- 1Faculty of Forestry and Environmental Management, University of New Brunswick, Fredericton, NB, Canada
- 2Natural Resources Canada, Canadian Forest Service - Atlantic Forestry Centre, Fredericton, NB, Canada
Increasing frequencies of severe heat waves and drought are expected to influence the composition and functioning of ecosystems globally. Our ability to predict and mitigate these impacts depends on our understanding of species- and age-specific responses to these stressors. To assess the adaptive capacity of balsam fir to climate change, a cold-adapted boreal tree species, we conducted a climate-controlled greenhouse experiment with four provenances originating from across the species biogeographic range, 12 temperature treatments ensuring a minimum of +11°C warming, and five drought treatment intensities. We found considerable acclimation to temperature and drought treatments across all provenances, with steady gains in biomass under temperatures well-beyond the “worst-case” (RCP 8.5) climate forcing scenario within the species natural range. Acclimation was supported by high phenotypic plasticity in root:shoot ratio (RSR) and photosynthesis, which were greatly increased with warming, but were not affected by drought. Our results suggest that regardless of the observed provenance variation, drought and heat are not limiting factors of the current-year balsam fir seedling growth, instead, these factors may be more impactful on later stages of regeneration or previously stressed individuals, thus highlighting the necessity of incorporating the factors of ontogeny and provenance origin in future research regarding plant and climate interactions.
1 Introduction
Climate change is increasing the intensity and variability of temperature and precipitation regimes throughout Canada (Zhang et al., 2019). As a result, forest model projections predict significant decreases in the abundance of cold-adapted boreal species such as balsam fir [Abies balsamea (L.) Mill] in the majority of their southern range from increasingly unfavorable growing conditions due to rising heat and drought (Boulanger et al., 2017; Taylor et al., 2017), while in the north, these species are expected to have generally large growth increases (D’Orangeville et al., 2018a; Wang et al., in press). The large potential ecological and economic impacts of such changes within Canada’s forests (Rodenhouse et al., 2008; Ochuodho et al., 2012; Rustad et al., 2012) require a better understanding of the adaptative capacity of the region’s most abundant tree species. Current observational studies of species responses to climate change have been asynchronous with some model predictions (Zhu et al., 2012; Fei et al., 2017), an issue that highlights the current lack of empirical data regarding age-specific and both inter- and intra-species responses to climate change across environmental gradients (Hijmans and Graham, 2006; Garzón et al., 2011; Aubin et al., 2016; Urban et al., 2016).
Low radial growth of balsam fir is correlated with low humidity (Duchesne and Houle, 2011), extreme low or high temperatures, and low soil moisture (Goldblum and Rigg, 2005; D’Orangeville et al., 2018a). However, seedlings differ from mature trees in their response to climate (Fisichelli et al., 2012, 2014; Gray and Brady, 2016) due to their small size and position in the understory (McDowell et al., 2008; Rollinson et al., 2021), and are at a higher risk for hydraulic failure and mortality from extreme climate events due to limited root volume and photosynthetic area (McDowell et al., 2008). Young balsam fir are particularly reliant on consistent soil moisture levels, likely partially due to the species’ proclivity for shallow rooting (Burns and Honkala, 1990; Taylor et al., 2020). When exposed to short-term moisture depletions, balsam fir seedlings show tolerance and plasticity in xylem growth and apical growth (Rossi et al., 2009), while long-term droughts decrease metabolic functions that limit growth in the current and following years (D’Orangeville et al., 2013; Vaughn et al., 2021).
While mean summer precipitation rates are projected to increase throughout the majority of balsam fir’s range (Zhang et al., 2019), higher frequencies of droughts and heatwaves will increase the prevalence of periods of low soil moisture, resulting in moisture stress and growth declines (Goldblum and Rigg, 2005; Way and Sage, 2008; Rossi et al., 2009; McDowell et al., 2011). Increases in atmospheric vapor pressure deficit (VPD) with warming and drying (Yuan et al., 2019) are expected to modify tree stomatal conductance and evapotranspiration rates (Novick et al., 2016; Grossiord et al., 2020). Limited soil moisture and a high transpirative demand due to warming can provoke stomatal closure in trees as a defense mechanism to mitigate water loss, therefore preventing cavitation by maintaining hydraulic pressure (Domec et al., 2009; Choat et al., 2018). In doing so, trees limit their rates of photosynthesis (Grossiord et al., 2020), a factor also directly inhibited by high temperature (McMurtrie and Wang, 1993; Lin et al., 2012). In turn, this reduces their capacity to produce valuable carbohydrate stores that may be used to mitigate cell death, cavitation, and hydraulic failure (Hacke et al., 2001; McDowell et al., 2011; Klein et al., 2018; Sapes et al., 2019). Long-term, persistent droughts eventually break the hydraulic tension in trees regardless of drought-resistance mechanisms, causing cavitation, which reduces the ability to transport water and solutes, leading to mortality (Allen et al., 2010; Choat et al., 2018).
All trees have a capacity to adapt to climate change, but the likelihood of a species to persist by both resisting and recovering from these climatic stressors is largely determined by its level and type of phenotypic plasticity and genetic diversity (Aubin et al., 2016). For instance, with limited moisture, some species lengthen their roots to access deeper moisture reserves, thereby increasing their root:shoot ratio (RSR), (Janiak et al., 2016). Trees can also adapt by increasing their photosynthesis to transpiration ratio, or water use efficiency (WUE), to reduce risk of carbon starvation and water loss (Osakabe et al., 2014), and refill embolized xylem to improve water conductance post-drought (Klein et al., 2018). Genetic adaptations resulting from local forcing events create adaptative differences between populations of species (Ghalambor et al., 2007), ensuring higher chances of survival under stress in certain populations (Moran et al., 2017). Research into the genetic variance amongst balsam fir populations, or “provenances,” has demonstrated high phenotypic variability within mature and sapling stage trees (Fryer and Ledig, 1972; Lowe et al., 1977; Carter, 1996; Akalusi and Bourque, 2021). In mature balsam fir, WUE was significantly different amongst provenances, with positive correlations between WUE and diameter at breast height (cm) (Akalusi and Bourque, 2021). A balsam fir provenance trial along an altitudinal gradient demonstrated sizeable variance of phenotypic plasticity among provenances through the adjustment of photosynthetic temperature optimums to better suit their origin climate, with a 2.4°C decrease in optimum temperature per 305 m increase in altitude (Fryer and Ledig, 1972). Incorporating these provenance-specific adaptations to climate into stand distribution models has been shown to greatly reduce the projected declines of climate change on a species’ abundance (Garzón et al., 2011). Therefore, empirically evaluating the effects of balsam fir seedling genetic variance and phenotypic plasticity on performance and survival under a range of environmental conditions is warranted to better understand the role of climate change in the boreal and temperate forests of North America.
We conducted a controlled seedling provenance trial within 12 climate-controlled greenhouse phytotrons with the objective of (i) determining the response of balsam fir growth and leaf-level gas exchanges to wide gradients of heat and drought and (ii) assessing the variation of these responses between provenances. To achieve these objectives, we evaluated the rate of physiological change of each provenance when exposed to five levels of drought intensity, and a 12-level temperature gradient, ranging from an average temperature of 13.9–30.9°C. To determine how seedling growth varied among treatments, we measured seedling biomass, RSRs, and CO2 assimilation rates. We hypothesized that (i) rates of balsam fir seedling growth and photosynthesis will increase in response to moderate temperature increases but significant declines are expected under extreme warming conditions (i.e., a nonlinear response); (ii) balsam fir provenances from southern locations will experience less significant growth declines at higher temperatures when compared to northern provenances; and (iii) drought will limit the positive effects of moderate temperature increases, exacerbate heat-related growth declines, and increase seedling mortality.
2 Material and methods
2.1 Experimental design
In the spring of 2021, we established a controlled greenhouse experiment located at the Atlantic Forestry Centre (AFC) Greenhouse operated by Natural Resources Canada—Canadian Forest Service (CFS) (Fredericton, NB, Canada). Our experiment utilized the split-split plot design, with four balsam fir provenances, nested within five levels of drought intensity, nested within 12 temperature treatments (Altman and Krzywinski, 2015). Each of these 240 treatments were applied to fifteen 2-year-old seedlings, for a total of 3,600 seedlings. Instead of replicating a low number of categorical treatment levels in an ANOVA-type experimental design, we applied a larger, evenly distributed number of temperature levels to support a regression type analysis, permitting us to model non-linear response patterns (Schweiger et al., 2016; Kreyling et al., 2018). The temperature treatment phase of the experiment ran from May 1st to September 16th, with all treatment groups irrigated to field capacity once soil volumetric water content dropped below 20%. The drought trial component commenced on August 1st and finished on September 16th.
2.2 Study apparatus
For this experiment, we built 12 climate-controlled phytotrons using pressure-treated lumber for the frames, and clear greenhouse plastic as a coating. The core design and construction process of the phytotrons is detailed in Vaughn et al. (2021). The phytotrons were located inside the main AFC greenhouse and are arranged in two rows of six. The climate of each phytotron was regulated by a temperature controller (model ITC-310 T-B, Inkbird, Shenzhen, China) that cycles the attached heater and air conditioner to obtain the pre-set temperature level. The controllers were programmed to have 12-temperature levels that were timed to mimic diurnal temperature cycles. Soil moisture sensors (EC-5 Volumetric Water Content sensor, METER Group, Pullman, WA, USA) were installed within each unit to ensure a homogeneity in soil moisture levels within treatments. Two LED growing lights (HLG 100 V2, Horticulture Lighting Group, Knoxville, TN, USA) were installed within the interior of each phytotron to provide intra-chamber homogeneity of light quantity and distribution. The main AFC were painted with a darkening agent and the roof was covered with a 50% light reducing cloth to further reduce lighting variation among the phytotrons.
2.3 Seedling preparation
The seeds were cleaned, imbibed for two days, cold stratified at 5°C for 3 weeks, and were then direct sown into 45-cell trays that were filled with a 2:1 peat/vermiculite mixture. Due to the sub-optimal germination success rates advertised by the National Tree Seed Centre (NTSC), we filled each tray cell with five seeds with the intent of germinating a minimum of one seedling per cell. Therefore, we opted to subject the seedlings to the experimental treatments in their second year to ensure that each treatment group had 15, well-established, healthy seedlings rather than utilizing trays with empty or overcrowded cells and poorly situated seedlings. For the first year, seedlings grew under ambient temperatures for 50 days, fertilized with a water-based fertilizer at 100 parts per million (ppm) of 20:8:20 NPK (nitrogen, phosphorus, and potassium). To promote winter cold-hardiness, seedlings were then exposed to cooler temperatures and fertilized with a 35 ppm 8:20:30 mixture for 49 days. At the end of the first growing season, mean seedling height was 3.5 cm (SD = 0.7). Seedlings were then placed in cooling chambers until their chilling requirements of 1,000 h were met. Once dormancy requirements were met, seedlings were transplanted alongside their rooting medium to larger, 15-cell trays (approximately 440 cm3 of cell rooting volume) to ensure adequate rooting area and to minimize competition for light. Seedlings were then immediately placed into the phytotrons, thus commencing the experimental portion of the project.
2.4 Experimental factors
2.4.1 Provenance
Considering that heterogeneous growing conditions between populations can increase genetic variance, we selected among available seedlots to maximize the temperature gradient available while controlling for variations in moisture regime and altitude in an effort to understand how the influence of climate heterogeneity affects the variance of phenotypic plasticity among provenances of balsam fir. For each provenance, seeds were collected by the NTSC1 during high-masting years to ensure optimal gene crossing and were collected from stands that represented regional environmental variation. To obtain the necessary provenance-specific climate data, the location of each provenance was intersected with North American interpolated climate grids provided by CFS (McKenney et al., 2011). The 30-years (1981–2010) of extracted climate data provided maximum monthly temperature (TMAX), minimum monthly temperature (TMIN), and the monthly climate moisture index (CMI). With this data, mean summer TMAX and mean winter TMIN was calculated for the 126 balsam fir provenances available from the NTSC, and the provenances were then ordered from coldest climate to hottest climate. Due to the constraints imposed by the area of the seedling trays and phytotrons, we determined that utilizing four provenances in our experiment was an optimal trade-off between replicate count and representativeness of provenance variation. We first selected our hottest and coldest provenances, which had a summer TMAX difference of 5°C and a winter TMIN difference of 14°C. The two intermediate provenances were then selected based on their evenly spaced distance in climate rank from the hottest and coldest provenance, and their minimal variation of CMI and elevation (m) (Figure 1). The temperature intervals between each of the four selected provenances were 4.8°C (winter TMIN) and 1.6°C (summer TMAX). Finally, we ensured that there was minimal variation in soil types between provenances; all provenances were collected from sites with well-drained, rocky, acidic soil.
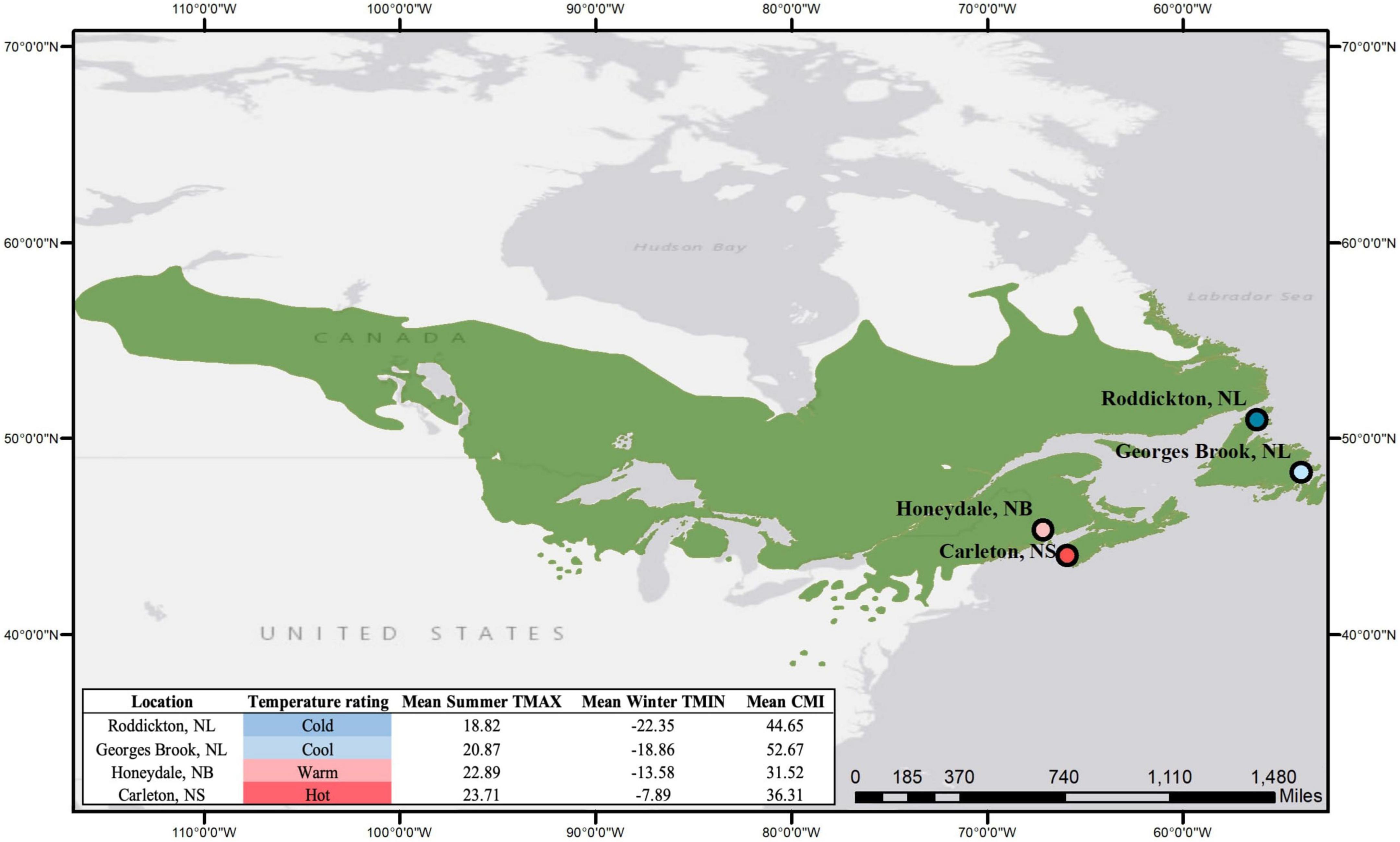
Figure 1. Balsam fir provenance locations overlayed with the geographic extent of the species (Little, 1971), accompanied with their corresponding climate values.
2.4.2 Temperature
To determine the extent of variation in provenance response to temperature increases, seedlings were exposed to twelve temperature treatment levels that covered a large temperature range. To emulate the natural variation of temperature throughout the growing season, we first averaged provenance-specific climate normals (Government of Canada, 2011) to create a baseline weekly temperature treatment schedule throughout the experiment growing season. We then adjusted the average temperature of this baseline schedule to cover a temperature range that was designed to (1) simulate the projected summer temperature increase under the “worst-case” Representative Concentration Pathway 8.5 forcing scenario (RCP; McKenney et al., 2011; van Vuuren et al., 2011) for each provenance, (2) simulate the mean summer temperature variation across the entire geographic range of balsam fir, and (3) expose the seedlings to warm enough temperatures that it may elicit a significant negative growth response in each provenance.
For eastern Canada, the projected mean annual temperature increases (compared to temperatures in 1986–2005) under RCP 8.5 is approximately 5.9°C (Zhang et al., 2019). The mean summer temperature difference between the southern and northern boundaries of balsam fir is 8.5°C (McKenney et al., 2011). Therefore, the treatment levels were created to ensure that each provenance was exposed to +8.5°C above their current origin climate, with an additional +2.5°C to account for our third objective of exposing them to extreme conditions. Based on this, we determined that temperature intervals of 1.81°C between the 12 temperature levels would ensure each provenance experienced a temperature increase of 11°C above its origin baseline summer climate. Our coldest treatment level corresponded to a scenario representing 3°C below the historical average growing season temperature at the coldest provenance location, while the warmest treatment corresponded to an 11°C increase above the summer climate of the warmest provenance (Table 1). For each phytotron, the 12 daily temperature levels were set to change every 2 h, with the coldest level occurring at 2 a.m.–4 a.m., and the hottest at 2 p.m.–4 p.m.
2.4.3 Drought
To evaluate the extent of variation in provenance response to different drought intensities, seedlings were exposed to five drought treatment levels. Drought treatments had evenly spaced intervals of soil water potential (SWP) (mPa) thresholds, with the driest group only watered once SWP reached a minimum of −2.5 mPa, a level associated with low plant water content, and near complete photosynthetic and transpirative shutdown within a range of conifer seedlings (Havranek and Benecke, 1978). We determined the tray SWP levels by determining the relationship between soil volumetric water content (VWC) and SWP. This was necessary because SWP measurements show the effort required by the plant roots to extract water from the soil while VWC measurements only show the water content within the soil (Papendick and Campbell, 1981), therefore making SWP a better indicator of drought severity for plants (see Supplementary material).
For all provenances in each phytotron, each of the five trays were designated a different SWP threshold. The four hottest treatments reached their SWP thresholds in 20 days, while the four intermediate and four cold treatments reached their thresholds in 25 and 32 days, respectively. Once the group thresholds were met, seedlings were watered to field capacity and were watered regularly until the end of the growing season.
2.5 Response variables
2.5.1 Photosynthetic response to temperature
The acclimation of photosynthesis to growing condition temperatures allows a plant to mitigate temperature-related growth inhibition, therefore enhancing plant resilience to climate variability (Berry and Björkman, 1980). Therefore, determining the extent of variation in photosynthetic acclimation to growing conditions within balsam fir seedlings will provide better insight of how balsam fir is able to adapt to climate change.
For the northernmost and southernmost provenance, we subjected two seedlings each from the coldest, intermediate, and hottest temperature treatments to temperatures ranging from 16 to 36°C and measured their rates of photosynthesis at each temperature level. Since adjusting the internal LiCOR temperatures is a time-intensive endeavor, this temperature range was considered an optimal trade-off between the number of seedlings measured and the extent of temperature range. Measurements were conducted with the LiCOR-6800 portable photosynthesis system, fit with a 6400-22 conifer chamber (Li-Cor, Lincoln, Nebraska, NE, USA). To ensure that internal LiCOR light levels were not limiting the measurements, we conducted two light response tests for both provenances. Based on these results, light levels within the LiCOR were set at a non-limiting level of 1,000 μmol m–2 s–1. Relative humidity levels were set to match the average noontime relative humidity level of the phytotrons. Measurements were logged once humidity, CO2, and temperature became stable within the chamber. Immediately after the measurement period, seedlings were harvested and frozen to prevent desiccation and damage, allowing for accurate leaf area (LA) measurements that accounted for the curvature of the needles.
Because the leaf area varies between seedlings, and area measurements cannot be conducted on a live seedling, a substitute area value was used while measurements were taken. Therefore, all seedlings that were measured with the LiCOR had their LAs measured to calculate leaf-gas exchange measurements that considered seedling-specific LA. We cut and scanned the cross-sections of three needles of each seedling, (SigmaScan Pro, Systat Software Inc., Chicago, IL, USA) and then measured the projected 2D needle surface area (A), cross-section circumference (c) and width (w) (WinSEEDLE, Régent Instruments, Quebec City, QC, Canada). Needles were then oven-dried at 70°C for a minimum of 48 h, and then weighed to determine oven-dried weight (ODW) and the specific ODW of the three measured needles.
The LA for each seedling was calculated by first determining the specific leaf area, which is the ratio of leaf surface area to dry mass, and then multiplying by the total ODW of the seedling needles. This process is represented with the following equation:
Where c is the cross-section circumference, w is the needle width, A is the 2D needle surface area, ML is the specific ODW of the three measured needles, and ODW is the total oven-dried weight of the needles.
2.5.2 Seedling needle damage and biomass measurements
For needle damage measurements, we performed a count of seedlings that had needle damage. Seedlings were visually analyzed to determine the percent of total LA that had needle damage. We isolated the seedlings damaged only by the drought by measuring damage levels before and after the drought. Based on these measurements, each seedling was assigned a damage intensity class ranging from 0 to 3, with 0 indicating no damage, 1 being 1–35%, 2 being 36–70%, and 3 representing 71–100% damage.
Immediately following the drought trial, two seedlings from each of the 240 treatment groups were harvested, and separated into needles, stems, and roots split. The 1,440 samples were dried in an oven at 65°C for a minimum of 48 h and were then measured for dry biomass (g). We also used these biomass measurements to calculate seedling RSR, which was calculated by dividing seedling belowground biomass by seedling aboveground biomass.
2.6 Statistical analyses
For three of our four response variables, we used linear-mixed effect models to understand the influence of temperature, provenance, and drought on numerous aspects of balsam fir seedling performance. The four model response variables were characterized as: biomass, RSR, needle damage, and seedling assimilation rate of CO2. The general fixed explanatory variables for all models were provenance location, average treatment temperature, and level of drought intensity. We also included a fixed variable for LiCOR temperature within the photosynthesis model. We scaled our two temperature variables to allow for easier model interpretation. To account for potential heterogeneity between treatment blocks, we assigned a random intercept for the phytotrons within each model.
For each response variable, we started with a full model including all measured variables and relevant interactions between the main variables. Save for the model-specific hypothesized interactions, we removed all non-significant (p > 0.05) predictors as well as non-significant two-way and three-way interactions within each model iteration to avoid overfitting and to simplify model interpretation. Our hypothesized interactions for the RSR and biomass models are temperature and drought, and temperature and provenance. In addition to the two prior interactions our photosynthesis model, we hypothesized that the internal LiCOR temperature would have a significant interaction with provenance. Finally, we included a single temperature and provenance interaction within our needle damage model. Our general model for biomass and RSR takes the following form:
Where T is the average temperature treatment, fit with an orthogonal polynomial term, P is the provenance, D is the drought intensity, β is the slope of the fixed effects, ∈ is the intercept of the phytotron j random effects. We only included H, a term for seedling height, for the RSR model because tree size is an important RSR moderator, where larger trees tend to have lower RSR levels (Ledo et al., 2018).
For our photosynthesis model, the general equation took the form of:
Where T is the internal LiCOR temperature at the time of measurement, fit with an orthogonal polynomial term, t is a three-factor phytotron temperature intensity variable. Unlike the other models, we considered phytotron temperature (t), as a factor as we only sampled from three distinct temperature treatments. We included three two-way interactions, which A term for drought is not included because these measurements were conducted prior to the drought.
Finally, to understand how the three general explanatory variables influenced the occurrences of seedling needle damage intensity, we used a Poisson linear mixed effects model. We used this type of analysis because our count data followed a Poisson distribution. This model took the following form:
Where λj represents the frequency of damaged seedling observations.
For these analyses, we used the R package “lme4” (Bates et al., 2015; R Development Core Team, 2022). We conducted a Breusch–Pagan test to assess levels of heteroscedasticity, calculated generalized variance inflation factors to test for multicollinearity, visually analyzed Q-Q plots for linearity. For post hoc comparisons, we conducted pairwise comparisons with the least-square means method using the “emmeans” package to determine the degree of difference in response between treatment groups (Lenth, 2021).
3 Results
3.1 Effects of drought and heat on seedling growth and damage
We observed a non-linear growth response to temperature amongst all provenances, with average seedling biomass levels increasing to an optimum average temperature level threshold of approximately 23°C (Figure 2A). These results support the hypothesis that seedling biomass is limited by current temperatures and responds positively to moderate warming. However, we report a positive response to temperature levels warmer than RCP 8.5 projections, suggesting a high tolerance to warming (Figure 2A). Pairwise comparisons revealed significant differences (p < 0.001) of total biomass (Figure 2A) and RSR (Figure 2B), however, the only significant interactions identified were the difference in RSR response to temperature between the north and south provenance (Table 2). Drought, applied late in the growing season to mimic July-August drought, had no impact on either aspect of growth (Table 2).
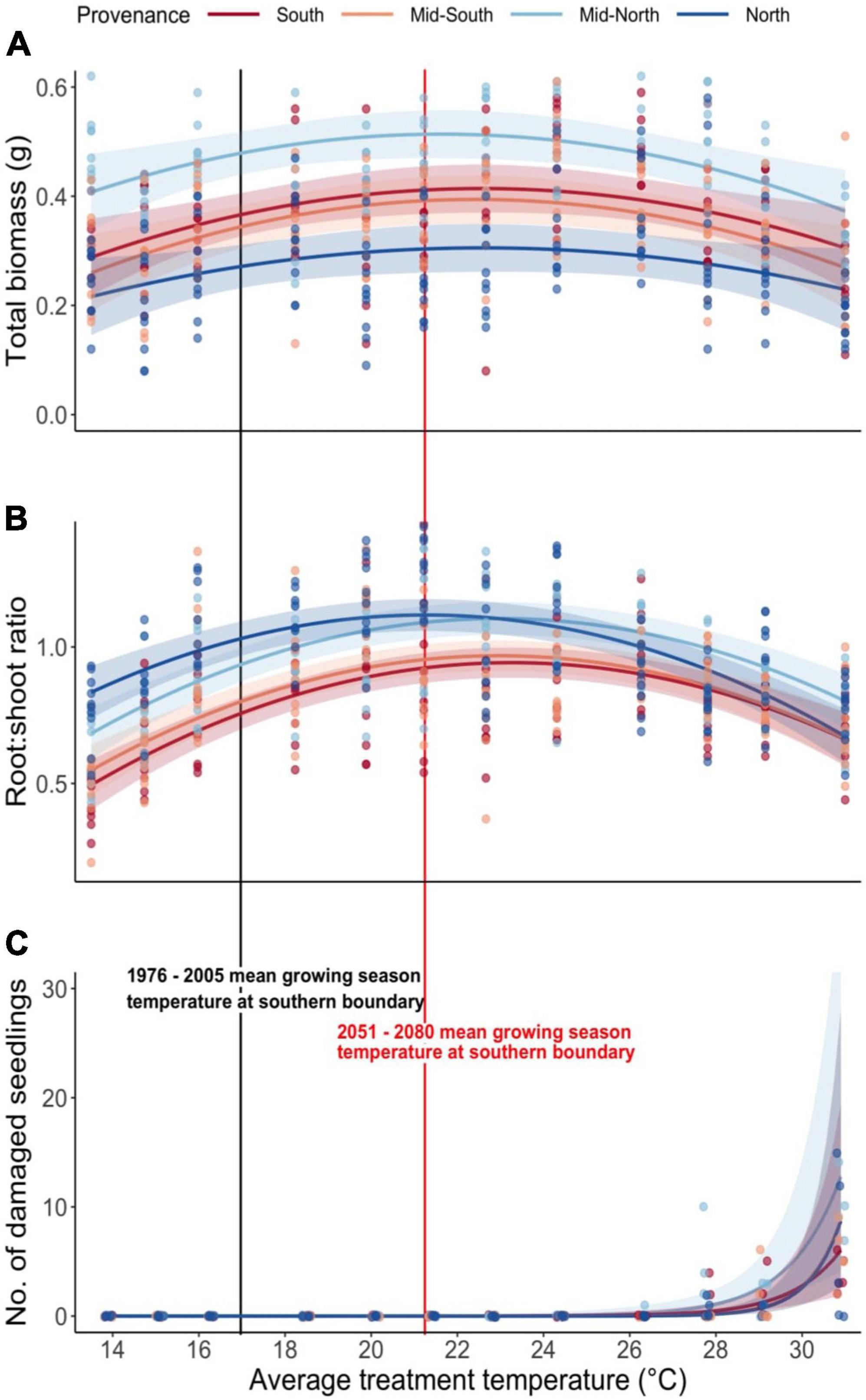
Figure 2. Interaction plots (Long, 2019) depicting the relationship between average treatment temperature and (A) the total seedling biomass (g), (B) seedling root:shoot ratio (RSR), and (C) the number of seedlings with severe needle damage. Colored bands represent 95% confidence intervals. The black vertical line shows the mean average daily temperature (17°C; May—September) within the hottest part of balsam firs range in the recent past. The red vertical line shows the RCP 8.5 projected average daily temperature (21.3°C; May—September) within the same region by 2051–2080 (Pacific Climate Impacts Consortium, 2014).
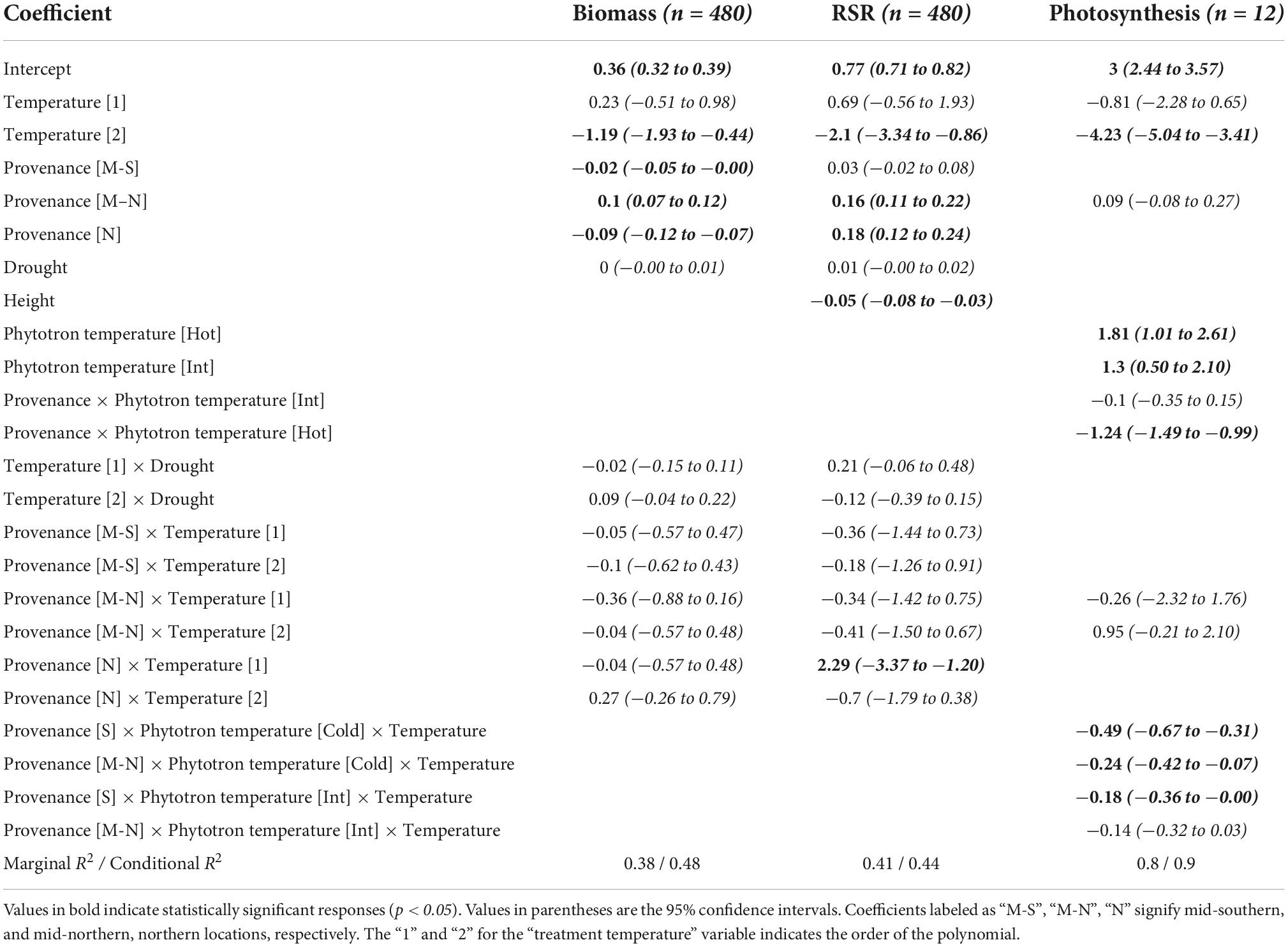
Table 2. General linear mixed-effects model coefficients for the biomass, root:shoot ratio (RSR), and photosynthesis models.
Severe needle damage was first observed at an average temperature of 26°C, with further temperature increases drastically raising incidence rates (Figure 2C and Table 2). The effect of temperature varied between provenances; the mid-north provenance exhibited a significantly higher sensitivity to temperature than the north and mid-south provenances (p < 0.05; Figure 2). Similar to biomass and RSR, drought had no impact on the severity of needle damage (p = 0.1).
3.2 Acclimation of photosynthesis to growing conditions
The south and north provenances both exhibit a considerable ability to acclimate their photosynthesis to their growing environment (Figure 3 and Table 2), each displaying a large, significant non-linear response curve to gradual changes in measurement temperature. For the south provenance only, we identified a consistent acclimation of seedling photosynthetic optimum temperatures; optimal photosynthesis shifted at a rate of 1°C for every 3.6°C shift in average treatment temperature (Figure 3). When we compare the effect of chronic differences in temperature, here represented by the comparison of trees grown under cold, intermediate, and hot temperature treatments, on the photosynthetic response curve, we detect multiple, significant interactions between provenances and treatment temperature indicating important effects of provenances and treatment temperature on the photosynthetic capacity of balsam fir. Specifically, when grown under hot conditions, we report a significant 29% higher photosynthetic rate when comparing the south provenance to the north provenance (p < 0.001; Figure 3), although the higher variation within the north-hot group may account for some of this difference.
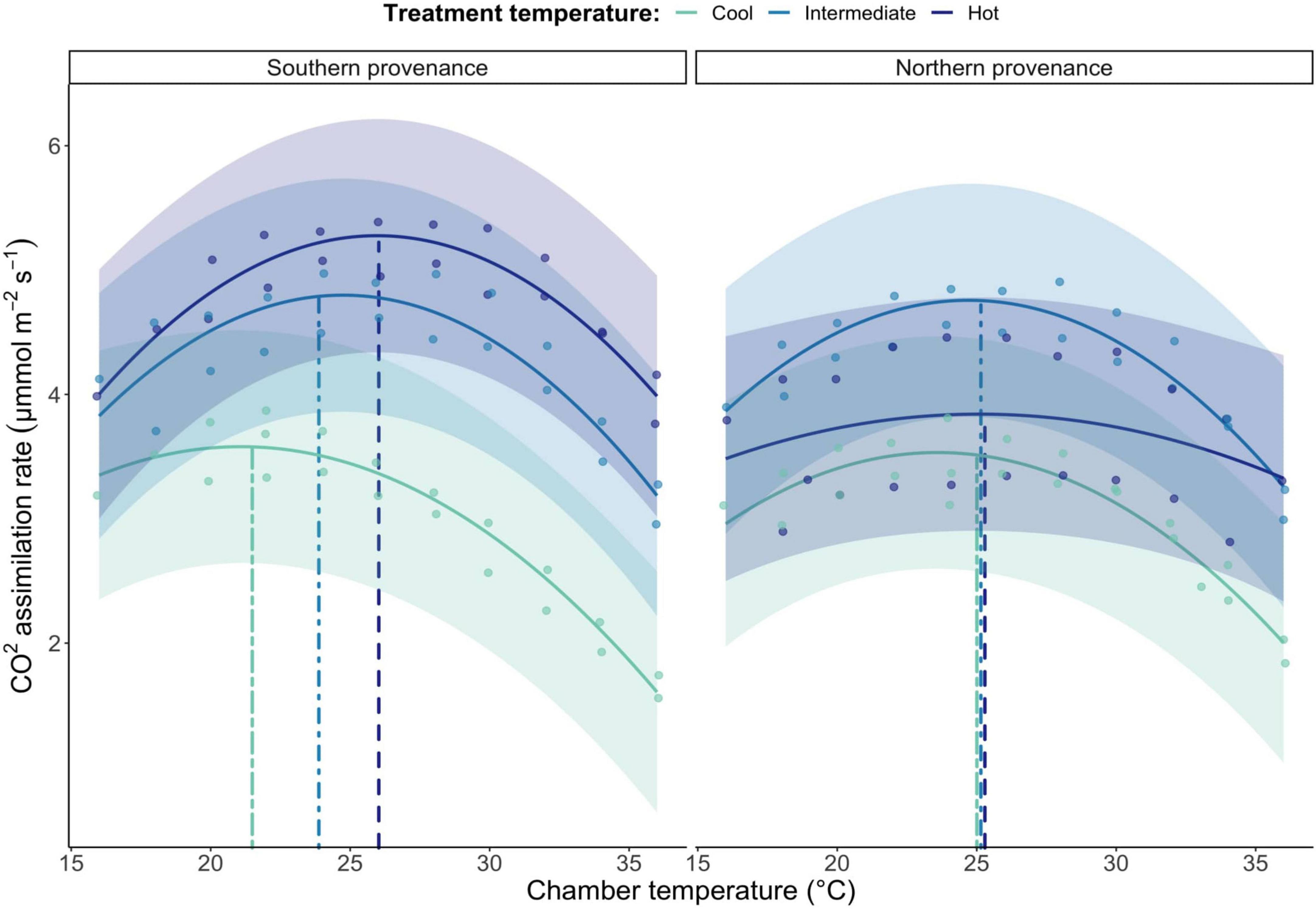
Figure 3. Seedling temperature response curves separated by provenance location and average temperature treatment level. The colored bands represent 95% confidence intervals. Vertical lines represent photosynthetic optimum temperatures for each treatment group. Grouped vertical lines indicate a shared photosynthetic optimum temperature associated with the rightmost line.
Finally, we observed differences in heat-tolerance between treatment groups for the southern provenance only. Specifically, photosynthesis rates in the cold treatment groups dropped faster when exposed to warmer measurement temperature, when compared to the hot (p < 0.001) and intermediate (p < 0.05) treatment groups (Figure 3 and Table 2).
4 Discussion
Our experiment revealed considerable phenotypic plasticity in photosynthesis and RSR among provenances, which seemed to moderate balsam fir seedling stress under the warming and drought treatments. Although we cannot exclude that provenances from drier, western parts of the species range may behave differently than the provenances studied here, the results suggest that regardless of adaptations to local climate regimes, the physiology of the balsam fir seedlings studied here is capable of adapting to a warming environment resulting from climate change, as evidenced by the uniform growth declines among all provenances observed only in temperature conditions well beyond RCP 8.5 forcing scenarios within the warmest regions of the species biogeographic range.
Optimizing resource acquisition in limiting environments is essential for survival in seedlings due to their limited size. The observed RSR increases alongside temperature indicate an important potential ability of balsam fir seedlings to allocate resources to below-ground structures to regulate greater evapotranspiration, a strategy regularly employed by plants in moisture-limiting conditions (Lopez-Iglesias et al., 2014; Aubin et al., 2016; Janiak et al., 2016; Moran et al., 2017; Ledo et al., 2018). The plastic response of seedling photosynthetic levels to increasing temperature may enable seedlings a greater capacity to mitigate heat stress in needles (Haider et al., 2021), maintain hydraulic transport integrity through the shifting of xylem anatomy (D’Orangeville et al., 2013) or through the refilling of embolized cells (Klein et al., 2018), thereby sustaining crucial carbohydrate distribution throughout the system (McDowell, 2011; Kono et al., 2019). Conversely, high rates of photosynthesis may exacerbate plant moisture loss through stomatal conductance, a risky but potentially beneficial strategy in hotter climates (McDowell et al., 2008; Sade et al., 2012; Skelton et al., 2015). In hot growing conditions, this risky behavior may have been amplified by a complete lack of water-use efficiency (WUE) acclimation to any intensity of drought (see Supplementary material). Although the drought treatment had no impact on seedling performance, the late application may impact growth in the following year as the majority of the current-year growth had already completed (Goldblum and Rigg, 2005; D’Orangeville et al., 2018b; Kannenberg et al., 2019). Regardless, the observed positive effects from moderate temperature increases supports our first hypothesis, while the lack of effect from the drought treatment fails to support our third hypothesis.
The emergence of genetic variation can result from the selective pressures of heterogeneity in growing conditions within a species geographic range, and from geographic isolation (Via and Lande, 1985; Lande, 2009). Advantageous phenotypic plasticity resulting from this genetic variation can potentially improve the persistence of a population in uncertain conditions (Donohue et al., 2000; Chevin and Lande, 2010; Nicotra et al., 2010), potentially promoting further population divergence (Via and Lande, 1985; Kelly, 2019). Our results indicate a low to moderate level of variation in phenotypic plasticity between provenances, here represented as RSR and photosynthesis. As hypothesized, the southern provenances exhibited greater performance in hot conditions when compared to the northern provenances; however, these divergences had limited impacts on growth and needle damage rates overall. This low variation between provenances may relate to the theorized ecological cost of adaptive phenotypic plasticity, where adaptations to a specific environment could limit a species plastic response to further change or cause unintended consequences within plant responses to other stimuli (DeWitt et al., 1998; Van Kleunen and Fischer, 2005; Ghalambor et al., 2015). Alternatively, considering that the magnitude and type of plasticity can change alongside tree development (Bouvet et al., 2005), advantageous phenotypic plasticity may have a more noticeable influence on provenance growth variation within older trees that may be more prone to moisture stress due to a greater exposure to climatic extremes (Tyree and Ewers, 1991; Domec et al., 2008; McDowell and Allen, 2015; McGregor et al., 2021; Rollinson et al., 2021).
Model projections of balsam fir’s future range distribution (Iverson et al., 2008; Hassan and Bourque, 2009), abundance and productivity (Boulanger et al., 2017; Taylor et al., 2017) under varying climate change scenarios fail to consider age-specific provenance growth responses to climate, which contributes to uncertainty in model projections. Considering responses to climate found in previous studies (Carter, 1996; Akalusi and Bourque, 2021) and our own findings, it is important to further highlight the two factors in modeling approaches.
Although our experiment was conducted in a controlled greenhouse setting, stressful factors found only in natural growing conditions such as competition (Rollinson et al., 2016), pests, and pathogens (Allen et al., 2010; McDowell et al., 2011) may limit the observed positive growth response to temperature. Nonetheless, our research indicates that the southward extent of balsam fir is not directly limited by temperature, as seedling growth increases well beyond the current average temperature of the species’ southern range boundary. Recent research has also reported similar temperature-related growth benefits in young balsam fir in natural conditions (Collier et al., 2022), and no effect of winter warming on germination success (Vaughn and Taylor, 2022). Interestingly, the southward range of balsam fir saplings was observed expanding with climate warming, while mature fir migrated poleward (Boisvert-Marsh et al., 2014), suggesting that balsam fir may be more sensitive to climate stress in later stages of life. Though plagued with a risky hydraulic framework (Sperry and Tyree, 1990), shorter balsam fir have less distance to transport water therefore avoiding the risk of embolism and moisture stress that larger, mature trees face due to greater xylem tension, a higher exposure to drought conditions, and a greater moisture requirement (Sperry and Tyree, 1990; Tyree and Ewers, 1991; Domec et al., 2008; McDowell and Allen, 2015; Aubin et al., 2018; McGregor et al., 2021; Rollinson et al., 2021). The moisture dependence of balsam fir has been noted in previous studies (D’Orangeville et al., 2013, 2018a; Collier et al., 2022), and may be explained by the growth efficiency trade-off between tracheid embolism resistance and hydraulic conductivity; in non-moisture limiting conditions, a low conductivity may reduce competitiveness (Sperry and Tyree, 1990; Tyree and Ewers, 1991; Domec et al., 2008). Therefore, the generally hydric conditions throughout the range of balsam fir promote a high-risk hydraulic framework, fast growth, and a subsequent moisture dependency, thereby potentially reducing climate resilience and competitiveness in conditions with high hydrological variability.
5 Conclusion
All balsam fir provenances exhibit a consistent, striking ability to acclimate their physiological traits via phenotypic plasticity, enabling high growth in considerable heat. This suggests that temperature and drought may not be the limiting factors that moderate the establishment and growth of balsam fir seedlings at range boundaries, instead, climatic stress may be exacerbated with age and cumulative abiotic and biotic stressors, thereby influencing the reproductive success and competitive vigor of older individuals. Although the implications of our research are limited by the controlled nature of our experimental design and can only be generalized to the eastern, wetter part of the species range where the species is most dominant, these findings support the integration of age- and provenance-specific growth responses into future modeling attempts aiming to evaluate species distributions and abundance under climate change scenarios. To do so, more empirical data relating to these interactions needs to be collected in both controlled and natural environments. Furthermore, while our research aimed to evaluate how range wide temperature heterogeneity influenced the variance of genetics and phenotypic plasticity between provenances, future research should incorporate greater provenance diversity to increase our holistic understanding of balsam fir climate resilience. Future research should also be conducted to decipher how competition, legacy effects, provenance origin, and biotic stressors influence the success of balsam fir under differing climatic conditions, and at different life stages.
Data availability statement
The raw data supporting the conclusions of this article will be made available by the authors, without undue reservation.
Author contributions
JR performed the experiments, analyzed the data, and prepared the manuscript. All authors conceived the study design and contributed to the final version of the manuscript.
Funding
Project funding was provided through an NSERC Discovery Grant (RGPIN-2019-04353), the New Brunswick Innovation Foundation (RIF 2019-029), and the Canadian Forest Service.
Acknowledgments
We greatly appreciate the support and resources provided by the Atlantic Forestry Centre throughout the project. We thank the National Tree Seed Centre for their generous contribution of the tree seed used in this project. Thanks to Rob Vaughn, John Letourneau, Gretta Goodine, Peter Tucker, and Rachel Aske for their guidance and help throughout the project.
Conflict of interest
The authors declare that the research was conducted in the absence of any commercial or financial relationships that could be construed as a potential conflict of interest.
Publisher’s note
All claims expressed in this article are solely those of the authors and do not necessarily represent those of their affiliated organizations, or those of the publisher, the editors and the reviewers. Any product that may be evaluated in this article, or claim that may be made by its manufacturer, is not guaranteed or endorsed by the publisher.
Supplementary material
The Supplementary Material for this article can be found online at: https://www.frontiersin.org/articles/10.3389/ffgc.2022.1075787/full#supplementary-material
Footnotes
References
Akalusi, M. E., and Bourque, C. P.-A. (2021). Physiological and morphological variation in balsam fir provenances growing in New Brunswick Canada. Forests 12:186. doi: 10.3390/f12020186
Allen, C. D., Macalady, A. K., Chenchouni, H., Bachelet, D., McDowell, N., Vennetier, M., et al. (2010). A global overview of drought and heat-induced tree mortality reveals emerging climate change risks for forests. For. Ecol. Manag. 259, 660–684. doi: 10.1016/j.foreco.2009.09.001
Altman, N., and Krzywinski, M. (2015). Split plot design. Nat. Methods 12, 165–166. doi: 10.1038/nmeth.3293
Aubin, I., Boisvert-Marsh, L., Kebli, H., McKenney, D., Pedlar, J., Lawrence, K., et al. (2018). Tree vulnerability to climate change: Improving exposure-based assessments using traits as indicators of sensitivity. Ecosphere 9:e02108. doi: 10.1002/ecs2.2108
Aubin, I., Munson, A. D., Cardou, F., Burton, P. J., Isabel, N., Pedlar, J. H., et al. (2016). Traits to stay, traits to move: A review of functional traits to assess sensitivity and adaptive capacity of temperate and boreal trees to climate change. Environ. Rev. 24, 164–186. doi: 10.1139/er-2015-0072
Bates, D., Mächler, M., Bolker, B., and Walker, S. (2015). Fitting linear mixed-effects models using lme4. J. Stat. Softw. 67, 1–48.
Berry, J., and Björkman, O. (1980). Photosynthetic response and adaptation to temperature in higher plants. Annu. Rev. Plant Physiol. 31, 491–543. doi: 10.1146/annurev.pp.31.060180.002423
Boisvert-Marsh, L. Périé, C., and de Blois, S. (2014). Shifting with climate? Evidence for recent changes in tree species distribution at high latitudes. Ecosphere 5:art83. doi: 10.1890/ES14-00111.1
Boulanger, Y., Taylor, A. R., Price, D. T., Cyr, D., McGarrigle, E., Rammer, W., et al. (2017). Climate change impacts on forest landscapes along the Canadian southern boreal forest transition zone. Landsc. Ecol. 32, 1415–1431. doi: 10.1007/s10980-016-0421-7
Bouvet, J.-M., Vigneron, P., and Saya, A. (2005). Phenotypic plasticity of growth trajectory and ontogenic allometry in response to density for eucalyptus hybrid clones and families. Ann. Bot. 96, 811–821. doi: 10.1093/aob/mci231
Burns, R. M., and Honkala, B. H. (1990). Silvics of North America: 1. Conifers. Agriculture handbook. Washington, DC: US Department of Agriculture, Forest Service.
Carter, K. K. (1996). Provenance tests as indicators of growth response to climate change in 10 north temperate tree species. Can. J. For. Res. 26, 1089–1095.
Chevin, L.-M., and Lande, R. (2010). When do adaptive plasticity and genetic evolution prevent extinction of a density-regulated population? Evolution 64, 1143–1150. doi: 10.1111/j.1558-5646.2009.00875.x
Choat, B., Brodribb, T. J., Brodersen, C. R., Duursma, R. A., López, R., and Medlyn, B. E. (2018). Triggers of tree mortality under drought. Nature 558, 531–539. doi: 10.1038/s41586-018-0240-x
Collier, J., MacLean, D. A. D’Orangeville, L., and Taylor, A. R. (2022). A review of climate change effects on the regeneration dynamics of balsam fir. For. Chron. 98, 54–65. doi: 10.5558/tfc2022-005
D’Orangeville, L., Côté, B., Houle, D., and Morin, H. (2013). The effects of throughfall exclusion on xylogenesis of balsam fir. Tree Physiol. 33, 516–526. doi: 10.1093/treephys/tpt027
D’Orangeville, L., Houle, D., Duchesne, L., Phillips, R. P., Bergeron, Y., and Kneeshaw, D. (2018a). Beneficial effects of climate warming on boreal tree growth may be transitory. Nat. Commun. 9:3213. doi: 10.1038/s41467-018-05705-4
D’Orangeville, L., Maxwell, J., Kneeshaw, D., Pederson, N., Duchesne, L., Logan, T., et al. (2018b). Drought timing and local climate determine the sensitivity of eastern temperate forests to drought. Glob. Change Biol. 24, 2339–2351. doi: 10.1111/gcb.14096
DeWitt, T. J., Sih, A., and Wilson, D. S. (1998). Costs and limits of phenotypic plasticity. Trends Ecol. Evol. 13, 77–81. doi: 10.1016/S0169-5347(97)01274-3
Domec, J.-C., Lachenbruch, B., Meinzer, F., Woodruff, D., Warren, J., and McCulloh, K. (2008). Maximum height in a conifer is associated with conflicting requirements for xylem design. Proc. Natl. Acad. Sci. U.S.A. 105, 12069–12074. doi: 10.1073/pnas.0710418105
Domec, J.-C., Noormets, A., Sun, G., King, J., McNulty, S., Gavazzi, M., et al. (2009). Decoupling the influence of leaf and root hydraulic conductances on stomatal conductance and its sensitivity to vapour pressure deficit as soil dries in a drained loblolly pine plantation. Plant Cell Environ. 32, 980–991. doi: 10.1111/j.1365-3040.2009.01981.x
Donohue, K., Messiqua, D., Pyle, E. H., Heschel, M. S., and Schmitt, J. (2000). Evidence of adaptive divergence in plasticity: Density- and site-dependent selection on shade-avoidance responses in impatiens capensis. Evolution 54, 1956–1968. doi: 10.1111/j.0014-3820.2000.tb01240.x
Duchesne, L., and Houle, D. (2011). Modelling day-to-day stem diameter variation and annual growth of balsam fir (Abies balsamea (L.) Mill.) from daily climate. For. Ecol. Manag. 262, 863–872. doi: 10.1016/j.foreco.2011.05.027
Fei, S., Desprez, J. M., Potter, K. M., Jo, I., Knott, J. A., and Oswalt, C. M. (2017). Divergence of species responses to climate change. Sci. Adv. 3:e1603055. doi: 10.1126/sciadv.1603055
Fisichelli, N., Frelich, L. E., and Reich, P. B. (2012). Sapling growth responses to warmer temperatures ‘cooled’ by browse pressure. Glob. Change Biol. 18, 3455–3463. doi: 10.1111/j.1365-2486.2012.02785.x
Fisichelli, N., Wright, A., Rice, K., Mau, A., Buschena, C., and Reich, P. B. (2014). First-year seedlings and climate change: Species-specific responses of 15 North American tree species. Oikos 123, 1331–1340. doi: 10.1111/oik.01349
Fryer, J. H., and Ledig, F. T. (1972). Microevolution of the photosynthetic temperature optimum in relation to the elevational complex gradient. Can. J. Bot. 50, 1231–1235. doi: 10.1139/b72-149
Garzón, M. B., Alía, R., Robson, T. M., and Zavala, M. A. (2011). Intra-specific variability and plasticity influence potential tree species distributions under climate change. Glob. Ecol. Biogeogr. 20, 766–778. doi: 10.1111/j.1466-8238.2010.00646.x
Ghalambor, C. K., Hoke, K. L., Ruell, E. W., Fischer, E. K., Reznick, D. N., and Hughes, K. A. (2015). Non-adaptive plasticity potentiates rapid adaptive evolution of gene expression in nature. Nature 525, 372–375. doi: 10.1038/nature15256
Ghalambor, C. K., McKAY, J. K., Carroll, S. P., and Reznick, D. N. (2007). Adaptive versus non-adaptive phenotypic plasticity and the potential for contemporary adaptation in new environments. Funct. Ecol. 21, 394–407. doi: 10.1111/j.1365-2435.2007.01283.x
Goldblum, D., and Rigg, L. S. (2005). Tree growth response to climate change at the deciduous-boreal forest ecotone, Ontario, Canada. Can. J. For. Res. 35, 2709–2718. doi: 10.1139/x05-185
Government of Canada (2011). Historical data - Climate - Environment and climate change Canada. Available online at: https://climate.weather.gc.ca/historical_data/search_historic_data_e.html (accessed November 15, 2020).
Gray, S. B., and Brady, S. M. (2016). Plant developmental responses to climate change. Dev. Biol. 419, 64–77. doi: 10.1016/j.ydbio.2016.07.023
Grossiord, C., Buckley, T. N., Cernusak, L. A., Novick, K. A., Poulter, B., Siegwolf, R. T. W., et al. (2020). Plant responses to rising vapor pressure deficit. New Phytol. 226, 1550–1566. doi: 10.1111/nph.16485
Hacke, U. G., Sperry, J. S., Pockman, W. T., Davis, S. D., and McCulloh, K. A. (2001). Trends in wood density and structure are linked to prevention of xylem implosion by negative pressure. Oecologia 126, 457–461. doi: 10.1007/s004420100628
Haider, S., Iqbal, J., Naseer, S., Yaseen, T., Shaukat, M., Bibi, H., et al. (2021). Molecular mechanisms of plant tolerance to heat stress: Current landscape and future perspectives. Plant Cell Rep. 40, 2247–2271. doi: 10.1007/s00299-021-02696-3
Hassan, Q. K., and Bourque, C. P.-A. (2009). Potential species distribution of balsam fir based on the integration of biophysical variables derived with remote sensing and process-based methods. Remote Sens. 1, 393–407. doi: 10.3390/rs1030393
Havranek, W. M., and Benecke, U. (1978). The influence of soil moisture on water potential, transpiration and photosynthesis of conifer seedlings. Plant Soil 49, 91–103. doi: 10.1007/BF02149911
Hijmans, R. J., and Graham, C. H. (2006). The ability of climate envelope models to predict the effect of climate change on species distributions. Glob. Change Biol. 12, 2272–2281. doi: 10.1111/j.1365-2486.2006.01256.x
Iverson, L. R., Prasad, A. M., Matthews, S. N., and Peters, M. (2008). Estimating potential habitat for 134 eastern US tree species under six climate scenarios. For. Ecol. Manag. 254, 390–406. doi: 10.1016/j.foreco.2007.07.023
Janiak, A., Kwaśniewski, M., and Szarejko, I. (2016). Gene expression regulation in roots under drought. J. Exp. Bot. 67, 1003–1014. doi: 10.1093/jxb/erv512
Kannenberg, S. A., Maxwell, J. T., Pederson, N., D’Orangeville, L., Ficklin, D. L., and Phillips, R. P. (2019). Drought legacies are dependent on water table depth, wood anatomy and drought timing across the eastern US. Ecol. Lett. 22, 119–127. doi: 10.1111/ele.13173
Kelly, M. (2019). Adaptation to climate change through genetic accommodation and assimilation of plastic phenotypes. Philos. Trans. R. Soc. Lond. B Biol. Sci. 374:20180176. doi: 10.1098/rstb.2018.0176
Klein, T., Zeppel, M. J. B., Anderegg, W. R. L., Bloemen, J., De Kauwe, M. G., Hudson, P., et al. (2018). Xylem embolism refilling and resilience against drought-induced mortality in woody plants: Processes and trade-offs. Ecol. Res. 33, 839–855. doi: 10.1007/s11284-018-1588-y
Kono, Y., Ishida, A., Saiki, S.-T., Yoshimura, K., Dannoura, M., Yazaki, K., et al. (2019). Initial hydraulic failure followed by late-stage carbon starvation leads to drought-induced death in the tree Trema orientalis. Commun. Biol. 2:8. doi: 10.1038/s42003-018-0256-7
Kreyling, J., Schweiger, A. H., Bahn, M., Ineson, P., Migliavacca, M., Morel-Journel, T., et al. (2018). To replicate, or not to replicate – that is the question: How to tackle nonlinear responses in ecological experiments. Ecol. Lett. 21, 1629–1638. doi: 10.1111/ele.13134
Lande, R. (2009). Adaptation to an extraordinary environment by evolution of phenotypic plasticity and genetic assimilation. J. Evol. Biol. 22, 1435–1446. doi: 10.1111/j.1420-9101.2009.01754.x
Ledo, A., Paul, K. I., Burslem, D. F. R. P., Ewel, J. J., Barton, C., Battaglia, M., et al. (2018). Tree size and climatic water deficit control root to shoot ratio in individual trees globally. New Phytol. 217, 8–11. doi: 10.1111/nph.14863
Lenth, R. V. (2021). emmeans: Estimated marginal means, aka least-squares means. v1.5.4. Available online at: https://CRAN.R-project.org/package=emmeans (accessed October 27, 2021).
Lin, Y.-S., Medlyn, B. E., and Ellsworth, D. S. (2012). Temperature responses of leaf net photosynthesis: The role of component processes. Tree Physiol. 32, 219–231. doi: 10.1093/treephys/tpr141
Little, E. L. Jr. (1971). Atlas of United States trees. Conifers and important hardwoods, Vol. Volume 1. Washington, DC: U.S. Department of Agriculture, Forest Service.
Long, J. A. (2019). interactions: Comprehensive, user-friendly toolkit for probing interactions, v1.1.0. Available online at: https://cran.r-project.org/package=interactions (accessed July, 02).
Lopez-Iglesias, B., Villar, R., and Poorter, L. (2014). Functional traits predict drought performance and distribution of Mediterranean woody species. Acta Oecol. 56, 10–18. doi: 10.1016/j.actao.2014.01.003
Lowe, W. J., Hocker, H. W. Jr., and McCormack, M. L. Jr. (1977). Variation in balsam fir provenances planted in New England. Can. J. For. Res. 7, 63–67. doi: 10.1139/x77-009
McDowell, N. G. (2011). Mechanisms linking drought, hydraulics, carbon metabolism, and vegetation mortality. Plant Physiol. 155, 1051–1059. doi: 10.1104/pp.110.170704
McDowell, N. G., and Allen, C. D. (2015). Darcy’s law predicts widespread forest mortality under climate warming. Nat. Clim. Change 5, 669–672. doi: 10.1038/nclimate2641
McDowell, N. G., Beerling, D. J., Breshears, D. D., Fisher, R. A., Raffa, K. F., and Stitt, M. (2011). The interdependence of mechanisms underlying climate-driven vegetation mortality. Trends Ecol. Evol. 26, 523–532. doi: 10.1016/j.tree.2011.06.003
McDowell, N., Pockman, W. T., Allen, C. D., Breshears, D. D., Cobb, N., Kolb, T., et al. (2008). Mechanisms of plant survival and mortality during drought: Why do some plants survive while others succumb to drought? New Phytol. 178, 719–739. doi: 10.1111/j.1469-8137.2008.02436.x
McGregor, I. R., Helcoski, R., Kunert, N., Tepley, A. J., Gonzalez-Akre, E. B., Herrmann, V., et al. (2021). Tree height and leaf drought tolerance traits shape growth responses across droughts in a temperate broadleaf forest. New Phytol. 231, 601–616. doi: 10.1111/nph.16996
McKenney, D. W., Hutchinson, M. F., Papadopol, P., Lawrence, K., Pedlar, J., Campbell, K., et al. (2011). Customized spatial climate models for North America. Bull. Am. Meteorol. Soc. 92, 1611–1622. doi: 10.1175/2011BAMS3132.1
McMurtrie, R. E., and Wang, Y.-P. (1993). Mathematical models of the photosynthetic response of tree stands to rising CO2 concentrations and temperatures. Plant Cell Environ. 16, 1–13. doi: 10.1111/j.1365-3040.1993.tb00839.x
Moran, E., Lauder, J., Musser, C., Stathos, A., and Shu, M. (2017). The genetics of drought tolerance in conifers. New Phytol. 216, 1034–1048. doi: 10.1111/nph.14774
Nicotra, A. B., Atkin, O. K., Bonser, S. P., Davidson, A. M., Finnegan, E. J., Mathesius, U., et al. (2010). Plant phenotypic plasticity in a changing climate. Trends Plant Sci. 15, 684–692. doi: 10.1016/j.tplants.2010.09.008
Novick, K. A., Ficklin, D. L., Stoy, P. C., Williams, C. A., Bohrer, G., Oishi, A. C., et al. (2016). The increasing importance of atmospheric demand for ecosystem water and carbon fluxes. Nat. Clim. Change 6, 1023–1027. doi: 10.1038/nclimate3114
Ochuodho, T. O., Lantz, V. A., Lloyd-Smith, P., and Benitez, P. (2012). Regional economic impacts of climate change and adaptation in Canadian forests: A CGE modeling analysis. For. Policy Econ. 25, 100–112. doi: 10.1016/j.forpol.2012.08.007
Osakabe, Y., Osakabe, K., Shinozaki, K., and Tran, L.-S. P. (2014). Response of plants to water stress. Front. Plant Sci. 5:86. doi: 10.3389/fpls.2014.00086
Pacific Climate Impacts Consortium (2014). Statistically downscaled climate scenarios. University of Victoria. Available online at: https://www.pacificclimate.org/data/statistically-downscaled-climate-scenarios (accessed July 23, 2022).
Papendick, R. I., and Campbell, G. S. (1981). “Theory and measurement of water potential,” in Water potential relations in soil microbiology: SSSA special publications, eds J. F. Parr, W. R. Gardner, and L. F. Elliott (Madison, WI: Soil Science Society of America), 1–22. doi: 10.2136/sssaspecpub9.c1
R Development Core Team (2022). R: A language and environment for statistical computing, v.4.0. Vienna: R foundation for Statistical Computing.
Rodenhouse, N., Matthews, S., McFarland, K., Lambert, J., Iverson, L., Prasad, A., et al. (2008). Potential effects of climate change on birds of the Northeast. Mitig. Adapt. Strateg. Glob. Chang. 13, 517–540. doi: 10.1007/s11027-007-9126-1
Rollinson, C. R., Alexander, M. R., Dye, A. W., Moore, D. J. P., Pederson, N., and Trouet, V. (2021). Climate sensitivity of understory trees differs from overstory trees in temperate mesic forests. Ecology 102:e03264. doi: 10.1002/ecy.3264
Rollinson, C. R., Kaye, M. W., and Canham, C. D. (2016). Interspecific variation in growth responses to climate and competition of five eastern tree species. Ecology 97, 1003–1011. doi: 10.1890/15-1549.1
Rossi, S., Simard, S., Rathgeber, C. B. K., Deslauriers, A., and De Zan, C. (2009). Effects of a 20-day-long dry period on cambial and apical meristem growth in Abies balsamea seedlings. Trees 23, 85–93. doi: 10.1007/s00468-008-0257-0
Rustad, L., Campbell, J., Dukes, J. S., Huntington, T., Lambert, K. F., Mohan, J., et al. (2012). Changing climate, changing forests: The impacts of climate change on forests of the northeastern United States and eastern Canada. Gen. Tech. Rep. NRS-99, Vol. 99. Newtown Square, PA: U.S. Department of Agriculture, Forest Service, Northern Research Station, 1–48. doi: 10.2737/NRS-GTR-99
Sade, N., Gebremedhin, A., and Moshelion, M. (2012). Risk-taking plants. Plant Signal. Behav. 7, 767–770. doi: 10.4161/psb.20505
Sapes, G., Roskilly, B., Dobrowski, S., Maneta, M., Anderegg, W. R. L., Martinez-Vilalta, J., et al. (2019). Plant water content integrates hydraulics and carbon depletion to predict drought-induced seedling mortality. Tree Physiol. 39, 1300–1312. doi: 10.1093/treephys/tpz062
Schweiger, A. H., Irl, S. D. H., Steinbauer, M. J., Dengler, J., and Beierkuhnlein, C. (2016). Optimizing sampling approaches along ecological gradients. Methods Ecol. Evol. 7, 463–471. doi: 10.1111/2041-210X.12495
Skelton, R. P., West, A. G., and Dawson, T. E. (2015). Predicting plant vulnerability to drought in biodiverse regions using functional traits. Proc. Natl. Acad. Sci. U.S.A. 112, 5744–5749. doi: 10.1073/pnas.1503376112
Sperry, J. S., and Tyree, M. T. (1990). Water-stress-induced xylem embolism in three species of conifers. Plant, Cell & Environment 13, 427–436. doi: 10.1104/pp.014100
Taylor, A. R., Boulanger, Y., Price, D. T., Cyr, D., McGarrigle, E., Rammer, W., et al. (2017). Rapid 21st century climate change projected to shift composition and growth of Canada’s Acadian Forest Region. For. Ecol. Manag. 405, 284–294. doi: 10.1016/j.foreco.2017.07.033
Taylor, A. R., Endicott, S., and Hennigar, C. (2020). Disentangling mechanisms of early succession following harvest: Implications for climate change adaptation in Canada’s boreal-temperate forests. For. Ecol. Manag. 461:117926. doi: 10.1016/j.foreco.2020.117926
Tyree, M. T., and Ewers, F. W. (1991). The hydraulic architecture of trees and other woody plants. New Phytol. 119, 345–360. doi: 10.1111/j.1469-8137.1991.tb00035.x
Urban, M. C., Bocedi, G., Hendry, A. P., Mihoub, J.-B., Pe’er, G., Singer, A., et al. (2016). Improving the forecast for biodiversity under climate change. Science 353:aad8466. doi: 10.1126/science.aad8466
Van Kleunen, M., and Fischer, M. (2005). Constraints on the evolution of adaptive phenotypic plasticity in plants. New Phytol. 166, 49–60. doi: 10.1111/j.1469-8137.2004.01296.x
van Vuuren, D. P., Edmonds, J., Kainuma, M., Riahi, K., Thomson, A., Hibbard, K., et al. (2011). The representative concentration pathways: An overview. Clim. Change 109:5. doi: 10.1007/s10584-011-0148-z
Vaughn, R., and Taylor, A. (2022). Projected winter warming unlikely to affect germination success of balsam fir regeneration in Atlantic Canada. Forestry 95, 659–666. doi: 10.1093/forestry/cpac019
Vaughn, W. R., Taylor, A. R., MacLean, D. A., D’Orangeville, L., and Lavigne, M. B. (2021). Climate change experiment suggests divergent responses of tree seedlings in eastern North America’s Acadian Forest Region over the 21st century. Can. J. For. Res. 51, 1888–1902. doi: 10.1139/cjfr-2021-0047
Via, S., and Lande, R. (1985). Genotype-environment interaction and the evolution of phenotypic plasticity. Evolution 39, 505–522. doi: 10.1111/j.1558-5646.1985.tb00391.x
Wang, J., and Taylor, A. R.D’Orangeville, L. (in press). Large, near-term increases in climate-induced tree growth may help offset impacts of increasing disturbance across Canadian boreal forest. Proc. Natl. Acad. Sci. U.S.A.
Way, D. A., and Sage, R. F. (2008). Elevated growth temperatures reduce the carbon gain of black spruce [Picea mariana (Mill.) B.S.P.]. Glob. Change Biol. 14, 624–636. doi: 10.1111/j.1365-2486.2007.01513.x
Yuan, W., Zheng, Y., Piao, S., Ciais, P., Lombardozzi, D., Wang, Y., et al. (2019). Increased atmospheric vapor pressure deficit reduces global vegetation growth. Sci. Adv. 5:eaax1396. doi: 10.1126/sciadv.aax1396
Zhang, X., Flato, G., Kirchmeier-Young, M., Vincent Wan, H., Wang, X., Rong, R., et al. (2019). “Chapter 4—Canada’s changing climate report,” in Changes in temperature and precipitation across Canada, eds E. Bush and D. S. Lemmen (Ottawa, ON: Government of Canada).
Keywords: Abies balsamea (L.) Mill., balsam fir, climate change, drought, provenance, phenotypic plasticity, intraspecific variation, acclimation
Citation: Ravn J, D’Orangeville L, Lavigne MB and Taylor AR (2022) Phenotypic plasticity enables considerable acclimation to heat and drought in a cold-adapted boreal forest tree species. Front. For. Glob. Change 5:1075787. doi: 10.3389/ffgc.2022.1075787
Received: 20 October 2022; Accepted: 28 November 2022;
Published: 14 December 2022.
Edited by:
Guobao Xu, Northwest Institute of Eco-Environment and Resources (CAS), ChinaReviewed by:
Alexander G. Ivanov, Bulgarian Academy of Sciences, BulgariaYassine Messaoud, Université du Québec en Abitibi–- Témiscamingue, Canada
Copyright © 2022 Ravn, D’Orangeville, Lavigne and Taylor. This is an open-access article distributed under the terms of the Creative Commons Attribution License (CC BY). The use, distribution or reproduction in other forums is permitted, provided the original author(s) and the copyright owner(s) are credited and that the original publication in this journal is cited, in accordance with accepted academic practice. No use, distribution or reproduction is permitted which does not comply with these terms.
*Correspondence: Jacob Ravn, anJhdm5AdW5iLmNh