- 1Programa de Pós-Graduação em Ecologia e Conservação, Universidade Federal do Paraná, Curitiba, Brazil
- 2Departamento de Biologia Vegetal, Instituto de Biologia, Universidade de Campinas (UNICAMP), Campinas, Brazil
- 3Laboratório de Ecologia Funcional de Comunidades (LABEF), Departamento de Botânica, Universidade Federal do Paraná, Curitiba, Brazil
- 4Laboratório de Genética and Biodiversidade, Universidade Federal de Goiás, Goiânia, Brazil
- 5Department of Biological and Environmental Sciences, University of Gothenburg, Gothenburg, Sweden
- 6Gothenburg Global Biodiversity Centre, Gothenburg, Sweden
Biodiversity can be quantified by taxonomic, phylogenetic, and functional diversity. Current evidence points to a lack of congruence between the spatial distribution of these facets due to evolutionary and ecological constraints. A lack of congruence is especially evident between phylogenetic and taxonomic diversity since the name and number of species are an artificial, yet commonly used, way to measure biodiversity. Here we hypothesize that due to evolutionary constraints that link phylogenetic and functional diversity, areas with higher phylogenetic and functional diversity will be spatially congruent in Neotropical cocosoid palms, but neither will be congruent with areas of high taxonomic diversity. Also, we hypothesize that any congruent pattern differs between rainforests and seasonally dry forests, since these palms recently colonized and diversified in seasonally dry ecosystems. We use ecological niche modeling, a phylogenetic tree and a trait database to test the spatial congruence of the three facets of biodiversity. Taxonomic and phylogenetic diversity were negatively correlated. Phylogenetic and functional diversity were positively correlated, even though their spatial congruence was lower than expected at random. Taken together, our results suggest that studies focusing solely on large-scale patterns of taxonomic diversity are missing a wealth of information on diversification potential and ecosystem functioning.
Introduction
Understanding why and how biodiversity is distributed across space is fundamental to preventing its loss in an ever-changing world. Taxonomic diversity, measured as species richness, is the most used biodiversity index (Díaz and Cabido, 2001; Jarzyna and Jetz, 2016). Biodiversity encompasses not only species richness but also evolutionary history and species functions (Convention on Biological Diversity, 2006). Phylogenetic diversity (e.g., branch lengths between species) reflects the evolutionary potential of communities (Swenson, 2011), representing both past diversification as well as the potential to evolve new lineages (Forest et al., 2007). Functional diversity determines the species role in the ecosystem (Swenson, 2011; Hortal et al., 2015), is a predictor of productivity and ecosystem vulnerability (Hooper et al., 2005), and is directly related to species’ response to environmental variation (Violle et al., 2007). The use of taxonomic diversity as the single source of information might be insufficient in describing how biodiversity patterns vary across space since high species richness does not necessarily lead to greater evolutionary and functional diversity (Sobral et al., 2014; Dreiss et al., 2015), and on top of that, the different components of biodiversity may not even be spatially congruent (Lamoreux et al., 2006). In order to prevent biodiversity loss, it is paramount to understand spatial variation of phylogenetic relationships and functional traits since they represent facets of biodiversity that are not incorporated in species richness (Diniz-Filho et al., 2013, 2019).
The majority of studies on taxonomic, phylogenetic and functional diversities focus on the differences among them and their relationships with environmental conditions, such as in vertebrates (Lamoreux et al., 2006), birds (Safi et al., 2011; Mazel et al., 2014; Sobral et al., 2014), and freshwater fish communities (Strecker et al., 2011), but see one example for plants Echeverría-Londoño et al. (2018). From an evolutionary viewpoint, the spatial mismatch among the facets of biodiversity is related to the way the ecological niches of species evolve. In general, closely related species retain ancestral ecological traits, and are therefore more ecologically similar than expected by chance, leading to phylogenetic niche conservatism (PNC; Losos, 2008). Under the premise of PNC, we expect that phylogenetic diversity would be correlated with functional diversity (Dreiss et al., 2015). That expectation will not hold if sister species have not have time to fully diverge, and therefore functional diversity will not increase in proportion to taxonomic diversity (Swenson, 2011). On the other hand, species may adapt and evolve into new areas (Bacon et al., 2017, 2018a; Cássia-Silva et al., 2020a), causing a spatial mismatch between phylogenetic and functional diversity, due to ecological divergence between sister species. Environmental change leads to evolutionary shifts within clades, for instance, rapid climate change throughout the Miocene led to the establishment of the South American open diagonal, which includes ecosystems such as Chaco, Cerrado, and Caatinga (Pennington et al., 2004).
Palms are iconic elements of the tropical flora and there is rich information on their phylogenetic relationships (Faurby et al., 2016) and morphology (Kissling et al., 2019). Palms are keystone species that sustain local faunas with fruits and seeds for the whole year (Terborgh, 1986; Hill et al., 2021). Indeed, prolific and widespread palms are reliable sources of food and construction material not just for animals, but also for humans (Cámara-Leret et al., 2017). For plants, a wide range of ecological strategies and responses to competition, stress, and disturbance can be summarized by assessing the variation in species traits. Palms are ecosystem builders (Göldel et al., 2015) and are morphologically variable, they present erect and aerial stems, stems concealed underground (e.g., acaulescent) and even climbing forms, which reach up to 170 m in length or more (Dransfield et al., 2008). Thus, palms occupy the canopy, understory, as well as intermediate layers of the forest strata (Dransfield et al., 2008).
Cocoseae is a pantropical palm tribe distributed in the Afrotropics (Elaeis and Jubaeopsis in continental Africa, and Beccariophoenix and Voanioala in Madagascar), as well as throughout the region (i.e., Cocos nucifera), but the vast majority occur in the American tropics (the Neotropics) where ca. 98% (ca. 350 species) of Cocoseae diversity is found (Dransfield et al., 2008; Meerow et al., 2015). In the Neotropics, cocosoid palms occur across vastly different ecosystems, from the Atlantic Forest and Amazonia, to Andean valleys and the Caribbean Islands (Dransfield et al., 2008). At the same time, the tribe represents a successful example of the colonization of seasonally dry ecosystems (Cássia-Silva et al., 2019). For instance, Acrocomia, Allagoptera, and Syagrus are common in seasonally dry ecosystems, while Bactris and Astrocaryum are most conspicuous in Amazonia (Bacon et al., 2017; Noblick, 2017). Environmental drivers of taxonomic, functional, and phylogenetic diversity have been studied in Neotropical palms (Bjorholm et al., 2005; Svenning et al., 2008; Göldel et al., 2015; Freitas et al., 2019; Velazco et al., 2021), but how these facets interact across space remains unexplored.
Here, we leverage the evolutionary history and life history traits of a widespread and species-rich group of palms measured at different evolutionary scales to determine how taxonomic, phylogenetic, and functional diversity interact across space. As palm morphological architecture is evolutionarily conserved (Tomlinson, 1990) and there is strong evidence for PNC in palms (Cássia-Silva et al., 2019), we hypothesize that (H1) Cocoseae phylogenetic diversity (PD) will be more spatially congruent with functional diversity (FD) than with taxonomic diversity (TD). Owing to its Neotropical rainforest origin (Meerow et al., 2015), with a remarkable in situ diversification across seasonally dry ecosystems of South America (Kissling et al., 2012; Freitas et al., 2019), we also hypothesize that (H2) the different facets of diversity have varying spatial patterns between rainforests and seasonally dry ecosystems. From H1, we predict that FD and PD will show higher spatial congruence compared with the TD. If H2 holds, we predict different patterns of congruence in TD, PD, and FD among rainforests and seasonally dry ecosystems. Incongruences amongst biodiversity metrics mean that the conclusions based solely on taxonomic diversity lack evolutionary and ecological information and therefore inaccurately portrays how biodiversity varies across space.
Materials and Methods
Distribution Data and Niche Modeling
We obtained occurrence data for the 261 cocosoid palms species based on the taxonomy from Govaerts et al. (2011) (last consulted on February 2019) from the Neotropical region from a larger dataset downloaded from the GBIF (last consulted on January 20191; The Global Biodiversity Information Facility, 2019) database. We cleaned the occurrences by removing records with repeated geographic coordinates and located in the sea or countries/provinces/capital centroids with CoordinateCleaner (Zizka et al., 2019), and with coordinates with less than two decimal places. We verified spatial inconsistencies in distribution, and corrected them based on information on Palmweb (Palmweb, 2020). Distribution ranges were obtained using species distribution modeling. To avoid spatial sampling biases in the modeling processes (i.e., more than one occurrence point inside the same pixel), we thinned species occurrences using an independence distance of 10 km. Only species with more than 10 independent occurrences were used, a total of 120 species. Species with more than five and less than 10 independent occurrence points (82 species) had their distribution estimated by minimum convex polygon enclosing all occurrences of a species, with interior angles smaller than 180°, which yielded 202 total species.
As predictor variables for species distribution modeling, we used 19 climatic and 36 physical edaphic variables (Supplementary Table 1) from the Chelsa Climate (Karger et al., 2017) and SoilGrids (Hengl et al., 2017) databases, using a 0.1°× 0.1° resolution (c. 11 km). To avoid multicollinearity issues in the modeling processes, instead of using the variables per se, we reduced the variation of all 55 variables using Principal Components Analysis (PCA). The first five orthogonal axes represented 85% of the total variation from the original values and were used as predictor variables.
We used four different algorithms to model species distributions: Generalized Additive Model, Maximum Entropy, Random Forest, and Gaussian Processes. We randomly partitioned the occurrence data in 70% for calibration and 30% for model validation, and iterated five times. We used the same number of pseudo-absences as occurrence points, selected at random. The area for model calibration (Barve et al., 2011) was defined by a minimum convex polygon enclosing all occurrences of a species, plus a buffer zone of 110 km surrounding the polygons. The model performance was assessed using True Skill Statistics (TSS; Allouche et al., 2006), which ranges from −1 to + 1, where + 1 indicates perfect agreement and values of zero or less indicate a performance no better than random.
After the modeling procedure, the projections of the different algorithms were combined in an ensemble using weighted mean, using only models with TSS higher than the model average (the best models). For the creation of the presence and absence matrix per pixel cell we used a binary map, where the threshold was the value in which the sum of the sensitivity and specificity was the highest. The species distribution modeling was performed using the ENMTML package (de Andrade et al., 2020) in the R environment 4.0.3 (R Core Team, 2020).
We built the occurrence matrix for the Neotropical region by overlapping the distribution data (distribution models and minimum convex polygons) of the 202 cocosoid species, obtaining a matrix containing species occurrence in each pixel (Supplementary Figure 1). This occurrence matrix was used to compute taxonomic, phylogenetic, and functional diversity indices. The GBIF downloaded data is available in Supplementary Table 5 and the specie names and number of occurrences is available in Supplementary Table 6.
Phylogenetic Analysis
We obtained phylogenetic information for the 202 cocosoid palm species from a dated Maximum Clade Credibility tree (MCC) of the palm family (Cássia-Silva et al., 2019), which was calculated from 1,000 phylogenetic trees from the posterior distribution of the species-level supertree (Faurby et al., 2016). In comparison to other clades represented in the supertree, the deep phylogenetic relationships (i.e., subtribes Attaleinae, Bactridinae, and Elaeidineae) were well-resolved and supported by a previous study (Meerow et al., 2015). The phylogenetic incongruences in Cocoseae were mostly restricted to the terminal nodes (Meerow et al., 2015), which overall do not significantly influence phylogenetic structure metrics (Swenson, 2011).
We calculated the Mean Pairwise Distance (MPD) and the Mean Nearest Taxon Distance (MNTD; Webb, 2000) of communities using the MCC tree with the functions mpd and mntd in the picante R package (Kembel et al., 2010). MPD is the mean of the branch lengths between all species in a community divided by the number of species, whereas MNTD is the mean branch lengths between each species within communities and its closest relative (Webb, 2000). We used these two indices because they are complementary and calculate both phylogenetic and functional diversity. While MPD provides an “ancient” picture, the MNTD provides more “recent” information of phylogenetic structure in a focal community (e.g., Cássia-Silva et al., 2020b). Community is here defined as the species co-occurring in a particular pixel cell. To calculate both MPD and MNTD, we obtained the cophenetic distance matrix using the function cophenetic. Because we used a dated tree, MPD and MNTD are in My (millions of years before present).
Functional Trait Analysis
The cocosoid traits (Table 1) were compiled from the global palm trait database (PalmTraits 1.0 data set; Kissling et al., 2019). We expanded on Westoby’s leaf-height-seed scheme (i.e., LHS; Westoby, 1998; Table 1) and included more traits, approximating the functional space of ecosystem builders such as palms (Göldel et al., 2015). The LHS scheme describes the position of a determined species in the volume formed by three axes in the functional space comprising Specific Leaf Area (SLA), height of the canopy at maturity, and seed mass (Westoby, 1998). Together the LHS traits represent the ability of species to compete for light, as well as the ability to disperse. The size of the plant, their position in the forest strata, and growth form are related to plant competitive vigor, fecundity, carbon sequestration, accumulation of biomass, as well as to ecosystem complexity (Phillips et al., 2002; Pérez-Harguindeguy et al., 2013). Additionally, leaf blade size is related to energy acquisition and photosynthetic rate (Pérez-Harguindeguy et al., 2013; Jimenez et al., 2021), while fruit color and size is related to resource availability for frugivores and plant dispersal ability (Onstein et al., 2020; Hill et al., 2021). The length of the leaf blade was highly correlated with the rachis length (r = 0.96; p < 0.001) and petiole length (r = 0.83; p < 0.001), thus we excluded the latter two and used the length of the leaf blade.
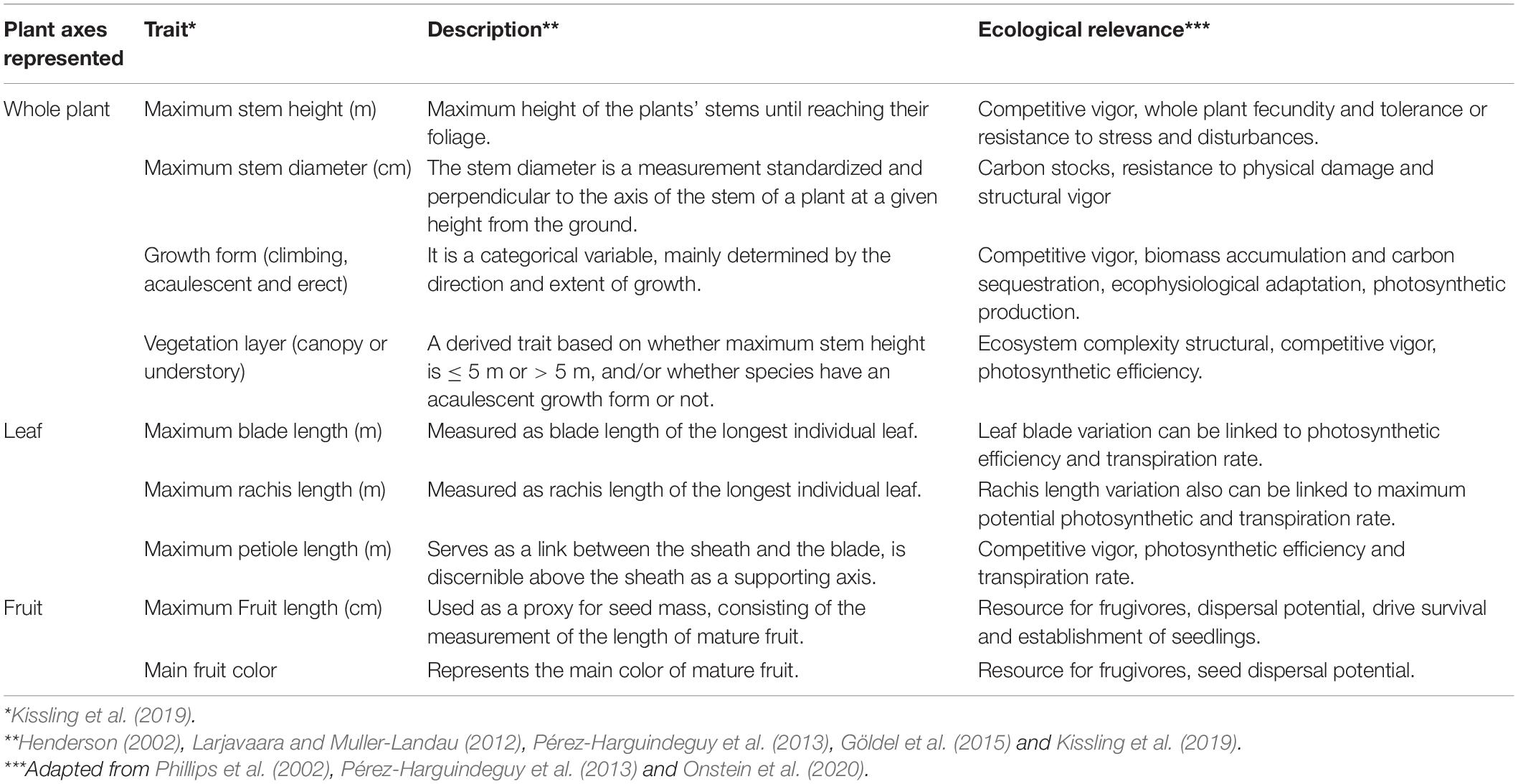
Table 1. Functional traits related to height, leaf and fruit characteristics used to obtain functional diversity in cocosoid palm species and their ecological relevance.
We used the matrix of 202 species to calculate functional MPD and MNTD as described above and following Hidasi-Neto et al. (2012), but here using trait distances instead of phylogenetic distances. We used the function dist.ktab from FD package to produce a mixed-variable coefficient of trait distances between species calculating a generalized version of Gower’s distance, which enabled the treatment of various statistically different variables (Pavoine et al., 2009). Maximum stem height, maximum stem diameter, maximum blade length, and maximum fruit length were treated as quantitative continuous variables, fruit color and growth form as nominal multiple state variables (i.e., a single species may assume one or more states), and vegetation layer as binary. Functional MPD and MNTD using Gower trait distances were computed using functions mpd and mntd in picante.
Finally, species with phenotypic variation in growth form (e.g., acaulescent and erect) were classified as erect since they may have this growth form during their lifespan (see Imputing missing data section and Supplementary Table 3 for details). This variation was present in the dataset in only three species: Astrocaryum ciliatum, A. farinosum, and A. sociale (see Supplementary Table 1 and Distribution of Communities section for details). All analyses were performed in the R statistical environment v. 3.4.3 (R Core Team, 2020).
Congruence Among Diversity Measures
We assessed the spatial congruence between taxonomic (TD), phylogenetic (PD), and functional diversity (FD) for the whole cocosoid palm distribution and between rainforests and seasonally dry ecosystems using a Geographically Weighted Correlation (GWC) with the function lcorrel implemented in the lctools package (Kalogirou, 2017). It is important to highlight that PD stands for phylogenetic diversity and is not related to the specific index called “PD” of Petchey and Gaston (2002). GWC is a measure of the correlation between variables while accounting for the spatial structure of the correlation, revealing the spatial congruence between them. GWC was plotted in heat maps of the Neotropics to show spatial variation. We also ordered our dataset from lower to higher values and divided it into quartiles, i.e., 1st (0–25%), 2nd (26–50%), 3rd (51–75%), and 4th quartile (76–100%). The congruences between the variables were converted to binary to be plotted in heat maps showing regions of congruence for the four quartiles using the raster package (Hijmans and van Etten, 2012). To test whether the spatial congruence patterns differed between rainforests and seasonally dry ecosystems for each pair of diversity index, we classified each grid cell into either rainforest or seasonally dry ecosystems using the WWF Ecoregion classification (Olson et al., 2001; Supplementary Table 2). We tested whether the mean geographic weighted correlation coefficients were different between rainforests and seasonally dry ecosystems for each comparison of the biodiversity facets using a t-test in the basic R package.
Results
Taxonomic, Phylogenetic, and Functional Diversity
Our models presented a satisfactory performance, with average value of 0.53 of TSS (0.17 SD, see Supporting Information Supplementary Figure 2). Considering our classification of areas into rainforests and seasonally dry ecosystems (Figure 1A) we describe our results below. Taxonomic diversity in cocosoid palms was especially high in rainforests such as Amazonia, inter-Andean valleys, and the northeastern portion of the Brazilian Atlantic Forest (Figure 1D). The taxonomic diversity was comparatively smaller in seasonally dry ecosystems such as in northeast Brazil, the Pacific coast of Central America, the south of Cocoseae distribution, and in the Andean slopes (Figure 1D). The Caribbean Islands and the Atlantic coast of Central America were especially species-poor. PDMPD had an opposite spatial pattern compared to species richness, being greater in the periphery of the Cocoseae distribution (Figure 1B). Phylogenetic diversity was especially high across the seasonally dry forests and the Brazilian Atlantic Forest. PDMNTD followed the same spatial pattern as the PDMPD (Figure 1C), except in seasonally dry ecosystems, where we found lower recent phylogenetic diversity compared with PDMPD patterns. On the other hand, recent phylogenetic diversity (PDMNTD) is higher in eastern Amazonia. Functional diversity (measured as FDMNTD) had a similar spatial pattern compared to PDMNTD (Figure 1F). However, FDMPD was higher in western Amazonia and seasonally dry ecosystems of the Andean slopes, Mesoamerica, and northeast Brazil and considerably lower in the seasonally dry ecosystems in central Brazil and the Atlantic Forest (Figures 1D,E).
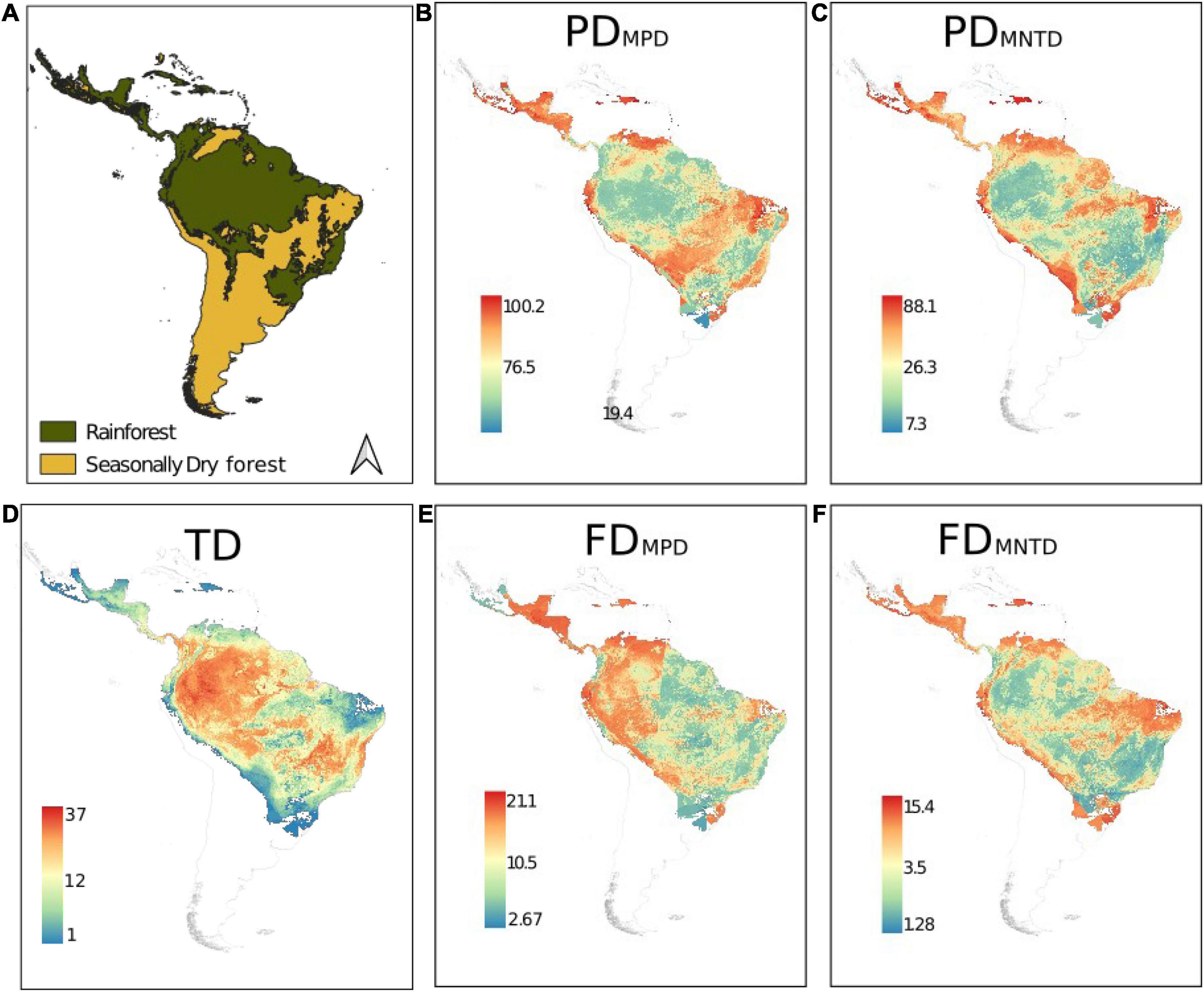
Figure 1. Geographical distribution of the taxonomic (TD), phylogenetic (PD), and functional (FD) diversity of cocosoid palm species in the Neotropics. MPD, Mean pairwise distance. MNTD, mean nearest taxon distance. (A) The classification of areas into rainforests and seasonally dry ecosystems. Phylogenetic diversity calculated using MPD (B), MNTD (C), and taxonomic diversity (D). Functional diversity calculated using MPD (E) and (F).
Spatial Congruence in the Neotropics
Overall, taxonomic diversity and PDMPD were negatively correlated across the Neotropics (Figure 2A). This pattern was most evident in Central America, the Caribbean islands, northern Andean valleys, and northern Amazonia. We found a positive but low correlation in central Brazil, the Chaco, and the southern Atlantic Forest (Figure 2A). The correlation between taxonomic diversity and FDMDP was also negative, but high only in northern Amazonia, and positive and low correlation was found in central Amazonia and central Brazil, and the southern regions of the distribution (Figure 2B). The correlation between PDMPD and FDMPD was largely positive and higher in Central America, Andean valleys, northern Amazon, Caribbean islands and Chocó (Figure 2C). Taxonomic diversity and PDMNTD and FDMNTD showed negative correlations (Figures 2D,E) across all Cocosaeae geographical distribution. We found a negative correlation between PDMNTD and FDMNTD (Figure 2F), with higher values across the seasonally dry ecosystems.
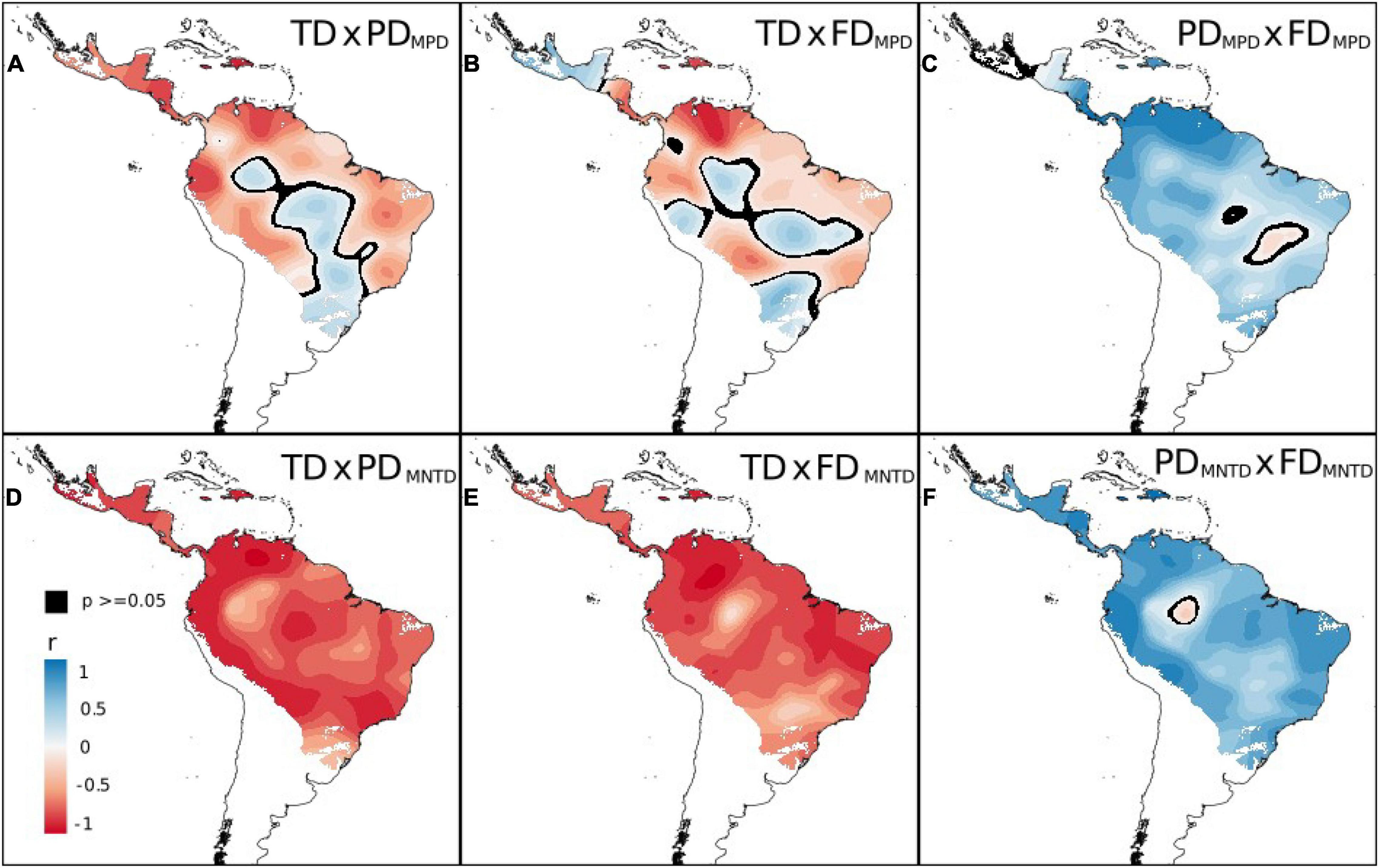
Figure 2. Spatial patterns in correlation between taxonomic (TD), phylogenetic (PD), and functional diversity (FD) of cocosoid palm species in the Neotropics using Geographically Weighted Correlation. Values range from 1 (positive—blue regions) to −1 (negative—red regions) in the map. MPD, Mean pairwise distance. MNTD, mean nearest taxon distance. In black, areas where p-value are above 0.05.
The three diversity indices of biodiversity, TD, PDMPD, and FDMPD, showed low spatial congruence in all four quartiles (Figure 3 and Table 2). The three indices were equally low (1st quartile) in the south of the Cocoseae distribution (Figure 3A and Table 2) and no region had high values (4th quartile) for the three indices (Figure 3D and Table 2). We found spatial congruence between two indices for low and intermediate values (2nd and 3rd quartiles) scattered throughout central and eastern Amazonia, and in central and eastern Brazil (Figures 3B,C and Table 2). However, PDMPD and FDMPD had high spatial congruence in the 4th quartile (Figure 3D and Table 2), mainly in Central America, the Caribbean islands, northern Amazonia, and in the Andean slopes. Similarly, we found low values of TD, PDMNTD, and FDMNTD (1st quartile) in the south of the Cocoseae distribution (Figure 4A and Table 2) and no region had high values (4th quartile) for the three indices (Figure 4D and Table 2). Congruences between two indices with intermediate values (2nd and 3rd quartiles) were found in central Brazil (Figure 4B and Table 2), western Amazonia, and in northeastern Brazil (Figure 4C and Table 2).
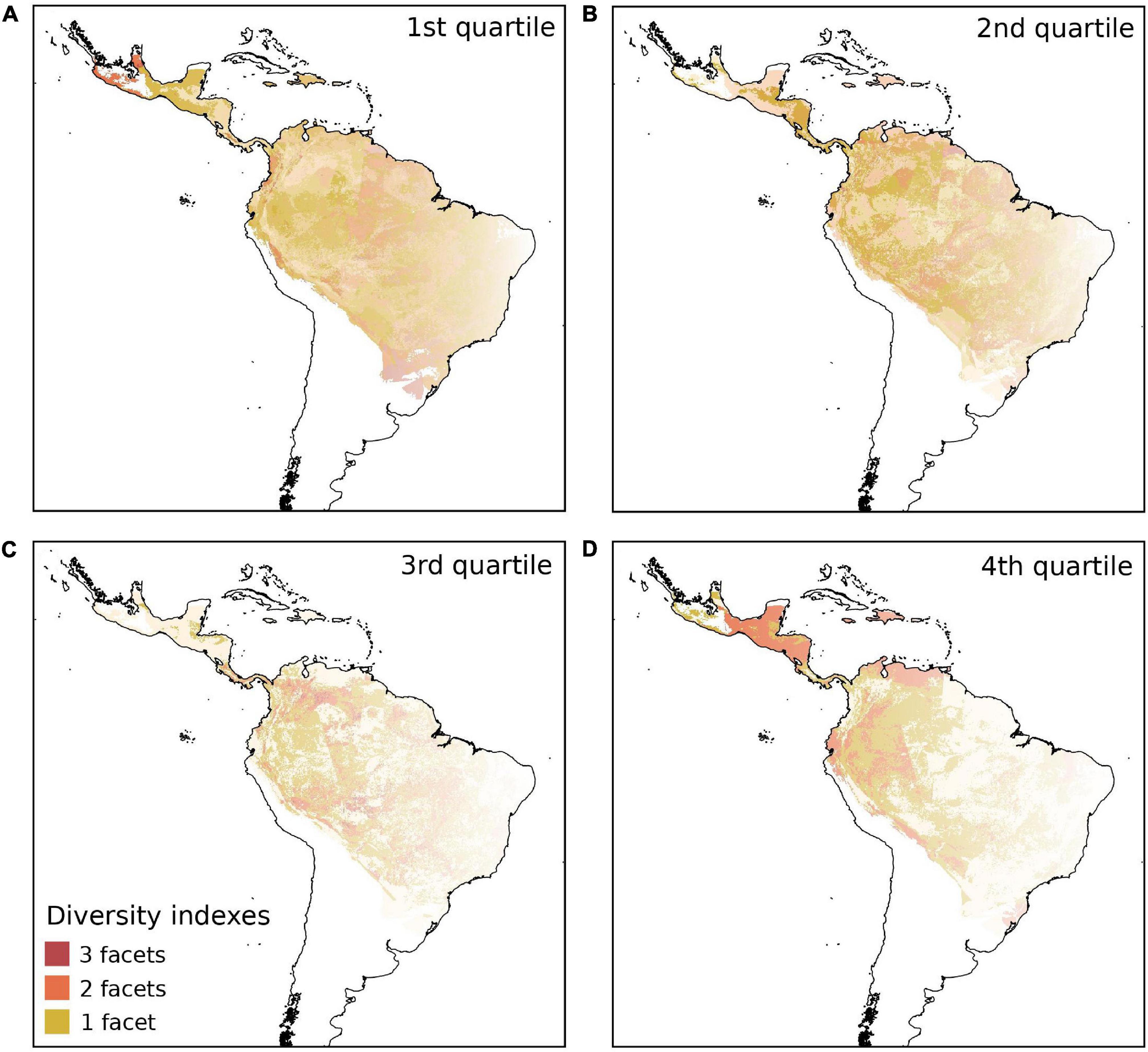
Figure 3. Spatial congruence in taxonomic, phylogenetic and functional diversity measured by Mean Pairwise Distance (MPD) of cocosoid palm species in the Neotropics, for the four quartiles; 1st quartile (A), 2nd quartile (B), 3rd quartile (C) and 4th quartile (D). Dark red shows 100% of spatial congruence among the three diversity indices, orange shows congruence between two indices, and yellow shows no congruence, i.e., the presence of only one of the indices analyzed.
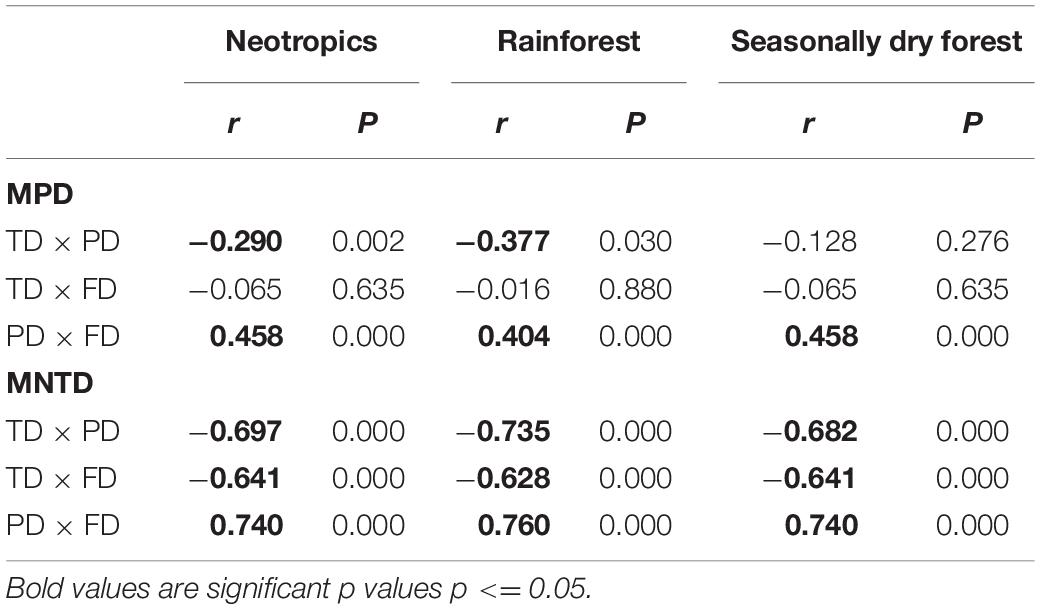
Table 2. Correlation values from geographic weighted correlations among taxonomic, phylogenetic and functional diversity between rainforests and seasonally dry forest using MPD and MNTD.
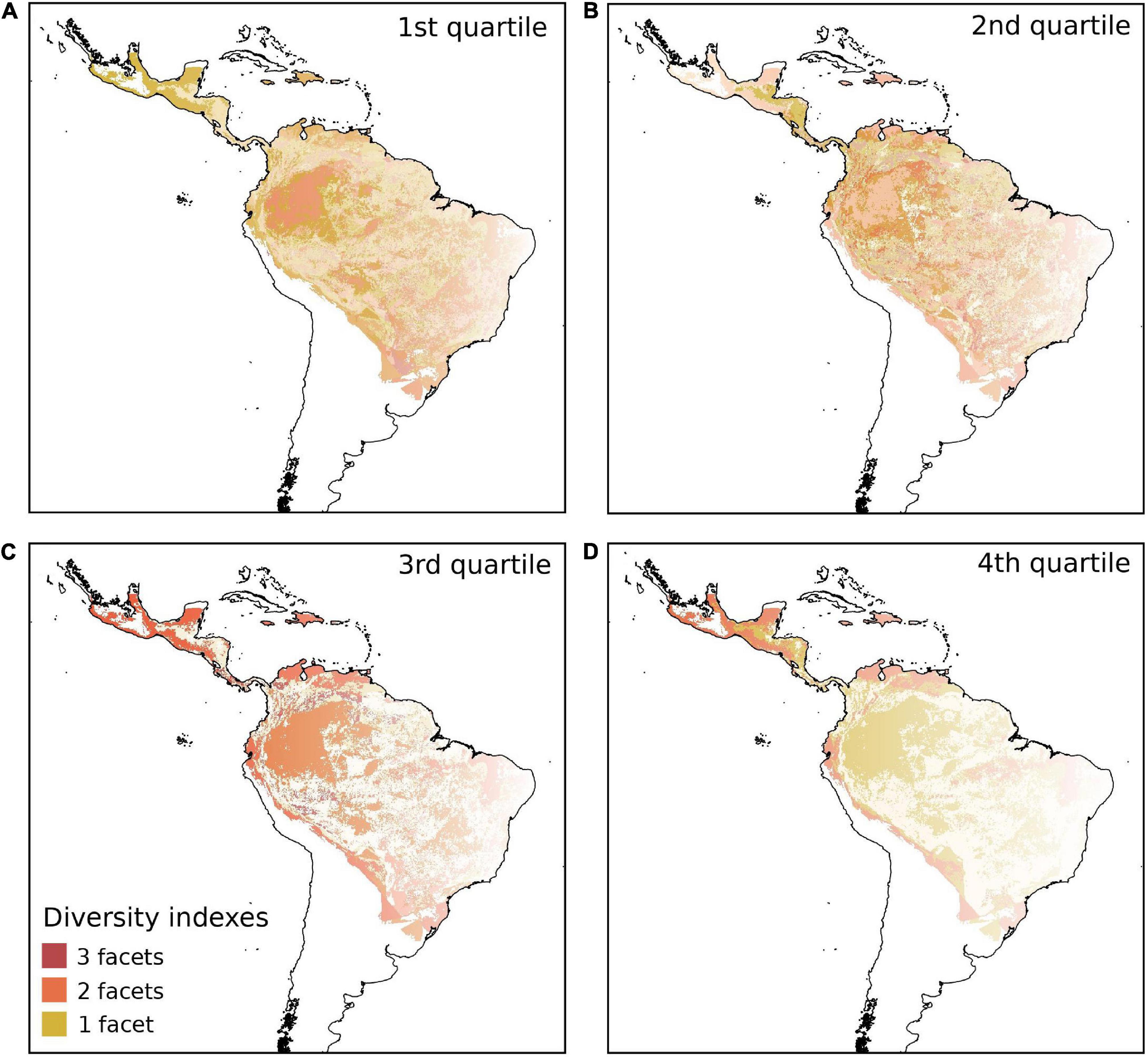
Figure 4. Spatial congruence in taxonomic, phylogenetic and functional diversity measured by Mean Nearest Taxon Distance (MNTD) of cocosoid palm species in the Neotropics, for the four quartiles; 1st quartile (A), 2nd quartile (B), 3rd quartile (C) and 4th quartile (D). Dark red shows 100% of spatial congruence among the three diversity indices, orange shows congruence between two facets two indices, and yellow shows no congruence, i.e., the presence of only one index.
Spatial Patterns of Congruence: Rainforests and Seasonally Dry Ecosystems
Overall, taxonomic diversity had a negative correlation with phylogenetic and functional diversity indices (MPD and MNTD) in rainforests and seasonally dry ecosystems (Figure 5 and Table 2). On the other hand, phylogenetic and functional diversity were positively correlated for both MPD and MNTD indices, both in rainforests and seasonally dry ecosystems (Figure 5 and Table 2).
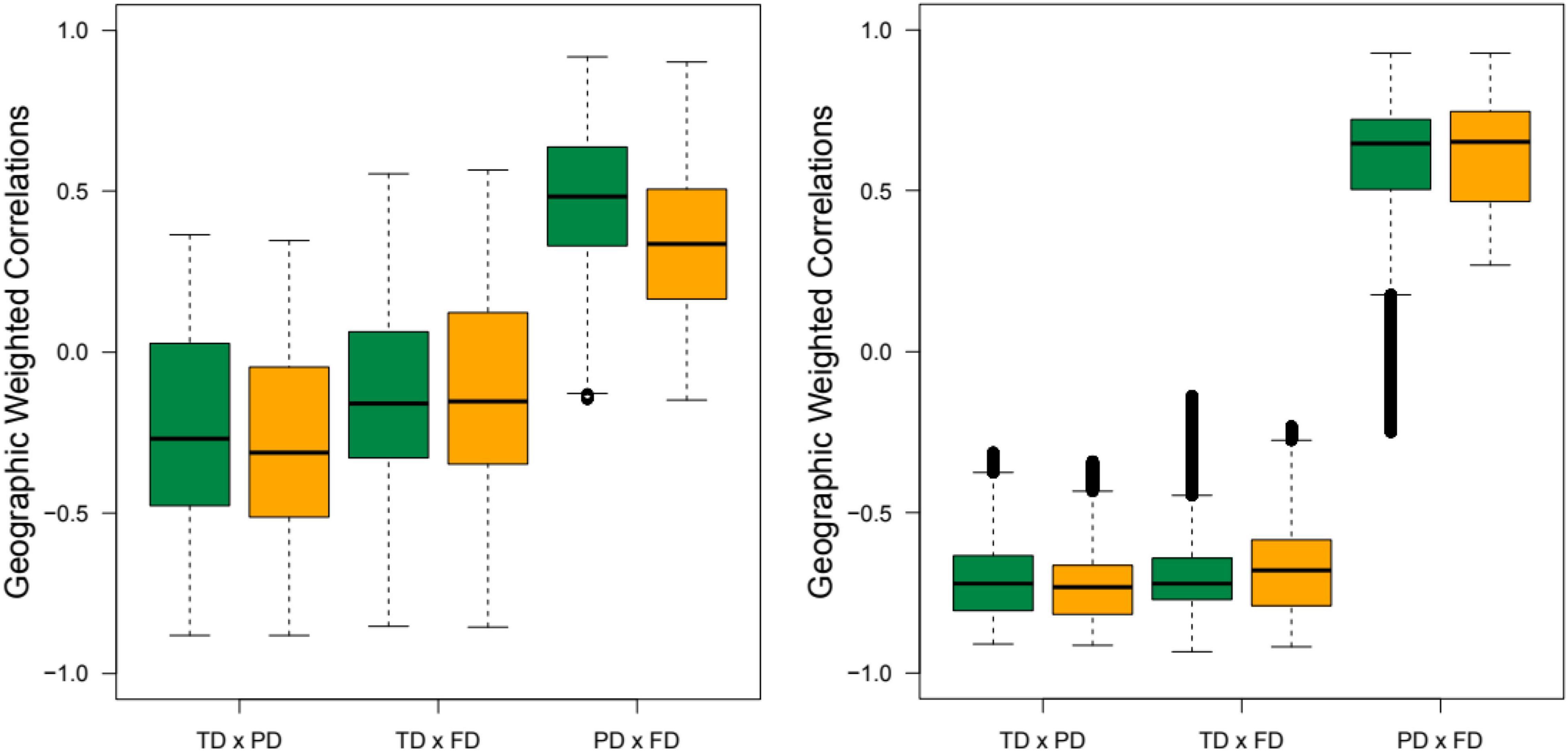
Figure 5. Geographic weighted correlations among taxonomic, phylogenetic and functional diversity between rainforests (green) and seasonally dry forests (yellow). The boxplot represents the mean correlation among the pixels classified in each ecosystem for MPD (right) and MNTD (left). All comparisons yielded a p < 0.001 (Supplementary Table 4).
Discussion
Biodiversity studies have traditionally focused on taxonomic diversity, but species names and numbers do not include fundamental information on evolutionary and ecological diversity. Here we use taxonomic, phylogenetic, and functional indices to investigate how different facets of biodiversity vary spatially. We hypothesized that phylogenetic and functional diversity have congruent spatial distributions that contrast with the spatial distribution of taxonomic diversity (H1), and that this pattern would differ between rainforests and seasonally dry forests for Cocoseae palms (H2). Our results show that taxonomic diversity is a poor predictor of phylogenetic and functional diversity, as few areas show spatial congruences between phylogenetic and functional with taxonomic diversity. Also, we found spatial congruence between functional and phylogenetic diversity only in a few geographic areas either considering ancient (MPD) and recent (MNTD) branch lengths of the phylogenetic tree. The relationship between phylogenetic and functional diversity is also apparent when data were split between rainforests and seasonally dry ecosystems.
As we expected (H1), taxonomic diversity is not a good predictor of phylogenetic or functional diversity. Indeed, species richness has little ecological and evolutionary power to decipher the mechanisms controlling species distributions, as previously shown in both birds and mammals (Mazel et al., 2014; Sobral et al., 2014). Here, we show a strong geographical mismatch among taxonomic, phylogenetic and functional diversity in plants. Thus, large-scale studies on biodiversity patterns that include solely taxonomic diversity are missing important information on both evolutionary and ecosystem functioning, since there is little spatial congruence between the three measures of biodiversity. Even focusing on the congruence of evolutionary and ecosystem functioning information in geographical space, we are ignoring relevant information, since there are only strong positive correlations in very specific areas of the Cocoseae distribution.
Palm phylogenetic diversity peaks from Costa Rica and northeastern South America toward the northern Amazon Basin (Velazco et al., 2021). By contrast, we found higher phylogenetic diversity of cocosoid palms across the open diagonal of South America (Prado and Gibbs, 1993), the Andean slopes, and the Atlantic Forest (Figure 1). This may be due to the fact that the Atlantic forest is thought to be the center of origin of Attaleinae, one of the three and more speciose subtribes of Cocoseae (Meerow et al., 2009). On the other hand, western Amazonia is suggested to be the center of origin of Bactridinae, another highly rich Cocoseae subtribe (Eiserhardt et al., 2011b), yet we found lower phylogenetic diversity compared to the former areas, indicating possibly a very recent diversification event (c.f. Roncal et al., 2013). We also found high phylogenetic diversity in the northern-most range of the Andean mountains, in agreement with evidence that this area is an important source of plant diversification for Amazonia (Bacon et al., 2018b; Gerhold et al., 2018). Also, our results show that cocosoid phylogenetic diversity congruence with taxonomic diversity is negligible, and that the correlation between the two variables is strong and negative, reinforcing our expectations that taxonomic diversity alone is not a good predictor of evolutionary history (H1).
We found more congruence between phylogenetic and functional diversity than either of them with taxonomic diversity, as expected. For instance, phylogenetic and functional diversity are congruently high in the Andean valleys, northeast Brazil, the Chaco and the Atlantic Forest in the southern Brazilian Coast. On the other hand, areas such as in the transition between Amazonia, the Cerrado, and the Caatinga showed low functional diversity, but high phylogenetic diversity considering ancient relationships (MPD; see Figures 1B,D), and recent relationships in the Guiana Shield (MNTD; Figures 1C,E). These opposing results do not support our expectations, as taxonomic and functional plant diversity was found to be highly congruent in space (Echeverría-Londoño et al., 2018). We suggest the lower level of functional diversity compared with both taxonomic and phylogenetic found here is linked to the fact that natural transition areas among ecosystems are more prone to ecological selection, filtering certain traits unfit for these higher level of stress (e.g., drought, increased solar radiation; Metcalfe et al., 2020). Finally, the higher functional diversity compared to phylogenetic diversity might be due to recent divergence events, increasing the variability among traits.
Both phylogenetic and functional diversities reflect evolutionary and ecological aspects hidden behind the species names in occurrence tables. The phylogenetic diversity of a particular clade tells the history of past diversifications and possible extinctions, and might lead to a better understanding of the species diversity changes. At the same time, phylogenetic diversity also provides information about the future of the lineages, acting as a “savings bank” of diversity needed in the face of environmental change. Functional diversity reflects the ecological role of species and is related to the potential of communities to sustain crucial ecological processes needed to keep ecosystems functioning properly. One important caveat here is that even carefully selecting important traits to reflect functional diversity in the trait space, one can only estimate the real functional diversity present in the community (Díaz and Cabido, 2001). Even so, our results lead to the conclusion that all components of biodiversity are needed to describe and conserve plant species diversity.
Under phylogenetic niche conservatism, species retain ecological traits similar to their ancestors, so functional diversity is expected to track evolutionary diversity (H2). Here we found that the spatial congruence between phylogenetic and functional diversity, in general, is unexpectedly low, however the level of congruence depends on the area, as we expected. The high phylogenetic diversity (i.e., MPD) across the open diagonal of South America found here suggests an increase in diversification of species adapted to seasonally dry conditions driven by Quaternary climate changes (Kissling et al., 2012). The Brazilian savanna (i.e., Cerrado), which is a prominent part of the open diagonal, presented low congruence between phylogenetic and functional diversity and shows lower functional diversity compared to phylogenetic diversity, which is potentially due to recent divergence events driven by climatic history (Pennington et al., 2004) leading to ecological evolution and adaptation to the new area. Further, lower functional diversity compared to phylogenetic diversity was also found in the transition between Amazonia, the Cerrado and Caatinga, in northeastern Brazil, and Chaco. A mismatch between phylogenetic and functional diversity might also be due to non-random species extinction (Purvis et al., 2000), where species are filtered out due to unfit traits (Cavender-Bares et al., 2004). On the other hand, another area of recent geological change, western Amazonia (Hoorn et al., 2010), presents an opposite pattern of higher functional compared to phylogenetic diversity, which is due to a disproportionate high functional diversity within the Bactridinae.
We found similar spatial patterns of congruence between phylogenetic and functional diversity in rainforests and seasonally dry ecosystems, contrary to our expectation (H2). However, spatial congruence between phylogenetic and functional diversity, described by MNTD, is slightly higher than that described by MPD. Humid and seasonally dry ecosystems have different, but interacting biogeographic histories that relate both to geological events, such as Andean uplift and the formation of the Amazon basin (Hoorn et al., 2010), the changes in air and ocean currents that led to inland aridification across the continent (Le Roux, 2012), and the closure of the Isthmus of Panama that led to the biological interchange between North and South America (Bacon et al., 2015). Even though historically older geological events have influenced Neotropical evolution, recent climatic and geological events have also had a crucial influence (Couvreur et al., 2011). Finally, much of the plant adaptations to dry and seasonal climates in rainforest-originating clades, such as Cocoseae, may be the result of convergent evolution of the same set of traits to deal with environmental filters such as drought and fire. Short or subterranean stems increase plant survival after seasonal fires and drought, for instance, are very common in savanna palms such as Acrocomia, Allagoptera, Butia, and especially Syagrus (Bacon et al., 2017; Noblick, 2017). The set of traits used here are widespread and have evolved several times across the plant tree of life (Simon and Pennington, 2012). A homogenization in functional strategies to cope with drought and fire (Hoffmann et al., 2012) can explain the low functional diversity in places where there is somewhat high phylogenetic diversity (i.e., South America open diagonal).
Temperature and precipitation are the main predictors of palm taxonomic diversity (Eiserhardt et al., 2011a). There is also evidence for historical and geographical factors modulating palm species distribution among ecosystems of South America (Roncal et al., 2010, 2011; Eiserhardt et al., 2011a; Freitas et al., 2016, 2019; Sanín et al., 2016). In this sense, evidence clearly points to recent episodes of species diversification into the open diagonal, including Attalea and Syagrus in the Brazilian Cerrado (Couvreur et al., 2011; Freitas et al., 2016).
Our results on the Cocoseae may reflect a general pattern for the palm family (Meerow et al., 2009, 2015; Eiserhardt et al., 2011a), and as a widespread, characteristic plant family in the Neotropics, may serve as a model to describe general patterns of plant diversity. Our results taken together show an unexpected lack of spatial congruence between the phylogenetic and functional patterns of palm diversity across space. We highlight the importance of incorporating different aspects of biodiversity to meet the biodiversity goals for the 2020–2030 agenda (Hoban et al., 2020). Species diversity is eroding quickly due to a variety of threats, and here we describe how evolutionary and functional diversity cannot be excluded from understanding the patterns of biodiversity across space. It is a goal of the Convention on Biological Diversity for 2030 to halt the loss of genetic diversity of all species, and here we provide an explicit overview on how the spatial relationships between the three facets of biodiversity are not congruent. In fact, concentrating efforts on studying large-scale patterns on taxonomic diversity on plants, biodiversity scientists are missing a wealth of information on diversification potential and ecosystem functioning.
Data Availability Statement
The original contributions presented in the study are included in the article/Supplementary Material, further inquiries can be directed to the corresponding author/s.
Author Contributions
CF and CC-S conceived the study. CC-S and LM organized the data. CF and FB analyzed the data. CF and CC-S wrote the manuscript with input from all authors.
Funding
CF received a CAPES PRINT fellowship (Coordenação de Aperfeiçoamento de Pessoal de Nível Superior do Brasil, grant # 88887.468290/2019-00). CC-S and LM were supported by São Paulo Research Foundation (FAPESP, grants #2020/09164-0 and #2020/06085-1, respectively). FB received a CAPES fellowship (finance Code 001). RC was continuously supported by productivity grants from CNPq, which we gratefully acknowledge. CB was supported by the Swedish Research Council (2017-04980).
Conflict of Interest
The authors declare that the research was conducted in the absence of any commercial or financial relationships that could be construed as a potential conflict of interest.
Publisher’s Note
All claims expressed in this article are solely those of the authors and do not necessarily represent those of their affiliated organizations, or those of the publisher, the editors and the reviewers. Any product that may be evaluated in this article, or claim that may be made by its manufacturer, is not guaranteed or endorsed by the publisher.
Acknowledgments
We thank INCT EECBio for providing the computer cluster to run spatial analyses.
Supplementary Material
The Supplementary Material for this article can be found online at: https://www.frontiersin.org/articles/10.3389/ffgc.2021.739468/full#supplementary-material
Footnotes
References
Allouche, O., Tsoar, A., and Kadmon, R. (2006). Assessing the accuracy of species distribution models: prevalence, kappa and the true skill statistic (TSS). J. Appl. Ecol. 43, 1223–1232. doi: 10.1111/j.1365-2664.2006.01214.x
Bacon, C. D., Moraes, R. M., Jaramillo, C., and Antonelli, A. (2017). Endemic palm species shed light on habitat shifts and the assembly of the Cerrado and Restinga floras. Mol. Phylogenet. Evol. 110, 127–133. doi: 10.1016/j.ympev.2017.03.013
Bacon, C. D., Silvestro, D., Jaramillo, C., Smith, B. T., Chakrabarty, P., and Antonelli, A. (2015). Biological evidence supports an early and complex emergence of the Isthmus of Panama. Proc. Natl. Acad. Sci. U.S.A. 112, 6110–6115. doi: 10.1073/pnas.1423853112
Bacon, C. D., Velásquez-Puentes, F. J., Hinojosa, L. F., Schwartz, T., Oxelman, B., Pfeil, B., et al. (2018a). Evolutionary persistence in Gunnera and the contribution of southern plant groups to the tropical Andes biodiversity hotspot. PeerJ 6:e4388. doi: 10.7717/peerj.4388
Bacon, C. D., Velásquez-Puentes, F. J., Hoorn, C., and Antonelli, A. (2018b). Iriarteeae palms tracked the uplift of Andean Cordilleras. J. Biogeogr. 45, 1653–1663. doi: 10.1111/jbi.13350
Barve, N., Barve, V., Jiménez-Valverde, A., Lira-Noriega, A., Maher, S. P., Peterson, A. T., et al. (2011). The crucial role of the accessible area in ecological niche modeling and species distribution modeling. Ecol. Modell. 222, 1810–1819. doi: 10.1016/j.ecolmodel.2011.02.011
Bjorholm, S., Svenning, J.-C., Skov, F., and Balslev, H. (2005). Environmental and spatial controls of palm (Arecaceae) species richness across the Americas. Glob. Ecol. Biogeogr. 14, 423–429. doi: 10.1111/j.1466-822x.2005.00167.x
Cámara-Leret, R., Faurby, S., Macía, M. J., Balslev, H., Göldel, B., Svenning, J.-C., et al. (2017). Fundamental species traits explain provisioning services of tropical American palms. Nat. Plants 3:16220. doi: 10.1038/nplants.2016.220
Cássia-Silva, C., Cianciaruso, M. V., Dias, P. A., Freitas, C. G., Souza-Neto, A. C., and Collevatti, R. G. (2020b). Among cradles and museums: seasonally dry forest promotes lineage exchanges between rain forest and savanna. Plant Eco. Div. 13, 1–13. doi: 10.1080/17550874.2019.1709103
Cássia-Silva, C., Freitas, C. G., Lemes, L. P., Paterno, G. B., Dias, P. A., Bacon, C. D., et al. (2020a). Higher evolutionary rates in life-history traits in insular than in mainland palms. Sci. Rep. 10:21125. doi: 10.1038/s41598-020-78267-5
Cássia-Silva, C., Freitas, C. G., Alves, D. M. C. C., Bacon, C. D., and Collevatti, R. G. (2019). Niche conservatism drives a global discrepancy in palm species richness between seasonally dry and moist habitats. Glob. Ecol. Biogeogr. 28, 814–825. doi: 10.1111/geb.12895
Cavender-Bares, J., Kitajima, K., and Bazzaz, F. A. (2004). Multiple trait associations in relation to habitat differentiation among 17 Floridian Oak species. Ecol. Monogr. 74, 635–662. doi: 10.1890/03-4007
Convention on Biological Diversity (2006). Global Biodiversity Outlook 2 Secretariat of the Convention on Biological Diversity. Montreal, QC: Convention on Biological Diversity.
Couvreur, T. L., Forest, F., and Baker, W. J. (2011). Origin and global diversification patterns of tropical rain forests: inferences from a complete genus-level phylogeny of palms. BMC Biol. 9:44. doi: 10.1186/1741-7007-9-44
de Andrade, A. F. A., Velazco, S. J. E., and De Marco Júnior, P. (2020). ENMTML: an R package for a straightforward construction of complex ecological niche models. Environ. Model. Softw. 125:104615. doi: 10.1016/j.envsoft.2019.104615
Diniz-Filho, J. A. F., Loyola, R. D., Raia, P., Mooers, A. O., and Bini, L. M. (2013). Darwinian shortfalls in biodiversity conservation. Trends Ecol. Evol. 28, 689–695. doi: 10.1016/j.tree.2013.09.003
Diniz-Filho, J. A. F., Souza, K. S., Bini, L. M., Loyola, R., Dobrovolski, R., Rodrigues, J. F. M., et al. (2019). A macroecological approach to evolutionary rescue and adaptation to climate change. Ecography 42, 1124–1141. doi: 10.1111/ecog.04264
Díaz, S., and Cabido, M. (2001). Vive la différence: plant functional diversity matters to ecosystem processes. Trends Ecol. Evol. 16, 646–655. doi: 10.1016/S0169-5347(01)02283-2
Dransfield, J., Uhl, N., Asmussen, C., Baker, W., Harley, M., and Lewis, C. E. (2008). Genera Palmarum. Kew: Royal Botanic Gardens.
Dreiss, L. M., Burgio, K. R., Cisneros, L. M., Klingbeil, B. T., Patterson, B. D., Presley, S. J., et al. (2015). Taxonomic, functional, and phylogenetic dimensions of rodent biodiversity along an extensive tropical elevational gradient. Ecography 38, 876–888. doi: 10.1111/ecog.00971
Echeverría-Londoño, S., Enquist, B. J., Neves, D. M., Violle, C., Boyle, B., Kraft, N. J., et al. (2018). Plant functional diversity and the biogeography of biomes in North and South America. Front. Ecol. Evol. 6:219. doi: 10.3389/fevo.2018.00219
Eiserhardt, W. L., Svenning, J.-C., Kissling, W. D., and Balslev, H. (2011a). Geographical ecology of the palms (Arecaceae): determinants of diversity and distributions across spatial scales. Ann. Bot. 108, 1391–1416. doi: 10.1093/aob/mcr146
Eiserhardt, W. L., Pintaud, J.-C., Asmussen-Lange, C., Hahn, W. J., Bernal, R., Balslev, H., et al. (2011b). Phylogeny and divergence times of Bactridinae (Arecaceae, Palmae) based on plastid and nuclear DNA sequences. Taxon 60, 485–498. doi: 10.1002/tax.602016
Faurby, S., Eiserhardt, W. L., Baker, W. J., and Svenning, J.-C. (2016). An all-evidence species-level supertree for the palms (Arecaceae). Mol. Phylogenet. Evol. 100, 57–69. doi: 10.1016/j.ympev.2016.03.002
Forest, F., Grenyer, R., Rouget, M., Davies, T. J., Cowling, R. M., Faith, D. P., et al. (2007). Preserving the evolutionary potential of floras in biodiversity hotspots. Nature 445, 757–760. doi: 10.1038/nature05587
Freitas, C., Meerow, A. W., Pintaud, J.-C., Henderson, A., Noblick, L., Costa, F. R. C., et al. (2016). Phylogenetic analysis of Attalea (Arecaceae): insights into the historical biogeography of a recently diversified Neotropical plant group. Bot. J. Linn. Soc. 182, 287–302. doi: 10.1111/boj.12466
Freitas, C. G., Bacon, C. D., Souza-Neto, A. C., and Collevatti, R. G. (2019). Adjacency and area explain species bioregional shifts in Neotropical palms. Front. Plant Sci. 10:55. doi: 10.3389/fpls.2019.00055
Gerhold, P., Carlucci, M. B., Proches, S., and Prinzing, A. (2018). The deep past controls the phylogenetic structure of present, local communities. Ann. Rev. Eco. Evol. Syst. 49, 477–497.
Göldel, B., Kissling, W. D., and Svenning, J.-C. (2015). Geographical variation and environmental correlates of functional trait distributions in palms (Arecaceae) across the New World. Bot. J. Linn. Soc. 179, 602–617. doi: 10.1111/boj.12349
Govaerts, R., Dransfield, J., Zona, S. F., Hodel, D. R., and Henderson, A. (2011). World Checklist of Arecaceae. Facilitated by the Royal Botanic Gardens, Kew. Available online at: http://apps.kew.org/wcsp/ (accessed February 2019).
Henderson, A. (2002). Evolution and Ecology of Palms. New York, NY: New York Botanical Garden Press.
Hengl, T., Mendes de Jesus, J., Heuvelink, G. B. M., Ruiperez Gonzalez, M., Kilibarda, M., Blagotić, A., et al. (2017). SoilGrids250m: global gridded soil information based on machine learning. PLoS One 12:e0169748. doi: 10.1371/journal.pone.0169748
Hidasi-Neto, J., Barlow, J., and Cianciaruso, M. V. (2012). Bird functional diversity and wildfires in the A mazon: the role of forest structure. Anim. Conserv. 15, 407–415. doi: 10.1111/j.1469-1795.2012.00528.x
Hijmans, R. J., and van Etten, J. (2012). raster: Geographic Analysis and Modeling with Raster Data. R package version 2.0-12.
Hill, A. P., Jiménez, M. F. T., Chazot, N., Cássia-Silva, C., Faurby, S., and Bacon, C. D. (2021). Fruit colour and range size interact to influence diversification. bioRxiv [Preprint]. doi: 10.1101/2021.10.26.465838
Hoban, S., Bruford, M., Jackson, J. D. U., Lopes-Fernandes, M., Heuertz, M., Hohenlohe, P. A., et al. (2020). Genetic diversity targets and indicators in the CBD post-2020 Global Biodiversity Framework must be improved. Biol. Conserv. 248:108654.
Hoffmann, W. A., Geiger, E. L., Gotsch, S. G., Rossatto, D. R., Silva, L. C. R., Lau, O. L., et al. (2012). Ecological thresholds at the savanna-forest boundary: how plant traits, resources and fire govern the distribution of tropical biomes. Ecol. Lett. 15, 759–768. doi: 10.1111/j.1461-0248.2012.01789.x
Hooper, D. U., Chapin, F. S., Ewel, J. J., Hector, A., Inchausti, P., Lavorel, S., et al. (2005). Effects of biodiversity on ecosystem functioning: a consensus of current knowledge. Ecol. Monogr. 75, 3–35. doi: 10.1890/04-0922
Hoorn, C., Wesselingh, F. P., ter Steege, H., Bermudez, M. A., Mora, A., Sevink, J., et al. (2010). Amazonia through time: Andean uplift, climate change, landscape evolution, and biodiversity. Science 330, 927–931. doi: 10.1126/science.1194585
Hortal, J., de Bello, F., Diniz-Filho, J. A. F., Lewinsohn, T. M., Lobo, J. M., and Ladle, R. J. (2015). Seven shortfalls that beset large-scale knowledge of biodiversity. Annu. Rev. Ecol. Evol. Syst. 46, 523–549. doi: 10.1146/annurev-ecolsys-112414-054400
Jarzyna, M. A., and Jetz, W. (2016). Detecting the multiple facets of biodiversity. Trends Ecol. Evol. 31, 527–538. doi: 10.1016/j.tree.2016.04.002
Jimenez, M. F. T., Chazot, N., Emilio, T., Fredin, J. U., Antonelli, A., Faurby, S., et al. (2021). Temperature predicts leaf shape in palms (Arecaceae). bioRxiv [Preprint]. doi: 10.1101/2021.10.26.465896
Kalogirou, S. (2017). lctools: Local Correlation, Spatial Inequalities, Geographically Weighted Regression and Other Tools. R Package Version 0.2-6.
Karger, D. N., Conrad, O., Böhner, J., Kawohl, T., Kreft, H., Soria-Auza, R. W., et al. (2017). Climatologies at high resolution for the earth’s land surface areas. Sci. Data 4:170122. doi: 10.1038/sdata.2017.122
Kembel, S. W., Cowan, P. D., Helmus, M. R., Cornwell, W. K., Morlon, H., Ackerly, D. D., et al. (2010). Picante: R tools for integrating phylogenies and ecology. Bioinformatics 26, 1463–1464. doi: 10.1093/bioinformatics/btq166
Kissling, W. D., Balslev, H., Baker, W. J., Dransfield, J., Göldel, B., Lim, J. Y., et al. (2019). PalmTraits 1.0, a species-level functional trait database of palms worldwide. Sci. Data 6:178. doi: 10.1038/s41597-019-0189-0
Kissling, W. D., Eiserhardt, W. L., Baker, W. J., Borchsenius, F., Couvreur, T. L. P., Balslev, H., et al. (2012). Cenozoic imprints on the phylogenetic structure of palm species assemblages worldwide. Proc. Natl. Acad. Sci. U.S.A. 109, 7379–7384. doi: 10.1073/pnas.1120467109
Lamoreux, J. F., Morrison, J. C., Ricketts, T. H., Olson, D. M., Dinerstein, E., McKnight, M. W., et al. (2006). Global tests of biodiversity concordance and the importance of endemism. Nature 440, 212–214. doi: 10.1038/nature04291
Larjavaara, M., and Muller-Landau, H. C. (2012). Still rethinking the value of high wood density. Am. J. Bot. 99, 165–168. doi: 10.3732/ajb.1100324
Le Roux, J. P. (2012). A review of Tertiary climate changes in southern South America and the Antarctic Peninsula. Part 1 Oceanic conditions. Sediment. Geol. 247–248, 1–20. doi: 10.1016/j.sedgeo.2011.12.014
Losos, J. B. (2008). Phylogenetic niche conservatism, phylogenetic signal and the relationship between phylogenetic relatedness and ecological similarity among species. Ecol. Lett. 11, 995–1003. doi: 10.1111/j.1461-0248.2008.01229.x
Mazel, F., Guilhaumon, F., Mouquet, N., Devictor, V., Gravel, D., Renaud, J., et al. (2014). Multifaceted diversity-area relationships reveal global hotspots of mammalian species, trait and lineage diversity. Glob. Ecol. Biogeogr. 23, 836–847. doi: 10.1111/geb.12158
Meerow, A. W., Noblick, L., Borrone, J. W., Couvreur, T. L. P., Mauro-Herrera, M., Hahn, W. J., et al. (2009). Phylogenetic analysis of seven WRKY genes across the palm subtribe Attaleinae (Arecaceae) identifies Syagrus as sister group of the coconut. PLoS One 4:e7353. doi: 10.1371/journal.pone.0007353
Meerow, A. W., Noblick, L., Salas-Leiva, D. E., Sanchez, V., Francisco-Ortega, J., Jestrow, B., et al. (2015). Phylogeny and historical biogeography of the cocosoid palms (Arecaceae, Arecoideae, Cocoseae) inferred from sequences of six WRKY gene family loci. Cladistics 31, 509–534. doi: 10.1111/cla.12100
Metcalfe, H., Milne, A. E., Deledalle, F., and Storkey, J. (2020). Using functional traits to model annual plant community dynamics. Ecology 101:e03167. doi: 10.1002/ecy.3167
Noblick, L. R. (2017). A revision of the genus Syagrus (Arecaceae). Phytotaxa 294:1. doi: 10.11646/phytotaxa.294.1.1
Olson, D. M., Dinerstein, E., Wikramanayake, E. D., Burgess, N. D., Powell, G. V. N., Underwood, E. C., et al. (2001). Terrestrial ecoregions of the world: a new map of life on earth. Bioscience 51, 933–938.
Onstein, R. E., Vink, D. N., Veen, J., Barratt, C. D., Flantua, S. G. A., Wich, S. A., et al. (2020). Palm fruit colours are linked to the broad-scale distribution and diversification of primate colour vision systems. Proc. R. Soc. B Biol. Sci. 287:20192731. doi: 10.1098/rspb.2019.2731
Palmweb (2020). Palmweb: Palms of the World Online. Available online at: http://www.palmweb.org (accessed December 20, 2020).
Pavoine, S., Vallet, J., Dufour, A.-B., Gachet, S., and Daniel, H. (2009). On the challenge of treating various types of variables: application for improving the measurement of functional diversity. Oikos 118, 391–402. doi: 10.1111/j.1600-0706.2008.16668.x
Pennington, R. T., Lavin, M., Prado, D. E., Pendry, C. A., Pell, S. K., and Butterworth, C. A. (2004). Historical climate change and speciation: neotropical seasonally dry forest plants show patterns of both tertiary and quaternary diversification. Philos. Trans. R. Soc. Lond. Ser. B Biol. Sci. 359, 515–538. doi: 10.1098/rstb.2003.1435
Pérez-Harguindeguy, N., Díaz, S., Garnier, E., Lavorel, S., Poorter, H., Jaureguiberry, P., et al. (2013). New handbook for standardised measurement of plant functional traits worldwide. Aust. J. Bot. 61, 167–234. doi: 10.1071/BT12225
Petchey, O. L., and Gaston, K. J. (2002). Functional diversity (FD), species richness and community composition. Ecol. Lett. 5, 402–411. doi: 10.1046/j.1461-0248.2002.00339.x
Phillips, O. L., Vásquez Martínez, R., Arroyo, L., Baker, T. R., Killeen, T., Lewis, S. L., et al. (2002). Increasing dominance of large lianas in Amazonian forests. Nature 418, 770–774. doi: 10.1038/nature00926
Prado, D. E., and Gibbs, P. E. (1993). Patterns of species distributions in the dry seasonal forests of South America. Ann. Miss. Bot. Gar. 80, 902–927.
Purvis, A., Gittleman, J. L., Cowlishaw, G., and Mace, G. M. (2000). Predicting extinction risk in declining species. Proc. R. Soc. Lond. Ser. B Biol. Sci. 267, 1947–1952. doi: 10.1098/rspb.2000.1234
R Core Team (2020). R: A Language and Environment for Statistical Computing. Vienna: R Foundation for Statistical Computing.
Roncal, J., Blach-Overgaard, A., Borchsenius, F., Balslev, H., and Svenning, J. C. (2011). A dated phylogeny complements macroecological analysis to explain the diversity patterns in Geonoma (Arecaceae). Biotropica 43, 324–334.
Roncal, J., Borchsenius, F., Asmussen-Lange, C. B., Balslev, H., Seberg, O., and Petersen, G. (2010). “Divergence times in the tribe Geonomateae (Arecaceae) coincide with tertiary geological events,” in Diversity, Phylogeny and Evolution of Monocotyledons, eds O. Seberg, G. Petersen, A. S. Barfod, and J. I. Davis (Aarhus: Aarhus University Press).
Roncal, J., Kahn, F., Millan, B., Couvreur, T. L., and Pintaud, J. C. (2013). Cenozoic colonization and diversification patterns of tropical American palms: evidence from Astrocaryum (Arecaceae). Bot. J. Linn. Soc. 171, 120–139.
Safi, K., Cianciaruso, M. V., Loyola, R. D., Brito, D., Armour-Marshall, K., and Diniz-Filho, J. A. F. (2011). Understanding global patterns of mammalian functional and phylogenetic diversity. Philos. Trans. R. Soc. Lond. B. Biol. Sci. 366, 2536–2544. doi: 10.1098/rstb.2011.0024
Sanín, M. J., Kissling, W. D., Bacon, C. D., Borchsenius, F., Galeano, G., Svenning, J. C., et al. (2016). The Neogene rise of the tropical Andes facilitated diversification of wax palms (Ceroxylon: Arecaceae) through geographical colonization and climatic niche separation. Bot. J. Linn. Soc. 182, 303–317. doi: 10.1111/boj.12419
Simon, M. F., and Pennington, T. (2012). Evidence for adaptation to fire regimes in the Tropical savannas of the Brazilian Cerrado. Int. J. Plant Sci. 173, 711–723. doi: 10.1086/665973
Sobral, F. L., Jardim, L., Lemes, P., Machado, N., Loyola, R., and Cianciaruso, M. V. (2014). Spatial conservation priorities for top predators reveal mismatches among taxonomic, phylogenetic and functional diversity. Nat. Conserv. 12, 150–155. doi: 10.1016/j.ncon.2014.09.008
Strecker, A. L., Olden, J. D., Whittier, J. B., and Paukert, C. P. (2011). Defining conservation priorities for freshwater fishes according to taxonomic, functional, and phylogenetic diversity. Ecol. Appl. 21, 3002–3013. doi: 10.1890/11-0599.1
Svenning, J.-C., Borchsenius, F., Bjorholm, S., and Balslev, H. (2008). High tropical net diversification drives the New World latitudinal gradient in palm (Arecaceae) species richness. J. Biogeogr. 35, 394–406. doi: 10.1111/j.1365-2699.2007.01841.x
Swenson, N. G. (2011). The role of evolutionary processes in producing biodiversity patterns, and the interrelationships between taxonomic, functional and phylogenetic biodiversity. Am. J. Bot. 98, 472–480. doi: 10.3732/ajb.1000289
Terborgh, J. (1986). “Keystone plant resources in the tropical forest,” in Conservation Biology, eds I. Soulé and E. Michael (Sunderland, MA: Sinauer Associates), 330–344.
The Global Biodiversity Information Facility (2019). GBIF.org. GBIF Occurrence Download. Available online at: https://doi.org/10.15468/dl.rjmqfy (accessed January 31, 2019).
Velazco, S. J. E., Svenning, J., Ribeiro, B. R., and Laureto, L. M. O. (2021). On opportunities and threats to conserve the phylogenetic diversity of Neotropical palms. Divers. Distrib. 27, 512–523. doi: 10.1111/ddi.13215
Violle, C., Navas, M. L., Vile, D., Kazakou, E., Fortunel, C., Hummel, I., et al. (2007). Let the concept of trait be functional! Oikos 116, 882–892.
Webb, C. O. (2000). Exploring the phylogenetic structure of ecological communities: an example for rain forest trees. Am. Nat. 156, 145–155. doi: 10.1086/303378
Westoby, M. (1998). A leaf-height-seed (LHS) plant ecology strategy scheme. Plant Soil 199, 213–227. doi: 10.1023/A:1004327224729
Keywords: Cocoseae, macroecology, Macroevolution, ecological niche modeling, spatial analysis
Citation: Freitas C, Brum FT, Cássia-Silva C, Maracahipes L, Carlucci MB, Collevatti RG and Bacon CD (2021) Incongruent Spatial Distribution of Taxonomic, Phylogenetic, and Functional Diversity in Neotropical Cocosoid Palms. Front. For. Glob. Change 4:739468. doi: 10.3389/ffgc.2021.739468
Received: 11 July 2021; Accepted: 03 December 2021;
Published: 23 December 2021.
Edited by:
Michael David Pirie, University Museum of Bergen, NorwayReviewed by:
William J. Baker, Royal Botanic Gardens, Kew, United KingdomThomas L. P. Couvreur, IRD UMR 232 Diversité, Adaptation, Développement des Plantes (DIADE), France
Copyright © 2021 Freitas, Brum, Cássia-Silva, Maracahipes, Carlucci, Collevatti and Bacon. This is an open-access article distributed under the terms of the Creative Commons Attribution License (CC BY). The use, distribution or reproduction in other forums is permitted, provided the original author(s) and the copyright owner(s) are credited and that the original publication in this journal is cited, in accordance with accepted academic practice. No use, distribution or reproduction is permitted which does not comply with these terms.
*Correspondence: Cintia Freitas, Y2ludHVjYUBnbWFpbC5jb20=