deciduous forests of different ages. Appl. Soil Ecol. 166:103986. doi: 10.1016/j.apsoil.2021.103986
Hu, Z., Michaletz, S. T., Johnson, D. J., Mcdowell, N. G., Huang, Z., Zhou, X., et al. (2018). Traits drive global wood decomposition rates more than climate. Glob. Chang. Biol. 24, 5259–5269. doi: 10.1111/gcb.14357
IPCC (2014). Climate Change 2014: Synthesis Report. Contribution of Working Groups I, II and III to the Fifth Assessment Report of the Intergovernmental Panel on Climate Change. Geneva: IPCC.
IPCC (2018). “Summary for policymakers,” in Global Warming of 1.5°C. An IPCC Special Report on the impacts of global warming of 1.5°C above pre-industrial levels and related global greenhouse gas emission pathways, in the context of strengthening the global response to the threat of climate change, eds V. MASSON-DELMOTTE, P. ZHAI, H.-O. PÖRTNER, D. ROBERTS, J. SKEA, P. R. SHAKULA, et al. (Geneva: World Meteorological Organization).
Jackson, R. B., Lajtha, K., Crow, S. E., Hugelius, G., Kramer, M. G., and Piñeiro, G. (2017). The Ecology of Soil Carbon: Pools, Vulnerabilities, and Biotic and Abiotic Controls. Annu. Rev. Ecol. Evol. Syst. 48, 419–445. doi: 10.1146/annurev-ecolsys-112414-054234
Juhos, K., Madarász, B., Kotroczó, Z., Béni, A., Makádi, M., and Fekete, I. (2021). Carbon sequestration of forest soils is reflected by changes in physicochemical soil indicators - A comprehensive discussion of a long-term experiment on a detritus manipulation. Geoder. 385:114918. doi: 10.1016/j.geoderma.2020.114918
Jurgensen, M. F., Miller, C. A., and Page-Dumroese, D. S. (2020). Wood decomposition after an aerial application of hydromulch following wildfire in a Southern California chaparral shrubland. Front. For. Glob. Change 2020:3.
Jurgensen, M. F., Reed, D. D., Page-Dumroese, D. S., Laks, P., Collins, A., Mroz, G., et al. (2006). Wood strength loss as a measure of decomposition in northern forest mineral soil. Eur. J. Soil Biol. 42, 23–31. doi: 10.1016/j.ejsobi.2005.09.001
Karger, D. N., Conrad, O., Böhner, J., Kawohl, T., Kreft, H., Soria-Auza, R. W., et al. (2017). Climatologies at high resolution for the earth’s land surface areas. Sci. Data 4, 170122–170122.
Karger, D. N., Conrad, O., Böhner, J., Kawohl, T., Kreft, H., Soria-Auza, R. W., et al. (2018). Data from: Climatologies at high resolution for the earth’s land surface areas. Dryad 2018:4. doi: 10.5061/dryad.kd1d4
Keuskamp, J. A., Dingemans, B. J. J., Lehtinen, T., Sarneel, J. M., and Hefting, M. M. (2013). Tea Bag Index: a novel approach to collect uniform decomposition data across ecosystems. Methods Ecol. Evol. 4, 1070–1075. doi: 10.1111/2041-210x.12097
Kielak, A. M., Scheublin, T. R., Mendes, L. W., Van Veen, J. A., and Kuramae, E. E. (2016). Bacterial community succession in pine-wood decomposition. Front. Microb. 2016:7. doi: 10.3389/fmicb.2016.00231
Latter, P. M., and Howson, G. (1977). The use of cotton strips to indicate cellulose decomposition in the field. Pedobiologia 1977, 145–155.
Latter, P. M., and Walton, D. W. H. (1988). “The cotton strip assay for cellulose decomposition studies in soil: history of the assay and development,” in ITE Symposium, Institute of Terrestrial Ecology, (Natural Environment Research Council).
Lefcheck, J. S. (2016). piecewiseSEM: piecewise structural equation modelling in R for ecology, evolution, and systematics. Methods Ecol. Evol. 7, 573–579. doi: 10.1111/2041-210x.12512
Lembrechts, J. J., Van Den Hoogen, J., Aalto, J., Ashcroft, M. B., De Frenne, P., Kemppinen, J., et al. (2022). Global maps of soil temperature. Glob. Chang. Biol. 2022:16060. doi: 10.1111/gcb.16060
Liu, G., Cornwell, W. K., Cao, K., Hu, Y., Van Logtestijn, R. S. P., Yang, S., et al. (2015). Termites amplify the effects of wood traits on decomposition rates among multiple bamboo and dicot woody species. J. Ecol. 103, 1214–1223. doi: 10.1111/1365-2745.12427
Lustenhouwer, N., Maynard, D. S., Bradford, M. A., Lindner, D. L., Oberle, B., Zanne, A. E., et al. (2020). A trait-based understanding of wood decomposition by fungi. Proc. Natl. Acad. Sci. 117, 11551–11558. doi: 10.1073/pnas.1909166117
Mackenzie, M. D., Deluca, T. H., and Sala, A. (2006). Fire exclusion and nitrogen mineralization in low elevation forests of western Montana. Soil Biol. Biochem. 38, 952–961. doi: 10.1016/j.soilbio.2005.08.008
Magnússon, R. I, Tietema, A., Cornelissen, J. H. C., Hefting, M. M., and Kalbitz, K. (2016). Tamm Review: sequestration of carbon from coarse woody debris in forest soils. For. Ecol. Manag. 377, 1–15. doi: 10.1016/j.foreco.2016.06.033
Mayer, M., Prescott, C. E., Abaker, W. E. A., Augusto, L., Cécillon, L., Ferreira, G. W. D., et al. (2020). Tamm Review: influence of forest management activities on soil organic carbon stocks: a knowledge synthesis. For. Ecol. Manag. 466:118127. doi: 10.1016/j.foreco.2020.118127
Meentemeyer, V. (1978). Macroclimate and Lignin Control of Litter Decomposition Rates. Ecology 59, 465–472. doi: 10.2307/1936576
Moroni, M. T., Morris, D. M., Shaw, C., Stokland, J. N., Harmon, M. E., Fenton, N. J., et al. (2015). Buried Wood: A Common Yet Poorly Documented Form of Deadwood. Ecosystems 18, 605–628. doi: 10.1007/s10021-015-9850-4
Nave, L., Marín-Spiotta, E., Ontl, T., Peters, M., and Swanston, C. (2019). “Chapter 11 - Soil carbon management,” in Developments in Soil Science, eds M. Busse, C. P. Giardina, D. M. Morris, and D. S. Page-Dumroese (Amsterdam: Elsevier).
Oberle, B., Lee, M. R., Myers, J. A., Osazuwa-Peters, O. L., Spasojevic, M. J., Walton, M. L., et al. (2020). Accurate forest projections require long-term wood decay experiments because plant trait effects change through time. Glob. Chang. Biol. 26, 864–875. doi: 10.1111/gcb.14873
Page-Dumroese, D. S., Brown, R. E., Jurgensen, M. F., and Mroz, G. D. (1999). Comparison of methods for determining bulk densities of rocky forest soils. Soil Sci. Soc. Am. J. 63, 379–383. doi: 10.2136/sssaj1999.03615995006300020016x
Page-Dumroese, D. S., Jurgensen, M. F., Miller, C. A., Busse, M. D., Curran, M. P., Terry, T. A., et al. (2021). Decomposition of wood stakes in the Pacific Northwest after soil compaction and organic matter removal. For. Ecol. Manag. 494:119362. doi: 10.1016/j.foreco.2021.119362
Page-Dumroese, D. S., Jurgensen, M. F., Miller, C. A., Pickens, J. B., and Tirocke, J. M. (2019). Wildfire alters belowground and surface wood decomposition on two national forests in Montana, USA. Internat. J. Wildland Fire 28, 456–469. doi: 10.1071/wf18218
Pan, Y., Birdsey, R. A., Fang, J., Houghton, R., Kauppi, P. E., Kurz, W. A., et al. (2011). A Large and Persistent Carbon Sink in the World’s Forests. Science 333, 988L–993. doi: 10.1126/science.1201609
Parton, W., Silver, W. L., Burke, I. C., Grassens, L., Harmon, M. E., Currie, W. S., et al. (2007). Global-Scale Similarities in Nitrogen Release Patterns During Long-Term Decomposition. Science 315, 361–364. doi: 10.1126/science.1134853
Pinheiro, J., Bates, D., Debroy, S., and Sarkar, D. (2021). nlme: Linear and nonlinear mixed effect models. R package version 3.1-153.
Reed, D., Breymeyer, A., Degorski, M., and Noble, R. (2003). Scots pine (Pinus sylvestris) ecosystem response to climate on a temperate to boreal forest transect: Project description. Polish J. Ecol. 51, 399–401.
Risch, A. C., Jurgensen, M. F., Page-Dumroese, D. S., and Schütz, M. (2013). Initial turnover rates of two standard wood substrates following land-use change in subalpine ecosystems in the Swiss Alps. Can. J. For. Res. 43, 901–910. doi: 10.1139/cjfr-2013-0109
Ruehlmann, J., and Körschens, M. (2009). Calculating the effect of soil organic matter concentration on soil bulk density. Soil Sci. Soc. Am. J. 73, 876–885. doi: 10.1371/journal.pone.0215223
Russell, M. B., Fraver, S., Aakala, T., Gove, J. H., Woodall, C. W., D’amato, A. W., et al. (2015). Quantifying carbon stores and decomposition in dead wood: a review. For. Ecol. Manag. 350, 107–128. doi: 10.1016/j.foreco.2015.04.033
Schimel, J. P. (1995). Terrestrial ecosystems and the carbon cycle. Glob. Chang. Biol. 1, 77–91. doi: 10.1111/j.1365-2486.1995.tb00008.x
Scott, N. A., and Binkley, D. (1997). Foliage litter quality and annual net N mineralization: comparison across North American forest sites. Oecologia 111, 151–159. doi: 10.1007/s004420050219
Seibold, S., Rammer, W., Hothorn, T., Seidl, R., Ulyshen, M. D., Lorz, J., et al. (2021). The contribution of insects to global forest deadwood decomposition. Nature 597, 77–81. doi: 10.1038/s41586-021-03740-8
Shipley, B. (2009). Confirmatory path analysis in a generalized multilevel context. Ecology 90, 363–368. doi: 10.1890/08-1034.1
Smyth, C. E., Kurz, W. A., and Trofymow, J. A. (2011). Including the effects of water stress on decomposition in the Carbon Budget Model of the Canadian Forest Sector CBM-CFS3. Ecol. Mod. 222, 1080–1091. doi: 10.1016/j.ecolmodel.2010.12.005
Smyth, C. E., Titus, B., Trofymow, J. A., Moore, T. R., Preston, C. M., Prescott, C. E., et al. (2016). Patterns of carbon, nitrogen and phosphorus dynamics in decomposing wood blocks in Canadian forests. Plant Soil 409, 459–477. doi: 10.1007/s11104-016-2972-4
Talbot, J. M., and Treseder, K. K. (2012). Interactions among lignin, cellulose, and nitrogen drive litter chemistry–decay relationships. Ecology 93, 345–354. doi: 10.1890/11-0843.1
Toljander, Y. K., Lindahl, B. D., Holmer, L., and Högberg, N. O. S. (2006). Environmental fluctuations facilitate species co-existence and increase decomposition in communities of wood decay fungi. Oecologia 148, 625–631. doi: 10.1007/s00442-006-0406-3
Tóth, J. A., Lajtha, K., Kotroczó, Z., Krakomperger, Z., Caldwell, B., Bowden, R., et al. (2007). The effect of climate change on soil organic matter decomposition. Acta Silvatica et Lignaria Hungarica 3, 75–85.
Trofymow, J. A. (1998). Detrital carbon fluxes and microbial activity in successional Douglas-fir forests. Northwest Sci. 72, 51–53.
Van Der Wal, A., De Boer, W., Smant, W., and Van Veen, J. A. (2007). Initial decay of woody fragments in soil is influenced by size, vertical position, nitrogen availability and soil origin. Plant Soil 301, 189–201. doi: 10.1007/s11104-007-9437-8
Wang, W., Lindner, D., Jusino, M. A., Page-Dumroese, D. S., Palmer, J. M., Jurgensen, M. F., et al. (2020). Wood-colonizing fungal community response to forest restoration thinnings in a Pinus tabuliformis plantation in northern China. For. Ecol. Manag. 476:118459. doi: 10.1016/j.foreco.2020.118459
Wang, W., Page-Dumroese, D., Jurgensen, M., Miller, C., Walitalo, J., Chen, X., et al. (2019). Restoration thinning impacts surface and belowground wood decomposition. Forest Ecol. Manag. 449:117451. doi: 10.1016/j.foreco.2019.117451
Wang, W., Page-Dumroese, D., Jurgensen, M., Tirocke, J., and Liu, Y. (2018). Effect of forest thinning and wood quality on the short-term wood decomposition rate in a Pinus tabuliformis plantation. J. Plant Res. 131, 897–905. doi: 10.1007/s10265-018-1069-y
Weedon, J. T., Cornwell, W. K., Cornelissen, J. H. C., Zanne, A. E., Wirth, C., and Coomes, D. A. (2009). Global meta-analysis of wood decomposition rates: a role for trait variation among tree species? Ecol. Lett. 12, 45–56. doi: 10.1111/j.1461-0248.2008.01259.x
Wei, X., Shao, M., Gale, W., and Li, L. (2014). Global pattern of soil carbon losses due to the conversion of forests to agricultural land. Sci. Rep. 4:4062. doi: 10.1038/srep04062
Wiesmeier, M., Urbanski, L., Hobley, E., Lang, B., Von Lützow, M., Marin-Spiotta, E., et al. (2019). Soil organic carbon storage as a key function of soils - A review of drivers and indicators at various scales. Geoderma 333, 149–162. doi: 10.1016/j.geoderma.2018.07.026
Woodward, B. M., Hatfield, C. A., and Lebow, S. T. (2011). Comparison of wood preservatives in stake tests : 2011 progress report. Madison, WI: U.S. Dept. of Agriculture, Forest Service.
Zhang, X., and Wang, W. (2015). The decomposition of fine and coarse roots: their global patterns and controlling factors. Sci. Rep. 5:9940. doi: 10.1038/srep09940
Keywords: climate properties, soil properties, aspen, pine, incubation location, in-situ incubation, mass loss, unharvested forest stands
Citation: Risch AC, Page-Dumroese DS, Schweiger AK, Beattie JR, Curran MP, Finér L, Hyslop MD, Liu Y, Schütz M, Terry TA, Wang W and Jurgensen MF (2022) Controls of Initial Wood Decomposition on and in Forest Soils Using Standard Material. Front. For. Glob. Change 5:829810. doi: 10.3389/ffgc.2022.829810
Received: 06 December 2021; Accepted: 04 March 2022;
Published: 31 March 2022.
Edited by:
Andreas Schindlbacher, Austrian Research Centre for Forests (BFW), AustriaReviewed by:
Werner Borken, University of Bayreuth, GermanyLaurent Augusto, INRA Centre Bordeaux-Aquitaine, France
Copyright © 2022 Risch, Page-Dumroese, Schweiger, Beattie, Curran, Finér, Hyslop, Liu, Schütz, Terry, Wang and Jurgensen. This is an open-access article distributed under the terms of the Creative Commons Attribution License (CC BY). The use, distribution or reproduction in other forums is permitted, provided the original author(s) and the copyright owner(s) are credited and that the original publication in this journal is cited, in accordance with accepted academic practice. No use, distribution or reproduction is permitted which does not comply with these terms.
*Correspondence: Anita C. Risch, YW5pdGEucmlzY2hAd3NsLmNo