- 1BPMP, CNRS, INRAE, Institut Agro, Université de Montpellier, Montpellier, France
- 2Department of Crop and Soil Sciences, North Carolina State University, Raleigh, NC, United States
- 3Excellence Unit AGRIENVIRONMENT, Agribiotechnology Research Institute, University of Salamanca, Salamanca, Spain
Global climate changes have serious consequences on natural ecosystems and cause diverse environmental abiotic stressors that negatively affect plant growth and development. Trees are dependent on their symbiosis with mycorrhizal fungi, as the hyphal network significantly improves the uptake of water and essential mineral nutrients by colonized roots. A number of recent studies has enhanced our knowledge on the functions of mycorrhizal associations between fungi and plant roots. Moreover, a series of timely studies have investigated the impact and benefit of root symbioses on the adaptation of plants to climate change-associated stressors. Trees in temperate and boreal forests are increasingly exposed to adverse environmental conditions, thus affecting their durable growth. In this mini-review, we focus our attention on the role mycorrhizal symbioses play in attenuating abiotic stressors imposed on trees facing climatic changes, such as high temperatures, drought, salinity, and flooding.
Introduction
Plant growth and development are dependent on intimate interspecific interactions and co-adaptation between plant species and soil microorganisms, and will be increasingly affected by environmental and climatic changes (van der Putten, 2012; Steidinger et al., 2019; Suz et al., 2021; Van Nuland et al., 2021). Trees, requiring sustained growth in fluctuating environmental conditions, are particularly vulnerable to increasing stress. Functional plant-microbe interactions are determinant drivers for plant adaptation to environmental stressors, among them severe and frequent climate-caused factors (Gehring et al., 2017; Lau et al., 2017). Such increasing abiotic stress conditions include high temperature waves associated with increasing solar radiation (Teskey et al., 2015), intensifying periods of drought (Cook et al., 2018), high levels of salinity (Corwin, 2021), and unpredictable events of flooding (Alfieri et al., 2017; Tabari, 2020; Figure 1A). Often, these conditions are tightly connected to poor soils with nutrient shortages. In temperate and boreal forest ecosystems, belowground beneficial interactions with plant roots are dominated by mycorrhizal symbioses. Trees are dependent on mycorrhizal fungi because they rely on the hyphal network, extending the root area of exploration beyond the rhizosphere, to increase the acquisition of essential macro- and microelements and water by colonized roots (Smith and Read, 2010; Becquer et al., 2019; Xu and Zwiazek, 2020).
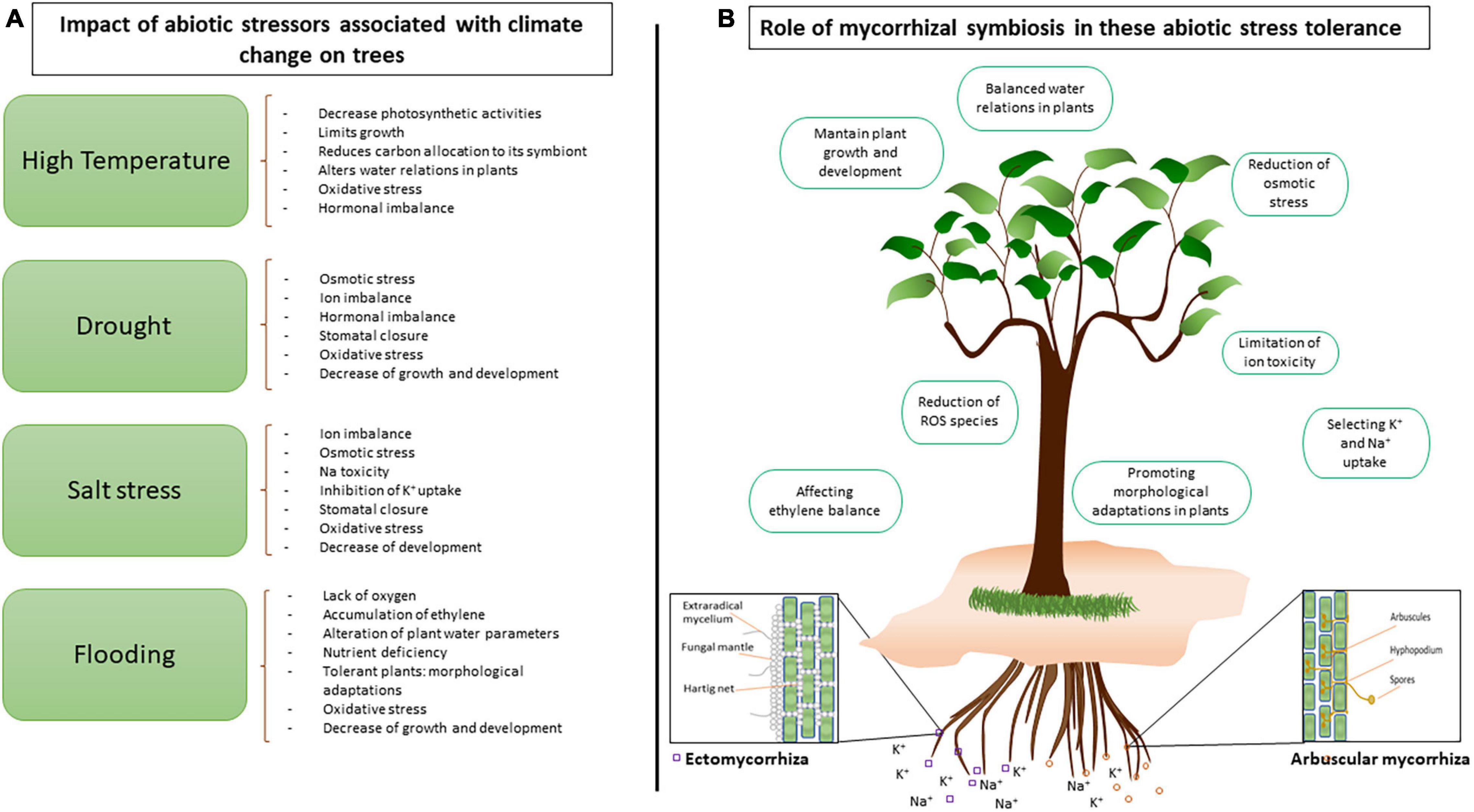
Figure 1. Mycorrhizal fungi alleviate climate change-linked abiotic stress affecting tree growth in temperate and boreal forests. (A) Trees are affected by increasing abiotic stress linked to climate change such as high temperatures, drought, salt stress, and flooding. (B) However, tree-associated mycorrhizal (ECM, ectomycorrhizal and AM, arbuscular mycorrhizal) fungi improve plant water and mineral nutrition (among them potassium K+) and help to adapt to stressful environmental conditions (among them elevated sodium Na+ in saline conditions).
Aside from the well described contribution to plant nutrition (Garcia et al., 2016, 2020), these root-associated beneficial symbionts are expected to improve the ability of their host trees to adapt to the stressor associated with climate change (Classen et al., 2015; van der Linde et al., 2018). Ectomycorrhizal (ECM) and arbuscular mycorrhizal (AM) fungi are widely associated with trees in natural forests and plantations (Figure 1B). ECM fungi, interacting with 60% of all trees (Steidinger et al., 2019) form a fungal sheath around fine roots, thus protecting the plant root and mediating direct interactions with the soil. AM fungi are more present in tropical environments than temperate and boreal forests, but do interact with several tree species, such as poplars (Fang et al., 2020), alders (Kilpeläinen et al., 2019), eucalyptus (Adjoud et al., 1996), or olive trees (Calvo-Polanco et al., 2016).
Herewith, we summarize recent reports on the role mycorrhizal symbioses play in tree growth and survival under increasingly prevalent climate change-driven abiotic stress conditions in temperate and boreal forests. In particular, we focus on publications, mainly from last few years, concerning those stressors that pose the most significant and increasing threat, namely high temperatures, drought, salinity, and flooding.
Plant Tolerance to Abiotic Stress Mediated by Mycorrhizal Interactions
Global climate change is primarily described by quantification of CO2 rise causing increase of mean temperatures. Other abiotic stresses, such as drought, salt stress or flooding, are tightly linked and affect plants and associated microorganisms (Figure 1). Whether CO2 rise will affect mycorrhizal tree symbioses in an indirect way by more carbon uptake (Godbold et al., 1997), other consequential stressors deteriorating plant growth might be alleviated by beneficial microbial root associations.
Increasing Temperatures
Frequency and intensity of high temperatures are increasing due to global warming. It can result in irreversible damages for many tree species, altering their growth and production, and sometimes leading to mortality (Teskey et al., 2015). To date, minimal research has explicitly explored the role of mycorrhizal fungi in heat stress alleviation in trees. Indeed, most studies have focused primarily on how increasing temperatures affect tree and fungal populations individually (Deslippe et al., 2011; Morgado et al., 2015; Treseder et al., 2016). However, the impacts of climate change on tree, fungal, and ecosystem health are connected and interdependent, and mycorrhizal fungi can also be directly affected by increasing soil temperatures (Kipfer et al., 2010; Gavito and Azcón–Aguilar, 2012). Higher temperatures can lower a tree’s photosynthetic activity, thereby limiting its growth and reducing carbon allocation to its ECM symbionts (Fernandez et al., 2017). Recently, Song et al. (2015) examined fungal partner changes in reaction to heat stress in two tree species, Douglas fir (Pseudotsuga menziesii) and ponderosa pine (Pinus ponderosae), and found that defoliation of the former resulted in stress signaling and resource sharing to the latter. In response to defoliation from heat and insect stress, photosynthetic carbon from P. menziesii was transferred to P. ponderosae plants via shared mycorrhizal networks (Song et al., 2015). Mueller et al. (2019) investigated growth-promoting effects of mature tree mortality on seedlings of pinyon pines and revealed increases in plant growth after mature trees died, and saw no significant loss of seedling establishment. One reason for this could be the inoculum of ECM symbionts provided by the older trees that the seedlings can utilize for acquiring the newly available resources. This indicates the importance of ECM communities in promoting the success of further generations, even as tree life expectancy may be cut short, as well as their role in promoting growth of other species following mortality. On the other hand, this ecosystem service depends on the presence of compatible species, and mortality from increasing temperatures simultaneous with heat-induced fungal community shifts, may threaten this resilience. Indeed, Fernandez et al. (2017) reported fungal taxonomic changes as a result of warming temperatures. An increased reliance on Ascomycetes and fungi that provided optimum benefits as photosynthesis capacity decreased was observed (Fernandez et al., 2017).
From these observations, it seems imperative that more research is conducted to understand how the positive effects conferred by mycorrhizal fungi will help host trees tackle the upcoming climatic variability. In particular: (i) identifying how ECM fungal communities will evolve, (ii) determining which tree-fungus combinations will be better adapted, and (iii) characterizing the underlying molecular mechanisms in both plant and fungi that prevent deleterious effects triggered by rising soil and ambient temperatures, could be promising ways to explore. Indeed, plant and ecosystem health are facing the pressure of changing global temperatures and will continue to do so to a greater extent in coming years. The respective success of each plant and microbial populations is integral in the success of the other, and further research into how ECM and AM fungi alleviate plant stress to climate change could shed light on how these interdependencies may provide ecosystem resilience through temperature increases.
Drought
Drought stress, partly linked to high temperatures, is one of the major abiotic stressors caused by climate change, and contributes to forest decline at a global level (Choat et al., 2012). Soil water deficiencies diminish plant physiological processes, including photosynthesis, enzyme structure, nutrient uptake and transport, and hormone balancing. This results in the triggering of other stressors such as nutritional, osmotic and oxidative stress. At the belowground level, roots have developed a variety of strategies to avoid and tolerate drought conditions, including root biomass adjustments, anatomical alterations and physiological acclimation, and interaction with mycorrhizal fungi.
Different mechanisms have been suggested for the improvement of plant drought tolerance by mycorrhizas. The most obvious direct mechanism to cope with water shortage is the increase of the absorbing surface in the soil through the development and ramification of an extraradical mycelium (Bogeat-Triboulot et al., 2004; Ruth et al., 2011; Zhang et al., 2018). Mycorrhizal fungi improve soil aggregate stability thanks to the structure of the hyphal network which, as part of the soil matrix, directly contributes to the formation and maintenance of soil water-macroaggregates (Ji et al., 2019), providing a better infiltration and storage of water. In the case of AM fungi, another well-identified mechanism that improves soil aggregate stability by carbon sequestration is the release of the glycoprotein glomalin by the fungus acting as soil-superglue (Kumar Singh et al., 2020; Cheng H. Q. et al., 2021).
Mycorrhizal colonization also has the ability to modify the root architecture to both enhance soil aggregate stability and increase the water and nutrient absorbing surface area, especially under drought conditions. For example, several studies in trifoliate orange suggested that mycorrhizal trees possess greater root hair growth to tolerate drought stress (Wu et al., 2016; Zou et al., 2017; Liu et al., 2018; Zhang et al., 2019). ECM fungi are reported to trigger structural changes in the root system of poplar trees by inducing lateral root formation (Felten et al., 2009; Wu et al., 2012). Finally, the modulation of root water transport under drought conditions in mycorrhizal plants has been commonly attributed to the aquaporin-mediated transport, as mycorrhizal colonization has been often shown to affect plant aquaporin gene expression (Calvo-Polanco et al., 2016, 2019; He et al., 2020).
In addition to the increased water uptake, mycorrhizal symbioses can indirectly improve nutrient acquisition under drought (Lehto and Zwiazek, 2011; Ouledali et al., 2018; Behrooz et al., 2019; Boutasknit et al., 2020). This is not only due to the increased absorption area of mycorrhizal roots, but also to factors such as the improved secretion of phosphatase to the soil, either directly by the extraradical hyphae as described for Rhizophagus clarus (Sato et al., 2019), or by stimulating the exudation of root phosphatase as observed in mycorrhizal citrus trees (Cheng H. Q. et al., 2021). These mechanisms could stimulate the decomposition and acquisition of organic orthophosphate and might thus partially alleviate the plant responses to drought stress.
Finally, mycorrhizal symbiosis also triggers numerous biochemical and physiological modifications in the plants that enhance plant drought tolerance. For example, some reports exist about the enhanced production of enzymatic (He et al., 2020; Huang et al., 2020) and non-enzymatic (Essahibi et al., 2018) antioxidants in mycorrhizal trees such as carob, trifoliate orange and apple trees under drought conditions. These enzymes and compounds may decrease the oxidative damage in the structure of carbohydrates, lipids, proteins, and DNA that result from prolonged drought exposure. It has also been suggested that mycorrhizal symbiosis has the potential to lower the osmotic damage through the accumulation of soluble sugars and proline (Yin et al., 2018; Behrooz et al., 2019; Boutasknit et al., 2020; Huang et al., 2020; Wang et al., 2021). Similarly, the water-use efficiency is in most cases improved in colonized plants, as observed in AM poplar and in ECM pine and oak trees exposed to drought (Li L. et al., 2021; Li M. et al., 2021; Li Y. et al., 2021). This could be related to a better modulation of stomata closure and abscisic acid concentration and signaling (Wang et al., 2017; Ouledali et al., 2018; Chen et al., 2020).
Salt Stress
Climate change-associated salinity stress causes land degradation, decreased crop yields, and afforestation, thus severely impacting the global economy (Leisner, 2020; Zandalinas et al., 2021). In addition, due to deep changes in environmental conditions and the expansion of irrigated land (>20% worldwide), the negative impact of salinity is considerably increasing and will continue to do so (Yuan et al., 2016). Excessive sodium (Na+) in soil competes for potassium (K+) uptake by plant roots due to ion similarity (Liang et al., 2018; Wu, 2018). Moreover, within the cytoplasm, Na+ has toxic effects on cellular functions and enzyme activation. Several endangered tree species (Bothe et al., 2010) evolved adaptations (Kijowska-Oberc et al., 2020), such as the exclusion or accumulation of the excess of Na+ within the different plant tissues (Ottow et al., 2005; Thiem et al., 2018), the control of transpiration (Negrão et al., 2017), or the increase of vessel frequencies (Janz et al., 2012).
Although many plant species have evolved salt tolerance mechanisms, interaction with soil microorganisms, and particularly mycorrhizal fungi, can greatly improve plant growth and development under saline conditions (Weissenhorn, 2002; Yi et al., 2008; Yang et al., 2014; Hrynkiewicz et al., 2015; Zwiazek et al., 2019; Klinsukon et al., 2021). Improved water (Lee et al., 2010) and nutrient (Li et al., 2012) uptake, as well as the exclusion of Na+ from roots and, consequently, shoots by mycorrhizal fungi are mechanisms that have been proposed to contribute to salt stress tolerance in colonized trees (Guerrero-Galán et al., 2019).
Improved acquisition of K+ as an essential macroelement by AM and ECM fungi (Garcia and Zimmermann, 2014; Frank and Garcia, 2021) helps maintain a desirable K+/Na+ ratio. By contrast, the mobilization of Na+ and chloride (Cl–) by plant roots was attenuated by the presence of mycorrhizal fungi, particularly under salt stress (Giri et al., 2007; Calvo-Polanco et al., 2008; Hrynkiewicz et al., 2015; Garcia et al., 2017; Hashem et al., 2018; Abdelhamid et al., 2019). To elude the translocation of excessive Na+ in photosynthetic organs, plants have adopted mechanisms to store Na+ in intraradical mycelium or in root cell vacuoles (Acosta-Motos et al., 2020). Moreover, defense mechanisms using antioxidant enzymes in plants might be improved by AM symbiosis lifting up their activities to mitigate salt stress, like catalase, peroxidase, glutathione reductase, superoxide dismutase, and ascorbate peroxidase. Ait-El-Mokhtar et al. (2019) demonstrated this mechanism when Phoenix dactylifera L. was colonized by AM fungi in salt stress conditions. Improvement of physiological plant parameters by AM and ECM symbioses has been shown by several studies in recent years, however, mechanisms have been discussed mainly for AM symbioses in non-woody plants (Porcel et al., 2012; Evelin et al., 2019). In trees, Behmanesh et al. (2019) reported that pistachio seedlings (Pistacia vera L.) inoculated by Rhizophagus irregularis (formerly Glomus intraradices) and Glomus mosseae displayed improved vegetative growth, chlorophyll contents, and less deposition of Na+ in shoots as compared to non-inoculated seedlings. Similar findings were reported by Frosi et al. (2018), when Cenostigma pyramidale was inoculated with AM fungi Acaulospora longula and Claroideoglomus etunicatum. Increases in shoot dry weight and chlorophyll contents, and less accumulation of Na+ and Cl– in photosynthetic organs were reported. Salt tolerance of almond rootstocks was increased by the AM fungi R. intraradices and Funneliformis mosseae through the improvement of diverse physiological parameters like chlorophyll, soluble sugars and proline content, osmotic parameters, and increased activity of antioxidant enzymes (Shahvali et al., 2020).
Likewise, several studies have shown improved salt tolerance in ECM trees as in white spruce in association with Hebeloma crustuliniforme (Muhsin and Zwiazek, 2002). When Coccoloba uvifera L., inoculated by Scleroderma bermudense, was grown in up to 500 mM of salt, the plants exhibited improved growth, water uptake, and shoot phosphorus and K+ concentrations, while the upward movement of Na+ and Cl– ions was limited in comparison to non-inoculated plants (Bandou et al., 2006). Improved ECM-driven uptake of essential nutrients along with water was observed in poplar roots colonized by Paxillus involutus under saline conditions (Luo et al., 2011). Involvement of aquaporin-mediated water transport was shown in jack pine in association with Suillus tomentosus (Lee et al., 2010). A recent study revealing improved growth of Alnus glutinosa when inoculated by the ECM strain P. involutus OW-5 under saline conditions, showed proline accumulation in plant tissues that acts as an endogenous osmotic regulator to tolerate salt stress effects (Thiem et al., 2020).
Altogether, mechanisms behind plant salt tolerance in AM and ECM symbiosis are rather complex, including several direct and indirect effects, and need further studies.
Flooding
Extreme precipitation and floods are expected to intensify due to climate change (Alfieri et al., 2017; Tabari, 2020), with consequences in the structure, function and production of forest ecosystems, and their interactions with soil microorganisms (Barnes et al., 2018). The adaptation of plants to flooding is dependent on the accumulation of ethylene within the plant tissues (Reynoso et al., 2019). In the most tolerant species, plants display extensive morphological modifications, such as the formation of aerenchyma (Sou et al., 2021) and adventitious roots, the reduction of the endodermis (Calvo-Polanco et al., 2012; Voesenek and Bailey-Serres, 2015), and the formation of hypertrophied lenticels (Fougnies et al., 2007).
In addition to these plant adaptations, the associated microbiome is crucial. The tolerance of mycorrhizal fungal species to flooding varies according to their host tree species and the severity and length of the stress (Lodge, 1989; Yang et al., 2016; Barnes et al., 2018; Johnson, 2018). In general, AM fungi are believed to be more present in waterlogged environments than ECM fungi, as they have been shown to survive anaerobic conditions due to the presence of well-developed aerenchyma in the roots (Wang et al., 2010). However, recent studies have determined that ECM also display a certain degree of resilience dependent on the species (Cho et al., 2021). Differences between hydrophobic and hydrophilic ECM fungi have been observed (Barnes et al., 2018), and several fungal species recovered after long periods of waterlogged field conditions (Thomas, 2021), as was shown for Thelephora terrestris, Laccaria laccata, and H. crustuliniforme (Stenström, 1991).
The few studies on AM trees under flood conditions are related to improving growth and development (e.g., citrus, peach, or trifoliate orange trees) by acting on the phosphorus and nitrogen acquisition (Neto et al., 2006; Fougnies et al., 2007; Wu et al., 2012; Zou et al., 2014; Zheng et al., 2020) and on the expression of aquaporins (Cheng X. F. et al., 2021). Even that it is commonly known that ethylene accumulation within the plant tissues is one of the main responses of plants to waterlogged conditions, this information on mycorrhizal trees is largely lacking. Only one study reported for mycorrhizal tomato plants showed that AM fungi reduced root ethylene concentrations, while improving their water status through the action of fungal and tomato aquaporins and the regulation of ACC synthase and oxidase genes (Calvo-Polanco et al., 2014).
In conclusion, there is immense potential to improve our knowledge on how mycorrhizal fungi help trees to cope with flooding events, and their effect in the main mechanism of ethylene regulation for the initial sensing of flood, that involves the oxygen-dependent degradation of group VII Ethylene Response Factor transcription factors and Plant Cysteine Oxidase enzymes (van Dongen and Licausi, 2015). This information will contribute to the development of new technologies and management procedures to overcome flood stress in agricultural and forestry settings.
Conclusion
Overall, mycorrhizal fungi have the potential to conserve and restore temperate and boreal forest ecosystems and to maintain sustainable forestry crops under stress due to global changes (Bothe et al., 2010; Field et al., 2020; Li et al., 2020). Numerous studies have shown improved tolerance of mycorrhizal trees to numerous abiotic stresses, such as high temperatures, drought, high salinity, and flooding (Figure 1). In general, the identified mechanisms are similar to those ones found in non-woody plants. However, we need to consider that most of these experiments have been performed with tree seedlings grown in pots under controlled conditions, so further research is required to unravel the underlying mechanisms in natural environments and in mature trees. The influence of natural soil microbiome interactions on complex ecosystem functioning linked to conditions of climate change has been recently analyzed taking growth and foliar development of a tree species (Populus angustifolia) as a model (Van Nuland et al., 2021). Moreover, microbiome manipulation experiments in forest conditions (e.g., adding inoculum of adapted species isolated from not stressed forest environments) could be feasible and bring further insight.
One of the most outstanding particularities of large trees is that they possess a root system that penetrates several meters into the ground. Although mycorrhizas have been commonly described to be mostly concentrated in the top layer of the soil, some reports show that they are also present several meters underground (de Araujo Pereira et al., 2018; Robin et al., 2019). With this respect, further research is required to unravel other relevant roles of mycorrhizas in deeper soil horizons and in large trees, probably more related with ecosystem services, such as carbon storage, that may also help trees to cope with environmental stresses.
Clear evidence exists to consider local beneficial mycorrhizal fungi as an environmentally friendly tool with a high potential to protect trees, from both forest and sustainable agricultural systems, against environmental stressors that will only increase in intensity and frequency due to global climate change. Although our review is focused on the negative effects of climate change on trees, it is worth mentioning that the mycorrhizal fungi decline occurring nowadays might be also linked to climate change (Bennett and Classen, 2020). However, it is unclear if mycorrhizal populations are directly affected by climate change-derived stresses or if, by contrast, they are negatively affected by a lower performance of trees exposed to stresses (Sapsford et al., 2017). Thus, further research is required to decipher the links between trees and mycorrhizal fungi under stress conditions in a climate change scenario in order to design good strategies aimed at supporting the health of both partners, trees and mycorrhizal fungi.
Author Contributions
MU, TH-P, HERF, MC-P, KG, and SDZ wrote the manuscript. MC-P, IG, KG, and SDZ revised and supervised this work. All authors contributed to the article and approved the submitted version.
Conflict of Interest
The authors declare that the research was conducted in the absence of any commercial or financial relationships that could be construed as a potential conflict of interest.
Publisher’s Note
All claims expressed in this article are solely those of the authors and do not necessarily represent those of their affiliated organizations, or those of the publisher, the editors and the reviewers. Any product that may be evaluated in this article, or claim that may be made by its manufacturer, is not guaranteed or endorsed by the publisher.
Funding
SDZ and TH-P are supported by the French ANR project “MYCOTRANS” (AAP Générique 2019 PRC N° 193112). MU is financially supported by a Ph.D. fellowship from the Pakistan Higher Education Commission. MC-P acknowledges the support from FEDER-Junta de Castilla y León, Escalera de Excelencia (RIS3; CLU-2018-04). KG and HERF acknowledge support by the AFRI program (grant no. 2020-67013-31800) from the USDA National Institute of Food and Agriculture, the North Carolina Soybean Producers Association (2019–1656), and the North Carolina Agriculture Research Service (NCARS). The funders had no role in the decision to publish or in the preparation of the manuscript.
Acknowledgments
We thank the whole research community contributing to the field and we apologize for further publications that we could have overlooked or are not included for space limitations. Contribution to research projects of former Ph.D. students Carmen Guerrero-Galán and Gabriella Houdinet is acknowledged.
References
Abdelhamid, M. T., El-Masry, R. R., Darwish, D. S., Abdalla, M. M., Oba, S., and Ragab, R. (2019). “The mechanisms involved in improving the tolerance of plants to salt stress using arbuscular mycorrhizal fungi,” in Microorganisms in Saline Environments: Strategies and Functions, eds B. Giri and A. Varma (Berlin: Springer), 303–327. doi: 10.1007/978-3-030-18975-4_13
Acosta-Motos, J. R., Penella, C., Hernández, J. A., Díaz-Vivancos, P., Sánchez-Blanco, M. J., Navarro, J. M., et al. (2020). Towards a sustainable agriculture: strategies involving phytoprotectants against salt stress. Agronomy 10:194. doi: 10.3390/agronomy10020194
Adjoud, D., Plenchette, C., Halli-Hargas, R., and Lapeyrie, F. (1996). Response of 11 eucalyptus species to inoculation with three arbuscular mycorrhizal fungi. Mycorrhiza 6, 129–135. doi: 10.1007/s005720050117
Ait-El-Mokhtar, M., Laouane, R. B., Anli, M., Boutasknit, A., Wahbi, S., and Meddich, A. (2019). Use of mycorrhizal fungi in improving tolerance of the date palm (Phoenix dactylifera L.) seedlings to salt stress. Sci. Hortic. 253, 429–438. doi: 10.1016/j.scienta.2019.04.066
Alfieri, L., Bisselink, B., Dottori, F., Naumann, G., de Roo, A., Salamon, P., et al. (2017). Global projections of river flood risk in a warmer world. Earths Future 5, 171–182. doi: 10.1002/2016EF000485
Bandou, E., Lebailly, F., Muller, F., Dulormne, M., Toribio, A., Chabrol, J., et al. (2006). The ectomycorrhizal fungus Scleroderma bermudense alleviates salt stress in seagrape (Coccoloba uvifera L.) seedlings. Mycorrhiza 16, 559–565. doi: 10.1007/s00572-006-0073-6
Barnes, C. J., van der Gast, C. J., McNamara, N. P., Rowe, R., and Bending, G. D. (2018). Extreme rainfall affects assembly of the root-associated fungal community. New Phytol. 220, 1172–1184. doi: 10.1111/nph.14990
Becquer, A., Guerrero-Galán, C., Eibensteiner, J. L., Houdinet, G., Bücking, H., Zimmermann, S. D., et al. (2019). The ectomycorrhizal contribution to tree nutrition. Adv. Bot. Res. 89, 77–126. doi: 10.1016/bs.abr.2018.11.003
Behmanesh, Z., Alaei, H., Mohammadi, A. H., and Dashti, H. (2019). Effect of arbuscular mycorrhizas Glomus intraradices and Glomus mosseae on pistachio root rot caused by Phytophthora under salinity stress. Iran. J. Plant Protect. Sci. 50, 197–212.
Behrooz, A., Vahdati, K., Rejali, F., Lotfi, M., Sarikhani, S., and Leslie, C. (2019). Arbuscular mycorrhiza and plant growth-promoting bacteria alleviate drought stress in walnut. HortScience 54, 1087–1092. doi: 10.21273/HORTSCI13961-19
Bennett, A. E., and Classen, A. T. (2020). Climate change influences mycorrhizal fungal–plant interactions, but conclusions are limited by geographical study bias. Ecology 101:e02978. doi: 10.1002/ecy.2978
Bogeat-Triboulot, M.-B., Bartoli, F., Garbaye, J., Marmeisse, R., and Tagu, D. (2004). Fungal ectomycorrhizal community and drought affect root hydraulic properties and soil adherence to roots of Pinus pinaster seedlings. Plant Soil 267, 213–223. doi: 10.1007/s11104-005-5349-7
Bothe, H., Turnau, K., and Regvar, M. (2010). The potential role of arbuscular mycorrhizal fungi in protecting endangered plants and habitats. Mycorrhiza 20, 445–457. doi: 10.1007/s00572-010-0332-4
Boutasknit, A., Baslam, M., Ait-El-Mokhtar, M., Anli, M., Ben-Laouane, R., Douira, A., et al. (2020). Arbuscular mycorrhizal fungi mediate drought tolerance and recovery in two contrasting carob (Ceratonia siliqua L.) ecotypes by regulating stomatal, water relations, and (in) organic adjustments. Plants 9:80. doi: 10.3390/plants9010080
Calvo-Polanco, M., Armada, E., Zamarreńo, A. M., García-Mina, J. M., and Aroca, R. (2019). Local root ABA/cytokinin status and aquaporins regulate poplar responses to mild drought stress independently of the ectomycorrhizal fungus Laccaria bicolor. J. Exp. Bot. 70, 6437–6446. doi: 10.1093/jxb/erz389
Calvo-Polanco, M., Molina, S., Zamarreńo, A. M., García-Mina, J. M., and Aroca, R. (2014). The symbiosis with the arbuscular mycorrhizal fungus Rhizophagus irregularis drives root water transport in flooded tomato plants. Plant Cell Physiol. 55, 1017–1029. doi: 10.1093/pcp/pcu035
Calvo-Polanco, M., Sánchez-Castro, I., Cantos, M., García, J. L., Azcón, R., Ruiz-Lozano, J. M., et al. (2016). Effects of different arbuscular mycorrhizal fungal backgrounds and soils on olive plants growth and water relation properties under well–watered and drought conditions. Plant Cell Environ. 39, 2498–2514. doi: 10.1111/pce.12807
Calvo-Polanco, M., Seńorans, J., and Zwiazek, J. J. (2012). Role of adventitious roots in water relations of tamarack (Larix laricina) seedlings exposed to flooding. BMC Plant Biol. 12:99. doi: 10.1186/1471-2229-12-99
Calvo-Polanco, M., Zwiazek, J. J., and Voicu, M. C. (2008). Responses of ectomycorrhizal American elm (Ulmus americana) seedlings to salinity and soil compaction. Plant Soil 308, 189–200. doi: 10.1007/s11104-008-9619-z
Chen, W., Meng, P., Feng, H., and Wang, C. (2020). Effects of arbuscular mycorrhizal fungi on growth and physiological performance of Catalpa bungei CA Mey. under drought stress. Forests 11:1117. doi: 10.3390/f11101117
Cheng, H. Q., Giri, B., Wu, Q. S., Zou, Y. N., and Kuča, K. (2021). Arbuscular mycorrhizal fungi mitigate drought stress in citrus by modulating root microenvironment. Arch. Agron. Soil Sci. [Epub ahead of print]. doi: 10.1080/03650340.2021.1878497
Cheng, X.-F., Wu, H.-H., Zou, Y.-N., Wu, Q.-S., and Kuča, K. (2021). Mycorrhizal response strategies of trifoliate orange under well-watered, salt stress, and waterlogging stress by regulating leaf aquaporin expression. Plant Physiol. Biochem. 162, 27–35. doi: 10.1016/j.plaphy.2021.02.026
Cho, Y., Shinnam, Y., Park, M. S., Kim, J. S., Kim, C. S., and Lim, Y. W. (2021). Ectomycorrhizal fungi associated with Pinus densiflora seedlings under flooding stress. Sustainability 13:4367. doi: 10.3390/su13084367
Choat, B., Jansen, S., Brodribb, T. J., Cochard, H., Delzon, S., Bhaskar, R., et al. (2012). Global convergence in the vulnerability of forests to drought. Nature 491, 752–755. doi: 10.1038/nature11688
Classen, A. T., Sundqvist, M. K., Henning, J. A., Newman, G. S., Moore, J. A., Cregger, M. A., et al. (2015). Direct and indirect effects of climate change on soil microbial and soil microbial-plant interactions: what lies ahead? Ecosphere 6, 1–21. doi: 10.1890/ES15-00217.1
Cook, B. I., Mankin, J. S., and Anchukaitis, K. J. (2018). Climate change and drought: from past to future. Curr. Clim. Change Rep. 4, 164–179. doi: 10.1007/s40641-018-0093-2
Corwin, D. L. (2021). Climate change impacts on soil salinity in agricultural areas. Eur. J. Soil Sci. 72, 842–862. doi: 10.1111/ejss.13010
de Araujo Pereira, A. P., Santana, M. C., Bonfim, J. A., de Lourdes Mescolotti, D., and Cardoso, E. J. B. N. (2018). Digging deeper to study the distribution of mycorrhizal arbuscular fungi along the soil profile in pure and mixed Eucalyptus grandis and Acacia mangium plantations. Appl. Soil Ecol. 128, 1–11. doi: 10.1016/j.apsoil.2018.03.015
Deslippe, J. R., Hartmann, M., Mohn, W. W., and Simard, S. W. (2011). Long–term experimental manipulation of climate alters the ectomycorrhizal community of Betula nana in Arctic tundra. Glob. Change Biol. 17, 1625–1636. doi: 10.1111/j.1365-2486.2010.02318.x
Essahibi, A., Benhiba, L., Babram, M. A., Ghoulam, C., and Qaddoury, A. (2018). Influence of arbuscular mycorrhizal fungi on the functional mechanisms associated with drought tolerance in carob (Ceratonia siliqua L.). Trees 32, 87–97. doi: 10.1007/s00468-017-1613-8
Evelin, H., Devi, T. S., Gupta, S., and Kapoor, R. (2019). Mitigation of salinity stress in plants by arbuscular mycorrhizal symbiosis: current understanding and new challenges. Front. Plant Sci. 10:470. doi: 10.3389/fpls.2019.00470
Fang, F., Wang, C., Wu, F., Tang, M., and Doughty, R. (2020). Arbuscular mycorrhizal fungi mitigate nitrogen leaching under poplar seedlings. Forests 11:325. doi: 10.3390/f11030325
Felten, J., Kohler, A., Morin, E., Bhalerao, R. P., Palme, K., Martin, F., et al. (2009). The ectomycorrhizal fungus Laccaria bicolor stimulates lateral root formation in poplar and Arabidopsis through auxin transport and signaling. Plant Physiol. 151, 1991–2005. doi: 10.1104/pp.109.147231
Fernandez, C. W., Nguyen, N. H., Stefanski, A., Han, Y., Hobbie, S. E., Montgomery, R. A., et al. (2017). Ectomycorrhizal fungal response to warming is linked to poor host performance at the boreal–temperate ecotone. Glob. Change Biol. 23, 1598–1609. doi: 10.1111/gcb.13510
Field, K. J., Daniell, T., Johnson, D., and Helgason, T. (2020). Mycorrhizas for a changing world: sustainability, conservation, and society. Plants People Planet 2, 98–103. doi: 10.1002/ppp3.10092
Fougnies, L., Renciot, S., Muller, F., Plenchette, C., Prin, Y., de Faria, S. M., et al. (2007). Arbuscular mycorrhizal colonization and nodulation improve flooding tolerance in Pterocarpus officinalis Jacq. seedlings. Mycorrhiza 17, 159–166. doi: 10.1007/s00572-006-0085-2
Frank, H. E. R., and Garcia, K. (2021). Benefits provided by four ectomycorrhizal fungi to Pinus taeda under different external potassium availabilities. Mycorrhiza. doi: 10.1007/s00572-021-01048-z
Frosi, G., Barros, V. A., Oliveira, M. T., Santos, M., Ramos, D. G., Maia, L. C., et al. (2018). Arbuscular mycorrhizal fungi and foliar phosphorus inorganic supply alleviate salt stress effects in physiological attributes, but only arbuscular mycorrhizal fungi increase biomass in woody species of a semiarid environment. Tree Physiol. 38, 25–36. doi: 10.1093/treephys/tpx105
Garcia, K., Bücking, H., and Zimmermann, S. D. (2020). Editorial: importance of root symbiomes for plant nutrition: new insights, perspectives, and future challenges. Front. Plant Sci. 11:594. doi: 10.3389/fpls.2020.00594
Garcia, K., Chasman, D., Roy, S., and Ané, J. M. (2017). Physiological responses and gene co-expression network of mycorrhizal roots under K+ deprivation. Plant Physiol. 173, 1811–1823. doi: 10.1104/pp.16.01959
Garcia, K., Doidy, J., Zimmermann, S. D., Wipf, D., and Courty, P.-E. (2016). Take a trip through the plant and fungal transportome of mycorrhiza. Trends Plant Sci. 21, 937–950. doi: 10.1016/j.tplants.2016.07.010
Garcia, K., and Zimmermann, S. D. (2014). The role of mycorrhizal associations in plant potassium nutrition. Front. Plant Sci. 5:337. doi: 10.3389/fpls.2014.00337
Gavito, M. E., and Azcón–Aguilar, C. (2012). Temperature stress in arbuscular mycorrhizal fungi: a test for adaptation to soil temperature in three isolates of Funneliformis mosseae from different climates. Agric. Food Sci. 21, 2–11. doi: 10.23986/afsci.4994
Gehring, C. A., Sthultz, C. M., Flores-Rentería, L., Whipple, A. V., and Whitham, T. G. (2017). Tree genetics defines fungal partner communities that may confer drought tolerance. Proc. Nat. Acad. Sci. U.S.A. 114, 11169–11174. doi: 10.1073/pnas.1704022114
Giri, B., Kapoor, R., and Mukerji, K. G. (2007). Improved tolerance of Acacia nilotica to salt stress by Arbuscular mycorrhiza, Glomus fasciculatum may be partly related to elevated K/Na ratios in root and shoot tissues. Microb. Ecol. 54, 753–760. doi: 10.1007/s00248-007-9239-9
Godbold, D. L., Berntson, G. M., and Bazzaz, F. A. (1997). Growth and mycorrhizal colonization of three North American tree species under elevated atmospheric CO2. New Phytol. 137, 433–440. doi: 10.1046/j.1469-8137.1997.00842.x
Guerrero-Galán, C., Calvo-Polanco, M., and Zimmermann, S. D. (2019). Ectomycorrhizal symbiosis helps plants to challenge salt stress conditions. Mycorrhiza 29, 291–301. doi: 10.1007/s00572-019-00894-2
Hashem, A., Abd-Allah, E. F., Alqarawi, A. A., and Egamberdieva, D. (2018). “Arbuscular mycorrhizal fungi and plant stress tolerance,” in Plant Microbiome: Stress Response, Microorganisms for Sustainability, eds D. Egamberdieva and P. Ahmad (Berlin: Springer), 81–103. doi: 10.1007/978-981-10-5514-0_4
He, J. D., Zou, Y. N., Wu, Q. S., and Kuča, K. (2020). Mycorrhizas enhance drought tolerance of trifoliate orange by enhancing activities and gene expression of antioxidant enzymes. Sci. Hortic. 262:108745. doi: 10.1016/j.scienta.2019.108745
Hrynkiewicz, K., Szymańska, S., Piernik, A., and Thiem, D. (2015). Ectomycorrhizal community structure of Salix and Betula spp. at a saline site in central Poland in relation to the seasons and soil parameters. Water Air Soil Pollut. 226, 1–15. doi: 10.1007/s11270-015-2308-7
Huang, D., Ma, M., Wang, Q., Zhang, M., Jing, G., Li, C., et al. (2020). Arbuscular mycorrhizal fungi enhanced drought resistance in apple by regulating genes in the MAPK pathway. Plant Physiol. Biochem. 149, 245–255. doi: 10.1016/j.plaphy.2020.02.020
Janz, D., Lautner, S., Wildhagen, H., Behnke, K., Schnitzler, J. P., Rennenberg, H., et al. (2012). Salt stress induces the formation of a novel type of ‘pressure wood’ in two Populus species. New Phytol. 194, 129–141. doi: 10.1111/j.1469-8137.2011.03975.x
Ji, L., Tan, W., and Chen, X. (2019). Arbuscular mycorrhizal mycelial networks and glomalin-related soil protein increase soil aggregation in Calcaric Regosol under well-watered and drought stress conditions. Soil Tillage Res. 185, 1–8. doi: 10.1016/j.still.2018.08.010
Johnson, D. (2018). Water, water everywhere…but how does it affect the functional diversity of ectomycorrhizal fungi? New Phytol. 220, 950–951. doi: 10.1111/nph.15086
Kijowska-Oberc, J., Staszak, A. M., Kamiński, J., and Ratajczak, E. (2020). Adaptation of forest trees to rapidly changing climate. Forests 11:123. doi: 10.3390/f11020123
Kilpeläinen, J., Barbero-López, A., Adamczyk, B., Aphalo, P. J., and Lehto, T. (2019). Morphological and ecophysiological root and leaf traits in ectomycorrhizal, arbuscular-mycorrhizal and non-mycorrhizal Alnus incana seedlings. Plant Soil 436, 283–297. doi: 10.1007/s11104-018-03922-w
Kipfer, T., Egli, S., Ghazoul, J., Moser, B., and Wohlgemuth, T. (2010). Susceptibility of ectomycorrhizal fungi to soil heating. Fungal Biol. 114, 467–472. doi: 10.1016/j.funbio.2010.03.008
Klinsukon, C., Lumyong, S., Kuyper, T. W., and Boonlue, S. (2021). Colonization by arbuscular mycorrhizal fungi improves salinity tolerance of eucalyptus (Eucalyptus camaldulensis) seedlings. Sci. Rep. 11:4362. doi: 10.1038/s41598-021-84002-5
Kumar Singh, A., Zhu, X., Chen, C., Wu, J., Yang, B., Zakari, S., et al. (2020). The role of glomalin in mitigation of multiple soil degradation problems. Crit. Rev. Env. Sci. Technol. doi: 10.1080/10643389.2020.1862561 [Epub ahead of print].
Lau, J. A., Lennon, J. T., and Heath, K. D. (2017). Trees harness the power of microbes to survive climate change. Proc. Nat. Acad. Sci. U.S.A. 114, 11009–11011. doi: 10.1073/pnas.1715417114
Lee, S. H., Calvo-Polanco, M., Chung, G. C., and Zwiazek, J. J. (2010). Role of aquaporins in root water transport of ectomycorrhizal jack pine (Pinus banksiana) seedlings exposed to NaCl and fluoride. Plant Cell Environ. 33, 769–780. doi: 10.1111/j.1365-3040.2009.02103.x
Lehto, T., and Zwiazek, J. J. (2011). Ectomycorrhizas and water relations of trees: a review. Mycorrhiza 21, 71–90. doi: 10.1007/s00572-010-0348-9
Leisner, C. P. (2020). Climate change impacts on food security-focus on perennial cropping systems and nutritional value. Plant Sci. 293:110412. doi: 10.1016/j.plantsci.2020.110412
Li, J., Bao, S., Zhang, Y., Ma, X., Mishra-Knyrim, M., Sun, J., et al. (2012). Paxillus involutus strains MAJ and NAU mediate K+/Na+ homeostasis in ectomycorrhizal Populus × canescens under sodium chloride stress. Plant Physiol. 159, 1771–1786. doi: 10.1104/pp.112.195370
Li, L., Zhang, H., Tang, M., and Chen, H. (2021). Nutrient uptake and distribution in mycorrhizal cuttings of Populus× canadensis ‘Neva’ under drought stress. J. Soil Sci. Plant Nutr. doi: 10.1007/s42729-021-00523-y [Epub ahead of print].
Li, M., Wang, H., Zhao, X., Lu, Z., Sun, X., and Ding, G. (2021). Role of Suillus placidus in improving the drought tolerance of Masson pine (Pinus massoniana Lamb.) seedlings. Forests 12:332. doi: 10.3390/f12030332
Li, Y., Zhang, T., Zhou, Y., Zou, X., Yin, Y., Li, H., et al. (2021). Ectomycorrhizal symbioses increase soil calcium availability and water use efficiency of Quercus acutissima seedlings under drought stress. Eur. J. For. Res. doi: 10.1007/s10342-021-01383-y [Epub ahead of print].
Li, Z., Wu, N., Meng, S., Wu, F., and Liu, T. (2020). Arbuscular mycorrhizal fungi (AMF) enhance the tolerance of Euonymus maackii Rupr. at a moderate level of salinity. PLoS One 15:e0231497. doi: 10.1371/journal.pone.0231497
Liang, W. J., Ma, X. L., Wan, P., and Liu, L. Y. (2018). Plant salt-tolerance mechanism: a review. Biochem. Biophys. Res. Comm. 495, 286–291. doi: 10.1016/j.bbrc.2017.11.043
Liu, C. Y., Zhang, F., Zhang, D. J., Srivastava, A., Wu, Q. S., and Zou, Y. N. (2018). Mycorrhiza stimulates root-hair growth and IAA synthesis and transport in trifoliate orange under drought stress. Sci. Rep. 8, 1–9. doi: 10.1038/s41598-018-20456-4
Lodge, D. (1989). The influence of soil moisture and flooding on formation of VA-endo-and ectomycorrhizae in Populus and Salix. Plant Soil 117, 243–253. doi: 10.1007/BF02220718
Luo, Z. B., Li, K., Gai, Y., Göbel, C., Wildhagen, H., Jiang, X., et al. (2011). The ectomycorrhizal fungus (Paxillus involutus) modulates leaf physiology of poplar towards improved salt tolerance. Environ. Exp. Bot. 72, 304–311. doi: 10.1016/j.envexpbot.2011.04.008
Morgado, L. N., Semenova, T. A., Welker, J. M., Walker, M. D., Smets, E., and Geml, J. (2015). Summer temperature increase has distinct effects on the ectomycorrhizal fungal communities of moist tussock and dry tundra in Arctic Alaska. Glob. Change Biol. 21, 959–972. doi: 10.1111/gcb.12716
Mueller, R. C., Scudder, C. M., Whitham, T. G., and Gehring, C. A. (2019). Legacy effects of tree mortality mediated by ectomycorrhizal fungal communities. New Phytol. 224, 155–165. doi: 10.1111/nph.15993
Muhsin, T. M., and Zwiazek, J. J. (2002). Colonization with Hebeloma crustuliniforme increases water conductance and limits shoot sodium uptake in white spruce (Picea glauca) seedlings. Plant Soil 238, 217–225. doi: 10.1023/A:1014435407735
Negrão, S., Schmöckel, S. M., and Tester, M. (2017). Evaluating physiological responses of plants to salinity stress. Ann. Bot. 119, 1–11. doi: 10.1093/aob/mcw191
Neto, D., Carvalho, L. M., Cruz, C., and Martins-Loucao, M. A. (2006). How do mycorrhizas affect C and N relationships in flooded Aster tripolium plants? Plant Soil 279, 51–63. doi: 10.1007/s11104-005-6333-y
Ottow, E. A., Brinker, M., Teichmann, T., Fritz, E., Kaiser, W., Brosché, M., et al. (2005). Populus euphratica displays apoplastic sodium accumulation, osmotic adjustment by decreases in calcium and soluble carbohydrates, and develops leaf succulence under salt stress. Plant Physiol. 139, 1762–1772. doi: 10.1104/pp.105.069971
Ouledali, S., Ennajeh, M., Zrig, A., Gianinazzi, S., and Khemira, H. (2018). Estimating the contribution of arbuscular mycorrhizal fungi to drought tolerance of potted olive trees (Olea europaea). Acta Physiol. Plant. 40, 1–13. doi: 10.1007/s11738-018-2656-1
Porcel, R., Aroca, R., and Ruiz-Lozano, J. M. (2012). Salinity stress alleviation using arbuscular mycorrhizal fungi. A review. Agron. Sust. Dev. 32, 181–200. doi: 10.1007/s13593-011-0029-x
Reynoso, M. A., Kajala, K., Bajic, M., West, D. A., Pauluzzi, G., Yao, A. I., et al. (2019). Evolutionary flexibility in flooding response circuitry in angiosperms. Science 20, 1291–1295. doi: 10.1126/science.aax8862
Robin, A., Pradier, C., Sanguin, H., Mahé, F., Lambais, G. R., de Araujo Pereira, A. P., et al. (2019). How deep can ectomycorrhizas go? A case study on Pisolithus down to 4 meters in a Brazilian eucalypt plantation. Mycorrhiza 29, 637–648. doi: 10.1007/s00572-019-00917-y
Ruth, B., Khalvati, M., and Schmidhalter, U. (2011). Quantification of mycorrhizal water uptake via high-resolution on-line water content sensors. Plant Soil 342, 459–468. doi: 10.1007/s11104-010-0709-3
Sapsford, S. J., Paap, T., Hardy, G. E. S. J., and Burgess, T. I. (2017). The ‘chicken or the egg’: which comes first, forest tree decline or loss of mycorrhizae? Plant Ecol. 218, 1093–1106. doi: 10.1007/s11258-017-0754-6
Sato, T., Hachiya, S., Inamura, N., Ezawa, T., Cheng, W., and Tawaraya, K. (2019). Secretion of acid phosphatase from extraradical hyphae of the arbuscular mycorrhizal fungus Rhizophagus clarus is regulated in response to phosphate availability. Mycorrhiza 29, 599–605. doi: 10.1007/s00572-019-00923-0
Shahvali, R., Shiran, B., Ravash, R., Fallahi, H., and Banović Đeri, B. (2020). Effect of symbiosis with arbuscular mycorrhizal fungi on salt stress tolerance in GF677 (peach×almond) rootstock. Scientia Horticulturae 272, 109535. doi: 10.1016/j.scienta.2020.109535
Song, Y. Y., Simard, S. W., Carroll, A., Mohn, W. W., and Zeng, R. S. (2015). Defoliation of interior Douglas-fir elicits carbon transfer and stress signalling to ponderosa pine neighbors through ectomycorrhizal networks. Sci. Rep. 5, 1–9. doi: 10.1038/srep08495
Sou, H. D., Masumori, M., Yamanoshita, T., and Tange, T. (2021). Primary and secondary aerenchyma oxygen transportation pathways of Syzygium kunstleri (King) Bahadur & R. C. Gaur adventitious roots in hypoxic conditions. Sci. Rep. 11:4520. doi: 10.1038/s41598-021-84183-z
Steidinger, B. S., Crowther, T. W., Liang, J., Van Nuland, M. E., Werner, G., Reich, P. B., et al. (2019). Climatic controls of decomposition drive the global biogeography of forest-tree symbioses. Nature 569, 404–408. doi: 10.1038/s41586-019-1128-0
Stenström, E. (1991). The effects of flooding on the formation of ectomycorrhizae in Pinus sylvestris seedlings. Plant Soil 131, 247–250. doi: 10.1007/BF00009455
Suz, L. M., Bidartondo, M. I., van der Linde, S., and Kuyper, T. W. (2021). Ectomycorrhizas and tipping points in forest ecosystems. New Phytol. 231, 1700–1707. doi: 10.1111/nph.17547
Tabari, H. (2020). Climate change impact on flood and extreme precipitation increases with water availability. Sci. Rep. 10:13768. doi: 10.1038/s41598-020-74038-4
Teskey, R., Wertin, T., Bauweraerts, I., Ameye, M., McGuire, M. A., and Steppe, K. (2015). Responses of tree species to heat waves and extreme heat events. Plant Cell Environ. 38, 1699–1712. doi: 10.1111/pce.12417
Thiem, D., Piernik, A., and Hrynkiewicz, K. (2018). Ectomycorrhizal and endophytic fungi associated with Alnus glutinosa growing in a saline area of central Poland. Symbiosis 75, 17–28. doi: 10.1007/s13199-017-0512-5
Thiem, D., Tyburski, J., Gołębiewski, M., and Hrynkiewicz, K. (2020). Halotolerant fungi stimulate growth and mitigate salt stress in Alnus glutinosa Gaertn. Dendrobiology 83, 30–42. doi: 10.12657/denbio.083.003
Thomas, P. W. (2021). Ectomycorrhiza resilience and recovery to extreme flood events in Tuber aestivum and Quercus robur. Mycorrhiza 31, 511–517. doi: 10.1007/s00572-021-01035-4
Treseder, K. K., Marusenko, Y., Romero-Olivares, A. L., and Maltz, M. R. (2016). Experimental warming alters potential function of the fungal community in boreal forest. Glob. Change Biol. 22, 3395–3404. doi: 10.1111/gcb.13238
van der Linde, S., Suz, L. M., Orme, C. D. L., Cox, F., Andreae, H., Asi, E., et al. (2018). Environment and host as large-scale controls of ectomycorrizal fungi. Nature 558, 243–248. doi: 10.1038/s41586-018-0189-9
van der Putten, W. H. (2012). Climate change, aboveground–belowground interactions, and species’ range shifts. Ann. Rev. Ecol. Evol. Syst. 43, 365–383. doi: 10.1146/annurev-ecolsys-110411-160423
van Dongen, J. T., and Licausi, F. (2015). Oxygen sensing and signaling. Ann. Rev. Plant Biol. 66, 345–367. doi: 10.1146/annurev-arplant-043014-114813
Van Nuland, M. E., Ware, I. M., Schadt, C. W., Yang, Z., Bailey, J. K., and Schweitzer, J. A. (2021). Natural soil microbiome variation affects spring foliar phenology with consequences for plant productivity and climate-driven range shifts. New Phytol. [Epub ahead of print]. doi: 10.1111/nph.17599
Voesenek, L. A. C. J., and Bailey-Serres, J. (2015). Flood adaptive traits and processes: an overview. New Phytol. 206, 57–73. doi: 10.1111/nph.13209
Wang, J., Zhang, H., Gao, J., Zhang, Y., Liu, Y., and Tang, M. (2021). Effects of ectomycorrhizal fungi (Suillus variegatus) on the growth, hydraulic function, and non-structural carbohydrates of Pinus tabulaeformis under drought stress. BMC Plant Biol. 21:171. doi: 10.1186/s12870-021-02945-3
Wang, W. X., Zhang, F., Chen, Z. L., Liu, J., Guo, C., He, J. D., et al. (2017). Responses of phytohormones and gas exchange to mycorrhizal colonization in trifoliate orange subjected to drought stress. Arch. Agron. Soil Sci. 63, 14–23. doi: 10.1080/03650340.2016.1175556
Wang, Y., Qiu, Q., Yang, Z., Hu, Z., Tam, N. F., and Xin, G. (2010). Arbuscular mycorrhizal fungi in two mangroves in South China. Plant Soil 331, 181–191. doi: 10.1007/s11104-009-0244-2
Weissenhorn, I. (2002). Mycorrhiza and Salt Tolerance of Trees. EU-project MYCOREM (QLK3-1999-00097) the Use of Mycorrhizal Fungi in Phytoremediation. Final Report of Partner 9. Available online at: https://www.servaplant.nl/new/wp-content/uploads/2015/10/MycoremReport.pdf (accessed June 2021).
Wu, A. S., Zou, Y. N., and Huang, Y. M. (2012). The arbuscular mycorrhizal fungus Diversispora spurca ameliorates effects of waterlogginig on growth, root system architecture and antioxidant enzyme activities of citrus seedlings. Fungal Ecol. 6, 37–43. doi: 10.1016/j.funeco.2012.09.002
Wu, H. (2018). Plant salt tolerance and Na+ sensing and transport. Crop J. 6, 215–225. doi: 10.1016/j.cj.2018.01.003
Wu, Q. S., Liu, C. Y., Zhang, D. J., Zou, Y. N., He, X. H., and Wu, Q. H. (2016). Mycorrhiza alters the profile of root hairs in trifoliate orange. Mycorrhiza 26, 237–247. doi: 10.1007/s00572-015-0666-z
Xu, H., and Zwiazek, J. J. (2020). Fungal aquaporins in ectomycorrhizal root water uptake. Front. Plant Sci. 11:302. doi: 10.3389/fpls.2020.00302
Yang, H., Koide, R. T., and Zhang, Q. (2016). Short-term waterlogging increases arbuscular mycorrhizal fungal species richness and shifts community composition. Plant Soil 404, 373–384. doi: 10.1007/s11104-016-2850-0
Yang, S. J., Zhang, Z. L., Xue, Y. X., Zhang, Z. F., and Shi, S. Y. (2014). Arbuscular mycorrhizal fungi increase salt tolerance of apple seedlings. Bot. Stud. 55, 1–7. doi: 10.1186/s40529-014-0070-6
Yi, H., Calvo-Polanco, M., MacKinnon, M. D., and Zwiazek, J. J. (2008). Responses of ectomycorrhizal Populus tremuloides and Betula papyrifera seedlings to salinity. Env. Exp. Bot. 62, 357–363. doi: 10.1016/j.envexpbot.2007.10.008
Yin, D., Song, R., Qi, J., and Deng, X. (2018). Ectomycorrhizal fungus enhances drought tolerance of Pinus sylvestris var. mongolica seedlings and improves soil condition. J. For. Res. 29, 1775–1788. doi: 10.1007/10.1007/s11676-017-0583-4
Yuan, F., Leng, B., and Wang, B. (2016). Progress in studying salt secretion from the salt glands in Recretohalophytes: how do plants secrete salt? Front. Plant Sci. 7:977. doi: 10.3389/fpls.2016.00977
Zandalinas, S. I., Fritschi, F. B., and Mittler, R. (2021). Global warming, climate change, and environmental pollution: recipe for a multifactorial stress combination disaster. Trends Plant Sci. 26, 588–599. doi: 10.1016/j.tplants.2021.02.011
Zhang, F., Wang, P., Zou, Y. N., Wu, Q. S., and Kuča, K. (2019). Effects of mycorrhizal fungi on root-hair growth and hormone levels of taproot and lateral roots in trifoliate orange under drought stress. Arch. Agron. Soil Sci. 65, 1316–1330. doi: 10.1080/03650340.2018.1563780
Zhang, F., Zou, Y. N., and Wu, Q. S. (2018). Quantitative estimation of water uptake by mycorrhizal extraradical hyphae in citrus under drought stress. Sci. Hortic. 229, 132–136. doi: 10.1016/j.scienta.2017.10.038
Zheng, F.-L., Liang, S.-M., Chu, X.-N., Yang, Y. L., and Wu, Q. S. (2020). Mycorrhizal fungi enhance flooding tolerance of peach through inducing proline accumulation and improving root architecture. Plant Soil Environ. 66, 624–631. doi: 10.17221/520/2020-PSE
Zou, Y. N., Srivastava, K., Wu, Q. S., and Huang, Y. M. (2014). Increased tolerance of trifoliate orange (Poncirus trifoliata) seedlings to waterlogging after inoculation with arbuscular mycorrhizal fungi. J. Anim. Plant Sci. 24, 1415–1420.
Zou, Y. N., Wang, P., Liu, C. Y., Ni, Q. D., Zhang, D. J., and Wu, Q. S. (2017). Mycorrhizal trifoliate orange has greater root adaptation of morphology and phytohormones in response to drought stress. Sci. Rep. 7, 1–10. doi: 10.1038/srep41134
Keywords: mycorrhizal symbioses, trees, climate change, environmental abiotic stress, high temperature, drought, salinity, flooding
Citation: Usman M, Ho-Plágaro T, Frank HER, Calvo-Polanco M, Gaillard I, Garcia K and Zimmermann SD (2021) Mycorrhizal Symbiosis for Better Adaptation of Trees to Abiotic Stress Caused by Climate Change in Temperate and Boreal Forests. Front. For. Glob. Change 4:742392. doi: 10.3389/ffgc.2021.742392
Received: 16 July 2021; Accepted: 30 August 2021;
Published: 20 September 2021.
Edited by:
Raffaella Balestrini, Institute for Sustainable Plant Protection, National Research Council (CNR), ItalyReviewed by:
Walter Chitarra, Council for Agricultural and Economics Research (CREA), ItalyAntonella Gori, University of Florence, Italy
Copyright © 2021 Usman, Ho-Plágaro, Frank, Calvo-Polanco, Gaillard, Garcia and Zimmermann. This is an open-access article distributed under the terms of the Creative Commons Attribution License (CC BY). The use, distribution or reproduction in other forums is permitted, provided the original author(s) and the copyright owner(s) are credited and that the original publication in this journal is cited, in accordance with accepted academic practice. No use, distribution or reproduction is permitted which does not comply with these terms.
*Correspondence: Sabine D. Zimmermann, c2FiaW5lLnppbW1lcm1hbm5AY25ycy5mcg==