- 1Department of Natural Resources and Environmental Science, University of Nevada, Reno, NV, United States
- 2Baruch Institute of Coastal Ecology and Forest Science, Clemson University, Georgetown, SC, United States
The interception of precipitation by plant canopies can alter the amount and spatial distribution of water inputs to ecosystems. We asked whether canopy interception could locally augment water inputs to shrubs by their crowns funneling (freshwater) precipitation as stemflow to their bases, in a wetland where relict overstory trees are dying and persisting shrubs only grow on small hummocks that sit above mesohaline floodwaters. Precipitation, throughfall, and stemflow were measured across 69 events over a 15-months period in a salinity-degraded freshwater swamp in coastal South Carolina, United States. Evaporation of intercepted water from the overstory and shrub canopies reduced net precipitation (stemflow plus throughfall) across the site to 91% of gross (open) precipitation amounts. However, interception by the shrub layer resulted in increased routing of precipitation down the shrub stems to hummocks – this stemflow yielded depths that were over 14 times larger than that of gross precipitation across an area equal to the shrub stem cross-sectional areas. Through dimensional analysis, we inferred that stemflow resulted in local augmentation of net precipitation, with effective precipitation inputs to hummocks equaling 100–135% of gross precipitation. Given that these shrubs (wax myrtle, Morella cerifera) are sensitive to mesohaline salinities, our novel findings prompt the hypothesis that stemflow funneling is an ecophysiologically important mechanism that increases freshwater availability and facilitates shrub persistence in this otherwise stressful environment.
Introduction
In flooded wetlands, microtopographic high areas (e.g., hummocks) can serve as local refugia. Microtopographic highs can foster seed germination and seedling survival in environments where they would otherwise be excluded by flooding (Conner et al., 1986; Souther and Shaffer, 2000); this is especially true when floodwaters are saline (Rheinhardt and Faser, 2001) because salinity is often a critical physiological stressor to both juvenile and mature trees (Allen et al., 2019). Thus, hummocks, which are often composed of partially decomposed organic material, such as stumps or logs, and are common in diverse wetland types (Andrus et al., 1983; Jones et al., 1996; Shimamura and Momose, 2005; Stine et al., 2011), can affect community structure by providing a preferential zone for establishment (Huenneke and Sharitz, 1986; Duberstein and Conner, 2009). Hummocks allow roots to avoid and sit above saline waters (Light et al., 2007). Hsueh et al. (2016) showed that salinity sensitive trees occupying hummocks in a mesohaline wetland used water from the hummocks which was less saline than the surrounding floodwaters; others have also observed coastal woody plants avoiding uptake of saline water despite its availability and proximity to roots (Ish-Shalom et al., 1992). In this study, we examine the input of freshwater to hummocks, which can facilitate plants’ avoidance of saline conditions.
Precipitation is often the primary freshwater input to ecosystems, although the amount and spatial distributions of that input can be substantially modified by canopy interception before it reaches plant roots. Interception can substantially reduce precipitation inputs, with interception losses in closed-canopy forests often exceeding 10–20% of gross precipitation (Carlyle-Moses and Gash, 2011). Thus, throughfall and stemflow, which together constitute the net precipitation input to soils, can be considerably less than gross precipitation. However, precipitation inputs to specific locations can be substantially augmented by interception processes because tree crowns can redistribute water and result in temporally stable spots of especially high precipitation inputs (Keim et al., 2005). Perhaps the greatest localized input is that of stemflow, which is routed down stems to the stem-soil interface and, hypothetically, to roots (Martinez-Meza and Whitford, 1996; Liang et al., 2009). Thus, a consequence of canopies routing throughfall and stemflow may be local augmentations to the amount of water that infiltrates near stems (Li et al., 2009).
In this study, we investigate how canopy interception affects precipitation inputs to a permanently flooded wetland where salinity intrusion has yielded an ecosystem composed of sparse relict trees with submerged roots (Williams et al., 2014) and midstory shrubs rooted on hummocks. The dominant shrub, wax myrtle (Morella cerifera) can have wide crowns and smooth bark, which are characteristics associated with high stemflow rates (Levia and Germer, 2015). In our study site, their crowns extended far beyond the extent of the above-water hummock areas (here defined as microtopographic highs supporting woody vegetation). Thus, we hypothesized that stemflow inputs to hummocks could augment the net precipitation, and thus the influx of freshwater, to exceed that of gross precipitation. If this were true, this could be ecologically important given that wax-myrtle shrubs are stressed by salinities as low as five practical salinity units (PSU) (Tolliver et al., 1997), which is within the range of values observed in floodwaters of the study site (Duberstein et al., 2018).
Materials and Methods
Site Description
The study site was Strawberry Swamp in coastal South Carolina (33°19′49″N, 79°14′54″W), a permanently flooded wetland with ∼30 ha watershed (Williams et al., 2014) that drains into the Winyah Bay estuary (1.2 km to the west); the site is also proximal to the Atlantic Ocean (8 km to the east). The region’s climate can be classified as humid subtropical with a mean July temperature of 27.6°C, a mean January temperature of 8.2°C, and an annual total precipitation of 133 cm, which is highest from July–September (mean of 17 cm month–1; from the National Climate Data Center). Salinity enters the site from periodic storm surges or by backflooding from the estuary during severe events. This salinity is associated with widespread tree mortality and replacement by shrub cover (Williams et al., 2014), as well as reduced biomass (Liu et al., 2017) and transpiration (Duberstein et al., 2020).
The throughfall and stemflow measurement locations spanned freshwater and mesohaline conditions (Table 1). This salinity gradient occurs over a roughly 50–100 m distance so freshwater and saltier zones are not discrete or spatiotemporally constant. The sampling design was oriented around a 500-m-long, 0.3-m-wide boardwalk. The boardwalk is composed of two transects in a “v” shape (Figure 1) from freshwater to mesohaline to freshwater zones. While the boardwalk location is not random, its placement was for spanning the salinity gradient and not with attention to small-scale biases regarding its position under the canopy.
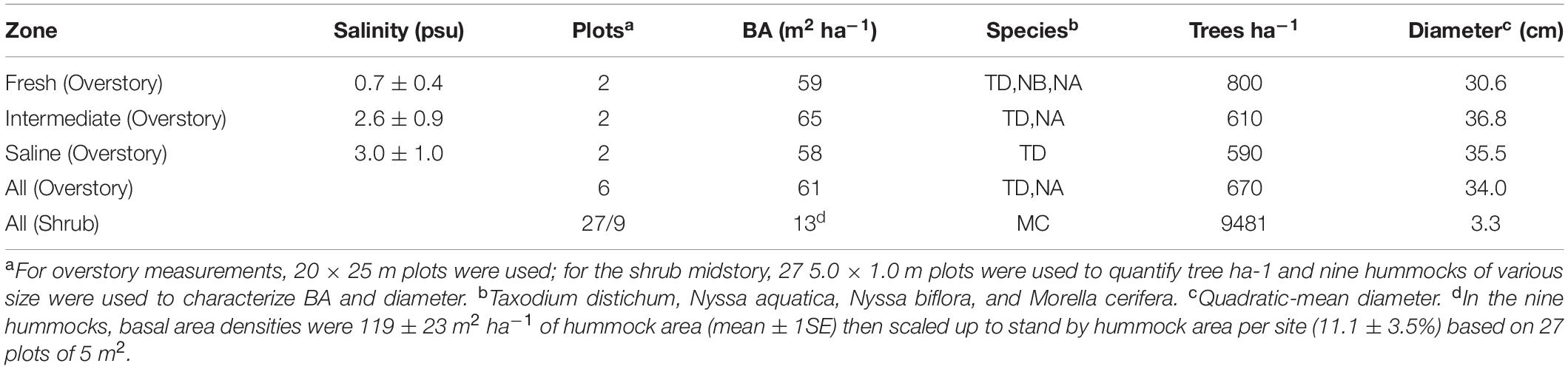
Table 1. Forest characteristics—salinity, basal area (BA), dominant species (>15% of BA), density, and size—for the overstory trees by zone, and shrub characteristics across the whole site (Liu et al., 2017; Duberstein et al., 2020).
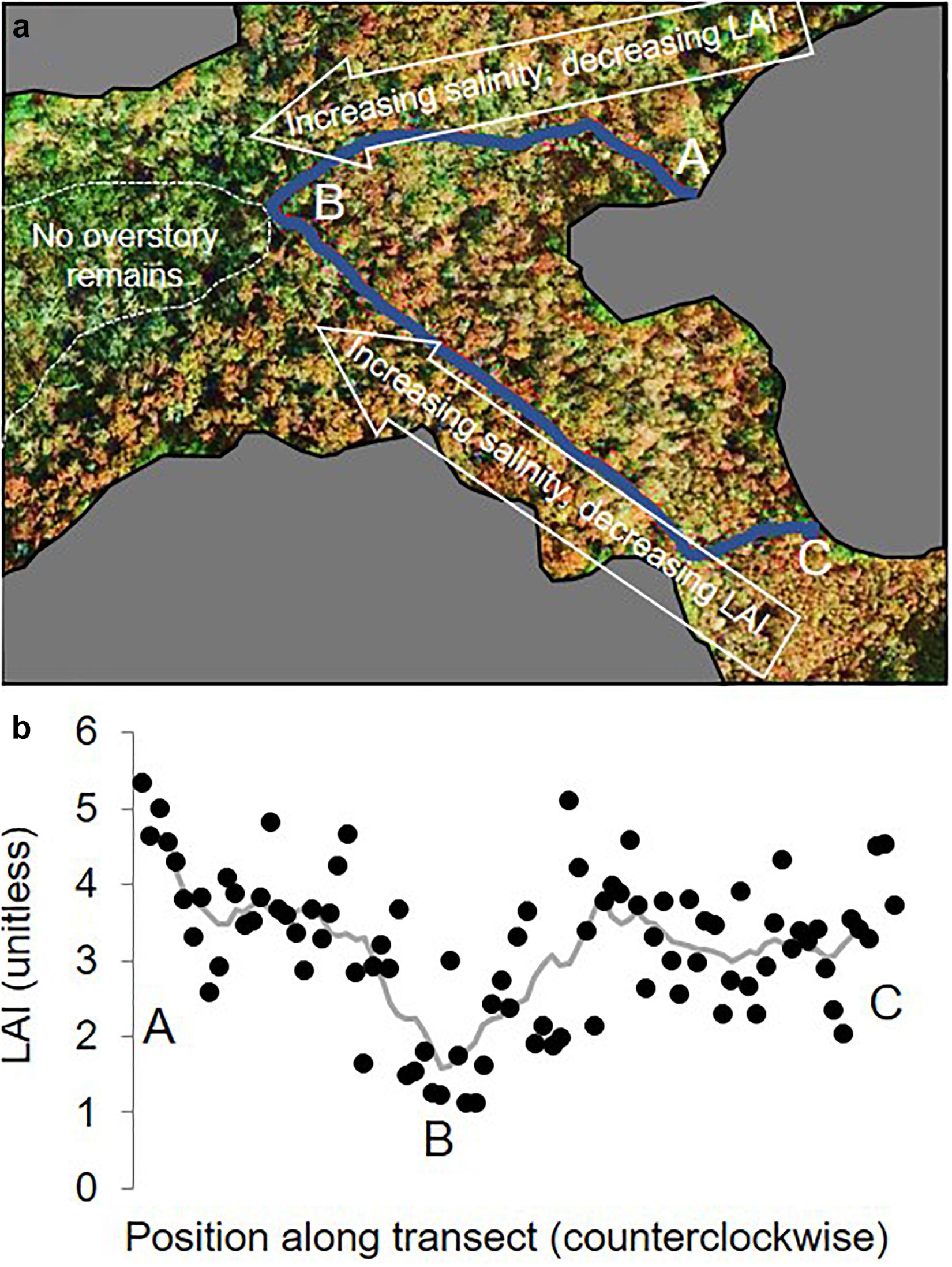
Figure 1. (a) Aerial image of site (with upland areas excluded), showing the boardwalk along which sampling was conducted (in blue) and showing the shift in structure with salinity. The marked “A”, “B”, and “C” correspond with the LAI measurements in (b), which are measured at 6-m intervals along the site boardwalk. Imagery was downloaded from Google Earth (Google Inc., Mountain View, CA, United States). The gray line in panel (b) is a running mean using a nine-measurement moving window.
Canopy and tree or shrub characteristics were quantified. Uncorrected leaf area index (LAI) was quantified by light attenuation using paired canopy analyzers (LAI-2000, LI-COR, Lincoln, NE) with 76° view angles (Cutini et al., 1998), which yields reasonable relativistic values in baldcypress swamps (Allen et al., 2015). Measurements were taken on an approximately 6-m interval over the length of the boardwalk (88 measurements) in July 2015 (Figure 1). LAI values ranged from one, in the mesohaline zone, to over five, in the freshwater zone (Figure 1). Stand basal areas were quantified across this gradient in previous studies (Liu et al., 2017; Duberstein et al., 2020) that discretized the site into zones classified as freshwater, intermediate, and mesohaline; those studies measured trees and shrubs with diameters at breast height (dbh, at 1.3 m) over 10 cm in two 20 × 25 m plots in each zone. In this study, we use site-averaged basal areas (BA), using the average of all six plots from these previous studies. We also refer to “Freshwater” and “Mesohaline” zones, which is an ad hoc classification used for communication purposes. Despite similarities in basal areas among zones (Table 1), the freshwater and mesohaline zones differed substantially in ecosystem structure: baldcypress (Taxodium distichum var. distichum) and water tupelo (Nyssa aquatica) occurred in both zones, but swamp tupelo (Nyssa biflora) and sweetgum (Liquidambar styraciflua) only occurred in the freshwater zone. In the mesohaline zone, there were fewer, larger trees and they were predominantly baldcypress (Table 1). The midstory was composed of wax myrtle shrubs (Morella cerifera) in both zones, although the freshwater zone midstory also had younger cohorts of baldcypress.
This wetland is always flooded, although wetter conditions reduce the above-water ground area. Based on our experience wading through the floodwaters, we estimate water depths to largely range between 0.5 and 1.5 m. However, they are not easily quantified because they vary tremendously in space. Furthermore, the soils are unconsolidated and contain substantial amounts of coarse woody debris, further challenging a quantitative description of water depth. Both the freshwater and mesohaline zones have hummocks, and both have deep (>1.0 m) water. In general, water levels vary with major events, seasons, and prolonged droughts, but are minimally responsive to small events in summer. For example, in the week before our hummock survey, 3.8 and 1.6 cm precipitation events resulted in 3.5 and 2.3-cm water level rises that receded back to prior conditions in less than a week. However, a historic 60-cm precipitation event in 2015 caused water levels to rise to 1.1 m over the median depth.
All observed shrub stems were rooted in hummocks (e.g., Figure 2), not in soils under the floodwater as was generally the case for large, mature trees; this pattern has been reported elsewhere (Rheinhardt and Faser, 2001).
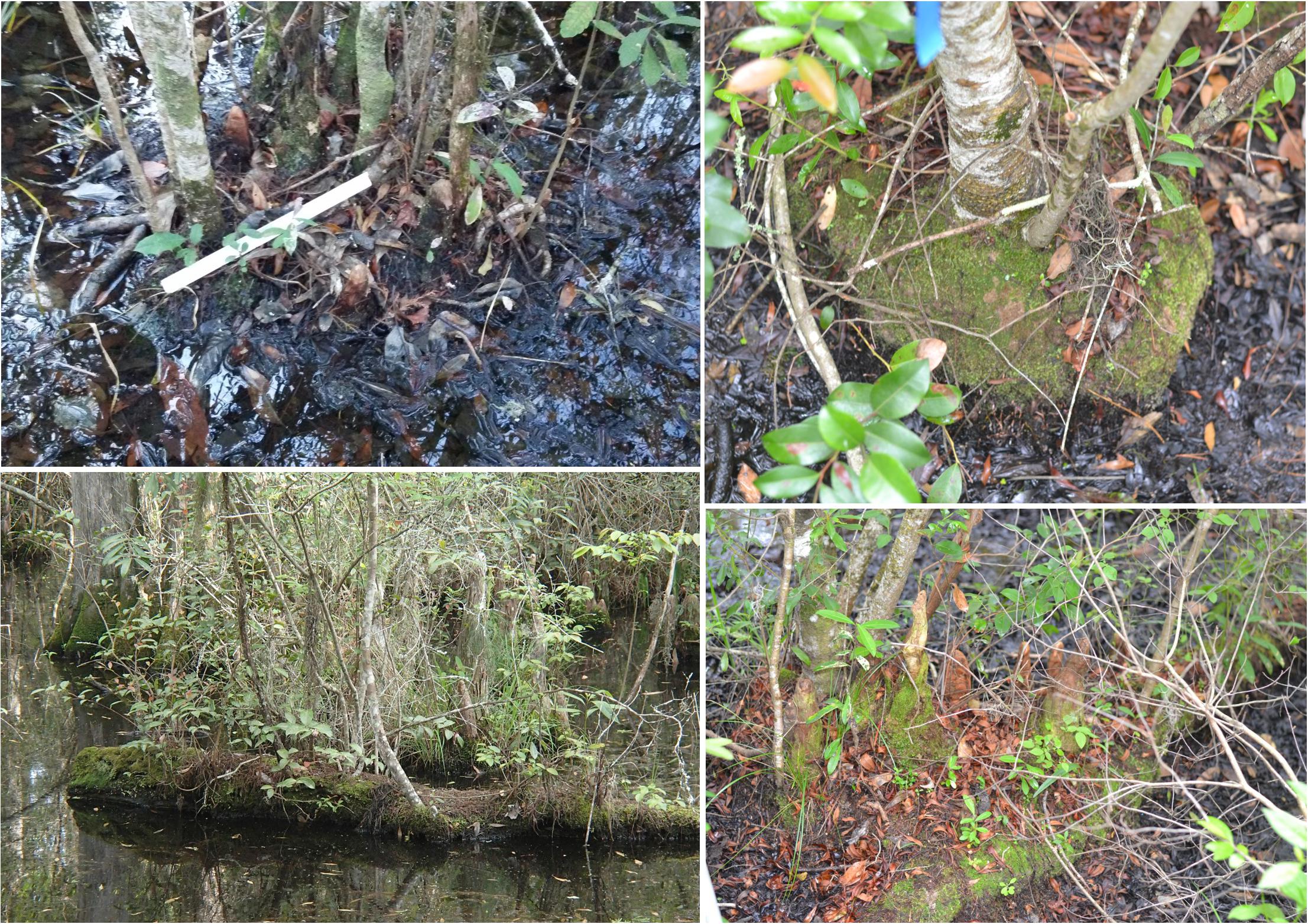
Figure 2. Wax myrtle stems on hummocks of different heights and sizes at the Strawberry Swamp field site; in the top left panel, a ∼0.3 m ruler is shown.
Net and Gross Precipitation Measurements
To measure gross precipitation, a precipitation collector was placed in an open field 500 m away from the study site. This precipitation collector, as well as each of the throughfall collectors, was composed of a 2.0-liter plastic jug with a 11.7 cm diameter funnel; water passed through a >5-cm length of tubing to impede evaporation from the collectors. Collectors were placed on a pole that was roughly one meter above the soil or floodwater surface. They were designed so that the collectors could haphazardly pivot around the poles, so that they would not be in an identical position each time they were sampled and put back on the pole; non-stationary sampling strategies such as this reduce uncertainties because they mitigate the likelihood of drip points being consistently represented or missed as a function of collector location (Holwerda et al., 2006).
To measure throughfall, 29 collectors were placed along the boardwalk. They were placed to span both fresh and mesohaline zones, up to the edge of the entirely treeless zone (Figure 1a). In addition to the previously described LAI measurements along the boardwalk, LAI was also characterized above each collector, using the LAI-2000 instrument. In the field, each collector was categorized as being associated with the freshwater or mesohaline zones as a function of its position along the gradient and the change in canopy (i.e., with minimal evidence of tree recruitment in recent decades in the mesohaline zone). These classifications were admittedly subjective; however, they were established prior to any precipitation sampling and they reflected our best visual assessment of a step-shift in forest structure.
Stemflow was measured on six wax-myrtle shrub stems. The stems varied from 5.0 to 7.5 cm dbh. Stemflow was collected using an approximately 5-cm thick ring of wax inside of a plastic cylinder sealed around them (Safeeq and Fares, 2014); a length of tubing conveyed water to a 2.0- or 4.0-liter container (depending on tree size). We did not measure overstory-tree stemflow because they all have rough bark, which is generally associated with low SF (Rothacher, 1963; Van Stan and Gordon, 2018); its potential effects were not ignored in calculations, however (as described in section “Analyses”).
Overall, precipitation from 69 events was collected from 14 April 2015 to 8 July 2016. The stemflow collectors were installed on 3 July 2015. Occasionally, back-to-back rain events occurred, preventing collection in between events; thus, some so-called events included multiple storms. The measurements were continuous, with the exception of one excluded record precipitation event of 60 cm in 5 days in Oct 2015. Over the study, 2% of throughfall samples were lost due to collectors coming off the poles or mis-transferring water into the measuring graduated cylinder. In all cases where cumulative throughfall or stemflow depths are compared to cumulative gross precipitation, only matched respective events are used (i.e., if a collector failed for one event, gross precipitation is also omitted for that event when, e.g., quantifying ratios of throughfall to gross precipitation).
Hummock and Shrub Measurements
Intensive measurements of shrubs and hummocks were made to scale stemflow measurements and quantify those fluxes across the site, across hummocks, and across shrubs.
To determine fractional hummock area for the swamp, we assumed the boardwalk to represent an unbiased transect through the swamp and took systematic measurements of hummocks along that track. A 5.0-by-1.0-m plot was established precisely every 18-m of length along the boardwalk (27 plots in total), extending perpendicularly on the outside of the boardwalk loop. In each plot, area of water versus area of above-water-level ground (hummocks) was quantified; water levels at the time of the survey were 5 cm below the median water level over the study duration, and water levels were within 5 cm of this height for 55% of the growing season, so they were assumed to reasonably represent typical conditions. Hummocks were defined as any ground above the water level that had accumulated soil or litter and had vegetation growing on it. Hummock areas were approximated by assuming each hummock was rectangular in shape, with area calculated from average lengths and average widths measured using a measuring tape. The number of woody stems on hummocks within plots was counted. Across the 5.0 m2 plots, the number of hummocks per plot ranged from zero (in five of the 27 plots) to four, the individual hummock sizes (within the plot bounds) ranged from 0.01 to 2.5 m2, and the shrub stem density averaged 9 ± 5 stems per m2 of hummock and was as high as 17 per plot and 67 per m2 of hummock area. Hummocks averaged 11 ± 3.5% (Mean ± 1SE) of the area in plots (and thus floodwater and submerged tree stems were 89% of plot area); this was assumed to be representative of the site.
More intensive diameter inventories were measured on nine additional hummocks, selected because they contained or were near the wax-myrtle shrubs on which stemflow was measured. These nine hummocks are reasonably representative of the site, as indicated by the similarity in stem density (10 ± 4 stems per m2 of hummock; Mean ± 1SE) to that observed across the effectively-random hummock plots described above. Hummock area, hummock height, and total crown area of all shrubs on each hummock were measured (Supplementary Table 1). In these nine hummocks, the total crown area of shrubs was twenty times greater than the area of hummocks they occupied (Supplementary Table 1); shrub crowns also overlapped with shrubs on other hummocks. Diameters of every woody plant over 1.0 cm in dbh were measured, which were almost exclusively wax myrtle; no diameters exceeded 8 cm. Basal areas were calculated for each stem, and summed for the nine hummocks and divided by hummock areas, showing that stem areas were 1.2 ± 0.2% of hummock areas (mean ± 1 SE); this number was multiplied by the fractional area of hummocks across the site (previous paragraph) to yield the mean fractional shrub basal area (FBA–shrub), which was 0.13 ± 0.05% of the site area (or 13 ± 5 m2 ha–1 of site area), with the SE estimated by Gaussian error propagation.
Analyses
Interception loss was calculated across the site using the following equation:
where Pg is the gross precipitation measured in the clearing, TF is the throughfall across the site, SFshrub is the upscaled site-level wax-myrtle stemflow, and SFoverstory is the assumed overstory stemflow, and FBA–all is the fractional basal area of all trees and shrubs; FBA–all was calculated as the sum of FBA–shrub and overstory tree basal areas divided by respective plot areas (FBA–overstory). The “1- FBA–all “term is included because throughfall cannot fall where stems are located. To report all fluxes as depths, measured Pg and TF volumes were divided by the area of the collector funnels. For SFshrub, depths were first calculated by dividing cumulative collector volumes for each stem by its basal area. These depths were averaged across the six wax-myrtle shrubs and multiplied by FBA–shrub to yield SFshrub, integrated across the stand. To fill gaps of events where stemflow was not measured, SFshrub was estimated by multiplying event Pg by the mean shrub funneling ratio (FRshrub: cumulative SFshrub divided by cumulative Pg, over the same events). Values for SFoverstory are assumed by multiplying Pg by FBA–overstory and an assumed overstory funneling ratio (1.0); a higher value (3.0) and a lower value (0.0) were also used to understand the sensitivity of our findings to the overstory trees potentially having unexpectedly small or large funneling ratios (see, e.g., Van Stan and Gordon, 2018).
Interception losses were calculated as site-integrated, cumulative values, but we also stratified results among small events (<1 cm), medium events (≥1 and <3 cm), and large events (≥3 cm), as well as by growing and dormant season (April-October and November-March, respectively; readers should note that wax myrtle is evergreen). For error calculations in cumulative values, by-event standard errors (reflecting variability among collectors) were added in quadrature to propagate errors through analyses. Errors in overstory-basal-area and gross-precipitation values are treated as negligible compared to other sources of errors in eq. 1.
Stemflow volumes exceeded the collector capacities in many larger events, with potentially overtopped collectors representing 19% of the entire dataset. This is a substantial amount, and thus all reported observed stemflow values are likely underestimates. In collectors that overtopped, we assumed their values to be the observed maximum collectable volume (i.e., 1600 or 3600 ml for 2000 or 4000 ml containers). We assume all reported observed SFshrub values to underestimate actual values, but the magnitude of that bias is unknown, and thus, we preface stemflow values as being “at least” what we report them to be.
Analyses were performed in MATLAB (Mathworks, Natick, MA, United States). All uncertainties are expressed as ± one standard error (SE) unless described otherwise.
Results and Discussion
Precipitation Partitioning
Over the 15-months measurement period, net precipitation was 91.4 ± 0.7% of Pg (Table 2), implying that interception losses were 8.6 ± 0.7% of Pg, or less, given that stemflow was underestimated (as described in section “Analyses”). Interception losses were greatest for small storms (<1.0 cm) during the growing season (Table 2). Throughfall, the dominant component of net precipitation, ranged from 63% to 112% of Pg in storms larger than >1.0 cm and from 39% to 142% of Pg in storms <1.0 cm. Throughfall exceeded precipitation in only eight events, with an amount-weighted mean among those events of only 1.06% of Pg. Interception loss fractions were lower in the dormant season, as indicated by the several SEs of separation between dormant- and growing-season mean values (Table 2).
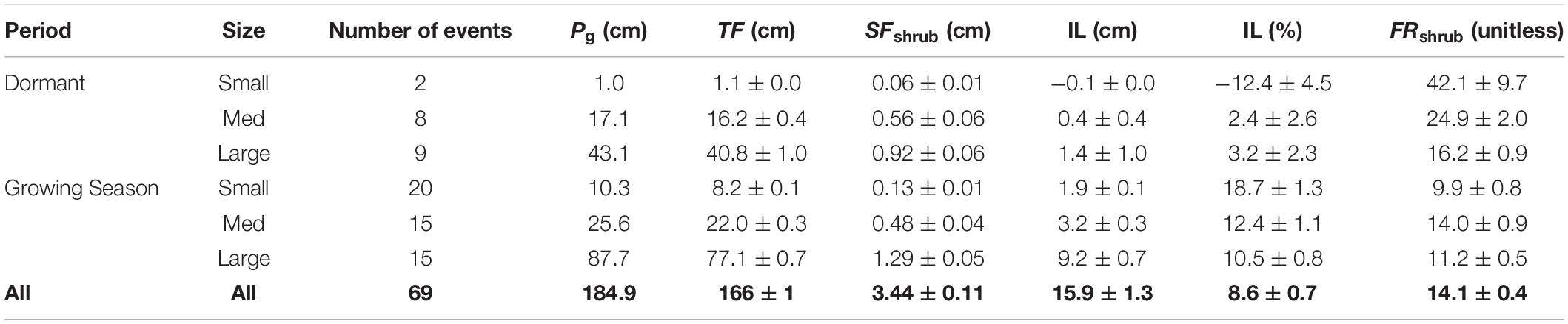
Table 2. Cumulative depth of gross precipitation (Pg), throughfall (TF), shrub stemflow (SFshrub), and shrub SF funneling ratio (FRshrub), and interception loss (IL) over the 15-months-measuring period, stratified by event size (small < 1 cm, 1 cm ≤ medium < 3 cm, and large ≥ 3 cm) and season (dormant versus growing seasons).
Across the salinity gradient, throughfall amounts increased in sparser-canopy zones (Figure 3). The TF:Pg ratios decreased with increasing LAI, although one outlier (i.e., a drip point in the mesohaline zone) weakened the least-squares-fit regression relationship (R2 = 0.04); however, by using a robust-fit regression to down-weight the influence of outliers, we found that TF:Pg decreased by 0.033 (mm mm–1) per unit of LAI (adjusted R2 = 0.25, p < 0.01). The collectors in the mesohaline zone yielded cumulative TF:Pg ratios of 0.92 ± 0.05 (n = 15; mean ± 1SE), compared to those in the freshwater zone of 0.87 ± 0.03 (n = 14), and thus they did not differ significantly (p = 0.4). Accordingly, we assume that TF is similar across the site in all site-level analyses, despite the relationships shown in Figures 1, 3.
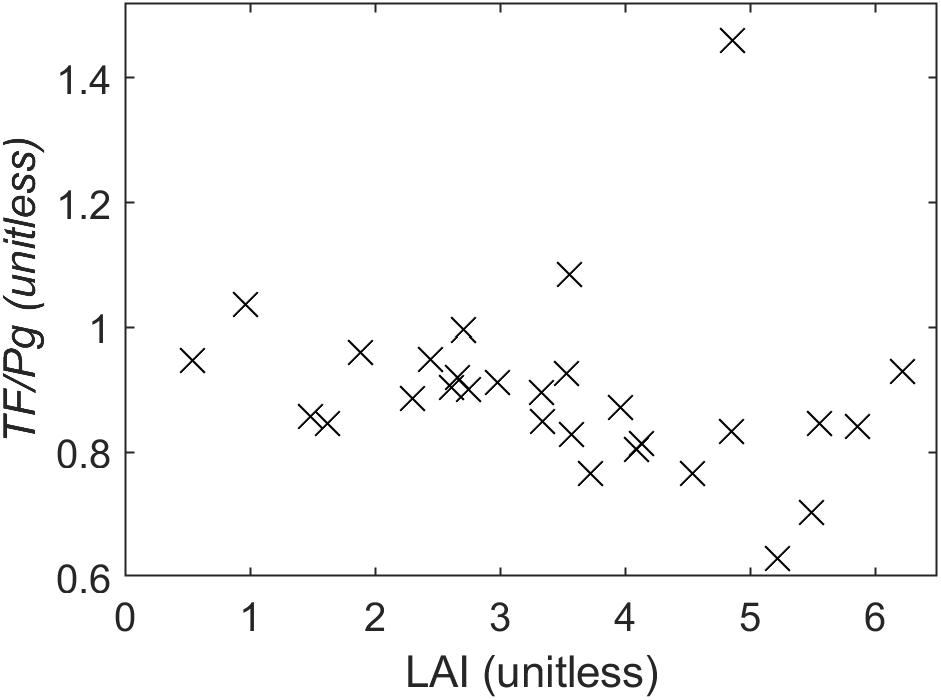
Figure 3. The ratio of cumulative throughfall (TF) to cumulative gross precipitation (Pg) by TF collector, regressed against LAI measured above each collector. Areas under denser canopy generally receive less throughfall.
To account for the uncertainties associated with assuming that overstory tree stemflow equaled Pg depths across the overstory tree BAs (i.e., an assumed funneling ratio of one), we evaluated the sensitivity of interception loss estimates to assuming overstory funneling ratios of zero and three; resulting interception losses were 9.2 and 7.4% of Pg, respectively, and thus errors in our interception loss calculations (reported above as ±0.7% of Pg) may be more on the scale of ± 2% of Pg.
Stemflow to Hummocks
Given that most precipitation must fall on the floodwater surface, as opposed to the hummock soils, the routing of stemflow to hummocks could be important for increasing freshwater availability to shrubs on those hummocks.
The mean cumulative funneling ratio (FRshrub) of the six instrumented wax-myrtle stems was 14.1 ± 0.4, implying that the depth of stemflow resulting from one mm of precipitation would be 14.1 mm across an area equal to the stem’s basal area. Although stemflow was only a small component (i.e., 2%) of net precipitation (Table 2), this input comes down shrub stems which only represent 0.13 ± 0.05% of the site area. Given that shrub crowns are much more expansive than stems, with a crown-area to stem-basal-area ratio of 2228 ± 198 m2 m–2 for shrubs on the nine intensively measured hummocks, only 0.6% of the precipitation potentially falling on those shrub crowns would need to be intercepted and routed to stemflow to yield funneling ratios of 14.1. Small amounts of precipitation collected across larger areas can be funneled to stems to locally augment inputs.
If we were to assume that all of shrub stemflow were to infiltrate into hummocks, stemflow can represent a substantial augmentation of freshwater, given that hummocks were small and the shrub stemflow was high. Hummocks were 11.1 ± 3.5% of the site area, and thus net precipitation can be calculated specifically for the hummocks by assuming that they receive all of the shrub stemflow (SFshrub) and the same depths of TF as the rest of the site. To do so, we re-calculate SFshrub for only the hummock area (i.e., SFhummocks is equal to SFshrub divided by 11.1 ± 3.5%) and then calculate hummock net precipitation as TF + SFhummocks. Using the mean fractional hummock area, we estimate that the cumulative SFhummocks would be 31.1 ± 1.0 cm, and that hummock net precipitation would be 197 ± 2 cm, representing 106% of Pg (Figure 4). To account for error in the fractional hummock area (a major source of uncertainty), we recalculate these values for the confidence intervals of fractional hummock area using the mean ± 2SE (i.e., with hummocks representing 4.1% and 18.1% of site area); this yields an interval of net precipitation to hummock of 185–250 cm, representing 100–135% of Pg. We remind readers that these estimates are coarse, but they are also underestimated because stemflow was under-captured by the collectors. Thus, net precipitation inputs to hummocks likely ranged from equaling to substantially exceeding Pg, despite site-integrated net precipitation being approximately 9% lower than Pg.
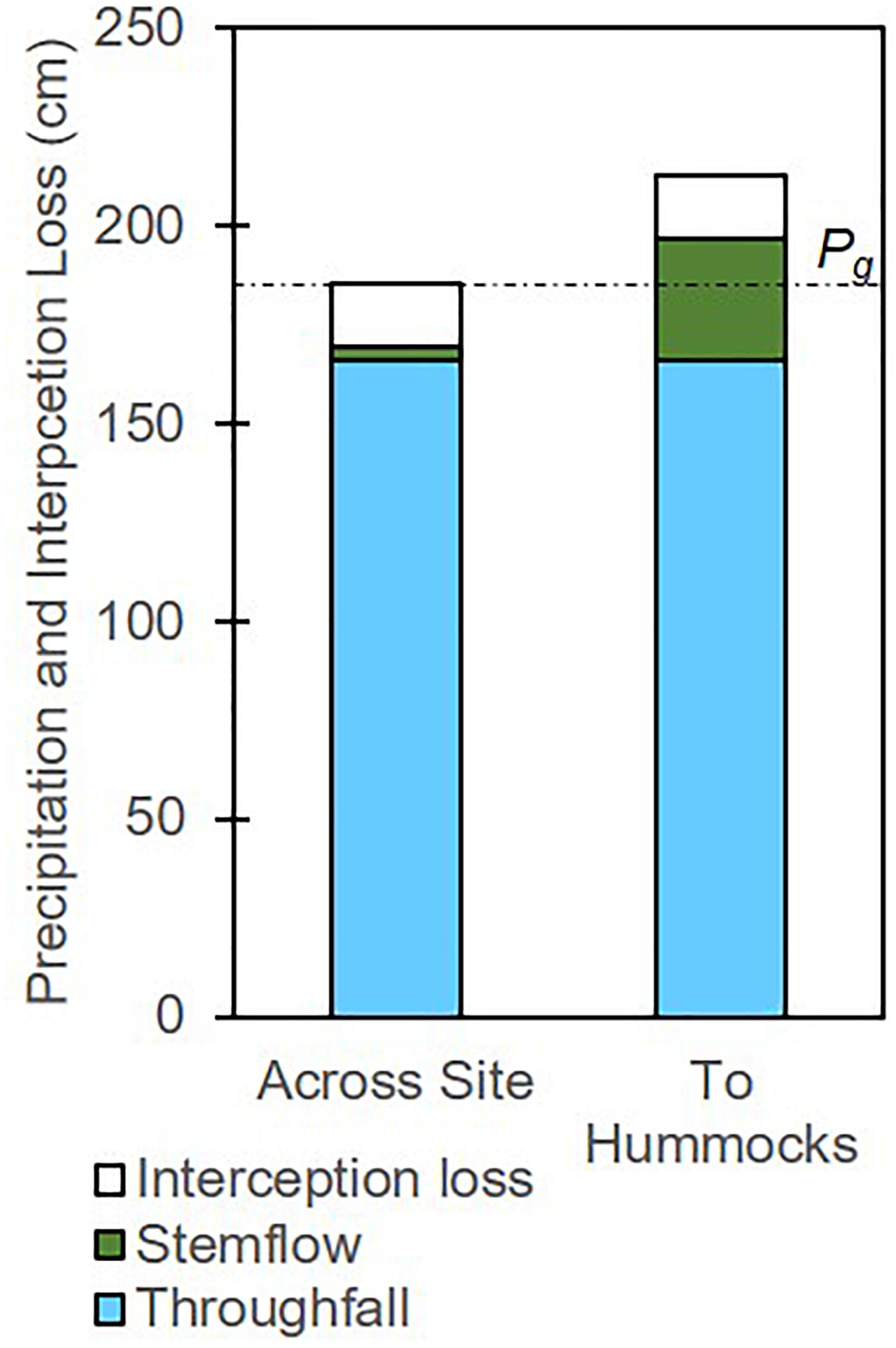
Figure 4. Partitioning of precipitation among interception loss, stemflow, and throughfall across the site and across hummock area, assuming all shrub stemflow infiltrates into hummocks. The dotted line indicates the depth of gross precipitation across the study period, equal to the sum of across-site stemflow, interception loss, and throughfall, but exceeded by the sum of those values to hummocks (because of stemflow funneling in shrubs on hummocks).
Implications and Synthesis
The effect of interception – accounting for losses and redistribution – involved net losses at the site level but local augmentation of precipitation to hummocks (Figure 4). These losses are not especially surprising, although the interception loss estimates (mean of 9%, assuming FRoverstory of one) were below average values of mixed forests (Carlyle-Moses and Gash, 2011), likely because the site included sparse canopy areas with low LAI (Figure 1). The denser canopy in the freshwater zone yielded TF:Pg ratios (Figure 3) that are comparable to global mean interception losses from forests (13–19% in broadleaf forests and 22% in conifers; Miralles et al., 2010). Both the effects of season (TF during leafed period > leafless) and event size (TF fraction during small events was less than during large events) were also consistent with others’ observations (e.g., Mużyło et al., 2012). Given that the observed patterns in interception loss are typical, we focus this discussion on the previously undescribed phenomenon of stemflow funneling to hummocks.
The augmentation of net precipitation to hummocks was likely attributable to (1) high funneling ratios, (2) stems restricted to rooting on small hummocks, and (3) broad crowns extending well beyond the spatial extents of the hummock (Figure 5 and Supplementary Table 1).
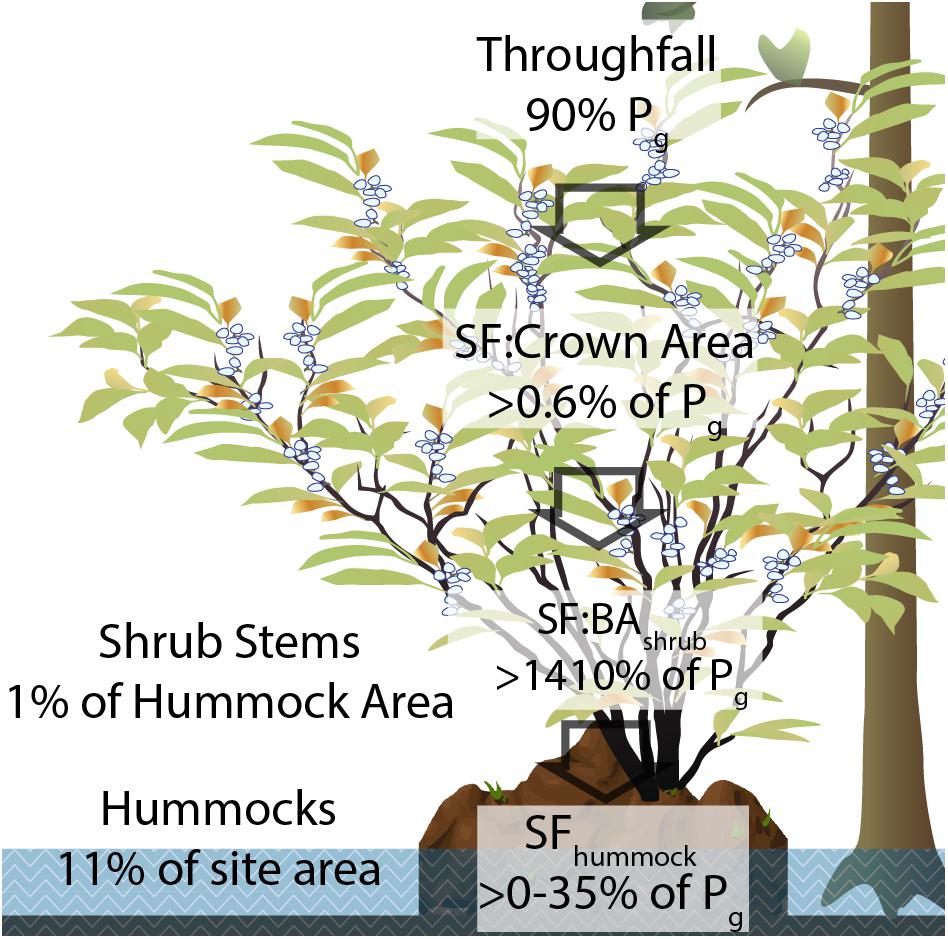
Figure 5. Diagram of stemflow input scaling in the shrub layer, where input is TF from the overstory (90% of gross precipitation, Pg). Across shrub crown areas, 0.6% of Pg is routed to stemflow, which equates to over 14.1 times the depth of Pg across shrub stem basal areas. Across hummocks, stemflow averaged 17% of Pg depths, amounting to hummock net precipitation inputs that were 106% of Pg. The plant illustrations are adapted from artwork by Jane Hawkey (Integration and Application Network, University of Maryland Center for Environmental Science; ian.umces.edu/imagelibrary/).
The observed cumulative funneling ratio of wax-myrtle shrubs (14 ± 0.4) was high, although funneling ratios over 20 are not uncommon for smooth-barked or large-crowned species (Levia and Germer, 2015). Moreover, the reported funneling ratios may be severely underestimated by us using too-small collectors (i.e., with maximum volumes of 3600 and 1600 ml for four and two of the collectors, respectively). More evidence that these values are underestimated is shown in Table 2: smaller events (less likely to overflow the collectors) had larger funneling ratios, which is a pattern that contrasts with other studies (Mużyło et al., 2012; Siegert and Levia, 2014). Collector overtopping even occurred in events as small as 1.6 cm, indicating that the under-catch of stemflow in larger events could have been substantial. Thus, actual net precipitation to hummocks may far exceed the 100–135% of Pg that we can infer from our data.
The association of wax-myrtle shrubs with hummocks may represent a system that benefits from stemflow-augmented net precipitation. Although wax myrtle is commonly associated with mesohaline environments, it is stressed by salinities as low as five PSU and it is often absent from zones of higher salinities (Young et al., 1994; Tolliver et al., 1997). In the mesohaline zones, 9% of measurements across the study exceeded 5 PSUs (see salinity measurements for stations “St4”, “St5”, and “T” in this dataset: Duberstein et al., 2018). Thus, the above-floodwater volume of these hummocks, which could store freshwater, may hypothetically provide a freshwater refuge to salinity-intolerant shrubs that enables of separation of roots from the surrounding mesohaline floodwaters. The measured hummock elevations (Supplementary Table 1) were similar in magnitude to the rooting depths of wax myrtle and other coastal shrubs reported elsewhere (Young et al., 1994). Others have used stable-isotope tracing to demonstrate that coastal vegetation can use hummock and shallow-soil water to avoid using saline floodwaters or groundwater (Sternberg et al., 1991; Hsueh et al., 2016). Given the limited heights and spatial extents of hummocks, and thus their limited potential volume of freshwater storage, we hypothesize that canopy-interception-induced precipitation augmentation to hummocks could be a mechanism by which plant-water status and site favorability is improved.
Potential ecohydrological implications of stemflow routing have been discussed and demonstrated elsewhere. The routing of stemflow has been shown to augment moisture locally around stems (Návar and Bryan, 1990; Liang et al., 2009), notably in water-limited shrublands (Martinez-Meza and Whitford, 1996). Researchers have described a “double funneling” effect where not only does stemflow funnel from a broad canopy funnel to a stem, it also preferentially infiltrates around the stem as a high-flux-density input (Martinez-Meza and Whitford, 1996; Levia et al., 2011; Spencer and Van Meerveld, 2016). There is a paucity of evidence showing how stemflow infiltrates or influences plant-water relations (see discussions in Allen et al., 2020; Carlyle-Moses et al., 2020; Van Stan and Allen, 2020); however, the potential physiological benefits of augmented net precipitation to hummocks with limited water storage capacities seem likely, given that precipitation is the freshwater source to the shrubs located in the mesohaline zone. Otherwise, unfortunately, our study was not designed to directly measure the roles of stemflow in hummock water balances, the pore-water salinities in hummocks, or wax-myrtle water uptake sources; perhaps a manipulative experiment in which stemflow is diverted from shrubs and hummocks in a subset of stems could elucidate the ecophysiological importance of stemflow to wax myrtle on hummocks.
Ultimately, this first study showing the potential magnitude of net precipitation to hummocks prompts new questions about the importance of this phenomenon. For example, given that dormant season events (i.e., when less overstory canopy is intercepting precipitation) showed much higher wax-myrtle funneling ratios, we wonder whether progressive mortality of overstory trees will increase shrub funneling ratios (year-round) and thereby further increase hummock net precipitation. For another example, these findings prompt us to ask how much water can be stored above floodwaters in these hummock soils, and how much of the net precipitation inputs are retained and held against gravity. More mechanistic research is warranted to understand the importance of hummock water balances in maintaining this precipitation-controlled and potentially water-limited ecotone.
Conclusion
Canopy interception losses were 9% of gross precipitation in a disturbed forested wetland in coastal South Carolina, measured across 69 storm events over a 15-months period. Despite these losses, net precipitation to hummocks equaled or exceeded gross precipitation (by an additional 0–35% of gross precipitation). These augmentations were a result of high stemflow rates in the shrub layer, which is assumed to have infiltrated into the hummocks on which the shrubs were rooted. Given that these shrubs are generally stressed by salinity, we present a novel hypothesis that the additional freshwater inputs provided by this localized net precipitation augmentation can facilitate the shrubs’ avoidance of using the mesohaline waters that surrounded the hummocks.
Data Availability Statement
The raw data supporting the conclusion of this article will be made available by the authors, without undue reservation.
Author Contributions
SA conceived and established the study, conducted the analysis, and wrote this manuscript. WC developed the study site and facilitated on-site activities, led the field sampling, and contributed to the editing of the manuscript. All authors contributed to the article and approved the submitted version.
Funding
Funding was provided by the Wetland Foundation Research Travel Grant, the CUAHSI Pathfinder Fellowship, and by the National Institute of Food and Agriculture, United States Department of Agriculture, under award number SCZ-1700590. Technical Contribution No. 6967 of the Clemson University Experiment Station.
Conflict of Interest
The authors declare that the research was conducted in the absence of any commercial or financial relationships that could be construed as a potential conflict of interest.
The handling editor declared a past co-authorship with one of the author SA.
Acknowledgments
Special thanks to the Baruch Foundation for use of their land during the study. We thank and remember Stephen “Hutch” Hutchinson for his assistance. Ryan Marsh and other Clemson University staff members also provided assistance. We also thank reviewers for their thoughtful comments and suggestions, which improved the manuscript.
Supplementary Material
The Supplementary Material for this article can be found online at: https://www.frontiersin.org/articles/10.3389/ffgc.2021.691321/full#supplementary-material
References
Allen, S. T., Aubrey, D. P., Bader, M. Y., Coenders-Gerrits, M., Friesen, J., Gutmann, E. D., et al. (2020). “Key questions on the evaporation and transport of intercepted precipitation,” in Precipitation Partitioning by Vegetation: A Global Synthesis, eds I. Van Stan, T. John, E. Gutmann, and J. Friesen (New York, NY: Springer International Publishing), 269–280. doi: 10.1007/978-3-030-29702-2_16
Allen, S. T., Keim, R. F., and Dean, T. J. (2019). Contrasting effects of flooding on tree growth and stand density determine aboveground production, in baldcypress forests. For. Ecol. Manage. 432, 345–355. doi: 10.1016/j.foreco.2018.09.041
Allen, S. T., Whitsell, M. L., and Keim, R. F. (2015). Leaf area allometrics and morphometrics in baldcypress. Can. J. For. Res. 45, 963–969. doi: 10.1139/cjfr-2015-0039
Andrus, R. E., Wagner, D. J., and Titus, J. E. (1983). Vertical zonation of Sphagnum mosses along hummock-hollow gradients. Can. J. Bot. 61, 3128–3139. doi: 10.1139/b83-352
Carlyle-Moses, D. E., and Gash, J. H. (2011). Rainfall interception loss by forest canopies, in: Forest Hydrology and Biogeochemistry. Netherlands: Springer, 407–423.
Carlyle-Moses, D. E., Iida, S., Germer, S., Llorens, P., Michalzik, B., Nanko, K., et al. (2020). Commentary: What we know about stemflow’s infiltration area. Front. For. Glob. Change 3:577247. doi: 10.3389/ffgc.2020.577247
Conner, W. H., Toliver, J. R., and Sklar, F. H. (1986). Natural regeneration of baldcypress (Taxodium distichum (L.) Rich.) in a Louisiana swamp. For. Ecol. Manage 14, 305–317. doi: 10.1016/0378-1127(86)90176-3
Cutini, A., Matteucci, G., and Mugnozza, G. S. (1998). Estimation of leaf area index with the Li-Cor LAI 2000 in deciduous forests. For. Ecol. Manage 105, 55–65. doi: 10.1016/S0378-1127(97)00269-7
Duberstein, J. A., and Conner, W. H. (2009). Use of hummocks and hollows by trees in tidal freshwater forested wetlands along the Savannah River. For. Ecol. Manage 258, 1613–1618. doi: 10.1016/j.foreco.2009.07.018
Duberstein, J. A., Krauss, K. W., Allen, S. T., and Baldwin, M. J. (2018). Sap flow data from a long-hydroperiod forested wetland undergoing salinity intrusion in South Carolina, USA: U.S. Geological Survey data release. doi: 10.5066/P9IR2XUO
Duberstein, J. A., Krauss, K. W., Baldwin, M. J., Allen, S. T., Conner, W. H., Salter, J. S., et al. (2020). Small gradients in salinity have large effects on stand water use in freshwater wetland forests. For. Ecol. Manage 473:118308. doi: 10.1016/j.foreco.2020.118308
Holwerda, F., Scatena, F. N., and Bruijnzeel, L. A. (2006). Throughfall in a Puerto Rican lower montane rain forest: A comparison of sampling strategies. J. Hydro. 327, 592–602. doi: 10.1016/j.jhydrol.2005.12.014
Hsueh, Y.-H., Chambers, J. L., Krauss, K. W., Allen, S. T., and Keim, R. F. (2016). Hydrologic exchanges and baldcypress water use on deltaic hummocks, Louisiana, USA. Ecohydrol 2016:1738. doi: 10.1002/eco.1738
Huenneke, L. F., and Sharitz, R. R. (1986). Microsite abundance and distribution of woody seedlings in a South Carolina cypress-tupelo swamp. Am. Midl. Nat. 115, 328–335. doi: 10.2307/2425869
Ish-Shalom, N., Sternberg, L., da, S. L., Ross, M., O’Brien, J., and Flynn, L. (1992). Water utilization of tropical hardwood hammocks of the Lower Florida Keys. Oecologia 92, 108–112. doi: 10.1007/BF00317270
Jones, R. H., Lockaby, B. G., and Somers, G. L. (1996). Effects of Microtopography and Disturbance on Fine-Root Dynamics in Wetland Forests of Low-Order Stream Floodplains. Am. Midl. Nat. 136, 57–71. doi: 10.2307/2426631
Keim, R. F., Skaugset, A. E., and Weiler, M. (2005). Temporal persistence of spatial patterns in throughfall. J. Hydrol. 314, 263–274. doi: 10.1016/j.jhydrol.2005.03.021
Levia, D. F., and Germer, S. (2015). A review of stemflow generation dynamics and stemflow-environment interactions in forests and shrublands. Rev. Geophys. 53:2015RG000479. doi: 10.1002/2015RG000479
Levia, D. F., Keim, R. F., Carlyle-Moses, D. E., and Frost, E. E. (2011). “Throughfall and stemflow in wooded ecosystems,” in Forest Hydrology and Biogeochemistry, Ecological Studies, eds D. F. Levia, D. Carlyle-Moses, and T. Tanaka (Netherlands: Springer), 425–443. doi: 10.1007/978-94-007-1363-5_21
Li, X.-Y., Yang, Z.-P., Li, Y.-T., and Lin, H. (2009). Connecting ecohydrology and hydropedology in desert shrubs: stemflow as a source of preferential flow in soils. Hydrol. Earth Syst. Sci. Discuss. 6, 1551–1580.
Liang, W.-L., Kosugi, K., and Mizuyama, T. (2009). A three-dimensional model of the effect of stemflow on soil water dynamics around a tree on a hillslope. J. Hydrol. 366, 62–75. doi: 10.1016/j.jhydrol.2008.12.009
Light, H. M., Darst, M. R., and Mattson, R. A. (2007). Ecological characteristics of tidal freshwater forests along the lower Suwannee River, Florida, in: Ecology of Tidal Freshwater Forested Wetlands of the Southeastern United States. Netherlands: Springer, 291–320. doi: 10.1007/978-1-4020-5095-4_11
Liu, X., Conner, W. H., Song, B., and Jayakaran, A. D. (2017). Forest composition and growth in a freshwater forested wetland community across a salinity gradient in South Carolina. USA. For. Ecol. Manage. 389, 211–219. doi: 10.1016/j.foreco.2016.12.022
Martinez-Meza, E., and Whitford, W. G. (1996). Stemflow, throughfall and channelization of stemflow by roots in three Chihuahuan desert shrubs. J. Arid. Environ. 32, 271–287. doi: 10.1006/jare.1996.0023
Miralles, D. G., Gash, J. H., Holmes, T. R. H., de Jeu, R. A. M., and Dolman, A. J. (2010). Global canopy interception from satellite observations. J. Geophys. Res. 115:D16122. doi: 10.1029/2009JD013530
Mużyło, A., Llorens, P., and Domingo, F. (2012). Rainfall partitioning in a deciduous forest plot in leafed and leafless periods. Ecohydrology 5, 759–767. doi: 10.1002/eco.266
Návar, J., and Bryan, R. (1990). Interception loss and rainfall redistribution by three semi-arid growing shrubs in northeastern Mexico. J. Hydrol. 115, 51–63. doi: 10.1016/0022-1694(90)90197-6
Rheinhardt, R. D., and Faser, K. (2001). Relationship between hydrology and zonation of freshwater swale wetlands on lower Hatteras Island, North Carolina, USA. Wetlands 21, 265–273. doi: 10.1672/0277-5212(2001)021[0265:rbhazo]2.0.co;2
Safeeq, M., and Fares, A. (2014). Interception losses in three non-native Hawaiian forest stands. Hydrol. Process. 28, 237–254. doi: 10.1002/hyp.9557
Shimamura, T., and Momose, K. (2005). Organic matter dynamics control plant species coexistence in a tropical peat swamp forest. Proc. R. Soc. Lon. B: Biol. Sci. 272, 1503–1510. doi: 10.1098/rspb.2005.3095
Siegert, C. M., and Levia, D. F. (2014). Seasonal and meteorological effects on differential stemflow funneling ratios for two deciduous tree species. J. Hydrol. 519, 446–454. doi: 10.1016/j.jhydrol.2014.07.038
Souther, R. F., and Shaffer, G. P. (2000). The effects of submergence and light on two age classes of baldcypress (Taxodium distichum (L.) Richard) seedlings. Wetlands 20, 697–706. doi: 10.1672/0277-5212(2000)020[0697:teosal]2.0.co;2
Spencer, S. A., and Van Meerveld, H. J. (2016). Double funnelling in a mature coastal British Columbia forest: spatial patterns of stemflow after infiltration. Hydrol. Process. 30, 4185–4201. doi: 10.1002/hyp.10936
Sternberg, L., da, S. L., Ish-Shalom-Gordon, N., Ross, M., and O’Brien, J. (1991). Water relations of coastal plant communities near the ocean/freshwater boundary. Oecologia 88, 305–310. doi: 10.1007/bf00317571
Stine, M. B., Resler, L. M., and Campbell, J. B. (2011). Ecotone characteristics of a southern Appalachian Mountain wetland. CATENA 86, 57–65. doi: 10.1016/j.catena.2011.02.006
Tolliver, K. S., Martin, D. W., and Young, D. R. (1997). Freshwater and saltwater flooding response for woody species common to barrier island swales. Wetlands 17, 10–18. doi: 10.1007/BF03160714
Van Stan, J. T. I., and Allen, S. T. (2020). What We Know About Stemflow’s Infiltration Area. Front. For. Glob. Change 3:61. doi: 10.3389/ffgc.2020.00061
Van Stan, J. T., and Gordon, D. A. (2018). Mini-Review: Stemflow as a resource limitation to near-stem soils. Front. Plant Sci 9:248. doi: 10.3389/fpls.2018.00248
Williams, B. J., Song, B., Williams, T. M., and Chow, A. (2014). “GIS analysis of impact of sea level change on a freshwater tidal forested wetland,” in Proceedings of the 9th Southern Forestry and Natural Resource Management GIS Conference. Presented at the Proceedings of the 9th Southern Forestry and Natural Resource Management GIS Conference, eds K. Merry, P. Bettinger, T. Brown, C. Cieszewski, I.-K. Hung, and Q. Meng (Athens, GA).
Keywords: ecohydrology, critical zone, precipitation partitioning, microtopography, coastal wetland, canopy interception
Citation: Allen ST and Conner WH (2021) Localized Augmentation of Net Precipitation to Shrubs: A Case Study of Stemflow Funneling to Hummocks in a Salinity-Intruded Swamp. Front. For. Glob. Change 4:691321. doi: 10.3389/ffgc.2021.691321
Received: 06 April 2021; Accepted: 15 June 2021;
Published: 07 July 2021.
Edited by:
Anna Klamerus-Iwan, University of Agriculture in Krakow, PolandReviewed by:
Krzysztof Owsiak, University of Agriculture in Krakow, PolandKelly Cristina Tonello, Federal University of São Carlos, Brazil
Copyright © 2021 Allen and Conner. This is an open-access article distributed under the terms of the Creative Commons Attribution License (CC BY). The use, distribution or reproduction in other forums is permitted, provided the original author(s) and the copyright owner(s) are credited and that the original publication in this journal is cited, in accordance with accepted academic practice. No use, distribution or reproduction is permitted which does not comply with these terms.
*Correspondence: Scott T. Allen, c2NvdHRhbGxlbkB1bnIuZWR1