- CIIDIR Oaxaca, Instituto Politécnico Nacional, Oaxaca, Mexico
Three upheavals shaped southern Mexico to Panama (SMP) biodiversity:
1. The Great American Interchange that allowed migrations between the Neotropical and the Nearctic biogeographic realms;
2. human colonization with the generation of Mesoamerican cultures; and
3. the Spaniards’ arrival and globalization.
Tectonic events generated a narrow piece of land with steep topography and high environmental heterogeneity, demanding high levels of local adaptation. Habitat size is usually restricted and reduced by frequent disturbances. Topography imposes few options for individuals forced to displace. Thus, extinction risks should be unusually high. Humans initiated an ongoing defaunation process and introduced the maize and the milpa, an itinerant maize-based slash-and-burn polyculture, which depends on revegetation to re-establish soil fertility. Also, the milpa is a most important pre-Hispanic legacy, a biocultural and landrace reservoir actively affecting landscape configuration, succession, soil development, and the genetic architecture of the species. Unprecedented human epidemics and soil, biodiversity, and culture erosion followed behind the Spanish aftermath and the subsequent globalization. > 63 million people and ≈100 ethnic groups inhabit SMP in 2020, which, with the biota, share the same problems of climate change, disturbance, and acculturation. SMP has been the scenario of severe climate change, fastest and deadliest extinction events (amphibians), a most spectacular exotic-species invasion (Africanized honeybees), and accelerated deforestation, defaunation, and acculturation. Biocultural conflicts between native and non-native people are globalization byproducts and sources of habitat destruction and species decline. Bottom-up initiatives are likely the best option for conservation in indigenous areas, whereas honest (i.e., with truly conservation intentions) top-down initiatives are helpful if the affected people are considered subjects (no objects) of conservation plans. We suggest some unique areas requiring conservation attention and analyzed current conservation initiatives. Not a single initiative is best suited for all conservation needs in SMP. Protection of all successional stages is critical for resilience and revegetation. Conservation of the milpa system (crop fields and subsequent fallows) is an optimal option for minimizing tradeoffs between conservation and people needs and safeguarding traditional culture and local landraces but is limited to areas with indigenous people and may not work for species with large home ranges.
Introduction
The biota is an essential and unique earth component. Human-triggered disturbances have accelerated extinction rates unprecedentedly, severely affecting human welfare. Proposing effective ways to ameliorate such effects requires a deep understanding of the abiotic, biological, and social components on a regional and historical base. Such components are very complex in Mesoamerica and have generated extremely rich biodiversity far from being wholly described. Standley and Steyermark (1946) estimated 8,000 plant species just for Guatemala. Currently, important biodiversity projects have been underway. Flora Mesoamericana is perhaps the most ambitious, aiming to describe all the vascular plants in this region (Anonymous, 2022). The available information about biodiversity confirms early statements suggesting that Mesoamerica is among the world’s richest biodiversity hotspots (Gaston and Williams, 1996; Thomas, 1999; Myers et al., 2000). Nevertheless, 300 endemic species of flora and fauna are threatened with extinction, of which 107 are critically endangered (Conservation International [CI], 2007). The Mesoamerica limits are arbitrary. Aside from the Yucatan Peninsula, the landscape is shaped by mountains that play a crucial role in their peculiar biodiversity and ecology. We focus our attention mainly on Southern Mexico mountain areas south of the Trans-Mexican Volcanic Belt, essentially from Puebla, Oaxaca, and Chiapas states, in Mexico, to Panama (SMP). The Trans-Mexican Volcanic Belt and the areas north of this mountain chain are different in geographic length and climate and so populous that they deserve separate analysis. The Yucatan peninsula also deserves separate analyses due to its flat terrain, distinct geology, and rich culture. This review examines the singularities of the biodiversity and conservation strategies of SMP from a historical, biophysical, and biocultural perspective.
The Intricacies of Adaptation from Southern Mexico to Panama
Adaptation in SMP mountains is particularly challenging for many species. Adaptation is an evolutionary response driven by natural selection, allowing sufficient survival, reproductive, and dispersal capacity to persist in time. However, adaptation is environmental contingent: an improvement to a particular environment usually is achieved at the expense of adaptation losses in other environments because of constraints or trade-offs (Kassen, 2002; Agrawal et al., 2010; Poisot et al., 2011). Spatial and temporal heterogeneities are expected to foster conflicting evolutionary responses because organisms usually have limited resources to perform their vital activities. Heterogeneous temporal or spatial environments (fine-grained environments) are commonly associated with generalist species, while specialization is expected in environments with few spatial or temporal changes (coarse-grained environments) (Kassen, 2002; Poisot et al., 2011). These trends should be calibrated by the dispersal capabilities of the organisms and the dispersal barriers that the habitat imposes, limiting the organisms’ displacements (O’Neill, 2001; Poisot et al., 2011). Limited migration facilitates specialization (Lenormand, 2002).
Habitat size is also a concern for conservation because it imposes the maximum limits on population size in each place. Reduced population size increases extinction risks by inbreeding, depression, loss of genetic diversity, and Allee effects (Frankham et al., 2002). Small habitat size can be caused by a steep orography generating significant environmental changes at few distances (Rull, 2011; Rahbek et al., 2019); spatiotemporal fluctuation in environmental conditions, often unpredictable, such as earthquakes (Garwood et al., 1979); anthropogenic causes, such as habitat loss and fragmentation (Fahrig, 2003); or long-term and continuous climatic events, such as global warming (Bell and Collins, 2008). The factors mentioned above, coupled with an intricate past, limited migration, and socioeconomic conflicts, make the SMP biota prone to extinction.
Physiography
Examining Myers et al. (2000) biodiversity hotspot list, three factors are relevant. First, a significant fraction of the hotspots has or are mountain areas (e.g., Tropical Andes, Central Chile, California Floristic Province, Madagascar, Mediterranean Basin, New Guinea, New Zealand, South Central China, West African Forests). Second, most are tropical (e.g., the Tropical Andes, Amazonia, Madagascar, and West African Forests). Third, some resulted from the collision of two continents (e.g., Sundaland, Hall, 2001; Indo-Burma, Uddin and Lundberg, 2004). Mesoamerica combines these three factors in a very narrow piece of land.
Mesoamerica is a dynamic geological landscape shaped by complex Earth surface processes relevant to biodiversity (Marshall, 2007). The most relevant are the tectonic collision of South America with the North American plates along a SE-NW axis; and the SW-NE collision between the Rivera, Cocos, and Nazca plates with the North American and Caribbean plates generated a subduction zone, the Middle America Trench, on the Pacific side and a very rough relief on the continent (Marshall, 2007). We used a digital elevation model to elaborate a topographic profile of different cuts along SMP to illustrate the complex SMP topography using the QGIS 3.2 software (QGIS Development Team, 2017; Figure 1). On the Atlantic side, the mountains start from an often-narrow plain coast, if any, up to 150 m wide and reaching between 70 and 100 m elevation, followed by a sharp slope change (Figure 1). Elevation (1,700 m on average) changes dramatically at short distances. Most areas (71.5%) are above 100 m elevation: Mountains usually rise below the sea level on the Pacific side. The Atlantic side could be less steep with a plain coast. Low-steepness piedmont areas can be found along the coast, as on the Mexico/Guatemala border (Love, 2007). Also, the SMP terrain is shaped in part by the rivers’ drainage (Marshall, 2007). Finally, sites above 3,000 m elevation are present but uncommon (0.6%).
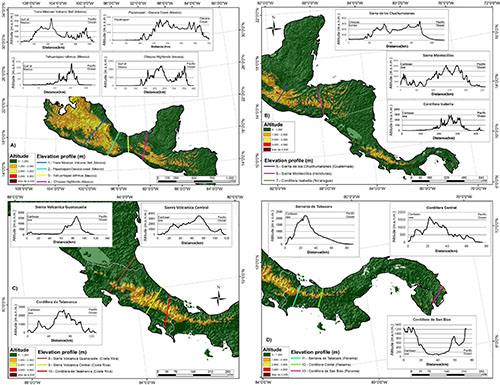
Figure 1. Maps of the SMP with width and elevation profiles at selected points. The entire area was divided in four sections: (A) Mexico, (B) Guatemala, Honduras, Salvador, and Nicaragua, (C) Costa Rica, (D) Panama.
From the above information, we can advance the following conservation recommendations. First, high elevation sites (>3,000 m) should be among the most endangered in SMP because they are uncommon and have a reduced surface. Second, the abundance of river systems (see Marshall, 2007) and the complex topography in SMP suggest vicariance and endemism in the freshwater biota as detected in fishes (Smith and Bermingham, 2005). Third, the biota of crater lakes in SMP has been developed in isolation and are abundant in endemic fishes, as in the Nicaragua crater lakes (Arkonada, 2019). Fourth, riparian ecosystems alongside waterways deserve particular attention because they are biodiversity-rich and might constitute an essential refuge for mesic flora before long-term drought events, such as climate change (Meave et al., 1991). Alnus acuminata (Betulaceae) is an example. This tree is common in humid secondary cloud forests (Velasco-Murguía et al., 2021), but it can become established in drier climates in gallery forests (e.g., Aguilar Luna, 2018).
Fifth, flat and low-steepness areas are scarce and ideal for urbanization or farming. In such places, native biodiversity should be highly endangered. Indeed, intermontane valleys are often the first places for human settlements (e.g., El Paraíso Valley, Honduras, Canuto and Bell, 2013). High fertility, high-soil moisture, and a relatively flat area make alluvial fans convenient for farming (Bull, 1968). Also, alluvial fans may generate terraces associated with distinct vegetation types that, in turn, influence ant diversity (Tehuacán Valley, Puebla, Ríos-Casanova et al., 2006). Thus, the biodiversity of alluvial fans and flat or low-steepen areas in SMP deserves more attention and conservation actions.
Climate
Macroclimate
The interaction between global climate and regional factors generates a strong climatic spatial heterogeneity in SMP. The Pacific slope is usually drier than the Caribbean slope by the winter regimes and the Caribbean low-level jet in summer (Sáenz and Durán-Quesada, 2015). This regional trend is locally modified by the complex orography producing significant climate differences at low geographical scales (Klinges and Scheffers, 2021). Mountain passes channel low-level winds and orographic rain. On the east side of the mountains, moist winds are often retained; humidity is concentrated and condensed as fog or rain partially blocking the summer humid wind flow from the Caribbean Sea, usually the primary rain source. On the opposite side, the leeward, the air is dry; the mountains’ cooling effect makes the air descend and turn warm, reducing the probability of rain (Sáenz and Durán-Quesada, 2015). Indeed, the precipitation gradient from the Caribbean Sea to the Pacific Ocean is reflected in paleoclimatic studies using δ18O isotopic records, as estimator of the surface water content, and explained by the rain shadow effect on the Pacific side (Lachniet and Patterson, 2009). Summer convective storms and mid-latitude cold fronts (“nortes”) contribute to the seasonal climatic variation (Poveda et al., 2006).
The role of vegetation on climate is important. In the tropics, vegetation modulates hydrological variability and the severity of interannual climatic variations (Poveda et al., 2006). One notorious example of the role of vegetation on climate is the low-cloud interception by tree canopies in tropical montane cloud forest areas (horizontal rain), having a significant impact on the hydrological cycle (Bruijnzeel and Proctor, 1995). The water trapped from the fog by the vegetation canopy eventually drops to the soil contributing between 2.4 and 60.6% of the total water input, based on estimations performed on cloud forests areas of the Central Cordillera of Panama (Cavelier et al., 1996). Horizontal rain can be irreversible destabilized with forest degradation in cloud forest areas (Hildebrandt and Eltahir, 2008).
Forest fires and pollutants can dramatically alter weather conditions, and their consequences may take place hundreds of kilometers away from their sources. Atmospheric pollutants such as smoke particles mainly derived from forest fires in Southern Mexico, for instance, can travel long distances causing severe weather effects in the United States, such as hail and lightning (Wang et al., 2009).
Large-scale fluctuations in air pressure between the western and eastern tropical Pacific, the southern oscillation index, generates warm events (ENSO, El Niño) or cold events (La Niña), disrupting SMP climate in complex ways (Poveda et al., 2006). These events affect ecosystems, agriculture, and the people livelihoods (Cane, 2005). However, land-surface atmospheric interactions can modulate the effects of such fluctuations. Lower evapotranspiration caused by El Niño event, for instance, can be exacerbated by deforestation reducing even more cloud production contributing to increase drought severity (Poveda et al., 2006). Thus, vegetation alterations can impact climate at local and trans-regional scales, implying that locally preserving forest ecosystems can have positive impacts at multiple spatial scales.
Long-term climate change has been so widespread in Mesoamerica and their putative effects are often dramatic, devastating, and an active area of research (e.g., Comisión Económica para América Latina y el Caribe [CEPAL], 2015). Studying climate change has been mostly implemented throughout the use of models, although the complex topography makes it difficult to provide accurate projections at local scales. Nevertheless, global warming and precipitation decreases are expected particularly during the dry season (Karmalkar et al., 2011). Climate change may interact with other climate events. The extreme 1997–1998 El Niño event caused an unusual severe drought in SMP, probably accentuated by global warming (Cai et al., 2015). In the following sections, we provide some examples of the impacts of El Niño and global warming in SMP, and their interactions with other factors.
Mesoclimate and Biodiversity
Mountain areas are tightly associated with high biodiversity, but the underlying processes are poorly known (Perrigo et al., 2020). Microclimatic variations contribute to explaining the high diversity. Slope angle, aspect, and elevation in montane areas generate tremendous spatial heterogeneity at shorter distances, affecting temperature and moisture, thus influencing species distribution, vegetation zonation, and ecosystem processes (Singh, 2018). In the tropics, such effects probably are more accentuated. Overlaps in temperature between different elevations are more reduced than in temperate areas where diurnal and seasonal variations are wider. Thus, because the tropics are less seasonal, tropical plants and animals should be adapted to a narrower temperature and elevational ranges than species living in temperate habitats (Janzen, 1967). Although not a rule (e.g., Gadek et al., 2018), this trend is common (Smith et al., 2014; Sheldon et al., 2018). The combination of very high within-site environmental homogeneity with very high-between site heterogeneity likely fostered adaptations to only a subset of all the possible habitats in SMP. Thus, SMP species may face tradeoffs associated with climate, such as surviving moist areas or displaying drought adaptations to dry areas, or adapting to a warm environment in the lowlands or a cool climate in the highlands. Tropical plants may face these tradeoffs even at short distances favoring endemism. Indeed, the frequency of endemisms is high, as in tropical montane cloud forests (Rzedowski, 1991). Therefore, even if abundant in their habitats, species in the neotropics often do not have extended distributional ranges (e.g., Lieberman et al., 1996; Williams et al., 2010).
One consequence of this specialization is the diverse ecosystems of SMP mountains, likely climate-related, and often related to elevation (e.g., Taylor, 1963). Many vegetation classification systems have been suggested (e.g., Velázquez et al., 2016). Holdridge (1967) proposed a simple way to classify world life zones based on temperature, precipitation, and expected climax vegetation. Except for very extreme habitats, such as tundra or desert, all world plant formations described by Holdridge (1967) are present in SMP mountains (e.g., Rzedowski, 1978; Greller, 2000) and likely are explained by the wide climatic variation described above. Most vegetation types in SMP are tree-dominated. The height of the vegetation and the fraction of trees that lost their leaves during the dry season have been helpful for classification purposes. Evergreen tropical rain forests prevail in moist lowland areas characterized by their highest biodiversity and tallest trees. As elevation increases, sub-montane or montane cloud forests with a shorter stature are typical vegetation on the humid side of the mountains. At very high elevations, shorter alpine and paramo vegetations can be found. The deciduousness of the vegetation often increases as annual rainfall decreases. Tropical deciduous dry forests or scrublands occupy dry lowlands, and their composition is often linked to the substrate, as seen below. In contrast, pine, pine-oak forests, or shrublands are often common in less humid areas at mid-elevations (Taylor, 1963; Sawyer and Lindsey, 1971; Rzedowski, 1978). Because of the high rate of anthropogenic and natural disturbances, it is not always easy to discover what the original vegetation was.
Mountains display a high β floristic diversity between distinct elevation belts, in agreement with Janzen’s (1967) statement (e.g., Lieberman et al., 1996; Zacarías-Eslava and del Castillo, 2010; Salas-Morales and Meave, 2012; Ramirez-Bautista and Williams, 2018; Martin et al., 2021).
Slope aspect effects can be significant for plants and vegetation in tropical areas, as reviewed by Singh (2018). These effects are expected to be more severe as steepness increases, and north-facing slopes are cooler and wetter in the Northern Hemisphere than south-facing slopes (Holland and Steyn, 1975). Slope aspect effects have been detected even at a low scale (10–100’s of m) in a tropical dry forest in Central Valleys, Oaxaca, where succulent plants displayed the lowest floristic similarity between north and south slopes (Luis-Martínez et al., 2020). Considering slope orientation has been helpful for forest protection. For example, in Yeguare, Honduras, the slope aspect is essential regarding fire risks, which tend to be highest on south-facing slopes (Cáceres, 2011).
High specialization in environmental conditions associated with elevation helps explain the vegetational altitudinal displacements detected by paleoclimatic studies. In the Cordillera de Talamanca, Costa Rica, the tree line displayed altitudinal shifts following the warming and cooling cycles of the last glaciations (Hooghiemstra et al., 1992). Fluctuating glacial and interglacial climates brought about changes in species ranges resulting in endemism and disjunctions (e.g., Wendt, 1987; Nixon, 2006). Such fluctuations may have left a genetic signature: reductions in population size associated with habitat cutbacks significantly reducing population genetic variation (Allendorf and Luikart, 2007). For example, Pinus chiapensis of Holarctic origin and endemic to southern Mexico and Guatemala (Farjon, 2018), shows an unusually low population genetic diversity compared with other pine species, including its nearest closest extant relatives: the North American white pines, P. monticola and P. strobus (Syring et al., 2007). Genetic bottlenecks derived from glacial fluctuations and anthropogenic habitat losses may explain this result (del Castillo et al., 2011).
Quaternary climatic oscillations also affected species of Gondwana (southern hemisphere) origin. The highland Podocarpus matudae and P. oleifolius (Podocarpaceae) displayed strong evidence of population expansion during the coldest last glacial maximum; following glacial retreat, the populations contracted and became fragmented (Ornelas et al., 2019). The small population size of species whose populations declined after the last glaciation, coupled with current global warming and habitat destruction, likely put these species at higher extinction rates. Population size is correlated with genetic diversity, the raw material for adaptation (Allendorf and Luikart, 2007).
Microclimate, Microcosms, and Biodiversity
The complex three-dimensional variation observed in the mountains takes place in trees at a lower scale. The tree canopy creates multiple niches for various organisms (Ozanne et al., 2003). By being the SMP vegetation tree-dominated, the arboreal component of biodiversity is crucial. Batke et al. (2016) estimate 2,781 species of flowering plant epiphytes for Central America, likely contributing to ∼20% of the vascular flora based on Cusuco National Park, Honduras, assessments. The extraordinary capacity of forest canopies to retain humidity and maintain a rich epiphyte community provides an additional reason for forest protection.
Arboreal organisms are small-scale ecosystems that may sustain multitrophic species assemblages. SMP harbors notable examples. The unusual pelage of the two-fingered (Choloepus spp., Choloepodidae) and three-fingered (Bradypus spp., Bradypodidae) sloths maintains a large community of epibionts including fungi, Cyanobacteria, algae, beetles that feed upon the algae and moths whose larvae consume the sloth dung; birds consumed some of such insects (Kaup et al., 2021). Tank epiphytes are another example: their rosette stores water and generate a micro-ecosystem. For instance, five Tillandsia (Bromeliaceae) species are associated with the lizard Abronia oaxacae (Anguidae), which feed upon insects that live in their tanks in Oaxacan temperate forests of Cruz-Ruiz et al. (2012).
Soil
Soil is essential in defining vegetation types and biodiversity. Soil properties are strongly correlated with climate variations associated with elevation, as detected in montane oak forest gradients in Costa Rica (Kappelle and van Uffelen, 2006). Even at the same elevation, soil dramatically changes. The thickness of the O horizon increased, whereas soil pH and N/P ratio decreased toward older stands in a tropical montane cloud forest area (Bautista-Cruz and del Castillo, 2005). Soil pH was found to explain species discontinuities of cloud forest species in Sierra Norte, Oaxaca (Domínguez-Yescas et al., 2020). Soil properties depend on the surface lithology, which in SMP is among the world’s most complex (Marshall, 2007). For instance, pine forests in west-central Honduras are abundant on thin soils derived from ultramafic rocks (Greller, 2000).
Igneous soils derived from volcanic activity or schists may occur close to limestone soils derived from calcium carbonate outcrops and platforms (Marshall, 2007). Limestone soils are chemically and physically different from those derived from siliceous rocks, often more acidic. First, they are more water-permeable, creating drier conditions than siliceous soils (Larcher, 1983). The edaphic aridity that generates limestone outcrops was evident by their higher proportion of xerophytic plants than that observed in adjacent vegetation growing on schist-derived soils (Nizanda, Oaxaca, Pérez-García and Meave, 2005). Second, high concentrations of CaCO3 make calcareous soils very alkaline, imposing severe nutrient limitations for plants relative to soils derived from siliceous rocks with neutral or slightly acidic pH (Taiz et al., 2015). Litter production releases organic acids buffering the alkalinity of limestone-derived soils, thus reducing pH differences between calcareous and siliceous soils toward more humid areas. Not surprisingly, the vegetation in dry areas is very different even under the same climate when the geological substrate changes (e.g., Rzedowski, 1978).
Species’ soil preference in semiarid habitats is a relatively well-known phenomenon (Goettsch and Hernández, 2006). The Tehuacán-Cuicatlán Valley (Puebla, Oaxaca) abundant in geological substrates (Mendoza-Rosales et al., 2013), endemisms, and the world richest columnar cactus diversity is an example (Dávila et al., 2002). Many of such species are endemic and edaphic specialists. Cephalocereus columna trajani, Mitrocereus fulviceps, and Neobuxbaumia mezcalaensis are examples of calcicolous species, whereas Pachycereus weberi, Polaskia chende grow on siliceous soils (Meyrán-García, 1973; del Castillo, 1996; Arias-Montes et al., 1997). At least 90 bird species, of which 56 are resident, have been found in columnar cactus forests in this valley (Arizmendi and de Los Monteros, 1996). Climate change and habitat destruction may severely impact the Tehuacán-Cuicatlán biota, particularly low mobility species or edaphic-specialist species. The complex orography and limited areas with similar edaphic conditions significantly reduce the opportunities for finding a similar place elsewhere.
Migration Options Are Restricted From Souhtern Mexico to Panama
The SMP complex orography in a very narrow piece of land and its SE-NW oriented continental main axis generates a dispersal bottleneck (Figure 1). These factors may account for the limited detected migration opportunities between North and South American landmasses, particularly the few historical human cultural contacts and diffusion of domesticated species (Diamond, 1999). For a North American species adapted to mid-elevations, migrating to South America would require passing to tropical areas with a warmer climate and few migration options.
Other geographic accidents in SMP may also function as dispersal barriers generating a phylogeographic structure, even those with flying capabilities. One example is the Nicaraguan Depression, a gene flow obstacle to the spot-crowned woodcreeper, Lepidocolaptes affinis (Furnariidae) (Arbelaez-Cortés et al., 2010). Limited gene flow and high spatial heterogeneity may explain SMP mountains’ great diversity and endemism. For instance, dispersal limitations could explain why the fresh-water fish species richness of small ranges exceeded large range fishes in south Mesoamerica (Smith and Bermingham, 2005). The Tehuantepec Isthmus was likely a significant source of lineage divergence and geographic discontinuity among selected plant, bird, and rodent species whose ranges are separated by this narrow piece of land (Ornelas et al., 2013). Topography complexity is not only an obstacle to migration and gene flow but also to cultural transmission as expressed in language (Cavalli-Sforza and Feldman, 1981). The given Zapotec names given to Pinus chiapensis (Pinaceae) in Sierra Norte, Oaxaca changes from village to village, separated a few kilometers from each other: Suy du do, Ya yieri do, Ya guieri do, Ya guir do, (approximate spelling) revealing a sort of linguistic drift akin to genetic drift (del Castillo and Acosta-Castellanos, 2002).
If species specialization is favored when the probability of a successful migration is reduced, then the frequency of specialists should increase with altitude. At high elevations, finding another similar or higher elevation place should decrease because high altitudinal belts are uncommon (Figure 1). The frequency of amphibian endemism, for instance, is higher in Mesoamerican uplands (Lips et al., 2005).
Migration is often the species’ last resource before climate change. Temperature tends to decrease with both latitude and altitude. Thus, migration to higher latitudes or altitudes is a typical response for many plants and animals affected by global warming (Parmesan and Yohe, 2003; Colwell et al., 2008; Wilson and Gutiérrez, 2012). The common assumption that migration is an easy task should not take for granted. Latitudinal displacements fostered by global warming are prone to be bottlenecked by the orography and climate in SMP (Figure 1). For example, for an endemic species of the Chiapas Highlands affected by global warming, migrating to higher latitudes requires first passing to the low and warm areas of the Tehuantepec Isthmus before finding cooler northern regions. Global warming has forced the upward migration of species, such as Pinus patula, which displays morphological and physiological evidence of better performance at elevations higher than those at which this species is commonly distributed (del Castillo et al., 2020).
Furthermore, upslope displacement implies a higher exposure to UV-B light, which is more elevated at tropical latitudes (Caldwell et al., 1980). UV-B light induces mutagenic and cytotoxic DNA lesions (Rastogi et al., 2010). The mutagenic impact of displacements to upland areas needs to be studied but likely accelerates extinction rates. The low availability of migration routes and the lack or scarcity of better alternatives to become established reinforce the relevance of implementing conservation actions at high elevation areas.
Natural Disturbances
Disturbance events may permanently or temporarily disable the affected land to be eventually colonized by the original species, reducing the potential sites that a species can occupy. The expected distributional response to global warming based only on thermal biology overestimates the potential area that the focus species can occupy in the future (see Peterson et al., 2011). Considering such an overestimate is particularly relevant in SMP since disturbances are common in SMP. Regardless of their origin, human activities usually magnify disturbance effects.
Earthquakes, Hurricanes, Landslides, and Volcanoes
SMP is a seismic area. Consequently, earthquakes are common and critical stochastic events disrupting the biota. Steep slopes triggering landslides often magnify earthquake effects with disastrous consequences (Malamud et al., 2004). Land cover change by landslides in SMP mountains is significant and challenging to estimate (Restrepo and Alvarez, 2006). In 1976, earthquake landslides denuded 54 km2 of tropical areas in Panama (Garwood et al., 1979). Also, landslide effects interact with rainfall and soil type (Bommer and Rodríguez, 2002). Hurricane Mitch generated more than 500,000 landslides in Honduras, with a high death toll and economic impacts (Harp et al., 2002; Perotto-Baldiviezo et al., 2004). Landslides are frequent on slopes higher than 40% (Figure 2); however, slope instability caused by deforestation magnifies landslide effects (Alcántara-Ayala et al., 2006). Landslide damages may not be permanent, but revegetation is slower than in less-steep slopes, even in humid areas (Dalling, 1994).
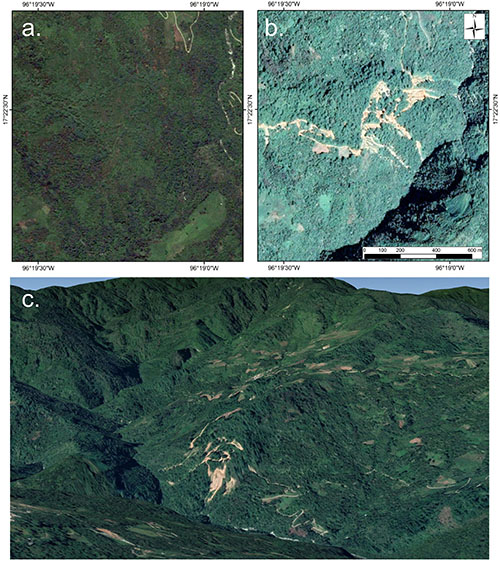
Figure 2. A landslide in Sierra Norte, Oaxaca, Mexico as detected by satellite images (Google Earth®). (a) Before (2014), and (b,c) after the landslide (2019).
Eruptive activity is a common disturbance in SMP tightly linked to earthquakes. Its multiple manifestations involve lava effusions, gas-and-ash explosions, pyroclastic density currents, and lahars, as in Guatemala’s Santiaguito lava-dome complex (Lamb et al., 2019). The destructive effects of lahars and pyroclastic density currents was detected by changes in the normalized difference vegetation index (NDVI) before and after the Santiaguito volcanic event; the sediment deposits affected tens of km2 of land (Harris et al., 2006). Due to episodic releases of ashes, volcanic eruptions significantly decreased tree survival and growth near the Tacaná Volcano, Guatemala-Mexico border (Allende et al., 2020). The ashes and hot gases released often kill or damage the plants (e.g., Poas volcano, Costa Rica, Figure 3). Unusual baldness probably caused by volcanic ash ingestion was observed in the spear-nosed bat (Phyllostomus hastatus, Phyllostomidae) (Masca, Honduras, Divoll and Buck, 2013).
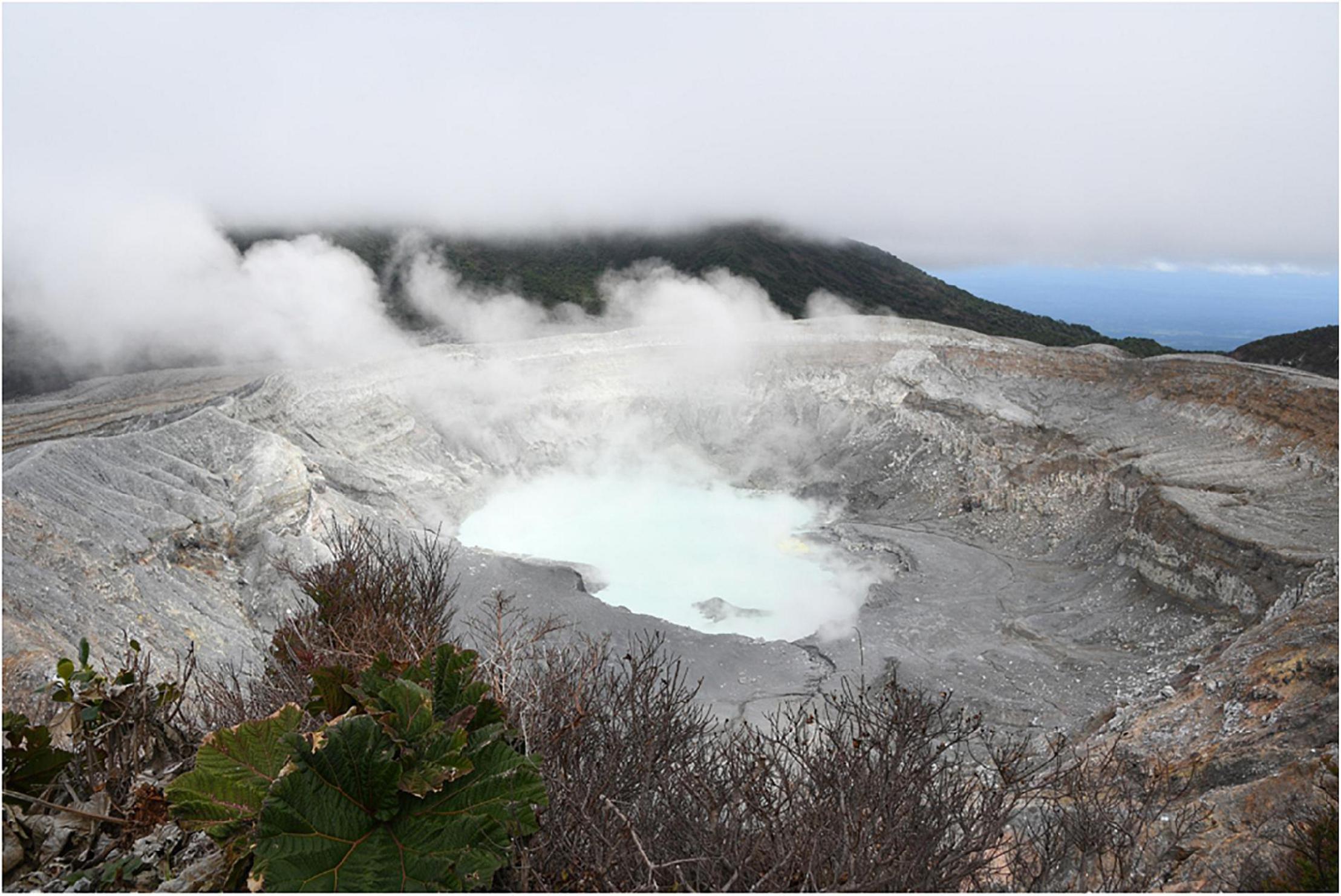
Figure 3. Poas volcano crater and plants damaged by the volcanic activity, Costa Rica (photo by R.F. del Castillo).
Strong winds often associated with hurricanes are common in SMP and interact with other disturbance factors such as landslides. The complex orography can locally increase wind speed (Wagenbrenner et al., 2016). The resulting power of the winds can destroy significant vegetation areas. This little-studied phenomenon in Sierra Norte, Oaxaca, Mexico, affected upper sections of the mountains’ east side in 2016 (Figure 4).
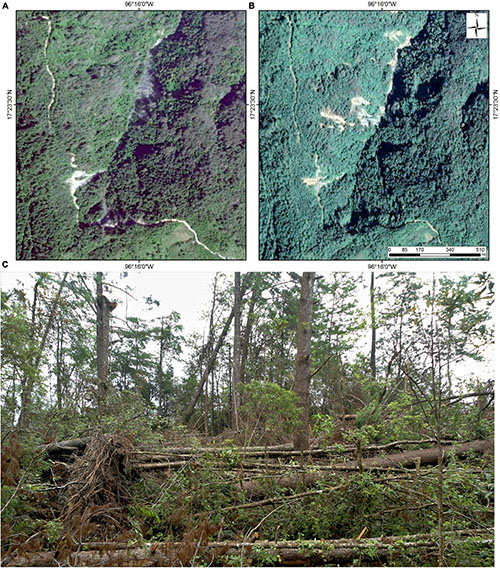
Figure 4. Satellite images taken before (2014) (A), and after a gust that took place on March 9th, 2016 (B). (C) in situ photograph of one of the affected sites in Sierra Norte, Oaxaca (photograph by Hermilo Martínez). Several sites were simultaneously affected by this strong wind in Sierra Norte.
Fires
Forest fires are frequent and often very destructive in SMP. The abundance of fire-adapted species suggests that fires are common in some semidry environments. In a frequently burned stand of Pinus oocarpa forest in Honduras, Clewell (1973) reported 124 species of flowering plants. High intensity fires are associated with abundant seedling density of this pine (Sola de Vega, Oaxaca, Juárez-Martínez and Rodríguez-Trejo, 2003). Furthermore, prescribed low-intensity burnings may reduce fire-hazards (Hudson et al., 1983). Nevertheless, not all semi-dry areas in SMP are rich in fire-adapted species, as the Guanacaste dry forest, in which case, fires can be disastrous. The high frequency of fires in this forest appears to be anthropogenic and linked to agricultural practices. In this case, fire controlling is essential for biodiversity conservation (Moline, 1999). Uncontrolled fires are a significant concern because of their high destructive power, outstanding aerosols, and CO2 released into the atmosphere. Fire frequency significantly increased from 1995 to 2020 in Puebla, Oaxaca, and Chiapas (Comisión Nacional Forestal [CONAFOR] and Secretaría de Medio Ambiente y Recursos Naturales [SEMARNAT], 2021; Figure 5). Climate-related factors, including climate change, often exacerbated by human activities, could explain these increases.
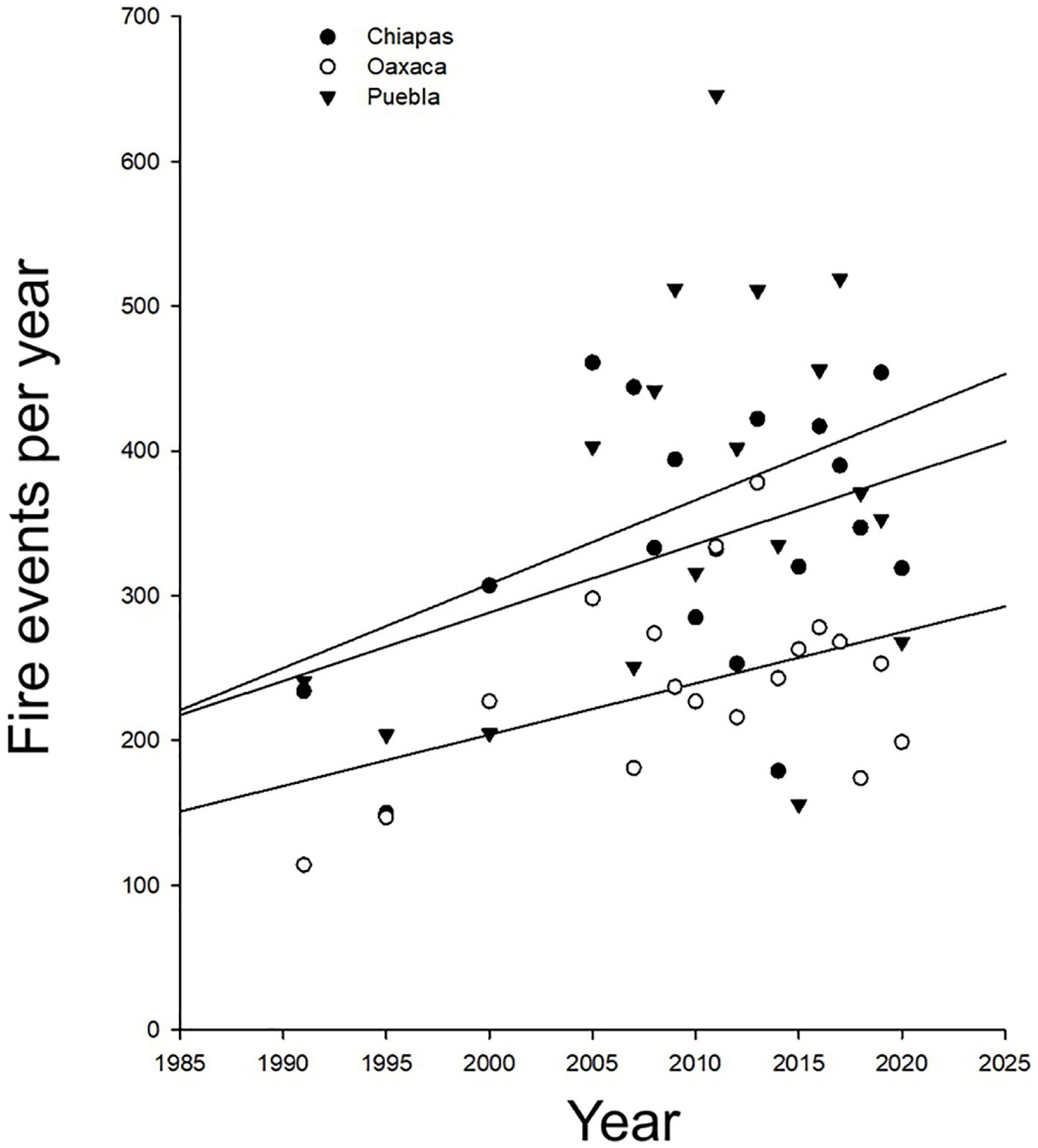
Figure 5. Forest fires per year in the three mountainous states of southern Mexico: Puebla, Oaxaca, and Chiapas from 1995 to 2020. Data from Comisión Nacional Forestal [CONAFOR], and Secretaría de Medio Ambiente y Recursos Naturales [SEMANART] (2021). Fire frequency has significantly increased in recent decades, from 1995 to 2020, in Puebla, Oaxaca, and Chiapas [F(1, 50) = 10.26, P = 0.002]. Fire incidence was significantly different among these states [F(2, 50) = 9.11, P = 0.0004]. However, the non-significant state-by-year interaction suggests that common factors act in similar ways in the observed increase in fire events in these states.
Drought can trigger and amplify fire events. So far, the worst year in forest fires was in 1998 in northern SMP (Comisión Nacional Forestal [CONAFOR] and Secretaría de Medio Ambiente y Recursos Naturales [SEMARNAT], 2021). This year was anomalous dry owing to the 1997–98 El Niño event and is probably associated with an unusual increase in atmospheric CO2 (Zeng et al., 2005). Under extreme climatic events, fires can be catastrophic in humid areas. Drought transformed the high amounts of litter accumulated by the slow decomposition rates in the humid forests’ acid soils into a potent fuel that can become easily ignited, as detected in a tropical montane cloud forest, by Asbjornsen et al. (2005, Chimalapas, Oaxaca). The 1997–98 El Niño event likely triggered fires that destroyed 82–100% of the above-ground tree biomass in the areas they studied, being the elfin forest the most impacted. Fine-root live biomass was more affected in plants growing on the sedimentary than on metamorphic substrates (Asbjornsen et al., 2005). Thus, the effects of same disturbance event can significantly vary at local scales.
Pioneer Plants and Secondary Vegetation
Natural and anthropogenic disturbance events are inherent to SMP. Thus, preserving the biota involved in naturally restoring disturbed areas is essential for conservation. Successional ecosystems, such as landslides and lava domes, are included in the Nicaraguan FAO ecosystem classification (Arkonada, 2019). Secondary vegetation assures ecosystem recovery before disturbance events. Often, few species are common between young and old-growth stands suggesting that disturbances are a source of mortality for some species and establishment for others (Denslow, 1980). Seed germination can be accelerated after deforestation in an old forest in Costa Rica (Young et al., 1987) or in open seral stages or forest gaps in Chiapas (González-Espinosa et al., 2006).
It is easy to assume that pioneer plants have few ecological requirements owing to their capability to become established in open places. Nevertheless, climate change and habitat destruction may increase their extinction risks. Colonization potential was associated with heterozygosity, genetic diversity, and population size in the pioneer pine Pinus chiapensis, Pinaceae (del Castillo et al., 2011), some of whose small populations are at high extinction risk (e.g., Martínez-Carrasco, 1998). Lack of ectomycorrhizal fungi (EMF) in soil deter the establishment of pine species in open areas, requiring EMF inoculation from forest soils (e.g., Sánchez-Montalvo et al., 2005). Also, seed richness in early successional depends on seeds dispersed from nearby stands in Costa Rica (Young et al., 1987). Chiapas highlands’ late-successional stage forests may harbor remarkable diversity of EMF fungi, displaying high turnover rates between nearby sites suggesting the importance of older forests as EMF reservoirs (Pérez-López et al., 2021). Consequently, successful revegetation should depend on two factors: First, intrinsic characteristics of potential colonizers, such as their viability as influenced by genetic factors, and second, habitat configuration, through the spatial distribution of nearby sites as suppliers of essential seeds and spores. Patches of young forests scattered in the landscape can maintain rare habitats through high stand turnover rates (Denslow, 1995).
Secondary neotropical forests have a relatively high resilience (Derroire et al., 2016; Poorter et al., 2016, 2021). Fostering early successional and old-growth forests offers the best balance between biodiversity conservation and landscape resilience. Vegetation recovery is affected by opposing factors such as disturbance frequencies, tending to decrease biodiversity and equitability, and soil seed bank inputs which tend to decrease with the age of successional stands (Young et al., 1987; Poorter et al., 2021). Thus, not a simple and universal method is likely effective for conserving frequently disturbed areas. Disturbance administration can be an option helping to protect biodiversity. For example, prescribed fires may reduce the intensity of other and more destructive fires and increase soil fertility (Nigh and Diemont, 2013; Alcañiz et al., 2018). A profound understanding of the successional process in SMP mountains is urgently needed, also providing an excellent opportunity for ecologists to have deep insights into such an important phenomenon.
Historical Upheavels
The Great American Interchange
Several Neogene tectonic events shaped the complex biogeography of Mesoamerica and its rich biodiversity (Rull, 2011). The northward displacement of South America that eventually joined North America through the Panama Isthmus in the Pliocene, the Great American Interchange, facilitated the encounter between the Neotropical and the Nearctic biogeographical realms, resulting in an impressive exchange of flora and fauna between the two subcontinents (Cox et al., 2019). This exchange generated an exceedingly high regional species pool (sensu Cornell and Harrison, 2014). The combination of high diversity in a heterogeneous habitat fosters more opportunities for species interactions and, thus, the generation of new niches, as Darwin (1859) and Wallace (1878) first noticed.
Climate change, particularly during glaciations and interglacial periods, played a significant role in the observed asymmetrical outcomes in mammalian exchanges when the land bridge was opened. During the humid interglacial period, in the late Pliocene, rainforests prevailed, facilitating south-north migrations from Amazonia into SMP. Through the following dry and cold glaciation period, savannas extended to tropical latitudes, and mammalian migration from North America outnumbered those from the south, probably because the temperate source area was larger than the south area (Webb, 1991). Also, invading North American carnivorous mammals spread into SMP more than their South American marsupial counterparts, probably because they were more effective predators (Leigh et al., 2014). In birds, migration across the land bridge mainly was from south to north (Weir et al., 2009). In plants, ecological and phylogenetic analyses rather than paleontological records are used because of the paucity of plant fossils. Early studies suggest that plant exchanges between North American and South America were not as sharply outlined as birds and mammals (Simpson and Neff, 1985). A recent molecular phylogenetic meta-analysis revealed that angiosperms dispersed earlier than animals, before the isthmus of Panama was closed (Cody et al., 2010). Similarly, the divergence between North American and South American Diadasia bees (Apidae) took place before the formation of the Panama Isthmus and is consistent with that of angiosperms, based on phylogenetical studies (Wilson et al., 2014). The contrasting migration patterns detected among the studied taxa and the lack of information of many others warn about generalizing and urge more data and integrative research to explain the origin and diversification of the incomparable Neotropical biodiversity (Antonelli et al., 2018).
Human Colonization
Humans should severely impact the SMP mountain pre-Columbian environment. Early evidence of human settlements in SMP was found in Santa Martha, Chiapas ∼11,340–11,280 BP, during the Pleistocene/Holocene boundary by Solís-Torres et al. (2021). The authors argue that the diversity of tropical ecosystems and species facilitated successful foraging and eventual transitions to agriculture. The same statement can be applied to most SMP, including semiarid areas (e.g., Valley of Oaxaca, Feinman and Nicholas, 2020). Many plants, including maize, squash, and beans, were poly-cultivated, as revealed by palaeoecological studies in Joya de Cerén, Salvador (Slotten et al., 2020). Still, more studies and multiple approaches are needed to provide accurate quantitative assessments of such impacts in Mesoamerica (Harvey et al., 2021a) and to understand the complex biocultural interactions that caused the rise and decline of pre-Columbian human settlements (Castanet et al., 2022).
Defaunation
Anthropogenic species extinction likely started very early after human colonization in SMP. Large animals characterized by low natality rates (small offspring number and longer time to attain reproductive maturity) are most threatened (cf., Begon et al., 1996). Radiocarbon dating studies conducted at La Estanzuela, Guatemala, suggest the possibility that the giant extinct gomphothere (Cuvieronius hyodon, Gomphotheriidae) coexisted with humans (Dávila et al., 2019). Also, the North-South displacements of early human colonizers through the American continent coincide with transgressive declines of the extinct megafauna consistent with Martin’s (1973) overkill hypothesis (Surovell et al., 2016). The invention of agriculture might boost the megafauna extinction (Alford, 1970).
Ethnic Diversity
As of 2020, the total human population of SMP comprised > 63 million people and ∼100 ethnic groups (Table 1). The ethnic diversity of SMP can be explained by: (a) the long occupation history; (b) the complex orography hindering migration, as migration routes were essential for agriculture dissemination (Zizumbo-Villarreal and Colunga-GarcíaMarín, 2010; González-Martín et al., 2015); and (c) habitat heterogeneity demanding local specific survival adaptations. These factors may have fostered habitat specialization by human groups in this coarse-grained environment, just as expected for any other species. Despite such a high ethnic diversity, most SMP ethnic groups have in common the maize (Zea mays: Poaceae) as the base of their subsistence (e.g., Boege, 2008; Hünemeier et al., 2012). By comparing modern words of related indigenous languages, Brown (2010) estimates that before 7,000 BP, maize and other managed plants became important enough to receive specific names by the speakers of Mesoamerican highlands.
Maize and the Milpa System
The Invention of Maize and Milpas
Maize cultivation in SMP has millennial roots: the eldest palaeoecological record stretches back to 6,250 BP in the Guilá-Naquitz Cave, Oaxaca (Benz, 2001; Piperno and Flannery, 2001). Also, ancient vestiges of maize cultivation have been detected throughout SMP: Panama (Piperno et al., 1985), Costa Rica and Nicaragua (Horn, 2016), Salvador (Dull, 2016), Honduras (Kennett et al., 2017), Guatemala (Castanet et al., 2022), Chiapas (Hammond, 2001; Rosenswig et al., 2015), and Puebla (Long and Fritz, 2001; Torres-Rodríguez et al., 2018). The presence of maize in palaeoecological records correlates with human genomic changes, sedentary lifestyle development, and farming techniques (Hünemeier et al., 2012). The traditional way of maize cultivation, the milpa, has been practiced by indigenous farmers till the present, giving a clue to how maize was cultivated during Pre-Columbian times (Horst, 1989; Bellon et al., 2018). Traditional milpa practices currently involve slashing and burning 1–2 Ha of forest fragments, little or null use of agrochemicals, no or few plugging, and long fallow periods (e.g., Pérez-García and del Castillo, 2016). A similar process of forest clearing and burning for maize cultivation was practiced during > 3,000 years in SMP as detected by palaeoecological studies (e.g., near Las Cruces Biological Station, Costa Rica, Clement and Horn, 2001).
The milpa is often defined as an itinerant, slash-and-burn, rainfed polyculture in which maize is cultivated with beans (Phaseolus spp., Fabaceae), squashes (Cucurbita spp., Cucurbitaceae), and other crops, with the tolerance of semi-domesticated and wild species, depending on the region (e.g., Altieri et al., 2012). It is a system of continuous improvement, locally adapted based on the conditions of the farmers’ territory: sowing time and cultivated landraces depend on elevation, rainfall, and soil, even within the same mountain range (e.g., González, 2001). The farmer selects seeds from plants in their field during each cultivation cycle with the desired qualities for the next cultivation cycle (e.g., González, 2001). Since maize is an annual plant, the domestication process has been continued for several thousands of selection rounds, likely explaining the differences in the maize genome compared with those of their closest extant wild relatives, the teosinte (Zea mays ssp.) (Tian et al., 2009). Also, genetic drift and founder events, through historical population contractions and expansions and genetic exchange between teosinte, shaped the maize genome (Wang et al., 2017). Consequently, the maize landrace diversity is enormous and distributed over a wide array of populations as this crop is broadly cultivated in highly heterogenous Mesoamerica (Sánchez et al., 2000). However, novel techniques involving genotyping and phenotyping are needed to fully characterize the great variety of landraces (Prasanna, 2012).
Pedogenetic Processes
The milpa system generates a cycle of soil acidification during revegetation and soil de-acidification during vegetation burning (Bautista-Cruz and del Castillo, 2005). Soil acidification may contribute to the release of nutrients to the soil from the parent material, while vegetation burning makes them available to crop plants by increasing soil pH. By not relying on chemical fertilizers, soil nutrients in milpas are often depleted after 2–4 cultivation cycles. Then, a piece of forestland is slashed and burned to initiate a new cropping cycle elsewhere (Boege, 2008; Toledo et al., 2019). The pace of soil recovery is slow and may take between ∼45 and > 100 years after the cultivation phase in tropical montane cloud forest areas, depending on the soil property (Bautista-Cruz and del Castillo, 2005). Therefore, the milpa requires large areas of secondary forest stands for soil recovery (Watters, 1971).
Secondary Succession
Soil recovery is associated with long-lasting revegetation generating fragments of successional stages of different ages in the landscape. Guariguata and Ostertag (2001) provided the general patterns of secondary succession in the Neotropics, which basically are the same as those observed in post-milpa fallows. Only a few highlights are shown here. Herbs, shrubs, and tree saplings dominate the first vegetation stage, commonly called acahual. Traditional milpas that do not use plowing leave tree stumps from the previous fallow, enhancing a rapid revegetation (Pérez-García and del Castillo, 2017). The acahual is eventually replaced by pioneer heliophilous tree species, constituting the earlier forest stage, commonly dominated by pines (Pinus spp.) (González-Espinosa et al., 1991, 2000; Almazán-Núñez et al., 2016; Velasco-Murguía et al., 2021), probably because pines are pioneer trees distributed from the sea level to > 3,000 m elevation from dry to humid areas north of Costa Rica in SMP (Farjon, 2018). Alternatively, other tropical trees are abundant in the first arboreal successional stage, such as the balsa tree Ochroma pyramidale (Malvaceae) (low humid lands, Vleut et al., 2013) or Mimosa acantholoba: Mimosaceae, in lowland dry forest (Lebrija-Trejos et al., 2008). Pioneer species often facilitate (sensu Connell and Slatyer, 1977) the establishing of shade-tolerant species dominated by angiosperm trees (e.g., González-Espinosa et al., 1991, 2006; de la Luz Avendaño-Yáñez et al., 2014; Almazán-Núñez et al., 2016; Velasco-Murguía et al., 2021). Tropical ecosystems, such as dry forests, are resilient before disturbances but with a slow recovery rate in species composition (Derroire et al., 2016).
Landscape Configuration Changes
SMP landscapes appear to result from two opposing factors: common and diverse disturbance events and the subsequent revegetation. Assuming that most disturbances were localized, pre-human landscapes in SMP should be mainly composed of late-successional forests and, at a lower frequency, different fragments of early and mid-successional stages resulting from past disturbances and recently disturbed areas (Figure 6A). Two significant events altered this successional dynamic: first, the colonization and dissemination of indigenous groups into virtually all Mesoamerica; second, the Spaniards’ introduction of new species and land-use methods.
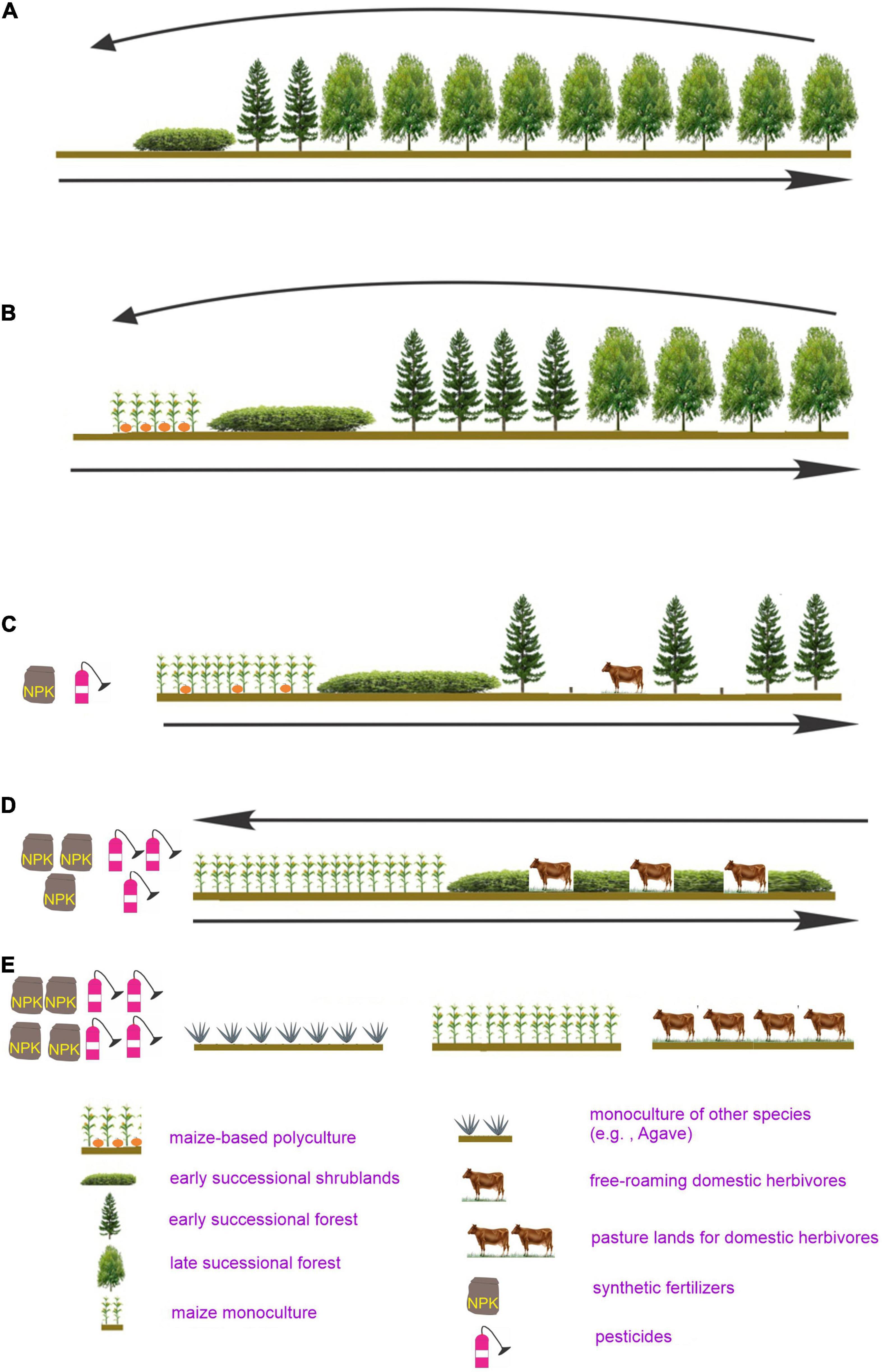
Figure 6. Successional changes expected in the SMP mountains. (A) Before human occupation. (B) After the human occupation before the Spanish conquest, and in contemporary-traditional milpas. In the Spanish aftermath, the simplification of the successional process became increasingly common and exacerbated by human population growth, resulting in (C) the elimination or reduction of old-growth forests and the dominance of fallows composed of early successional forests and successional shrublands (acahuales), (D) the elimination or reduction of pioneer forests and the prevalence of acahuales as the main fallow land. Finally (E), the elimination of acahaules and their replacement be maize fields and other agricultural lands, accompanied by the increasing use of agrochemicals.
Milpa itinerancy and the long-lasting successional processes required for soil recovery alter the pre-human SMP landscape in three ways: (a) introducing cropland fragments; (b) increasing early successional shrublands and forest fallow lands, and (c) reducing old-growth forestlands (Figure 6B). Thus, the landscape was transformed into a checkerboard of field crops and associated fallows that constitute the milpa system (Figure 7). Landscape patchiness generates diverse niches for many species, as observed in other regions (e.g., Fuhlendorf et al., 2006). Indeed, the fraction of shared species among different-aged fallows is meager (e.g., González-Espinosa et al., 2006). Species of small non-flying mammals have different peaks of abundance at distinct successional stages (e.g., Santos Moreno, 2008). The species richness of shrubs, geophytes, and other low plants peaks very early during succession, in contrast to lianas and trees, which peak at later successional stages in cloud forest areas (del Castillo and Blanco-Macías, 2007).
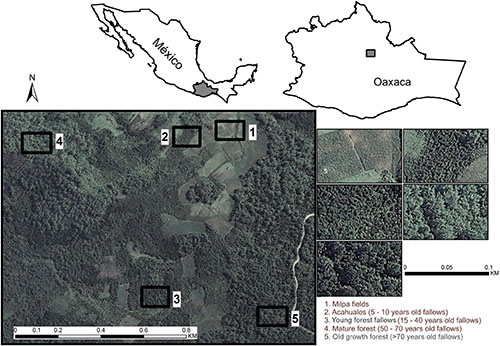
Figure 7. The appearance of landscapes communicates cultural values (Iverson Nassauer, 1995), as on the windward side of the Sierra Norte mountains, Oaxaca, Mexico (1,300–1,800 m elevation). The milpas and their associated secondary vegetation constitute a biocultural landscape resulting from the cultural transmission and adaptive management that have prevailed for > 5,000 years. The landscape is a mosaic of crop fields and vegetation fragments of different ages resulting from small land pieces previously cultivated and set idle at different times in the past after the cultivation phase. Young forest fallows are dominated by Pinus chiapensis. Old-growth fallows are typical montane cloud forests. Reproduced from del Castillo (2015) by permission of Ecological Applications© John Wiley and Sons (2015).
Biotic Relationships, Domestication, and Co-domestication
The invention of the milpa fostered novel adaptations to the newcomer humans in the local biota. For instance, squash seeds were found in Mastodon (Mammutidae) dung deposits, suggesting that this extinct mammal was the original seed disperser. Domestication likely saved some Cucurbita species from extinction by humans fulfilling their dispersal needs, likely contributing to the survival of humans and squashes (Kistler et al., 2015). Cucurbita, milpas’ common companion plants, are pollinated by specialist bees (Peponapis spp., Apidae). The fields where squashes are cultivated maintain a significantly different bee composition compared with adjacent non-cultivated areas (Baja Verapaz, Guatemala, Enríquez et al., 2015). As species richness increases in the maize agroecosystem, maize root colonization by arbuscular mycorrhizal fungi, AMF, and soil fertility (N, P) increases (Negrete-Yankelevich et al., 2013). The rhizospheric bacterial community associated with milpa maize changes at different cultivation stages (Rebollar et al., 2017). The huitlacoche or corn smuth disease (Ustilago maydis, Ustilaginaceae) is an obligate and highly appreciated edible maize fungal pathogen. Molecular analyses demonstrate that domestication and early maize cultivation changed the huitlacoche population’s genetic structure (Munkacsi et al., 2008). A maize landrace from Sierra Mixe, Oaxaca, displays extensive development of adventitious roots that secrete a sugar-rich mucilage that sustains nitrogen-fixing bacteria, according to Van Deynze et al. (2018). The authors estimate that 29–82% of this maize landrace nitrogen is of atmospheric origin, opening the possibility of developing nitrogen fixation for commercial maize agriculture (Sheoran et al., 2021). The roots of milpa-associated beans, such as Phaseolus vulgaris, host a great diversity of Rhizobium spp. (Rhizobiaceae) nitrogen-fixing bacteria (Martinez-Romero, 2003). Maize-bean intercropping increased Rhizobium nodulation, rhizosphere-soil P availability, and grain yield relative to monocultures on phosphorus-deficient soils (Latati et al., 2016). The above examples suggest that domestication in milpas have generated significant transformation in the genetic architecture of many species of different life kingdoms.
The fallows associated with the milpas are not only land pieces left idle for revegetation and pedogenesis. They are part of the milpa system in which the domestication of valuable species occurs. This process involves the forest species’ manipulation through selection processes aiming to enhance the desired properties of forest plants and, together with the entire milpa practice, constitute the co-domestication of forest and tree species (Wiersum, 1997).
Indeed, the lands adjacent to crop areas, often fallows, were domestication scenarios of a great variety of plants in Mesoamerica, such as agave (Agave spp., Asparagaceae, semi-dry shrublands), avocado (Persea americana, Lauraceae, humid tropical forest), guava (Psidium guajava, Myrtaceae, warm secondary forest), tejocote (Crataegus mexicana: Rosaceae, temperate oak forest), and papaya (Carica papaya, Caricaceae, disturbed tropical rain forest) to mention few examples of the > 600 plant species domesticated in Mesoamerica (see Nieto-Angel et al., 1997; Caballero et al., 1998; Pennington and Sarukhán, 1998; Casas et al., 2007; Delgado-Lemus et al., 2014; Chávez-Pesqueira and Núñez-Farfán, 2017). In addition to the abovementioned example of Cucurbita, Janzen and Martin (1982) suggested other possible examples of neotropical anachronisms in plants such as avocado and cacao (Theobroma cacao: Malvaceae), which domestication could have helped to avoid their extinction and likely expanded their distribution ranges (Van Zonneveld et al., 2018).
Other Ecosystem Services
Many of the species’ fallows are medicine, firewood, and construction material sources for the local people (Aguilar-Santelises, 2007; Diemont and Martin, 2009). Spatially altering polyculture fields within several vegetation types likely reduce pest numbers relative to a solid monoculture (Horn, 2012). Also, the movement of wind-dispersed weeds between different crop fields is likely hampered by vegetation fragments that lie between crop fields, probably reducing unwanted weeds (see Cousens et al., 2008). Properly managed milpas may contribute to soil fertility and long-term carbon sequestration (Nigh and Diemont, 2013). Milpa is also an adaptive system subject to improvement. Yield, for instance, can be improved by changing sowing density (van der Wal et al., 2006).
The Spanish Conquest and Globalization
The Spaniard’s arrival initiated the last biological upheaval, implying a tremendous cultural supplantation through globalization. Like the other two previous upheavals, the Spaniards altered the landscape, life kingdoms, biotic relationships, and the genetic architecture of some species. In a few decades, mining, building new roads and settlements, the introduction of exotic animals, crops, and diseases started an ongoing transformation of the Americas (Challenger, 1998; Diamond, 1999). One of the fastest, earliest, and most dramatic biotic impacts was the 16th-century series of disease outbreaks that decimated the native human population, considered one of the worst and deadliest epidemics (Diamond, 1999). There are many gaps and speculations regarding these outbreaks. However, they were of continental dimensions and multifactorial (Cook and Lovell, 2001). After a 1,519–1,520 smallpox epidemic, a 1,545–1,576 outbreak followed, probably caused by a hemorrhagic fever that the Indians called cocolitzi, the word for pest in the Nahuatl language; these two outbreaks killed between 12 and 25 million people in central Mexico (Acuña-Soto et al., 2002). Salmonella enterica (Enterobacteriaceae), a bacterium that causes enteric fever, is the most likely culprit of the 1,545 cocolitzli outbreak, based on a genome-wide study on pulp teeth skeletons from possible victims from the Mixteca Alta, Oaxaca (Vågene et al., 2018). Ten lesser epidemics followed, but the 1,545 severe epidemic coincided with an unusual drought after a wet period, which likely favored the proliferation of cocolitzi-infected rodents, and their subsequent displacements to human settlements searching for food (Acuña-Soto et al., 2002). The decimation of the Indian populations paved the way for a fast cultural and biotic transformation, through the ongoing globalization process, with two significant stages: biocultural reduction followed by biocultural homogenization (Rozzi, 2018). Earlier effects of such changes have been documented in SMP. Severe deforestation caused by pine over-exploitation for shipbuilding wood was detected in the eighteenth century in Chimalapas, Oaxaca (De Bethencourt Massieu, 1960). Palynological records attested to the mixed hardwood forest decline in Chuchumatanes Highlands, Guatemala, after the Spaniards’ arrival (Harvey et al., 2021b). The Spanish conquest time coincides with a significant global decline in terrestrial vertebrates (Dirzo et al., 2014).
Landscape Changes: Simplification of Successional Dynamics and Ecosystem Losses
Globalization and high food demand have simplified the indigenous successional process by substituting old-growth hardwood forests with early secondary forests. This process has been referred to as “pinarization” as pines usually dominate early secondary forests north of Costa Rica (sensu González Espinosa, 2000; Galindo-Jaimes et al., 2002; Figure 6C). The litter derived from pines gives lower crop yields than old-growth forest plants (García-Barrios and González-Espinosa, 2004). This decrease would have augmented the demand for more croplands at the expense of forestlands, enhanced the use of synthetic fertilizers, or both. Increasing crop field connectivity by reducing forestlands should have boosted the dispersal of weeds and crop pests, thus increasing pesticide demand. Insecticide applications probably favor the decimation of squash bee pollinators, favoring monoculture. Eventually, forestlands might disappear, remaining acahuales and croplands, or only croplands, encouraging, even more, the use of agrochemicals. In the meantime, the pressure on forestlands should have increased by logging, firewood extraction, and freely roaming domestic herbivores (Figure 6D). The above factors should have diminished the capability of pioneer forest plants to revegetate by reducing their effective population size, as described above, thus accelerating deforestation. Indeed, intensive agriculture and high human population density have transformed vast SMP forestlands into croplands, pasturelands, and monocultures (e.g., Chiapas Highlands, Luna, 2019; Figure 6E). However, estimating deforestation rates are challenging because of the complex dynamics of land use in forestlands, involving traditional land management practices, shade tree coffee, and sustainable management of forests for timber production (Noble and Dirzo, 1997; Bray, 2009).
Soil erosion could have been intensified by indigenous agriculture and aggravated by drought periods during pre-Hispanic times (Nochixtlán valley, Oaxaca, Mueller et al., 2012). This problem, however, was exacerbated by domestic animal overgrazing, deforestation, and agriculture after the Spanish conquest. For instance, El Salvador had lost more than 50% of the arable land by the end of the last century, remaining few forestlands (Leonard, 1987). Neotropical mountain forests have vanished in many places or were simplified by humans, causing a severe decline of species of which large-sized animals were the most evident cases (e.g., Figel et al., 2009), but not the only ones (e.g., Janzen and Hallwachs, 2019). Agricultural encroachment in the Bosawas Biosphere Reserve and Wawashan Nature Reserve corridor in Nicaragua has contributed to the decline of the jaguar, the largest feline in America (Panthera onca, Felidae), and six large-bodied prey species, including white-lipped peccary (Tayassu pecari, Tayassuidae), collared peccary (Pecari tajacu, Tayassuidae), and red brocket deer (Mazama americana, Cervidae) (Petracca et al., 2014).
Mammal defaunation, particularly of large-sized animals, has augmented since the 1970s. Nicaragua and Honduras, in the Neotropics, are the countries with the heaviest defaunation burden, according to Bogoni et al. (2020). The authors found that forest reduction, hunting, and increasing accessibility to wild areas explain this decline. Similarly, increasing secondary vegetation and croplands in Oaxaca cloud forest areas likely have enhanced the jaguar extinction risk (Briones-Salas et al., 2012). Also, old-growth forest decline should contribute to reducing epiphyte populations. Epiphyte colonization is a slow process observed in cloud forest areas in Guatemala (Catling and Lefkovitch, 1989) and Oaxaca; vascular plant epiphytes were the latest groups to colonize the tree trunks in a successional gradient (Cordova and del Castillo, 2001). Finally, old-growth forest plants and relict species probably are other essential groups deserving urgent conservation efforts.
The Complexity of Species Declining
SMP has been the scenario of accelerating species declines in the last decades, but the factors involved other than humans are unclear. The amphibian decline after the 1970s is perhaps the most dramatic (Alroy, 2015). The interactions between disturbance and pathogens might act synergistically to reduce amphibian populations (Whitfield et al., 2016). The rapid amphibians decline has been displaced from Costa Rica to Panama, becoming more severe in the mountains (Lips et al., 2006). The emergence of the fungal pathogen Batrachochytrium dendrobatidis (Chytridiomycota), which spread from Mexico to Costa Rica, coincides with the decline of the golden toad Bufo periglenes (Bufonidae) (Cheng et al., 2011). Multiple migration events and factors may account for the amphibian decline, likely transforming Mesoamerica into one of the world’s worst centers of frog and squamate extinctions (Alroy, 2015).
Bark-beetle (Dendroctonus spp., Coleoptera) neotropical pine outbreaks exemplify how multifactorial interactions magnify species decline. Drought events may weaken and increase the vulnerability to the bark beetle in Pinus pseudostrobus trees (Gómez-Pineda et al., 2022). Temperature increases might account for south displacements of this insect, augmenting its distribution area (Armendáriz, 2012). In 2003, in the department of Santa Ana, El Salvador, a wildfire burned 80 Ha of Pinus oocarpa forest. The beetle severely affected the surviving pines the following year, whereas no affected pines were detected in adjacent unburned forests (Billings et al., 2004). Agrarian conflicts between municipalities magnify bark beetle infestation in Oaxaca by impeding the arrival of forest technicians to the affected areas; from 2010 to 2020, this plague affected 5,000 Ha of forestlands in this state (Agencia NEC, 2020).
Fire incidence increases fostered by global warming and human activities in dry forests of the Lomas Barbudal, Costa Rica, have reduced the supply of essential resources for bees, undoubtedly impacting other species, such as the plants they pollinate (Barthell et al., 1993). Fragmentation has augmented the perimeter/area of the vegetation patches. Thus, the canopy exposure of the host trees to open areas in Sierra Norte oak forests, Oaxaca favors tree colonization by the epiphyte Catopsis compacta (Bromeliaceae). However, its long-term survival may be compromised by reductions in host regeneration by global warming and deforestation (del Castillo et al., 2013). Also, increasing the fragment’s perimeter/area might favor the fragment shrub/tree species composition, probably because of the higher preference for shrubs to more exposed areas (Aguilar-Santelises and del Castillo, 2013). Deforestation and bat diversity decreases in Costa Rica appear to enhance male-biased parasitism in bat assemblages (Frank et al., 2016). Sex ratio bias diminishes the effective population size, increasing extinction probabilities (Frankham, 2005). Thus, disturbances affect the biota in complex ways.
Genetic Erosion in Crops
The replacement of landraces with modern cultivars causes genetic erosion and threatens food biosecurity (Van de Wouw et al., 2010). The presence of transgenes in local maize landraces is an example in SMP. Transgenic plants generated by transnational companies are common cultivars with high-added value. Transgenic maize is commonly cultivated in many countries since it is allegedly insect-resistant, herbicide-tolerant, non-allergenic, or toxic to animals and humans (Baktavachalam et al., 2015). In Mexico, its cultivation is forbidden, but transgenic maize is widely imported for consumption and inadvertently cultivated (McAfee, 2008). Since maize pollen is wind-pollinated, pollen flow between transgenic maize and local landraces is possible, mainly if cultivated in proximity (Bellon and Berthaud, 2004). However, seed flow carried out by farmers appears to be more critical (van Heerwaarden et al., 2012). Indeed, transgenes in local landraces were unequivocally detected in Sierra Norte, Oaxaca (Piñeyro-Nelson et al., 2009).
The proliferation of maize monoculture should have accelerated genetic erosion. On the humid side, at mid-elevations (1,400–1,900 m), yellow and blue maize landraces are cultivated in traditional milpas (Pérez-García and del Castillo, 2016). However, the state laws impose punishments upon anyone who, in forestlands, perform any other activity than forestry (Gobierno del Estado de Oaxaca, 2020). As such, a semi-permanent system has been implemented in the same areas. This system is characterized by fire suppression, agrochemical usage, and a monoculture using the white local landrace (Pérez-García and del Castillo, 2016). The substitution of milpas by maize monoculture may disrupt biotic interactions such as the ancient maize-microbe interactions involving bacteria of three phyla, which may benefit the plants (Aguirre-von-Wobeser et al., 2018). The risks of genetic introgression with transgenic maize could be enhanced as both the kernels of the transgenic maize and the white local landrace are similar and complex to sort out.
Exotic Species
The Spaniards’ introduction of exotic mammals, mainly cattle, sheep, and goats, has two significant implications for the biota and the landscape. The natural vegetation or croplands are transformed into grasslands for raising these animals (Figure 7; Guevara and Laborde, 2014). This conversion implies significant organic matter losses resulting in greenhouse gas emissions (Aryal et al., 2018), likely altering the species composition of many species groups, including AMF (e.g., Alvarez-Lopeztello et al., 2019). Abandoned pasture lands in Panama, Costa Rica, Nicaragua, and Mexico may regenerate into the original vegetation. However, fires, long histories of forest clearance, and reduced abundance of remnant trees are limiting factors (Griscom and Ashton, 2011). Overgrazing and inadequate agriculture result in land erosion, which is challenging to lessen due to the lack of coordination between different political systems (e.g., Rio Lempa Basin, Honduras-Salvador border, Kim et al., 2005).
Second, when domestic mammals are left in natural ecosystems to roam freely, they compete with native herbivores, usually accelerating land degradation and extinction (Gillespie et al., 2000). However, domestic animals might contribute to the disperse seeds of native plants such as jicaro (Crescentia alata: Bignoniaceae) and guanacaste (Enterolobium cyclocarpum: Fabaceae) that presumably were initially dispersed by Pleistocene extinct megafauna (Janzen and Martin, 1982, but see Blanco et al., 2019).
There are many other examples of exotic species’ introduction in SMP. That of honeybees (Apis mellifera: Apidae) is remarkable. First, they spread widely into the Americas and might outcompete social-native stingless bees (Roubik et al., 1986). Second, an africanized variety later escaped from a Brazilian laboratory and rapidly expanded and hybridized with resident honeybees on the continent, considered “the most spectacular biological invasions yet documented” (Pinto et al., 2005). The africanized honeybees are more aggressive and adapted to tropical climates than their European counterparts, which genes were common in bees of the first invasion. Recent molecular genetics studies indicate that, after the second invasion, most genes of honeybees from Panama, Costa Rica, and Mexico are of African origin, suggesting an adaptive advantage of those genes over the original European genes (Zárate et al., 2022). Studies of the impact of such invasions on native bees are needed. Of particular importance is that non-native species introduction is another element that erode local culture (e.g., Simberloff, 2018).
Cultural Erosion and Bio-Cultural Conflicts
Landscape simplification decreases biodiversity and impacts culture and local human populations in SMP. Small indigenous landholders are frequently the most affected. For indigenous people, deforestation signifies losses of valuable species and services. A species’ local extinction in an indigenous territory is of cultural impact, meaning the loss of a pedagogical element for the cultural transmission from parents to offspring, contributing to the erosion of the cultural heritage, and a loss of an ingredient to justify any attempt to preserve the ecosystem. Formal education has contributed to this erosion, as usually little or no information provides about the peoples’ local habitat. As such, the estimated traditional knowledge, for instance, tends to increase with the fraction of native-language speakers and to decrease with the level of formal education in Mixtecan municipalities, Oaxaca (Aguilar-Santelises and del Castillo, 2015). Therefore, one of the benefits of biodiversity conservation is restoring to the people what they have lost through the acculturation process (Allen, 1988).
Cultural conflicts between local people, and newcomer immigrants or non-indigenous people, are common in SMP. These conflicts increase deforestation and biodiversity losses. Illegal logging and fauna trafficking are examples (e.g., Richards et al., 2003; Honey-Rosés, 2009; Masés-García et al., 2021). In Chimalapas, indigenous people using community-based rules for forest management and conservation conflict with recent immigrants fomenting cattle rising (Payne, 2002). These examples stress the importance of the biocultural heritage for biodiversity conservation (Toledo, 2001).
Disturbances and Climate Change Impact Humans and Other Species
A recent survey across six different landscapes reveals that coffee and maize producers have noticed global warming and unpredictable rains reduce crop production (Harvey et al., 2018). As is the case of other species, migration could be an option for farmers facing climate change (see Lustgarten, 2020). In mountain areas, upslope displacements of agricultural lands are expected, probably at a higher pace than anticipated for many local species (Bush, 2002). Consequently, upslope migrating species may find reduced colonization options because of the small areas of high elevation belts and the risks that such areas could be partially or totally anthropized. Thus, humans, small landholders, and biodiversity are severely affected by common problems: disturbances and climate change. Holistic approaches seeking human welfare and biodiversity conservation can be challenging to design and apply, but their probability of success should be high (Harvey et al., 2008; Rozzi, 2018).
Conservation Challenges
Mesoamerican countries are officially aware of the importance of biological diversity, have ratified the Biological Diversity Convention, and implementing actions favoring conservation (Aguilar-Støen and Dhillion, 2003; Toly, 2004; Harvey et al., 2008). A deep analysis of conservation initiatives in SMP is beyond the scope of this review. However, two conservation kinds of initiatives are worth mentioning. They are entirely different, often yielding conflicting results reflecting the contrasting interests of the actors and economic sectors involved in formulating such initiatives.
Top-Down and Bottom-Up Initiatives
Top-down initiatives are mainly derived from top-level government agencies, often with the participation of foreign agencies. Perhaps the best example is the Costa Rican National Park Network, whose implementation began in the early 1970s (Toly, 2004). This network has been recognized worldwide owing to its success in helping to protect critical biodiversity reservoirs and generating income through ecotourism (Valverde-Sánchez, 2018).
Some top-down initiatives aim to legitimize regional access to biodiversity resources and estimate their market exchange value (Brand and Görg, 2003). One of the missions of the Costa Rica National Institute of Biodiversity, established in 1992, is the production of diversity derivatives, such as genetic and biochemical resources that can be exploited by large multinational companies (Toly, 2004). Two different initiatives, the Plan Puebla-Panama, seeking regional free trade and development, and the Mesoamerican Biological Corridor with conservation goals, are additional examples aiming at the entire Mesoamerican region. These initiatives include the systematic search for biochemical and genetic information in nature to develop commercially valuable products (Toly, 2004). Such initiatives may promote biodiversity conservation, but the probability of success is low unless local people are considered the main actors and beneficiaries.
Bottom-up initiatives are based on the local people’s participation and initiatives targeting biodiversity conservation, sustainability, and community benefits. Some examples include traditional (Pascual-Mendoza et al., 2020) or novel agroforestry techniques, sometimes with the involvement of academics (e.g., Rosales Adame et al., 2020); in other cases, design and maintain communal voluntary conservation reserves (Anta, 2007), perform forestry practices, balanced by processes of forest recovery, maintenance, and protection (Van Vleet et al., 2016; Pazos-Almada and Bray, 2018); or develop a network of communitarian germplasm banks (Mejía Lacayo, 2019). Most of the above-mentioned bottom-up examples are ruled by indigenous people. Bottom-up initiatives seldom are recognized outside the frontiers of the land at which they are aimed, even among the conservation scientific community (Van Vleet et al., 2016). The lack of recognizance leads to the false conclusion that the problems and threats to biodiversity are because of the dearth of conservation initiatives. Instead, most conservation problems result from conflicting perspectives and interests between the actual or potential primary users of biological resources and between the people who formulate the conservation initiatives.
Another difference between bottom-up and top-down initiatives is the conception of indigenous people’s roles in conservation. For top-down initiatives, the presence of the people in areas to preserve is a nuisance. Thus, rural-urban migration, common in SMP, is considered an opportunity for forest recovery (Aide and Grau, 2004). However, urban migration of people practicing traditional forms of land management might clear the way for easy access to their lands, facilitating aggressive land uses, including intensive land exploitation and illegal logging, mining, hunting, and trafficking of species and drugs by reducing the number of local people who can safeguard the land. Holistic and more efficient conservation agendas must consider how humans should coexist with nature (Rozzi et al., 2013). Conservation policies should include the diversity’s biological and cultural components since both are tightly linked (Pretty et al., 2009).
Shade coffee plantations are another important non-traditional conservation and land management initiative practiced at mid-elevation in the humid areas of SMP mountains. This strategy may involve top-down and bottom-up initiatives, which, properly integrated, can benefit biodiversity and households (Méndez et al., 2010). Shade coffee plantations may maintain similar composition and structure to native vegetation, generating profits (Alvarez-Alvarez et al., 2021). However, the common use of Inga (Fabaceae) as a shade plant may decrease the proportion of native species, making it essential to fomenting the importance of these species in plantations among coffee farmers (Valencia et al., 2016). Also, the livelihood strategies and suitable types may influence the success of coffee plantations in maintaining biodiversity (Méndez et al., 2010). Coffee yield may negatively correlate with shade coffee plantations’ tree diversity, thus requiring some compensation for yield losses (Perfecto et al., 1996). Furthermore, conflicts between economic revenues and conservation may transform more forests into coffee plantations (e.g., Tejeda-Cruz et al., 2010). Thus, shade coffee plantations can be a good conservation option but need careful monitoring and improvement.
Social Contradictions in Conservation Programs
In Costa Rica, laws that ban forest conversion into other land uses are valuable for conservation and are included in the conservation agenda (Harvey et al., 2008). As we mentioned above, such laws may not work in indigenous territories in SMP. For indigenous-dominated communities banning agriculture practices in forest areas imply banning traditional milpas and is against their biocultural heritage. Such regulations favor monoculture practices, reduce the land’s capacity to revegetate after cultivation (Pérez-García and del Castillo, 2017), and provide basic nutrient requirements for the people (e.g., López-Ridaura et al., 2021; Novotny et al., 2021). Please recall that in Costa Rica, 2.4% of the population is indigenous, compared to 41.0%, in Guatemala, for instance, and many dominated-indigenous communities live partially isolated in the mountains (Banco Mundial, 2015). Thus, the same conservation policies may have opposing effects depending on where and when they are applied.
Disagreements between local people and top-down initiatives often arise because local people are commonly ignored during the decision-making process (Toly, 2004). Certification politics of fair-trade initiatives such as the Fair-trade USA coffee plantations have generated economic insecurity and housing problems in Nicaragua (Raynolds and Rosty, 2021). Complex regulations against logging and institutional corruption in Nicaragua and Honduras, for instance, have fostered illegal logging. At the same time, the landowners of the affected forests, mostly indigenous groups, become the most prejudiced (Richards et al., 2003). The effects of land use regulation on biological resources may have transnational implications. More rigid and more efficient rules against logging in Costa Rica have generated timber shortfalls partially covered by illegal woods smuggled from Nicaragua (Richards et al., 2003). Furthermore, laws forbidding the commerce of threatened species may become very difficult to enforce. Some of such species have long been traditionally traded in local markets, as is the case of some orchids in Mexico (Cruz-Garcia et al., 2015).
At first glance, recent initiatives such as the Mesoamerican Corridor are promising. This initiative comprises establishing connectivity between ecosystems, landscapes, and the evolutionary processes from southern Mexico to Panama (DeClerck et al., 2010; Comisión Nacional para el Conocimiento y Uso de la Biodiversidad, México [CONABIO], 2021). However, the local people’s participation in the planning and execution of this kind of project is crucial. This is not always the case, as shown in the Nicaraguan section of the Mesoamerican Corridor, where the participation of the local government and the impacted human groups is reduced (Finley-Brook, 2007). The main objective of such kinds of initiatives should be attending to real conservation problems and not a bureaucratic shield to mask other intentions (Grandia, 2007).
Other conservation alternatives, such as the payment for environmental services, may generate tradeoffs between people’s needs and environmental goals in SMP (Kosoy et al., 2007; Calvo-Alvarado et al., 2009). Also, without considering people’s needs, afforestation may increase forest cover and social marginalization (e.g., Costa Rica, Kull et al., 2007). The participation of local people in monitoring species is also an efficient alternative to protect wildlife, particularly large animals in danger of extinction, such as the jaguar in Oaxaca (Lavariega et al., 2020). The successful establishment of reserves coupled with economic and political changes have fostered the recovery of tropical dry forest areas in Costa Rica (Hilje et al., 2015).
Local people participation in conservation initiatives likely guarantees long-lasting conservation efforts bringing genuine opportunities for involving bottom-up and top-down initiatives. The Área de Conservación Guanacaste is a promising example (Janzen and Hallwachs, 2020). Attending to local people’s needs is essential in any serious attempt to protect natural environments; moreover, people practicing traditional farming have a profound ecological knowledge of their land (Altieri and Toledo, 2011). Therefore, studies of biological and cultural diversity, including traditional land management, ecological processes, and community-based social practices, are required to lay the foundations of effective conservation methods that simultaneously protect biological and cultural diversity, ameliorate disturbance events, and assure the welfare of the local people.
Conclusion
The biodiversity of SMP is the product of three upheavals:
1. The Great American Interchange and the subjacent tectonic events
2. Human colonization with the generation of Mesoamerican cultures and the maize-milpa system
3. The Spaniards’ arrival and subsequent globalization
These events and frequent disturbances have dramatically altered the landscape spatial configuration, successional dynamics, biotic relationships, and the genetic architecture of some species. Frequent disturbances, habitat heterogeneity, poor habitat connectivity, and habitat size likely reduce the capability to respond to environmental stresses.
The milpa system (crop fields and associated fallows) is one of the most important legacies of the pre-Hispanic human occupation in Mesoamerica. It is also an ecological and genetic engineering system actively affecting landscape configuration, successional, and pedogenetic processes, and an agent that modifies the genetic architecture of species of different life kingdoms through domestication. It is also a biocultural reservoir, a product of close links between biological and cultural diversity (Perales et al., 2005). Thus, conservation of the milpa system should positively impact food biosecurity, culture, and biodiversity. Also, preserving the milpa system is concordant with the recently promoted biocultural ethics that try to understand and foment coherent links between human habits and habitats (Rozzi et al., 2013), a challenging task in SMP.
The Spanish conquest and globalization led to unprecedented human epidemics and the erosion of soil, culture, and biodiversity. SMP has been the scenario of severe global warming, one of the fastest and deadliest extinction events (amphibians), one of the most spectacular exotic-species invasions (Afrikanized honeybees), and accelerated deforestation, defaunation, and acculturation.
Biocultural conflicts are expressed in conservation initiatives based on different perspectives and interests between the involved stakeholders, often ignoring the affected people. Local bottom-up initiatives can be the best suited for conservation in indigenous areas, whereas honest (i.e., with truly conservation intentions) top-down initiatives can be helpful if the affected people are considered subjects (no objects) of conservation plans.
We suggest some often-neglected unique habitats requiring conservation attention based on habitat size, expected probabilities of the protected species to migrate to similar habitats in the event of habitat destruction or climate change; habitat refuges, potential threatening factors; and social/biocultural peculiarities (e.g., requiring the involvement of local indigenous people or coordination between different authorities along the target area) (Table 2). We analyzed current conservation initiatives based on the target species and habitats, biocultural dimension, resilience, goods and cash generated, and applicability (Table 3).
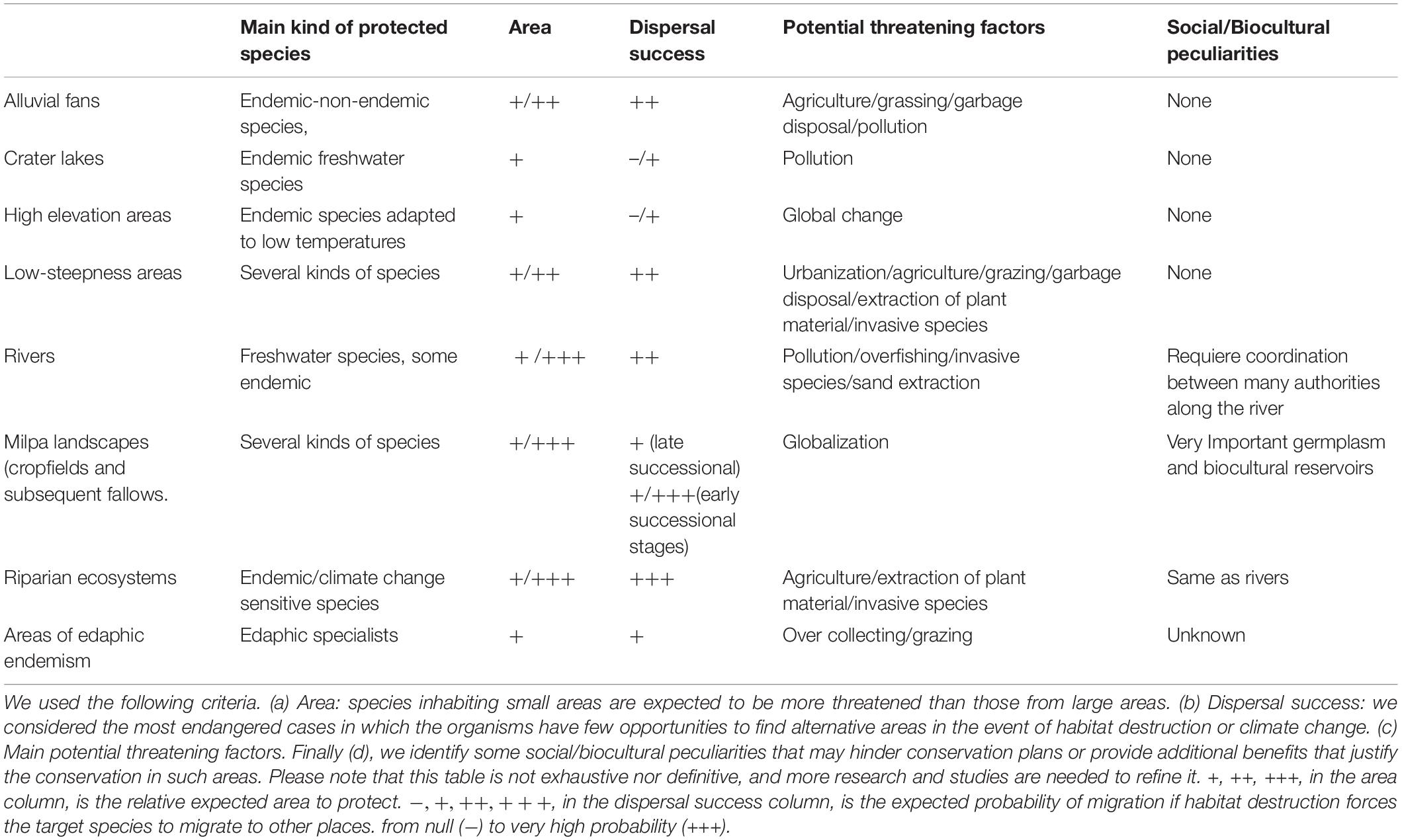
Table 2. Conservation sites of SMP that require urgent conservation and often are not considered, or conservation is logistically challenging.
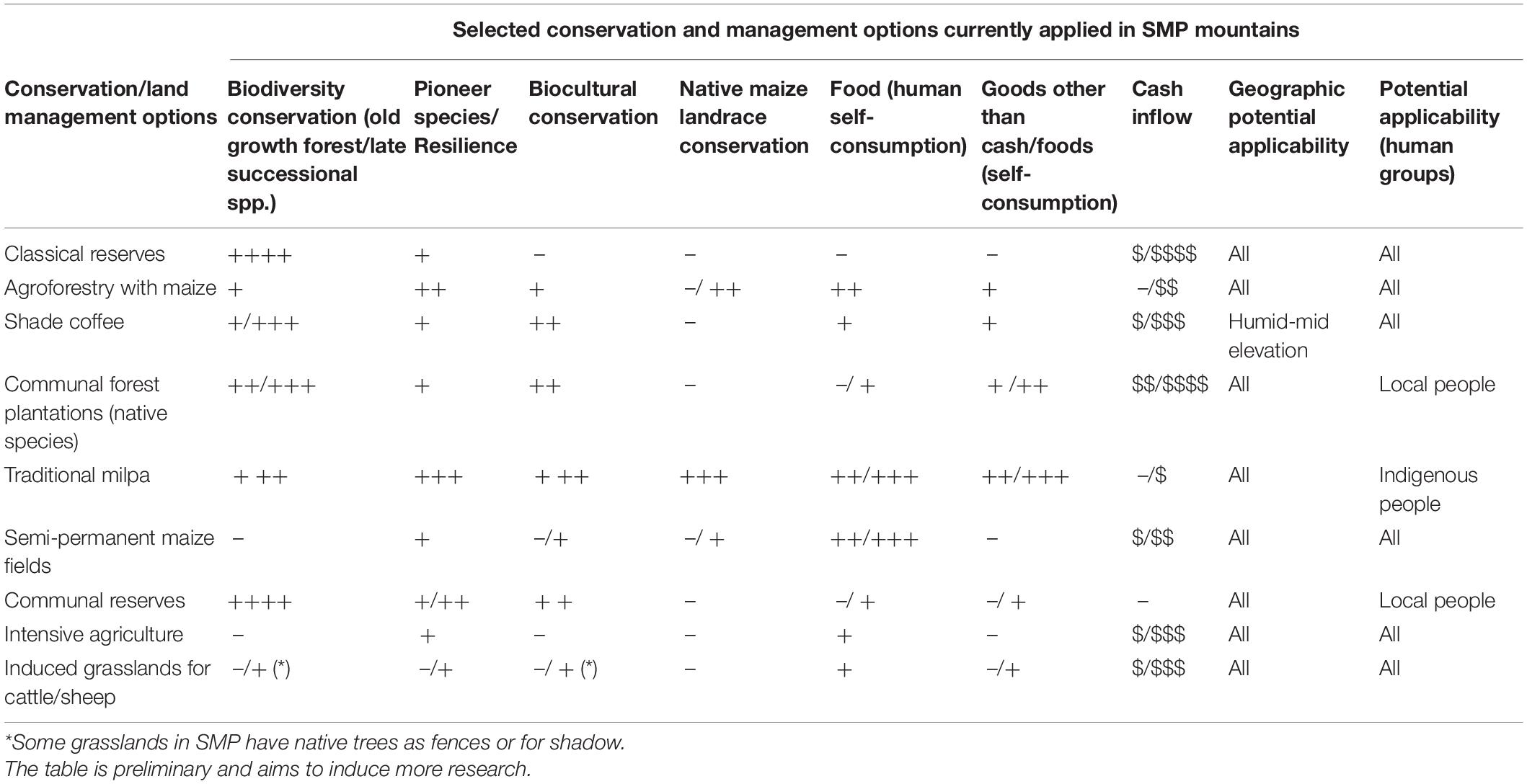
Table 3. Comparative of selected conservation and management options currently applied in SMP mountains regarding their conservation potential, resilience, biocultural conservation, the potential for providing foods and other goods for human self-consumption (low, +, to very high, ++++), cash inflow ($, low to very high $$$$), potential geographic applicability (e.g., elevation, climate), and potential applicability to be conducted by different human groups (all, local or outsiders; local, local people regardless their cultural background; indigenous, local people with local traditional backgrounds).
Not a single conservation initiative is best suited for all conservation needs in SMP. Protection of all successional stages is imperative to assure enough resilience and genetic material for natural restoration. Conservation of the milpa system (crop fields and associated fallows) is an optimal option for minimizing tradeoffs between conservation and people needs and safeguarding traditional culture and local landraces but is probably limited to areas with indigenous people and may not work for species with large home ranges.
Author Contributions
RC wrote the manuscript. RR-G analyzed the satellite imagery, designed the maps, and conducted documentary research. Both authors contributed to the article and approved the submitted version.
Funding
This work was supported by the Instituto Politecnico Nacional, SIP 20201673, awarded to RC. European Commission FP6-2004-INCO-DEV-3 PROP N° 032132 (awarded to A. C. Newton). European Commission, BIOCORES project contract no. ICA4-CT 2001-10095 (awarded to A. C. Newton). Biodiversity, conservation, and sustainable use in Mexican cloud forest*, Darwin Initiative for the Survival of Species (awarded to A. C. Newton).
Conflict of Interest
The authors declare that the research was conducted in the absence of any commercial or financial relationships that could be construed as a potential conflict of interest.
Publisher’s Note
All claims expressed in this article are solely those of the authors and do not necessarily represent those of their affiliated organizations, or those of the publisher, the editors and the reviewers. Any product that may be evaluated in this article, or claim that may be made by its manufacturer, is not guaranteed or endorsed by the publisher.
Acknowledgments
RC was most thankful to the editors for their invitation to participate in the Research Topic “Environmental Gradients and Neotropical Forest Dynamics Under Climate Change.” We are most thankful with previous discussions with A. C. Newton, the researchers of the REFORLAN and BIOCORES projects, and the del Castillo lab group. Three reviewers careful read the manuscript and provided helpful comments.
References
Acuña-Soto, R., Stahle, D. W., Cleaveland, M. K., and Therrell, M. D. (2002). Megadrought and megadeath in 16th century Mexico. Emerg. Infect. Dis. 8:360. doi: 10.3201/eid0804.010175
Agencia NEC (2020). Líos Agrarios Limitan el Combate de Plagas Forestales: CONAFOR. Oaxaca: Diario Marca.
Agrawal, A. A., Conner, J. K., and Rasmann, S. (2010). “Tradeoffs and negative correlations in evolutionary ecology,” in Evolution Since Darwin: The first 150 years, eds M. A. Bell, D. J. Futuyama, W. F. Eanes, and J. S. Levinton (Sunderland, MA: Sinauer Associates), 243–268.
Aguilar Luna, J. M. E. (2018). Estructura y diversidad de la vegetación arbórea de un bosque de galería en el estado de Puebla. Rev. Mex. Cienc. Forest. 9, 230–252.
Aguilar-Santelises, R., and del Castillo, R. F. (2013). Factors affecting woody plant species diversity of fragmented seasonally dry oak forests in the Mixteca Alta, Oaxaca, Mexico. Rev. Mex. Biodiv. 84, 575–590.
Aguilar-Santelises, R., and del Castillo, R. F. (2015). Demographic and socio-economic determinants of traditional plant knowledge among the Mixtecs of Oaxaca, Southern Mexico. Hum. Ecol. 43, 655–667.
Aguilar-Santelises, R. (2007). Etnobotánica Cuantitativa de una Región de Bosque de Niebla de Sierra Norte, Oaxaca. M.Sc. Thesis. México: Instituto Politécnico Nacional.
Aguilar-Støen, M., and Dhillion, S. S. (2003). Implementation of the convention on biological diversity in Mesoamerica: environmental and developmental perspectives. Environ. Conserv. 30, 131–138.
Aguirre-von-Wobeser, E., Rocha-Estrada, J., Shapiro, L. R., and de la Torre, M. (2018). Enrichment of verrucomicrobia, actinobacteria and burkholderiales drives selection of bacterial community from soil by maize roots in a traditional Milpa agroecosystem. PLoS One 13:e0208852. doi: 10.1371/journal.pone.0208852
Aide, T. M., and Grau, H. R. (2004). Globalization, migration, and Latin American ecosystems. Science 305, 1915–1916. doi: 10.1126/science.1103179
Alcañiz, M., Outeiro, L., Francos, M., and Úbeda, X. (2018). Effects of prescribed fires on soil properties: a review. Sci. Total Environ. 613, 944–957. doi: 10.1016/j.scitotenv.2017.09.144
Alcántara-Ayala, I. O., Esteban-Chávez, O., and Parrot, J. F. (2006). “Landsliding related to land-cover change: a diachronic analysis of hillslope instability distribution in the Sierra Norte, Puebla, Mexico”. Catena 65, 152–165.
Alford, J. J. (1970). Extinction as a possible factor in the invention of New World agriculture. Prof. Geogr. 22, 120–123.
Allen, W. H. (1988). Biocultural restoration of a tropical forest. BioScience 38, 156–161. doi: 10.1016/j.jenvman.2021.113014
Allende, T. C., Macias, J. L., Mendoza, M. E., and Diaz, J. V. (2020). Evidence of volcanic activity in the growth rings of trees at the Tacana volcano, Mexico-Guatemala border. Can. J. For. Res. 50, 65–73.
Allendorf, F. W., and Luikart, G. (2007). Conservation and the Genetics of Populations. Malden, MA: Blackwell Publishing.
Almazán-Núñez, R. C., Corcuera, P., Parra-Juárez, L., Jiménez-Hernández, J., and Charre, G. M. (2016). Changes in structure and diversity of woody plants in a secondary mixed pine-oak forest in the Sierra Madre del Sur of Mexico. Forests 7:90.
Alroy, J. (2015). Current extinction rates of reptiles and amphibians. Proc. Natl. Acad. Sci. U.S.A. 112, 13003–13008. doi: 10.1073/pnas.1508681112
Altieri, M. A., and Toledo, V. M. (2011). The agroecological revolution in Latin America: rescuing nature, ensuring food sovereignty and empowering peasants. J. Peasant Stud. 38, 587–612.
Altieri, M. A., Funes-Monzote, F. R., and Petersen, P. (2012). Agroecologically efficient agricultural systems for smallholder farmers: contributions to food sovereignty. Agron. Sustain. Dev. 32, 1–13.
Alvarez-Alvarez, E. A., Almazán-Núñez, R. C., Gonzalez-Garcia, F., Brito-Millan, M., Mendez-Bahena, A., and Garcia-Ibanez, S. (2021). Shade coffee plantations maintain woody plant diversity and structure in a cloud forest landscape of southern Mexico. J. Forest. Res. 32, 637–648.
Alvarez-Lopeztello, J., del Castillo, R. F., Robles, C., and Hernández-Cuevas, L. V. (2019). Spore diversity of arbuscular mycorrhizal fungi in human-modified neotropical ecosystems. Ecol. Res. 34, 394–405.
Anonymous (2022). Flora Mesoamericana, Missouri Botanical Garden, Universidad Autónoma de México. London: The Natural History Museum.
Anta, S. (2007). Áreas Naturales de Conservación Voluntaria, Vol. 182. México: Consejo Civil Mexicano para la Silvicultura Sostenible, A.C, 1–23.
Antonelli, A., Ariza, M., Albert, J., Andermann, T., Azevedo, J., Bacon, C., et al. (2018). Conceptual and empirical advances in Neotropical biodiversity research. PeerJ 6:e5644. doi: 10.7717/peerj.5644
Arbelaez-Cortés, E., Nyari, A. S., and Navarro-Sigüenza, A. G. (2010). The differential effect of lowlands on the phylogeographic pattern of a Mesoamerican montane species (Lepidocolaptes affinis, Aves: Furnariidae). Mol. Phylogenet. Evol. 57, 658–668. doi: 10.1016/j.ympev.2010.06.013
Arias-Montes, S., Gama-López, S., and Guzman-Cruz, L. (1997). Cactaceae. Flora del Valle de Tehuacan-Cuicatlan. Fasciculo 14. Mexico: Universidad Nacional Autónoma de México.
Arizmendi, M. D. C., and de Los Monteros, A. E. (1996). Avifauna de los bosques de cactáceas columnares del Valle de Tehuacán, Puebla. Acta Zool. Mex. 67, 25–46.
Arkonada, K. (2019). Cinco ecosistemas únicos de Nicaragua en riesgo de desaparecer. Temas Nicaraguenses 137, 44–53.
Armendáriz, T. F. (2012). New record and extension of the distribution range of the bark beetle Dendroctonus rhizophagus (Curculionidae: Scolytinae). Rev. Mex. Biodiv. 83, 850–853.
Aryal, D. R., Morales Ruiz, D. E., Tondopó Marroquín, C. N. R., Pinto Ruiz, R., Guevara Hernández, F., Venegas Venegas, J. A., et al. (2018). Soil organic carbon depletion from forests to grasslands conversion in Mexico: a review. Agriculture 8:181.
Asbjornsen, H., Velázquez-Rosas, N., García-Soriano, R., and Gallardo-Hernández, C. (2005). Deep ground fires cause massive above-and below-ground biomass losses in tropical montane cloud forests in Oaxaca, Mexico. J. Trop. Ecol. 21, 427–434.
Baktavachalam, G. B., Delaney, B., Fisher, T. L., Ladics, G. S., Layton, R. J., Locke, M. E., et al. (2015). Transgenic maize event TC1507: global status of food, feed, and environmental safety. GM Crops Food 6, 80–102. doi: 10.1080/21645698.2015.1054093
Barthell, J., Bradleigh Vinson, S., Frankie, G. W., La Salle, J., and Gauld, I. D. (1993). Threats to the Diversity of Solitary Bees in a Neotropical Dry Forest in Central America Hymenoptera and Biodiversity. Wallingford: CAB International.
Batke, S. V. E. N., Cascante-Marin, A., and Kelly, D. (2016). Epiphytes in Honduras: a geographical analysis of the vascular epiphyte flora and its floristic affinities to other Central American countries. Trop. Ecol. 57, 663–675.
Bautista-Cruz, M. A., and del Castillo, R. F. (2005). Soil changes during secondary succession in a tropical montane cloud forest area. Soil Sci. Soc. Am. J. 69, 906–914.
Begon, M., Mortimer, M., and Thompson, D. J. (1996). ). Population Ecology. An Unified Study of Animals and Plants. Oxford: Blackwell.
Bell, G., and Collins, S. (2008). Adaptation, extinction, and global change. Evol. Appl. 1, 3–16. doi: 10.1111/j.1752-4571.2007.00011.x
Bellon, M. R., and Berthaud, J. (2004). Transgenic maize and the evolution of landrace diversity in Mexico. The importance of farmers’ behavior. Plant Physiol. 134, 883–888. doi: 10.1104/pp.103.038331
Bellon, M. R., Mastretta-Yanes, A., Ponce-Mendoza, A., Ortiz-Santamaría, D., Oliveros-Galindo, O., Perales, H., et al. (2018). Evolutionary and food supply implications of ongoing maize domestication by Mexican campesinos. Proc. R. Soc. Lond. B 285:20181049. doi: 10.1098/rspb.2018.1049
Benz, B. F. (2001). Archaeological evidence of teosinte domestication from Guilá Naquitz, Oaxaca. Proc. Natl. Acad. Sci. U.S.A. 98, 2104–2106. doi: 10.1073/pnas.98.4.2104
Billings, R. F., Clarke, S. R., Espino-Mendoza, V., Cordón Cabrera, P., Meléndez Figueroa, B., Ramón Campos, J., et al. (2004). Bark beetle outbreaks and fire: a devastating combination for Central America’s pine forests. Unasylva 217, 15–21.
Blanco, G., Tella, J. L., Díaz-Luque, J. A., and Hiraldo, F. (2019). Multiple external seed dispersers challenge the megafaunal syndrome anachronism and the surrogate ecological function of livestock. Front. Ecol. Evol. 7:328. doi: 10.3389/fevo.2019.00328
Boege, E. (2008). El patrimonio Biocultural de los Pueblos Indígenas de México. México: Instituto Nacional de Antropología e Historia.
Bogoni, J. A., Peres, C. A., and Ferraz, K. M. (2020). Extent, intensity and drivers of mammal defaunation: a continental-scale analysis across the Neotropics. Sci. Rep. 10:14750. doi: 10.1038/s41598-020-72010-w
Bommer, J. J., and Rodríguez, C. E. X. (2002). Earthquake-induced landslides in Central America. Eng. Geol. 63, 189–220.
Brand, U., and Görg, C. (2003). The state and the regulation of biodiversity: international biopolitics and the case of Mexico. Geoforum 34, 221–233.
Bray, D. B. (2009). “Forest cover dynamics and forest transitions in Mexico and Central America: towards a great restoration,” in Reforesting Landscapes: Linking pattern and process, eds H. Nagendra and J. Southwood (Dordrecht: Springer), 85–120.
Briones-Salas, M., Lavariega, M. C., and Lira-Torres, I. (2012). Distribución actual y potencial del jaguar (Panthera onca) en Oaxaca, México. Rev. Mex. Biodiv. 83, 246–257.
Birf-aif (2021). Banco Mundial BIF -AIF. Available online at: https://datos.bancomundial.org/indicador/SP.POP.TOTL?end=2020&locations=SV&start=1960 (accessed August 5, 2021).
Brown, C. H. (2010). “Development of agriculture in prehistoric Mesoamerica. The linguistic evidence,” in Pre-Columbian Foodways, eds J. Staller and M. Carrasco (New York, NY: Springer), 71–107.
Bruijnzeel, L. A., and Proctor, J. (1995). “Hydrology and biogeochemistry of tropical montane cloud forest: what do we really know?,” in Tropical Montane Forests, eds L. S. Hamilton, J. O. Juvick, and F. N. Scatena (New York, NY: Springer Verlag), 38–78.
Bush, M. B. (2002). Distributional change and conservation on the Andean flank: a palaeoecological perspective. Glob. Ecol. Biogeogr. 11, 463–473.
Caballero, J., Casas, A., Cortés, L., and Mapes, C. (1998). Patrones en el conocimiento, uso y manejo de plantas en pueblos indígenas de México. Rev. Estud. Atacameños 16, 181–196.
Cáceres, C. F. (2011). Using GIS in hotspots analysis and for forest fire risk zones mapping in the Yeguare Region, Southeastern Honduras. Pap. Resour. Anal. 13, 1–14.
Cai, W., Wang, G., Santoso, A., McPhaden, M. J., Wu, L., Jin, F. F., et al. (2015). Increased frequency of extreme La Niña events under greenhouse warming. Nat. Clim. Change 5, 132–137. doi: 10.1016/j.etap.2020.103508
Caldwell, M. M., Robberecht, R., and Billings, W. D. (1980). A steep latitudinal gradient of solar ultraviolet-B radiation in the arctic-alpine life zone. Ecology 61, 600–611.
Calvo-Alvarado, J., McLennan, B., ánchez-Azofeifa, A. S., and Garvin, T. (2009). Deforestation and forest restoration in Guanacaste, Costa Rica: putting conservation policies in context. For. Ecol. Manag. 258, 931–940.
Cane, M. A. (2005). The evolution of El Niño, past and future. Earth Planet. Sci. Lett. 230, 227–240.
Canuto, M. A., and Bell, E. E. (2013). Archaeological investigations in the El Paraíso Valley: the role of secondary centers in the multiethnic landscape of Classic period Copan. Anc. Mesoam. 24, 1–24.
Casas, A., Otero-Arnaiz, A., érez-Negrón, E. P., and Valiente-Banuet, A. (2007). In situ management and domestication of plants in Mesoamerica. Ann. Bot. 100, 1101–1115. doi: 10.1093/aob/mcm126
Castanet, C., Purdue, L., Testé, M., Garnier, A., Develle-Vincent, A. L., Mokadem, F., et al. (2022). Multi-millennial human impacts and climate change during the Maya early Anthropocene: implications on hydro-sedimentary dynamics and socio-environmental trajectories (Naachtun, Guatemala). Quat. Sci. Rev. 283, 107458.
Catling, P. M., and Lefkovitch, L. P. (1989). Associations of vascular epiphytes in a Guatemalan cloud forest. Biotropica 21, 35–40.
Cavalli-Sforza, L. L., and Feldman, M. W. (1981). Cultural Transmission and Evolution: A Quantitative Approach. Princeton, NJ: Princeton University Press.
Cavelier, J., Solis, D., and Jaramillo, M. A. (1996). Fog interception in montane forest across the Central cordillera of Panamá. J. Trop. Ecol. 12, 357–369.
Challenger, A. (1998). Utilización y Conservación de los Ecosistemas Terrestres de México, Pasado, Presente y Futuro. México D.F: Comisión Nacional para el Conocimiento y Uso de la Biodiversidad, Instituto de Biología, Universidad Nacional Autónoma de Mèxico, Agrupación Sierra Madre, S.C.
Chávez-Pesqueira, M., and Núñez-Farfán, J. (2017). Domestication and genetics of papaya: a review. Front. Ecol. Evol. 5:155. doi: 10.3389/fevo.2017.00155
Cheng, T. L., Rovito, S. M., Wake, D. B., and Vredenburg, V. T. (2011). Coincident mass extirpation of neotropical amphibians with the emergence of the infectious fungal pathogen Batrachochytrium dendrobatidis. Proc. Natl. Acad. Sci. U.S.A. 108, 9502–9507. doi: 10.1073/pnas.1105538108
Clement, R. M., and Horn, S. P. (2001). Pre-Columbian land-use history in Costa Rica: a 3000-year record of forest clearance, agriculture and fires from Laguna Zoncho. Holocene 11, 419–426.
Clewell, A. F. (1973). Floristic composition of a stand of Pinus oocarpa in Honduras. Biotropica 5, 175–182.
Coba, L. Y. (2005). Los Pueblos Indígenas de Panamá: Diagnóstico Sociodemográfico a Partir del Censo del 2000. Santiago de Chile: Comisión Económica para América Latina y el Caribe (CEPAL).
Cody, S., Richardson, J. E., Rull, V., Ellis, C., and Pennington, R. T. (2010). The great American biotic interchange revisited. Ecography 33, 326–332.
Colwell, R. K., Brehm, G., Cardelús, C.-L., Gilman, A. C., and Longino, J. T. (2008). Global warming, elevational range shifts, and lowland biotic attrition in the wet tropics. Science 322, 258–261. doi: 10.1126/science.1162547
Comisión Económica para América Latina y el Caribe [CEPAL] (2015). Estudio Económico de América Latina y el Caribe 2015: Desafíos Para Impulsar el Ciclo de Inversión con Miras a Reactivar el Crecimiento. Available online at: https://www.cepal.org/es/publicaciones/38713-estudio-economico-america-latina-caribe-2015-desafios-impulsar-ciclo-inversion (accessed April 8, 2021).
Comisión Nacional Forestal [CONAFOR], and Secretaría de Medio Ambiente y Recursos Naturales [SEMARNAT] (2021). Número de Incendios Forestales. Mexico: SEMARNAT.
Comisión Nacional para el Conocimiento y Uso de la Biodiversidad [CONABIO] (2021). El Corredor Biológico Mesoamericano. Available online at: https://www.biodiversidad.gob.mx/corredor/corredoresbio.html (accessed August 16, 2021).
Connell, J. H., and Slatyer, R. O. (1977). Mechanisms of succession in natural communities and their role in community stability and organization. Am. Nat. 111, 1119–1144. doi: 10.1002/hpm.3005
Conservation International [CI] (2007). Biodiversity Hotspots: The Most Remarkable Places on Earth Are also the Most Threatened. Arlington, VA: Conservation International.
Cook, N. D., and Lovell, W. G. (2001). Secret Judgments of God: Old World Disease in Colonial Spanish 530 America. Norman: University of Oklahoma Press.
Cordova, J., and del Castillo, R. F. (2001). “Changes in epiphyte cover in three chronosequences in a tropical montane cloud forest in Mexico,” in Life Forms and Dynamics in Tropical Forests. Dissertationes Botanicae, Vol. 346, eds G. Gottsberger and S. Liede (Berlin: J. Cramer in der Gebrüder Borntraeger Verlagsbuchhandlung), 79–94.
Cornell, H. V., and Harrison, S. P. (2014). What are species pools and when are they important? Annu. Rev. Ecol. Evol. Syst. 45, 45–67.
Cousens, R., Dytham, C., and Law, R. (2008). Dispersal in Plants a Population Perspective. Oxford: Oxford University.
Cox, C. J., Ladle, R. J., and Moore, P. D. (2019). Biogeography, an Ecological and Evolutionary Approach, 10 Edn. New York, NY: Wiley.
Cruz-Garcia, G., Lagunez-Rivera, L., Chavez-Angeles, M. G., and Solano-Gómez, R. (2015). The wild orchid trade in a Mexican local market: diversity and economics. Econ. Bot. 69, 291–305.
Cruz-Ruiz, G. I. D., Mondragón, D., and Santos-Moreno, A. (2012). The presence of Abronia oaxacae (Squamata: Anguidae) in tank bromeliads in temperate forests of Oaxaca, Mexico. Braz. J. Biol. 72, 337–341. doi: 10.1590/s1519-69842012000200015
Dalling, J. W. (1994). Vegetation colonization of landslides in the blue mountains, Jamaica. Biotropica 26, 392–399.
Darwin, C. (1859). On the Origin of Species by Means of Natural Selection, or the Preservation of Favoured Races in the Struggle for Life. London: John Murray.
Dávila, P., Arizmendi, M. D. C., Valiente-Banuet, A., Villaseñor, J. L., Casas, A., and Lira, R. (2002). Biological diversity in the Tehuacán-Cuicatlán valley, México. Biodiv. Conserv. 11, 421–442.
Dávila, S. L., Stinnesbeck, S. R., Gonzalez, S., Lindauer, S., Escamilla, J., and Stinnesbeck, W. (2019). Guatemala’s late Pleistocene (Rancholabrean) fauna: revision and interpretation. Quat. Sci. Rev. 219, 277–296.
De Bethencourt Massieu, A. (1960). Arboladura de Santa María de Chimalapa-Tehuantepec en las construcciones navales indianas (1730-1750). Rev. Indias 20:79.
de la Luz Avendaño-Yáñez, M., Sánchez-Velásquez, L. R., Meave, J. A., and del Rosario Pineda-López, M. (2014). Is facilitation a promising strategy for cloud forest restoration? Forest Ecol. Manag. 329, 328–333.
DeClerck, F. A., Chazdon, R., Holl, K. D., Milder, J. C., Finegan, B., Martinez-Salinas, A., et al. (2010). Biodiversity conservation in human-modified landscapes of Mesoamerica: past, present and future. Biol. Conserv. 143, 2301–2313.
del Castillo, R. F. (1996). Ensayo sobre el fenómeno calcícola-calcífuga en cactáceas mexicanas. Cact. Suculent. Mex. 41, 3–11.
del Castillo, R. F. (2015). A conceptual framework to describe the ecology of fragmented landscapes and implications for conservation and management. Ecol. Appl. 25, 1447–1455. doi: 10.1890/14-1964.1
del Castillo, R. F., and Acosta-Castellanos, S. (2002). Ethnobotanical notes on Pinus strobus v. chiapensis. Anal. Inst. Biol. Univ. Nacl. Autón. Méx. Ser. Bot. 7, 319–327.
del Castillo, R. F., and Blanco-Macías, A. (2007). “Secondary succession under a slash-and-burn regime in a tropical montane cloud forest: soil and vegetation characteristics,” in Biodiversity Loss and Conservation in Fragmented Forest Landscapes. Evidence from the Forests of Montane Mexico and Temperate South America, ed. A. C. Newton (Wallingford: CAB International), 158–180.
del Castillo, R. F., Terrazas, T., Trujillo, A. S., and Rivera García, R. (2020). Use of remote sensing and anatomical evidence at contrasting elevations to infer climate change sensitivity: preliminary results in Pinus patula. Bot. Sci. 98, 248–263.
del Castillo, R. F., Trujillo, A. S., Rivera García, R., Gómez-Ocampo, Z., and Mondragón Chaparro, D. (2013). Possible combined effects of climate change, deforestation, and harvesting on the epiphyte Catopsis compacta: a multidisciplinary approach. Ecol. Evol. 3, 3935–3945. doi: 10.1002/ece3.765
del Castillo, R. F., Trujillo, A. S., Sánchez-Vargas, N., and Newton, A. C. (2011). Genetic factors associated with population size may increase extinction risks and decrease colonization potential in a key stone tropical pine. Evol. Appl. 4, 574–588. doi: 10.1111/j.1752-4571.2010.00177.x
Delgado-Lemus, A., Torres, I., Blancas, J., and Casas, A. (2014). Vulnerability and risk management of Agave species in the Tehuacan Valley, México. J. Ethnobiol. Ethnomed. 10:53. doi: 10.1186/1746-4269-10-53
Denslow, J. S. (1980). Paterns of plant species diversity during succession under different disturbance regimes. Oecologia 46, 18–21.
Denslow, J. S. (1995). Disturbance and diversity in tropical rain forests: the density effect. Ecol. Appl. 5, 962–968.
Derroire, G., Balvanera, P., Castellanos-Castro, C., Decocq, G., Kennard, D. K., Lebrija-Trejos, E., et al. (2016). Resilience of tropical dry forests–a meta-analysis of changes in species diversity and composition during secondary succession. Oikos 125, 1386–1397.
Diemont, S. A., and Martin, J. F. (2009). Lacandon Maya ecosystem management: sustainable design for subsistence and environmental restoration. Ecol. Appl. 19, 254–266. doi: 10.1890/08-0176.1
Dirzo, R., Young, H. S., Galetti, M., Ceballos, G., Isaac, N. J., and Collen, B. (2014). Defaunation in the Anthropocene. Science 345, 401–406. doi: 10.1126/science.1251817
Divoll, T. J., and Buck, D. G. (2013). Noteworthy field observations of cave roosting bats in Honduras. Mastozool. Neotrop. 20, 149–151.
Domínguez-Yescas, R., Vázquez-García, J. A., Muñiz-Castro, M. Á, Hernández-Vera, G., Salcedo-Pérez, E., Rodríguez-Pérez, C., et al. (2020). Small-scale environmental drivers of plant community structure and diversity in neotropical montane cloud forests harboring threatened Magnolia dealbata in Southern Mexico. Diversity 12:444.
Dull, R. A. (2016). The Maize Revolution A View from El Salvador. Histories of Maize in Mesoamerica: Multidisciplinary Approaches. Walnut Creek, CA: Left Coast Press.
Enríquez, E., Ayala, R., Gonzalez, V. H., and Núñez-Farfán, J. (2015). Alpha and beta diversity of bees and their pollination role on Cucurbita pepo L. kaup (Cucurbitaceae) in the Guatemalan cloud forest. Pan Pac. Entomol. 91, 211–222.
EspacioHonduras (2021). espaciohonduras.net. Available online at: https://www.espaciohonduras.net/cultura/grupos-etnicos-de-honduras (accessed August 5, 2021).
Fahrig, L. (2003). Effects of habitat fragmentation on biodiversity. Annu. Rev. Ecol. Evol. Syst. 34, 487–515.
Fedrick, J. M. (2003). Información Sobre los Pueblos Indígenas de Nicaragua como Insumo para el Proyecto Regional de Manejo Integrado de Ecosistemas por Pueblos Indígenas y Comunidades de Centroamerica. Nicaragua: RUTA.
Feinman, G. M., and Nicholas, L. M. (2020). Relict plant communities at prehispanic sites in Oaxaca. México: historical implications. Hum. Ecol. 48, 539–555.
Figel, J. J., Durán, E. L., Bray, D. B., and Priscilano-Vázquez, J. R. (2009). New jaguar records from montane forest at a priority site in southern Mexico. CATnews 50, 14–15.
Finley-Brook, M. (2007). Green neoliberal space: the Mesoamerican biological corridor. J. Latin Am. Geogr. 6, 101–124.
Frank, H. K., Mendenhall, C. D., Judson, S. D., Daily, G. C., and Hadly, E. A. (2016). Anthropogenic impacts on Costa Rican bat parasitism are sex specific. Ecol. Evol. 6, 4898–4909. doi: 10.1002/ece3.2245
Frankham, R., Ballou, J. D., and Briscoe, D. A. (2002). Introduction to Conservation Genetics. Cambridge: Cambridge University Press.
Fuhlendorf, S. D., Harrell, W. C., Engle, D. M., Hamilton, R. G., Davis, C. A., and Leslie, D. M. Jr. (2006). Should heterogeneity be the basis for conservation? Grassland bird response to fire and grazing. Ecol. Appl. 16, 1706–1716. doi: 10.1890/1051-0761(2006)016[1706:shbtbf]2.0.co;2
Gadek, C. R., Newsome, S. D., Beckman, E. J., Chavez, A. N., Galen, S. C., Bautista, E., et al. (2018). Why are tropical mountain passes “low” for some species? Genetic and stable-isotope tests for differentiation, migration and expansion in elevational generalist songbirds. J. Anim. Ecol. 87, 741–753. doi: 10.1111/1365-2656.12779
Galindo-Jaimes, L., González-Espinosa, M., Quintana-Ascencio, P. F., and García Barrios, L. (2002). Tree composition and structure in disturbed stands with varying dominance by Pinus spp. in the highlands of Chiapas, México. Plant Ecol. 162, 259–272.
García-Barrios, L., and González-Espinosa, M. (2004). Change in oak to pine dominance in secondary forests may reduce shifting agriculture yields: experimental evidence from Chiapas, Mexico. Agric. Ecosyst. Environ. 102, 389–401.
Garwood, N. C., Janos, D. P., and Brokaw, N. (1979). Earthquake-caused landslides: a major disturbance to tropical forests. Science. 205, 997–999. doi: 10.1126/science.205.4410.997
Gaston, K. J., and Williams, P. H. (1996). “Spatial patterns in taxonomic diversity,” in Biodiversity: A Biology of Numbers and Difference, ed. K. J. Gaston (Oxford: Blackwell Science, Limited), 202–229.
Gillespie, T. W., Grijalva, A., and Farris, C. N. (2000). Diversity, composition, and structure of tropical dry forests in Central America. Plant Ecol. 147, 37–47.
Gobierno del Estado de Oaxaca (2020). Ley de Desarrollo Forestal Sustentable del Estado de Oaxaca, Decreto 1711, LXIV Legislatura Constitucional, Periódico Oficial del Gobierno del Estado de Oaxaca. Oaxaca: Gobierno del Estado de Oaxaca location.
Goettsch, B., and Hernández, H. M. (2006). Beta diversity and similarity among cactus assemblages in the Chihuahuan Desert. J. Arid Environ. 65, 513–528.
Gómez-Pineda, E., Hammond, W. M., Trejo-Ramirez, O., Gil-Fernández, M., Allen, C. D., Blanco-García, A., et al. (2022). Drought years promote bark beetle outbreaks in Mexican forests of Abies religiosa and Pinus pseudostrobus. For. Ecol. Manag. 505:119944.
González, R. J. (2001). Zapotec Science. Farming and Food in the Northern Sierra of Oaxaca. Austin, TX: University of Texas Press.
González Espinosa, M. (2000). Invasión de Pinus spp. y la conservación de la diversidad florística en Los Altos de Chiapas, México: Informe final SNIB-CONABIO Proyecto No. L329. México: El Colegio de la Frontera Sur, 105.
González-Espinosa, M., Quintana-Ascencio, P. F., Ramírez-Marcial, N., and Gaytán-Guzmán, P. (1991). Secondary succession in disturbed Pinus-Quercus forests in the highlands of Chiapas, Mexico. J. Veg. Sci. 2, 351–360.
González-Espinosa, M., Ramirez-Marcial, N., and Galindo-Jaimes, L. (2006). “Secondary succession in montane pine-oak forests of Chiapas, México,” in Ecology and Conservation of Neotropical Montane Oak Forests, ed. M. Kappelle (Berlin: Springer), 209–221.
González-Martín, A., Gorostiza, A., Regalado-Liu, L., Arroyo-Peña, S., Tirado, S., Nuño-Arana, I., et al. (2015). Demographic history of indigenous populations in Mesoamerica based on mtDNA sequence data. PLoS One 10:e0131791. doi: 10.1371/journal.pone.0131791
Grandia, L. (2007). Between bolivar and bureaucracy: the Mesoamerican biological corridor. Conserv. Soc. 5, 478–503.
Greller, A. M. (2000). “Vegetation in the floristic regions of North and Central America,” in Imperfect Balance, ed. D. L. Lentz (New York, NY: Columbia University Press), 39–88. doi: 10.1073/pnas.1714977115
Griscom, H. P., and Ashton, M. S. (2011). Restoration of dry tropical forests in Central America: a review of pattern and process. For. Ecol. Manag. 261, 1564–1579.
Guariguata, M. R., and Ostertag, R. (2001). Neotropical secondary forest succession: changes in structural and functional characteristics. For. Ecol. Manag. 148, 185–206.
Guevara, S., and Laborde, J. (2014). The Mesoamerican rain forest environmental history. Livestock and landscape biodiversity at Los Tuxtlas, México. Pastos 42, 219–248.
Hall, R. (2001). Faunal and Floral Migrations and Evolution in SE Asia-Australasia, eds I. Metcalfe, J. M. B. Smith, M. Morwood, and I. Davidson (Lisse: A.A. Balkema Publishers).
Hammond, N. (2001). “Los orígenes de la cultura maya y la formación de las comunidades rurales,” in Los Mayas una Civilización Milenaria, eds N. Grube, E. Eggebrecht, and M. Scidel (Cologne: Könemann), 34–47.
Harp, E. L., Hagaman, K. W., Held, M. D., and McKenna, J. P. (2002). Digital Inventory of Landslides and Related Deposits in Honduras Triggered by Hurricane Mitch. Reston, VA: U.S. Geological Survey.
Harris, A. J., Vallance, J. W., Kimberly, P., Rose, W. I., Matáas, O., Bunzendahl, E., et al. (2006). Downstream aggradation owing to lava dome extrusion and rainfall runoff at Volcan Santiaguito, Guatemala. Spec. Pap. Geol. Soc. Am. 412:85.
Harvey, C. A., Komar, O., Chazdon, R., Ferguson, B. G., Finegan, B., Griffith, D. M., et al. (2008). Integrating agricultural landscapes with biodiversity conservation in the Mesoamerican hotspot. Conserv. Biol. 22, 8–15. doi: 10.1111/j.1523-1739.2007.00863.x
Harvey, C. A., Saborio-Rodríguez, M., Martínez-Rodríguez, M. R., Viguera, B., Chain-Guadarrama, A., Vignola, R., et al. (2018). Climate change impacts and adaptation among smallholder farmers in Central America. Agric. Food Sec. 7, 1–20. doi: 10.1007/s11027-015-9696-2
Harvey, W. J., Nogué, S., Stansell, N., Adolf, C., Long, P. R., and Willis, K. (2021b). A palynological perspective on the impacts of European contact: historic deforestation, ranching and agriculture surrounding the Cuchumatanes Highlands, Guatemala. Veg. Hist. Archaeobot. 30, 395–408.
Harvey, W. J., Petrokofsky, G., Stansell, N., Nogué, S., Petrokofsky, L., and Willis, K. J. (2021a). Forests, water, and land use change across the Central American Isthmus: mapping the evidence base for terrestrial holocene palaeoenvironmental proxies. Forests 12:1057.
Hildebrandt, A., and Eltahir, E. A. (2008). Using a horizontal precipitation model to investigate the role of turbulent cloud deposition in survival of a seasonal cloud forest in Dhofar. J. Geophys. Res. Biogeosci. 113:G04028.
Hilje, B., Calvo-Alvarado, J., Jiménez-Rodríguez, C., and ánchez-Azofeifa, A. S. (2015). Tree species composition, breeding systems, and pollination and dispersal syndromes in three forest successional stages in a tropical dry forest in Mesoamerica. Trop. Conserv. Sci. 8, 76–94.
Holland, P. G., and Steyn, D. G. (1975). Vegetational responses to latitudinal variations in slope angle and aspect. J. Biogeogr. 2, 179–183.
Hooghiemstra, H., Cleef, A. M., Noldus, C. W., and Kappelle, M. (1992). Upper quaternary vegetation dynamics and palaeoclimatology of the La Chonta bog area (Cordillera de Talamanca, Costa Rica). J. Quat. Sci. 7, 205–225.
Horn, D. J. (2012). “Pest management: ecological aspects,” in Encyclopedia of Environmental Management, ed. S. E. Jorgensen (Boca Raton, FL: CRC Press), 1934–1936.
Horn, S. P. (2016). “Pre-Columbian maize agriculture in Costa Rica,” in Histories of Maize in Mesoamerica: Multidisciplinary Approaches, eds J. E. Staller, R. H. Tykot, and B. F. Benz (London: Routledge), 104–117. doi: 10.1111/nph.16936
Horst, O. (1989). The persistence of milpa agriculture in highland Guatemala. J. Cult. Geogr. 9, 13–29.
Hudson, J., Kellman, M., Sanmugadas, K., and Alvarado, C. (1983). Prescribed burning of Pinus oocarpa in Honduras I. Effects on surface runoff and sediment loss. For. Ecol. Manag. 5, 269–281.
Hünemeier, T., Amorim, C. E. G., Azevedo, S., Contini, V., Acuña-Alonzo, V., Rothhammer, F., et al. (2012). Evolutionary responses to a constructed niche: ancient Mesoamericans as a model of gene-culture coevolution. PLoS One 7:e38862. doi: 10.1371/journal.pone.0038862
INEGI (2020). Censo de Población y Vivienda. IBEGI. Available online at: https://www.inegi.org.mx/programas/ccpv/2020 (accessed August 8, 2021).
INE-Guatemala (2021). Instituto Nacional de Estadística – Guatemala. Available online at: https://www.ine.gob.gt/ine/ (accessed May 8, 2021).
IWGIA (2021). El Grupo Internacional de Trabajo sobre Asuntos Indígenas (IWGIA). Available online at: https://www.iwgia.org/es/costa-rica/3740-mi-2020-costa-rica.html#:~:text=En%20el%20pa%C3%ADs%20habitan%20ocho,4%25%20de%20la%20poblaci%C3%B3n%20total (accessed August 5, 2021).
Janzen, D. H., and Hallwachs, W. (2019). Perspective: where might be many tropical insects? Biol. Conserv. 233, 102–108.
Janzen, D. H., and Hallwachs, W. (2020). Área de conservación guanacaste, northwestern Costa Rica: converting a tropical national park to conservation via biodevelopment. Biotropica 52, 1017–1029.
Janzen, D. H., and Martin, P. S. (1982). Neotropical anachronisms: the fruits the gomphotheres ate. Science 15, 19–27. doi: 10.1126/science.215.4528.19
Juárez-Martínez, A., and Rodríguez-Trejo, D. A. (2003). Efecto de los incendios forestales en la regeneración de Pinus oocarpa var. ochoterenae. Revista Chapingo. Ser. Cienc. Forest. Amb. 9, 125–130.
Kappelle, M., and van Uffelen, J. G. (2006). “Altitudinal zonation of montane oak forests along climate and soil gradients in Costa Rica,” in Ecology and Conservation of Neotropical Montane Oak Forests, ed. M. Kappelle (Berlin: Springer), 39–54.
Karmalkar, A. V., Bradley, R. S., and Diaz, H. F. (2011). Climate change in Central America and Mexico: regional climate model validation and climate change projections. Clim. Dyn. 37, 605–629. doi: 10.1073/pnas.2331584100
Kassen, R. (2002). The experimental evolution of specialists, generalists, and the maintenance of diversity. J. Evol. Biol. 15, 173–190.
Kaup, M., Trull, S., and Hom, E. F. (2021). On the move: sloths and their epibionts as model mobile ecosystems. Biol. Rev. 96, 2638–2660. doi: 10.1111/brv.12773
Kennett, D. J., Thakar, H. B., VanDerwarker, A. M., Webster, D. L., Culleton, B. J., Harper, T. K., et al. (2017). High-precision chronology for Central American maize diversification from El Gigante rockshelter, Honduras. Proc. Natl. Acad. Sci. U.S.A. 114, 9026–9031. doi: 10.1073/pnas.1705052114
Kim, J. B., Saunders, P., and Finn, J. T. (2005). Rapid assessment of soil erosion in the Rio Lempa Basin, Central America, using the universal soil loss equation and geographic information systems. Environ. Manag. 36, 872–885. doi: 10.1007/s00267-002-0065-z
Kistler, L., Newsom, L. A., Ryan, T. M., Clarke, A. C., Smith, B. D., and Perry, G. H. (2015). Gourds and squashes (Cucurbita spp.) adapted to megafaunal extinction and ecological anachronism through domestication. Proc. Natl. Acad. Sci. U.S.A. 112, 15107–15112. doi: 10.1073/pnas.1516109112
Klinges, D. H., and Scheffers, B. R. (2021). Microgeography, not just latitude, drives climate overlap on mountains from tropical to polar ecosystems. Am. Nat. 197, 75–92. doi: 10.1086/711873
Kosoy, N., Martinez-Tuna, M., Muradian, R., and Martinez-Alier, J. (2007). Payments for environmental services in watersheds: insights from a comparative study of three cases in Central America. Ecol. Econ. 61, 446–455.
Kull, C. A., Ibrahim, C. K., and Meredith, T. C. (2007). Tropical forest transitions and globalization: neo-liberalism, migration, tourism, and international conservation agendas. Soc. Nat. Resour. 20, 723–737.
Lachniet, M. S., and Patterson, W. P. (2009). Oxygen isotope values of precipitation and surface waters in northern central America (Belize and Guatemala) are dominated by temperature and amount effects. Earth Planet. Sci. Lett. 284, 435–446.
Lamb, O. D., Lamur, A., Diaz-Moreno, A., De Angelis, S., Hornby, A. J., von Aulock, F. W., et al. (2019). Disruption of long-term effusive-explosive activity at Santiaguito, Guatemala. Front. Earth Sci. 6:253. doi: 10.3389/feart.2018.00253
Latati, M., Bargaz, A., Belarbi, B., Lazali, M., Benlahrech, S., Tellah, S., et al. (2016). The intercropping common bean with maize improves the rhizobial efficiency, resource use and grain yield under low phosphorus availability. Eur. J. Agron. 72, 80–90.
Lavariega, M. C., Ríos-Solís, J. A., Flores-Martínez, J. J., Galindo-Aguilar, R. E., ánchez-Cordero, V. S., Juan-Albino, S., et al. (2020). Community-based monitoring of jaguar (Panthera onca) in the Chinantla region, Mexico. Trop. Conserv. Sci. 13:1940082920917825.
Lebrija-Trejos, E., Bongers, F., Pérez-García, E. A., and Meave, J. A. (2008). Successional change and resilience of a very dry tropical deciduous forest following shifting agriculture. Biotropica 40, 422–431.
Leigh, E. G., O’Dea, A., and Vermeij, G. J. (2014). Historical biogeography of the Isthmus of Panama. Biol. Rev. 89, 148–172. doi: 10.1111/brv.12048
Lenormand, T. (2002). Gene flow and the limits to natural selection. Trends Ecol. Evol. 17, 183–189.
Leonard, H. (1987). Natural Resource and Economic Development in Central America. Washington, DC: International Institute for Environment and Development.
Lieberman, D., Lieberman, M., Peralta, R., and Hartshorn, G. S. (1996). Tropical forest structure and composition on a large-scale altitudinal gradient in Costa Rica. J. Ecol. 84, 137–152.
Lips, K. R., Brem, F., Brenes, R., Reeve, J. D., Alford, R. A., Voyles, J., et al. (2006). Emerging infectious disease and the loss of biodiversity in a Neotropical amphibian community. Proc. Natl. Acad. Sci. 103, 3165–3170. doi: 10.1073/pnas.0506889103
Lips, K. R., Burrowes, P. A., Mendelson, J. R. III, and Parra-Olea, G. (2005). Amphibian declines in Latin America: widespread population declines, extinctions, and impacts 1 Biotropica. J. Biol. Conserv. 37, 163–165.
Long, A., and Fritz, G. J. (2001). Validity of AMS dates on maize from the Tehuacán Valley: a comment on MacNeish and Eubanks. Latin Am. Antiq. 12, 87–90.
López-Ridaura, S., Barba-Escoto, L., Reyna-Ramirez, C. A., Sum, C., Palacios-Rojas, N., and Gerard, B. (2021). Maize intercropping in the milpa system. Diversity, extent and importance for nutritional security in the Western Highlands of Guatemala. Sci. Rep. 11:3696. doi: 10.1038/s41598-021-82784-2
Love, M. (2007). Recent research in the southern Highlands and Pacific Coast of Mesoamerica. J. Archaeol. Res. 15, 275–328.
Luis-Martínez, J. C., Luna-Cavazos, M., Vibrans, H., and Flores-Cruz, M. (2020). Atributos ecológicos y de hábitat de las especies suculentas del área natural protegida monumento natural Yagul, Oaxaca, México. Bot. Sci. 98, 36–49.
Luna, M. M. S. (2019). Mapping Small Scale Farming in Heterogeneous Landscapes: A Case Study of Smallholder Shade Coffee and Plastic Agriculture Farmers in the Chiapas Highlands, Ph.D. dissertation. Miami, FL: Miami University.
Malamud, B. D., Turcotte, D. L., Guzzetti, F., and Reichenbach, P. (2004). Landslides, earthquakes, and erosion. Earth Planet. Sci. Lett. 229, 45–59.
Marshall, J. S. (2007). The geomorphology and physiographic provinces of Central America. Cent. Am. Geol. Resour. Hazards 1, 75–121.
Martin, M. P., Peters, C. M., Asbjornsen, H., and Ashton, M. S. (2021). Diversity and niche differentiation of a mixed pine–oak forest in the Sierra Norte, Oaxaca, Mexico. Ecosphere 12:e03475.
Martin, P. S. (1973). The discovery of America: the first Americans may have swept the Western hemisphere and decimated its fauna within 1000 years. Science 179, 969–974. doi: 10.1126/science.179.4077.969
Martínez-Carrasco, N. (1998). Atributos poblacionales y reproductivos de Pinus chiapensis en Chiapas, México. Anales del Instituto de Biología de la Universidad Nacional Autónoma de México. Ser. Bot. 69, 119–134.
Martinez-Romero, E. (2003). Diversity of Rhizobium-Phaseolus vulgaris symbiosis: overview and perspectives. Plant Soil 252, 11–23.
Masés-García, C. A., Briones-Salas, M., and Sosa-Escalante, J. E. (2021). Assessment of wildlife crime in a high-biodiversity region of Mexico. J. Nat. Conserv. 59:125932.
McAfee, K. (2008). Beyond techno-science: transgenic maize in the fight over Mexico’s future. Geoforum 39, 148–160.
Meave, J., Kellman, M., MacDougall, A., and Rosales, J. (1991). Riparian habitats as tropical forest refugia. Glob. Ecol. Biogeogr. Lett. 1, 69–76.
Méndez, V. E., Bacon, C. M., Olson, M., Morris, K. S., and Shattuck, A. (2010). Agrobiodiversity and shade coffee smallholder livelihoods: a review and synthesis of ten years of research in Central America. Prof. Geogr. 62, 357–376.
Mendoza-Rosales, C. C., Silva-Romo, G., Centeno-García, E., Campos-Madrigal, E., and Rodríguez-Otero, M. (2013). La formación Chivillas en Tehuacán, Puebla, México: definición, análisis de facies y procedencia. Bolet. Soc. Geol. Mex. 65, 457–480.
Meyrán-García, J. (1973). Guía Botánica de Cactáceas y Otras Suculentas del Valle de Tehuacán. Mexico City: Sociedad Mexicana de Cactología.
Ministerio de Cultura (2021). Available online at: https://www.cultura.gob.sv (accessed August 8, 2021).
Moline, A. (1999). Tropical dry forest restoration in the Guanacaste Conservation Area, Costa Rica. Restor. Reclam. Rev. 4, 1–7.
Mueller, R. G., Joyce, A. A., and Borejsza, A. (2012). Alluvial archives of the Nochixtlan valley, Oaxaca, Mexico: age and significance for reconstructions of environmental change. Palaeogeogr. Palaeoclimatol. Palaeoecol. 321, 121–136.
Munkacsi, A. B., Stoxen, S., and May, G. (2008). Ustilago maydis populations tracked maize through domestication and cultivation in the Americas. Proc. R. Soc. Lond. B Biol. Sci. 275, 1037–1046. doi: 10.1098/rspb.2007.1636
Myers, N., Mittermeier, R. A., Mittermeier, C. G., da Fonseca, G. A. B., and Kent, J. (2000). Biodiversity hotspots for conservation priorities. Nature 403, 853–858.
Negrete-Yankelevich, S., Maldonado-Mendoza, I. E., Lázaro-Castellanos, J. O., Sangabriel-Conde, W., and Martínez-Álvarez, J. C. (2013). Arbuscular mycorrhizal root colonization and soil P availability are positively related to agrodiversity in Mexican maize polycultures. Biol. Fertil. Soils 49, 201–212.
Nieto-Angel, R., Ortiz, J., González-Andrés, F., and Borys, M. W. (1997). Endocarp morphology as an aid for discriminating wild and cultivated Mexican hawthorn (Crataegus mexicana Moc and Sessé). Fruits 5, 317–324.
Nigh, R., and Diemont, S. A. (2013). The Maya milpa: fire and the legacy of living soil. Front. Ecol. Environ. 1:e45–e54. doi: 10.1890/120344
Nixon, K. C. (2006). “Global and neotropical distribution and diversity of Oak (genus Quercus) and Oak forests,” in Ecology and Conservation of Neotropical Montane Oak Forests: Ecological Studies, Vol. 185, ed. M. Kappelle (Berlin: Springer), 3–13.
Novotny, I. P., Tittonell, P., Fuentes-Ponce, M. H., López-Ridaura, S., and Rossing, W. A. (2021). The importance of the traditional milpa in food security and nutritional self-sufficiency in the highlands of Oaxaca, Mexico. PLoS One 16:e0246281. doi: 10.1371/journal.pone.0246281
O’Neill, R. V. (2001). Is it time to bury the ecosystem concept? (with full military honors, of course!). Ecology 82, 3275–3284.
Ornelas, J. F., Ortiz-Rodriguez, A. E., Ruiz-Sanchez, E., Sosa, V., and Pérez-Farrera, M. Á (2019). Ups and downs: genetic differentiation among populations of the Podocarpus (Podocarpaceae) species in Mesoamerica. Mol. Phylogenet. Evol. 138, 17–30. doi: 10.1016/j.ympev.2019.05.025
Ornelas, J. F., Sosa, V., Soltis, D. E., Daza, J. M., González, C., Soltis, P. S., et al. (2013). Comparative phylogeographic analyses illustrate the complex evolutionary history of threatened cloud forests of northern Mesoamerica. PLoS One 8:e56283. doi: 10.1371/journal.pone.0056283
Ozanne, C. M. P., Anhuf, D. S., Boulter, L., Keller, M., Kitching, R. L., Körner, C., et al. (2003). Biodiversity meets the atmosphere: global view of forest Canopies. Science 301, 183–186. doi: 10.1126/science.1084507
Parmesan, C., and Yohe, G. (2003). A globally coherent fingerprint of climate change impacts across natural systems. Nature 421, 37–42. doi: 10.1038/nature01286
Pascual-Mendoza, S., Manzanero-Medina, G. I., Saynes-Vásquez, A., and ásquez-Davila, M. A. V. (2020). Sistemas agroforestales de una comunidad zapoteca de la Sierra Norte de Oaxaca, México. Bot. Sci. 98, 128–144.
Payne, R. (2002). Community forestry and the politics of agrarian reform in the Chimalapas, Oaxaca. J. Sust. Forest. 15, 95–112.
Pazos-Almada, B., and Bray, D. B. (2018). Community-based land sparing: territorial land-use zoning and forest management in the Sierra Norte of Oaxaca, Mexico. Land Use Policy 78, 219–226.
Pennington, T. D., and Sarukhán, J. (1998). Árboles tropicales de México. Manual para la Identificación de las Principales Especies. Mexico: Universidad Nacional Autónoma de México, Fondo de Cultura Económica.
Perales, H. R., Benz, B. F., and Brush, S. B. (2005). Maize diversity and ethnolinguistic diversity in Chiapas, Mexico. Proc. Natl. Acad. Sci. U.S.A. 102, 949–954. doi: 10.1073/pnas.0408701102
Pérez-García, E. A., and Meave, J. A. (2005). Heterogeneity of xerophytic vegetation of limestone outcrops in a tropical deciduous forest region in southern México. Plant Ecol. 175, 147–163.
Pérez-García, O., and del Castillo, R. F. (2016). The decline of the itinerant milpa and the maintenance of traditional agrobiodiversity: crops and weeds coexistence in a tropical cloud forest area in Oaxaca, Mexico. Agric. Ecosyst. Environ. 228, 30–37.
Pérez-García, O., and del Castillo, R. F. (2017). Shifts in swidden agriculture alter the diversity of young fallows: is the regeneration of cloud forest at stake in southern Mexico? Agric. Ecosyst. Environ. 248, 162–174.
Pérez-López, R. I., González-Espinosa, M., Ramírez-Marcial, N., Pérez-Moreno, J., and Toledo-Aceves, T. (2021). Forest management effects on the ectomycorrhizal macromycete community in tropical montane forests in Mexico. Forest Ecol. Manag. 501:119670.
Perfecto, I., Rice, R. A., Greenberg, R., and Van der Voort, M. E. (1996). Shade coffee: a disappearing refuge for biodiversity: shade coffee plantations can contain as much biodiversity as forest habitats. BioScience 46, 598–608.
Perotto-Baldiviezo, H. L., Thurow, T. L., Smith, C. T., Fisher, R. F., and Wu, X. B. (2004). GIS-based spatial analysis and modeling for landslide hazard assessment in steeplands, southern Honduras. Agric. Ecosyst. Environ. 103, 165–176.
Perrigo, A., Hoorn, C., and Antonelli, A. (2020). Why mountains matter for biodiversity. J. Biogeogr. 47, 315–325. doi: 10.1111/gcb.15951
Peterson, A. T., Soberón, J., Pearson, R. G., Anderson, R. S., Martínez-Meyer, E., Nakamura, M., et al. (2011). Ecological Niches and Geographic Distributions. Princeton, NJ: Princeton University Press.
Petracca, L. S., Hernández-Potosme, S., Obando-Sampson, L., Salom-Pérez, R., Quigley, H., and Robinson, H. S. (2014). Agricultural encroachment and lack of enforcement threaten connectivity of range-wide jaguar (Panthera onca) corridor. J. Nat. Conserv. 22, 436–444.
Piñeyro-Nelson, A., Van Heerwaarden, J., Perales, H. R., Serratos-Hernández, J. A., Rangel, A., Hufford, M. B., et al. (2009). Transgenes in Mexican maize: molecular evidence and methodological considerations for GMO detection in landrace populations. Mol. Ecol. 18, 750–761. doi: 10.1111/j.1365-294X.2008.03993.x
Pinto, M. A., Rubink, W. L., Patton, J. C., Coulsen, R. N., and Johnston, J. S. (2005). Africanization in the United States: replacement of feral European honeybees (Apis mellifera L.) by an African hybrid swarm. Genetics 170, 1653–1665. doi: 10.1534/genetics.104.035030
Piperno, D. R., and Flannery, K. V. (2001). The earliest archaeological maize (Zea mays L.) from highland Mexico: new accelerator mass spectrometry dates and their implications. Proc. Natl. Acad. Sci. U.S.A. 98, 2101–2103. doi: 10.1073/pnas.98.4.2101
Piperno, D. R., Clary, K. H., Cooke, R. G., Ranere, A. J., and Weiland, D. (1985). Preceramic maize in central Panama: phytolith and pollen evidence. Am. Anthropol. 87, 871–878.
Poisot, T., Bever, J. D., Nemri, A., Thrall, P. H., and Hochberg, M. E. (2011). A conceptual framework for the evolution of ecological specialisation. Ecol. Lett. 14, 841–851. doi: 10.1111/j.1461-0248.2011.01645.x
Poorter, L., Bongers, F., Aide, T. M., Zambrano, A. M. A., Balvanera, P., Becknell, J. M., et al. (2016). Biomass resilience of Neotropical secondary forests. Nature 530, 211–214. doi: 10.1038/nature16512
Poorter, L., Craven, D., Jakovac, C. C., van der Sande, M. T., Amissah, L., Bongers, F., et al. (2021). Multidimensional tropical forest recovery. Science 374, 1370–1376. doi: 10.1126/science.abh3629
Poveda, G., Waylen, P. R., and Pulwarty, R. S. (2006). Annual and inter-annual variability of the present climate in northern South America and southern Mesoamerica. Palaeogeogr. Palaeoclimatol. Palaeoecol. 234, 3–27.
Prasanna, B. M. (2012). Diversity in global maize germplasm: characterization and utilization. J. Biosci. 37, 1–13. doi: 10.1007/s12038-012-9227-1
Pretty, J., Adams, B., Berkes, F., De Athayde, S. F., Dudley, N., Hunn, E., et al. (2009). The intersections of biological diversity and cultural diversity: towards integration. Conserv. Soc. 7, 100–112.
QGIS Development Team (2017). QGIS Un Sistema de Información Geográfica Libre y de Código Abierto. Available online at: https://qgis.org/es/site/ (accessed April 5, 2021).
Rahbek, C., Borregaard, M. K., Colwell, R. K., Dalsgaard, B., Holt, B. G., Morueta-Holme, N., et al. (2019). Humboldt’s enigma: what causes global patterns of mountain biodiversity? Science 365, 1108–1113. doi: 10.1126/science.aax0149
Ramirez-Bautista, A., and Williams, J. N. (2018). The importance of productivity and seasonality for structuring small rodent diversity across a tropical elevation gradient. Oecologia 190, 275-28. doi: 10.1007/s00442-018-4287-z
Rastogi, R. P., Kumar, A., Tyagi, M. B., and Sinha, R. P. (2010). Molecular mechanisms of ultraviolet radiation-induced DNA damage and repair. J. Nucleic Acids 2010:592980. doi: 10.4061/2010/592980
Raynolds, L. T., and Rosty, C. (2021). Fair Trade USA coffee plantation certification: ramifications for workers in Nicaragua. Dev. Policy Rev. 39, O102–O121.
Rebollar, E. A., Sandoval-Castellanos, E., Roessler, K., Gaut, B. S., Alcaraz, L. D., Benítez, M., et al. (2017). Seasonal changes in a maize-based polyculture of central Mexico reshape the co-occurrence networks of soil bacterial communities. Front. Microbiol. 8:2478. doi: 10.3389/fmicb.2017.02478
Restrepo, C., and Alvarez, N. (2006). Landslides and their contribution to land-cover change in the mountains of Mexico and Central America 1. Biotropica 38, 446–457.
Richards, M., Wells, A., Del Gatto, F., Contreras-Hermosilla, A., and Pommier, D. (2003). Impacts of illegality and barriers to legality: a diagnostic analysis of illegal logging in Honduras and Nicaragua. Int. Forest. Rev. 5, 282–292.
Ríos-Casanova, L., Valiente-Banuet, A., and Rico-Gray, V. (2006). Ant diversity and its relationship with vegetation and soil factors in an alluvial fan of the Tehuacán Valley, Mexico. Acta Oecol. 29, 316–323.
Rosales Adame, J. J., Cevallos Espinosa, J., Castillo y Dzul, ÁA., Caamal Canto, A. R., Dzib Castillo, B. B., Herrera Dzul, ÁJ., et al. (2020). Experiencias de Agroforestería en México. Mexico city: Secretaría de Medio Ambiente y Recursos Naturales.
Rosenswig, R. M., VanDerwarker, A. M., Culleton, B. J., and Kennett, D. J. (2015). Is it agriculture yet? Intensified maize-use at 1000 cal BC in the Soconusco and Mesoamerica. J. Anthropol. Archaeol. 40, 89–108.
Roubik, D. W., Moreno, J. E., Vergara, C., and Wittmann, D. (1986). Sporadic food competition with the African honey bee: projected impact on neotropical social bees. J. Trop. Ecol. 2, 97–111.
Rozzi, R., Pickett, S. T., Palmer, C., Armesto, J. J., and Callicott, J. B. (2013). Linking Ecology and Ethics for a Changing World. New York, NY: Springer.
Rozzi, R. (2018). “Biocultural homogenization: a wicked problem in the Anthropocene,” in From Biocultural Homogenization to Biocultural Conservation, eds R. Rozzi and R. H. May Jr., F. S. Chapin III, F. Massardo, M. C. Gavin, I. J. Klaver, et al. (Cham: Springer Nature), 21–48.
Rull, V. (2011). Neotropical biodiversity: timing and potential drivers. Trends Ecol. Evol. 26, 508–513. doi: 10.1016/j.tree.2011.05.011
Rzedowski, J. (1991). El endemismo en la flora fanerogámica mexicana: una apreciación preliminar. Acta Bot. Mex. 15, 47–64.
Sáenz, F., and Durán-Quesada, A. (2015). A climatology of low-level wind regimes over Central America using a weather type classification approach. Front. Earth Sci. 3:15. doi: 10.3389/feart.2015.00015
Salas-Morales, S. H., and Meave, J. A. (2012). Elevational patterns in the vascular flora of a highly diverse region in southern Mexico. Plant Ecol. 213, 1209–1220.
Sánchez, G. J. J., Stuber, C. W., and Goodman, M. M. (2000). Isozymatic diversity in the races of maize of the Americas. Maydica 45, 185–203.
Sánchez-Montalvo, G., Hernández-Rea, R. A., Leyva-López, J. C., and Mathus-Morales, M. A. (2005). Experiencias de Investigación Forestal en la Plantación de Pino “Ing. Jorge L. Tamayo”. México: Instituto Tecnológico del Valle de Oaxaca, Oaxaca.
Santos Moreno, J. A. (2008). Ecología de Comunidades y Pequeños Mamíferos Terrestres en tres Estados Sucesionales de Bosque Mesófilo de Montaña en la Sierra Norte de Oaxaca, Mexico. Mexico City: Universidad Autónoma Metropolitana.
Sawyer, J. O., and Lindsey, A. A. (1971). Vegetation of the Life Zones in Costa Rica. Muncie, IN: Indiana Academy of Science.
Sheldon, K. S., Huey, R. B., Kaspari, M., and Sanders, N. J. (2018). Fifty years of mountain passes: a perspective on Dan Janzen’s classic article. Am. Nat. 191, 553–565. doi: 10.1086/697046
Sheoran, S., Kumar, S., Kumar, P., Meena, R. S., and Rakshit, S. (2021). Nitrogen fixation in maize: breeding opportunities. Theor. Appl. Genet. 134, 1263–1280. doi: 10.1007/s00122-021-03791-5
SIC-MEXICO (2021). Sistema de INformacion Cultural (SIC MEXICO). Available online at: https://sic.cultura.gob.mx/lista.php?table=inali_li&estado_id=7&municipio_id=-1 (accessed August 5, 2021).
Simberloff, D. (2018). “Nature, culture, and nature culture: the role of nonnative species in biocultures,” in From Biocultural Homogenization to Biocultural Conservation. Ecology and Ethics, Vol. 3, eds R. Rozzi and R. H. May Jr., F. S. Chapin III, F. Massardo, M. Gavin, I. Klaver, et al. (Dordrecht: Springer), 207–218.
Simpson, B. B., and Neff, J. L. (1985). “Plants, their pollinating bees, and the Great American Interchange. I,” in The Great American Biotic Interchange, eds F. G. Stehli and S. D. Webb (Boston, MA: Springer), 427–452.
Singh, S. (2018). Understanding the role of slope aspect in shaping the vegetation attributes and soil properties in Montane ecosystems. Trop. Ecol. 59, 417–430.
Slotten, V., Lentz, D., and Sheets, P. (2020). Landscape management and polyculture in the ancient gardens and fields at Joya de Cerén, El Salvador. J. Anthropol. Archaeol. 59:101191.
Smith, M. A., Hallwachs, W., and Janzen, D. H. (2014). Diversity and phylogenetic community structure of ants along a Costa Rican elevational gradient. Ecography 37, 720–731.
Smith, S. A., and Bermingham, E. (2005). The biogeography of lower Mesoamerican freshwater fishes. J. Biogeogr. 32, 1835–1854.
Solís-Torres, ÓR., Acosta-Ochoa, G., Arroyo-Cabrales, J., Flores-Granados, F., and Roberts, P. (2021). Pleistocene-Holocene human palaeoecology in southern Mexico: stable isotopic evidence from the Santa Marta Cave, Chiapas. J. Archaeol. Sci. Rep. 39:103131.
Standley, P. C., and Steyermark, J. A. (1946). Flora of Guatemala. Botanical Series 24. Chicago, IL: Field Museum of Natural History.
Surovell, T. A., Pelton, S. R., Anderson-Sprecher, R., and Myers, A. D. (2016). Test of Martin’s overkill hypothesis using radiocarbon dates on extinct megafauna. Proc. Natl. Acad. Sci. 113, 886–891. doi: 10.1073/pnas.1504020112
Syring, J., del Castillo, R. F., Cronn, R., and Liston, A. (2007). Multiple nuclear loci reveal the distinctiveness of the threatened neotropical pine Pinus chiapensis. Syst. Bot. 32, 703–727.
Taiz, L., Zeiger, E., Mller, I. M., and Murphy, A. (2015). Plant Physiology and Development, 6th Edn. Sunderland, MA: Sinauer.
Tejeda-Cruz, C., Silva-Rivera, E., Barton, J. R., and Sutherland, W. J. (2010). Why shade coffee does not guarantee biodiversity conservation. Ecol. Soc. 15:13.
Thomas, W. W. (1999). Conservation and monographic research on the flora of Tropical America. Biodiv. Conserv. 8, 1007–1015.
Tian, F., Stevens, N. M., and Buckler, E. S. (2009). Tracking footprints of maize domestication and evidence for a massive selective sweep on chromosome 10. Proc. Natl. Acad. Sci. U.S.A. 106, (Suppl. 1) 9979–9986. doi: 10.1073/pnas.0901122106
Toledo, V., Barrera-Bassols, N., and Camejo Pereira, M. V. (2019). “La milpa y la memoria biocultural de Mesoamérica,” in A Conservação das Sementes Crioulas: Uma Visão Interdisciplinar da Agrobiodiversidade, ed. F. Kessler Dal Soglio (Rio Grande do Sul: Universidade Federal do Rio Grande do Sul Série Ensino, Aprendizagens e Tecnologias (UFRGS), Série Ensino, Aprendizagens e tecnologías), 63–81.
Toly, N. J. (2004). Globalization and the capitalization of nature: a political ecology of biodiversity in Mesoamerica. Bull. Sci.Technol. Soc. 24, 47–54.
Torres-Rodríguez, E., Vallebueno-Estrada, M., González, J. M., Cook, A. G., Montiel, R., and Vielle-Calzada, J. P. (2018). AMS dates of new maize specimens found in rock shelters of the Tehuacán Valley. Radiocarbon 60, 975–987.
Uddin, A., and Lundberg, N. (2004). Miocene sedimentation and subsidence during continent–continent collision, Bengal basin, Bangladesh. Sediment. Geol. 164, 131–146.
Vågene, ÅJ., Herbig, A., Campana, M. G., Robles García, N. M., Warinner, C., Sabin, S., et al. (2018). Salmonella enterica genomes from victims of a major sixteenth-century epidemic in Mexico. Nat. Ecol. Evol. 2, 520–528. doi: 10.1038/s41559-017-0446-6
Valencia, V., Naeem, S., García-Barrios, L., West, P., and Sterling, E. J. (2016). Conservation of tree species of late succession and conservation concern in coffee agroforestry systems. Agric. Ecosyst. Environ. 219, 32–41.
Valverde-Sánchez, R. (2018). Conservation strategies, protected areas, and ecotourism in Costa Rica. J. Park Recreat. Admin. 36, 115–128.
Van de Wouw, M., Kik, C., van Hintum, T., van Treuren, R., and Visser, B. (2010). Genetic erosion in crops: concept, research results and challenges. Plant Genet. Resour. 8, 1–15.
van der Wal, H., Golicher, J. D., Caudillo, S. C., and Domínguez, M. V. (2006). Plant densities, yields and area demands for maize under shifting cultivation in the Chinantla, Mexico. Agrociencia 40, 449–460.
Van Deynze, A., Zamora, P., Delaux, P. M., Heitmann, C., Jayaraman, D., Rajasekar, S., et al. (2018). Nitrogen fixation in a landrace of maize is supported by a mucilage-associated diazotrophic microbiota. PLoS Biol. 16:e2006352. doi: 10.1371/journal.pbio.2006352
van Heerwaarden, J., Hufford, M. B., and Ross-Ibarra, J. (2012). Historical genomics of North American maize. Proc. Natl. Acad. Sci. U.S.A. 109, 12420–12425. doi: 10.1073/pnas.1209275109
Van Vleet, E., Bray, D. B., and Durán, E. (2016). Knowing but not knowing: systematic conservation planning and community conservation in the Sierra Norte of Oaxaca, Mexico. Land Use Policy 59, 504–515.
Van Zonneveld, M., Larranaga, N., Blonder, B., Coradin, L., Hormaza, J. I., and Hunter, D. (2018). Human diets drive range expansion of megafauna-dispersed fruit species. Proc. Natl. Acad. Sci. U.S.A. 115, 3326–3331. doi: 10.1073/pnas.1718045115
Velasco-Murguía, A., del Castillo, R. F., Rös, M., and Rivera-García, R. (2021). Successional pathways of post-milpa fallows in Oaxaca, Mexico. Forest Ecol. Manag. 500;119644.
Velázquez, A., Medina García, C., Durán Medina, E., Amador, A., and Gopar Merino, L. F. (2016). Standardized Hierarchical Vegetation Classification. Cham: Springer International Publishing.
Vleut, I., Levy-Tacher, S. I., De Boer, W. F., Galindo-Gonzalez, J., and Ramirez-Marcial, N. (2013). Can a fast-growing early-successional tree (Ochroma pyramidale, Malvaceae) accelerate forest succession? J. Trop. Ecol. 29, 173–180.
Wagenbrenner, N. S., Forthofer, J. M., Lamb, B. K., Shannon, K. S., and Butler, B. W. (2016). Downscaling surface wind predictions from numerical weather prediction models in complex terrain with WindNinja. Atmos. Chem. Phys. 16, 5229–5241.
Wang, J., Van den Heever, S. C., and Reid, J. S. (2009). A conceptual model for the link between Central American biomass burning aerosols and severe weather over the south-central United States. Environ. Res. Lett. 4:015003.
Wang, L., Beissinger, T. M., Lorant, A., Ross-Ibarra, C., Ross-Ibarra, J., and Hufford, M. B. (2017). The interplay of demography and selection during maize domestication and expansion. Genome Biol. 18:215. doi: 10.1186/s13059-017-1346-4
Watters, R. F. (1971). Shifting Cultivation in Latin America. Forestry Development Paper# 17. Rome: Food and Agriculture Organization (FAO).
Weir, J. T., Bermingham, E., and Schluter, D. (2009). The great American biotic interchange in birds. Proc. Natl. Acad. Sci. U.S.A. 106, 21737–21742. doi: 10.1073/pnas.0903811106
Wendt, T. (1987). Las selvas de Uxpanapa, Veracruz-Oaxaca, México: evidencia de refugios florísticos cenozoicos. Anal. Inst. Biol. Univ. Nacl. Autón. Méx. 58, 29–54.
Whitfield, S. M., Lips, K. R., and Donnelly, M. A. (2016). Amphibian decline and conservation in Central America. Copeia 104, 351–379.
Wiersum, K. F. (1997). From natural forest to tree crops, co-domestication of forests and tree species, an overview. Netherl. J. Agric. Sci. 45, 425–438.
Williams, J. N., Viers, J. H., and Schwartz, M. W. (2010). Tropical dry forest trees and the relationship between local abundance and geographic range. J. Biogeogr. 37, 951–959.
Wilson, J. S., Carril, O. M., and Sipes, S. D. (2014). Revisiting the Great American biotic interchange through analyses of amphitropical bees. Ecography 37, 791–796.
Wilson, R. J., and Gutiérrez, D. (2012). “Effects of climate change on the elevational limits of species range,” in Ecological Consequences of Climate Change. Mechanisms, Conservation and Management, eds E. A. Beever and J. L. Belant (Boca Raton, FL: CRC), 107–131.
Young, K. R., Ewel, J. J., and Brown, B. J. (1987). Seed dynamics during forest succession in Costa Rica. Vegetatio 71, 157–173.
Zacarías-Eslava, Y., and del Castillo, R. F. (2010). Comunidades vegetales templadas de la Sierra Juárez Oaxaca: pisos altitudinales y sus posibles implicaciones ante el cambio climático. Bolet. Soc. Bot. Méx. 87, 13–28.
Zárate, D., Lima, T. G., Poole, J. D., Calfee, E., Burton, R. S., and Kohn, J. R. (2022). Admixture in Africanized honey bees (Apis mellifera) from Panamá to San Diego, CA. Ecol. Evol. 12:e8580. doi: 10.1002/ece3.8580
Zeng, N., Qian, H., Roedenbeck, C., and Heimann, M. (2005). Impact of 1998–2002 midlatitude drought and warming on terrestrial ecosystem and the global carbon cycle. Geophys. Res. Lett. 32:L22709.
Keywords: Central America, climate change, extinction, Mexico, migration, secondary succession, traditional agriculture, bottom-up conservation initiatives
Citation: del Castillo RF and Rivera-García R (2022) Biophysical and Biocultural Upheavals in Mesoamerica, a Conservation Perspective: Mountains, Maize-Milpa, and Globalization. Front. For. Glob. Change 5:763009. doi: 10.3389/ffgc.2022.763009
Received: 23 August 2021; Accepted: 08 June 2022;
Published: 24 June 2022.
Edited by:
H. Mauricio Ortega Andrade, Universidad Regional Amazónica Ikiam, EcuadorReviewed by:
Eduardo J. Chacon, University of Costa Rica, Costa RicaRicardo Rozzi, University of North Texas, United States
Alejandra Tauro, Cape Horn International Center (CHIC-Chile), United States, contributed to the review of RR
Copyright © 2022 del Castillo and Rivera-García. This is an open-access article distributed under the terms of the Creative Commons Attribution License (CC BY). The use, distribution or reproduction in other forums is permitted, provided the original author(s) and the copyright owner(s) are credited and that the original publication in this journal is cited, in accordance with accepted academic practice. No use, distribution or reproduction is permitted which does not comply with these terms.
*Correspondence: Rafael F. del Castillo, ZnNhbmNoZXpAaXBuLm14