- 1Departamento de Botânica, Instituto de Biociências, Universidade de São Paulo, São Paulo, Brazil
- 2Instituto Nacional de Pesquisas da Amazônia, Manaus, Brazil
- 3Royal Botanic Gardens, Kew, Kew Green, Richmond, United Kingdom
- 4Departamento de Biologia, Universidade Federal do Amazonas, Manaus, Brazil
- 5Instituto de Pesquisa Ambiental da Amazônia, Canarana, Brazil
- 6Centro de Ciências Naturais e Humanas, Universidade Federal do ABC, São Bernardo do Campo, Brazil
Plant distribution patterns may indicate habitat specialization either by closely related species with conserved traits or by phylogenetically distant species with converging traits. Lianas represent a large proportion of the overall tropical species diversity and abundance. Despite their importance, little is known about the relationship between habitat specialization and the phylogenetic structure of lianas, especially at the landscape scale where forest disturbances and hydro-edaphic gradients are crucial. To explore this knowledge gap, we used one of the most diverse lineages of Neotropical lianas to test whether (i) landscape environmental gradients explain liana species diversity and composition, (ii) habitat specialization is phylogenetically conserved along ecological gradients, and (iii) closely related liana species have more similar distribution patterns. We hypothesized that hydro-edaphic and forest disturbance gradients determine the compartmentalization of a subset of closely related species in different portions of the ecological gradients. To test our hypothesis, we examined the distribution of the tribe Bignonieae on 34 1-ha permanent plots systematically distributed over a 42 km2 forested landscape area in Central Amazon. We used proxies for the hydro-edaphic, forest disturbance, and soil nutrient gradients. Liana diversity increased along the hydro-edaphic gradient (i.e., toward dry plateaus), but slightly decreased along the forest disturbance gradient. Further, we found evidence of habitat specialization along the hydro-edaphic gradient on plateaus with deeper water tables, where liana assemblages are subsets of closely related species, exhibiting phylogenetic clustering. The opposite pattern was found on valleys, where liana assemblages were phylogenetically overdispersed. Our results support the role of phylogenetic niche conservatism on plateaus and a stronger environmental filter within the hydrologically dynamic valleys, associated with a functional convergence of more distantly related species. The selection of more distantly related species on hydrologically dynamic areas is a general pattern among trees, palms and now lianas. We conclude that ecological filters and phylogenetic history have played fundamental roles in structuring liana assemblages unevenly at the landscape scale. Fine-scale hydrology determines several aspects of plant community organization, whose mechanisms need to be experimentally investigated in the Amazon basin.
Introduction
Patterns of species distribution and richness along environmental gradients have intrigued ecologists and evolutionary biologists for a long time (Darwin, 1859; Hawkins, 2001; Hawkins and Diniz-Filho, 2004; Ricklefs, 2005). During the past decades, several studies have described plant species distribution patterns at different spatial scales in tropical forests (Soares-Filho et al., 2006; Laurance et al., 2011; Bullock et al., 2020). On a regional scale, the Amazon basin includes a variety of forest types, such as terra firme forests, floodplain/inundated forests, and white-sand areas. The broad environmental heterogeneity between these vegetation types contributes to high basin-wide species richness and high turnover in plant species composition (Gentry, 1988; Fine et al., 2005). Within vegetation types, topographic and soil heterogeneity drives species richness and species turnover due to specific abiotic conditions and habitat-specific biotic interactions favoring the survival, growth, and reproduction of species subsets (Pitman et al., 2001; Schietti et al., 2014; Tuomisto et al., 2017). Overall, the environmental heterogeneity of tropical forests can promote ecologically mediated speciation and habitat specialization (Fine et al., 2005; Fine and Kembel, 2011). However, habitat specialization can also be shuffled by stochastic events, such as blowdowns (Bordon et al., 2019), and constrained by the evolutionary history of plant lineages (Webb and Peart, 1999; Webb et al., 2002; Graham and Fine, 2008), determining the current diversity patterns found in tropical forests.
From an eco-evolutionary perspective, a major challenge to be investigated is whether habitats filter species subsets by their traits because of their common recent history. It has long been held that the most closely related species should have greater ecological similarity due to the common ancestry (Darwin, 1859; Ricklefs and Schluter, 1993; Webb, 2000; Wiens and Graham, 2005). Therefore, if shared traits enhance fitness in particular environments, then environmental filters could generate local communities composed of closely related species, creating a pattern of phylogenetic clustering. On the other hand, interspecific competition and character displacement, or convergent evolution mediated by extreme environmental conditions, could lead to an assemblage structure made up of local groupings of distantly related species, thereby creating a pattern of phylogenetic overdispersion (Webb et al., 2002; Wiens and Donoghue, 2004; Cavender-Bares et al., 2009).
Phylogenetic patterns of habitat specialization have often been described for tree and palm assemblages in tropical forests (Fine and Kembel, 2011; Eiserhardt et al., 2013; Freitas et al., 2014). However, the prevalence of phylogenetic clustering or overdispersion cannot be generalized. For example, Amazonian tree and palm assemblages were shown to be formed by more closely related species than expected by chance within terra-firme, floodplain, and pre-montane forests (Fine and Kembel, 2011; Eiserhardt et al., 2013; Muscarella et al., 2019), indicating a pattern of phylogenetic clustering (synonym of phylogenetic niche conservatism here; Webb et al., 2002). This pattern has been explained by environmental filtering under hydro-edaphic conditions of each habitat and limited seed dispersal across habitats (Fine and Kembel, 2011; Eiserhardt et al., 2013; Muscarella et al., 2019). On the other hand, Amazonian tree and palm assemblages on more stressful restinga and white-sand vegetation are composed of more distantly related species (Fine and Kembel, 2011; Eiserhardt et al., 2013; Muscarella et al., 2019). Moreover, palm assemblages of terra firme forests shift their phylogenetic structure from overdispersion in the white-sand valleys to a phylogenetically random structure toward upland plateaus (Freitas et al., 2014). Plant assemblages composed of more distantly related species have been associated with the action of environmental filters, convergent plant evolution, and, less likely, by competitive exclusion between ecologically similar close relatives (Eiserhardt et al., 2013; Freitas et al., 2014). Therefore, while some habitats are composed of more closely related plant species, others are composed of more distantly related species. Notably, both patterns are shared by trees and palms throughout the Amazon Forest landscape (Supplementary Table 1).
Few studies have focused on the diversity patterns and drivers of assemblage structure of neglected plant habits, such as lianas, even though these plants represent a large proportion of the overall tropical species diversity and abundance (Putz and Mooney, 1991; Schnitzer and Bongers, 2002; Burnham, 2004; Schnitzer, 2018). While studies of trees and palms have shown that environmental filtering generates edaphic specialization patterns (Fine et al., 2005; Fine and Kembel, 2011; Zuquim et al., 2012; Oliveira et al., 2014), it remains unclear whether lianas also show specialization to portions of gradients driven by forest disturbance, soil nutrients, and water availability. Moreover, we do not know if such specialization in lianas is due to the filtering of functional traits, allowing plant survival and reproduction in habitats with extreme environmental conditions (resulting in phylogenetic overdispersion), or from a historical legacy owing to trait conservatism within each environment (resulting in phylogenetic clustering). To address these unknowns, we investigated the relationship between habitat specialization and the phylogenetic structure of liana assemblages on 34 1-ha plots distributed over the landscape scale (∼42 km2) of Reserva Ducke in Central Amazon, where forest disturbances and hydro-edaphic gradients are crucial to species distribution (Toledo et al., 2012; Schietti et al., 2014). We used one of the most diverse lineages of Neotropical lianas (Bignonieae tribe, Bignoniaceae), a clade that shows species composition patterns that are congruent with those documented for other plant and animal groups (Landeiro et al., 2012; Schietti et al., 2014) and the whole liana assemblage in Central Amazonia (Rocha et al., 2021). We tested whether (i) landscape environmental gradients, such as soil water condition, forest disturbance, and soil fertility, explain the patterns of liana species diversity and composition; (ii) habitat specialization is associated with a pattern of phylogenetically clustering along these ecological gradients, and (iii) closely related liana species have more similar distribution patterns than expected by chance. We hypothesized that the three independent factors–soil water condition, forest disturbance, and soil nutrients gradients–determine the compartmentalization of subsets of more closely related species within different portions of ecological gradients. Although specialization and phylogenetic structure within habitats do not allow straightforward generalizations, the clustering pattern has been the more frequently reported for other Amazonian plant assemblages (e.g., Fine and Kembel, 2011; Eiserhardt et al., 2013; Muscarella et al., 2019) under a large set of explanatory processes (Webb, 2000; Cavender-Bares et al., 2009; Vamosi et al., 2009). Under this scenario, the most likely explanation would be phylogenetic clustering linked to environmental filtering as a basis for habitat specialization.
Materials and Methods
Study Area and Liana Sampling Design
This study was conducted at Ducke Reserve managed by the National Institute of Amazonian Research (INPA), located 26 km northwest of Manaus (02°55′S, 59°59′W at reserve headquarters) in the state of Amazonas, Brazil. The Reserve houses 10,000 ha of lowland tropical terra firme rainforest. The vegetation is characterized by a closed canopy 30–37 m tall, but with emergent trees up to 45 m high and generally low light levels in the understory (Guillaumet and Kahn, 1982; Ribeiro et al., 1999; Oliveira et al., 2008). The soils represent a continuum from clayey latosols in the highest flat plateaus to increasing sand content on the slopes and turning into pure sand on valley bottoms (Chauvel et al., 1987; Mertens, 2004). The mean annual temperature is around 26°C, and the mean annual precipitation is 2,300 mm. The rainy season occurs from October to June and the dry season from July to September when rainfall is generally < 100 mm.month–1 (Marques Filho et al., 1981).
The Reserve has a 64 km2 grid of trails established by the LTER-Brazil Program (Long-term Ecological Research) and PPBio (the Brazilian Biodiversity Research Program). Our liana inventory was conducted in 34 plots systematically distributed over the landscape of 42 km2 with plots at least 1 km distant from each other. Each permanent plot was 250 m long and 40 m wide (1 ha), following the altitudinal contour (see Costa et al., 2005 and Costa and Magnusson, 2010, for further sampling design details). This sampling design aimed to reduce internal variation in the hydro-edaphic features of each plot (Costa and Magnusson, 2010). From August 2004 to October 2005, every individual liana stem rooted inside the plot with >1 cm in diameter at 130 cm from the rooting point was tagged and then counted and identified. We measured the stem diameter according to Gerwing et al. (2006). For lianas with non-cylindrical stems, we measured the minimum section (Smin) and maximum section (Smax) and applied the equation to obtain a corrected estimate of stem diameter. Lianas of the tribe Bignonieae (from now on called Bignonieae lianas) were identified at the species level in the study area by A. Nogueira, L. G. Lohmann and A. R. Zuntini. Bignonieae lianas represent ∼25% of Ducke’s lianas species and are the most diverse lineage of lianas at Ducke reserve (Ribeiro et al., 1999; Costa et al., 2008). All lianas with a diameter (D) ≥ 5 cm were sampled in 1 ha (40 × 250 m), and lianas with 1 cm ≤ D ≤ 4.9 cm were subsampled in 0.25 ha (10 × 250 m) (Nogueira et al., 2011). The botanical nomenclature of all Bignonieae liana species follows Lohmann et al. (2020). For each species, liana density was expressed as the number of stems per hectare. Liana plots inventory is available in the data repository at ppbio.inpa.gov.br/en/DataBank and ForestPlots.net.
Hydro-Edaphic Gradient, Tree Turnover, and Soil Phosphorus
Several organisms, including different plant groups, have a concordant pattern of species turnover along the edaphic and topographic gradients in Central Amazonia (Landeiro et al., 2012). Variation of height above the nearest drainage (HAND) appears to be the most robust available metric describing a hydro-edaphic gradient at Ducke Reserve (Schietti et al., 2014). Therefore, we used HAND to represent one of the environmental gradients at Ducke Reserve. HAND describes the vertical height to the nearest drainage of each plot, which is an indirect estimate of the distance to the water table derived from a Shuttle Radar Topography Mission (SRTM) digital elevation model (DEM; Rennó et al., 2008; Schietti et al., 2014). Plots with high HAND values are vertically far from the water table and have clayey soils (plateaus) at Ducke Reserve, while plots with low HAND values are in lowland areas closer to the water table and have sandy soils (valleys). The HAND gradient varied from 1.4 to 52.5 m across the sampled plots. At the Ducke Reserve, HAND describes both soil texture and hydrological aspects associated with the topography, generating a strong local hydro-edaphic gradient, given the convergence of water toward the lower sandy grounds predominantly through groundwater flow (Fan et al., 2013; Schietti et al., 2014).
At Ducke Reserve, the HAND gradient is also positively correlated with the concentration of exchangeable bases (sum of bases–sodium, magnesium, potassium, and calcium) and nitrogen, but not with soil phosphorus (P; mg.kg–1) concentration, commonly scarce and nutritional restrictive for plants in the Amazon basin (Dalling et al., 2016). The soil phosphorus is a macronutrient essential for energy storage and metabolic reactions in plants (Jennifer et al., 2013; Malhotra et al., 2018). Therefore, we used the phosphorus concentration (data from Castilho et al., 2006) as another environmental gradient at Ducke Reserve. In each plot, six superficial soil samples (0–5 cm deep) were collected at 50-m intervals, mixed into a composite sample, dried at 105°C, sieved (2 mm mesh size), and analyzed at the INPA Soil Laboratory (Castilho et al., 2006). The soil phosphorus gradient varied from 0.77 to 6.71 mg.kg–1 across the sampled plots.
Additionally, lianas use trees as supports to reach the canopy; therefore, changes in the dynamics of trees can correspondingly affect the dynamics of lianas (Schnitzer and Bongers, 2002). Thus, estimates of tree turnover per plot (average of annual mortality and recruitment rates) were calculated using the data from Castilho et al. (2010), considering the total number of dead and recruited trees with diameter at breast height (DBH) > 1 cm for 2 years. We used tree censuses undertaken between 2001/2003 and 2003/2005 to calculate the average of annualized rates of tree mortality and recruitment (Phillips and Gentry, 1994). These data were collected in the same 34 permanent plots where we sampled Bignonieae lianas. Plot width sampled for trees varied according to size class. More specifically, trees with DBH ≥ 30 cm were sampled at 40 × 250 m (1 ha). Trees with DBH ≥ 10 cm were sub-sampled in 20 × 250 m plots (0.5 ha), and trees with DBH ≥ 1 cm were sampled in 4 × 250 m plots (0.1 ha). Tree turnover rate calculations were standardized for the 1 ha area (Castilho et al., 2006), the same area used for the liana plots. We used the tree turnover rate, varying from 0.30 to 1.38 across the sampled plots, as the third environmental gradient at Ducke Reserve.
Phylogeny of the Tribe Bignonieae
We estimated the phylogeny of the tribe Bignonieae (Bignoniaceae) based on DNA sequences (i.e., ndhF and PepC genes) from 133 liana species. Sequences from 118 species were obtained from Lohmann (2006), while 15 Bignonieae liana species were newly sequenced for this study (Supplementary Table 2). The complete list of accessions used can be found in Supplementary Table 3. The new sequences were obtained from DNA extractions from fresh leaves collected at Ducke Reserve. The details of DNA extraction, PCR amplification, cloning (PepC gene), and sequencing are presented in Zuntini et al. (2013). Of the 133 liana species of the tribe Bignonieae included in the phylogeny, 38 were also sampled in the assembled community dataset.
We aligned each marker using Muscle (Edgar, 2004), followed by visual inspection and manual corrections when needed. A Bayesian phylogenetic tree was inferred using BEAST 1.8 (Drummond et al., 2012) using the substitution models selected by the JModelTest 2 (Darriba et al., 2012). Seven substitution schemes were used, including Bayesian information criteria. The best-fit models were TVM+G and HKY+G for the ndhF and pepC markers, respectively. The analyses were conducted using the Yule speciation model and uncorrelated lognormal clocks for each marker. Two independent runs, each with 10,000,000 generations, were performed, sampling trees every 1,000 generations. To confirm stationarity and effective sampling of parameter distribution, the logs were analyzed in Tracer 1.6 (Rambaut et al., 2014). After discarding 10% of the initial trees and combining the results from runs, we obtained the species tree in TreeAnnotator using maximum clade credibility. Posterior probabilities (pp) were used to evaluate the support of each node. Phylogeny reconstruction was run in CIPRES.
Liana Species Diversity and Composition
To characterize the species diversity and composition of the liana assemblages of the tribe Bignonieae, we used Fisher’s alpha, Faith’s phylogenetic diversity index and a single Non-Metric Multidimensional Scaling (NMDS) dimension describing the species composition of each of the 34 forest plots sampled.
The Fisher’s alpha diversity index (α) was calculated as
where S and N are the total number of Bignonieae liana species and individuals in the sample, respectively (Fisher et al., 1943; Magurran, 1988). Faith’s phylogenetic diversity index was calculated by the sum of the lengths of the phylogenetic branches joining the basal node to the tips of all the species in the forest plots (Faith, 1992).
Liana species composition of the tribe Bignonieae distributed on the 34 plots was reduced to a single dimension using the Non-Metric Multidimensional Scaling (NMDS) ordination method, based on a dissimilarity matrix constructed with the Bray-Curtis index. The NMDS is a flexible and robust ordination technique that accommodates many distance measures with a unique assumption that the recovered dissimilarity among objects has a monotonic relationship with original distances (Minchin, 1987). The NMDS ordination performs better than other multivariate methods in recovering known structures, describing well especially ecological gradients (Minchin, 1987; Clarke, 1993). We choose the Bray-Curtis index, which is also pointed as one of the best to recover compositional similarity when applied over site-standardized data (Clarke, 1993; Legendre and Legendre, 1998; Ricotta and Podani, 2017). This standardization (species abundance transformed to relative abundance in each plot) avoids the caveats indicated by Jost et al. (2011) and was adopted here. In the NMDS, the number of dimensions in which distances among objects will be recovered is chosen before analysis. To evaluate the quality of the NMDS ordination and decide the number of axes to be used, we initially checked if the stress value was equal or less than 0.20 in the NMDS ordination with one, two, and three dimensions (Clarke, 1993). As the single NMDS dimension was within the acceptable stress limit, we present the single-dimension ordination analysis, facilitating biological interpretation in further hypothesis testing. Finally, we constructed the Shepard stress plot (Supplementary Figure 1) showing the relationship between the observed dissimilarities between plots and the ordination distances and estimated the linear and non-linear fit coefficient of determination to evaluate the NMDS goodness-of-fit (Clarke, 1993; Legendre and Legendre, 1998).
Phylogenetic Community Structure
To describe the phylogenetic structure of Bignonieae liana assemblages, we used four indexes of community phylogenetic structure applied to each plot (local communities): Net Relatedness Index (NRI), Nearest Taxon Index (NTI), Phylogenetic Species Variability (PSV), and Phylogenetic Species Clustering (PSC) (Webb et al., 2002; Pearse et al., 2014).
Net Relatedness Index and Nearest Taxon Index measure differences between the observed local and random communities generated by a null model of the independent swap algorithm proposed by Gotelli and Entsminger (2003). This null model randomizes the observed community matrix, keeping fixed the number of species and the frequency of occurrence in each plot (constrained null model). This null model is recommended to detect niche-based community assembly and is preferable to other null models (Webb et al., 2008; Kembel, 2009). The Net Relatedness Index (NRI) compares the phylogenetic distance among all community members, while the Nearest Taxon Index (NTI) examines only distances among nearest relatives. We calculated the NRI and NTI as
where MPD and MNTD represent the Mean Pairwise Distance and the Mean Nearest Taxon Distance among co-occurring species in the plot, respectively. MPDobserved and MNTDobserved refer to values for the observed local community in the plot, whereas MPDrandomized and MNTDrandomized are the mean MPD/MNTD of the randomized assemblages (n = 10,000), and sd is the standard deviation of the MPDrandomized/MNTDrandomized. Zero values are expected for the NRI and NTI in plots without any phylogenetic structure, while positive values indicate phylogenetic clustering and negative values indicate phylogenetic overdispersion.
We calculated Phylogenetic Species Variability (PSV), which measures whether the distribution of species across the phylogeny differs from the expectation under a Brownian null model. PSV equals one when all species in the community are less closely related than expected by chance and approaches zero when species are more closely related. Phylogenetic Species Clustering (PSC) is the same as PSV, but modified to reveal how species in an assembly are clustered toward the tip of the phylogeny. PSC approaches one when species are less related to each other at the tips (Helmus et al., 2007). PSV and PSC are analogous to NRI and NTI, respectively (Miller et al., 2017).
The species pool considered was composed of 38 liana species of the tribe Bignonieae distributed across the 34 sampled plots. Alternatively, we considered a species pool consisting of 50 liana species of the tribe as described in the flora of the Ducke Reserve (Oliveira et al., 2008) and other local surveys. However, the results using this larger species pool did not differ from the species pool used initially (data not shown).
Similarity in the Distribution Curves of Liana Species
We modeled the distribution of each Bignonieae liana species along the HAND gradient, applying the kernel density curve on the occurrence data of each species across the 34 plots. We initially replaced species occurrence by the respective HAND value of the plot where the species occurred. Therefore, the HAND values recovered for each species were modeled with the kernel density function, a non-parametric method that calculates population density distributions in the niche or functional trait space (Mouillot et al., 2005; Mason et al., 2011). This method attributes a bell-shaped density distribution to each datum, in our case to each HAND value, using the following kernel function:
where DxXi is the density (ordinal height of the bell-shaped curve) at functional trait value x = HAND for datum Xi, n is the number of data points (measurements), and h is the bandwidth that controls the smoothness of the estimator and defines the default value as 1.06σn − 1/5 with σ being the standard deviation of the trait values (Stine and Heyse, 2001). Species probability density at the HAND value is calculated as the sum of kernel density functions for each data point. Once the density distribution of each species was generated, the niche overlap between each pair of species (Oij) was calculated as the area of overlapping (%) between two probability density functions using the equation:
where fit and fjt are kernel-generated probability density functions for species i and j, respectively, and x is the HAND gradient. See Supplementary Figure 2 to visually observe the area of overlap between pairs of species (shaded area). In addition, we extracted the HAND value associated with the peak of the kernel density curve (niche optimum sensu Carscadden et al., 2020) for each species (see Supplementary Figure 2) and calculated the distance between the peaks of the curves of each species pair. We used the distance (in HAND units) as a measure of dissimilarity between niche optimums.
Statistical Analyses
To assess if hydro-edaphic (i.e., HAND), tree turnover rate, and soil phosphorus concentration gradients explain liana species diversity and composition, we used generalized linear multiple models (GLMs) with the Gaussian probabilistic distribution. We used Fisher’s alpha index, Faith’s phylogenetic diversity index and species composition summarized by the first and unique NMDS dimension as response variables, while the three ecological gradients were used as continuous fixed factors.
To investigate whether the phylogenetic structure of the liana assembly was related to the ecological gradients at Ducke Reserve, we used generalized linear multiple models (GLMs) with Gaussian probabilistic distribution. We used the four indexes of community phylogenetic structure (NRI, NTI, PSV, and PSC) as the response variables and the same three ecological gradients as fixed continuous factors.
Lastly, to test if closely related Bignonieae liana species have more similar distribution patterns than expected by chance, we applied matrix correlation tests (Mantel tests). In this case, the metrics for similarity of species distribution curves for each species pair were (i) the overlapping distribution area (%) and (ii) the distance between niche optimums. We used patristic phylogenetic distance among species. Similarity and distance matrices were constructed for the Mantel tests, and the significance level was determined with 10,000 permutations. Although the Mantel test is not the most suitable analytical method for testing phylogenetic signals, it is the only option when data are only expressed in pairwise distances among taxa, as suggested by Harmon and Glor (2010). This situation is the case with our measure of species overlap. To maintain the consistency of our analyses, we also applied the Mantel test to the species’ niche optimum. We only considered Bignonieae liana species in the Mantel tests that occurred in at least four plots (24 species).
All statistical analyses were performed in the R environment (v. 3.2.1; R Foundation for Statistical Computing, Vienna, Austria). Fisher’s alpha index and NMDS were calculated using the Vegan package (Oksanen et al., 2019). Faith’s Phylogenetic Diversity, MPD, MNTD, PSV, and PSC indexes were calculated using the Picante package (Helmus et al., 2007; Kembel et al., 2010). Normality and homoscedasticity of the residuals were checked in all models using plot inspection. Multicollinearity among independent variables was always checked, evaluating each model’s Variance Inflation Factor (VIF). We considered VIF of less than 2.5 as low levels of multicollinearity (Zuur et al., 2009; Adeboye et al., 2014), which was true for all our cases. We also calculated the partial determination coefficients for each predictor in the models describing the variance partition using the rsq.partial function of the R rsq package. We calculated the Gaussian kernel density curves for each Bignonieae liana species along the HAND gradient using the density function of the R stats package.
Results
The Bignonieae liana assemblage was composed of 1,861 stems of 38 species in the 34 plots. On average, we found 54.7 (±37.6) stems.ha–1 and about 9 (±4.0) species.ha–1 of Bignonieae at Ducke Reserve. Most species were members of three main clades: (i) Adenocalymma Clade, composed of species of Adenocalymma exclusively (10 species); (ii) the Fridericia-and-Allies Clade, composed primarily of species of Fridericia (8), Cuspidaria (3), Tanaecium (2), and Tynanthus (2); and (iii) the Multiples-of-Four Clade, composed primarily of species of Bignonia (4), Amphilophium (4), and Anemopaegma (2). Apart from those clades, we also sampled species of Pachyptera (2), Callichlamys (1), and Pleonotoma (4). Adenocalymma validum, Anemopaegma robustum, Pachyptera aromatica, Adenocalymma longilineum, and Adenocalymma adenophorum had the highest stem density per hectare (>10 stems.ha–1, Figure 1). Adenocalymma longilineum, Pachyptera aromatica, and Fridericia triplinervia occur in more than 50% of the sampled plots (Figure 1).
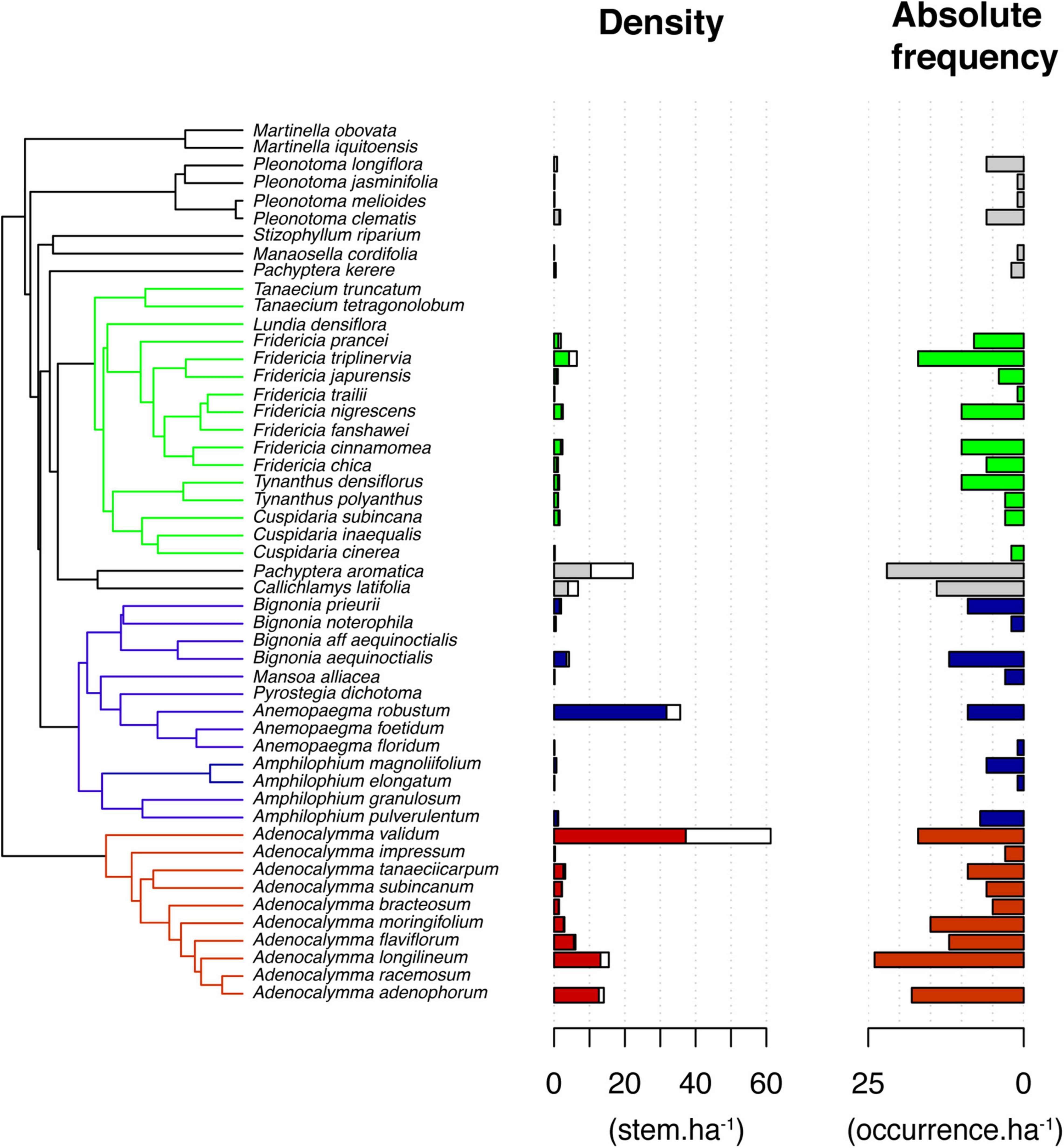
Figure 1. Phylogenetic relationships among the 38 liana species of the tribe Bignonieae (Bignoniaceae) and their stem density (genets in filled bar and ramets in the hollow bar) and absolute frequency in plots sampled at Ducke Reserve (Central Amazon, Brazil). Red, blue, and green highlight the three main clades of the tribe Bignonieae: Adenocalymma, Multiples-of-Four, and Fridericia-and-Allies, respectively.
Phylogeny of Bignonieae Lianas
The Bignonieae species tree obtained using a Bayesian algorithm was broadly congruent with previous topologies reconstructed for tribe Bignonieae (Lohmann, 2006; Pace et al., 2016), with Perianthomega vellozoi appearing as sister to all remaining Bignonieae and the Adenocalymma clade appearing as sister to the core Bignonieae. Within the latter, basal relationships are poorly resolved, although two well-supported clades emerged: the Multiples-of-Four Clade, formed by Anemopaegma, Bignonia, Mansoa, and Pyrostegia, and the Fridericia-and-Allies Clade formed by Cuspidaria, Fridericia, Lundia, Tanaecium, Tynanthus, and Xylophragma. All 15 new accessions fall into their expected genera.
Habitat Specialization of Lianas Along Environmental Gradients
The relative importance of environmental gradients in explaining taxonomic and phylogenetic diversity was tested for the complete liana assemblage of the tribe Bignonieae. We found that Fisher’s alpha diversity index was positively related to the HAND gradient (βst = 0.91, df = 25, r2partial = 0.21, p = 0.01; Figure 2A), but it was not associated with tree turnover (Figure 2B) nor with soil phosphorus concentration (Figure 2C). Faith’s phylogenetic diversity index was positively related to HAND (βst = 0.02, df = 25, r2partial = 0.52, p < 0.01; Figure 2D) and negatively related to tree turnover (βst = −0.009, df = 25, r2partial = 0.18, p = 0.02; Figure 2E), but not associated with soil phosphorus concentration (Figure 2F). Therefore, both taxonomic and phylogenetic diversity of Bignonieae lianas increased along the HAND gradient. At the same time, phylogenetic diversity decreased in areas with higher tree turnover. The partial determination coefficient showed the HAND gradient as the most important predictor of liana taxonomic and phylogenetic diversity, accounting for 21 and 52% of the variance explained by the linear models, respectively. Analytical details of the models in Figures 2, 3 are available in Supplementary Table 4.
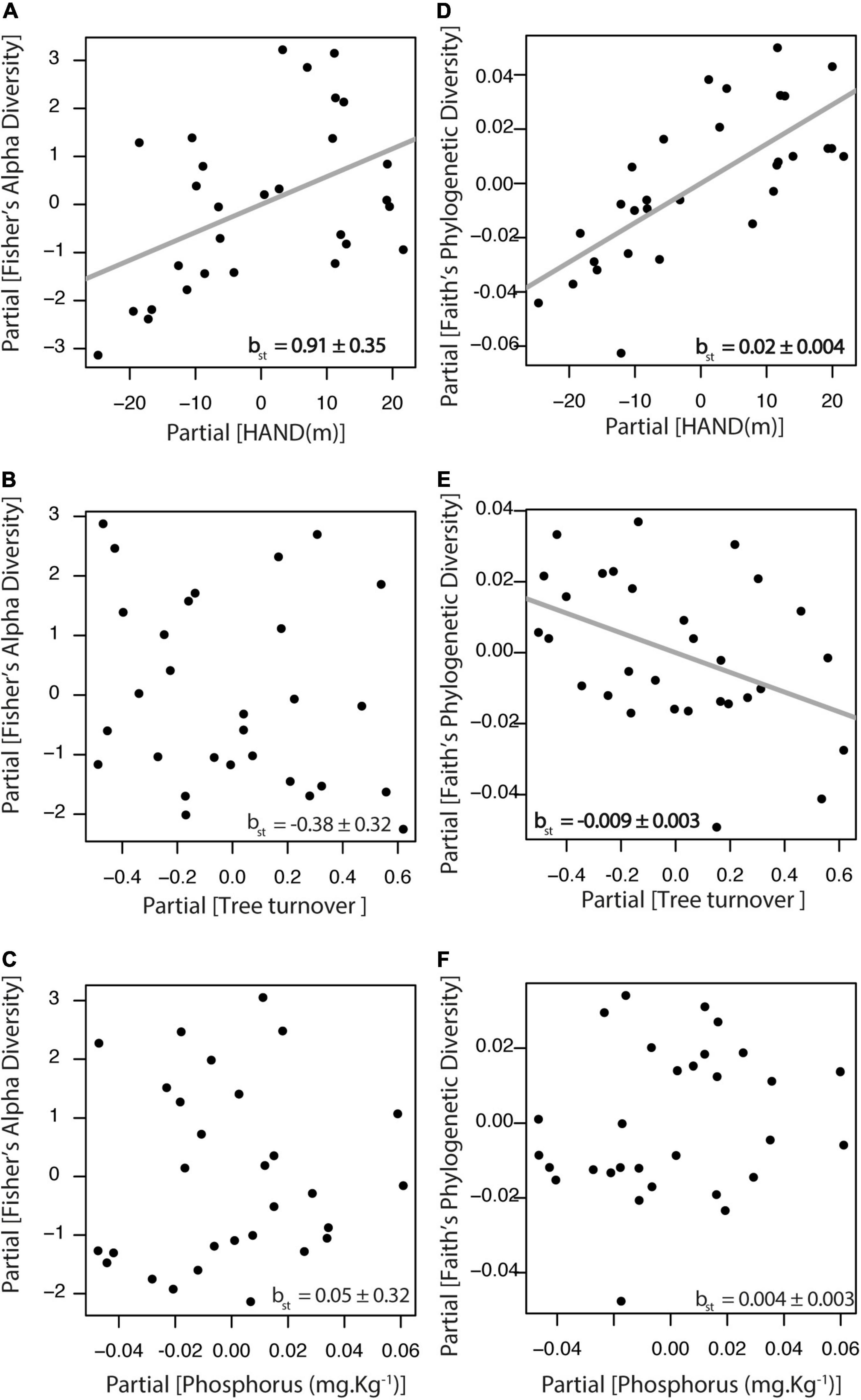
Figure 2. Relationship between liana species diversity and the three environmental gradients at Ducke Reserve (Central Amazon, Brazil). All relationships are partial regressions from models, including HAND (height above the nearest drainage), tree turnover and soil P content as predictive variables. (A–C) Fisher’s alpha diversity index; (D–F) Faith‘s phylogenetic diversity. Gray lines represent significant relationships (p < 0.05), and standardized multiple linear regression coefficients (βst ± SE) are shown in the graphs.
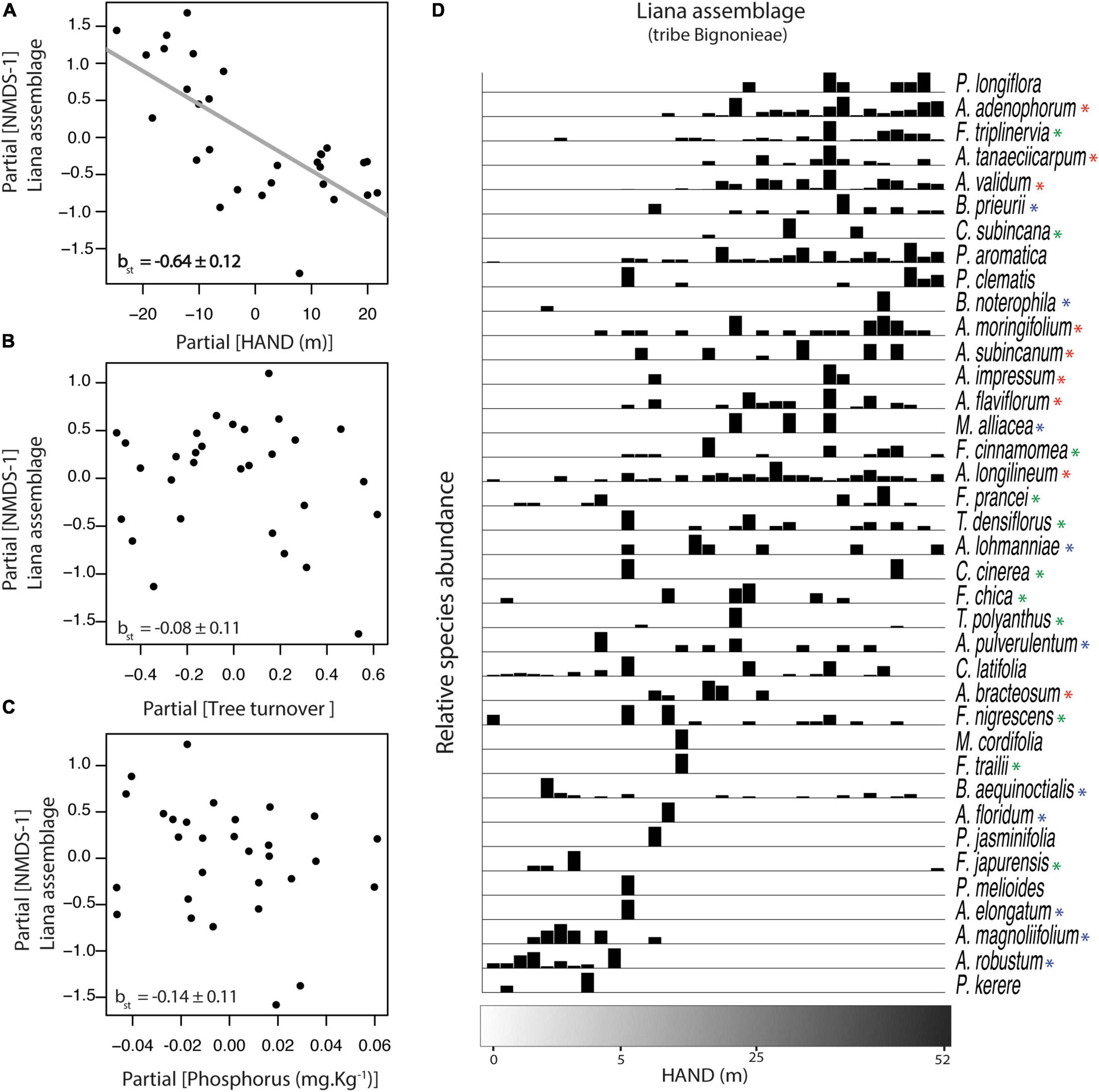
Figure 3. Relationship between liana species composition (NMDS-1) and the three environmental gradients at Ducke Reserve (Central Amazon, Brazil). Relationships (A–C) are partial regressions from models, including HAND (height above the nearest drainage), tree turnover and soil P concentration as predictive variables. The gray line represents a significant relationship (p < 0.05). Standardized multiple linear regression coefficients (βst ± SE) are shown in the graphs. (D) Species abundance distribution on the 34 plots is based on direct ordination along the HAND gradient. See Figure 1 for abbreviations of scientific terms. Red, blue, and green asterisks highlight the three main clades of the tribe Bignonieae: Adenocalymma, Multiples-of-Four, and Fridericia-and-Allies, respectively.
Species composition of the Bignonieae liana assemblage was represented in a single NMDS dimension with a satisfactory ordination goodness-of-fit (Stress = 0.20; linear r2= 0.89, non-linear r2= 0.96; see also the Shepard stress plot for visual inspection in the Supplementary Figure 1). The NMDS axis was correlated negatively with the HAND gradient (βst = −0.34, df = 25, p < 0.01; Figure 3A), but it was not associated with tree turnover (Figure 3B) nor with soil phosphorus concentration (Figure 3C). A partition of the relative importance of predictors showed the HAND gradient as the most important predictor of liana assemblage composition (r2partial = 0.47), accounting for 47% of the variance explained by the model.
Relative species abundance distributions along the HAND gradient showed that few Bignonieae liana species were consistently restricted to areas with lower HAND values (<13 m), i.e., those close to the valleys (Pachyptera kerere, Anemopaegma robustum, Amphilophium magnolipholium, and Amphilophium elongatum; Figure 3D). Toward plateau areas, a higher number of Bignonieae liana species co-occurred in areas with the highest HAND values (Pleonotoma longiflora, Adenocalymma adenophorum, Adenocalymma tanaeciicarpum, and Adenocalymma validum; Figure 3D). Overall, a gradual turnover of liana species composition along the HAND gradient was observed with some Bignonieae restricted to the gradient extremes, indicating habitat specialization along the hydro-edaphic gradient.
Different Phylogenetic Structures of Liana Assemblages Along the Hydro-Edaphic Gradient
We found that indices of species relatedness within plots (NTI) were positively related to the HAND gradient (βst = 0.41, df = 25, r2partial = 0.16, p = 0.01; Figure 4A), but not associated with tree turnover (Figure 4B) nor with soil phosphorus concentration (Figure 4C). Similarly, we found that PSC was negatively related to the HAND gradient (βst = −0.11, df = 25, r2partial = 0.57, p < 0.0001; Figure 4D), but not associated with tree turnover (Figure 4E) nor with soil phosphorus concentration (Figure 4F). In addition, PSV was negatively related to tree turnover gradient (βst = −0.011, df = 25, r2partial = 0.14, p = 0.047; Figure 4H), but not associated with HAND (Figure 4G) nor with soil phosphorus concentration (Figure 4I). In general, valleys (<13 m of HAND) had liana assemblages composed of less phylogenetically related Bignonieae species, switching to a more phylogenetically clustered liana assemblage in the plateaus with higher HAND values. NRI was unrelated to any of the three environmental gradients [F(3,25) = 0.81, r2 = 0.08, p = 0.50, Supplementary Figure 3]. Analytical details of the models in Figure 4 are available in Supplementary Table 5.
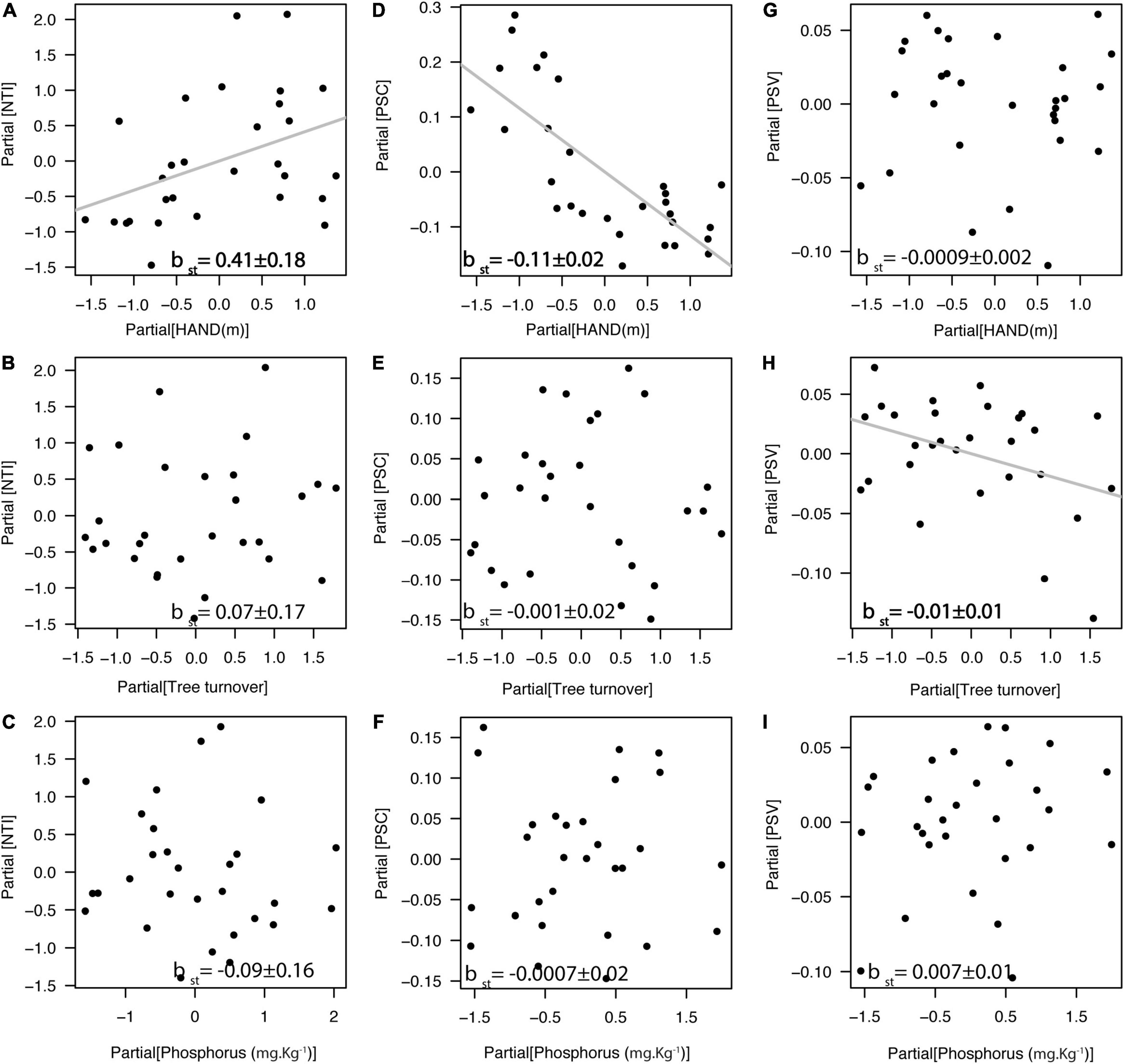
Figure 4. Relationship between the phylogenetic structure of the liana assemblage and the three environmental gradients at Ducke Reserve (Central Amazon, Brazil). All relationships are partial regressions from models, including HAND (height above the nearest drainage), tree turnover and soil P content as predictive variables. (A–C) Nearest taxon index (NTI); (D–F) Phylogenetic species clustering (PSC); (G–I) Phylogenetic species variability (PSV). Gray lines and bold values represent significant relationships (p < 0.05), and standardized multiple linear regression coefficients (βst ± SE) are shown in the graphs. Higher NTI values and lower PSC or PSV values indicate phylogenetic clustering, and lower NTI values and higher PSC or PSV values indicate phylogenetic overdispersion, relative to a null model of random shuffling of taxa labels across the tips of the phylogenetic tree including all taxa.
Modeling the distribution of each Bignonieae liana species along the hydro-edaphic gradient using the kernel density curve showed that liana species of the Adenocalymma Clade are more abundant in areas with higher HAND values. Similarly, closely related species in this clade are more abundant in more elevated portions of the HAND gradient with rare exceptions (Figure 5A). On the other hand, liana species of the Multiple-of-Four Clade were more often distributed in areas with lower HAND values with more exceptions in species distribution (Figure 5A). Species of the Fridericia-and-Allies Clade were more evenly distributed across the HAND gradient, although often associated with higher HAND values. In that case, closely related species often occupied higher portions of the HAND gradient (Figure 5A), although we found exceptions to this pattern. All other Bignonieae liana species outside these three major clades included closely related species that occupied contrasting portions of the HAND gradient (Supplementary Figure 4).
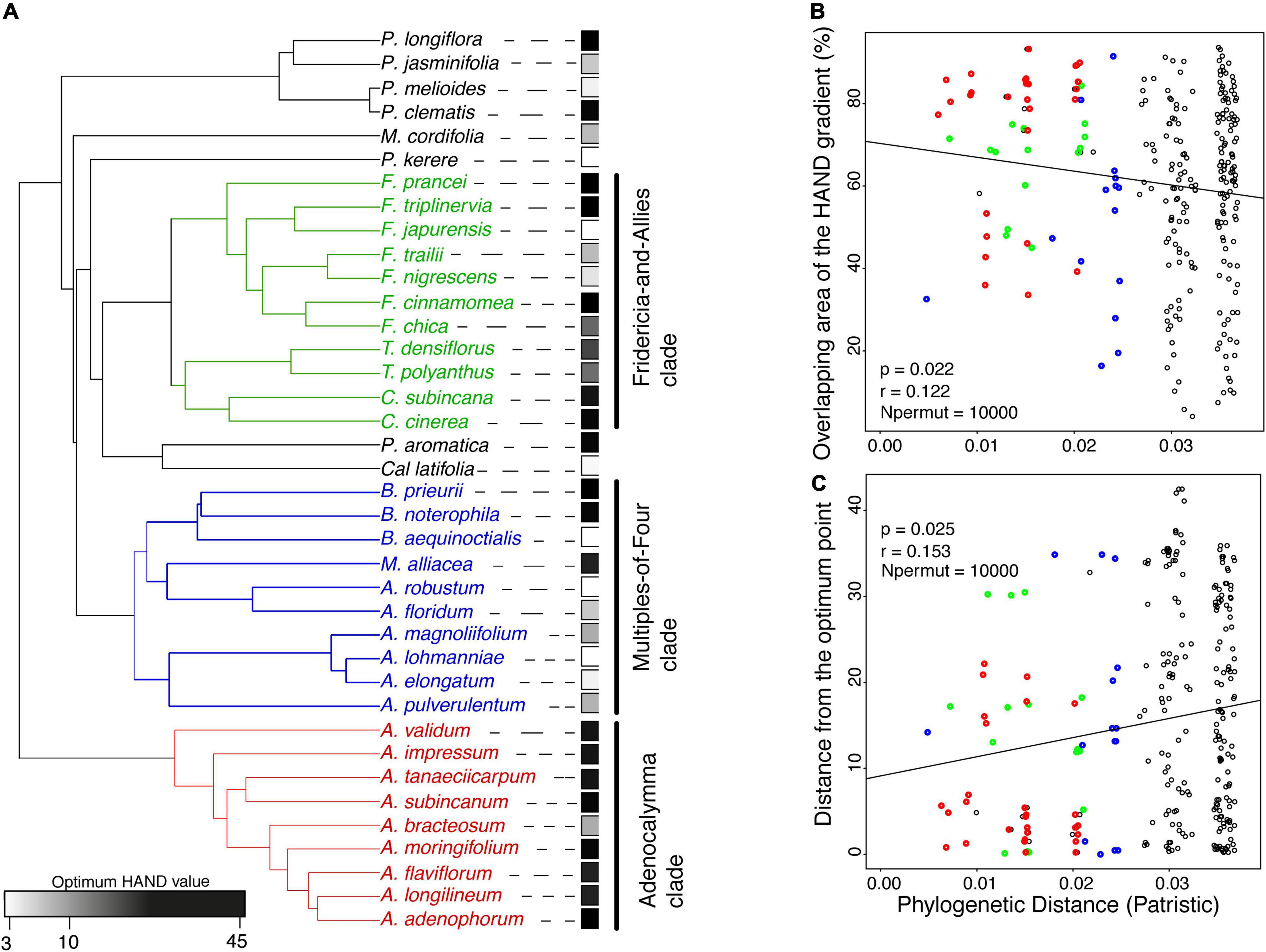
Figure 5. Relationship between phylogenetic pattern and liana species distribution of the tribe Bignonieae in the HAND gradient. (A) Phylogenetic relationship of the tribe Bignonieae with the optimum value of each species in the HAND gradient. (B) Negative relationship between the overlapping area of the HAND gradient and the Phylogenetic Distance (Patristic). (C) Positive relationship between the distance from the optimum value of each species in the HAND gradient and the phylogenetic distance (Patristic). See Figure 1 for abbreviations of scientific terms. Red, blue, and green highlight the three main clades of the tribe Bignonieae: Adenocalymma, Multiples-of-Four, and Fridericia-and-Allies, respectively. Black points represent other species of the tribe Bignonieae.
The Mantel test detected a negative relationship between the overlap of the species distribution curve along the HAND gradient and phylogenetic distance (r = −0.12, p = 0.02, Figure 5B). The Mantel test also detected a positive relationship between the pairwise distance of niche optimum in the HAND gradient and phylogenetic distance (r = 0.15, p = 0.02, Figure 5C). Therefore, closely related liana species of the tribe Bignonieae had a greater overlap in their distribution and smaller distance of niche optimum in the HAND gradient.
Discussion
Variation in forest disturbance, soil nutrients, and soil hydrology constitute gradients affecting the assemblage of different plant groups at the landscape scale. However, the diversity and composition of lianas in our study site were explained almost exclusively by the hydro-edaphic gradient, as defined by the height above the nearest drainage (HAND). We found that (1) liana species composition of the tribe Bignonieae changes along the hydro-edaphic gradient, partially owing to habitat specialization, with wet valleys holding a lower taxonomic diversity of phylogenetically dispersed species, while well-drained plateaus concentrated higher diversity of closely related taxa, and (2) in general, closely related liana species had a larger distribution overlap and smaller distances among hydro-edaphic niche optimum values. These findings indicate habitat filtering by different mechanisms depending on specific environmental conditions along the hydro-topographic gradient.
To understand the divergent patterns of habitat filtering in this forest, we need to understand the physicochemical conditions created by topography and how they affect forest structure. Topography generates a strong local hydro-edaphic gradient, given the convergence of water toward the lower grounds, predominantly through groundwater flow (Fan et al., 2013; Schietti et al., 2014; Costa et al., 2022). Where the water table is close to the surface, in sandy valley soils, water availability is more extensive through the year, although this condition also generates waterlogging at least seasonally (Hodnett et al., 1997). Waterlogging comes with anoxia and reduced nitrogen availability (Ponnamperuma, 1972; Luizão et al., 2004), both limiting conditions for plant development (Parent et al., 2008; Kreuzwieser and Gessler, 2010). Waterlogging also leads to a loose, less cohesive aggregation among soil particles which is summed to the sandy soil type and the shallow rooting imposed by shallow water tables (Fan et al., 2017), decreasing the soil capacity to anchor roots firmly (Fraser, 1962; Ennos, 2000). As we move away from valleys toward the top of hills, water table depth increases, and the soil becomes progressively clayey (Chauvel et al., 1987; Luizão et al., 2004). Clayey plateau soils hold more nutrients, such as nitrogen and exchangeable bases (Luizão et al., 2004; Costa et al., 2005), have lower permeability and higher water-holding capacity than sandy valley soils (Sanchez, 2019). However, during the dry season, these portions of the hydro-edaphic gradient have lower water availability for plants, especially in extreme drought years (Costa et al., 2020). The clayey soils on the plateaus are deep and well-structured, providing a good root anchoring (Quesada et al., 2009). Thus, the topography, hydrology, and edaphic factors comprise a complex scenario that creates distinct habitats for plants along the hydro-edaphic gradient of Ducke Reserve.
The habitats formed along the hydro-edaphic gradient, in turn, affect forest structure, determining potential support for lianas in the lower strata of the forest. Valleys are associated with a lower density of trees and a high density of palms (Kahn and Castro, 1985; Nogueira et al., 2011) that typically offer poor support for the canopy rise of lianas (Putz, 1984b; Nogueira et al., 2011). Compared to valleys, clayey plateaus on the opposite side of the hydro-edaphic gradient have a higher density of trees, including trees of different sizes and fewer canopy palms (Castilho et al., 2006; Nogueira et al., 2011). This forest structure in the plateaus creates favorable conditions with the greatest potential to support liana climbing during development and its maintenance after reaching the forest canopy (Nogueira et al., 2011). This set of favorable and unfavorable environmental conditions below and above-ground across the hydro-edaphic gradient constitute differential filters of the lianas species assemblage, affecting the diversity and distribution of plants, as discussed below.
Assembly Along the Hydro-Edaphic Gradient
The more restrictive conditions in valleys, as noted above, result in strong environmental filtering of a small set of species. Decreased plant species diversity is expected in more restrictive environments (Givnish, 1999), such as flooded forests with hypoxic to anoxic soils, limiting the growth, survival, and reproduction of non-edaphic specialists (Parolin, 2009; Parolin and Wittmann, 2010). Although wind-dispersed seeds of Bignonieae liana species may often arrive in valleys, our data highlight that only a few liana species can grow in these restrictive conditions. A lower diversity in wetlands and flooded forests compared to that in uplands was also found for liana and tree assemblages in other regions of the Amazon basin (Burnham, 2002; Muscarella et al., 2019). Functional traits required to be successful in these conditions may include the ability to germinate, establish and propagate in anoxic waterlogged soils (Parent et al., 2008; Parolin, 2009). The high rates of vegetative propagation of the few Bignonieae liana species that grow in valleys may contribute to maintaining individuals of these species locally (Rocha et al., 2020). In those species, such as the most abundant liana species of valleys Anemopaegma robustum, it is common the production of a high number of adventitious roots with denser lenticels (CG personal observations) which can improve root oxygenation during waterlogging. Also, other undocumented traits for lianas could be crucial under such conditions. For example, alternative energy production routes, such as fermentation, and as plants grow, the existence of aerenchyma (Parent et al., 2008; Parolin, 2009).
Non-exclusive mechanisms can explain the habitat specialization associated with a phylogenetically overdispersed pattern in valleys–evolutionary convergence in functional traits and biotic interactions. Repeated colonization through time by multiple species from different clades bearing particular and similar functional traits appropriate for this more restrictive environment, i.e., evolutionary convergence, may create an overdispersed phylogenetic pattern. Evolutionary convergence agrees well with the distribution of functional traits of lianas described along the hydro-edaphic gradient (Rocha et al., 2021). Liana species in valleys share similar traits, including Bignonieae lianas, such as lower wood density and specific leaf area, larger stomata size and self-supporting xylem area (Rocha et al., 2021), despite the large phylogenetic distance among those liana species. Low wood density species with cheaper stem construction tend to grow more rapidly in short but more favorable periods of the year (King et al., 2006; Larjavaara and Muller-Landau, 2010; Rüger et al., 2012), which may be advantageous in the seasonally waterlogged valleys, where the short dry season provide the most favorable mesic soil conditions for growth. In addition, the larger self-supporting xylem area in the stems allow lianas to behave as freestanding woody plants in the understory during unfavorable periods along development.
Biotic interactions may also be invoked to explain the overdispersion pattern in more restrictive habitats, although these are so far more speculative arguments. For example, interspecific competition and facilitation could both generate or reinforce an overdispersion pattern. Past competition could lead to local extinction of ecologically similar and closely related species if strong competitive interactions dominate (Webb et al., 2002; Cavender-Bares et al., 2004, 2009). However, under stressful conditions, positive interactions among plant species as facilitation are more likely than competition (Bertness and Callaway, 1994). Although the facilitation process is a speculative explanation here, a particular attachment structure found in Bignonieae liana associated with valleys–the adhesive tendrils (e.g., Amphilophium magnoliifolium and Bignonia aequinoctiales) allows this possibility. Adhesive tendrils enable lianas to attach and climb to vertical supports independently of their overall size and shape (Sousa-Baena et al., 2018; Sperotto et al., 2020), potentially overcoming the limitations of inadequate supports such as palms. After establishing themselves, these liana species can act as adequate support for other younger liana species with different attachment structures to climb, i.e., they facilitate their climbing process. If the facilitation process is more recurrent within valley liana species than in plateaus species, the presence of a first canopy Bignonieae liana established in inadequate support could contribute to the local establishment of newly arriving younger lianas of more distantly related species (e.g., Verdú et al., 2009; Valiente-Banuet and Verdú, 2013).
As we move away from the valleys toward the top of the hills, we detect an increase in liana species diversity that is composed of a different set of Bignonieae liana species. The greater soil fertility, higher density of adequate support, and the more stable and less restrictive conditions of plateaus allow the co-occurrence of a higher number of liana species (Rocha et al., 2021), including lianas of the tribe Bignonieae, despite the lower water availability than valleys. Soil water restriction in the dry season probably does not limit lianas at Ducke Reserve, as species associated with plateaus show higher embolism resistance than those of valleys (Gerolamo et al., 2018 unpublished data; Oliveira et al., 2019). Lianas under such conditions may also rely on deep roots, hydraulic redistribution associated with multifocal growing strategies, or higher water storage capacity (de Azevedo Amorim et al., 2018).
On plateaus, habitat specialization is associated with a high diversity of more closely related liana species of the tribe Bignonieae. This phylogenetically clustered pattern may have emerged from the weak interspecific competition and a predominance of environmental filtering of conserved traits (Webb et al., 2002; Cavender-Bares et al., 2004, 2009; Hubbell, 2005). Supporting this, we noticed similar functional traits (e.g., higher wood density) in the liana species co-occurring in the higher portions of the hydro-edaphic gradient (Rocha et al., 2021). The higher wood density is associated with the high hydraulic resistance (Hacke and Sperry, 2001; Jacobsen et al., 2005) probably required for liana maintenance in the plateaus. This pattern supports trait conservatism across Bignonieae liana species on the plateau.
Therefore, hydro-edaphic habitat specialization associated with different patterns of phylogenetic structure of lianas of the tribe Bignonieae is mediated by specific functional traits at Ducke Reserve, probably generated by a strong species filtering in the valleys and a distinct and soft species filtering in plateaus. This differential filtering pattern of liana species of the tribe Bignonieae associated to hydro-edaphic conditions here described is similar to the patterns of other plant groups found at the same site (Drucker et al., 2008; Landeiro et al., 2012; Freitas et al., 2014; Schietti et al., 2014; Cosme et al., 2017; Oliveira et al., 2019; Costa et al., 2020) and elsewhere (Fine et al., 2005; Eiserhardt et al., 2013; Muscarella et al., 2019; Supplementary Table 1). In addition, the overdispersed phylogenetic pattern observed for the Bignonieae lianas in the valleys of Ducke Reserve was also found for palm assemblages at the same site (Freitas et al., 2014). Overdispersion under such conditions appears to be shared among sites beyond Central Amazonia, e.g., trees and shrubs in flooded forest or white-sand habitats with periodic flooding (Fine et al., 2005; Fine and Kembel, 2011; Eiserhardt et al., 2013; Oliveira et al., 2014; Araújo and Santos, 2019), pointing to generality in these Amazonian systems (but see Baraloto et al., 2021 for a discussion at higher taxonomic levels). Under such conditions, we highlight that the convergence of functional traits to deal with more stressful environments and less likely facilitation among plant species could explain the phylogenetic structure of all plant groups beyond lianas.
Phylogenetic diversity of Bignonieae lianas was negatively related to tree turnover rate. Higher tree turnover rates occur in areas with a higher frequency of tree fall (canopy gaps) with a consequent increase in light availability (Canham et al., 1990). Light availability increases the recruitment of lianas and, thus, liana stem densities (Schnitzer et al., 2000; Dalling et al., 2012; Gerolamo et al., 2018; Schnitzer, 2018), but not liana diversity, as a few more opportunistic species quickly occupy patches with high light availability (Putz, 1984a). Bignonieae liana diversity and species composition were unrelated to soil phosphorus concentration, similar to other reports (e.g., Dalling et al., 2012). This pattern may simply be a consequence of the short extent of the phosphorus gradient in the landscape (Pansonato et al., 2013), which is valid for our study site (mean concentration of available soil phosphorus 3.2 ± SD2.3 mg.kg–1, Costa et al., 2005). The soil diversity in the Amazon basin is mainly related to differences in geology manifesting mostly at the regional rather than local to meso scales (Quesada et al., 2011). Liana species diversity and composition have been shown to respond to soil phosphorus in other sites (e.g., the Montane subtropical forest) (Malizia et al., 2010), but this effect has remained elusive in the Amazon forest.
Conclusion
We identified a general pattern of phylogenetic clustering among lianas of the tribe Bignonieae at Ducke Reserve. This pattern emerges from the fact that most liana species occur in the higher portions of the HAND gradient, where the phylogenetic structure of the liana assemblage is clustered. Thus, niche conservatism emerges as the most general pattern for this clade of lianas in this central Amazon forest, where more closely related species co-occur in higher portions of the hydro-edaphic gradient. However, valleys diverge from this pattern by having phylogenetically overdispersed assemblages.
We conclude that ecological filters and phylogenetic history have played fundamental roles in structuring liana assemblies at the landscape scale. In sum, the pattern of hydro-edaphic habitat specialization results from different ecological processes that have contributed to the assembly of local communities from valleys to plateaus, with the strength of environmental filters varying along this gradient. These findings highlight the need for future experimental studies to understand the causes behind the diversity of liana assemblies across habitats. For example, studies investigating how different liana strategies varying in leaf, stem, and root functional traits are responsible for the good performance of liana species along the hydro-edaphic gradient. Data of this nature will, in turn, help clarify the role of fine-scale soil hydrology in the evolutionary history of Amazonian flora.
Data Availability Statement
The raw data supporting the conclusions of this article will be made available by the authors, without undue reservation.
Author Contributions
AN, AV, and FC conceived the ideas and designed the experiments. AN and AZ collected the data. AN, LL, and AZ identified the plant species. CG, AZ, and AN analyzed the data and led the writing of the manuscript. All authors contributed to the manuscript writing and revision and approved the final version.
Funding
This study was supported by the Coordenação de Aperfeiçoamento de Pessoal de Nível Superior - Brasil (CAPES) Finance code 88882.333016/2019-01, São Paulo Research Foundation (FAPESP 2018/06917-7, 2018/23899-2), and Conselho Nacional de Desenvolvimento Científico e Tecnológico (CNPq Grants 441282/2016-4, 403764/2012-2, and 558244/2009-2) that funded the Central Amazon Long-term Ecological Project, the plots of which were used for this study. Additional funds were provided to LL by CNPq through a Pq-1B grant (310871/2017-4) and to AN by CNPq through a Postdoctoral Fellowship (CNPq Grant 234000/2014-7) and Research Grant (434692/2018-2), and by the FAPESP through a Young Investigators Grant (FAPESP 2019/19544-7).
Conflict of Interest
The authors declare that the research was conducted in the absence of any commercial or financial relationships that could be construed as a potential conflict of interest.
Publisher’s Note
All claims expressed in this article are solely those of the authors and do not necessarily represent those of their affiliated organizations, or those of the publisher, the editors and the reviewers. Any product that may be evaluated in this article, or claim that may be made by its manufacturer, is not guaranteed or endorsed by the publisher.
Acknowledgments
We thank Paulo Inacio for the valuable discussion on data analyses in the first ideas of this work. We also thank all staff at the Department of Reserves (DSER) of the National Institute for Amazonian Research (INPA) for logistical support, as well as everyone involved in fieldwork, especially João Batista da Silva, Raimunda Nazaré Oliveira de Araújo, and Sebastião Salvino de Souza.
Supplementary Material
The Supplementary Material for this article can be found online at: https://www.frontiersin.org/articles/10.3389/ffgc.2022.809904/full#supplementary-material
References
Adeboye, N. O., Fagoyinbo, I. S., and Olatayo, T. (2014). Estimation of the Effect of Multicollinearity on the Standard Error for Regression Coefficients. IOSR J. Math. 10, 16–20. doi: 10.9790/5728-10411620
Araújo, F. D. C., and Santos, R. M. (2019). Different degrees of water-related stress affect evolutionary diversity in a seasonally dry biome. Oecologia 189, 795–802. doi: 10.1007/s00442-019-04358-4
Baraloto, C., Vleminckx, J., Engel, J., Petronelli, P., Dávila, N., RÍos, M., et al. (2021). Biogeographic history and habitat specialization shape floristic and phylogenetic composition across Amazonian forests. Ecol. Monogr. 91:e01473. doi: 10.1002/ecm.1473.
Bertness, M. D., and Callaway, R. (1994). Positive interactions in communities. Trends Ecol. Evol. 9, 191–193. doi: 10.1201/9780203738559
Bordon, N. G., Nogueira, A., Leal Filho, N., and Higuchi, N. (2019). Blowdown disturbance effect on the density, richness and species composition of the seed bank in Central Amazonia. For. Ecol. Manage. 453:117633. doi: 10.1016/j.foreco.2019.117633
Bullock, E. L., Woodcock, C. E., Souza, C., and Olofsson, P. (2020). Satellite-based estimates reveal widespread forest degradation in the Amazon. Glob. Chang. Biol. 26, 2956–2969. doi: 10.1111/gcb.15029
Burnham, R. J. (2002). Dominance, diversity and distribution of lianas in Yasuní, Ecuador: Who is on top? J. Trop. Ecol. 18, 845–864. doi: 10.1017/S0266467402002559
Burnham, R. J. (2004). Alpha and beta diversity of Lianas in Yasuní, Ecuador. For. Ecol. Manage. 190, 43–55. doi: 10.1016/j.foreco.2003.10.005
Canham, C. D., Denslow, J. S., Platt, W. J., Runkle, J. R., Spies, T. A., and White, P. S. (1990). Light regimes beneath closed canopies and tree-fall gaps in temperate and tropical forests. Can. J. For. Res. 20, 620–631.
Carscadden, K. A., Emery, N. C., Arnillas, C. A., and Cadotte, M. W. (2020). Niche breadth: Causes and consequences for ecology, evolution, and conservation. Q. Rev. Biol. 953, 179–214.
Castilho, C. V., Magnusson, W. E., De Araújo, R. N. O., and Luizão, F. J. (2010). Short-term temporal changes in tree live biomass in a central amazonian forest, Brazil. Biotropica 42, 95–103. doi: 10.1111/j.1744-7429.2009.00543.x
Castilho, C. V., Magnusson, W. E., de Araújo, R. N. O., Luizão, R. C. C., Luizão, F. J., Lima, A. P., et al. (2006). Variation in aboveground tree live biomass in a central Amazonian Forest: Effects of soil and topography. For. Ecol. Manage. 234, 85–96. doi: 10.1016/j.foreco.2006.06.024
Cavender-Bares, J., Kitajima, K., and Bazzaz, F. A. (2004). Multiple trait associations in relation to habitat differentiation among 17 Floridian oak species. Ecol. Monogr. 74, 635–662. doi: 10.1890/03-4007
Cavender-Bares, J., Kozak, K. H., Fine, P. V. A., and Kembel, S. W. (2009). The merging of community ecology and phylogenetic biology. Ecol. Lett. 12, 693–715. doi: 10.1111/j.1461-0248.2009.01314.x
Chauvel, A., Lucas, Y., and Boulet, R. (1987). On the genesis of the soil mantle of the region of Manaus. Experientia 43, 234–241.
Clarke, K. R. (1993). Non-parametric multivariate analyses of changes in community structure. Aust. J. Ecol. 18, 117–143.
Cosme, L. H. M., Schietti, J., Costa, F. R. C., and Oliveira, R. S. (2017). The importance of hydraulic architecture to the distribution patterns of trees in a central Amazonian forest. New Phytol. 215, 113–125. doi: 10.1111/nph.14508
Costa, F. R. C., Castilho, C., Drucker, D. P., Kinupp, V., Nogueira, A., and Spironello, W. (2008). “Flora,” in Reserva Ducke: A biodiversidade Amazônica Através de Uma Grade, eds M. L. de Oliveira, F. B. Baccaro, R. Braga-Neto, and W. E. Magnusson (Manaus, Brazil: Áttema Design Editoria), 23–30.
Costa, F. R. C., and Magnusson, W. E. (2010). The need for large-scale, integrated studies of biodiversity - the experience of the program for biodiversity research in Brazilian Amazonia. Nat. Conserv. 8, 3–12. doi: 10.4322/natcon.00801001
Costa, F. R. C., Magnusson, W. E., and Luizao, R. C. (2005). Mesoscale distribution patterns of Amazonian understorey herbs in relation to topography, soil and watersheds. J. Ecol. 93, 863–878. doi: 10.1111/j.1365-2745.2005.01020.x
Costa, F. R. C., Zuanon, J. A. S., Baccaro, F. B., de Almeida, J. S., Menger, J. da S., Souza, J. L. P., et al. (2020). Effects of climate change on central amazonian forests: A two decades synthesis of monitoring tropical biodiversity. Oecologia Aust. 24, 317–335. doi: 10.4257/oeco.2020.2402.07
Costa, F. R., Schietti, J., Stark, S. C., and Smith, M. N. (2022). The other side of tropical forest drought: Do shallow water table regions of Amazonia act as large-scale hydrological refugia from drought? New Phytol. 3–29. doi: 10.1111/nph.17914
Dalling, J. W., Heineman, K., Lopez, O. R., Wright, S. J., and Turner, B. L. (2016). “Nutrient Availability in Tropical Rain Forests: The Paradigm of Phosphorus Limitation,” in Tropical Tree Physiology, eds. G. Goldstein and L. Santiago (New York: Springer), 261–273. doi: 10.1038/248302c0.
Dalling, J. W., Schnitzer, S. A., Baldeck, C., Harms, K. E., John, R., Mangan, S. A., et al. (2012). Resource-based habitat associations in a neotropical liana community. J. Ecol. 100, 1174–1182. doi: 10.1111/j.1365-2745.2012.01989.x
Darriba, D., Taboada, G. L., Doallo, R., and Posada, D. (2012). JModelTest 2: More models, new heuristics and parallel computing. Nat. Methods 9:772. doi: 10.1038/nmeth.2109
de Azevedo Amorim, T., Nunes-Freitas, A. F., and Rosado, B. H. P. (2018). Revisiting the hypothesis for increasing liana abundance in seasonal forests: a theoretical review. Plant Soil 430, 1–6. doi: 10.1007/s11104-018-3730-6.
Drucker, D. P., Costa, F. R. C., and Magnusson, W. E. (2008). How wide is the riparian zone of small streams in tropical forests? A test with terrestrial herbs. J. Trop. Ecol. 24, 65–74. doi: 10.1017/S0266467407004701
Drummond, A. J., Suchard, M. A., Xie, D., and Rambaut, A. (2012). Bayesian phylogenetics with BEAUti and the BEAST 1.7. Mol. Biol. Evol. 29, 1969–1973. doi: 10.1093/molbev/mss075
Edgar, R. C. (2004). MUSCLE: Multiple sequence alignment with high accuracy and high throughput. Nucleic Acids Res. 32, 1792–1797. doi: 10.1093/nar/gkh340
Eiserhardt, W. L., Svenning, J. C., Borchsenius, F., Kristiansen, T., and Balslev, H. (2013). Separating environmental and geographical determinants of phylogenetic community structure in Amazonian palms (Arecaceae). Bot. J. Linn. Soc. 171, 244–259. doi: 10.1111/j.1095-8339.2012.01276.x
Ennos, A. R. (2000). The mechanics of root anchorage. Adv. Bot. Res. 33, 133–157. doi: 10.1016/s0065-2296(00)33042-7
Faith, D. P. (1992). Conservation evaluation and phylogenetic diversity. Biol. Conserv. 61, 1–10. doi: 10.1016/0006-3207(92)91201-3
Fan, Y., Li, H., and Miguez-Macho, G. (2013). Global patterns of groundwater table depth. Science 339, 940–943. doi: 10.1126/science.1229881
Fan, Y., Miguez-Macho, G., Jobbágy, E. G., Jackson, R. B., and Otero-Casal, C. (2017). Hydrologic regulation of plant rooting depth. Proc. Natl. Acad. Sci. U. S. A. 114, 10572–10577. doi: 10.1073/pnas.1712381114
Fine, P. V. A., Daly, D. C., Muñoz, G. V., Mesones, I., and Cameron, K. M. (2005). The Contribution of Edaphic Heterogeneity To the Evolution and Diversity of Burseraceae Trees in the Western Amazon. Evolution 59, 1464. doi: 10.1554/04-745
Fine, P. V. A., and Kembel, S. W. (2011). Phylogenetic community structure and phylogenetic turnover across space and edaphic gradients in western Amazonian tree communities. Ecography (Cop.). 34, 552–565. doi: 10.1111/j.1600-0587.2010.06548.x
Fisher, R. A., Corbet, A. S., and Williams, C. B. (1943). The Relation Between the Number of Species and the Number of Individuals in a Random Sample of an Animal Population. J. Anim. Ecol. 12, 42. doi: 10.2307/1411
Fraser, A. I. (1962). The soil and roots as factors in tree stability. Forestry 34, 117–127. doi: 10.1093/forestry/34.2.117
Freitas, C. G., de Sales Dambros, C., Eiserhardt, W. L., Costa, F. R. C., Svenning, J. C., and Balslev, H. (2014). Phylogenetic structure of a palm community in the central Amazon: changes along a hydro-edaphic gradient. Plant Ecol. 215, 1173–1185. doi: 10.1007/s11258-014-0376-1
Gentry, A. (1988). Changes in Plant Community Diversity and Floristic Composition on Environmental and Geographical Gradients. Ann. Missouri Bot. Gard. 75, 1–34.
Gerolamo, C. S., Nogueira, A., Costa, F. R. C., de Castilho, C. V., and Angyalossy, V. (2018). Local dynamic variation of lianas along topography maintains unchanging abundance at the landscape scale in central Amazonia. J. Veg. Sci. 29, 651-661. doi: 10.1111/jvs.12644.
Gerwing, J. J., Schnitzer, S. A., Burnham, R. J., Bongers, F., Chave, J., DeWalt, S. J., et al. (2006). A Standard Protocol for Liana Censuses. Ecology 38, 256–261.
Givnish, T. J. (1999). On the causes of gradients in tropical tree diversity. J. Ecol. 87, 193–210. doi: 10.1046/j.1365-2745.1999.00333.x
Gotelli, N. J., and Entsminger, G. L. (2003). Swap Algorithms in Null Model Analysis. Ecology 84, 532–535.
Graham, C. H., and Fine, P. V. A. (2008). Phylogenetic beta diversity: Linking ecological and evolutionary processes across space in time. Ecol. Lett. 11, 1265–1277. doi: 10.1111/j.1461-0248.2008.01256.x
Guillaumet, J.-L., and Kahn, F. (1982). Structure et dynamisme de la forêt. Acta Amaz. 12, 61–77. doi: 10.1590/1809-43921982123s061
Hacke, U. G., and Sperry, J. S. (2001). Functional and ecological xylem anatomy. Funct. Ecol. xylem Anat. 4/2, 97–115.
Harmon, L. J., and Glor, R. E. (2010). Poor statistical performance of the mantel test in phylogenetic comparative analyses. Evolution 64, 2173–2178. doi: 10.1111/j.1558-5646.2010.00973.x
Hawkins, B. A., and Diniz-Filho, J. A. F. (2004). “Latitude” and Geographic Patterns in Species Richness. Ecography 27, 268–272.
Helmus, M. R., Bland, T. J., Williams, C. K., and Ives, A. R. (2007). Phylogenetic measures of biodiversity. Am. Nat. 169, E68-83. doi: 10.1086/511334.
Hodnett, M. G., Vendrame, I., De O. Marques Filho, A., Oyama, M. D., Tomasella, J, et al. (1997). Soil water storage and groundwater behaviour in a catenary sequence beneath forest in central Amazonia: I. Comparisons between plateau, slope and valley floor. Hydrol. Earth Syst. Sci. 1, 265–277. doi: 10.5194/hess-1-265-1997
Hubbell, S. P. (2005). Neutral theory in community ecology and the hypothesis of functional equivalence. Funct. Ecol. 19, 166–172. doi: 10.1111/j.0269-8463.2005.00965.x
Jacobsen, A. L., Ewers, F. W., Pratt, R. B., Paddock, W. A., and Davis, S. D. (2005). Do xylem fibers affect vessel cavitation resistance? Plant Physiol. 139, 546–556. doi: 10.1104/pp.104.058404
Jennifer, Morgan, B., and Connolly, E. L. (2013). Plant-Soil Interactions : Nutrient Uptake. Nat. Educ. Knowl. 4, 2–7.
Jost, L., Chao, A., and Chazdon, R. L. (2011). “Compositional similarity and β (beta) diversity,” in Biological Diversity: Frontiers in Measurement and Assessment, eds B. A. Maurer and B. J. McGill. (Oxford: Oxford University Press), 66–84.
Kahn, F., and Castro, A. (1985). The Palm Community in a Forest of Central Amazonia, Brazil. Biotropica 17, 210–216.
Kembel, S. W. (2009). Disentangling niche and neutral influences on community assembly: Assessing the performance of community phylogenetic structure tests. Ecol. Lett. 12, 949–960. doi: 10.1111/j.1461-0248.2009.01354.x
Kembel, S. W., Cowan, P. D., Helmus, M. R., Cornwell, W. K., Morlon, H., Ackerly, D. D., et al. (2010). Picante: R tools for integrating phylogenies and ecology. Bioinformatics 26, 1463–1464. doi: 10.1093/bioinformatics/btq166
King, D. A., Davies, S. J., Tan, S., and Noor, N. S. M. (2006). The role of wood density and stem support costs in the growth and mortality of tropical trees. J. Ecol. 94, 670–680. doi: 10.1111/j.1365-2745.2006.01112.x
Kreuzwieser, J., and Gessler, A. (2010). Global climate change and tree nutrition: Influence of water availability. Tree Physiol. 30, 1221–1234. doi: 10.1093/treephys/tpq055
Landeiro, V. L., Bini, L. M., Costa, F. R. C., Franklin, E., Nogueira, A., De Souza, J. L. P., et al. (2012). How far can we go in simplifying biomonitoring assessments? An integrated analysis of taxonomic surrogacy, taxonomic sufficiency and numerical resolution in a megadiverse region. Ecol. Indic. 23, 366–373. doi: 10.1016/j.ecolind.2012.04.023
Larjavaara, M., and Muller-Landau, H. C. (2010). Rethinking the value of high wood density. Funct. Ecol. 24, 701–705. doi: 10.1111/j.1365-2435.2010.01698.x
Laurance, W. F., Camargo, J. L. C., Luizão, R. C. C., Laurance, S. G., Pimm, S. L., Bruna, E. M., et al. (2011). The fate of Amazonian forest fragments: A 32-year investigation. Biol. Conserv. 144, 56–67. doi: 10.1016/j.biocon.2010.09.021
Legendre, P., and Legendre, L. (1998). Numerical Ecology. second edi. Amsterdam: Elsevier Scince B.V. doi: 10.1007/978-94-017-9484-8.
Lohmann, L. (2006). Untangling the phylogeny of neotropical lianas (Bignonieae, Bignoniaceae). Am. J. Bot. 93, 304–318.
Lohmann, L. G., Kaehler, M., Fonseca, L.H.M., Farias-Singer, R., Firetti, F., Silva-Castro, M. M, et al. (2020). Bignoniaceae in Flora do Brasil 2020. Jard: Botânico do Rio Janeiro.
Luizão, R. C. C., Luizao, F. J., Paiva, R. Q., Monteiro, T. F., Sousa, L. S., and Kruijt, B. (2004). Variation of carbon and nitrogen cycling processes along a topographic gradient in a central Amazonian forest. Glob. Chang. Biol. 10, 592–600. doi: 10.1111/j.1529-8817.2003.00757.x
Magurran, A. E. (1988). Ecological Diversity and Its Measurement. Princeton: Princeton university press doi: 10.1007/978-94-015-7358-0.
Malhotra, H., Vandana, Sharma, S., and Pandey, R. (2018). “Phosphorus Nutrition: Plant Growth in Response to Deficiency and Excess,” in Plant Nutrients and Abiotic Stress Tolerance, eds M. Hasanuzzaman, M. Fujita, H. Oku, K. Nahar, and B. Hawrylak-Nowak (New York: Springer Nature Singapore Pte Ltd.), 1–590. doi: 10.1007/978-981-10-9044-8.
Malizia, A., Grau, H. R., and Lichstein, J. W. (2010). Soil phosphorus and disturbance influence liana communities in a subtropical montane forest. J. Veg. Sci. 21, 551–560. doi: 10.1111/j.1654-1103.2009.01162.x
Marques Filho, A. de O., Ribeiro, M. de N. G., Santos, H. M. Santos, J. M, et al. (1981). Estudos climatológicos da Reserva Florestal Ducke - Manaus-AM. IV. Precipitação. Acta Amaz. 11, 759–768. doi: 10.1590/1809-43921981114759
Mason, N. W. H., De Bello, F., Doležal, J., and Lepš, J. (2011). Niche overlap reveals the effects of competition, disturbance and contrasting assembly processes in experimental grassland communities. J. Ecol. 99, 788–796. doi: 10.1111/j.1365-2745.2011.01801.x
Mertens, J. (2004). The Characterization of Selected Physical and Chemical Soil Properties of the Surface Soil Layer in the “‘Reserva Ducke”’, Manaus, Brazil, with Emphasis on Their Special Distribution. [P.h.D.thesis]. Berlin: Humboldht University
Miller, E. T., Farine, D. R., and Trisos, C. H. (2017). Phylogenetic community structure metrics and null models: a review with new methods and software. Ecography 40, 461–477. doi: 10.1111/ecog.02070
Minchin, P. R. (1987). “An evaluation of the relative robustness of techniques for ecological ordination,” in Theory and Models in Vegetation Science, ed. E. Van Der Maareu (Dordrecht, The Netherlands: Springer), 89–107. doi: 10.1007/978-94-009-4061-1.
Mouillot, D., Stubbs, W., Faure, M., Dumay, O., Tomasini, J. A., Wilson, J. B., et al. (2005). Niche overlap estimates based on quantitative functional traits: A new family of non-parametric indices. Oecologia 145, 345–353. doi: 10.1007/s00442-005-0151-z
Muscarella, R., Bacon, C. D., Faurby, S., Antonelli, A., Kristiansen, S. M., Svenning, J. C., et al. (2019). Soil fertility and flood regime are correlated with phylogenetic structure of Amazonian palm communities. Ann. Bot. 123, 641–655. doi: 10.1093/aob/mcy196
Nogueira, A., Costa, F. R. C., and Castilho, C. V. (2011). Liana Abundance Patterns: The Role of Ecological Filters during Development. Biotropica 43, 442–449. doi: 10.1111/j.1744-7429.2010.00722.x
Oksanen, A. J., Blanchet, F. G., Friendly, M., Kindt, R., Legendre, P., Mcglinn, D., et al. (2019). Vegan. Encycl. Food Agric. Ethics, 2395–2396. doi: 10.1007/978-94-024-1179-9_301576.
Oliveira, A. A., Vicentini, A., Chave, J., Castanho, C. D. T., Davies, S. J., Martini, A. M. Z., et al. (2014). Habitat specialization and phylogenetic structure of tree species in a coastal Brazilian white-sand forest. J. Plant Ecol. 7, 134–144. doi: 10.1093/jpe/rtt073
Oliveira, M. L., Baccaro, F. B., Braga-Neto, R., and Magnusson, W. E. (2008). Reserva Ducke: a biodiversidade amazônica através de uma grade. Manaus, Brazil: Átema Desing. doi: 10.1007/978-3-642-41714-6_190602.
Oliveira, R. S., Costa, F. R. C., van Baalen, E., de Jonge, A., Bittencourt, P. R., Almanza, Y., et al. (2019). Embolism resistance drives the distribution of Amazonian rainforest tree species along hydro-topographic gradients. N. Phytol. 221, 1457–1465. doi: 10.1111/nph.15463
Pace, M. R., Zuntini, A. R., Lohmann, L. G., and Angyalossy, V. (2016). Phylogenetic relationships of enigmatic Sphingiphila (Bignoniaceae) based on molecular and wood anatomical data. Taxon 65, 1050–1063. doi: 10.12705/655.7
Pansonato, M. P., Costa, F. R. C., de Castilho, C. V., Carvalho, F. A., and Zuquim, G. (2013). Spatial scale or amplitude of predictors as determinants of the relative importance of environmental factors to plant community structure. Biotropica 45, 299–307. doi: 10.1111/btp.12008
Parent, C., Capelli, N., Berger, A., Crèvecoeur, M., and Dat, J. (2008). An overview of plant responses to soil waterlogging. Plant Stress 2, 20–27.
Parolin, P. (2009). Submerged in darkness: Adaptations to prolonged submergence by woody species of the Amazonian floodplains. Ann. Bot. 103, 359–376. doi: 10.1093/aob/mcn216
Parolin, P., and Wittmann, F. (2010). Struggle in the flood: tree responses to flooding stress in four tropical floodplain systems. AoB Plants 2010, 1–19. doi: 10.1093/aobpla/plq003
Pearse, W. D., Purvis, A., Cavender-Bares, J., and Helmus, M. R. (2014). “Metrics and Models of Community Phylogenetics,” in Modern Phylogenetic Comparative Methods and their Application in Evolutionary Biology, ed. L. Z. Garamszegi. Berlin: Springer-Verlag. doi: 10.1007/978-3-662-43550-2 1–552
Phillips, O. L., and Gentry, A. H. (1994). Increasing turnover through time in tropical. Science 263, 954–958. doi: 10.1126/science.263.5149.954.
Pitman, N. C. A., Terborgh, J. W., Silman, M. R., Núñez, P. V., Neill, D. A., Cerón, C. E., et al. (2001). Dominance and distribution of tree species in upper Amazonian terra firme forests. Ecology 82, 2101–2117. doi: 10.1890/0012-96582001082
Putz, F. E., and Mooney, H. A. (1991). The Biology of Vines. Cambridge: Cambridge University Press. doi: 10.5860/choice.30-0291.
Quesada, C. A., Lloyd, J., Anderson, L. O., Fyllas, N. M., Schwarz, M., and Czimczik, C. I. (2011). Soils of Amazonia with particular reference to the RAINFOR sites. Biogeosciences 8, 1415–1440. doi: 10.5194/bg-8-1415-2011
Quesada, C. A., Lloyd, J., Schwarz, M., Baker, T. R., Phillips, O. L., Patiño, S., et al. (2009). Regional and large-scale patterns in Amazon forest structure and function are mediated by variations in soil physical and chemical properties. Biogeosciences Discuss. 6, 3993–4057. doi: 10.5194/bgd-6-3993-2009
Rambaut, A., Suchard, M., and Drummond, A. (2014). TRACER v1.6. Available online at http://beast.bio.ed.ac.uk/software/tracer/ (accessed October, 2020).
Rennó, C. D., Nobre, A. D., Cuartas, L. A., Soares, J. V., Hodnett, M. G., Tomasella, J., et al. (2008). HAND, a new terrain descriptor using SRTM-DEM: Mapping terra-firme rainforest environments in Amazonia. Remote Sens. Environ. 112, 3469–3481. doi: 10.1016/j.rse.2008.03.018
Ribeiro, J. E. L. S., Hopkins, M. J. G., Vicentini, A., Sothers, C. A., Costa, M. A. S., Brito, J. M., et al. (1999). Flora da Reserva Ducke. Manaus, Brazil: Instituto Nacional de Pesquisas da Amazônia (INPA) & Department for International Development (DFID).
Ricklefs, R. E. (2005). Historical and ecological dimensions of global patterns in plant diversity. Biol. Skr. 55, 583–603.
Ricklefs, R. E., and Schluter, D. (1993). Species Diversity in Ecological Communities. Chicago, Illinois, USA: Chicago University Press.
Ricotta, C., and Podani, J. (2017). On some properties of the Bray-Curtis dissimilarity and their ecological meaning. Ecol. Complex. 31, 201–205. doi: 10.1016/j.ecocom.2017.07.003
Rocha, E. da X., Nogueira, A., Costa, F. R. C., Burnham, R., Gerolamo, C, et al. (2021). Liana functional assembly along the hydrological gradient in Central Amazonia. Res. Sq. [preprint]. doi: 10.21203/rs.3.rs-1083529/v1.
Rocha, E. X., Schietti, J., Gerolamo, C. S., Burnham, R. J., and Nogueira, A. (2020). Higher rates of liana regeneration after canopy fall drives species abundance patterns in central Amazonia. J. Ecol. 108, 1311-1321. doi: 10.1111/1365-2745.13345.
Rüger, N., Wirth, C., Wright, S. J., and Condit, R. (2012). Functional traits explain light and size response of growth rates in tropical tree species. Ecology 93, 2626–2636. doi: 10.1890/12-0622.1
Sanchez, P. A. (2019). “Soil Physical Properties,” in Properties and Management of Soils in the Tropics (Cambridge University Press: Cambridge, United Kingdom), 21–254. doi: 10.13031/2013.22670.
Schietti, J., Emilio, T., Rennó, C. D., Drucker, D. P., Costa, F. R., Nogueira, A., et al. (2014). Vertical distance from drainage drives floristic composition changes in an Amazonian rainforest. Plant Ecol. Divers. 7, 241–253. doi: 10.1080/17550874.2013.783642
Schnitzer, S. A. (2018). Testing ecological theory with lianas. N. Phytol. 220, 366–380. doi: 10.1111/nph.15431
Schnitzer, S. A., and Bongers, F. (2002). The ecology of lianas and their role in forests. Trends Ecol. Evol. 17, 223–230. doi: 10.1016/S0169-5347(02)02491-6
Schnitzer, S. A., Dalling, J. W., and Carson, W. P. (2000). The impact of lianas on tree regeneration in tropical forest canopy gaps: Evidence for an alternative pathway of gap-phase regeneration. J. Ecol. 88, 655–666. doi: 10.1046/j.1365-2745.2000.00489.x
Soares-Filho, B. S., Nepstad, D. C., Curran, L. M., Cerqueira, G. C., Garcia, R. A., Ramos, C. A., et al. (2006). Modelling conservation in the Amazon basin. Nature 440, 520–523. doi: 10.1038/nature04389
Sousa-Baena, M. S., Sinha, N. R., Hernandes-Lopes, J., and Lohmann, L. G. (2018). Convergent evolution and the diverse ontogenetic origins of tendrils in angiosperms. Front. Plant Sci. 9, 1–19. doi: 10.3389/fpls.2018.00403
Sperotto, P., Acevedo-Rodríguez, P., Vasconcelos, T. N. C., and Roque, N. (2020). Towards a Standardization of Terminology of the Climbing Habit in Plants. Bot. Rev. 86, 180–210. doi: 10.1007/s12229-020-09218-y.
Stine, R. A., and Heyse, J. F. (2001). Non-parametric estimates of overlap. Stat. Med. 20, 215–236. doi: 10.1002/1097-0258(20010130)20:2.
Toledo, J. J., Magnusson, W. E., Castilho, C. V., and Nascimento, H. E. M. (2012). Tree mode of death in Central Amazonia: Effects of soil and topography on tree mortality associated with storm disturbances. For. Ecol. Manage. 263, 253–261. doi: 10.1016/j.foreco.2011.09.017
Tuomisto, H., Ruokolainen, K., Vormisto, J., Duque, A., Sánchez, M., Paredes, V. V., et al. (2017). Effect of sampling grain on patterns of species richness and turnover in Amazonian forests. Ecography 40, 840–852. doi: 10.1111/ecog.02453.
Valiente-Banuet, A., and Verdú, M. (2013). Plant facilitation and phylogenetics. Annu. Rev. Ecol. Evol. Syst. 44, 347–366. doi: 10.1146/annurev-ecolsys-110512-135855
Vamosi, S. M., Heard, S. B., Vamosi, J. C., and Webb, C. O. (2009). Emerging patterns in the comparative analysis of phylogenetic community structure. Mol. Ecol. 18, 572–592. doi: 10.1111/j.1365-294X.2008.04001.x
Verdú, M., Rey, P. J., Alcántara, J. M., Siles, G., and Valiente-Banuet, A. (2009). Phylogenetic signatures of facilitation and competition in successional communities. J. Ecol. 97, 1171–1180. doi: 10.1111/j.1365-2745.2009.01565.x
Webb (2000). Exploring the Phylogenetic Structure of Ecological Communities: An Example for Rain Forest Trees. Am. Nat. 156:145. doi: 10.2307/3079215
Webb, C. O., Ackerly, D. D., and Kembel, S. W. (2008). Phylocom: Software for the analysis of phylogenetic community structure and trait evolution. Bioinformatics 24, 2098–2100. doi: 10.1093/bioinformatics/btn358
Webb, C. O., Ackerly, D. D., McPeek, M. A., and Donoghue, M. J. (2002). Phylogenies and community ecology. Annu. Rev. Ecol. Syst. 33, 475–505. doi: 10.1146/annurev.ecolsys.33.010802.150448
Webb, C. O., and Peart, D. R. (1999). Seedling Density Dependence Promotes Coexistence of Borean Rain Forest Trees. Ecology 80, 2006–2017.
Wiens, J. J., and Donoghue, M. J. (2004). Historical biogeography, ecology and species richness. Trends Ecol. Evol. 19, 639–644. doi: 10.1016/j.tree.2004.09.011
Wiens, J. J., and Graham, C. H. (2005). Niche conservatism: Integrating evolution, ecology, and conservation biology. Annu. Rev. Ecol. Evol. Syst. 36, 519–539. doi: 10.1146/annurev.ecolsys.36.102803.095431
Zuntini, A. R., Fonseca, L. H. M., and Lohmann, L. G. (2013). Primers for Phylogeny Reconstruction in Bignonieae (Bignoniaceae) Using Herbarium Samples. Appl. Plant Sci. 1:1300018. doi: 10.3732/apps.1300018
Zuquim, G., Tuomisto, H., Costa, F. R. C., Prado, J., Magnusson, W. E., Pimentel, T., et al. (2012). Broad Scale Distribution of Ferns and Lycophytes along Environmental Gradients in Central and Northern Amazonia, Brazil. Biotropica 44, 752–762. doi: 10.1111/j.1744-7429.2012.00880.x
Keywords: gradient analyses, habitat specialization, liana diversity, phylogenetic community structure, terra firme forest, vines
Citation: Gerolamo CS, Costa FRC, Zuntini AR, Vicentini A, Lohmann LG, Schietti J, Rocha EX, Angyalossy V and Nogueira A (2022) Hydro-Edaphic Gradient and Phylogenetic History Explain the Landscape Distribution of a Highly Diverse Clade of Lianas in the Brazilian Amazon. Front. For. Glob. Change 5:809904. doi: 10.3389/ffgc.2022.809904
Received: 05 November 2021; Accepted: 23 March 2022;
Published: 15 April 2022.
Edited by:
Stefan A. Schnitzer, Marquette University, United StatesReviewed by:
Sergio Estrada Villegas, Yale University, United StatesJulia Sfair, Federal University of Ceará, Brazil
Copyright © 2022 Gerolamo, Costa, Zuntini, Vicentini, Lohmann, Schietti, Rocha, Angyalossy and Nogueira. This is an open-access article distributed under the terms of the Creative Commons Attribution License (CC BY). The use, distribution or reproduction in other forums is permitted, provided the original author(s) and the copyright owner(s) are credited and that the original publication in this journal is cited, in accordance with accepted academic practice. No use, distribution or reproduction is permitted which does not comply with these terms.
*Correspondence: Caian Souza Gerolamo, Y2FpYW4uZ2Vyb2xhbW9AdXNwLmJy; Anselmo Nogueira, YS5ub2d1ZWlyYUB1ZmFiYy5lZHUuYnI=