- 1RESOLVE, Washington, DC, United States
- 2Osprey Insights LLC, Seattle, WA, United States
- 3Alaska Venture Fund, Anchorage, AK, United States
- 44 International Arctic Research Center (IARC), University of Alaska Fairbanks, Fairbanks, AK, United States
- 5Department of Environmental Science, Alaska Pacific University, Anchorage, AK, United States
- 6National Audubon Society, Anchorage, AK, United States
- 7Conservation Sciences Graduate Program, College of Food, Agricultural, and Natural Resource Sciences, University of Minnesota, Minneapolis, MN, United States
- 8Department of Forest Ecosystems & Society, Oregon State University, Corvallis, OR, United States
- 9The School of Resource and Environmental Management, Simon Fraser University, Burnaby, BC, Canada
- 10Renewable Resources, University of Alberta, Edmonton, AB, Canada
- 11Renewable Resources, Yukon University, Whitehorse, YT, Canada
- 12Environmental Science, Policy, & Sustainability, Southern Oregon University, Ashland, OR, United States
- 13Geography and Environmental Studies, University of Alaska Anchorage, Anchorage, AK, United States
Alaska is globally significant for its large tracts of intact habitats, which support complete wildlife assemblages and many of the world’s healthiest wild fisheries, while also storing significant amounts of carbon. Alaska has 1/3 of United States federal lands, the bulk of the United States’ intact and wild lands, and over half of the country’s total terrestrial ecosystem carbon on federal lands. Managing Alaska’s public lands for climate and biodiversity conservation purposes over the next 30–50 years would provide meaningful and irreplaceable climate benefits for the United States and globe. Doing so via a co-management approach with Alaska’s 229 federally recognized tribes is likely not only to be more effective but also more socially just. This paper lays out the scientific case for managing Alaska’s public lands for climate stabilization and resilience and addresses three primary questions: Why is Alaska globally meaningful for biodiversity and climate stabilization? Why should Alaska be considered as a key element of a climate stabilization and biodiversity conservation strategy for the United States? What do we need to know to better understand the role of Alaska given future scenarios? We summarize evidence for the role Alaska’s lands play in climate stabilization, as well as what is known about the role of land management in influencing carbon storage and sequestration. Finally, we summarize priority research that is needed to improve understanding of how policy and management prescriptions are likely to influence the role Alaska plays in global climate stabilization and adaptation.
Introduction
Alaska is globally significant for its large tracts of intact habitats and their role in conserving biodiversity and storing carbon while supporting traditional and cultural uses (Kofinas et al., 2010; Reynolds et al., 2018; Dinerstein et al., 2020). Alaska holds one third of United States federal lands, the bulk of the United States’ intact and wild lands, and 62 percent of the country’s terrestrial ecosystem carbon stocks on federal lands (Merrill et al., 2018). The Alaska Native Claims Settlement Act of 1971 (ANCSA) established twelve (later thirteen) Alaska Native regional corporations and over 200 local village corporations, representing a total of 44 million acres (17,806 km2) of land (Figure 1). Alaska is home to 229 federally recognized tribes and it is the United States state with the highest proportion of American Indian and Alaska Native people1. Alaska has >30% of the United States coastline2 and 63% of the nation’s wetlands (Hall et al., 1994). Most of Alaska far exceeds landscape condition values of even the most protected places in the contiguous United States (Reynolds et al., 2018; Figure 2). The intactness of Alaska’s systems as part of a national climate change mitigation strategy has been underexplored in both science and public policy.
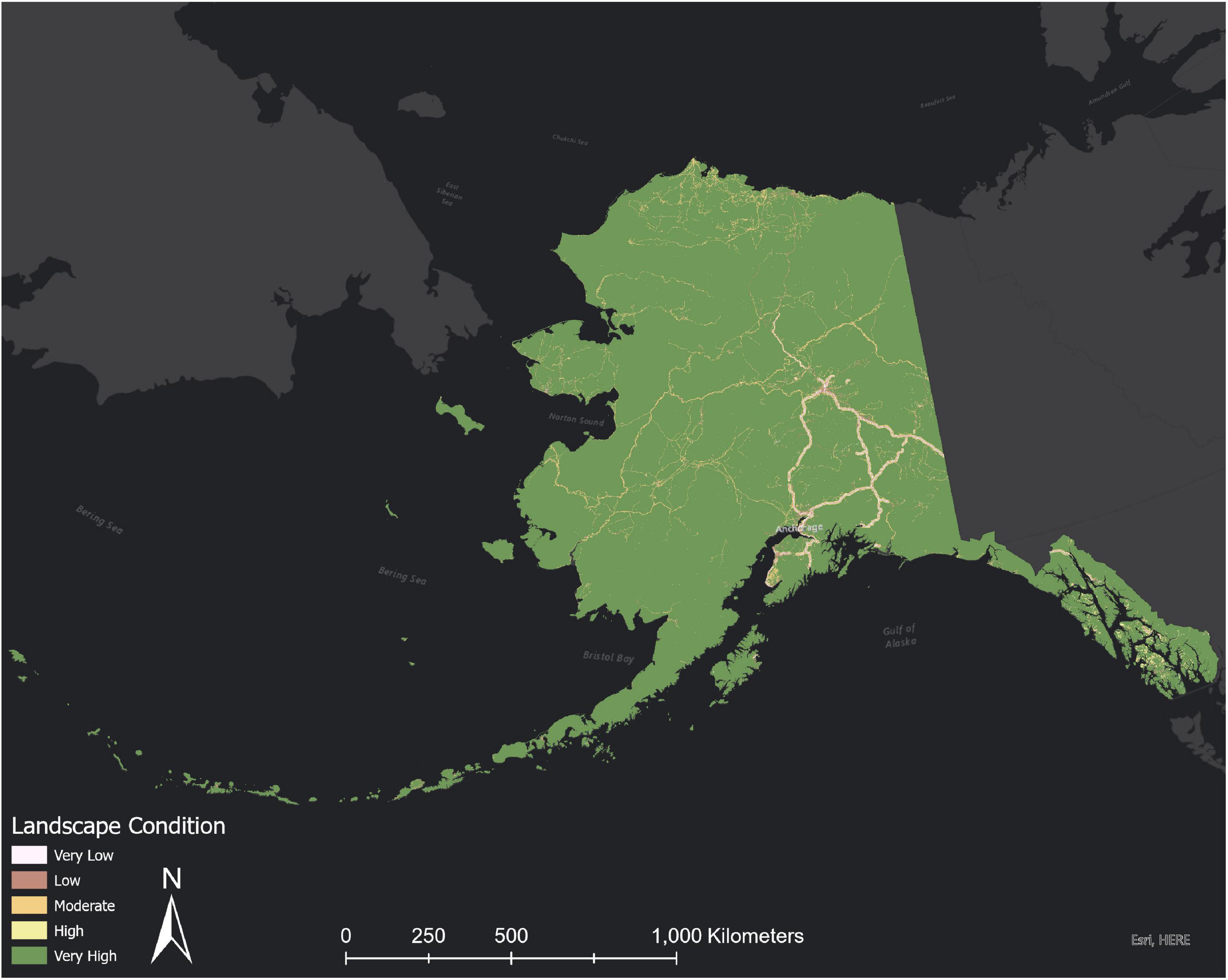
Figure 2. Landscape condition of Alaska; data from Trammell and Aisu (2015). Landscape condition uses a method developed by Hak and Comer (2017) but was modified to better represent the effect of human development on systems in Alaska. Each type of human footprint (e.g., major highways or logging) is assigned a site impact score, which are a relative and scaled measure of the overall impact of a human activity on the landscape, and a decay score to represent the gradual decreasing impact as you move away from the activity (Trammell and Aisu, 2015). This is meant to represent the physical impact of human activities to the landscape, not necessarily all the ecological impacts.
In discussing the role of Alaska’s intact systems in fostering biodiversity and storing carbon, it is important to recognize that the region is already experiencing and will continue to experience climatic changes that will influence carbon sequestration and storage capacity across landscapes. While physically Alaska’s landscapes are largely undeveloped (Figure 3), the region is changing quickly. Presently, climate change is the biggest driver of change for Alaska’s ecosystems, species, and people, and this has been well documented (Stone et al., 2002; Chapin et al., 2004; Hinzman et al., 2005; Brubaker et al., 2011). Likewise, cultural and economic climate change impacts across Alaska have been reviewed elsewhere (e.g., Hinzman et al., 2005; Wolken et al., 2011; Cochran et al., 2013; Berman and Schmidt, 2019). With reference to these previous reviews, this analysis focuses on carbon dynamics and ecosystem integrity.
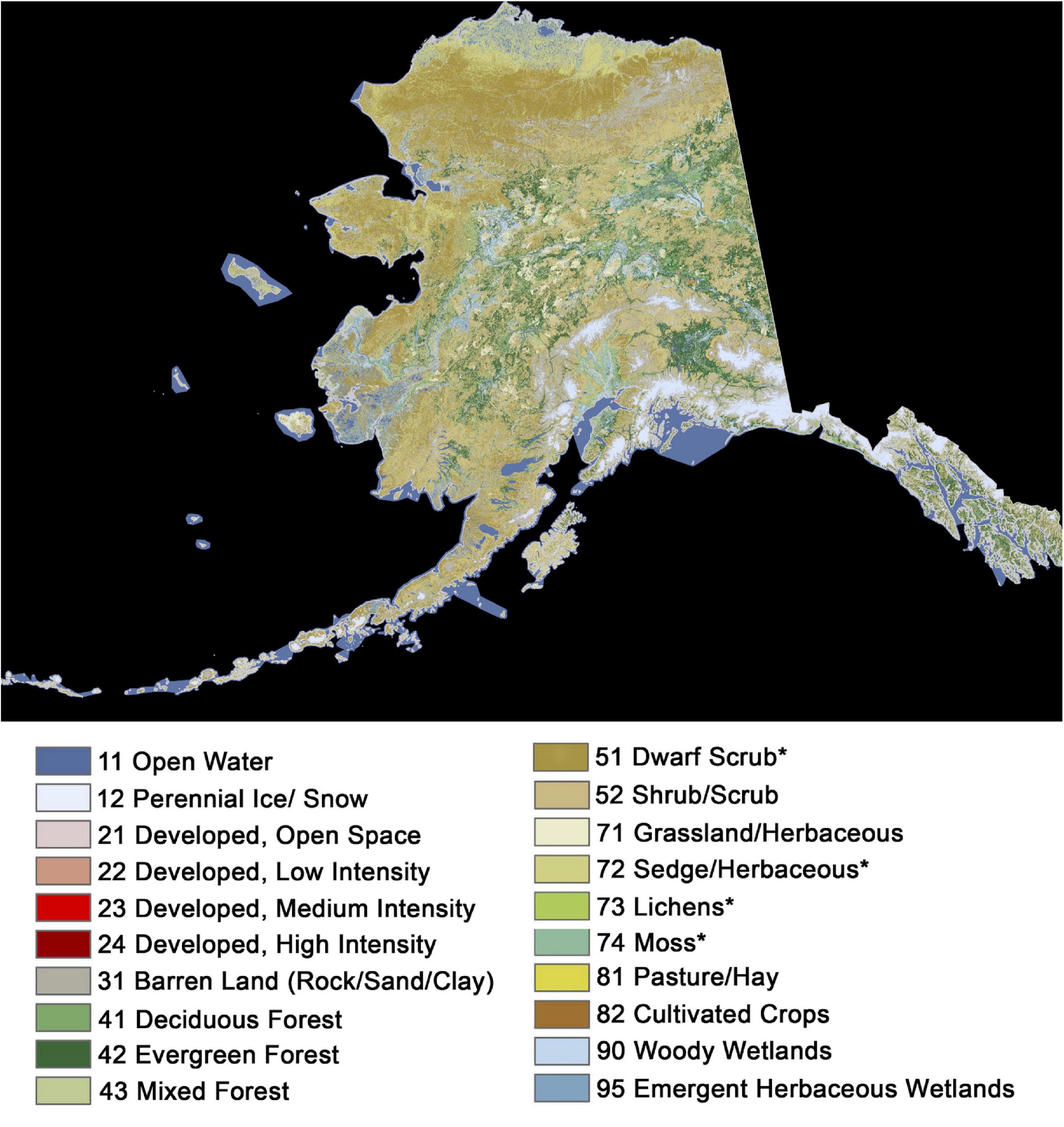
Figure 3. Land Cover for Alaska according to the National Land Cover Database maintained by the United States Geological Service. * Alaska only.
Due to the geographic size and spatial extent of Alaska’s landscapes, along with the relative intactness of many of its ecological systems, recent reviews suggest that Alaska could contribute over 50% of total carbon storage for the entire United States (Zhu and McGuire, 2016). Northern permafrost soils contain almost two times the amount of stored carbon as that found within the atmosphere. However, soil carbon concentrations will change with the melting of permafrost (Hugelius et al., 2014; Jones et al., 2017). The decomposition of permafrost carbon could accelerate climate warming through the loss of stored carbon, and methane releases have been widely documented from warming lakes (Jorgenson et al., 2001). The interaction of marine and terrestrial systems across seasonal variations makes predicting changes to total carbon storage challenging (Parmentier et al., 2017). Likewise, as will be discussed, the effects of climate change on the frequency and intensity of wildfires add complexity to issues of carbon balance. Here we focus on the role that Alaska’s intact systems and species might play in maintaining biodiversity, storing carbon, and mitigating climate change – even as climate change continues to affect those systems.
Global climate targets have been established in order to prevent the worst effects of climate change, including disruption to weather patterns, extreme heat and flood events, water and food shortages, and loss of biodiversity and essential ecosystem services (IPCC, 2018). Naturally functioning ecosystems are critical for mitigating greenhouse gas emissions and meeting global climate targets via both protecting existing carbon stocks and new sequestration (Griscom et al., 2017; Teske, 2019). These natural-climate solutions (NCS) include proforestation (allowing forests to continue to grow), which is the most rapid means to accumulate additional carbon in forests and out of the atmosphere (Law et al., 2018; Moomaw et al., 2019). Other strategies—including conservation and restoration that increase carbon storage and/or reduce greenhouse gas emissions—are increasingly recognized as essential to meeting global climate targets (Griscom et al., 2017; Seddon et al., 2020). Nearly all pathways to staying below 2°C rise in average global temperature require preventing conversion of the vast majority of Earth’s remaining, terrestrial habitats (Teske, 2019). Significantly, much of the land essential to achieving carbon storage objectives is also essential for protecting biodiversity (Dinerstein et al., 2019, 2020; Buotte et al., 2020).
Protection of intact and low human-impact forests is one of the most cost-effective ways to mitigate climate change. Globally, forests store 662 Gt C, or 75% of the amount currently in the atmosphere (Food and Agricultural Organization, 2020), and terrestrial ecosystems (primarily forests) annually remove 30% of excess carbon emitted by human activities. A large portion of this carbon is found in the globally important forest and wetland areas of the tropics and boreal region (Bradshaw and Warkentin, 2015). Protection of intact ecosystems from industrial land uses is particularly important since carbon storage values are diminished as natural areas are degraded or converted (Grünzweig et al., 2004; Martin and Watson, 2016).
Though the impacts to Alaska systems from climate change are well established, there has been little focus on the potential contribution of Alaska land and water management to climate stabilization (Zhu and McGuire, 2016). Though Alaska’s marine areas are also extremely significant for global biodiversity and carbon budgets, we focus here on terrestrial ecosystems. We synthesize existing literature and provide expert input to answer three primary questions: Why is Alaska globally meaningful (for biodiversity and climate)? Why should Alaska be a key element of a climate stabilization and biodiversity conservation strategy for the United States? What do we need to know to better understand the role Alaska will play in climate stabilization and resilience given projected changes?
The Global Significance of Alaska for Biodiversity, Climate Stabilization, and Resilience: Intact Habitats and Biodiversity
Alaska is recognized for its naturally functioning ecosystems, undammed rivers, complete wildlife assemblages, and healthy populations of fish and wildlife. Much of this is attributed to its large tracts of intact or undeveloped habitat, which are important for sustaining ecological and evolutionary processes central to the long-term persistence of biodiversity (Figure 2; Locke et al., 2019). The majority of the world’s most significant congregations of species, remaining migrations, and sheer numbers of individuals of many species occur in the world’s most intact landscapes (Betts et al., 2017). Ecologically intact ecosystems are becoming increasingly limited on the planet, making their identification and conservation an important priority (Plumptre et al., 2019). These remaining intact landscapes contain the last strongholds for many imperiled species (Watson et al., 2016). In Alaska, they also support Alaska Native peoples, who currently and historically maintain complex and vibrant traditions of subsistence hunting, fishing, and gathering. These traditions are key to not only the cultures and people themselves, but also to public policy (Wheeler and Thornton, 2005).
Large tracts of undeveloped areas also support a majority of the world’s remaining intact mega-fauna assemblages, as well as species sensitive to exploitation or conflicts with humans (Morrison et al., 2007; Ripple et al., 2014, 2015). Alaska is the only location in the United States outside of the Yellowstone Ecosystem that has landscapes with their full roster of historically present (AD1500) large mammals (Morrison et al., 2007). The state as a whole is a global priority for conserving or restoring large mammal assemblages, as all of Alaska’s ecoregions contain either complete or near-complete rosters of their historically present large mammals (Dinerstein et al., 2020). Alaska is also a priority for conserving the increasingly rare phenomenon of mass migration of large terrestrial mammals, with a number of significant caribou migrations occurring throughout the region (Harris et al., 2009).
Alaska hosts a number of globally significant sites for large aggregations of breeding shorebirds. The state provides breeding habitat for more shorebirds than any other state in the United States and hosts between 7 and 12 million shorebirds, or as much as 50% of all the shorebirds that occur in North America3. Most of the world’s population of three shorebird species, entire populations of five subspecies, and large portions of North America’s populations of six other species or subspecies depend on Alaska’s habitats2. The Copper River Delta supports what is thought to be the world’s largest concentration of shorebirds, with between 5 and 8 million shorebirds visiting each spring. Concentrations of over one million birds each occur at ten key migration or stopover sites in Alaska3. 155 Key Biodiversity Areas, globally significant sites for species conservation, occur in the state (Birdlife International, 2019). According to the National land cover database, 24% of Alaska is forested, and 74% is vegetated (i.e., not all ice and barren land (Figure 3).
Salmon-producing regions of Alaska are highly diverse, ranging from rainforest, to boreal forest, to Arctic tundra lowlands.4 Alaska’s contribution to global salmon catch is profound; roughly half of the world’s sockeye salmon spawn in the Bristol Bay watershed and rivers along the Gulf of Alaska alone produce one-third of the world’s wild salmon (Schoen et al., 2017). Salmon are known to use approximately 36,000 km of streams in the state, which equates to nearly three trips around the globe; and cataloging of salmon occurrence in Alaska is incomplete and ongoing due, in part, to insufficient funding. No other place on Earth has as many healthy and diverse populations of wild salmon.
In Alaska, large tracts of intact habitat support multiple runs of salmon. Maintaining multiple populations with diverse responses to environmental fluctuations within a landscape provides greater ecological resilience to threats and stochastic events (Schindler et al., 2010). The Tongass and Chugach National Forests in southeastern and southcentral Alaska represent some of the largest tracts of intact rainforest in the world (Orians and Schoen, 2017) and these forests support diverse and productive Pacific salmon fisheries (Johnson et al., 2019). Intact landscapes support habitat diversity allowing, for instance, the peak migration timing of the same species in nearby streams to vary by 3 weeks or more due in part to temperature differences. This variability allows trout, bears, and various birds (e.g., gulls) to eat salmon and salmon eggs for months rather than weeks by moving progressively from colder to warmer streams (Ruff et al., 2011; Schoen et al., 2017). Diversity in the timing of migration can also extend fishing seasons and improve food security for Indigenous and other people (Nesbitt and Moore, 2016). Further, salmon provide a bridge between marine, freshwater, and terrestrial ecosystems, enriching adjacent forests through nutrient transfer that enhances tree growth (Quinn et al., 2018), and carbon sequestration.
The coastal temperate rainforests of coastal British Columbia and southeast Alaska exhibit a distinct aspect of “intactness” that is rarely replicated globally: river systems and their surrounding watersheds with no history of industrial exploitation or modern urban development (Lertzman and MacKinnon, 2013). These watershed ecosystems represent not just ecological communities, seral stage distributions, and carbon stores in their historic proportions and configurations on the landscape, but also are more likely to maintain the hydrological, geomorphic, and other physical processes that sustain the ecosystems over time. These undeveloped watersheds represent a globally rare and significant, process-based conservation opportunity.
Intact Ecosystems and Carbon Storage
The temperate rainforests of the Pacific Northwest, including those in the southeast of Alaska, contain the most extensive examples of undeveloped and semi-natural temperate rainforest remaining globally, and are recognized for their significance in storing large amounts of carbon (Hudiburg et al., 2009; Law et al., 2018). Trees in the Pacific Northwest often live for 800 years or more, storing carbon in boles and roots for that length of time, and they continue to accumulate carbon annually (Hudiburg et al., 2009; Stephenson et al., 2014; Lutz et al., 2018). In Oregon and Washington, forests east of the Cascade crest, large trees (greater than 21 inches in diameter) are only 3% of the trees but account for almost half of the total tree biomass (Mildrexler et al., 2020). Protecting more forest carbon on public lands, lengthening harvest cycles, and reforestation contribute the most to increase forest carbon storage and sequestration (Law et al., 2018). These findings led to the promotion of proforestation, or letting forests grow, as a high priority climate mitigation strategy globally (Moomaw et al., 2019, 2020).
The rainforests of southeast Alaska are notable for supporting large tracts of old growth forest, where tree size and biomass are higher than in younger forests. There is more old-growth than young forest area in Alaska (Pan et al., 2011), and the most effective measure to protect existing carbon stocks is to protect and maintain existing old growth stands. The total carbon stock in the forest and soils of the Tongass National Forest in southeast Alaska alone is 8% of that of the forests in the conterminous United States (Leighty et al., 2006). Protecting forests with old trees will also confer co-benefits to wildlife, biodiversity, and other ecosystem services including the provision of microclimate buffering under future extreme climate conditions and various cultural ecosystem services (Sutherland et al., 2016).
The boreal forest biome, which covers much of interior Alaska, is increasingly recognized for the important role it plays in carbon storage (Dinerstein et al., 2020; Wells et al., 2020). The forests in Alaska have relatively low carbon density in living vegetation compared with coastal forests (Domke et al., 2018), but when maps of total biomass (including soil) are included, these areas stand out as globally significant (Dinerstein et al., 2020; Soto-Navarro et al., 2020; Figures 4, 5). Half the carbon in Alaska and Canada’s boreal zone is stored in coniferous forests (Schuur et al., 2018). The boreal forests of Alaska show growth increases in productivity at the boreal-tundra ecotones where it is colder and sparsely forested, and drought-induced declines in productivity throughout interior Alaska, indicating a biome shift is underway (Beck et al., 2011). These forests also lose a substantial amount of carbon in wildfires, primarily from the burning of soils and duff (Goetz et al., 2012; Kasischke and Hoy, 2012).
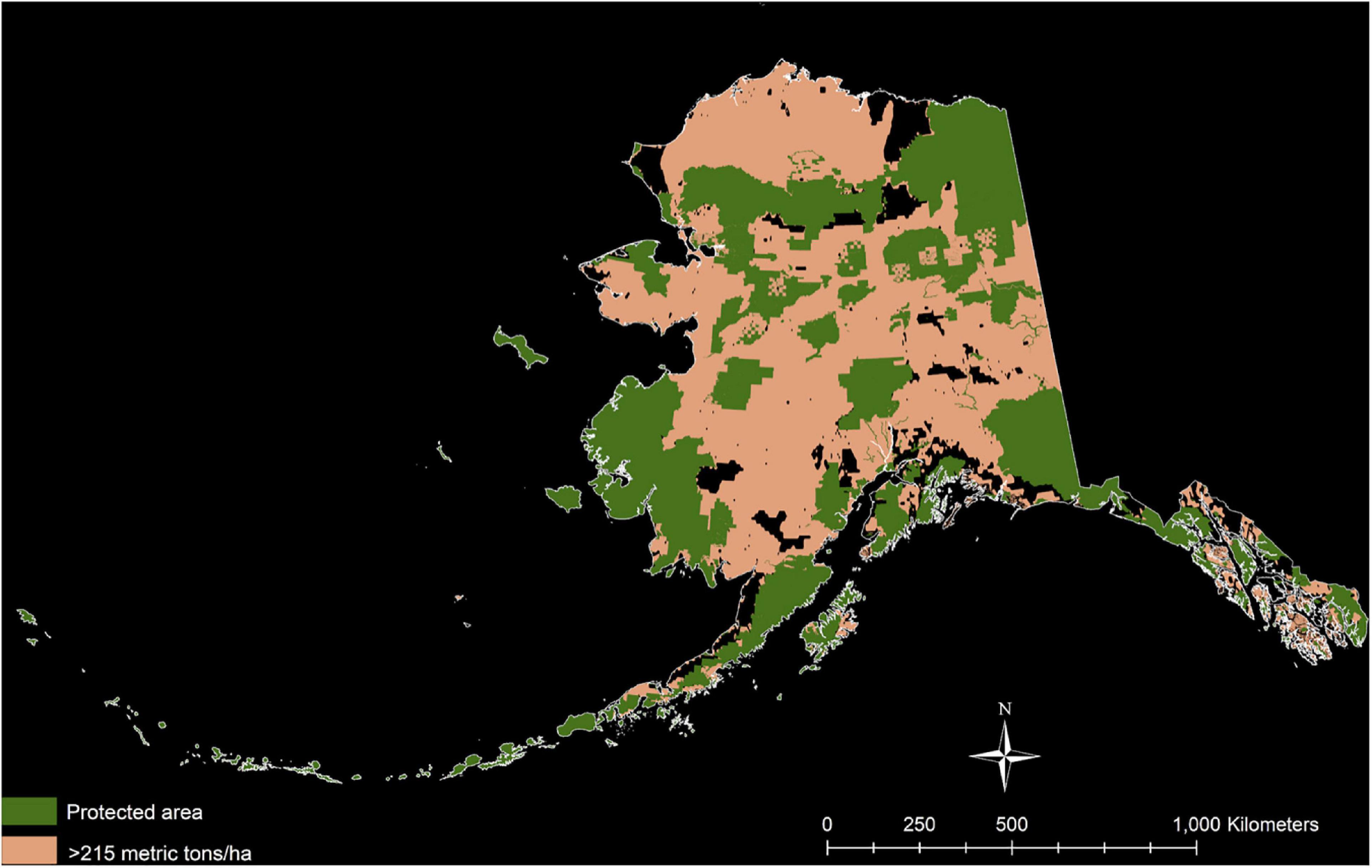
Figure 4. IUCN I-VI Protected Areas in Alaska, according to the Protected Areas Database of the United States, maintained by the United States Geological Survey, and areas outside of the protected areas system where total carbon biomass is above the global median.
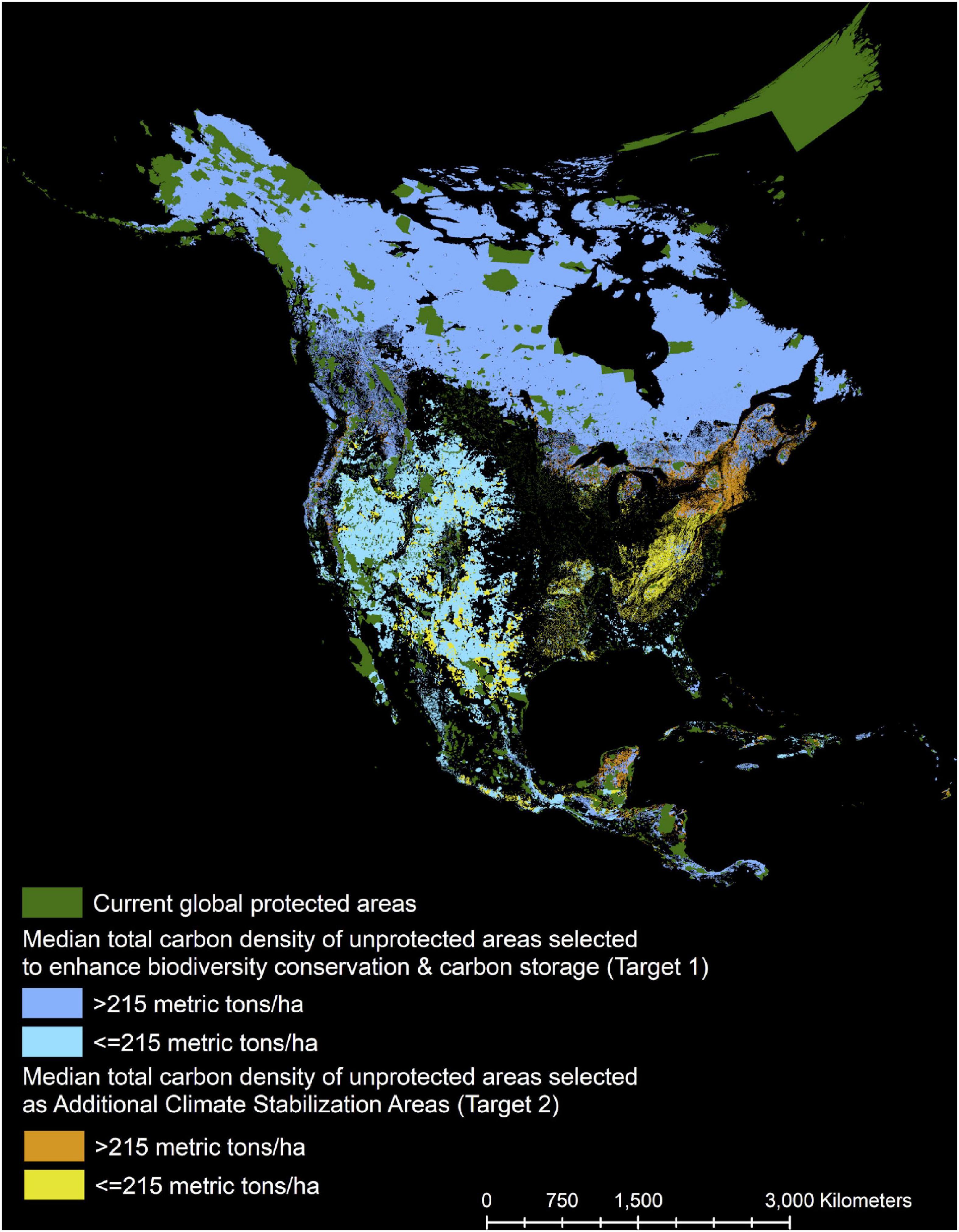
Figure 5. Median total carbon distribution in the Nearctic realm [from Dinerstein et al. (2020)].
Disturbances reduce the net carbon sink, yet have been challenging to quantify in this region. Satellite and field observations showed that the Alaska Boreal Interior ecoregion’s above ground biomass carbon averaged 29.3 MgC ha–1 from 1984 to 2014, and 36.8 MgC ha–1 in the Boreal Cordillera ecoregion (Wang et al., 2021). The total biomass stocks in the Alaska Boreal Interior averaged 1,410 TgC for the same period. The net change in above ground biomass carbon was +60 and +143 TgC for the ecoregions, respectively, though most of the Boreal Cordillera ecoregion is in Canada. This indicates that the above ground carbon of these forests was still a net carbon sink after accounting for disturbance losses from primarily wildfires and harvest. On an area basis, harvest impacts on above ground carbon were nearly two times larger than from wildfire (Wang et al., 2021).
While the forests of Alaska are globally significant carbon storehouses, the vast majority of carbon in Alaska is stored in undisturbed soils. Globally, peatlands-wetlands whose soils consist almost entirely of organic matter-cover just 3% of the Earth’s surface but store more carbon than any other types of ecosystems and for longer periods of time. Due to rising temperatures, permafrost is thawing rapidly and is thus altering carbon storage dynamics with potentially dire consequences. But as new unfrozen peatlands form, more carbon may be taken from the atmosphere due to increased plant growth. The carbon balance of these peatlands is changing rapidly with as yet unknown consequences for carbon storage and hence the global climate (McGuire et al., 2012; Graven et al., 2013; Commane et al., 2017). While long-term warming will likely restructure Arctic tundra, there are multiple lines of evidence of Arctic greening and increasing productivity (Berner et al., 2020).
The amount of carbon stored on federal lands in Alaska is approximately 62% of the total carbon stored on all United States federal lands (Merrill et al., 2018). Average carbon storage in live vegetation and soils (2005–2014) was 131,675 MMT CO2 Eq. on Alaska’s federal lands, with 92 percent stored in soils and eight percent stored in live vegetation (Merrill et al., 2018). In the Tongass National Forest, 26 percent is stored in live vegetation (Leighty et al., 2006). Maps of total biomass carbon, which integrate above and below ground sources, indicate that nearly all of Alaska is above the global median (Figures 4, 5). Protecting these carbon-storing intact systems in Alaska is important because the ability of these ecosystems to recover carbon once lost is very slow (Goldstein et al., 2020). It takes hundreds to thousands of years to accumulate carbon in trees and soils to their ecological potential, respectively, and it takes that long to regain that amount of carbon once removed, if ever (Sun et al., 2004; Luyssaert et al., 2008; Hudiburg et al., 2009). Ecosystem types that host large amounts of this “irrecoverable” carbon dominate much of Alaska, and include boreal forest, boreal peatlands, and seagrasses (Goldstein et al., 2020). Even partial “carbon recovery-ability” in the boreal and temperate peatlands could take millennia (Leifeld and Menichetti, 2018).
In a recent global analysis of remaining intact habitats and essential places to conserve to stabilize climate and avoid species extinctions, 93.6% of Alaska’s lands were identified as essential (Dinerstein et al., 2020). Besides the existing protected areas (IUCN Category I-VI designations), the role of intact areas and high carbon storage areas were central to Alaska featuring prominently in this analysis (Dinerstein et al., 2020). Intact large mammal assemblages-places where rosters of the historically present (as of AD 1500) large mammals still remain-occur across much of Alaska (Morrison et al., 2007; Dinerstein et al., 2020). Presence of healthy populations of large mammals contributes to healthy ecosystem functioning and carbon storage (Ripple et al., 2014; Cromsigt et al., 2018). Conserving and restoring robust populations of large mammals that occur in Alaska is an opportunity to maximize carbon storage, as well as to confer other benefits that the presence of large mammals brings for Indigenous, local, and visiting human communities (Colt, 2001; Colt et al., 2002; Chapin et al., 2004; Wolfe, 2004; Ballew et al., 2006).
Though this paper primarily focuses on synthesizing information on terrestrial systems, coastal systems are also a key component of Alaska’s globally significant carbon storage capacity. Blue carbon refers to the carbon stored in coastal wetlands and estuaries. Seagrass and salt marshes can store up to 2.5 times the buried carbon as terrestrial boreal and temperate forests (Mcleod et al., 2011). Salt marshes and seagrass estuaries are losing up to 7% of their global distribution on an annual basis, however, most of the coastal seagrass estuaries in Alaska remain intact (Short et al., 2007). Alaska has among the largest eelgrass beds in the world (Ward et al., 1997). Though the blue carbon potential of peatlands, wetlands, and coastal estuaries has been studied in the Pacific coast region of Washington State (Kauffman et al., 2020), no such studies have been completed for Alaska.
Carbon Emission Risks in Alaska
Wildfire is the dominant driver of ecosystem change in much of Alaska, particularly the boreal region, and is strongly linked to climate. The area that burns in Alaska has increased significantly in recent decades (Kasischke et al., 2010), and points to the importance of accounting for the potential changes in the Alaska fire regime with respect to carbon storage and fluxes. Recent large burns put Alaska as contributing to approximately half of United States fire carbon emissions in certain years (Veraverbeke et al., 2015, 2017). As the changes in future climate will affect the landscape configuration of vegetation types throughout fire-initiated secondary succession, there are potential feedbacks to the fire regime and hence differences in modeled projections (Wolken et al., 2011; Zhu and McGuire, 2016). In some cases, such as in white spruce forests of south-central and interior Alaska, old forests are better able to withstand fire than young forests due to their higher water content, taller canopies and thicker bark. However, in black spruce forests, old stands are highly fire prone. The extent to which mature forest stands can escape or withstand fire and other disturbances will therefore be a key factor in determining their near-term climate-change resilience. Hotter and more intense fires are also likely to burn more of the organic layer, further increasing emissions.
Despite short-term releases of carbon due to more intense and more frequent fires, shifts in dominant plant species catalyzed by severe fire could mitigate feedback to climate warming if future deciduous-dominated boreal forests are managed to reduce fire activity. Fast-growing deciduous trees that replace slow-growing black spruce following severe boreal forest burns has been shown to result in a net increase in carbon storage by a factor of five over the disturbance cycle (Mack et al., 2021).
A thorough review of baseline and projected future carbon storage and greenhouse-gas fluxes in Alaska was done by Zhu and McGuire (2016). They found that across upland and wetland ecosystems, Alaska will likely see substantial increases in carbon sequestration potential between now and 2099. This is due primarily to increases in net primary productivity and is predicted despite likely and substantial increases in carbon emissions due to wildfire. The temperate forests in south-central and southeast coastal Alaska, which store significant carbon in live and dead tree biomass, are also projected to increase storage potential. Susceptibility to climate change in lowland shrub tundra ecotypes is significant due to large amounts of soil organic carbon becoming available for loss by decomposition. It should be noted, however, that systems that are more intact and less disturbed are expected to maintain higher carbon storage levels (Martin and Watson, 2016).
Alaska’s Importance in Climate Adaptation and Resilience
Beyond biodiversity and carbon storage sequestration, Alaska’s intact ecosystems provide other essential ecosystem functions such as regulating hydrological regimes or buffering against major storm events. These systems provide services directly essential to human health, including provisioning of clean air and water. Globally, intact ecosystems are especially important for many Indigenous communities (Watson et al., 2018; Plumptre et al., 2019). In Alaska, dependence on wild resources is cultural, social, and economic across communities. Rural residents harvest about 18,000 tons of wild foods each year, with fish making up about 56 percent of this harvest5. Rural communities in Alaska are geographically isolated by waterways, varied and steep terrain, and lack of road infrastructure, and hence are particularly reliant on wild foods.
For Indigenous peoples in particular, wild foods such as salmon are a central facet of culture and social relationships as well as subsistence6. Because of the conjunction of widespread intact ecosystems with Indigenous communities retaining their strong connections to place-based culture, Alaska provides opportunities for supporting healthy relationships between cultures, communities, and ecosystems that are rare in a global context (Fernández-Llamazares et al., 2021; Thornton and Moss, 2021). Even elsewhere in the temperate rainforests of the Pacific Northwest, many critical resources for these communities have become dramatically reduced in abundance, negatively impacting the resilience of human communities (e.g., Benner et al., 2021). Salmon, herring, and other marine foods are iconic in this regard (Thornton and Moss, 2021), but access to a diverse array of terrestrial and marine plant and animal foods contribute to nutritional health, cultural continuity, and resilience to modern crises (Moss, 1993; Hunn et al., 2003; Fernández-Llamazares et al., 2021).
Ecologically, Alaska could provide key refugia for species whose distributions will shift northward under climate change. Southeast Alaska will, for example, provide key refugia for temperate rainforest communities of the Pacific Coast (DellaSala et al., 2015), and future habitat for boreal species that presently occur further south and east (Stralberg et al., 2015). Land management decisions will affect how species, ecosystems and their services, and the people of Alaska and the nation as a whole, will adapt to climate-related changes. Conservation and informed management of areas of high refugia potential may help species and ecosystems persist, facilitate adaptation to new conditions, and allow for proactive management. This is consequential as many land managers are struggling to keep up with the accelerating consequences of climate change (Stralberg et al., 2020). Intact systems and the robust populations of species that occur in them can be viewed as an insurance policy against having to address the problems and associated costs that occur in trying to manage at-risk species in altered habitat in the future.
Leveraging the relatively intact nature of the Kenai peninsula in Alaska, the Kenai National Wildlife Refuge (KNWR) has been actively promoting proactive management to protect future anticipated ecosystem function (Magness and Morton, 2017). Leveraging climate envelope models developed for the region, managers at the KNWR have developed a portfolio approach that works with climate change trajectories to promote species more suited to future anticipated climates (Magness and Morton, 2018). Directing ecosystem change, in conjunction with resisting or accepting ecosystem change, is more attainable in intact ecosystems (Thompson et al., 2021).
Alaska is home to many of the last remaining intact and largely unaltered Pacific salmon (genus Oncorhynchus) producing ecosystems. The persistence of salmon and their supporting habitats has sustained the connection between Alaskans and salmon for over 10,000 years (Halffman et al., 2015). In contemporary times, salmon support multi-billion industries in Alaska through harvest by commercial fishermen and recreational anglers (Johnson et al., 2019). Salmon are harbingers of climate change and are increasingly observed in Arctic regions where Indigenous Peoples have little or no experience interacting with salmon (Dunmall et al., 2013) and where community reception to the newcomers is mixed (Carothers et al., 2019). Although Alaska is in a prolonged period of low abundance of the large-bodied and iconic Chinook salmon (O. tshawytscha), the numbers and biomass of wild pink salmon, chum salmon, and sockeye salmon in the North Pacific Ocean are as high as they have been since the 1920s (Ruggerone and Irvine, 2018).
Evidence points to major ocean changes as the primary drivers of these shifts in abundance (e.g., Cunningham et al., 2018), though changes in streamflow and freshwater discharge also play a role. Restoring and maintaining intact landscapes and flexible options for salmon to adapt in the future will determine where and how salmon are distributed across the state moving forward. In the rainforest region of Southeast Alaska, it is a priority to understand the efficacy of forest restoration from historical logging and if and how this can improve. For example, restoration and management to buffer against higher frequency flooding events, drought, and variability has been prioritized by disciplinary experts (Shanley et al., 2015). Maintaining flexible options for salmon—by maintaining a diversity of habitats and keeping these as intact as possible—will increase the likelihood that salmon productivity can be maintained despite large-scale shifts that will occur (Cline et al., 2017; Schoen et al., 2017).
Mitigating climate change and maintaining flexible options into the future will be essential to minimize forced migration of human populations as climate refugees, loss of access to food resources, and other uncertain risks (Hamilton et al., 2016). This is particularly important in Alaska as communities deriving significant elements of their daily needs from subsistence economies and ecologies are particularly at risk from climate change (Savo et al., 2016). In Alaska, climate change could add $5–6 billion to future costs for public infrastructure from now to 2080 (Larsen et al., 2008; Melvin et al., 2016). A recent report estimates that some of the most certain consequences of climate change in Alaska will cost between $340 million and $700 million per year over the next three to five decades (Berman and Schmidt, 2019), and this estimate included only a limited set of costs. Such costs may be borne by United States taxpayers more generally as well as Alaskans. Rural Alaskans will be disproportionately affected by changes to subsistence harvest cycles and reduced barge service as rivers become too shallow. Implementing plausible adaptation strategies could offset impacts by up to 45% over the long-run (Larsen et al., 2008).
The Importance of Alaska to a United States Strategy for Climate Stabilization and Biodiversity Conservation
The sheer size of Alaska and proportion of intact systems, much of which is public land, makes Alaska a singularly important and opportune place for the United States to consider natural climate solutions within its own borders. Approximately 60% (900,000 km2 of 1.5 M km2) of Alaska’s terrestrial surface area is federal land (Figure 1). Nearly 2/3 of this is within an IUCN protected category I-VI, with management ranging from protections for wildlife and recreational values [e.g., Fish and Wildlife Service (FWS) Refuges, National Park Service (NPS)], to managing lands for some oil and gas, mineral, and timber development [e.g., United States Forest Service (USFS), Bureau of Land Management (BLM)]. The State of Alaska manages another 100 million acres (404,685 km2), an area the size of the State of California, for an equally wide range of purposes. Together, 88% of Alaska is managed either by the federal or state government. No United States state has a larger amount of public lands nor the combined intactness, carbon storage, and native biodiversity importance of Alaska.
As noted, ANCSA, enacted in 1971, transferred 44 million acres (178,062 km2) of land (nearly 12% of the State) to newly created Alaska Native regional and village corporations. These lands are managed for the benefit of the corporations’ Alaska Native shareholders. In addition, the Alaska National Interest Lands Conservation Act (ANILCA), passed in 1980, provided varying protections to 157 million acres of land set aside as National Parks, National Forests, and other conservation areas. It also established subsistence priority in hunting, fishing, and gathering activities for rural residents, Alaska Native or otherwise, stating that, “the continuation of the opportunity for subsistence is essential to Native physical, economic, traditional, and cultural existence.” Roughly 80% of the population of remote rural Alaska residents are Indigenous People7, and federal and state public lands comprise much of the traditional territory of Alaska’s 229 federally recognized tribes—creating an unusual opportunity for a small number of landowners and sovereign tribes to work together on coordinated land management strategies across large regions of the state. Only about 1% of Alaska is in general private land ownership.
Many intact systems in Alaska face a variety of proposed industrial land uses that either increase carbon emissions through the extraction and production of fossil fuels or diminish carbon storage values and system intactness through degradation or conversion of natural areas. Among these are expanded fossil fuel development in the Brooks Foothills and Beaufort Coastal Plain (Figure 6), clear-cut old-growth logging in forest systems in southeast Alaska, and large-scale, fossil-fuel based mining operations and associated infrastructure in otherwise intact regions such as the Bristol Bay watershed. Land conversion from forest to agriculture in Alaska’s boreal region would be likely to result in net carbon release (Grünzweig et al., 2004). In Canada, agricultural conversion is the greatest source of historical loss of boreal forests, and remains an important driver of deforestation in some boreal zones (Hobson et al., 2002). Such conversion is not however, occurring at anything approaching large scales in Alaska (Figure 3).
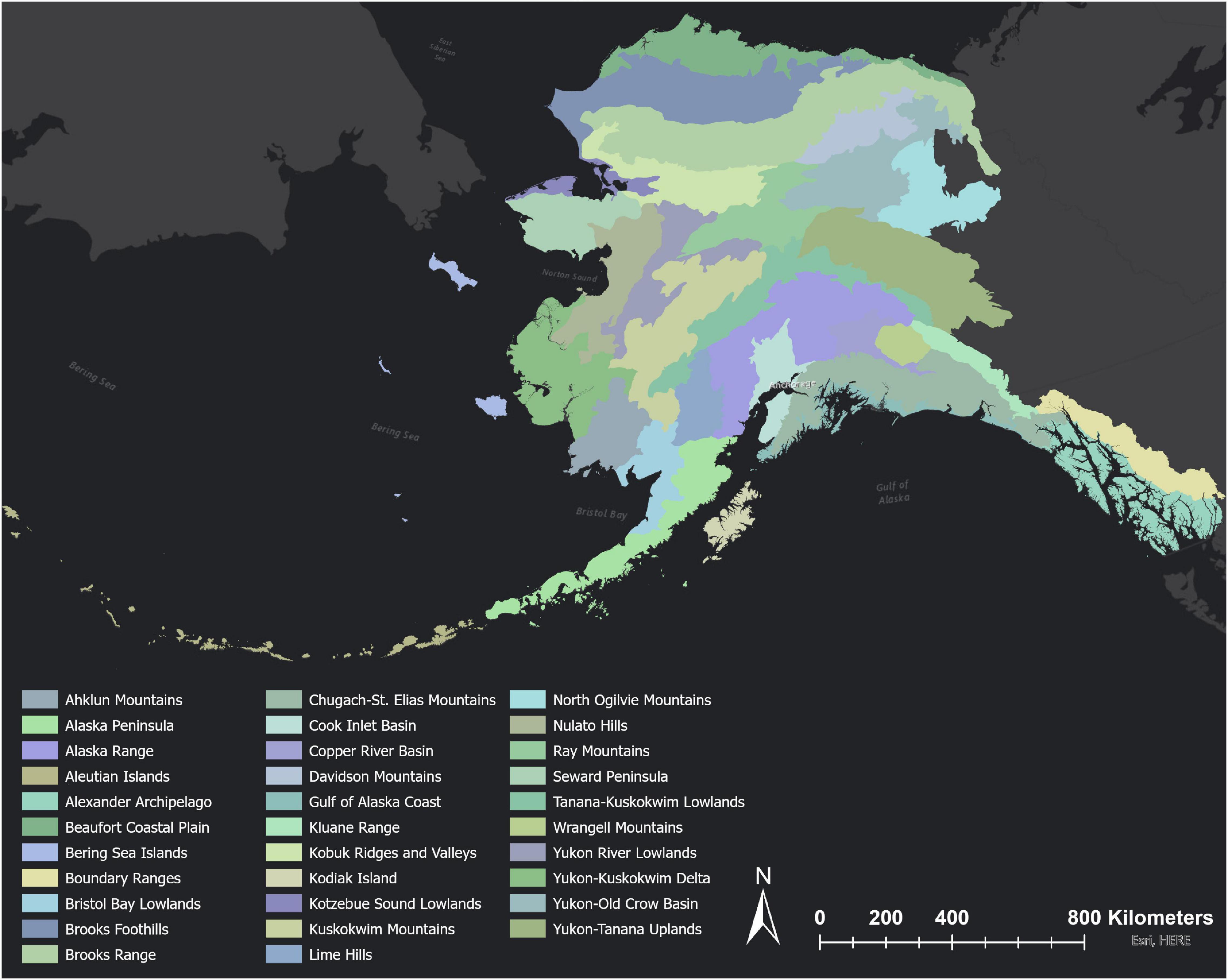
Figure 6. Ecoregions of Alaska [from Nowacki et al. (2003)].
Recommendations
While the tundra, forests, and other ecosystems of Alaska all face some degree of risk, there is potential for these to be managed through local land-use decisions and actions (Grünzweig et al., 2004; Goldstein et al., 2020). Managing for climate stabilization and adaptation benefits in Alaska may provide an opportunity to scale land conservation and augment management on the rapid timescale that is necessary to meet global climate and biodiversity objectives (Dinerstein et al., 2019). Land management has a significant role to play in how Alaska’s systems, species, and people contribute to climate stabilization and adapt to climate change. For example, forest carbon storage potentially could triple with management focused on limiting harvest in the high biomass forests of Southeast Alaska (Zhu and McGuire, 2016). Further, processes internal to an ecosystem can lead to a decoupling from regional temperature and moisture regimes, and relatively undisturbed systems can confer resistance to climate change (Stralberg et al., 2020). Management of fire in Alaska has also been suggested as a potentially important climate mitigation strategy, as well as being important to directly protect human communities (Phillips et al., 2019). New evidence that shifting species dominance following severe burns in boreal forests is important as this has the potential to reduce likelihood of future fires, increase the tenure of this carbon on the landscape, and provide negative or stabilizing feedback to climate warming (Mack et al., 2021).
The singular opportunity that Alaska’s relatively intact systems present, particularly the irreplaceability of its accumulated carbon stores, merits consideration of an approach to the management of Alaska’s public lands that prioritizes climate stabilization, adaptation, and resilience. Such an approach might include a wide range of management actions. The identification and designation of areas important for climate stabilization and resilience could provide an opportunity to increase the use of nature-based approaches to mitigating climate change. These designations could include large intact areas and areas that are essential carbon storehouses, particularly vital in climate mitigation, and which could be managed to maintain and enhance conditions (e.g., vegetative cover, soil condition) for carbon storage and sequestration. Co-developing ideas and means to manage these areas with local people, particularly federally recognized tribes, could also increase stewardship and the efficacy of these areas in meeting not only conservation and climate objectives but also local economic, social, and cultural concerns.
Though additional IUCN-defined protected areas (categories I-VI) may be warranted in specific places, climate stabilization designations may simply shape management plans in a way that maximizes carbon storage and biodiversity conservation. Achieving expanded conservation objectives may also be achieved through other effective area-based conservation measures (OECMs) and Indigenous and Community Conserved Areas (ICCAs; Wells et al., 2020). Long-term or indefinite-termed withdrawal of areas of public land and waters from specific activities that directly contribute to emissions (e.g., expanded fossil fuel development) or that diminish carbon storage, intact systems, and biodiversity values through significant degradation or conversion of natural areas (e.g., clear-cut old growth logging and large-scale hard rock mining) could be considered (Wells et al., 2020). Such targeted withdrawals provide near-term biodiversity and climate stabilization benefits while still meeting other human needs and retaining the option to adjust management in the future.
Targeted withdrawals or designations may be area-based or they may be ownership-based, such as withdrawals of categories of federally-owned or managed lands and waters from fossil fuel extraction, for example. The Northern Bering Sea Climate Resilience Area8 (NBSCRA) is an example of an area-based, targeted withdrawal of oil extraction activities. This designation, created in partnership between regional Indigenous entities, including over 70 federally recognized tribes, and the federal government, was also an example of a collaboratively-developed designation that increases authority, recognition, flexibility, and benefits to local stakeholders, particularly Indigenous peoples. Notably, the NBSCRA required for the first time that Indigenous knowledge be included in federal decision-making. Co-development of climate stabilization and resilience designations and approaches with federally-recognized tribes could provide a means of recognizing tribal sovereignty, tapping Indigenous knowledge and governance, addressing the needs of Indigenous communities, and achieving climate and biodiversity objectives in an effective and socially just manner (Artelle et al., 2019). Designations might also include or be complemented by programs designed to be expanded to ensure multiple resource management benefits–such as water and food security and economic and educational opportunities–for resource users.
Prioritization of a climate stabilization, adaptation, and resilience approach may also be attractive to Alaska Native corporate landowners if the benefits to shareholders were to outweigh the benefits of resource extraction that diminishes carbon storage and biodiversity conservation but currently provides needed economic activity. Sealaska, the regional Alaska Native corporation for Southeast Alaska, established a carbon bank of 165,000 acres (667.7 km2) of its lands in Southeast Alaska. No commercial timber harvest will occur on those lands for 100 years. Federal programs could be designed in partnership with Alaska Native corporate landowners, federally-recognized tribes, and Alaskan communities that complement market-based actions and further incentivize climate stabilization and resilience investments on Alaska Native corporate lands and federal lands. These programs could also provide economic opportunities related to stewardship of carbon, food security, and other ecosystem services. Similarly, federal, state, and private partnerships may incentivize state climate stabilization investments and enable coordinated climate stabilization strategies over intact systems with fragmented land ownership.
Based on the information synthesized above, such an approach in Alaska should consider:
1. Maintaining intactness values: evaluate costs and benefits of developing industrial projects in intact areas as maintaining intact landscapes helps mitigate climate change as well as buffer its impacts.
2. Promoting proforestation—letting forests grow—as a forest management strategy and a component of ecosystem-based management.
3. Conserving forests with old trees.
4. Together with local communities, addressing and developing wildfire response strategies and stewardship and restoration strategies that promote carbon storage.
5. Prioritizing conservation and restoration of intact large mammal faunas and healthy salmon streams; presence of viable populations of these species confer systems-level carbon storage, and a myriad of other benefits for people and nature.
A climate stabilization and resilience approach could also involve greater focus of public resources and funding for a research agenda to better inform land managers in stewarding Alaska’s systems. Such an approach should also facilitate greater support and funding for Indigenous-led research and co-production of knowledge with Indigenous Peoples. According to a recent report assessing natural climate solutions across the United States, priority areas to maintain in Alaska comprise 29% of total carbon storage across all ecosystems, and are extremely important in terms of the United States commitment to draw down greenhouse gas emissions9. Filling key research gaps, particularly related to how the role of wildfire and ecosystem shifts may affect the carbon balance in Alaska, is particularly important. We elaborate on this and other research priorities in the next section.
Research Priorities
Indigenous-led research and research needs prioritized by Indigenous Peoples in Alaska should be a central component of research priorities. Collaboration with Indigenous knowledge-holders is consistent with internationally recognized Indigenous rights and generates more impactful research outcomes (Ban et al., 2018). Such cooperation among scientific and Indigenous perspectives will both improve understanding of system dynamics and better inform both scenario planning and the design of any climate stabilization and resilience approach to Alaska’s lands and waters.
Fire is a key ecosystem driver across the boreal region of Alaska. In this century, it has increased in range, with more frequent fires occurring further north, west, and south, as compared to historical records. Fire has also increased in frequency (leading to shorter average fire return intervals), intensity and severity, leading to more complete burning of biomass in trees and in soils, associated greater losses in carbon, and slower post-fire recouping and regenerating (Turetsky et al., 2011). Model results suggest that the boreal forest is particular at risk of carbon losses via fire, and that changes in fire regime will cause net carbon loss from deep organic soils and near−surface permafrost (O’Donnell et al., 2011; Genet et al., 2013). Carbon is lost to the atmosphere not only during fire events, but for years afterward, as well—but this effect is poorly understood. Postfire changes in absorption of solar radiation and organic decay in soils alter rates of carbon turnover in boreal forests. These post-fire releases may have been vastly underestimated, in part due to poor understanding of “mass wasting” and carbon decomposition in the mineral soil layer in the 2 years post-fire (O’Neill et al., 2003; Potter, 2018). The magnitude of these changes, their overall impact on carbon balance, and the practical ability of human management strategies to alter their trajectory all merit additional research and modeling.
While it has already been noted that the increased temperatures and permafrost thaw associated with past and ongoing climate change have already accelerated carbon loss from soils via decomposition, the magnitude of past and future carbon losses are far from clear—as are potential management strategies that might limit or mitigate such losses. Decay rates and rates of carbon loss are temperature sensitive and occur differently at different depths and locations. More data are needed on the effects of climate change on soil organic carbon in boreal permafrost soils, especially from depths greater than one meter (Kane et al., 2005; Johnson et al., 2011). A meta-analysis of existing literature on the anticipated response of Alaska’s ecosystems to climate change is a research priority. Synthesizing scientific conclusions and quantifying how projected changes are likely to influence boreal forest and tundra ecosystems, in particular, would benefit the research community and land managers. This meta-analysis could help inform a quantitative assessment of the potential for Alaska’s natural climate solutions to help the United States meet national and global climate commitments, as was recently assessed for Canada (Drever et al., 2021).
Ecological shifts have seen some recent research, but much remains to be examined with regard to the impacts of shrub encroachment in tundra systems and increases in forest biomass in areas previously sparsely forested, particularly on land areas where permafrost has recently thawed (Loranty et al., 2018). Complex patterns of soil drainage, currently unmapped for the state, add to the difficulties of predicting these land cover changes. Of particular importance is to understand and monitor how shrub species in tundra ecosystems will vary in response to climate and environmental change, and how herbivores and their shifting distributions may limit this change (Myers-Smith et al., 2011). Filling data gaps so that analyses such as that of Fargione et al. (2018) on natural climate solutions for the United States may be completed for Alaska is a priority. Similarly, filling gaps and completing mapping and inventorying of blue carbon networks is also a pressing research priority so that Alaska’s blue carbon potential can be adequately assessed.
The ecoregions of Alaska have many close parallels in the ecoregions of Canada, particularly with regard to permafrost tundra, vast boreal forests underlain with discontinuous permafrost southern boreal forests (aspen parkland), and temperate coastal rainforests (Figure 6). While in some cases the management of these lands has been similar in Alaska and in Canada, in many cases there have been key differences. For example, in Canada, there has been a policy of active clear-cutting and regeneration (large-scale forestry) in much of the boreal zone, while the same has not been true in Alaska. Research focused on the differing outcomes of these differing strategies, with regard to carbon storage and uptake, is a little-explored avenue. Another parallel is a shift from large-scale clearcutting in coastal rainforests of British Columbia to ecosystem-based management. Most of the coastal rainforest of British Columbia’s central and north coast—which are ecologically similar to the rainforests of southeast Alaska—are now managed under an ecosystem-based regime.
Besides basic research, there is a need to conduct more work on scenario planning to inform policy-making and management. Scenario planning can identify opportunities to shift land management and to meet desired states, or to avoid, mitigate, or enhance certain outcomes. Geodesign is one potential approach to move this type of integrated carbon futures assessment forward. Based upon deep stakeholder engagement (Steinitz, 2012), geospatial science, and scenarios that are socially and environmentally plausible (Trammell et al., 2018), geodesign futures can identify priority landscapes for a range of carbon storage solutions. Currently, the International Geodesign Collaborative is exploring the feasibility of the Trillion Trees Initiative using the geodesign principles of stakeholder-informed landscape planning. Regardless of the tool, the early involvement of local communities and stakeholders in identifying options for developing a climate stabilization and adaptation approach is likely to result in stronger, more durable outcomes for both biodiversity conservation and community wellbeing (Salomon et al., 2018). Additionally, and as information becomes available, quantitative assessments of how nature-based solutions can contribute to sequestration targets will be helpful to help guide investments and decision-making.
Discussion
Avoiding the worst effects of climate change requires rapid decarbonization and improved ecosystem stewardship. Alaska, with its intact ecosystems and ecoregions with full rosters of native species—many with relatively healthy populations—provides a globally exceptional opportunity for protecting biodiversity in a context linked to natural climate solutions. As climate warms, intact systems will become more valuable as they have greater potential to provide superior resilience to climate change and will help buffer against frequent droughts and other disturbance events. Analysis of vegetation cover data for Alaska shows that significant changes are occurring, with implications for both the biodiversity and carbon storage potential of the nation. Natural climate solutions offer win-win solutions to the linked crises of biodiversity loss, accompanying cultural and traditional losses, and climate change. Managing lands for climate stabilization, adaptation, and resilience provides an opportunity to prevent degradation of ecosystems and to increase the ability of ecosystems to remove CO2 from the atmosphere. Because Alaska is also globally significant for its large contribution to carbon storage, incentivizing land management to conserve and enhance this storage presents a significant opportunity for the United States to lead in natural climate solutions.
Quantification of the near-term contribution of various land management scenarios to climate emissions reduction and to long-term climate stabilization in Alaska is difficult due to significant data gaps. In 2018, researchers identified and analyzed 21 natural climate solutions in the contiguous United States and found that combined they could reduce global warming emissions by an amount equivalent to nearly 21% of United States net emissions in 2016 (Fargione et al., 2018). No such study has been performed for Alaska, which accounts for 19.3% of the United States land mass (US Census Bureau, 2012). Given the size and importance of Alaska’s lands and waters and the magnitude of its accumulated carbon stores and potential climate stabilization services over time, however, it is clear that Alaska must play a prominent role in any knowledge-based, United States climate stabilization strategy.
Design of a natural climate solutions approach for Alaska should consider both western-scientific and Indigenous perspectives and be developed within a framework that acknowledges the value of human-environment relationships in fostering ecosystem integrity and human well-being. The early involvement of local communities and stakeholders in identifying options is likely to result in stronger, more durable outcomes. In particular, collaboration with Alaska’s Indigenous communities, tribes, and landowners is necessary to develop an effective and socially just approach. Worldwide, there is a growing recognition that ecological knowledge and stewardship practices of Indigenous peoples can offer pathways for conservation and resources management that is not only effective but also socially just (Atlas et al., 2020). A stewardship approach that acknowledges the value of human-environment relationships in fostering ecosystem integrity and human well-being provides a framework for such collaboration (Chapin et al., 2015).
New research from the World Economic Forum ties half the world’s GDP—$44 trillion dollars—directly to nature and its services10. The recent COVID-19 crisis has demonstrated the ability of the world’s governments to mobilize trillions of dollars and there are a number of proposals emerging to tie environmental restoration and climate response to economic recovery. A climate stabilization and adaptation approach offers one framework to move beyond the incrementalism of protected area designation over the past couple of decades and to incentivize protection of intact lands that are already providing a myriad of benefits for biodiversity and humanity. Alaska, with its largely intact landscapes, wildlife values, and high carbon storage potential, is an opportunity for the United States to lead both in global climate and biodiversity conservation efforts.
Author Contributions
CV and ED conceived of and drafted the manuscript. NF, ND, AJ, BL, KL, SR, FS, and ET contributed to ideas, writing, and editing of the manuscript. AJ and ET produced the figures. All authors contributed to the article and approved the submitted version.
Funding
Funding was provided by Wilburforce Foundation and Campion Foundation as a grant to Alaska Venture Fund, a project of the New Venture Fund.
Conflict of Interest
CV was employed by company Osprey Insights LLC.
The remaining authors declare that the research was conducted in the absence of any commercial or financial relationships that could be construed as a potential conflict of interest.
Publisher’s Note
All claims expressed in this article are solely those of the authors and do not necessarily represent those of their affiliated organizations, or those of the publisher, the editors and the reviewers. Any product that may be evaluated in this article, or claim that may be made by its manufacturer, is not guaranteed or endorsed by the publisher.
Acknowledgments
We would like to thank Nikoosh Carlo, Colin Shanley, and Peter Wesley for their constructive comments on the draft manuscript.
Footnotes
- ^ https://www.bia.gov/regional-offices/alaska
- ^ www.coast.noaa.gov
- ^ www.fws.gov/alaska/pages/migratory-birds/shorebirds; accessed 1 October 2020
- ^ www.alaskasalmonandpeople.org
- ^ https://www.doi.gov/subsistence; accessed 19 November 2020
- ^ https://alaskasalmonandpeople.org; accessed 4 February 2021
- ^ https://iseralaska.org/static/legacy_publication_links/researchsumm/UA_RS10.pdf; accessed 4 February 2021
- ^ https://obamawhitehouse.archives.gov/the-press-office/2016/12/09/executive-order-northern-bering-sea-climate-resilience; accessed November 20, 2020
- ^ https://www.audubon.org/conservation/climate/naturalsolutions; accessed 9 July 2021
- ^ https://www.weforum.org/press/2020/01/half-of-world-s-gdp-moderately-or-highly-dependent-on-nature-says-new-report/
References
Artelle, K. A., Zurba, M., Bhattacharyya, J., Chan, D. E., Kelly, B., Hosty, J., et al. (2019). Supporting resurgent Indigenous-led governance: a nascent mechanism for just and effective conservation. Biol. Conserv. 240:108284. doi: 10.1016/j.biocon.2019.108284
Atlas, W. I., Ban, N. C., Moore, J. W., Tuohy, A. M., Greening, S., Reid, A. J., et al. (2020). Indigenous Systems of Management for Culturally and Ecologically Resilient Pacific Salmon (Oncorhynchus spp.) Fisheries. Bioscience. 71, 186–204 doi: 10.1093/biosci/biaa144
Ballew, C., Ross Tzilkowski, A., Hamrick, K., and Nobmann, E. D. (2006). The contribution of subsistence foods to the total diet of Alaska natives in 13 rural communities. Ecol. Food Nutr. 45, 1–26. doi: 10.1080/03670240500408302
Ban, N. C., Frid, A., Reid, M., Edgar, B., Shaw, D., and Siwallace, P. (2018). Incorporate Indigenous perspectives for impactful research and effective management. Nat. Ecol. Evol. 2, 1680–1683. doi: 10.1038/s41559-018-0706-0
Beck, P. S. A., Juday, G. P., Alix, C., Barber, V. A., Winslow, S. E., Sousa, E. E., et al. (2011). Changes in forest productivity across Alaska consistent with biome shift. Ecol. Lett. 4, 373–379 doi: 10.1111/j.1461-0248.2011.01598.x
Benner, J., Nielsen, J., and Lertzman, K. (2021). Using Traditional Ecological Knowledge to Understand the Diversity and Abundance of Culturally Important Trees. J. Ethnobiol. 41, 209–228.
Berman, M., and Schmidt, J. I. (2019). Economic effects of climate change in Alaska. Weather Clim. Soc. 11, 245–258. doi: 10.1175/WCAS-D-18-0056.1
Berner, L. T., Massey, R., Jantz, P., Forbes, B. C., Macias-Fauria, M., Myers-Smith, I., et al. (2020). Summer warming explains widespread but not uniform greening in the Arctic tundra biome. Nat. Commun. 11:4621. doi: 10.1038/s41467-020-18479-5
Betts, M. G., Wolf, C., Ripple, W. J., Phalan, B., Millers, K. A., Duarte, A., et al. (2017). Global forest loss disproportionately erodes biodiversity in intact landscapes. Nature 547, 441–444. doi: 10.1038/nature23285
Bradshaw, C. J. A., and Warkentin, I. G. (2015). Global estimates of boreal forest carbon stocks and flux. Glob. Planet. Change 128, 24–30. doi: 10.1016/j.gloplacha.2015.02.004
Brubaker, M., Berner, J., Chavan, R., and Warren, J. (2011). Climate change and health effects in Northwest Alaska. Glob. Health Action. 4:8445. doi: 10.3402/gha.v4i0.8445
Buotte, P. C., Law, B. E., Ripple, W. J., and Berner, L. T. (2020). Carbon sequestration and biodiversity co-benefits of preserving forests in the western United States. Ecol. Appl. 30:e02039. doi: 10.1002/eap.2039
Carothers, C., Sformo, T. L., Cotton, S., George, J. C., and Westley, P. A. (2019). Pacific salmon in the rapidly changing Arctic: exploring local knowledge and emerging fisheries in Utqiaġvik and Nuiqsut, Alaska. Arctic 72, 273–288. doi: 10.14430/arctic68876
Chapin, F. S. III, Peterson, G., Berkes, F., Callaghan, T. V., Angelstam, P., Apps, M., et al. (2004). Resilience and vulnerability of northern regions to social and environmental change. Ambio 33, 344–349. doi: 10.1579/0044-7447-33.6.344
Chapin, F. S. III, Sommerkorn, M., Robards, M. D., and Hillmer-Pegram, K. (2015). Ecosystem stewardship: a resilience framework for arctic conservation. Glob. Envir. Change 34, 207–217. doi: 10.1016/j.gloenvcha.2015.07.003
Cline, T. J., Schindler, D. E., and Hilborn, R. (2017). Fisheries portfolio diversification and turnover buffer Alaskan fishing communities from abrupt resource and market changes. Nat. Commun. 8:14042. doi: 10.1038/ncomms14042
Cochran, P., Huntington, O. H., Pungowiyi, C., Stanley, T., Chapin, F. S. III, Huntington, H. P., et al. (2013). “Indigenous frameworks for observing and responding to climate change in Alaska,” in Climate Change and Indigenous Peoples in the United States, eds J. K. Maldonado, B. Colombi, and R. Pandya (Cham: Springer).
Colt, S. (2001). The economic importance of healthy Alaska ecosystems. Anchorage: Institute of Social and Economic Research, University of Alaska Anchorage.
Colt, S., Martin, S., Mieren, J., and Tomeo, M. (2002). Recreation and tourism in south-central Alaska: patterns and prospects. Gen. Tech. Rep. PNW-GTR-551. Portland, OR: U.S. Department of Agriculture, Pacific Northwest Research Station.
Commane, R., Lindaas, J., Benmergui, J., Luus, K. A., Chang, R. Y.-W., Daube, B. C., et al. (2017). Carbon dioxide sources from Alaska driven by increasing early winter respiration from Arctic tundra. Proc. Natl. Acad. Sci. U. S. A. 114, 5361–5366. doi: 10.1073/pnas.1618567114
Cromsigt, J. P., te Beest, M., Kerley, G. I., Landman, M., Le Roux, E., and Smith, F. A. (2018). Trophic rewilding as a climate change mitigation strategy? Phil. Trans. R. Soc. A 373:20170440. doi: 10.1098/rstb.2017.0440
Cunningham, C. J., Westley, P. A. H., and Adkison, M. D. (2018). Signals of large scale climate drivers, hatcher enhancement, and marine factors on Yukon River Chinook salmon survival revealed with a Bayesian life history model. Glob. Chang. Biol. 24, 4399–4416. doi: 10.1111/gcb.14315
DellaSala, D. A., Brandt, P., Koopman, M., Leonard, J., Meisch, C., Herzog, P., et al. (2015). “Climate Change May Trigger Broad Shifts in North America’s Pacific Coastal Rainforests,” in Encyclopedia of the Anthropocene. Volume 2: Climate Change. Reference Module in Earth Systems and Environmental Sciences, eds D. A. DellaSala., and M. I. Goldstein Oxford, UK: Elsevier: 233–244. doi: 10.1016/b978-0-12-409548-9.09367-2
Dinerstein, E., Joshi, A., Vynne, C., Lee, A. T., Pharand-Deschênes, F., França, M., et al. (2020). A ‘Global Safety Net’ to Reverse Biodiversity Loss and Stabilize the Earth’s Climate. Sci. Adv. 6, 1–14. doi: 10.1007/978-3-030-25594-7_1
Dinerstein, E., Vynne, C., Sala, E., Joshi, A. R., Fernando, S., Lovejoy, T. E., et al. (2019). A Global Deal for Nature: guiding Principles. Milestones, and Targets. Sci. Adv. 5:eaaw2869. doi: 10.1126/sciadv.aaw2869
Domke, G., Williams, C. A., Birdsey, R., Coulston, J., Finzi, A., Gough, C., et al. (2018). “Chapter 9: Forests,” in In Second state of the Carbon Cycle Report (SOCR2): A Sustained Assessment Report, eds N. Cavallaro, G. Shrestha, R. Birdsey, M. A. Mayes, R. G. Rajjar, S. C. Reed, et al. (Washington, DC, U.S.A: U. S. Global Change Research Program), 365–398.
Drever, D. R., Cook-Patton, S. C., Akhter, F., Badious, P. H., Chmura, G. L., Davidson, S. J., et al. (2021). Natural climate solutions for Canada. Sci. Adv. 7:eabd6034.
Dunmall, K. M., Reist, J. D., Carmack, E. C., Babaluk, J. A., Heide-Jørgensen, M. P., and Docker, M. F. (2013). Pacific salmon in the Arctic: harbingers of change. Responses of arctic marine ecosystems to climate change. Fairbanks, Alaska, United: Alaska Sea Grant, University of Alaska Fairbanks, 141–160.
Fargione, J., Bassett, S., Boucher, T., Bridgham, S., Conant, R. T., Cook-Patton, S., et al. (2018). Natural Climate Solutions for the United States. Sci. Adv. 4:e1869.
Fernández-Llamazares, A., Lepofsky, D., Lertzman, K., Geralda Armstrong, C., Brondizio, E. S., Gavin, M. C., et al. (2021). Scientists’ Warning to Humanity on Threats to Indigenous and Local Knowledge Systems. J. Ethnobiol. 41, 144–171.
Food and Agricultural Organization. (2020). Global Forest Resources Assessment 2020 – Key findings. Rome: Food and Agricultural Organization, doi: 10.4060/ca8753en
Genet, H., McGuire, A. D., Barrett, K., Breen, A., Euskirchen, E. S., Johnston, F. F., et al. (2013). Modeling the effects of fire severity and climate warming on active layer thickness and soil carbon storage of black spruce forests across the landscape in interior Alaska. Environ. Res. Lett. 8:045009. doi: 10.1088/1748-9326/8/4/045016
Goetz, S. J., Bond-Lamberty, B., Law, B. E., Hicke, J. A., and Huang, C. (2012). Observations and assessment of forest carbon dynamics following disturbance in North America. J. Geophys. Res. 117, 1–17. doi: 10.1029/2011JG001733
Goldstein, A., Turner, W. R., Spawn, S. A., Anderson-Teixeira, K. J., Cook-Patton, S., Fargione, J., et al. (2020). Protecting irrecoverable carbon in Earth’s ecosystems. Nat. Clim. Chang. 10, 287–295. doi: 10.1038/s41558-020-0738-8
Graven, H. D., Keeling, R. F., Piper, S. C., Patra, P. K., Stephens, B. B., Wofsy, S. C., et al. (2013). Enhanced Seasonal Exchange of CO2 by Northern Ecosystems Since 1960. Science 341, 1085–1089. doi: 10.1126/science.1239207
Griscom, B. W., Adams, J., Ellis, P. W., Houghton, R. A., Lomas, G., Miteva, D. A., et al. (2017). Natural Climate Solutions. Proc. Natl. Acad. Sci. U. S. A. 114, 11645–11650.
Grünzweig, J. M., Sparrow, S. D., Yakir, D., and Chapin, F. S. III (2004). Impact of Agricultural Land-use Change on Carbon Storage in Boreal Alaska. Glob. Chang. Biol. 10, 452–472. doi: 10.1111/j.1365-2486.2004.00738.x
Hak, J. C., and Comer, P. J. (2017). Modeling landscape condition for biodiversity assessment - Application in temperate North America. Ecol. Indic. 82, 206–216. doi: 10.1016/j.ecolind.2017.06.049
Halffman, C. M., Potter, B. A., McKinney, H. J., Finney, B. P., Rodrigues, A. T., Yang, D. Y., et al. (2015). Early human use of anadromous salmon in North America at 11,500 y ago. Proc. Natl. Acad. Sci. U. S. A. 112, 12344–12348. doi: 10.1073/pnas.1509747112
Hall, J. V., Frayer, W. E., and Wilen, B. O. (1994). Status of Alaska Wetlands. Anchorage, Alaska: U.S. Fish and Wildlife Service Alaska Region.
Hamilton, L. C., Saito, K., Loring, P., Lammers, R. B., and Huntington, H. P. (2016). Climigration? Population and climate change in Arctic Alaska. Popul. Environ. 38, 115–133. doi: 10.1007/s11111-016-0259-6
Harris, G., Thirgood, S., Hopcraft, J. G. C., Cromsigt, J. P. G. M., and Berger, J. (2009). Global decline in aggregated migrations of large terrestrial mammals. Endang Species Res. 7, 55–76. doi: 10.3354/esr00173
Hinzman, L. D., Bettez, N. D., Bolton, W. R., Chapin, F. S. III, Dyurgerov, M. B., Fastie, C. L., et al. (2005). Evidence and Implications of Recent Climate Change in Northern Alaska and Other Arctic Regions. Clim. Change 72, 251–298.
Hobson, K. A., Bayne, E. M., and Van Wilgenburg, S. L. (2002). Large-Scale Conversion of Forest to Agriculture in the Boreal Plains of Saskatchewan. Conserv. Biol. 16, 1530–1541. doi: 10.1046/j.1523-1739.2002.01199.x
Hudiburg, T., Law, B. E., Turner, D. P., Campbell, J., Donato, D., and Duane, M. (2009). Carbon dynamics of Oregon and Northern California forests and potential land-based carbon storage. Ecol. Appl. 19, 163–180. doi: 10.1890/07-2006.1
Hugelius, G., Strauss, J., Zubrzycki, S., Harden, J. W., Schuur, E. A. G., Ping, C. L., et al. (2014). Estimated stocks of circumpolar permafrost carbon with quantified uncertainty ranges and identified data gaps. Biogeosciences 11, 6573–6593. doi: 10.5194/bg-11-6573-2014
Hunn, E., Johnson, D., Russell, P., and Thornton, T. (2003). Huna Tlingit Traditional Environmental Knowledge, Conservation, and the Management of a “Wilderness” Park. Curr. Anthropol. 44, S79–S103.
IPCC. (2018). Summary for Policymakers, Global Warming of 1.5°C. An IPCC Special Report on the impacts of global warming of 1.5°C above pre-industrial levels and related global greenhouse gas emission pathways, in the context of strengthening the global response to the threat of climate change, sustainable development, and efforts to eradicate poverty. Geneva, Switzerland: World Meteorological Organization
Johnson, A. C., Bellmore, J. R., Haught, S., and Medel, R. (2019). Quantifying the Monetary Value of Alaska National Forests to Commercial Pacific Salmon Fisheries. North Am. J. Fish. Manag. 39, 1119–1131. doi: 10.1002/nafm.10364
Johnson, K. D., Harden, J., McGuire, A. D., Bliss, N. B., Bockheim, J. G., and Clark, et al. (2011). Soil carbon distribution in Alaska in relation to soil-forming factors. Geoderma 167–168, 71–84. doi: 10.1016/j.geoderma.2011.10.006
Jones, M. C., Harden, J., O’Donnell, J., Manies, K., Jorgenson, T., Treat, C., et al. (2017). Rapid carbon loss and slow recovery following permafrost thaw in boreal peatlands. Glob. Chang. Biol. 23, 1109–1127. doi: 10.1111/gcb.13403
Jorgenson, M. T., Racine, C. H., Walters, J. C., and Osterkamp, T. E. (2001). Permafrost Degradation and Ecological Changes Associated with a WarmingClimate in Central Alaska. Clim. Change 48, 551–579. doi: 10.1023/A:1005667424292
Kane, E. S., Valentine, D. W., Schuur, E. A. G., and Dutta, K. (2005). Soil carbon stabilization along climate and stand productivity gradients in black spruce forests of interior Alaska. Can. J. For. Res. 35, 2118–2129. doi: 10.1139/x05-093
Kasischke, E. S., and Hoy, E. E. (2012). Controls on carbon consumption during Alaskan wildland fires. Glob. Chang. Biol. 18, 685–699. doi: 10.1111/j.1365-2486.2011.02573.x
Kasischke, E. S., Verbyla, D. L., Scott, R. T., Mcguire, A. D., Murphy, K. A., Randi, J., et al. (2010). Alaska ’s changing fire regime - implications for the vulnerability of its boreal forests. Can. J. For. Res. 40, 1313–1324. doi: 10.1139/x10-098
Kauffman, J. B., Giovanonni, L., Kelly, J., Dunstan, N., Borde, A., Diefenderfer, H., et al. (2020). Total ecosystem carbon stocks at the marine-terrestrial interface: blue carbon of the Pacific Northwest Coast, United States. Glob. Chang. Biol. 26, 5679–5692. doi: 10.1111/gcb.1524800
Kofinas, G. P., Chapin, F. S. III., BurnSilver, S., Schmidt, J. I., Fresco, N. L., Kielland, K., et al. (2010). Resilience of Athabascan subsistence systems to interior Alaska’s changing climate. Can. J. of For. Res. 40, 1347–1359. doi: 10.1139/x10-108
Larsen, P. H., Goldsmith, S., Smith, O., Wilson, M. L., Strzepek, K., Chinowsky, P., et al. (2008). Estimating future costs for Alaska public infrastructure at risk from climate change. Glob. Environ. Change 18, 442–457. doi: 10.1016/j.gloenvcha.2008.03.005
Law, B. E., Hudiburg, T. W., Berner, L. T., Kent, J. J., Buotte, P. C., and Harmon, M. (2018). Land use strategies to mitigate climate change in carbon dense temperate forests. Proc. Natl. Acad. Sci. U. S. A. 115, 3663–3668. doi: 10.1073/pnas.1720064115
Leifeld, J., and Menichetti, L. (2018). The underappreciated potential of peatlands in global climate change mitigation strategies. Nat. Commun. 9:1071.
Leighty, W. W., Hamburg, S. P., and Caouette, J. P. (2006). Effects of management on carbon sequestration in forest biomass in southeast Alaska. Ecosystem 9, 1051–1065. doi: 10.1007/s10021-005-0028-3
Lertzman, K., and MacKinnon, A. (2013). “Why Watersheds: Evaluating the Protection of Undeveloped Watersheds as a Conservation Strategy in Northwestern North America,” in Ecology and Conservation of North Pacific Rainforests, eds G. H. Orians and J. W. Schoen (Seattle, WA: University of Washington Press).
Locke, H., Ellis, E. C., Venter, O., Schuster, R., Ma, K., Shen, X., et al. (2019). Three global conditions for biodiversity conservation and sustainable use: an implementation framework. Nat. Sci. Rev. 6, 1080–1082. doi: 10.1093/nsr/nwz136
Loranty, M. M., Abbott, B. W., Blok, D., Douglas, T. A., Epstein, H. E., Forbes, B. C., et al. (2018). Reviews and syntheses: changing ecosystem influences on soil thermal regimes in northern high-latitude permafrost regions. Biogeoscience 15, 5287–5313. doi: 10.5194/bg-15-5287-2018
Lutz, J. A., Furniss, T. J., Johnson, D. J., Davies, S. J., Allen, D., Alonso, A., et al. (2018). Global importance of large-diameter trees. Glob. Ecol. Biogeogr. 27, 849–864.
Luyssaert, S., Schulze, E. D., Börner, A., Knohl, A., Hessenmöller, D., Law, B. E., et al. (2008). Old-growth forests as global carbon sinks. Nature 455, 213–215 doi: 10.1038/nature07276
Mack, M. C., Xanthe, W. J., Johnstone, J. F., Alexander, H. D., Melvin, A. M., et al. (2021). Carbon loss from boreal forest wildfires offset by increased dominance of deciduous trees. Science. 372, 280–283 doi: 10.1126/science.abf3903
Magness, D. R., and Morton, J. M. (2017). Implementing Portfolios of Adaptation Strategies on U.S. Conservation Lands in the Anthropocene, Reference Module in Earth Systems and Environmental Sciences. Amsterdam: Elsevier. doi: 10.1016/B978-0-12-409548-9.10504-4
Magness, D. R., and Morton, J. M. (2018). Using climate envelope models to identify potential ecological trajectories on the Kenai Peninsula, Alaska. PLoS One 13:e0208883. doi: 10.1371/journal.pone.0208883
Martin, T. G., and Watson, J. E. M. (2016). Intact ecosystems provide best defense against climate change. Nat. Clim. Change 6, 122–124. doi: 10.1038/nclimate2918
McGuire, A. D., Christensen, T. R., Hayes, D., Heroult, A., Euskirchen, E., Kimball, J. S., et al. (2012). An assessment of the carbon balance of Arctic tundra: comparisons among observations, process models, and atmospheric inversions. Biogeoscience 9, 3185–3204. doi: 10.5194/bg-9-3185-2012
Mcleod, E., Chmura, G. L., Bouillon, S., Salm, R., Bjork, M., Duarte, C. M., et al. (2011). A blueprint for blue carbon: toward an improved understanding of the role of vegetated coastal habitats in sequestering CO2. Front. Ecol. Environ. 10:552–560. doi: 10.1890/110004
Melvin, A. M., Larsen, P., Boehlert, B., Neumann, J. E., Chinowsky, P., Espinet, X., et al. (2016). Climate change damages to Alaska public infrastructure and the economics of proactive adaptation. Proc. Natl. Acad. Sci. U. S. A. 114, E122–E131. doi: 10.1073/pnas.1611056113
Merrill, M. D., Sleeter, B. M., Freeman, P. A., Liu, J., Warwick, P. D., and Reed, B. C. (2018). Federal lands greenhouse emissions and sequestration in the United States—Estimates for 2005–14. U.S. Geol. Survey Sci. Invest. Rep. 31, 2018–5131. doi: 10.3133/sir20185131
Mildrexler, D., Berner, L. T., Law, B. E., Birdsey, R. A., and Moomaw, W. R. (2020). Large trees dominate carbon storage east of the Cascade crest in the U.S. Pacific Northwest. Front. For. Clim. Change 3:594274. doi: 10.3389/ffgc.2020.594274
Moomaw, W. R., Masino, S. A., and Faison, E. K. (2019). Intact Forests in the United States: proforestation Mitigates Climate Change and Serves the Greatest Good. Front. For. Glob. Change 2:27. doi: 10.3389/ffgc.2019.00027
Moomaw, W., Law, B. E., and Goetz, S. (2020). Focus on the role of forests and soils in meeting climate change mitigation goals: summary. Environ. Res. Lett. 15:045009. doi: 10.1088/1748-9326/ab6b38
Morrison, J. C., Sechrest, W., Dinerstein, E., Wilcove, D. S., and Lamoreux, J. F. (2007). Persistence of Large Mammal Faunas as Indicators of Global Human Impacts. J. Mammal. 88, 1363–1380. doi: 10.1644/06-mamm-a-124r2.1
Moss, M. L. (1993). Shellfish, Gender, and Status on the Northwest Coast of North America: reconciling Archeological, Ethnographic and Ethnohistorical Records of the Tlingit. Am. Anthropol. 95, 631–652. doi: 10.1525/aa.1993.95.3.02a00050
Myers-Smith, I. H., Forbes, B. C., Wilmking, M., Hallinger, M., Lantz, T., Blok, D., et al. (2011). Shrub expansion in tundra ecosystems: dynamics, impacts and research priorities. Environ. Res. Lett. 6:045509. doi: 10.1088/1748-9326/6/4/045509
Nesbitt, H. K., and Moore, J. W. (2016). Species and population diversity in Pacific salmon fisheries underpin indigenous food security. J. Appl. Ecol. 53, 1489–1499. doi: 10.1111/1365-2664.12717
Nowacki, G. J., Spencer, P., Fleming, M., Brock, T., and Jorgenson, T. (2003). Unified Ecoregions of Alaska: 2001. Open-File Report. Virginia, U.S: Geological Survey (U.S.). doi: 10.3133/ofr2002297
O’Donnell, J. A., Harden, J. W., McGuire, A. D., Kanevskiy, M. Z., Jorgenson, M. T., and Xu, X. (2011). The effect of fire and permafrost interactions on soil carbon accumulation in an upland black spruce ecosystem of interior Alaska: implications for post-thaw carbon loss. Glob. Chang. Biol. 17, 1461–1474. doi: 10.1111/j.1365-2486.2010.02358.x
O’Neill, K. P., Kasischke, E. S., and Richter, D. D. (2003). Seasonal and decadal patterns of soil carbon uptake and emission along an age sequence of burned black spruce stands in interior Alaska. J. Geophys. Res 108:FFR 11-1–FFR 11-15. doi: 10.1029/2001JD000443
Orians, G., and Schoen, J. (2017). North Pacific Temperate Rainforests: Ecology and Conservation. Seattle: University of Washington Press.
Pan, Y., Chen, J. M., Birdsey, R., McCullough, K., He, L., and Deng, F. (2011). Age structure and disturbance legacy of North American forests. Biogeosciences 8, 715–732. doi: 10.5194/bg-8-715-2011
Parmentier, F. J. W., Christensen, T. R., Rysgaard, S., Bendtsen, J. Glud, R. N., Else, B., van Huissteden, J., et al. (2017). A synthesis of the arctic terrestrial and marine carbon cycles under pressure from a dwindling cryosphere. Ambio 46, 53–69. doi: 10.1007/s13280-016-0872-8
Phillips, C. A., Rogers, B. M., and Frumhoff, P. C. (2019). Managing boreal wildfires as a climate mitigation strategy. in AGU Fall Meeting Abstracts vol. 2019 B31B-03. Washington, DC: AGU.
Plumptre, A. J., Baisero, D., Jędrzejewski, W., Kühl, H., Maisels, F., Ray, J. C., et al. (2019). Are We Capturing Faunal Intactness? A Comparison of Intact Forest Landscapes and the “Last of the Wild in Each Ecoregion”. Front. For. Glob. Change 2:24. doi: 10.3389/ffgc.2019.00024
Potter, C. (2018). Ecosystem carbon emissions from 2015 forest fires in interior Alaska. Carbon Balance Manag. 13:2. doi: 10.1186/s13021-017-0090-0
Quinn, T. P., Helfield, J. M., Austin, C. S., Hovel, R. A., and Bunn, A. G. (2018). A multidecade experiment shows that fertilization by salmon carcasses enhanced tree growth in the riparian zone. Ecology 99, 2433–2441. doi: 10.1002/ecy.2453
Reynolds, J. H., Trammell, E. J., and Taylor, J. J. (2018). Migration’s Foundation: ecological Intactness of Alaska’s Ecosystems. Alaska Park Sci. 17, 85–91.
Ripple, W. J., Estes, J. A., Beschta, R. L., Wilmers, C. C., Ritchie, E. G., Hebblewhite, M., et al. (2014). Status and Ecological Effects of the World’s Largest Carnivores. Science 343, 151–163.
Ripple, W. J., Newsome, T., Wolf, C., Dirzo, R., Everatt, K. T., Galetti, M., et al. (2015). Collapse of the world’s largest herbivores. Sci. Adv. 1:e1400103. doi: 10.1126/sciadv.1400103
Ruff, C. P., Schindler, D. E., Armstrong, J. B., Bentley, K. T., Brooks, G. T., Holtgrieve, G. W., et al. (2011). Temperature-associated population diversity in salmon confers benefits to mobile consumers. Ecology 92, 2073–2084. doi: 10.1890/10-1762.1
Ruggerone, G. T., and Irvine, J. R. (2018). Numbers and biomass of natural-and hatchery-origin pink salmon, chum salmon, and sockeye salmon in the north Pacific Ocean, 1925–2015. Mar. Coast. Fish. 10, 152–168. doi: 10.1002/mcf2.10023
Salomon, A. K., Lertzman, K., Brown, K., Wilson, K. B., Secord, D., and McKechie, I. (2018). Democratizing conservation science and practice. Ecol. Soc. 23:44.
Savo, V., Lepofsky, D. S., Benner, J. P., Kohfeld, K. E., Bailey, H. J., and Lertzman, K. P. (2016). Observations of climate change among subsistence-oriented communities around the world. Nat. Clim. Change 6, 462–473. doi: 10.1038/nclimate2958
Schindler, D. E., Hilborn, R., Chasco, B., Boatright, C. P., Quinn, T. P., Rogers, L. A., et al. (2010). Population diversity and the portfolio effect in an exploited species. Nature 465, 609–612. doi: 10.1038/nature09060
Schoen, E. R., Wipfli, M. S., Trammell, E. J., Rinella, D. J., Floyd, A. L., Grunblatt, J., et al. (2017). Future of Pacific Salmon in the Face of Environmental Change: lessons from One of the World’s Remaining Productive Salmon Regions. Fisheries 42, 538–553. doi: 10.1080/03632415.2017.1374251
Schuur, E. A. G., McGuire, A. D., Romanovsky, V., Schädel, C., and Mack, M. (2018). “Chapter 11: Arctic and boreal carbon,” in Second State of the Carbon Cycle Report (SOCCR2): A Sustained Assessment Report, eds N. Cavallaro, G. Shrestha, R. Birdsey, M. A. Mayes, R. G. Najjar, S. C. Reed, et al. (Washington, DC, U.S.A: U.S. Global Change Research Program), 428–468.
Seddon, N., Chausson, A., Berry, P., Girardin, C. A. J., Smith, A., and Turner, B. (2020). Understanding the value and limits of nature-based solutions to climate change and other global challenges. Phil. Trans. R. Soc. B 375:1794. doi: 10.1098/rstb.2019.0120
Shanley, C. S., Pyare, S., Goldstein, M. I., Alaback, P. B., Albert, D. M., Beier, C. M., et al. (2015). Climate change implications in the northern coastal temperate rainforest of North America. Clim. Change 130, 155–170. doi: 10.1007/s10584-015-1355-9
Short, F., Carruthers, T., Dennison, W., and Waycott, M. (2007). Global seagrass distribution and diversity: a bioregional model. J. Exp. Mar. Biol. Ecol. 350, 3–20. doi: 10.1016/j.jembe.2007.06.012
Soto-Navarro, C., Ravilious, C., Arnell, A., de Lamo, X., Harfoot, M., Hill, S. L. L., et al. (2020). Mapping co-benefits for carbon storage and biodiversity to inform conservation policy and action. Phil. Trans. R. Soc. B Biol. Sci. 375:20190128. doi: 10.1098/rstb.2019.012
Steinitz, C. (2012). A framework for geodesign: Changing geography by design. Redlands, CA: ESRI, 208.
Stephenson, N. L., Das, A. J., Condit, R., Russo, S. E., Baker, P. J., Beckman, N. J., et al. (2014). Rate of tree carbon accumulation increases continuously with tree size. Nature 507, 90–93.
Stone, R. S., Dutton, E. G., Harris, J. M., and Longenecker, D. (2002). Earlier spring snowmelt in northern Alaska as an indicator of climate change. J. Geophys. Res. 107:4089.
Stralberg, D., Arseneault, D., Baltzer, J. L., Barber, Q. E., Bayne, E. M., Boulanger, Y., et al. (2020). Climate-change refugia in boreal North America: what, where, and for how long? Front. Ecol. Environ. 18:261–270. doi: 10.1002/fee.2188
Stralberg, D., Bayne, E. M., Cumming, S. G., Sólymos, P., Song, S. J., and Schmiegelow, F. K. A. (2015). Conservation of future boreal forest bird communities considering lags in vegetation response to climate change: a modified refugia approach. Divers. Distrib. 21, 1112–1128. doi: 10.1111/ddi.12356
Sun, O., Campbell, J., Law, B. E., and Wolf, V. (2004). Dynamics of carbon stocks in soils and detritus across chronosequences of different forest types in the Pacific Northwest. U.S.A. Glob. Change Biol. 10, 1470–1481. doi: 10.1111/j.1365-2486.2004.00829.x
Sutherland, I. J., Gergel, S. E., and Bennett, E. M. (2016). Seeing the forest for its multiple ecosystem services: indicators for cultural services in heterogeneous forests. Ecol. Indic. 17, 123–133. doi: 10.1016/j.ecolind.2016.06.037
Teske, S., (ed.) (2019). “Achieving the Paris Climate Agreement,” in Goals: Global and Regional 100% Renewable Energy Scenarios with Non-Energy GHG Pathways for +1.5C and +2C. (Berlin: Springer), doi: 10.1007/978-3-030-05843-2
Thompson, L. M., Lynch, A. J., Beever, E. A., Engman, A. C., Falke, J. A., Jackson, S. T., et al. (2021). Responding to Ecosystem Transformation: resist, Accept, or Direct? Fisheries 46, 8–21. doi: 10.1002/fsh.10506
Thornton, T. F., and Moss, M. L. (2021). Herring and people of the North Pacific. Seattle: University of Washington Press, 259
Trammell, E. J., and Aisu, M. (2015). Development of a Landscape Integrity dataset for the Alaska Crucial Habitat Assessment Tool. Juneau, AK: Prepared for the Alaska Department of Fish and Game.
Trammell, E. J., Thomas, J. S., Mouat, D., and Korbulic, Q., and Bassett, S., (2018). Developing alternative land-use scenarios to facilitate natural resource management across jurisdictional boundaries. J. Environ. Plan. Manage. 61, 64–85 doi: 10.1080/09640568.2017.1289901
Turetsky, M. R., Kane, E. S., Harden, J. W., Ottmar, R. D., Manies, K. L., Hoy, E., et al. (2011). Recent acceleration of biomass burning and carbon losses in Alaskan forests and peatlands. Nat. Geosci. 4, 27–31. doi: 10.1038/ngeo1027
US Census Bureau. (2012). 2010 Census of Population and Housing, Population and Housing Unit Counts, CPH-2-1, United States Summary. Washington, DC: U.S. Government Printing Office, 2012.
Veraverbeke, S., Rogers, B. M., and Randerson, J. T. (2015). Daily burned area and carbon emissions from boreal fires in Alaska. Biogeosciences 12, 3579–3601. doi: 10.5194/bg-12-3579-2015
Veraverbeke, S., Rogers, B. M., Goulden, M. L., Jandt, R. R., Miller, C. E., Wiggins, E. B., et al. (2017). Lightning as a major driver of recent large fire years in North American boreal forests. Nat. Clim. Chang. 7, 529–534. doi: 10.1038/nclimate3329
Wang, J. A., Baccini, A., Farina, M., Randerson, J. T., and Friedl, M. A. (2021). Disturbance suppresses the aboveground carbon sink in North American boreal forests. Nat. Clim. Chang. 11, 435–441. doi: 10.1038/s41558-021-01027-4
Ward, D. H., Markon, C. J., and Douglas, D. C. (1997). Distribution and stability of eelgrass beds at Izembek Lagoon, Alaska. Aquatic Bot. 58, 229–240. doi: 10.1016/s0304-3770(97)00037-5
Watson, J. E. M., Evans, T., Venter, O., Williams, B., Tulloch, A., Stewart, C., et al. (2018). The exceptional value of intact forest ecosystems. Nat. Ecol. Evol. 2, 599–610.
Watson, J. E. M., Shanahan, D. F., Di Marco, M., Allan, J., Laurance, W. F., Sanderson, E. W., et al. (2016). Catastrophic Declines in Wilderness Areas Undermine Global Environment Targets. Curr. Biol. 26, 2929–2934. doi: 10.1016/j.cub.2016.08.049
Wells, J. V., Dawson, N., Culver, N., Reid, F. A., and Morgan Siegers, S. (2020). The State of Conservation in North America’s Boreal Forest: issues and Opportunities. Front. For. Glob. Change. 3:90. doi: 10.3389/ffgc.2020.00090
Wheeler, P., and Thornton, T. F. (2005). Subsistence Research in Alaska: a thirty year retrospective. Alaska J. Anthropol. 3, 69–103.
Wolfe, R. J. (2004). Local traditions and subsistence: A synopsis from twenty-five years of research by the State of Alaska. Juneau, AK: Alaska Department of Fish and Game, Division of Subsistence.
Wolken, J. M., Hollingsworth, T. N., Rupp, T. S., Chapin, F. S. III, Trainor, S. F., Barrett, T. M., et al. (2011). Evidence and implications of recent and projected climate change in Alaska’s forest ecosystems. Ecosphere 2, 1–35. doi: 10.1890/ES11-00288.1
Keywords: Alaska, climate change mitigation, adaptation, biodiversity, carbon storage
Citation: Vynne C, Dovichin E, Fresco N, Dawson N, Joshi A, Law BE, Lertzman K, Rupp S, Schmiegelow F and Trammell EJ (2021) The Importance of Alaska for Climate Stabilization, Resilience, and Biodiversity Conservation. Front. For. Glob. Change 4:701277. doi: 10.3389/ffgc.2021.701277
Received: 27 April 2021; Accepted: 22 July 2021;
Published: 23 August 2021.
Edited by:
Brian J. Palik, United States Forest Service (USDA), United StatesReviewed by:
Xianliang Zhang, Agricultural University of Hebei, ChinaGuillaume Marie, Vrije Universiteit Amsterdam, Netherlands
Copyright © 2021 Vynne, Dovichin, Fresco, Dawson, Joshi, Law, Lertzman, Rupp, Schmiegelow and Trammell. This is an open-access article distributed under the terms of the Creative Commons Attribution License (CC BY). The use, distribution or reproduction in other forums is permitted, provided the original author(s) and the copyright owner(s) are credited and that the original publication in this journal is cited, in accordance with accepted academic practice. No use, distribution or reproduction is permitted which does not comply with these terms.
*Correspondence: Carly Vynne, Y2FybHlAb3NwcmV5aW5zaWdodHMuY29t; Natalie Dawson, bmF0YWxpZS5kYXdzb25AYXVkdWJvbi5vcmc=