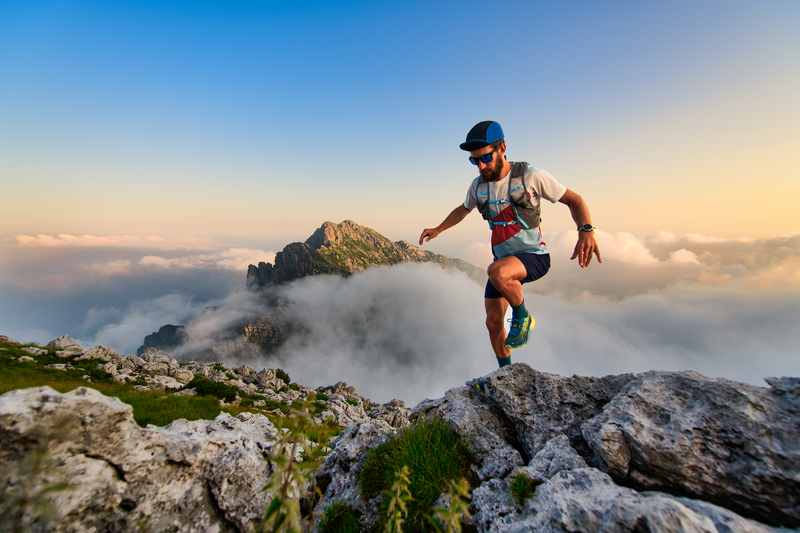
94% of researchers rate our articles as excellent or good
Learn more about the work of our research integrity team to safeguard the quality of each article we publish.
Find out more
ORIGINAL RESEARCH article
Front. For. Glob. Change , 02 December 2021
Sec. Forest Soils
Volume 4 - 2021 | https://doi.org/10.3389/ffgc.2021.691302
Warming can increase the efflux of carbon dioxide (CO2) from soils and can potentially feedback to climate change. In addition to warming, the input of labile carbon can enhance the microbial activity by stimulating the co-metabolism of recalcitrant soil organic matter (SOM). This is particularly true with SOM under invaded ecosystems where elevated CO2 and warming may increase the biomass of invasive species resulting in higher addition of labile substrates. We hypothesized that the input of labile carbon would instigate a greater soil organic carbon (SOC) loss with warming compared to the ambient temperature. We investigated this by incubating soils collected from a native pine (Pinus taeda) forest to which labile carbon from the invasive species kudzu (Pueraria lobata) was added. We evaluated the microbial extracellular enzyme activity, molecular composition of SOC and the temperature sensitivity of soil CO2 efflux under warming and labile carbon addition. After 14 months of soil incubation, the addition of labile C through kudzu extract increased the activity of β-1,4-glucosidase compared with the control. However, the activity of N-acetyl-β-D-glucosaminidase and fungal biomass (ergosterol) decreased with labile carbon addition. The activity of peroxidase increased with warming after 14 months of soil incubation. Although the carbon content of incubated soils did not vary with substrate and temperature treatments, the molecular composition of SOC indicated a general decrease in biopolymers such as cutin, suberin, long-chain fatty acids, and phytosterol with warming and an increasing trend of microbial-derived compounds with labile substrate addition. In soils that received an addition of labile C, the macro-aggregate stability was higher while the temperature sensitivity of soil C efflux was lower compared with the control. The increase in aggregate stability could enhance the physical protection of SOC from microbial decomposition potentially contributing to the observed pattern of temperature sensitivity. Our results suggest that warming could preferentially accelerate the decomposition of recalcitrant compounds while the addition of labile substrates could enhance microbial-derived compounds that are relatively resistant to further decomposition. Our study further emphasizes that global change factors such as plant invasion and climate change can differentially alter soil microbial activity and the composition of SOC.
Warming can accelerate the efflux of carbon dioxide (CO2) from the soil carbon pool to the atmospheric pool, potentially creating a positive feedback to climate change (Field et al., 2007; Wu et al., 2011). The magnitude of this feedback is partly determined by the temperature sensitivity of the microbial decomposition of soil organic matter (Conant et al., 2008; Suseela et al., 2012). Microbial metabolism is also positively influenced by the addition of labile carbon from plant litter. The input of labile carbon could subsidize the energy requirement of microbes for the production of oxidative enzymes. This in turn could stimulate the co-metabolism of relatively recalcitrant SOM (Kuzyakov et al., 2000) leading to the loss of soil C. Thus, both warming and the input of labile substrates could accelerate soil CO2 efflux, which could potentially feedback to climate change. Although the influence of warming and labile substrate addition on the quantity of soil carbon has been studied independently, less is known about the interactive effects of these two factors (Zhang et al., 2013) on soil carbon stocks and particularly the molecular composition of resulting SOC, which could have implications on the stability of soil carbon.
Global changes such as elevated CO2 and climate warming could differentially influence the primary producers and microbial decomposers that in turn could affect SOC storage. Elevated CO2 and warming often stimulates plant growth (Rustad et al., 2001; Jackson et al., 2009; Morgan et al., 2011) leading to a higher carbon input while warming directly influences microbial metabolism and extracellular enzyme activity through changes in soil temperature and/or moisture (Birgander et al., 2013). The interactive effects of these global change factors are particularly important for soil carbon pools in native ecosystems that have been subjected to the encroachment of exotic invasive plant species. This is because some of the invasive plant species possess greater physiological adaptability to warmer climates, enabling them to input a higher amount of labile biomass to the invaded ecosystems than the natives they displace (Barney et al., 2006; Liao et al., 2008; Laungani and Knops, 2009; Holcombe et al., 2010). Also, most of the invasive plant species exhibit a higher resource acquisition and resource use efficiency than the native species (Funk and Vitousek, 2007) producing a higher biomass per unit of available resources (Laungani and Knops, 2009). Moreover, the spread of invasive species is projected to increase exponentially with warmer climates due to climate-matching and superior physiology (Barney et al., 2006; Holcombe et al., 2010; Murray et al., 2012; Sorte et al., 2013). The longer growing season and better climate matching due to warming could result in increased input of fresh biomass that in turn facilitate higher microbial metabolism (Rothstein et al., 2004; Allison et al., 2010) reducing soil carbon storage in the invaded soils. Thus, the warming-induced increase in microbial metabolism can interact additively with the input of labile carbon by exotic plant species resulting in a greater impact on the storage of SOC in invaded ecosystems. The invasion of exotic plant species is a major global change factor that threatens the sustainability of native ecosystems. Previous studies have reported a decline in SOC in invaded ecosystems due to the input of labile carbon (Strickland et al., 2010; Tamura and Tharayil, 2014). However, the magnitude of this loss of soil C and subsequent composition of SOC as influenced by warmer temperature and labile C addition remains less known, as are the molecular-level changes in soil C composition due to microbial co-metabolism of native SOM.
Soil organic matter is a complex mixture of substrates with varying quality and decomposability. The chemical composition of substrates strongly influences the microbial C use efficiency (CUE), which is the ratio of mineralized vs. incorporated C as microbial biomass or by-product (Kallenbach et al., 2019). Substrates with lower quality (high C:N) and higher structural complexity have lower microbial carbon use efficiency (Cotrufo et al., 2013) leading to greater loss of carbon. The decomposition of these lower quality substrates (recalcitrant substrates) is more sensitive to temperature as they have higher activation energy (Davidson and Janssens, 2006). Thus, warming could accelerate the CO2 efflux from SOM by increasing the rate of enzymatic reaction and by reducing the microbial CUE (Conant et al., 2011). Considering the potential additive influence of the input of labile C and warmer temperatures on microbial metabolism, we hypothesized that the input of labile carbon would instigate a greater loss of soil carbon under warming than at ambient temperatures. Labile carbon addition would also alter the temperature sensitivity of soil carbon efflux. In the absence of labile carbon, a lower temperature sensitivity of microbially mediated soil carbon efflux has been reported (Yuste et al., 2003; Arevalo et al., 2010). However, other studies have reported that even under warming, the higher abundance of labile substrates in soil (Feng et al., 2008; Pisani et al., 2014) and plant litter (Suseela et al., 2013) reduced the decomposition of the recalcitrant fraction. In other words, the abundance of labile C may reduce the temperature sensitivity of SOM decomposition (Ghee et al., 2013). Moreover, the input of labile carbon and a higher rate of microbial by-product accumulation that enhances the aggregate stability may in turn reduce the temperature sensitivity of SOM degradation by reducing the accessibility of microbes to the substrate (Sollins et al., 1996; Park et al., 2007; Qin et al., 2019). Here we investigated the effect of warming and labile carbon addition on soil C efflux, the temperature sensitivity and the molecular composition of SOC. We also evaluated the combined effect of warming and addition of labile C substrates on microbial biomass and microbial extracellular enzyme activity. In this study, the difference in C quality between the labile substrate and the soil was achieved by adding labile litter-extracts (C:N = 19) from kudzu, a nitrogen-fixing invasive species (Pueraria lobata) to pine (Pinus taeda; C:N = 128) forest soil that was relatively enriched in recalcitrant compounds (Tamura et al., 2017).
We collected the soil samples for the incubation study from a Pinus taeda stand at the Clemson Experimental Forest, Clemson, SC, United States (SC34°44′N, 82°50′W). The top 5 cm of the mineral soil was collected using a soil corer (10 cm in diameter) after carefully removing the organic layer. Soils were then passed through a 2 mm-mesh sieve. A specimen cup (120 ml) was filled with 75 g of this soil and placed in a 1 L mason jar. At both temperature treatments (20 and 30°C), a set of 10 replicates received a substrate treatment of either water or kudzu extract every 2 months (see below for extract preparation) resulting in four treatments. We destructively harvested four and six replicates of each treatment at 6 and 14 months after incubation, respectively. The water content of the soil was maintained at 65% water holding capacity throughout the experiment by periodically weighing and adding nanopure water. The mason jars with the soil samples were periodically ventilated to maintain the CO2 concentration to <5% in the headspace.
A labile substrate was prepared from fresh kudzu leaves collected from the invaded site in Seneca, SC (SC34°41′N, 82°53′W). Briefly, 20 g of fresh kudzu leaves were blended with 250 ml of double deionized water and the slurry was sequentially filtered through a glass filter (1.6 μ m). To adjust the concentration of the leaf extract to be added to soils in each specimen cup, we added a known volume of the extract to a tin capsule, completely dried, and examined the C and N content using a continuous-flow isotope ratio mass spectrometer. The application of 2 ml of this extract provided 130 μg C g–1 soil that resulted in the maximum microbial activity before saturation (Bastida et al., 2013; Ghee et al., 2013). The amount was also equivalent to ∼0.25% of the total organic carbon in each specimen cup. The extract also provided 6.8 μg N g–1 at each application. The extract was added every 2 months for the entire 14-month incubation period. To compare the change in the quantity of native soil C and N in the samples after six and 14 months of incubation, the amount of C and N supplied through applications of kudzu extract was subtracted from the samples destructively harvested at six and 14 months of incubation.
The enzyme assays were conducted 14 days after substrate application at six and 14 months after incubation to capture the response of microbial activity to temperature and substrate addition treatments. The activity of hydrolase enzymes such as β-1,4-glucosidase (BG) that degrades cellulose and N-acetyl-β-D-glucosaminidase (NAG) that degrades chitin and peptidoglycan (Sinsabaugh et al., 1992) were quantified as per Sinsabaugh et al. (1999). The peroxidase enzyme was quantified to determine the lignolytic activity in the soil (Kirk and Farrell, 1987). Briefly, 800 mg of soil was combined with 150 ml of 50 mM sodium acetate (pH 5.5) using a high-speed blender for 1 min. Two hundred microliter of the soil slurry was transferred into a black 96-well plate. The plate also included 250 μl of the buffer as a blank sample. The hydrolase enzymes (BG and NAG) were quantified by adding 50 μl of 4-methylumbelliferyl β-D-glucopyranoside and 4-methylumbelliferyl N-acetyl β-D-glucosaminide as substrates, respectively. We used 200 μl of buffer and 50 μl of 4-methyl umbelliferone as a reference standard (Suseela et al., 2014). The enzyme activity was calculated following DeForest (2009). To quantify the activity of peroxidase, the above slurry containing buffer and soil (0.75 ml) was combined with 0.75 ml of the substrate L-3,4-dihydroxyphenylalanine (L-DOPA) and 0.2 ml of 0.3% hydrogen peroxide in a micro-centrifuge tube. For this assay, we used substrate with buffer (0.75 ml each) and slurry with buffer (0.75 ml each) as controls and a blank that contained 1.5 ml of the buffer alone. Both hydrolases and oxidases enzyme assay samples were incubated at 20°C for 2 h. At the end of 2 h of incubation, the hydrolase activity was quantified by measuring the absorbance at 365–450 nm (ex-em) using a microplate fluorimeter. The peroxidase activity was measured as absorption at 460 nm (ex) using a UV-VIS spectrophotometer.
We estimated bacterial biomass in the soils harvested at six months by quantifying the muramic acid. Fungal biomass was estimated by the abundance of ergosterol (Montgomery et al., 2000) at six and 14 months after incubation. To quantity the ergosterol content in soils, briefly, 5 g of soil was refluxed with 7.5 ml of 0.14 M methanoic potassium hydroxide at 82°C in glass tubes for 40 min. The supernatant (5 ml) was acidified to pH < 2 with 0.75 M HCl. The tubes were centrifuged at 2,500 × g for 15 min to sediment the humic precipitates and 6 ml of the supernatant was extracted with 1.2 ml of hexane on a rotary shaker for 5 min. One milliliter of the hexane phase was collected into a glass vial and dried completely under nitrogen gas. The dried content was further reconstituted in 100 μL of 0.1 M potassium hydroxide in isopropyl alcohol. The concentration of the extract was determined using high pressure liquid chromatography (HPLC). With an Onyx C18 column (monolithic silica, 130 Å; 100 mm × 4.6 mm I.D.; Phenomenex, Torrance, CA, United States) and a methanol-water (99:1, v/v) solvent at a flow rate of 1 ml min–1, the ergosterol was detected by the absorbance of conjugated double bond at 282 nm.
To extract muramic acid, briefly, 3 g of soil was hydrolyzed with 10 ml of 6 N HCl for 6 h at 100°C and were then acidified and centrifuged to remove the humic precipitates. Prior to analysis on HPLC, the samples were derivatized. We combined 400 μL of the extract with 50 μL of ortho-phthalaldehyde (OPA) reagent (1 M sodium borate containing 40 mM OPA and 0.01% of β -mercaptoethanol) and the reaction was ceased at 1 min using 30 μL of 10% acetic acid (Tharayil et al., 2013). The derivatized samples were immediately analyzed on HPLC using a Synergy Polar-RP column (ether-linked phenyl, 4 μm, 80 Å; 250 mm × 4.6 mm I.D.; Phenomenex, Torrance, CA, United States). We used a binary gradient elution of 5 mM ammonium acetate (mobile phase A) with a linear increase of mobile-phase B (100% methanol) from 0% at 0 min to 40% at 20 min at a flow rate of 0.5 ml min–1. Muramic acid was quantified using a fluorescence detector (Ex-Em = 340 nm and 455 nm, respectively). Using a triple quadrupole mass spectrometer (reaction monitoring m/z 252– > 144 and m/z 252- > 126), the muramic acid in samples was verified.
Lipids and phenolics were extracted using solvent extraction, base hydrolysis, and copper oxide oxidation of the mineral soils to compare the composition of soil organic C sources as either plant or microbial origin (Otto et al., 2005). The biomarker approach can provide compound-specific information and facilitate the classification of compounds of the extractable fraction in SOM to plant/microbial origins (Simpson et al., 2008; Zhang and Suseela, 2021). Kudzu litter extract did not contain any measurable amount of recalcitrant plant polymers, thus no adjustment was made for the addition of kudzu extract on the concentrations of the lipids and phenolics.
We combined 300 mg of dry soil sample with 3 mL of 100% methanol and sonicated in water bath for 30 min. The sample was centrifuged, and the supernatant was collected. The residue soil sample was sequentially extracted with 3 mL of dichloromethane/methanol (1:1 v/v) and 100% dichloromethane by following the same procedure, and all the solvent extracts were combined. We added 7 ml of DDI water to the extract and the content was gently shaken. The methylene chloride phase (1.5 ml) was collected and completely dried. The samples were stored at −20°C until analysis.
To the subsamples of solvent extracted soil, 3 ml of 1N methanolic sodium hydroxide was added and heated at 95°C for 3 h. After cooling to room temperature, the supernatant was collected. The residue soil was combined with 2 ml of 100% methanol and sonicated in a water bath for 30 min, and the supernatant was combined with the previous base solvent. The extraction was repeated with 2 mL of methylene chloride. To the combined extract sample, 100 μl of nonadecane (100 μg ml–1 in 100% methanol) was added as an internal standard. We added 2 ml of 50% HCl to the sample before adding 7 mL of DDI water. We collected 500 μl of the methylene chloride fraction and stored at −20°C until analysis.
Briefly, the base extracted soil equivalent to roughly 25 mg of soil C, was combined with 1 g CuO, 150 mg ferrous ammonium sulfate, and 15 ml of 2 M NaOH that had been sparged for 20 min with argon, into a 23 ml Teflon-lined acid digestion vessel. Further, each vessel was sparged for an additional 15 min before closing. The samples were heated to 160°C for 160 min and then allowed to cool to room temperature. Upon cooling, the supernatants were transferred and 100 μl of the internal standards (cinnamic acid and ethylvanillin prepared as 200 μg ml–1 in 100% methanol) were added to each sample, followed by the addition of 3 ml of 18 N H2SO4. Twelve milliliters of the supernatant was extracted with 1 ml of ethyl acetate, and the ethyl acetate fraction was collected and stored at −20°C for further analysis.
The dried extract from the solvent extraction was reconstituted in 1 ml of methanol and methylene chloride (1:1) solution. Fifty microliters each of free and bound lipid and lignin extract were each transferred separately to glass vials with 250 μl inserts and completely dried under nitrogen gas. The samples were then silylated with 200 μL of N-methyl-N-(trimethylsilyl) trifluoroacetamide (MSTFA) with 1% trimethylchlorosilane (TMCS) at 65°C for 30 min. The silylated samples were analyzed using an Agilent 7980A GC system coupled with a 5975 C Series mass detector. The separation of the compounds was achieved on a DB-5 MS fused-silica capillary column (30 m length × 0.25 mm internal diameter × 0.20 μm film thickness) using a split (1:10) injection (1 μl). For the free and bound lipids, the samples were run at 65°C for 2 min followed by a linear ramp of 6°C per min to 300°C and held at 300°C for 15 min. The samples for lignin estimation were analyzed at 80°C for 2 min followed by a linear ramp of 10°C per min to 300°C. The carrier gas (He) was maintained at a constant pressure of 10 psi, the injection port and the MS interphase were maintained at 280°C, the MS quad temperature was maintained at 150°C and MS source temperature was set at 230°C. The electron multiplier was operated at a constant gain of 10 (EMV = 1478V) and the scan range was set at 50–600 amu resulting in 2.66 scans sec–1.
Aggregate stability was assessed by chemical dispersion as per Wright and Upadhyaya (1998). Briefly, the soil was wet sieved using a 250 μm sieve to obtain the macro-aggregate fraction (250–2,000 μm). Four grams of the macro-aggregate fraction was then mixed with 50 ml of 5% sodium hexametaphosphate, and dispersed on a rotary shaker for 20 h at 20°C. The samples were centrifuged, and supernatant was discarded. The residue soil fraction was dried at 45°C and weighed. The initial and final weight of the macroaggregate sample was used to calculate the stable aggregate fraction. The aggregate stability was conducted only on soils harvested at 6 months since soils incubated for 14 months were prioritized for all other analyses.
We measured CO2 efflux from soils incubated for 12 months immediately after the application of substrate (kudzu extract or water treatments). Initially, the measurements were taken at times 0, 9, 24, 33, and 48 h with subsequent measurements taken every 48 h and terminated at 1,008 h (42 days). To measure the CO2 concentration, 5 ml of the head space of the mason jar was collected using a gas tight syringe and injected into an infrared gas analyzer (EGM-4, PP-Systems, Amesbury, MA, United States). The data was recorded as an average of two consecutive measurements for each sample. Temperature sensitivity of the water and kudzu applied soils expressed as Q10 was calculated using the following equation:
where T1 is the base temperature (20°C) and T2 is the elevated temperature (30°C). C1 and C2 are the CO2 concentrations of 20 and 30°C soil within the same substrate treatment, respectively.
The data were log transformed wherever necessary to meet the assumptions of normality. Two-way analysis of variance (ANOVA) was conducted to test the main and interactive effects of temperature (20 or 30°C) and substrate (water or kudzu extract) treatments on soil organic C, carbon to nitrogen ratio, enzyme activity, microbial biomass, plant and microbial biomarkers, and aggregate stability from the samples destructively harvested at six and 14 months of incubation. Statistically significant differences (α < 0.05) were further subjected to Tukey’s HSD multi-comparison test to obtain difference between individual treatments.
At six months, both temperature and substrate treatments did not affect the total carbon content of the incubated soils (Supplementary Figure 1). However, at 14 months, the carbon content of pine soil exhibited a decreasing trend in soils incubated at 30°C compared with soils at 20°C but was not significant (P = 0.13; Supplementary Figure 1).
Indicators of fungal and bacterial biomass (ergosterol and muramic acid, respectively) were quantified from the soil samples destructively harvested at six months after incubation. The concentration of ergosterol varied with the main effect of temperature (Figure 1A) and substrate treatments (Figure 1B). The ergosterol content of soils incubated at 30°C was 50% less than soils incubated at 20°C (Figure 1A; P = 0.02) and soils amended with kudzu extract had higher ergosterol content than soils added with water (control; Figure 1B; P = 0.05). The concentration of muramic acid showed a decreasing trend with the application of kudzu extract (Figure 1C; P = 0.07). At 14 months after incubation, higher amount of ergosterol was quantified from water applied soil (Figure 1D; 35%) than the kudzu applied soil (P = 0.03).
Figure 1. The amount of ergosterol (A,B) and muramic acid (C) in soil samples at 6-months and ergosterol (D) at 14-months after incubation. Bars with different lowercase letters indicate a difference (Tukey’s HSD) between treatments. Values represent means ± SE (n = 4).
At six months after incubation, β-glucosidase, a cellulolytic enzyme, exhibited higher activity (23%) in soils that received kudzu extract and incubated at 30°C (Figure 2A; P = 0.03). The activity of NAG, a chitinase enzyme had 27% higher activity than soils at 30°C (P = 0.03) and soils that received the input of kudzu extract had 25% higher activity than the control (P = 0.03, Figures 2B,C). However, the activity of peroxidase, one of the lignolytic oxidative enzymes did not vary with treatments (P > 0.05; data not shown) after six months of incubation.
Figure 2. Activity of enzymes at 2 weeks after labile extract addition on soils incubated for 6 months: (A) β-glucosidase and (B,C) N-acetyl-glucosaminidase (NAG; values represent means ± SE; n = 4) and the activity of enzymes at 2 weeks after labile extract addition on soils incubated for 14 months: (D,E) β-glucosidase, (F) N-acetyl-glucosaminidase (NAG), and (G) peroxidase (values represent means ± SE; n = 6). Bars with different lowercase letters indicate a difference (Tukey’s HSD) between treatments. Asterisk in Figure 1A indicates a difference (Tukey’s HSD) between substrate treatment at 30°C.
The enzyme activities in soils harvested at 14 months did not follow the trend as those at six months. The activity of BG was 20% lower in soils incubated at 30°C than those at 20°C (P < 0.02; Figure 2D) and was 16% higher with the addition of kudzu extract (P = 0.03; Figure 2E) compared to soils that received water. The substrate addition treatment affected the activity of NAG where soils that received kudzu extract had 20% lower activity than water applied soils (P = 0.01; Figure 2F). Unlike the activity at six months, after 14 months of incubation the peroxidase enzyme activity in soil incubated at 30°C was 43% higher compared to soils incubated at 20°C (P = 0.04; Figure 2G).
The mass of stable aggregate fraction was quantified by chemical dispersion of the macro-aggregate fraction in soils harvested at six months (Figure 3). The mass of macroaggregate that remained after dispersion was 13% higher in soils that received kudzu extract than the control soils (P < 0.01).
Figure 3. Stability of macro-aggregates in soils collected after 6 months of incubation. Bars with different lowercase letters indicate a difference (Tukey’s HSD) between treatments. Values represent means ± SE (n = 4).
Change in the molecular level composition of organic carbon was compared using the concentration of the extractable plant and microbial derived lipid biomarkers (Figures 4A–F). Among the five different compound classes that were identified, both suberin (19.8%; Figure 4B) and phytosterol (18%; Figure 4D) decreased significantly with higher temperature (P < 0.05) while cutin (13.5%; P = 0.06; Figure 4A) and LFA (17.4%; P = 0.07; Figure 4C) decreased marginally with higher temperature. Soils added with kudzu extract had marginally higher content of phytosterol (20%; Figure 4E) and SFA (17%; P = 0.06; Figure 4F) than the control soils. The concentration of lignin derived monomers such as syringyl, vanillyl, and cinnamyl phenols (SVC) were marginally lower (12%) in soils incubated at 30°C compared to soils at 20°C (P = 0.07; Figure 5).
Figure 4. Plant biomarkers quantified at 14 months after incubation. (A) ΣCutin, ([C14-C18 hydroxyalkanoic acid, C16-di-hydroalkanoic acid, ω- hydroxy-, and ω-hydroxy-epoxy alkanoic acids (C16-C18)]; (B) ΣSuberin, [α, ω-dicarboxilic acids (C16-C24; saturated and substituted) and ω-hydroxyalkanoic acids (C20-C30; saturated and substituted) Suseela et al., 2017]; (C) ΣLFA, long-chain fatty acids (>C24 alkanes, >C22 n-alkanoic acids, and alkanols); (D,E) ΣPhytosterols (stigmasterol and β-sitosterol) (F) ΣSFA, short-chain fatty acid, sum of [C10-C18 n-alkanoic and n-alkanoic acid, ω- hydroxy-, and ω-hydroxy-epoxy alkanoic acids (C16-C18) Tamura et al., 2017]. Asterisk indicate a difference (Tukey’s HSD) between treatments. Values represent means ± SE (n = 3).
Figure 5. Concentration of total lignin monomers in soils after CuO oxidation (SVC represents the sum of syringyl, vanillyl, and cinnamyl phenols). Values represent means ± SE (n = 4).
Temperature sensitivity of the CO2 efflux (Q10) between the substrate treatments (Figure 6) were compared using the cummulative CO2 efflux from the two temperatures (eq. 1). The higher Q10 value for kudzu applied soil was only observed during the first 24 h. After one week of the substrate addition (168 h), the Q10 value was stabilized at around 1.4 for soils that received kudzu input. The control (water) samples showed a linear increase of Q10 until 2 weeks after application (336 h) and stabilized at ∼ 2.4 at the end of the incubation.
Figure 6. Temperature sensitivity (Q10), based on the increase in cumulative CO2 efflux with 10°C increase in temperature. Opened and closed symbols represent the values from water and kudzu extract, respectively.
Warming is projected to increase the catalytic activity of enzymes (Davidson and Janssens, 2006) and decrease the microbial CUE while decomposing substrates of low quality (Conant et al., 2011) leading to the release of soil carbon. Furthermore, the higher input of labile carbon through litter fall promotes microbial decomposition of native SOM in turn reducing the C storage in invaded soils (Strickland et al., 2010; Tamura and Tharayil, 2014). Thus, combined warming and labile C input could have an additive effect on soil C loss. We hypothesized that the input of labile carbon would instigate a greater soil C loss under elevated temperature than at ambient temperature. However, after 14 months of incubation the addition of labile substrates did not additively decrease soil carbon content. Although field studies have exhibited greater decrease of native carbon content due to labile litter input from invasive species, the observed trend of carbon quantity may have been potentially due to the relatively shorter incubation time of the study (Dungait et al., 2012). Our results exhibited only a trend of decreasing carbon with warming treatment (Supplementary Figure 1) suggesting that a longer duration of incubation may result in significant differences in carbon stocks.
Although warming and substrate addition treatments did not affect the quantity of carbon, our study revealed differences in microbial community and enzyme activity at different temperatures that potentially affected the composition of SOC. At six months after incubation, an increase in the activity of BG with the addition of kudzu extract was observed only in soils that were incubated at 30°C indicating that labile substrate input could alter the temperature response of enzyme activity (Fissore et al., 2013). However, after 14 months of incubation when the soils became more recalcitrant, warming decreased the activity of BG while kudzu addition increased the activity. The addition of labile substrate would increase the soil microbial activity as evident from the higher activity of BG after 14 months of incubation in soils that received kudzu extract (Figure 2B). Similar, higher activity of glucosidase has been reported following the addition of substrates with low C:N ratios (Fog, 1988; Berg and Matzner, 1997; Bastida et al., 2013).
The increase in fungal biomass (ergosterol; Figure 1A) in soils that received kudzu extract, after six months of incubation subsequently increased the activity of NAG (Figure 2C) which releases N from chitin present in fungal cell walls (Leake and Read, 1990). As fungi are more capable of degrading recalcitrant fraction (plant heteropolymers) than bacteria (Sinsabaugh, 2010), the periodic input of kudzu (labile) substrate with high N may have suppressed the fungal biomass (Figure 1D) resulting in lower NAG activity at 14 months after incubation (Figure 2F). Previous study from the same pine forest site has reported a decrease in fungal biomass when pine soils were invaded by kudzu (Tamura and Tharayil, 2014). Soil peroxidase activity did not vary with the input of kudzu extract at either 6 or 14 months after incubation. However, at 14 months after incubation, the peroxidase activity increased with warming (Figure 2G) leading to a corresponding decrease in the content of lignin (Figure 5). A recent climate warming experiment reported a warming induced shift in fungal community by selecting fungi with a higher capacity to depolymerize recalcitrant carbon (Treseder et al., 2016).
As the initial study soils from pine forest were characterized by higher abundance of cutin, wax and suberin-derived lipids (Tamura and Tharayil, 2014), the molecular biomarker analysis of soils at 14 months after incubation delineated the change in SOC composition with temperature and substrate addition (Figure 4). The biomarker analysis revealed that warming resulted in the loss of plant derived compounds such as cutin, suberin, phytosterol and LFA (Figure 4), which are otherwise slower to decompose and often preserved in soil (von Lützow et al., 2006). Warming could increase the kinetics of reaction catalyzed by the microbes (Davidson and Janssens, 2006), thus higher response in degradation rate was anticipated for recalcitrant fraction that requires higher activation energy (Suseela et al., 2013). Similar reduction of aliphatic compounds such as cutin and suberin with warming was observed in the field manipulation experiment (Pisani et al., 2014). Moreover, the concentration of lignin monomers was also lower in soils incubated at 30°C than 20°C (Figure 5) potentially due to the observed higher activity of soil peroxidase (Figure 2G). Recent meta-analysis has also reported increased ligninase activity with warming (Chen et al., 2018). Similar, lower concentration of lignin with warming treatment was observed in previous studies (Feng et al., 2008; Pisani et al., 2014) and greater carbon loss due to warming was reported to be correlated with increased ratios of ligninase to cellulase activity (Chen et al., 2020). Although warming increased the degradation of plant heteropolymers, the input of labile C resulted in the accumulation of microbial products (Cotrufo et al., 2013; Tamura et al., 2017) evident from the marginally higher abundance of short-chain fatty acids (Figure 4F) in soils that received kudzu extract. The increase in SFA could indicate higher concentration of degradation products of plant-derived long-chain fatty acids and/or bacterial biomass (Otto et al., 2005). Overall, the molecular composition of resultant soil delineated the impact of warming in decreasing the abundance of recalcitrant fraction of soil C, while the input of labile extract increased the microbial products which are potentially more stable as it can form organo-mineral associations (Cotrufo et al., 2013, 2015; Tamura et al., 2017; Sokol et al., 2019). These results suggest that future warming and addition of labile substrates may affect soil carbon by differentially influencing the utilization of carbon compounds of varying lability.
Soil organic matter is a highly heterogeneous matrix composed of both labile and recalcitrant compounds. Although recalcitrant C has a higher temperature sensitivity (Davidson and Janssens, 2006), microbes could preferentially decompose labile fraction within the soil (Feng et al., 2008; Pisani et al., 2014) or litter matrix (Suseela et al., 2013). Thus, the labile C addition could reduce the temperature sensitivity of the SOC mineralization rate by hindering the decomposition of recalcitrant fraction. For example, the quantity of phytosterol was higher in soils that received kudzu-extract compared to the control (Figure 4E). Since kudzu leaf tissue contained negligible amount of phytosterol (Tamura and Tharayil, 2014), this observation could indicate that the abundance of labile C reduced the degradation of phytosterol. Therefore, decomposition of recalcitrant compounds could be hindered by labile C (kudzu-extract), and overall temperature sensitivity could be consequently lower for soils that received labile extract.
We observed a lower temperature coefficient (Q10) for the kudzu added soil compared to the control soils (Figure 6). The immediate increase in Q10 after the labile substrate input is often observed due to the activity of fast-growing bacteria responding to increased labile nutrient source, that diminish within the first 24 h (Dungait et al., 2013; Thiessen et al., 2013). The rate of carbon mineralization through microbial decomposition of SOC is influenced by the quality of the substrates, and the accessibility of microbes to the substrates (von Lützow et al., 2006). Soils that received kudzu substrate had greater macro-aggregate stability compared to the control (Figure 3). The enhancement of aggregate stability and reduced SOM mineralization rate (Park et al., 2007) and subsequent lower temperature sensitivity with the input of labile C was also reported previously (Qin et al., 2019). Therefore, these results suggest that labile substrate input could promote physical protection of the SOC that partly would have contributed to a lower temperature sensitivity of CO2 efflux.
Climate warming and invasion of exotic plant species are two major global change factors that threaten the sustainability of native ecosystems. Our study examined the interactive effects of warming and labile substrate input by invasive plants on the quantity and composition of native SOC through changes in microbial biomass and enzyme activity. Although the predicted additive impact of warming and input of labile C in reducing soil C was not evident, the study demonstrated that the input of labile C increased aggregate stability, abundance of short chain fatty acids and decreased the temperature sensitivity of soil C efflux. Conversely, warming increased peroxidase activity and altered the soil carbon composition by decreasing the abundance of recalcitrant plant polymers such as cutin, suberin and lignin consistent with the kinetics theory. The decomposition of chemically complex and recalcitrant carbon would increase the soil carbon efflux and have the potential to accelerate warming. Our results thus indicate that the input of labile C from invader biomass and warmer climates could affect microbial activity and the molecular-level soil carbon composition which could alter SOC storage in invaded ecosystems.
The raw data supporting the conclusions of this article will be made available by the authors, without undue reservation.
VS and MT conceived and designed the study. MT conducted the experiment and wrote the draft of the manuscript with input from VS. Both authors approved the submitted version.
This work was supported by the National Institute of Food and Agriculture, United States Department of Agriculture, under award number 2017-67014-26698. Clemson library has provided funding to cover the publication cost. This is Technical Contribution No. 6997 of the Clemson University Experiment Station.
The authors declare that the research was conducted in the absence of any commercial or financial relationships that could be construed as a potential conflict of interest.
All claims expressed in this article are solely those of the authors and do not necessarily represent those of their affiliated organizations, or those of the publisher, the editors and the reviewers. Any product that may be evaluated in this article, or claim that may be made by its manufacturer, is not guaranteed or endorsed by the publisher.
The Supplementary Material for this article can be found online at: https://www.frontiersin.org/articles/10.3389/ffgc.2021.691302/full#supplementary-material
Allison, S. D., Wallenstein, M. D., and Bradford, M. A. (2010). Soil-carbon response to warming dependent on microbial physiology. Nat. Geosci. 3, 336–340. doi: 10.1038/ngeo846
Arevalo, C. B. M., Bhatti, J. S., Chang, S. X., Jassal, R. S., and Sidders, D. (2010). Soil respiration in four different land use systems in north central Alberta, Canada. J. Geophys. Res.-Biogeosci. 115: G01003.
Barney, J. N., Tharayil, N., DiTommaso, A., and Bhowmik, P. C. (2006). Thebiology of invasive alien plants in Canada. 5. Polygonum cuspidatum Sieb. & Zucc. [= Fallopia japonica (Houtt.) Ronse Decr.]. Can. J. Plant Sci. 86, 887–905. doi: 10.4141/p05-170
Bastida, F., Torres, I. F., Hernández, T., Bombach, P., Richnow, H. H., and García, C. (2013). Can the labile carbon contribute to carbon immobilization in semiarid soils? Priming effects and microbial community dynamics. Soil Biol. Biochem. 57, 892–902. doi: 10.1016/j.soilbio.2012.10.037
Berg, B., and Matzner, E. (1997). Effect of N deposition on decomposition of plant litter and soil organic matter in forest systems. Environ. Rev. 5, 1–25. doi: 10.1139/a96-017
Birgander, J., Reischke, S., Jones, D. L., and Rousk, J. (2013). Temperature adaptation of bacterial growth and 14C-glucose mineralisation in a laboratory study. Soil Biol. Biochem. 65, 294–303. doi: 10.1016/j.soilbio.2013.06.006
Chen, J., Elsgaard, L., van Groenigen, K. J., Olesen, J. E., Liang, Z., Jiang, Y., et al. (2020). Soil carbon loss with warming: new evidence from carbon-degrading enzymes. Glob Change Biol. 26, 1944–1952. doi: 10.1111/gcb.14986
Chen, J., Luo, Y., García-Palacios, P., Cao, J., Dacal, M., Zhou, X., et al. (2018). Differential responses of carbon-degrading enzyme activities to warming: implications for soil respiration. Glob Change Biol. 24, 4816–4826. doi: 10.1111/gcb.14394
Conant, R. T., Ryan, M. G., Ågren, G. I., Birge, H. E., Davidson, E. A., Eliasson, P. E., et al. (2011). Temperature and soil organic matter decomposition rates: synthesis of current knowledge and a way forward. Global Change Biol. 17, 3392–3404. doi: 10.1111/j.1365-2486.2011.02496.x
Conant, R. T., Steinweg, J. M., Haddix, M. L., Paul, E. A., Plante, A. F., and Six, J. (2008). Experimental warming shows that decomposition temperature sensitivity increases with soil organic matter recalcitrance. Ecology 89, 2384–2391. doi: 10.1890/08-0137.1
Cotrufo, M. F., Soong, J. L., and Horton, A. J. (2015). Formation of soil organic matter via biochemical and physical pathways of litter mass loss. Nat. Geosci. 8, 776–779. doi: 10.1038/ngeo2520
Cotrufo, M. F., Wallenstein, M. D., Boot, C. M., Denef, K., and Paul, E. (2013). The microbial efficiency-matrix stabilization (MEMS) framework integrates plant litter decomposition with soil organic matter stabilization: do labile plant inputs form stable soil organic matter? Global Change Biol. 19, 988–995. doi: 10.1111/gcb.12113
Davidson, E., and Janssens, I. (2006). Temperature sensitivity of soil carbon decomposition and feedbacks to climate change. Nature 440, 165–173. doi: 10.1038/nature04514
DeForest, J. L. (2009). The influence of time, storage temperature, and substrate age on potential soil enzyme activity in acidic forest soils using MUB-linked substrates and L-DOPA. Soil Biol. Biochem. 41, 1180–1186.
Dungait, J. A., Kemmitt, S. J., Michallon, L., Guo, S., Wen, Q., Brookes, P., et al. (2013). The variable response of soil microorganisms to trace concentrations of low molecular weight organic substrates of increasing complexity. Soil Biol. Biochem. 64, 57–64. doi: 10.1016/j.soilbio.2013.03.036
Dungait, J. A. J., Hopkins, D. W., Gregory, A. S., and Whitmore, A. P. (2012). Soil organic matter turnover is governed by accessibility not recalcitrance. Global Change Biol. 18, 1781–1796. doi: 10.1111/j.1365-2486.2012.02665.x
Feng, X., Simpson, A. J., Wilson, K. P., Williams, D. D., and Simpson, M. J. (2008). Increased cuticular carbon sequestration and lignin oxidation in response to soil warming. Nat. Geosci. 1, 836–839. doi: 10.1038/ngeo361
Field, C. B., Lobell, D. B., Peters, H. A., and Chiariello, N. R. (2007). Feedbacks of terrestrial ecosystems to climate change. Annu. Rev. Env. Resour. 32, 1–29. doi: 10.1146/annurev.energy.32.053006.141119
Fissore, C., Giardina, C. P., and Kolka, R. K. (2013). Reduced substrate supply limits the temperature response of soil organic carbon decomposition. Soil Biol. Biochem. 67, 306–311. doi: 10.1016/j.soilbio.2013.09.007
Fog, K. (1988). The effect of added nitrogen on the rate of decomposition of organic matter. Biol. Rev. 63, 433–462. doi: 10.1111/j.1469-185x.1988.tb00725.x
Funk, J., and Vitousek, P. (2007). Resource-use efficiency and plant invasion in low-resource systems. Nature 446, 1079–1081. doi: 10.1038/nature05719
Ghee, C., Neilson, R., Hallett, P. D., Robinson, D., and Paterson, E. (2013). Priming of soil organic matter mineralisation is intrinsically insensitive to temperature. Soil Biol. Biochem. 66, 20–28. doi: 10.1016/j.soilbio.2013.06.020
Holcombe, T. R., Stohlgren, T. J., and Jarnevich, C. S. (2010). From points to forecasts: predicting invasive species habitat suitability in the near term. Diversity 2010, 738–767. doi: 10.3390/d2050738
Jackson, R. B., Cook, C. W., Pippen, J. S., and Palmer, S. M. (2009). Increased belowground biomass and soil CO2 fluxes after a decade of carbon dioxide enrichment in a warm-temperate forest. Ecology 90, 3352–3366. doi: 10.1890/08-1609.1
Kallenbach, C. M., Wallenstein, M. D., Schipanksi, M. E., and Grandy, S. A. (2019). Managing agroecosystems for soil microbial carbon use efficiency: ecological unknowns, potential outcomes, and a path forward. Front. Microbiol. 10:1146. doi: 10.3389/fmicb.2019.01146
Kirk, T. K., and Farrell, R. L. (1987). Enzymatic” combustion”: the microbial degradation of lignin. Annu. Rev. Microbiol. 41, 465–501. doi: 10.1146/annurev.mi.41.100187.002341
Kuzyakov, Y., Friedel, J., and Stahr, K. (2000). Review of mechanisms and quantification of priming effects. Soil Biol. Biochem. 32, 1485–1498. doi: 10.1016/s0038-0717(00)00084-5
Laungani, R., and Knops, J. M. H. (2009). Species-driven changes in nitrogen cycling can provide a mechanism for plant invasions. Proc. Natl. Acad. Sci. U.S.A. 106, 12400–12405. doi: 10.1073/pnas.0900921106
Leake, J. R., and Read, D. J. (1990). Chitin as a nitrogen source for mycorrhizal fungi. Mycol. Res. 94, 993–995. doi: 10.1016/s0953-7562(09)81318-x
Liao, C., Peng, R., Luo, Y., Zhou, X., Wu, X., Fang, C., et al. (2008). Altered ecosystem carbon and nitrogen cycles by plant invasion: a meta-analysis. N. Phytol. 177, 706–714. doi: 10.1111/j.1469-8137.2007.02290.x
Montgomery, H. J., Monreal, C. M., Young, J. C., and Seifert, K. A. (2000). Determination of soil fungal biomass from soil ergosterol analyses. Soil Biol. Biochem. 32, 1207–1217. doi: 10.1016/s0038-0717(00)00037-7
Morgan, J. A., LeCain, D. R., Pendall, E., Blumenthal, D. M., Kimball, B. A., Carrillo, Y., et al. (2011). C4 grasses prosper as carbon dioxide eliminates desiccation in warmed semi-arid grassland. Nature 476, 202–205. doi: 10.1038/nature10274
Murray, J. V., Stokes, K. E., and Klinken, R. D. (2012). Predicting the potential distribution of a riparian invasive plant: the effects of changing climate, flood regimes and land-use patterns. Global Change Biol. 18, 1738–1753. doi: 10.1111/j.1365-2486.2011.02621.x
Otto, A., Shunthirasingham, C., and Simpson, M. J. (2005). A comparison of plant and microbial biomarkers in grassland soils from the prairie ecozone of canada. Organic Geochem. 36, 425–448. doi: 10.1016/j.orggeochem.2004.09.008
Park, E., Sul, W. J., and Smucker, A. J. M. (2007). Glucose additions to aggregates subjected to drying/wetting cycles promote carbon sequestration and aggregate stability. Soil Biol. Biochem. 39, 2758–2768. doi: 10.1016/j.soilbio.2007.06.007
Pisani, O., Hills, K. M., Courtier-Murias, D., Haddix, M. L., Paul, E. A., Conant, R. T., et al. (2014). Accumulation of aliphatic compounds in soil with increasing mean annual temperature. Organic Geochem. 76, 118–127. doi: 10.1016/j.orggeochem.2014.07.009
Qin, A., Chen, L., Fang, K., Zhang, Q., Wang, J., Liu, F., et al. (2019). Temperature sensitivity of SOM decomposition governed by aggregate protection and microbial communities. Sci. Adv. 5:eaau1218. doi: 10.1126/sciadv.aau1218
Rothstein, D. E., Vitousek, P. M., and Simmons, B. L. (2004). An exotic tree alters decomposition and nutrient cycling in a Hawaiian montane forest. Ecosystems 7, 805–814. doi: 10.1007/s10021-004-0009-y
Rustad, L., Campbell, J., Marion, G., Norby, R., Mitchell, M., Hartley, A., et al. (2001). A meta-analysis of the response of soil respiration, net nitrogen mineralization, and aboveground plant growth to experimental ecosystem warming. Oecologia 126, 543–562. doi: 10.1007/s004420000544
Simpson, M. J., Otto, A., and Feng, X. (2008). Comparison of solid-state Carbon-13 nuclear magnetic resonance and organic matter biomarkers for assessing soil organic matter degradation. Soil Sci. Soc. Am. J. 72, 268–276. doi: 10.2136/sssaj2007.0045
Sinsabaugh, R. L. (2010). Phenol oxidase, peroxidase and organic matter dynamics of soil. Soil Biol. Biochem. 42, 391–404. doi: 10.1016/j.soilbio.2009.10.014
Sinsabaugh, R. L., Antibus, R. K., Linkins, A. E., Mclaugherty, C. A., Rayburn, L., Repert, D., et al. (1992). Wood decomposition over a first-order watershed: mass loss as a function of lignocellulase activity. Soil Biol. Biochem. 24, 743–749. doi: 10.1016/0038-0717(92)90248-v
Sinsabaugh, R. L., Klug, M. J., Collins, H. P., Yeager, P. E., and Petersen, S. O. (1999). “Characterizing soil microbial communities,” in Standard Soil Methods for Long-Term Ecological Research, eds G. P. Robertson, C. S. Bledsoe, D. C. Coleman, and P. Sollins (New York, NY: Oxford University Press), 318–348.
Sokol, N. W., Sanderman, J., and Bradford, M. A. (2019). Pathways of mineral-associated soil organic matter formation: integrating the role of plant carbon source, chemistry, and point of entry. Global Change Biol. 25, 12–24. doi: 10.1111/gcb.14482
Sollins, P., Homann, P., and Caldwell, B. A. (1996). Stabilization and destabilization of soil organic matter: mechanisms and controls. Geoderma 74, 65–105. doi: 10.1016/s0016-7061(96)00036-5
Sorte, C. J. B., Ibáñez, I., Blumenthal, D. M., Molinari, N. A., Miller, L. P., and Grosholz, E. D. (2013). Poised to prosper? A cross-system comparison of climate change effects on native and non-native species performance. Ecol. Lett. 16, 261–270. doi: 10.1111/ele.12017
Strickland, M. S., Devore, J. L., Maerz, J. C., and Bradford, M. A. (2010). Grass invasion of a hardwood forest is associated with declines in belowground carbon pools. Global Change Biol. 16, 1338–1350. doi: 10.1111/j.1365-2486.2009.02042.x
Suseela, V., Conant, R. T., Wallenstein, M. D., and Dukes, J. S. (2012). Effects of soil moisture on the temperature sensitivity of heterotrophic respiration vary seasonally in an old-field climate change experiment. Global Change Biol. 18, 336–348. doi: 10.1111/j.1365-2486.2011.02516.x
Suseela, V., Tharayil, N., Xing, B., and Dukes, J. S. (2013). Labile compounds in plant litter reduce the sensitivity of decomposition to warming and altered precipitation. N. Phytol. 200, 122–133. doi: 10.1111/nph.12376
Suseela, V., Tharayil, N., Xing, B. S., and Dukes, J. S. (2014). Warming alters potential enzyme activity but precipitation regulates chemical transformations in grass litter exposed to simulated climatic changes. Soil Biol. Biochem. 75, 102–112. doi: 10.1016/j.soilbio.2014.03.022
Suseela, V., Tharayil, N., Pendall, E., and Rao, A M. (2017). Warming and elevated CO2 alter the suberin chemistry in roots of photosynthetically divergent grass species. AoB Plants 9:plx041. doi: 10.1093/aobpla/plx041
Tamura, M., Suseela, V., Simpson, M., Powell, B., and Tharayil, N. (2017). Plant litter chemistry alters the content and composition of organic carbon associated with soil mineral and aggregate fractions in invaded ecosystems. Global Change Biol. 23, 4002–4018. doi: 10.1111/gcb.13752
Tamura, M., and Tharayil, N. (2014). Plant litter chemistry and microbial priming regulate the accrual, composition and stability of soil carbon in invaded ecosystems. N. Phytol. 203, 110–124. doi: 10.1111/nph.12795
Tharayil, N., Alpert, P., Bhowmik, P., and Gerard, P. (2013). Phenolic inputs by invasive species could impart seasonal variations in nitrogen pools in the introduced soils: a case study with Polygonum cuspidatum. Soil Biol. Biochem. 57, 858–867. doi: 10.1016/j.soilbio.2012.09.016
Thiessen, S., Gleixner, G., Wutzler, T., and Reichstein, M. (2013). Both priming and temperature sensitivity of soil organic matter decomposition depend on microbial biomass–An incubation study. Soil Biol. Biochem. 57, 739–748.
Treseder, K. K., Marusenko, Y., Romero-Olivares, A. L., and Maltz, M. R. (2016). Experimental warming alters potential function of the fungal community in boreal forest. Global Change Biol. 22, 3395–3404. doi: 10.1111/gcb.13238
von Lützow, M., Koegel-Knabner, I., Ekschmitt, K., Matzner, E., Guggenberger, G., Marschner, B., et al. (2006). Stabilization of organic matter in temperate soils: mechanisms and their relevance under different soil conditions - a review. Eur. J. Soil Sci. 57, 426–445. doi: 10.1111/j.1365-2389.2006.00809.x
Wright, S., and Upadhyaya, A. (1998). A survey of soils for aggregate stability and glomalin, a glycoprotein produced by hyphae of arbuscular mycorrhizal fungi. Plant Soil 198, 97–107.
Wu, Z., Dijkstra, P., Koch, G. W., Peñuelas, J., and Hungate, B. A. (2011). Responses of terrestrial ecosystems to temperature and precipitation change: a meta-analysis of experimental manipulation. Glob Change Biol. 17, 927–942. doi: 10.1111/j.1365-2486.2010.02302.x
Yuste, J. C., Janssens, I. A., Carrara, A., Meiresonne, L., and Ceulemans, R. (2003). Interactive effects of temperature and precipitation on soil respiration in a temperate maritíme pine forest. Tree Physiol. 23, 1263–1270. doi: 10.1093/treephys/23.18.1263
Zhang, W. D., Wang, X. F., and Wang, S. L. (2013). Addition of external organic carbon and native soil organic carbon decomposition: a meta-analysis. PLoS One 8:e54779. doi: 10.1371/journal.pone.0054779
Keywords: soil organic matter, suberin, cutin, warming, labile substrates, enzyme activity, invasion, temperature sensitivity
Citation: Tamura M and Suseela V (2021) Warming and Labile Substrate Addition Alter Enzyme Activities and Composition of Soil Organic Carbon. Front. For. Glob. Change 4:691302. doi: 10.3389/ffgc.2021.691302
Received: 06 April 2021; Accepted: 08 July 2021;
Published: 02 December 2021.
Edited by:
Andreas Schindlbacher, Austrian Research Centre for Forests (BFW), AustriaCopyright © 2021 Tamura and Suseela. This is an open-access article distributed under the terms of the Creative Commons Attribution License (CC BY). The use, distribution or reproduction in other forums is permitted, provided the original author(s) and the copyright owner(s) are credited and that the original publication in this journal is cited, in accordance with accepted academic practice. No use, distribution or reproduction is permitted which does not comply with these terms.
*Correspondence: Vidya Suseela, dnN1c2VlbEBjbGVtc29uLmVkdQ==
Disclaimer: All claims expressed in this article are solely those of the authors and do not necessarily represent those of their affiliated organizations, or those of the publisher, the editors and the reviewers. Any product that may be evaluated in this article or claim that may be made by its manufacturer is not guaranteed or endorsed by the publisher.
Research integrity at Frontiers
Learn more about the work of our research integrity team to safeguard the quality of each article we publish.