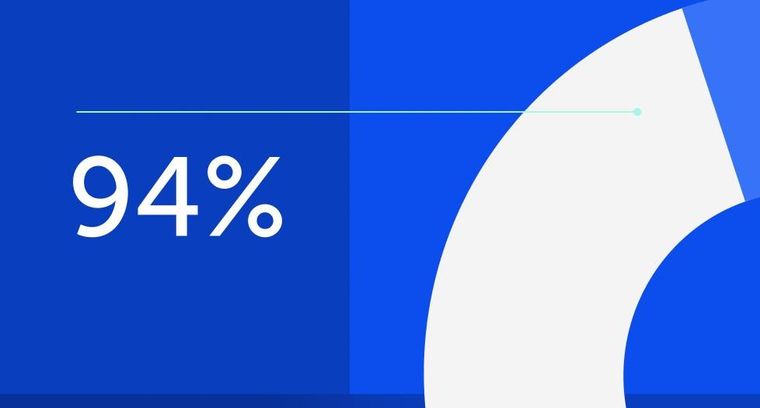
94% of researchers rate our articles as excellent or good
Learn more about the work of our research integrity team to safeguard the quality of each article we publish.
Find out more
ORIGINAL RESEARCH article
Front. For. Glob. Change, 27 September 2021
Sec. Forests and the Atmosphere
Volume 4 - 2021 | https://doi.org/10.3389/ffgc.2021.738303
This article is part of the Research TopicGreenhouse Gas Fluxes in Forest EcosystemsView all 5 articles
Riparian forests are often kept as buffers between rivers and oil palm plantations. Many benefits of riparian forests, such as increasing biodiversity and providing a travel corridor for wildlife have been documented. Conversely, data on fluxes of the greenhouse gases nitrous oxide (N2O) and methane (CH4) from riparian forests are sparse. Nitrogen (N) from fertilizer applied in the oil palm plantations leached to the adjacent riparian forests, may increase emissions of N2O. Methane (CH4) fluxes might also differ between oil palm plantations and riparian forests due to carbon (C) availability. In this scoping study, we installed transects from three mature oil palm plantations to adjacent riparian forests within the SAFE project landscape in Sabah, Malaysia (https://www.safeproject.net) for measurements of greenhouse gases and associated parameters every 2 months for 13 months. Emissions of N2O were higher from riparian forests with 40.4 [95% confidence intervals (CI): 35.7–44.6] μg N2O-N m–2 h–1 than from an equivalent area of oil palm plantation 27.6 (CI: 23.1–32.3) μg N2O-N m–2 h–1. Methane uptake was significantly higher from the riparian forest with −14.7 (CI: −21.1 to −8.3) μg CH4-C m–2 h–1 compared to slight positive emission in the oil palm plantations of 6.3 (CI: 1.1–11.4) μg CH4-C m–2 h–1. We are contributing urgently needed flux data for less well studied riparian forests in the Tropics, however, additional long-term studies are needed to be able to draw wider conclusions than possible from this scoping study alone.
Riparian buffers in agricultural landscapes exist in the transition area between agricultural and freshwater habitats (Cole et al., 2020). Riparian buffers, including riparian forests, are habitats with critical ecological and conservation value as they assemble diverse and unique species, contribute to regional species diversity (Sabo et al., 2005), act as dispersal corridors for flora and fauna (Knopf and Samson, 1994; Nilsson and Svedmark, 2002), and mediate exchanges of matter and energy between terrestrial and aquatic environments (Osborne and Kovacic, 1993). In tropical Southeast Asia, riparian forests are recognized as important for biodiversity conservation and for the reduction of pollutant transfer from terrestrial to aquatic habitats, particularly in areas dominated by oil palm cultivation (Luke et al., 2019). Unlike in intensively managed agricultural land in temperate regions, where riparian buffers are often retrospectively established, in the oil palm landscape riparian buffers are commonly remnants of tropical forests, consequently riparian forests. Legislation and policy seek to protect natural vegetation, such as riparian forests, in order to provide refuges for forest species and habitat connectivity among forest fragments. However, the effectiveness of current policy and practice in Southeast Asia is still in question (Luke et al., 2019).
It has been predicted that riparian buffers can be potential hotspots for greenhouse gas fluxes to the atmosphere (Hedin et al., 1998) but actual data are lacking. An essential ecological service provided by riparian buffers is the mitigation of nutrient pollution from surrounding agricultural areas (Kachenchart et al., 2012). The management is imperative of controlling nitrogen (N) loading to waterbodies and protecting or restoring ecosystems surrounding these waterbodies to maintain or enhance their N removal functions (Mulholland et al., 2008). Runoff and leaching of fertilizer from surrounding oil palm plantations are expected to raise concentrations of dissolved macro and micronutrients and promote nitrophilic plant species in riparian soils (Nagy et al., 2015). Increased concentrations of inorganic N in riparian forests are of particular concern, as enhanced N availability can increase emissions of nitrous oxide (N2O), a greenhouse gas with a global warming potential 298 times that of carbon dioxide (Myhre et al., 2013). The main processes leading to N2O emissions are nitrification and denitrification (Davidson et al., 2000) with main controlling factors being soil N availability, water content, bulk density, and temperature (Verchot et al., 2006). Nitrification, is an oxidative process, converting ammonium (NH4+) to nitrate (NO3–), with N2O production under O2 limiting conditions. Denitrification, the reduction of NO3– to the end products N2O and N2, requires anaerobic conditions as often found in wet and waterlogged soils (Butterbach-Bahl et al., 2013). The ability of riparian buffers to remove mineral and organic N compounds from runoff through denitrification is predominantly controlled by soil chemical and physical properties and the microbiological community compositions (Ma et al., 2020). The magnitude of indirect N2O emissions from fertilizer leaching into the riparian forests compared to direct N2O emissions from oil palm plantations receiving high rates of N fertilizers is currently unknown, as not many robust studies have been reported. However, there are first indications that due to nutrient translocation into riparian forests, greenhouse gases such as N2O can be emitted from these areas, often in quantities comparable to those from adjacent agricultural plantations themselves (Drewer et al., 2020, 2021). It is important to determine if riparian forest buffers are sinks or sources of N2O and their magnitude in relation to OP plantations (Mafa-Attoye et al., 2020).
Land-use change has been identified as driver for changes in soil and biomass carbon (C) and greenhouse gas emissions (Kachenchart et al., 2012; Brauman et al., 2015; Nagy et al., 2015). There is a severe lack of data, especially longer-term studies, on C storage, N2O and CH4 emissions from riparian forests to fully assess and quantify their potential benefits (Luke et al., 2019). Methane (CH4) is a potent greenhouse gas with a global warming potential 28 times higher than CO2 on a 100-year time horizon (Myhre et al., 2013). Methane is emitted or taken up by the soil depending on the balance between methanotrophy and methanogenesis. The latter is favored by anaerobic conditions and is the anaerobic microbial decomposition of organic material, which occurs in wet and organic rich soils; methanotrophy takes place in parts of the soil where oxygen is available (Dutaur and Verchot, 2007). For example, recent studies have suggested that CH4 uptake in oil palm and rubber plantation in Indonesia might be higher in riparian forests than plantations (Hassler et al., 2015; Lang et al., 2020).
The objective of this scoping study was to carry out preliminary field measurements of N2O and CH4 as well as basic soil physicochemical parameters in transects from oil palm plantations to riparian forests in Malaysian Borneo, Sabah. Here we provide much needed data to address the question whether riparian forests or oil palm plantations have higher N2O and CH4 emissions.
Three transects from oil palm plantations (OP) via riparian reserves (RR), in this case riparian forests, to the rivers were set up within the SAFE landscape (Stability of Altered Forest Ecosystems1) in Malaysian Borneo, Sabah (Ewers et al., 2011). To be in line with other SAFE publications we use the nomenclature RR (riparian reserve) for the riparian forests. The study area has a wet tropical climate with a wet season typically from October to February and a dry season typically from March to September (Drewer et al., 2021) with average monthly temperatures of 32.5°C (irrespective of season) and average monthly rainfall of 164.1 mm2. The soils are classed as orthic Acrisols or Ultisols (Riutta et al., 2018).
Transects 1 and 2 were installed on a 53 ha oil palm plantation established in 2007. Transect 1 consisted of an OP plantation draining into a very steep ∼40 m wide shady riparian mature forest. Transect 2, less than 500 m from Transect 1, drains into a flat riparian reserve and then into a small shallow tributary stream. The riparian reserve is vegetated mainly with the legume Mucuna bracteata, a typical cover crop in OP and rubber plantations, and planted with soft wood trees. Transect 3 was set up in a 32 ha OP plantation established in 2011 (Figure 1). The OP plantation drained through a 20 m wide Mucuna bracteata area into a mature riparian forest of 64 m width, which was slightly less steep compared to the riparian forest of Transect 1. Transect 1 and 3 forests are relatively good quality logged forests with high biodiversity, akin to logged forests within the rest of the SAFE landscape. All three riparian reserves drain into the same river, albeit for Transect 2 via the small stream.
Figure 1. Layout of the transects from oil palm plantation via riparian forest to the river, using transect 3 as an example. Chambers (CHB) 31–36 are in the oil palm plantation. Chambers 37–48 are in the riparian forest. There are six rows of three chambers each across the transect.
The oil palm plantations are terraced and almost devoid of ground vegetation, due to near canopy closure, and a planting density of 120 palms ha–1. Fertilizer management was similar at both Estates and typical for this region. A composite fertilizer of N, P, K, Magnesium and Boron is broadcast around the palm stem twice per year, at a rate of 4 kg N per palm. Our sampling frequency did not allow to investigate individual fertilizer events. The objective of this study was to capture general differences of GHG fluxes between OP plantations and RR. It has previously been reported that effects of N fertilizer application in an oil palm plantation lasted only a few days and were unlikely to have significant consequences for monthly means or annual budgets (Comeau et al., 2016). Due to difficult logistics and accessibility of the sites as well as financial restraints, we were only able to carry out field measurements every 2 months.
The static chamber method was used for greenhouse gas flux measurements as previously described in Drewer et al. (2021). Chambers were installed in triplicate at each transect point (see example in Figure 1); two rows of three (total six) in each OP plantation, followed by four rows of three (total 12) for transect 1 and 3, and two rows of three (6) for transect 2 (less wide buffer compared to the other two) in the riparian forests. In total there were 48 chambers; two transects with 18 and one with 12 chambers. For exact GPS locations see published dataset (Drewer et al., 2019).
The static chamber method was used for N2O and CH4 flux measurements as described previously (Drewer et al., 2017a,b). Round static chambers (diameter = 40 cm) consisting of opaque polypropylene bases of 10 cm height were inserted into the ground to a depth of approximately 5–10 cm for the entire 13 months study period (November 2016 to November 2017). Lids of 25 cm height were fastened onto the bases using four strong clips, only during the 45-min measurement periods. A strip of commercially available draft excluder glued onto the flange of the lid provided a gas tight seal between chamber and lid. The lids were fitted with a pressure compensation plug to maintain ambient pressure in the chambers during and after sample removal. Gas samples were taken at regular intervals (0, 15, 30, 45 min) from each chamber. A three-way tap was used for gas sample removal using a 100 ml syringe. 20 ml glass vials were filled with a double needle system to flush the vials with five times their volume and remained at ambient pressure (Drewer et al., 2017a, 2021). The sample vials were sent to UKCEH Edinburgh for analysis usually between 4 and 7 weeks after sampling. A specifically conducted storage test confirmed no significant loss of concentration during this time period. Samples and three sets of four certified standard concentrations (N2O, CH4 in N2 with 20% O2) were analyzed using a gas chromatograph (Agilent GC7890B with headspace autosampler 7697A; Agilent, Santa Clara, CA, United States) with micro electron capture detector (μECD) for N2O analysis and flame ionization detector (FID) for CH4 analysis. These detectors were setup in parallel allowing the analysis of the two GHGs at the same time. Limit of detection was 5 ppb for N2O and 40 ppb for CH4 (Drewer et al., 2020). Peak integration was carried out with OpenLab© Software Suite (Agilent, Santa Clara, CA, United States).
The flux F (μg m–2 s–1) for each sequence of gas samples from the different chambers was calculated according to Equation 1:
Where dC/dt is the concentration (C, μmol mol–1) change over time (t, in s), which was calculated by linear regression, ρV/A is the number of molecules in the enclosure volume to ground surface ratio, where ρ is the density of air (mol m–3), V (m3) is the air volume in the chamber and A (m2) is the surface area in the chamber (Levy et al., 2012). Fluxes were checked for linearity and all fluxes presented here had the best fit with linear interpolation. Applying the analytical limit of detection to the flux calculation, the resulting detection limits and therefore uncertainties associated with the flux measurements are 1.6 μg N m–2 h–1 for N2O and 5 μg C m–2 h–1 for CH4 in the units used in the “Results” section as described in Drewer et al. (2021).
Other environmental parameters were measured during time of chamber enclosure as possible explanatory variables for correlation with recorded GHG fluxes. Soil and air temperatures were measured using a handheld Omega HH370 temperature probe (Omega Engineering United Kingdom Ltd., Manchester, United Kingdom) at each chamber location at a soil depth of 10 cm and by holding the temperature sensor 30 cm above the soil surface at chamber height. Volumetric soil moisture content (VMC) was measured at a depth of 7 cm with a portable probe (Hydrosense 2; Campbell Scientific, Loughborough, United Kingdom). For determining KCl-extractable soil nitrogen (N) in the field, soil samples were collected to a depth of 10 cm around each of the chamber locations on each of the chamber measurement days, using a gouge auger. Soil KCl extractions were carried out in the field laboratory on the same day. Soil samples were mixed well, stones were removed, and subsamples of ca. 6 g soil (fresh weight) were transferred into 50 ml falcon tubes containing 25 ml 1 M KCl solution. The samples were shaken for 1 min every 15 min for 1 h, then filtered through Whatman 42© filter paper (GE Healthcare, Chicago, IL, United States) and kept in the fridge after addition of one drop of 75% H2SO4 as a preservative. Analysis of ammonium (NH4+) and nitrate (NO3–) concentrations from KCl-extracted soils were carried out at the Forest Research Center in Sandakan (Sabah, Malaysia) using a colorimetric method (Astoria 2 Analyzer, Astoria-Pacific Inc., United States).
The following parameters were measured once on the last sampling occasion. Soil samples for total C and total N were taken from the top 0–10 cm inside the chambers. The samples were air dried in the field laboratory and a subsample of each was dried at 105°C to constant weight in the laboratory to convert the results to oven-dry weight, ground and analyzed at the Forest Research Center in Sandakan on an elemental analyser [Vario Max CN Elemental Analyzer (Elementar Analysensysteme, Germany)]. Leaf litter was collected from the surface in each chamber (0.1257 m2), dried at 70°C and analyzed for total C and N as described above. For pH measurements 10 g of fresh soil was mixed with deionized H2O (ratio 1:2), and after 1 h analyzed on a MP 220 pH meter (Mettler Toledo GmbH, Schwerzenbach, Switzerland).
Mean and 95 percentile confidence intervals (C.I.s) reported in this study used the arithmetic means (normal distribution) for all variables, with the exception of N2O flux for which we used the Bayesian method as described below. When estimating confidence intervals on mean values in this study, the upper and lower C.I.s were calculated by multiplying the standard error by 1.96. Where 95 percentile confidence intervals do not overlap, comparisons of mean values between two variables are deemed significantly different (Di Stefano, 2004).
Environmental data, such as soil N2O fluxes, are often highly temporally and spatially variable, making their analysis challenging. Typically, they are strongly left skewed (containing a high number of very small fluxes), and approximate a log-normal distribution. This makes trying to detect effects of land-use very difficult. When variability is high and the sample size is small, as is generally the case with flux measurements, calculation of a confidence interval on the mean of a log-normal distribution is challenging. Hence, we applied a Bayesian methodology to address this problem, using a model similar to that described by Levy et al. (2017) and used in Drewer et al. (2021). Expressed in the current statistical terminology, this is a generalized linear mixed-effect model (GLMM) with a log-normal response and identity link function. The model consists of a fixed effect of land-use [oil palm (OP) vs. riparian forest (RR)], with a random effect representing the variation among sites within a land-use type. The parameters were estimated by the Markov chain Monte Carlo (MCMC) method, using Gibbs sampling as implemented in Just Another Gibbs Sampler (JAGS), described in more detail by Levy et al. (2017). This model is suitable for small samples sizes irrespective of numbers of samples per treatment (site/land-use). All statistical analyses were conducted using the R software package, version 3.4.3 (R Core Team, 2017) using the lme4 package for linear mixed-effects models (Bates et al., 2015).
We compared soil parameters and GHG fluxes from each of the three oil palm-riparian forest transects, as well as the land-use level [oil palm (OP) vs. riparian forest (RR)], in order to establish which land-use has the higher N2O (and CH4) emission rates. In this scoping study we collected a total of 126 individual flux measurements from OP and 210 from RR between November 2016 and November 2017.
Air and soil temperatures were constant at all sites throughout the year and seasons and averaged 28.4 ± 0.1°C and 26.7 ± 0.1°C (± standard error), respectively. There was no temporal or distinct spatial trend for soil moisture (Figure 2). Transect 2, with the shallower slope, had the least variable soil moisture content for both OP and RR, broadly between 30 and 40% throughout the study period.
Figure 2. Monthly soil temperature (left) and volumetric soil moisture content (right) from the three transects (1–3), OP, oil palm (red); RR, riparian forest (blue). The lower and upper hinges correspond to the first and third quartiles (the 25th and 75th percentiles), lower whisker = smallest observation greater than or equal to lower hinge –1.5 × IQR (inter quartile range), upper whisker = largest observation less than or equal to upper hinge +1.5 × IQR. The median is marked by a horizontal line inside the box.
Soil pH was slightly higher in the riparian forests compared to the oil palm plantations in all three transects, albeit with a high spatial variability as indicated by SD and 95% C.I. and hence no significant differences (Table 1). Transect 2 had a slightly higher mean pH of 5.1 for OP and 5.3 for RR compared with pH values of around 4.2–4.4 in the OP plantations and pH 4.6–4.7 in the RR in transects 1 and 3, respectively. Total soil C and N (%) contents were higher from RR than OP in all three transects, as was the amount of litter present and total C and N contents in the litter (Table 1). However, the difference in soil C between OP and RR was only significant (no overlap between C.I.) in transect 3, whilst the differences between land-uses for soil N were only significant for transects 2 and 3. Differences in C and N contents in both soil and litter were comparable between transects. Smallest amounts of litter (in dry weight) inside the chambers were in the OP plantations of transects 1 and 3, whereas highest amounts were measured in the riparian forests which was a significant difference in both transects. Transect 2 was an exception, in that the difference in the amount of litter between OP and RR was not that large and not significant but the litter C and N contents (%) were significantly larger in RR2 than OP2 (Table 1). Although litter samples were only taken once at the end of the measurement campaign, they were representative of the entire measurement period as confirmed by visual inspection on each sampling occasion.
Table 1. Soil and litter physicochemical parameters by transect (1–3) and land-use (OP, oil palm; RR, riparian forest) from measurements at the last sampling occasion in November 2017 from soil and litter inside the chambers (surface area 0.1257 m2).
Mineral N (NH4+ and NO3–) concentrations were highly spatially variable, with no clear temporal trends but occasional hotspots in both OP and RR (Figure 3). Most notable were very high NH4+-N concentrations in OP transect 3, however, with a very high spatial variability within and between the six chambers and two rows. These concentrations of 100–300 mg N kg–1 dry soil (Figure 3) were 100 times larger in March 2017 than at the other two transects on the same measurement occasion. Some hotspots for NO3– occurred for both OP and RR in all transects and were around 10 mg N kg–1 dry soil (Figure 3).
Figure 3. Monthly soil NH4-N (left) and NO3-N (right) (mg kg–1) concentrations from the three transects (1–3), OP, oil palm (red); RR, riparian forest (blue). The lower and upper hinges correspond to the first and third quartiles (the 25th and 75th percentiles), lower whisker = smallest observation greater than or equal to lower hinge –1.5 × IQR (inter quartile range), upper whisker = largest observation less than or equal to upper hinge +1.5 × IQR. The median is marked by a horizontal line inside the box. Note different y-axis scale for NH4-N (left) for the three different transects.
N2O fluxes were very variable with no clear temporal trend across all three transects (Figure 4). Hotspots were observed for both, OP and RR, most notably for RR in transect 1. Peak fluxes exceeded 100 μg m–2 h–1 N2O-N, with the majority of fluxes being within a few tens of μg m–2 h–1 N2O-N. There were no significant correlations between N2O-N and mineral N or other measured parameters. Mean N2O fluxes over the entire measurement period were only significantly different (larger from RR than OP with no overlap of the C.I.) for transect 1 (Table 2). The distribution of all measured N2O fluxes was log-normal. To answer our main research question “which land-use emitted higher N2O fluxes,” we therefore applied a GLMM as described in section “Data Analysis” to all available data (Levy et al., 2017; Drewer et al., 2021). The posterior probability density confirmed that N2O fluxes were indeed overall higher from RR than OP (Figure 5). By fitting the GLMM to the data, we estimated mean N2O fluxes to be 27.6 (95% C.I.: 23.1–32.3) μg N m–2 h–1 for OP and 40.0 (35.7–44.6) μg N m–2 h–1 for RR, respectively (Figure 5 and Table 2).
Figure 4. Monthly N2O-N fluxes (μg m–2 h–1) from the three transects (1–3), OP, oil palm (red); RR, riparian forest (blue). The lower and upper hinges correspond to the first and third quartiles (the 25th and 75th percentiles), lower whisker = smallest observation greater than or equal to lower hinge –1.5 × IQR (inter quartile range), upper whisker = largest observation less than or equal to upper hinge +1.5 × IQR. The median is marked by a horizontal line inside the box.
Table 2. Mean nitrous oxide (N2O) and methane (CH4) fluxes over the 13 month measurement period by transect (1–3) and land-use (OP, oil palm; RR, riparian forest).
Figure 5. Posterior probability density of the mean nitrous oxide flux from each land-use (oil palm, riparian forest), estimated by the Bayesian generalized linear mixed-effect model (GLMM).
Methane fluxes were normally distributed and fluctuated around zero in all transects (Figure 6). Highest positive fluxes were measured in transect 1 for both OP and RR and transect 2 in RR and exceeded 100 μg m–2 h–1 CH4-C. The highest net uptake (a.k.a. negative flux) was measured in the lowest transect point of transect 3 and exceeded −100 μg m–2 h–1 CH4-C. Results show that both oil palm plantations and riparian reserves can be net sinks as well as net sources of CH4. Due to CH4 being normally distributed and comprising negative fluxes, the posterior probability density as shown for N2O could not be calculated. Over the entire measurement period CH4 fluxes were significantly higher from OP than RR for transect 1 and 3 (Table 2) with no overlap of the C.I. Mean CH4 fluxes by land-use were 6.3 (95 % C.I.: 1.1–11.4) μg C m–2 h–1 and −14.7 (−21.1–8.3) μg C m–2 h–1 for OP and RR, respectively (Table 2), and implies that on average OP were a source and RR a sink of CH4.
Figure 6. Monthly CH4-C fluxes (μg m–2 h–1) from the three transects (1–3), OP, oil palm (red); RR, riparian forest (blue). The lower and upper hinges correspond to the first and third quartiles (the 25th and 75th percentiles), lower whisker = smallest observation greater than or equal to lower hinge –1.5 × IQR (inter quartile range), upper whisker = largest observation less than or equal to upper hinge +1.5 × IQR. The median is marked by a horizontal line inside the box.
All three oil palm/riparian forest transects were within one km2 of each other and received the same management; yet, we saw differences in N2O fluxes across the three transects. This highlights the inherent high spatial variability of soil N2O fluxes, as described many times in the literature (Davidson et al., 2000; Reay et al., 2012; Cowan et al., 2015, 2020; Drewer et al., 2021). The complexity of these two investigated ecosystems, oil palm plantations (OP) and riparian forests (RR) is very high, demonstrated by very high variability within sites as well as between sites. The Bayesian GLMM approach allowed us to deal with the nature of the approximately log-normal distributed fluxes to compare the land-uses. Looking at the three individual transects, there was only a significant difference between RR and OP for transect 1 (Table 2). However, by pooling all N2O data for each land-use, we were able to determine a difference between the different land-uses, namely that N2O emissions were higher from the riparian reserves [40.4 (95% CI: 35.7–44.6) μg N m–2 h–1] compared to the OP plantations [27.6 (23.1–32.3) μg N m–2 h–1] on a per area basis. The individual hotspots observed for N2O (and also CH4) could not be explained by any of the measured parameters. To overcome the lack of correlations between fluxes and their drivers, we calculated the posterior probability density (Figure 5). Considering temporal and spatial variability in our small dataset (126 individual measurement for OP and 210 for riparian forest), no clear trend in GHG fluxes or soil parameters could be established. This scoping study has demonstrated, that in order to better characterize N2O and CH4 emissions from different land-uses a much larger network of sites is required to accommodate the inherently large spatial and temporal variabilities. Due to logistical restraints, as mentioned above, we could only sample every 2 months. We never intended to capture individual fertilizer events, which only occurred twice per year. It is likely that all sampling events occurred outside of the fertilizer events, as we did not observe substantial increases in N2O flux rates. This resonates with a previous study of soil respiration rates from N fertilized peatlands in Sumatra. The authors reported that individual fertilizer events do not necessarily have a significant impact on mean monthly or annual fluxes (Comeau et al., 2016). Hotspots of N2O and CH4 as well as NH4 and NO3 were occasionally measured in both OP and RR (Figures 3, 4), but no clear temporal trends or clear correlations with other measured variables could be established for all three transects using conventional statistical data analysis.
A previous study in the SAFE region, carried out 2 years before prior to this present study, focused on differences between logged forests and OP plantations, and likewise noted highly variable fluxes as well as hotspots of N2O and CH4 (Drewer et al., 2021). Mean N2O and CH4 fluxes from the earlier study were slightly higher than in this present one, albeit with a similar large range.
We cannot be certain of the main drivers controlling N2O fluxes in the riparian forests, in our study, however, the significantly higher amounts of leaf litter in the riparian forests compared to the oil palm plantations (Table 1) contributed to continuous C and N supply to the forest floor and thereby stimulating microbial decomposition (Kerdraon et al., 2020). Typical oil palm plantations are relatively devoid of litter input, apart from old palm fronds piled up usually in rows. Consequently, litter amount and total soil C and N concentrations in the riparian reserves were significantly larger than in the oil palm plantations (Table 1), promoting organic matter production, and enhancing microbial activity, such as mineralization which provides the substrates for nitrification and denitrification and consequential N2O emissions (Butterbach-Bahl et al., 2013). As reported by Hefting et al. (2005), riparian reserves can provide ideal conditions for microbial denitrification, producing N2O if NO3– is in abundance, as was the case in our studied riparian forests.
As previously reported by Drewer et al. (2021) from measurements in the same area prior to this study, CH4 can be a net sink as well as a net source, from both, forests and oil palm plantations. Differences across the three land uses were not significantly different. In contrast, there was a significant difference in CH4 fluxes with uptake in the riparian forests and small emissions in the oil palm plantations in this present study. Elsewhere, reduction in soil CH4 uptake in oil palm and rubber plantations (ranging from −3.0 to −14.9 μg C m–2 h–1) compared to lowland forest in Indonesia (ranging from −20.8 to −40.3 μg C m–2 h–1) has been shown to be due to a decrease in soil N availability in the plantations (Hassler et al., 2015). This could not directly be confirmed in our study. Similarly, CH4 consumption at 0–5 cm soil depth was also significantly greater in natural forests than in rubber plantations on tropical soil in China, with a mean CH4 flux of −23.8 ± 1.0 and −14.4 ± 1.0 μg C m–2 h–1, respectively (Lang et al., 2020) indicating the same difference between agricultural plantation and (semi) natural forest as seen in our study.
Results from a comprehensive analysis of a large number of studies (53,976 manual static chamber measurements from 27 different sites) from agricultural mineral soil in temperate regions concluded that agricultural soils can be small sinks of CH4 (Cowan et al., 2021). We therefore conclude that in order to make a comparable and more robust assessment of CH4 fluxes a much larger network of sites is required. This applies also to other GHGs and climate zones.
The results of our scoping study support Reay et al. (2012) in their debate, that N2O emissions from nitrogen-rich semi-natural environments, as the riparian forest in our study, can contribute more to global N2O emissions than from directly N fertilized agricultural soils. N2O emissions from riparian forest should be regarded as indirect emissions and need to be included in the impact assessment of N fertilized plantations. Indirect emissions (from not directly fertilized land) currently account for a disproportionate share (approximately two thirds) of the uncertainty in the estimated N2O emission factors (IPCC, 2000), hence there is a need for data to address and potentially reduce these uncertainties.
Using Bayesian statistics (posterior probability density) on the dataset from our scoping study confirmed that N2O emissions from riparian forests in Malaysian Borneo can be higher than from adjacent oil palm plantations despite of no direct N fertilization. This indicates the need for further studies to investigate GHG flux rates from riparian forests and potential drivers. Methane uptake rates were significantly larger from riparian forests than oil palm plantations. These findings need to be explored in more detail to confirm whether this might be a general trend. In any case, benefits of riparian forests such as increasing biodiversity, providing travel corridors for wildlife and other ecosystems services have to be taken into account for a complete environmental assessment of the effectiveness of riparian buffers as these positive effects likely outweigh the negative impact of increased N2O emissions.
The datasets presented in this study can be found in online repositories. The names of the repository/repositories and accession number(s) can be found below: JD, HK, JS, and US, Soil greenhouse gas fluxes along transects from oil palm to riparian forests in the SAFE landscape, 2019, Zenodo; http://doi.org/10.5281/zenodo.3251886.
JD and US designed the experiment, in collaboration with HK and JS. HK carried out the field measurements and prepared soil for laboratory analysis. NM performed the soil laboratory analysis. NC and JD analyzed the flux and soil data. NC carried out statistical analysis. JD and US wrote the manuscript with comments from all co-authors. All authors contributed to the article and approved the submitted version.
This project was funded as LOMBOK (Land-use Options for Maintaining BiOdiversity and eKosystem functions) by the NERC Human Modified Tropical Forest (HMTF) research program (NE/K016091/1). This work was also supported by the Sustainable Use of Natural Resources to Improve Human Health and Support Economic Development (SUNRISE) Program (NE/R000131/1) (UKCEH).
The authors declare that the research was conducted in the absence of any commercial or financial relationships that could be construed as a potential conflict of interest.
All claims expressed in this article are solely those of the authors and do not necessarily represent those of their affiliated organizations, or those of the publisher, the editors and the reviewers. Any product that may be evaluated in this article, or claim that may be made by its manufacturer, is not guaranteed or endorsed by the publisher.
We would like to thank the LOMBOK research assistants (especially “Noy” Arnold James and “Loly” Lawlina Mansul) at SAFE for help with the field sampling, Fifilyana Abdulkarim for laboratory analysis at the Forest Research Center, Sepilok, and the managers of the Merbau and Mengarris estates for access to their land.
Bates, D., Mächler, M., Bolker, B., and Walker, S. (2015). Fitting linear mixed-effects models using lme4. J. Stat. Softw. 67, 1–48. doi: 10.18637/jss.v067.i01
Brauman, K. A., Freyberg, D. L., and Daily, G. C. (2015). Impacts of land-use change on groundwater supply: ecosystem services assessment in Kona, Hawaii. J. Water Resour. Plan. Manag. 141:A4014001. doi: 10.1061/(ASCE)WR.1943-5452.0000495
Butterbach-Bahl, K., Baggs, E. M., Dannenmann, M., Kiese, R., and Zechmeister-Boltenstern, S. (2013). Nitrous oxide emissions from soils: how well do we understand the processes and their controls? Philos. Trans. R. Soc. B Biol. Sci. 368:20130122. doi: 10.1098/rstb.2013.0122
Cole, L. J., Stockan, J., and Helliwell, R. (2020). Managing riparian buffer strips to optimise ecosystem services: a review. Agric. Ecosyst. Environ. 296:106891. doi: 10.1016/j.agee.2020.106891
Comeau, L.-P., Hergoualc’h, K., Hartill, J., Smith, J., Verchot, L. V., Peak, D., et al. (2016). How do the heterotrophic and the total soil respiration of an oil palm plantation on peat respond to nitrogen fertilizer application? Geoderma 268, 41–51. doi: 10.1016/j.geoderma.2016.01.016
Cowan, N., Carnell, E., Skiba, U., Dragosits, U., Drewer, J., and Levy, P. (2020). Nitrous oxide emission factors of mineral fertilisers in the UK and Ireland: a Bayesian analysis of 20 years of experimental data. Environ. Int. 135:105366. doi: 10.1016/j.envint.2019.105366
Cowan, N., Maire, J., Krol, D., Cloy, J. M., Hargreaves, P., Murphy, R., et al. (2021). Agricultural soils: a sink or source of methane across the British Isles? Eur. J. Soil Sci. 72, 1842–1862. doi: 10.1111/ejss.13075
Cowan, N. J., Norman, P., Famulari, D., Levy, P. E., Reay, D. S., and Skiba, U. M. (2015). Spatial variability and hotspots of soil N2O fluxes from intensively grazed grassland. Biogeosciences 12, 1585–1596. doi: 10.5194/bg-12-1585-2015
Davidson, E. A., Keller, M., Erickson, H. E., Verchot, L. V., and Veldkamp, E. (2000). Testing a conceptual model of soil emissions of nitrous and nitric oxides: using two functions based on soil nitrogen availability and soil water content, the hole-in-the-pipe model characterizes a large fraction of the observed variation of nitric oxide and nitrous oxide emissions from soils. BioScience 50, 667–680. doi: 10.1641/0006-3568(2000)050[0667:TACMOS]2.0.CO;2
Di Stefano, J. (2004). A confidence interval approach to data analysis. For. Ecol. Manag. 187, 173–183. doi: 10.1016/S0378-1127(03)00331-1
Drewer, J., Anderson, M., Levy, P. E., Scholtes, B., Helfter, C., Parker, J., et al. (2017a). The impact of ploughing intensively managed temperate grasslands on N2O, CH4 and CO2 fluxes. Plant Soil 411, 193–208. doi: 10.1007/s11104-016-3023-x
Drewer, J., Kuling, H. J., Sentian, J., and Skiba, U. (2019). Soil Greenhouse Gas Fluxes Along Transects From Oil Palm to Riparian Forests in the SAFE Landscape [Data set]. Zenodo, doi: 10.5281/zenodo.3251886
Drewer, J., Leduning, M. M., Griffiths, R. I., Goodall, T., Levy, P. E., Cowan, N., et al. (2021). Comparison of greenhouse gas fluxes from tropical forests and oil palm plantations on mineral soil. Biogeosciences 18, 1559–1575. doi: 10.5194/bg-18-1559-2021
Drewer, J., Yamulki, S., Leeson, S. R., Anderson, M., Perks, M. P., Skiba, U. M., et al. (2017b). Difference in soil methane (CH4) and nitrous oxide (N2O) fluxes from bioenergy crops SRC willow and SRF scots pine compared with adjacent arable and fallow in a temperate climate. Bioenergy Res. 10, 575–582. doi: 10.1007/s12155-017-9824-9
Drewer, J., Zhao, J., Leduning, M. M., Levy, P. E., Sentian, J., Gubry-Rangin, C., et al. (2020). Linking nitrous oxide and nitric oxide fluxes to microbial communities in tropical forest soils and oil palm plantations in malaysia in laboratory incubations. Front. For. Glob. Change 3:4. doi: 10.3389/ffgc.2020.00004
Dutaur, L., and Verchot, L. V. (2007). A global inventory of the soil CH4sink. Glob. Biogeochem. Cycles 21:GB4013. doi: 10.1029/2006GB002734
Ewers, R. M., Didham, R. K., Fahrig, L., Ferraz, G., Hector, A., Holt, R. D., et al. (2011). A large-scale forest fragmentation experiment: the stability of altered forest ecosystems project. Philos. Trans. R. Soc. Lond. Ser. B Biol. Sci. 366, 3292–3302. doi: 10.1098/rstb.2011.0049
Hassler, E., Corre, M. D., Tjoa, A., Damris, M., Utami, S. R., and Veldkamp, E. (2015). Soil fertility controls soil–atmosphere carbon dioxide and methane fluxes in a tropical landscape converted from lowland forest to rubber and oil palm plantations. Biogeosciences 12, 5831–5852. doi: 10.5194/bg-12-5831-2015
Hedin, L. O., von Fischer, J. C., Ostrom, N. E., Kennedy, B. P., Brown, M. G., and Robertson, G. P. (1998). Thermodynamic constraints on nitrogen transformations and other biogeochemical processes at soil-stream interfaces. Ecology 79, 684–703. doi: 10.2307/176963
Hefting, M. M., Clement, J.-C., Bienkowski, P., Dowrick, D., Guenat, C., Butturini, A., et al. (2005). The role of vegetation and litter in the nitrogen dynamics of riparian buffer zones in Europe. Ecol. Eng. 24, 465–482. doi: 10.1016/j.ecoleng.2005.01.003
IPCC (2000). “Indirect N2O emissions from agriculture,” in Good Practice Guidance and Uncertainty Management in National Greenhouse Gas Inventories, eds J. Penman, I. Galbally, T. Hiraishi, B. Nyenzi, S. Emmanul, L. Buendia, et al. (Geneva: IPCC), 381–397.
Kachenchart, B., Jones, D. L., Gajaseni, N., Edwards-Jones, G., and Limsakul, A. (2012). Seasonal nitrous oxide emissions from different land uses and their controlling factors in a tropical riparian ecosystem. Agric. Ecosyst. Environ. 158, 15–30. doi: 10.1016/j.agee.2012.05.008
Kerdraon, D., Drewer, J., Chung, A. Y. C., Majalap, N., Slade, E. M., Bréchet, L., et al. (2020). Litter inputs, but not litter diversity, maintain soil processes in degraded tropical forests—a cross-continental comparison. Front. For. Glob. Change 2:90. doi: 10.3389/ffgc.2019.00090
Knopf, F. L., and Samson, F. B. (1994). Scale perspectives on avian diversity in Western Riparian ecosystems. Conserv. Biol. 8, 669–676. doi: 10.1046/j.1523-1739.1994.08030669.x
Lang, R., Goldberg, S. D., Blagodatsky, S., Piepho, H.-P., Hoyt, A. M., Harrison, R. D., et al. (2020). Mechanism of methane uptake in profiles of tropical soils converted from forest to rubber plantations. Soil Biol. Biochem. 145:107796. doi: 10.1016/j.soilbio.2020.107796
Levy, P. E., Burden, A., Cooper, M. D. A., Dinsmore, K. J., Drewer, J., Evans, C., et al. (2012). Methane emissions from soils: synthesis and analysis of a large UK data set. Glob. Change Biol. 18, 1657–1669. doi: 10.1111/j.1365-2486.2011.02616.x
Levy, P. E., Cowan, N., van Oijen, M., Famulari, D., Drewer, J., and Skiba, U. (2017). Estimation of cumulative fluxes of nitrous oxide: uncertainty in temporal upscaling and emission factors. Eur. J. Soil Sci. 68, 400–411. doi: 10.1111/ejss.12432
Luke, S. H., Slade, E. M., Gray, C. L., Annammala, K. V., Drewer, J., Williamson, J., et al. (2019). Riparian buffers in tropical agriculture: scientific support, effectiveness and directions for policy. J. Appl. Ecol. 56, 85–92. doi: 10.1111/1365-2664.13280
Ma, L., Xiong, Z., Yao, L., Liu, G., Zhang, Q., and Liu, W. (2020). Soil properties alter plant and microbial communities to modulate denitrification rates in subtropical riparian wetlands. Land Degrad. Dev. 31, 1792–1802. doi: 10.1002/ldr.3569
Mafa-Attoye, T. G., Baskerville, M. A., Ofosu, E., Oelbermann, M., Thevathasan, N. V., and Dunfield, K. E. (2020). Riparian land-use systems impact soil microbial communities and nitrous oxide emissions in an agro-ecosystem. Sci. Total Environ. 724:138148. doi: 10.1016/j.scitotenv.2020.138148
Mulholland, P. J., Helton, A. M., Poole, G. C., Hall, R. O., Hamilton, S. K., Peterson, B. J., et al. (2008). Stream denitrification across biomes and its response to anthropogenic nitrate loading. Nature 452, 202–205. doi: 10.1038/nature06686
Myhre, G. D., Shindell, F.-M., Bréon, W., Collins, J., Fuglestvedt, J., Huang, D., et al. (2013). “Anthropogenic and natural radiative forcing,” in Climate Change 2013: The Physical Science Basis. Contribution of Working Group I to the Fifth Assessment Report of the Intergovernmental Panel on Climate Change, eds T. F. Stocker, D. Qin, G.-K. Plattner, M. Tignor, S. K. Allen, J. Boschung, et al. (Cambridge: Cambridge University Press).
Nagy, R. C., Porder, S., Neill, C., Brando, P., Quintino, R. M., and Nascimento, S. A. (2015). Structure and composition of altered riparian forests in an agricultural Amazonian landscape. Ecol. Appl. 25, 1725–1738. doi: 10.1890/14-1740.1
Nilsson, C., and Svedmark, M. (2002). Basic principles and ecological consequences of changing water regimes: riparian plant communities. Environ. Manag. 30, 468–480. doi: 10.1007/s00267-002-2735-2
Osborne, L. L., and Kovacic, D. A. (1993). Riparian vegetated buffer strips in water-quality restoration and stream management. Freshw. Biol. 29, 243–258. doi: 10.1111/j.1365-2427.1993.tb00761.x
R Core Team (2017). R: A Language and Environment for Statistical Computing. Vienna: R Foundation for Statistical Computing.
Reay, D. S., Davidson, E. A., Smith, K. A., Smith, P., Melillo, J. M., Dentener, F., et al. (2012). Global agriculture and nitrous oxide emissions. Nat. Clim. Change 2, 410–416. doi: 10.1038/nclimate1458
Riutta, T., Malhi, Y., Kho, L. K., Marthews, T. R., Huaraca Huasco, W., Khoo, M., et al. (2018). Logging disturbance shifts net primary productivity and its allocation in Bornean tropical forests. Glob. Change Biol. 24, 2913–2928. doi: 10.1111/gcb.14068
Sabo, J. L., Sponseller, R., Dixon, M., Gade, K., Harms, T., Heffernan, J., et al. (2005). Riparian zones increase regional species richness by harboring different, not more species. Ecology 86, 56–62. doi: 10.1890/04-0668
Keywords: greenhouse gases, riparian buffer, tropics, nitrate, ammonium, nitrogen
Citation: Drewer J, Kuling HJ, Cowan NJ, Majalap N, Sentian J and Skiba U (2021) Comparing Soil Nitrous Oxide and Methane Fluxes From Oil Palm Plantations and Adjacent Riparian Forests in Malaysian Borneo. Front. For. Glob. Change 4:738303. doi: 10.3389/ffgc.2021.738303
Received: 08 July 2021; Accepted: 07 September 2021;
Published: 27 September 2021.
Edited by:
Jinshu Chi, Swedish University of Agricultural Sciences, SwedenReviewed by:
Haofei Yu, University of Central Florida, United StatesCopyright © 2021 Drewer, Kuling, Cowan, Majalap, Sentian and Skiba. This is an open-access article distributed under the terms of the Creative Commons Attribution License (CC BY). The use, distribution or reproduction in other forums is permitted, provided the original author(s) and the copyright owner(s) are credited and that the original publication in this journal is cited, in accordance with accepted academic practice. No use, distribution or reproduction is permitted which does not comply with these terms.
*Correspondence: Julia Drewer, anVld0BjZWguYWMudWs=
Disclaimer: All claims expressed in this article are solely those of the authors and do not necessarily represent those of their affiliated organizations, or those of the publisher, the editors and the reviewers. Any product that may be evaluated in this article or claim that may be made by its manufacturer is not guaranteed or endorsed by the publisher.
Research integrity at Frontiers
Learn more about the work of our research integrity team to safeguard the quality of each article we publish.