- 1Isotope Ecology Laboratory, Center for Nuclear Energy in Agriculture, University of São Paulo, Piracicaba, Brazil
- 2Environmental Study and Research Centre, State University of Campinas, Campinas, Brazil
- 3Forest Management Laboratory, National Institute of Amazonian Research, Manaus, Brazil
- 4Ecology Department, Institute of Biological Sciences, University of Brasília, Brasília, Brazil
The Neotropics harbor some of the most diversified woody species in the world, and to understand the nutrient dynamics in these ecosystems, it is crucial to understand the role of plant taxonomy. In addition, biological nitrogen (N) fixation (BNF) in the tropics is one of the key processes affecting the global N cycle. Our objective was to (i) investigate the role of taxonomy and sampling site as predictors of foliar carbon (C) and N concentration and its stable isotopes (i.e., δ13C and δ15N); (ii) assess differences in foliar N, C:N ratio, and δ15N among three functional groups: species of N2-fixers and non-fixers of the Fabaceae family, as well as non-Fabaceae species; and (iii) examine the effect of wood density on tree foliar properties. We hypothesized that Fabaceae specimens in symbiosis with N2-fixers would possess a higher foliar N than non-fixing plants, including those of the Fabaceae family, as well as high-density trees would have higher foliar C and C:N ratio relative to low-density trees, where the latter invest in nutrients instead of structural C. We used a data set composed of 3,668 specimens sampled in three main biomes of Brazil: Amazon, Atlantic Forest, and Cerrado. The partitioning of variance had a higher influence of taxonomy on leaf C, N, and C:N ratio. Conversely, foliar δ13C and δ15N were environmentally constrained. While family was the most important taxonomy level for C, N, and C:N ratio, species played a major role for δ13C and δ15N. Foliar N followed the pattern fixers > non-fixers > non-Fabaceae, while C:N ratio had an opposite trend. In addition, foliar C was correlated with wood density, where high-density > medium-density and low-density woods. The large variability of δ15N was observed among Fabaceae species, demonstrates the complexity of using δ15N as an indicator of BNF. The higher foliar N of Fabaceae non-fixers than non-Fabaceae specimens support the hypothesis that an N-demanding lifestyle is an inherent pattern in this family. Lastly, although observed in some studies, the prediction of foliar properties using wood density is challenging, and future research on this topic is needed.
Introduction
The Neotropics harbor one of the most diversified plant and animal species in the world (Hughes et al., 2013; Ulloa et al., 2017; Meseguer et al., 2020). Almost 40% of the global seed plant populations, approximately 100,000 species, are found in this region (Antonelli and Sanmartín, 2011). Although the reasons for such richness of life are still debatable, it seems that at regional to continental level, past geological events affecting the elevation of the Earth surface (topography) and climate changes (or lack of them), together with biotic processes, like phylogenetic niche conservatism, shape the distribution of species in the Neotropics (Rull, 2011; Cheng et al., 2013; Hughes et al., 2013; Ledo and Colli, 2017; Rangel et al., 2018).
Plant species diversity is also highly controlled by soil nutrients, from the local to the regional level (Hulshof and Spasojevic, 2020). In recent years, it has been elucidated that biodiversity has a pivotal role in ecosystem functioning (Cardinale et al., 2012; Tilman et al., 2014), which is strongly regulated by how plants process nutrients, mainly through biomass accumulation and litterfall (Vitousek and Sanford, 1986). Therefore, plant biodiversity can increase nutrient cycling and its acquisition due to the complement effect among species with varied functional traits, where different spatial and temporal niches are accessed, decreasing nutrient loss from the system (Hooper et al., 2005; Oelmann et al., 2011). The rate at which nutrients will be available to plants also depends on how nitrogen (N)-rich the vegetal detritus is, since the more N-rich it is, the faster it decomposes, returning this limiting nutrient quicker to the soil for subsequent plant uptake (Melillo et al., 1982). That is the case of Fabaceae family, which usually has higher foliar N concentration than other families, being a particularly important botanical family in forests of the tropics (Vitousek et al., 2002). Some species of this family are also able to establish symbiosis relations with the bacteria of the genus Rhizobium, enabling atmospheric N2 fixation, known as biological N fixation (BNF), one of the key processes in the global N cycle and of high importance in the tropics (Hedin et al., 2009; Barron et al., 2011; Cleveland et al., 2011). There are many other plant families that support symbiotic N fixation. However, Fabaceae are considered the most important species regarding N acquisition due to their symbiotic relationship with rhizobia (Valentine et al., 2018). Potentially, the foliar N isotope ratio (i.e., δ15N) could be used to estimate BNF in plants, although there are several pitfalls in such use, especially in woody perennial plants (Boddey et al., 2000).
The uniqueness of the Fabaceae family highlights the importance of understanding the taxonomy of plant species and their role on nutrient flow and ecosystem functioning. Taxonomy-driven variances have been reported as a strong source of the variation of photosynthetic and structural traits (Oliveras et al., 2020). Additionally, taxonomy seems to be more limiting to leaf traits than the environment, showing the dominance of taxonomic turnover over the environmental variations (Fyllas et al., 2009; Anderegg et al., 2018; Oliveras et al., 2020). Many plant traits and associations between traits have been hypothesized to be under the control of a common genetic mechanism, reflecting a combination of evolutionary and community assembly process responding to biotic and abiotic constraints (Chapin et al., 1993; Vasseur et al., 2012; Oliveras et al., 2020).
In tropical regions, investigation of foliar carbon (C) and N concentrations as well as isotopic ratios seems to be appealing because there is limited evidence suggesting that N site availability is an important driver of foliar N isotopic ratio (Högberg, 1997; Martinelli et al., 1999; Schuur and Matson, 2001; Craine et al., 2009). Current scientific understanding state that N-site availability controls foliar N isotope composition. In addition, there is an understanding that the canopy C isotope ratio (i.e., δ13C) is mainly controlled by water availability, in which stomata tend to stay open longer in sites with higher water abundance than in the arid ones (Diefendorf et al., 2010; Basu et al., 2019). Consequently, the internal stomatal CO2 concentration (pi) tends to equilibrate with the atmospheric CO2 (pa), leading to more negative δ13C values related to the plants in arid sites (Farquhar et al., 1989). Additionally, it is already established that slow-growing trees are shade-tolerant species, where water is more available, but the light is more limiting, leading to leaves with more negative δ13C than fast-growing and light-demanding species (Farquhar and Sharkey, 1982).
In order to understand the life history of trees and their mechanical and physiological strategies, wood density can be an important trait to be considered, since it relates to the whole-plant performance (Westoby et al., 2002; Swenson and Enquist, 2007). Low-density species have higher N and lower C concentrations in comparison with high-density species, following the well-known “leaf economics spectrum” (LES), where low-density trees, being short-lived, invest more on nutrients and less on structural C, while high-density trees, being long-lived, invest less on nutrients and more on structural C (Wright et al., 2004). Therefore, wood density also appears to be a relevant tree trait to unravel the ecosystem functioning, since this trait is key to determine forest biomass and C (Baker et al., 2004).
As part of the Neotropics, Brazil is the country with the largest number of vascular plant species (Ulloa et al., 2017), comprising six major biomes that can be broadly divided into two major rainforests: Amazon and the Atlantic Forest. These two rainforests are separated along a northeast–southwest axis by the Caatinga and Cerrado, representing two major dry areas (Rangel et al., 2018). Besides the above biomes, there is the Pantanal, one of the main wetland areas of the Neotropics, and the Pampas, in the south tip of Brazil, representing the only truly sub-temperate biome of the country. According to a recent cross-taxonomic biogeographic analysis, Amazon stands out as the primary source of diversity in the Neotropics (Antonelli et al., 2018). However, as recognized by several authors, drier regions such as the Caatinga and Cerrado also play a vital role in the biodiversity of the country (Pennington et al., 2009; Antonelli et al., 2018; Rangel et al., 2018).
In a recent study of Martinelli et al. (2020), the foliar δ13C and δ15N were determined in ∼6,500 tree leaves sampled from 57 sites distributed across all major Brazilian biomes. This analysis showed that there is a large variation in foliar chemical properties among vegetation types, demonstrating that parallel to life diversity, there is also a “biogeochemical diversity,” as postulated by Townsend et al. (2008). It was also speculated that precipitation was the main environmental factor responsible for such variability. However, the weight of taxonomy in these foliar properties was not determined and remains poorly understood. Therefore, our main objective was to determine how much of the variance in canopy foliar traits is explained by taxonomy and site characteristics, considering foliar N and C isotopic ratios, but also on foliar N and C concentrations in a subset of the database provided by Martinelli et al. (2020), containing 3,668 foliar samples with complete taxonomy (family, genus, and species) from three major Brazilian biomes, i.e., Amazon, Atlantic Forest, and Cerrado, including 741 species, distributed in 298 genera and 81 botanical families. Our special interest in stable isotopes lies in the fact that there is limited information regarding taxonomic control on canopy δ13C (Fyllas et al., 2009), and to the best of our knowledge, this aspect has not yet been evaluated for canopy δ15N. If our study proves that taxonomy exerts a major role in determining foliar δ15N, the importance of N-site availability as a key factor will be challenged. The second objective of this study was to investigate foliar N concentration, C:N ratio, and δ15N in specimens of the Fabaceae family and also compare these parameters among Fabaceae N-fixing, non-fixing, and non-Fabaceae species of the Brazilian biomes mentioned above. Finally, we also assessed whether wood density correlates or not with foliar C and N concentration, as well as the C:N ratio.
Materials and Methods
Data Acquisition and Processing
We used a data set compiled by Martinelli et al. (2020) and available in a public repository at Mendeley1, where C and N concentration, C:N ratio, and isotopic ratios are available for each species. Due to the large number of publications present in this data set (including original data provided by the authors), the sampling procedure of leaves slightly differed among studies. First, while several studies used the abundance criteria, sampling the most common species, others prioritized plant species based on logistic facilities (Martinelli et al., 2020). Second, most of the studies followed the protocol of Cornelissen et al. (2003), where mature, fully expanded, and sunlit leaves found in the middle of the canopy were sampled. However, in some studies, sampling of sunlit leaves was constrained by tree height in tropical forests. In such cases, understory leaves were not included in our subset to reduce bias regarding 13C composition (Ometto et al., 2006).
Overall, sampled leaves were oven-dried at 60–65°C to a constant weight and finely ground in a Wiley mill, and a subsample was transferred to a tin capsule. Samples were analyzed for total C and N concentration as well as their isotopic composition (13C and 15N) in an elemental analyzer interfaced to an isotope ratio mass spectrometer. C and N concentrations are expressed in percent, while the stable isotopic ratio in the classical “δ” notation is expressed according to the following equation (Craig, 1953):
δnX = (Rsample/Rstandard – 1) × 1,000 (1)
where X is C or N; n is the mass number of the heavier isotope (13C or 15N); and Rsample and Rstandard are the isotopic ratio of the samples and the standard, respectively. The primary standards were Pee Dee Belemnite for C and atmospheric air for N.
Of a total of ∼6,500 foliar samples, 3,668 had botanical identification. We used a similar procedure described by Chave et al. (2006) and by Oliveira et al. (2019). We first compared species names with the currently accepted names using the R package “flora” (Carvalho, 2020), with the purpose of correcting spelling errors and checking species names that have changed. We found a high number of spelling errors, which seems to be a recurrent problem in large data sets (Chave et al., 2006), and a smaller number of invalid species names that were discarded. This process resulted in a subset composed of tree foliar samples with accurate botanical classification at the species level from three major Brazilian biomes (Figure 1 and Supplementary Data Sheet 1). Vegetation types found in the data set were those previously described in Martinelli et al. (2020) for Amazon, Atlantic Forest, and Cerrado, namely, Dense Ombrophilous Forest (DOF), Open Ombrophilous Forest (OOF), Mixed Ombrophilous Forest (MOF), Campinarana Forest (CAM), Restinga Forest (MV), and Savanna (SAV). DOF had sampling sites located in the Amazon as well as in the Atlantic biome.
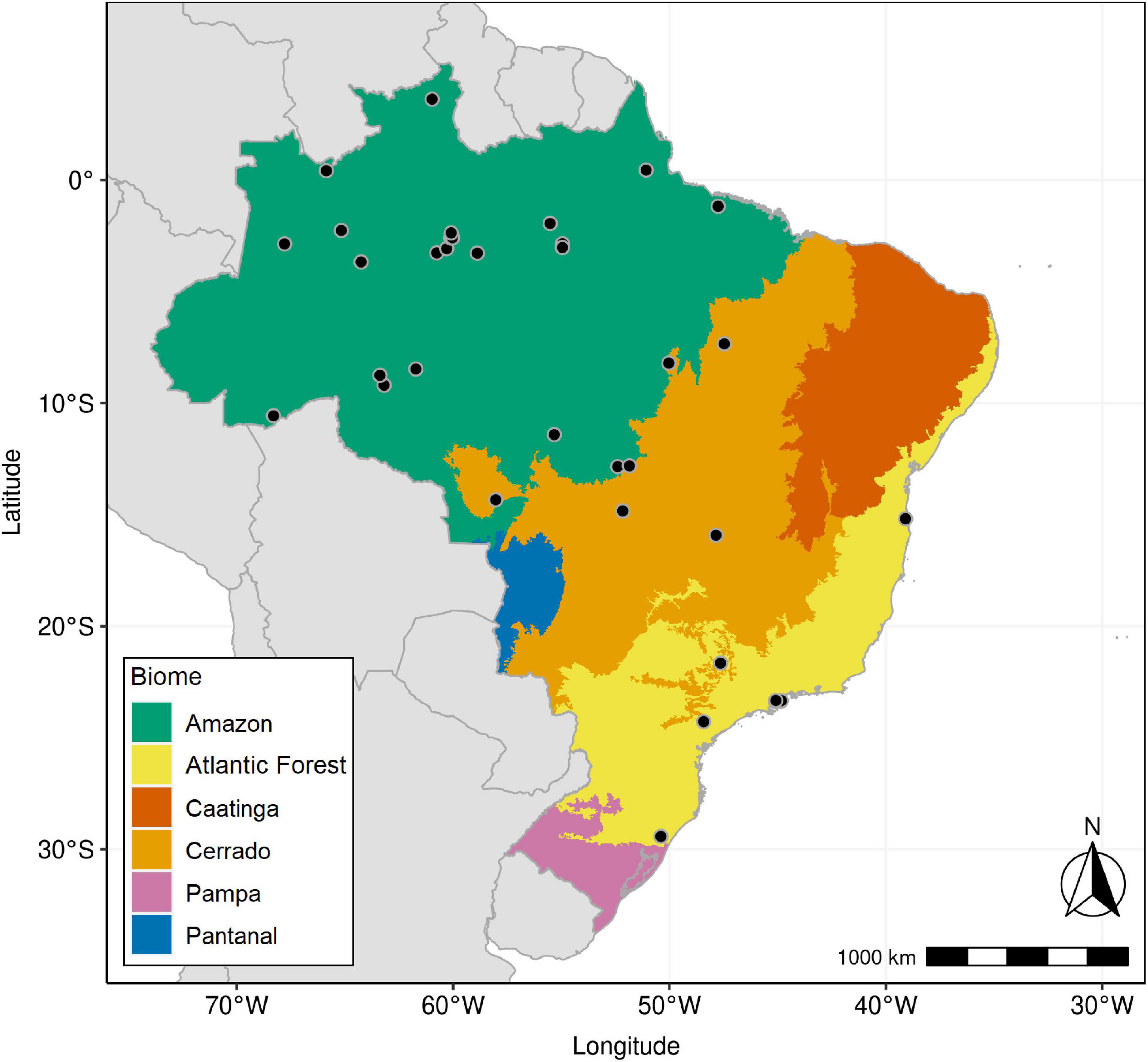
Figure 1. Map of Brazil with borders of its six major continental biomes: Amazon, Atlantic Forest, Caatinga, Cerrado, Pampa, and Pantanal. Black circles indicate sampling plots included in this study.
Variance Partitioning of Foliar Properties Into Taxonomy and Site
To partition the variance between taxonomy and site according to each foliar property, we used a similar statistical procedure employed by Fyllas et al. (2009) and Asner and Martin (2016), among others. A multilevel generalized linear mixed model (GLMM) was used, and model parameters were estimated by a residual maximum likelihood method (REML). To perform this analysis, we first checked normality of residuals using the R package “fitdistrplus” (Delignette-Muller and Dutang, 2015). Only foliar N concentration was log-transformed to ensure normality. The “lmerTest” R package was used to run the GLMM (Kuznetsova et al., 2017). To estimate the pseudo-R2 for GLMM (R2GLMM), the R package “MuMIn” was used (Bartón, 2020). This package estimated the variance explained by the fixed effect (marginal R2GLMM), the variance explained by the whole model, and the sum of fixed and random variables (conditional R2GLMM; Nakagawa et al., 2017).
The response variables (foliar properties) were modeled assuming the following random variables within the GLMM: (i) species, nested within genus and family; and (ii) site, to account for environmental filters. Adopting the same nomenclature as that found in previous studies (Fyllas et al., 2009; Asner and Martin, 2016; Oliveras et al., 2020), the total variance of the model for each response variable was then partitioned as follows:
where μ is the overall mean value of each foliar trait (T), presenting C and N elemental concentration and stable isotopic ratios; ss is the name of each sampling site; f/g/s represents the nested taxonomic structure of the data, species (s), nested in a genus (g) and nested in a family (f); and ε is the residual term that includes any variability not captured by the model.
Influence of the Fabaceae Family on Foliar Properties
To test the differences in foliar properties of species of Fabaceae, we first compared this family with each of the other families through multiple box-plots without conducting any statistical test since the main purpose here was to check, in descending order, how these traits varied among families. Second, we grouped Fabaceae according to their capability or not of establishing associations with N2-fixing bacteria (“fixers” and “non-fixers,” respectively), following Tedersoo et al. (2018). We compared these two groups with the third group consisting of species belonging to other botanical families (“non-Fabaceae”) with regard to foliar N, C:N, and δ15N. With the exception of foliar N, which was log-transformed before analysis, all variables were normally distributed. Then, we developed a GLMM considering these three groups as fixed effect and sampling site as a random effect. In case of significance (p ≤ 0.05), means were separated by Sidak’s post hoc test through the “emmeans” R package (Lenth et al., 2021). Given the often high foliar N concentration of legumes, a GLMM was used to assess the variance partitioning for foliar N of Fabaceae fixers and non-fixers into each sampling site, taxonomy (species nested in a genus), and residual, based on the statistical procedure described above (Equation 2).
Influence of Wood Density on Leaf Foliar Properties
Finally, we tested the effects of wood density on foliar properties by assigning a wood density (ρ) value to each species found in the data set published by Chave et al. (2006). Unfortunately, not all species included in our data set were found in the database provided by Chave et al. (2006). Accordingly, we ended up with 262 species belonging to 235 genera with values of ρ, with ∼35% of the total number of species included in our data set (Supplementary Data Sheet 2). As Chave et al. (2006) pointed out, most of the variance of ρ was explained at the genus level; accordingly, we grouped plants by their respective genera. We also grouped ρ in three classes considering the 33rd and 66th percentiles in the frequency distribution of ρ from Chave’s data set, as follows: low density (ρ < 0.54 g cm–3); medium density (0.54 < ρ < 0.71 g cm–3), and high density (ρ > 0.71 g cm–3). These values are similar to the ones used for classifying mechanical wood properties. However, we acknowledge that these classes of wood density were solely based on the frequency distribution of ρ found in Chave et al. (2006). We tested the effects of ρ on foliar composition through a simple linear model using the normal distribution, since residuals were normally distributed for all variables (foliar C, N, and C:N ratio). A similar procedure used to assess the influence of the Fabaceae family in foliar properties compared with other botanical families was adopted here as related to significances and post hoc tests. The R code used in this study can be found in Supplementary Data Sheet 3.
Results
Site had the lowest effect (∼21%) on the variance partitioning of foliar C, while ∼40% of the variance was explained by the sum of family, genus, and species, and ∼39% of the variance was attributed to the residual, not accounted by the above factors (Figure 2 and Table 1). With regard to foliar N, taxonomy explained ∼44% of the variance, while sampling site and residual represented 28% each (Figure 2 and Table 1). Approximately 26% of the variance of foliar C:N ratio was explained by site, but taxonomy still represented ∼44% of the variance, while the remaining percentage (∼30%) was not explained by the model (Figure 2 and Table 1). The effect of sampling site was the highest (∼40%) in explaining the variance of foliar δ13C, followed by residual (∼35%) and taxonomy (∼25%), with the lowest importance (Figure 2 and Table 1). Similar to foliar δ13C, most of the variance of δ15N was explained by site (∼63%), while taxonomy and residual accounted for ∼16 and ∼22%, respectively (Figure 2 and Table 1). Overall, the partitioning of leaf trait variance within the taxonomy levels indicated that botanical family explained mostly the total variance for C, N, and C:N ratio (∼23%, on average), while species was the most important group for δ13C and δ15N (∼13%, on average; Figure 2 and Table 1). The pseudo-R2 for GLMM of foliar properties ranged from 0.61 to 0.78 (Table 1).
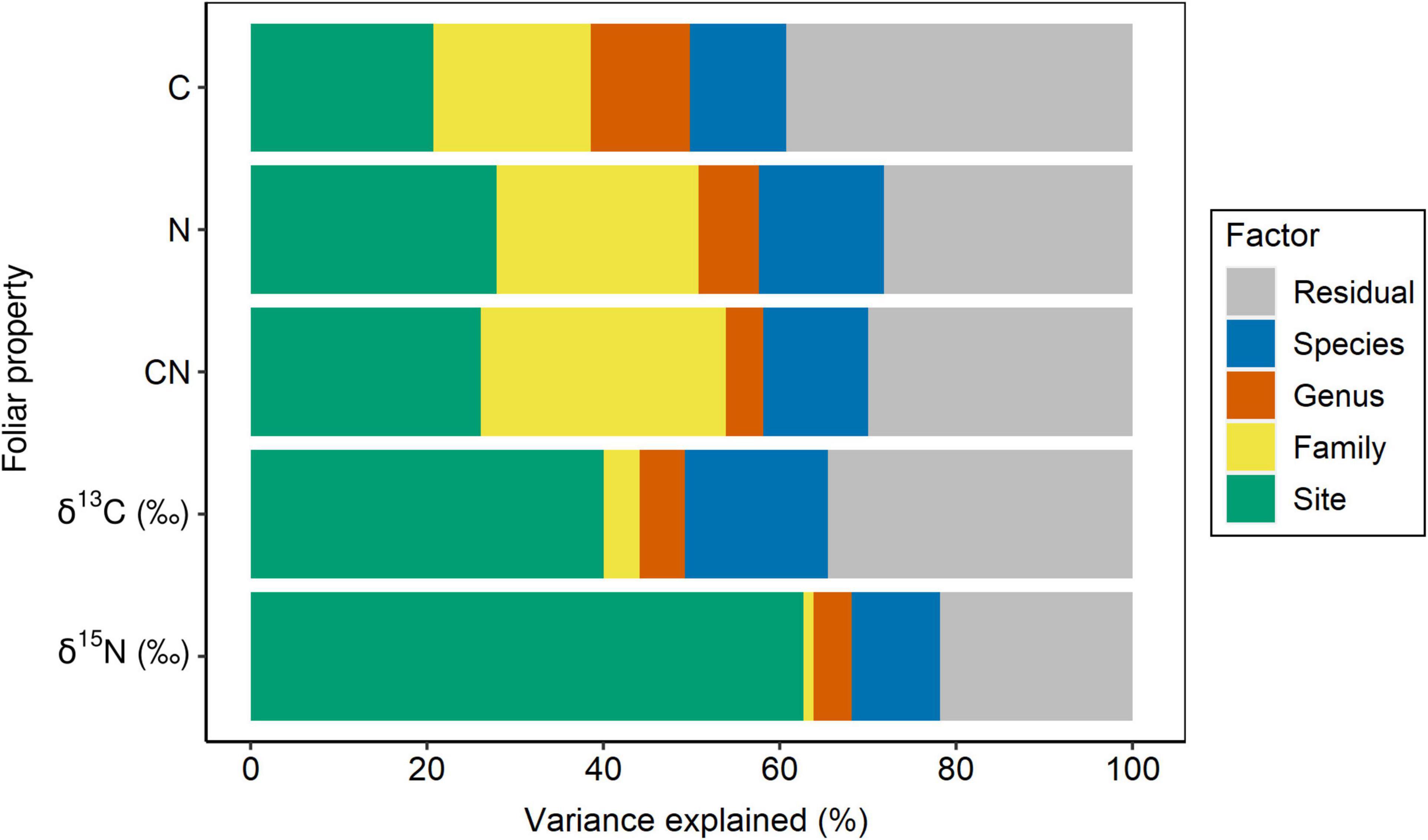
Figure 2. Partitioning of variance for foliar C and N concentration, C:N ratio, δ13C, δ15N into taxonomy (nested design: family/genus/species), site, and residual. Foliar properties are sorted from the less to more site constrained.
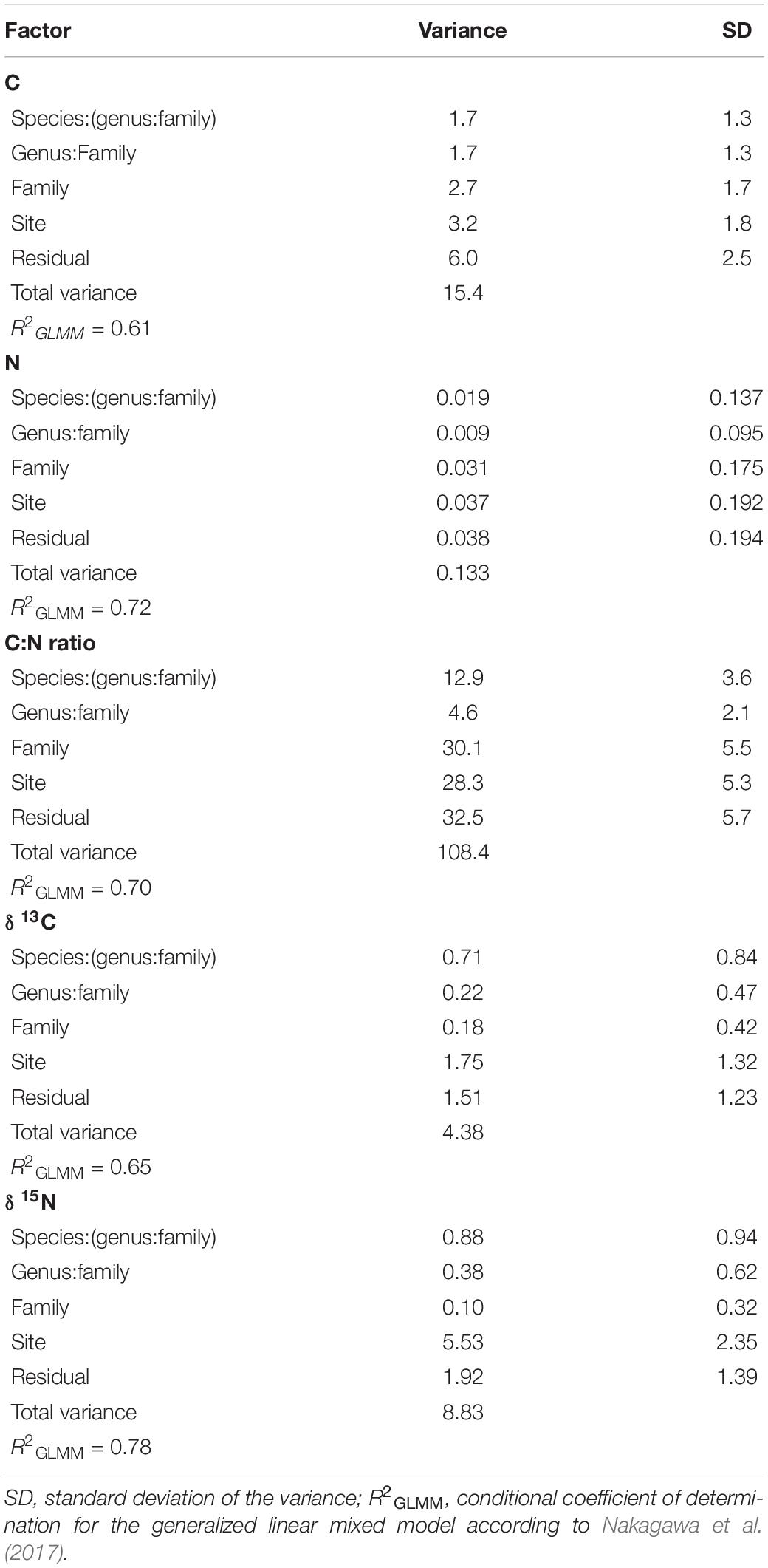
Table 1. Variance partitioning according to taxonomy (nested design: family/genus/species), site, and residual for foliar C, N, C:N ratio, δ13C, and δ15N.
Although Fabaceae ranked among the N-rich families, it was not the family with the highest foliar N concentration or even the lowest C:N ratio (Figures 3, 4). However, the family-median values for Fabaceae were higher and lower than the overall median for foliar N and C:N ratio, respectively. Finally, the family-median δ15N of Fabaceae was essentially similar to the overall median (Figure 5). By grouping Fabaceae family according to their N-fixing capacity at genera level, a clearer picture emerges. The foliar N concentration of fixers was significantly higher than the other two groups (Figure 6 and Supplementary Table 1). The foliar C:N ratio, as expected, followed an inverse trend, with fixers showing the lowest median values, followed by non-fixers and non-Fabaceae (Figure 6 and Supplementary Table 1). On the other hand, foliar δ15N was higher for non-fixers compared with non-Fabaceae and fixers (Figure 6 and Supplementary Table 1). The variance partitioning across the two groups of Fabaceae indicated that ∼50, ∼14, and ∼36% of the variance of foliar N was explained by taxonomy, site, and residual, respectively, for fixers, while for non-fixers, taxonomy and site represented ∼35 and ∼27% of the variance, respectively, with the remaining percentage (∼38%) attributed to residual (Figure 7 and Supplementary Table 2). For both groups, genus played a major role relative to species across the taxonomic levels. In addition, foliar composition varied among different classes of wood density (Figure 8 and Supplementary Table 3). However, differences were only significant for foliar C concentration. Thus, C concentration was the highest in the high-density class, exhibiting the following trend: high density > medium density ≈ low density (Figure 8 and Supplementary Table 3).
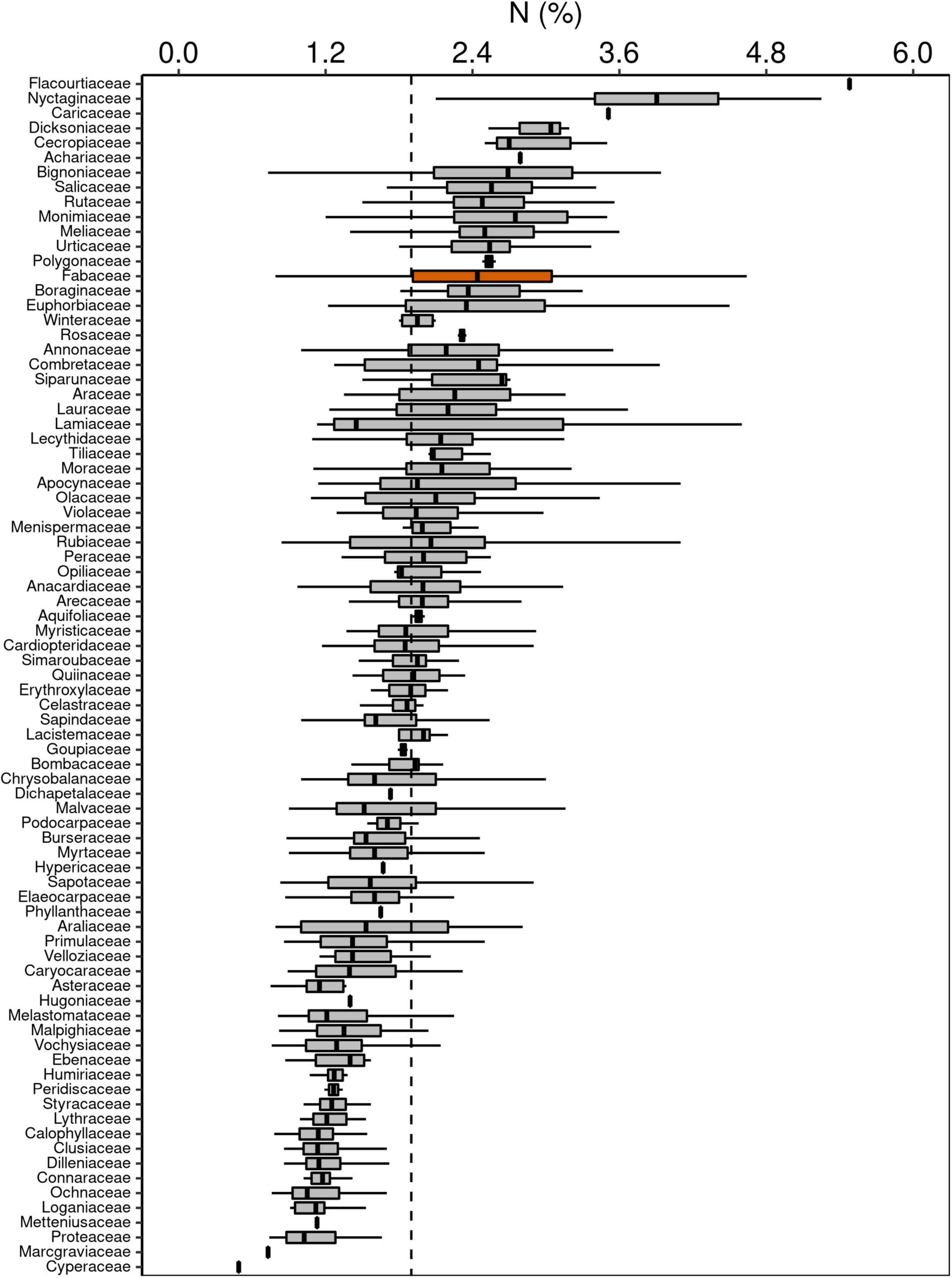
Figure 3. Box-plots of foliar N concentration of botanical families in decreasing order. The vertical lines inside each box represent the median; the box represents the first and third quartiles; and the bars represent the interquartile range. The vertical dashed line represents the mean of the entire population. The orange box is evidencing the Fabaceae family, where a notable part of the species is capable of fixing atmospheric N through symbiotic associations.
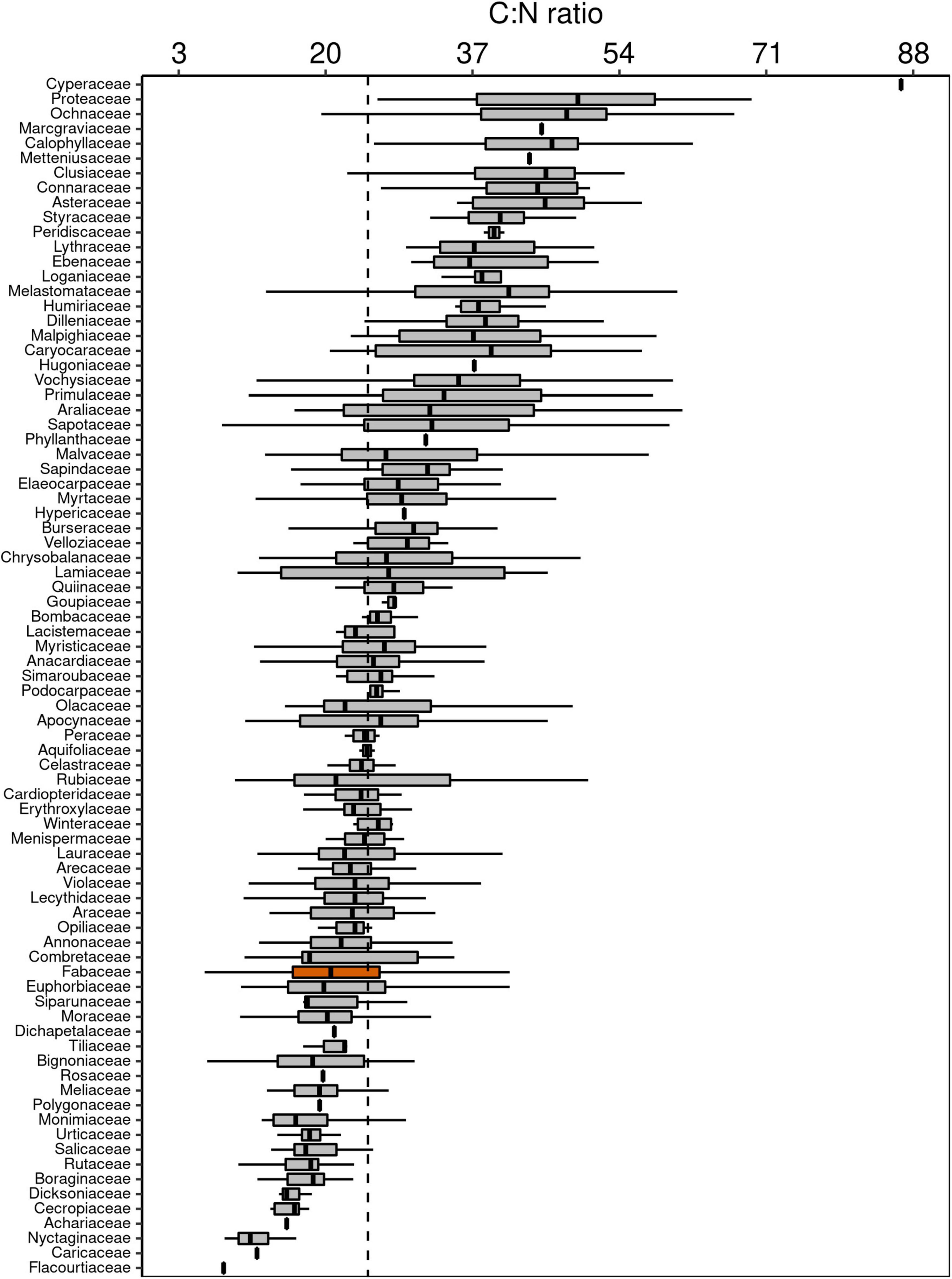
Figure 4. Box-plots of foliar C:N ratio of botanical families in decreasing order. The vertical lines inside each box represent the median; the box represents the first and third quartiles; and the bars represent the interquartile range. The vertical dashed line represents the mean of the entire population. The orange box is evidencing the Fabaceae family, where a notable part of the species is capable of fixing atmospheric N through symbiotic associations.
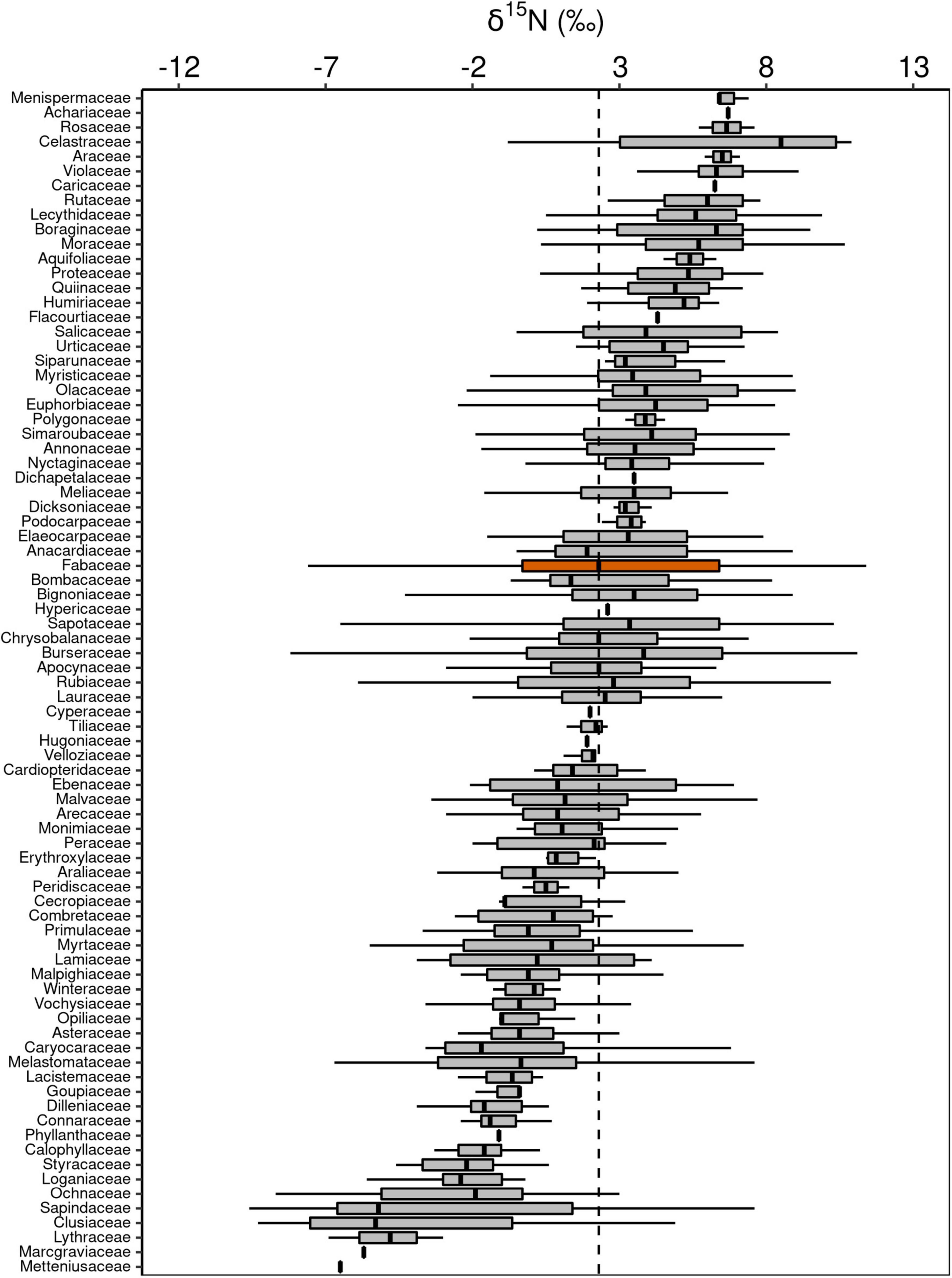
Figure 5. Box-plots of foliar δ15N of botanical families in decreasing order. The vertical lines inside each box represent the median; the box represents the first and third quartiles; and the bars represent the interquartile range. The vertical dashed line represents the mean of the entire population. The orange box is evidencing the Fabaceae family, where a notable part of the species is capable of fixing atmospheric N through symbiotic associations.
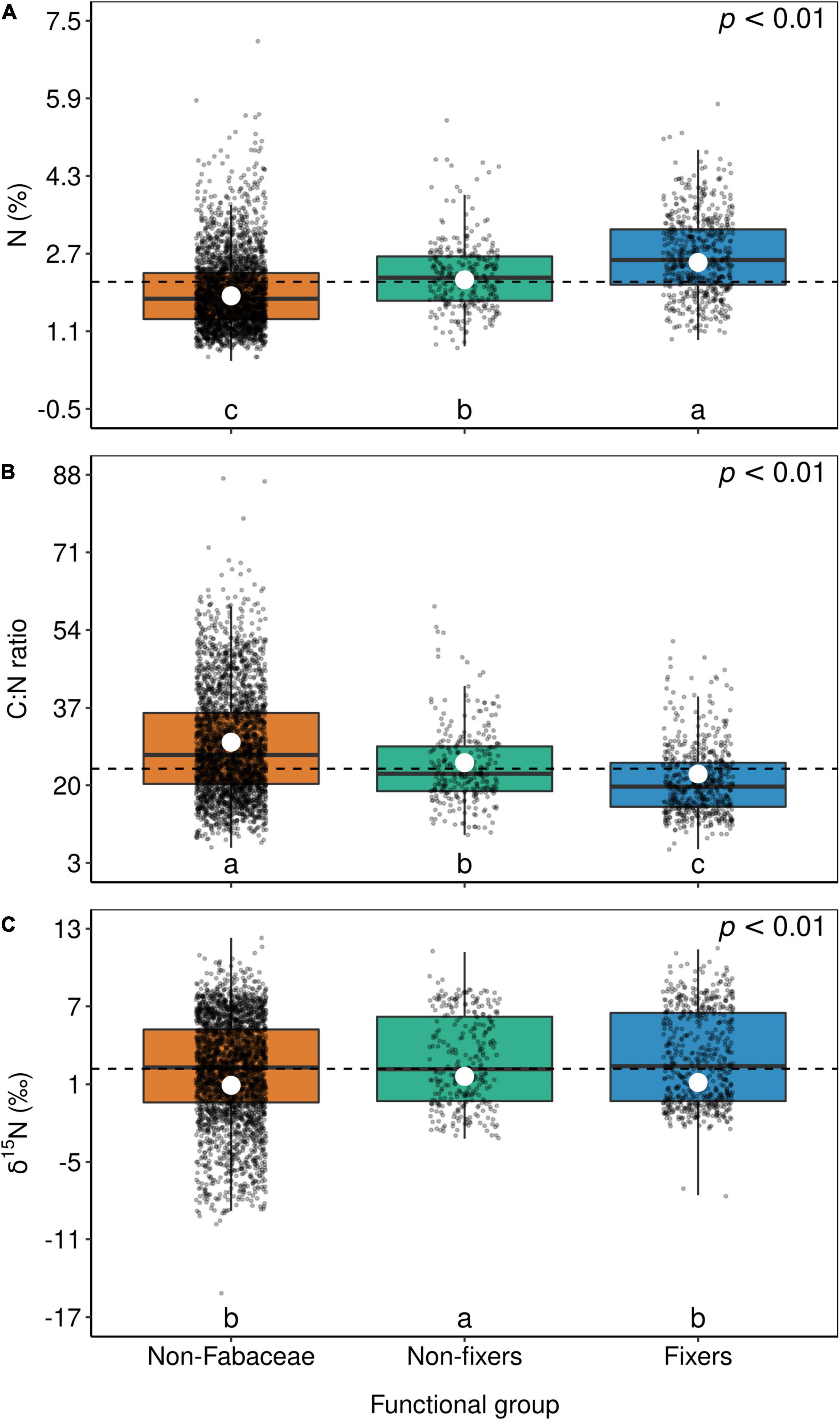
Figure 6. Box-plots of foliar N (A), C:N ratio (B), and δ15N (C) of non-Fabaceae species as well as non-fixer and N2-fixer species of the Fabaceae family. The horizontal lines inside each box represent the median; the box represents the first and third quartiles; and the bars represent the interquartile range. The horizontal dashed line represents the mean of the entire population. White circles represent estimate means obtained from the generalized linear mixed model. The summary of the statistical model can be seen in Supplementary Table 1. Levels followed by a common letter are not different according to the Sidak-test at p ≤ 0.05.
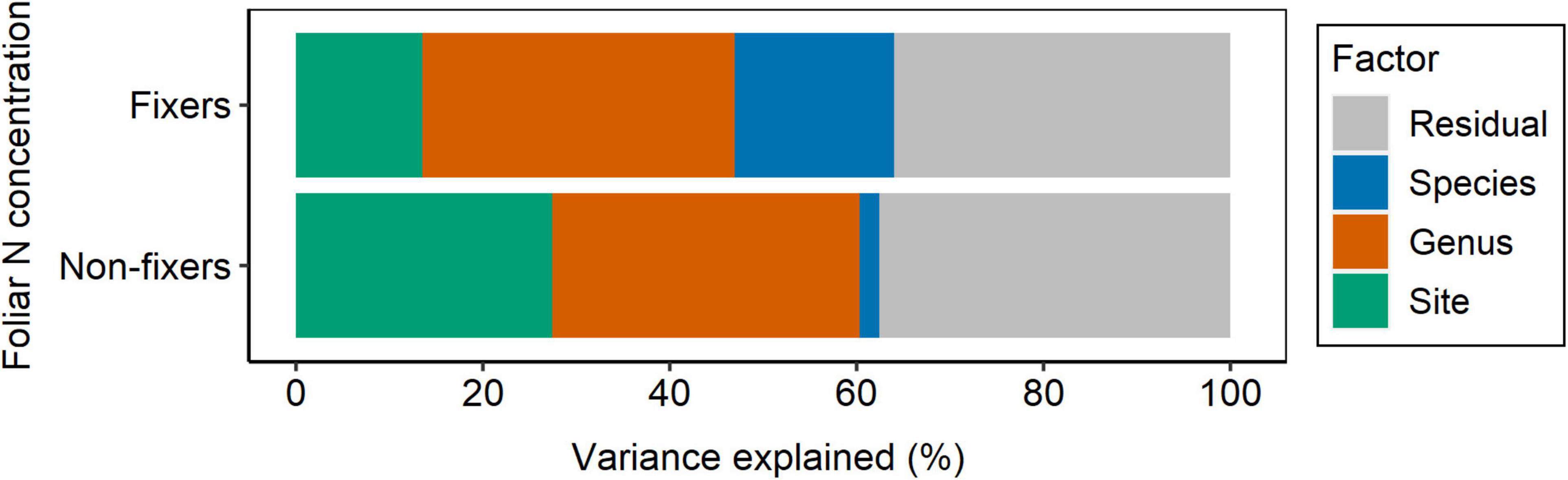
Figure 7. Partitioning of variance for foliar N concentration of N2-fixing and non-fixing Fabaceae into taxonomy (nested design: genus/species), site, and residual.
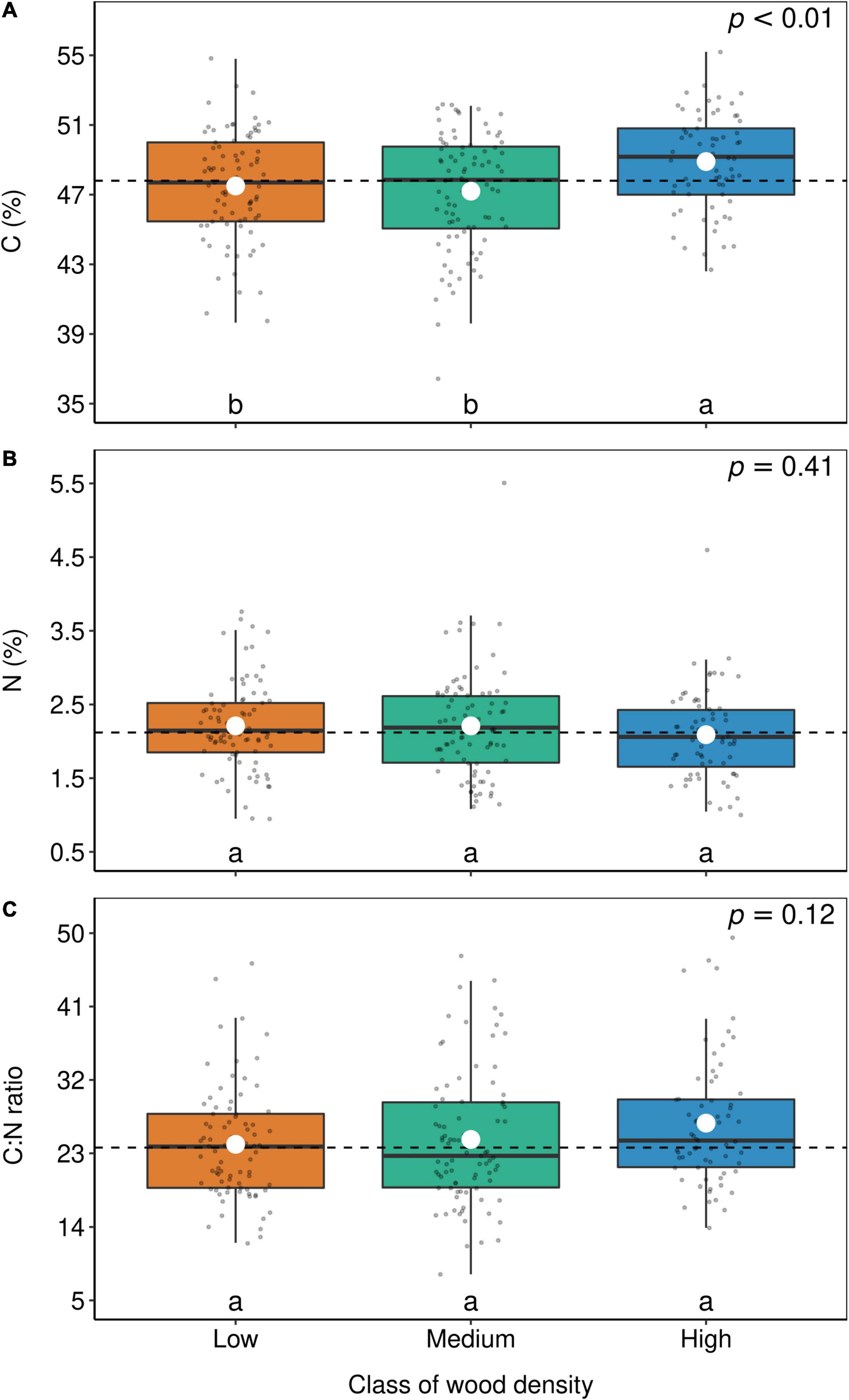
Figure 8. Box-plots of foliar C (A), N (B), and C:N ratio (C) according to wood density groups. Each dot represents the mean of a botanical genus. The horizontal lines inside each box represent the median; the box represents the first and third quartiles; and the bars represent the interquartile range. The horizontal dashed line represents the mean of the entire population. White circles represent estimate means obtained from the linear model. The summary of the statistical model can be seen in Supplementary Table 3. Levels followed by a common letter are not different according to the Sidak-test at p ≤ 0.05.
Discussion
Taxonomy and Site Affecting the Canopy Chemistry
We observed that foliar δ15N was strongly environmentally constrained, therefore presenting a high level of plasticity, with taxonomy playing a minor role in the respective variation (Figure 2). This finding indicates that local abiotic conditions, represented here by the site factor within the variance partitioning model, are tightly associated with δ15N of leaf trees in comparison with the genetic (i.e., taxonomic levels) component. Despite being unable to identify the specific abiotic factors contributing to this strong relationship, soil N availability at the local scale and precipitation at a larger scale have been proven to be the key in controlling the δ15N of plants (Craine et al., 2009, 2015). Many studies postulate that foliar δ15N tends to be higher in sites where the N availability (i.e., mineral N forms) is also high (Högberg, 1997; Martinelli et al., 1999; Craine et al., 2009), in addition to the lower soil available N in wet than dryer areas (Amundson et al., 2003; Pardo et al., 2006). Moreover, a recent study developed an isoscape of soil δ15N for South America, suggesting a high variation of values across the Brazilian territory (Sena-Souza et al., 2020), which also helps to explain the dominance of the environmental filter on predicting foliar δ15N.
It is important to notice that still ∼16% of the variance of foliar δ15N was explained by taxonomy (Figure 2). Plant species can be important in driving N isotopic ratios in several ways (Högberg, 1997), such as “preferential” uptake of a particular mineral; atmospheric or organic N form (Chalk and Smith, 2020); and metabolic-induced changes of N isotopic ratios (Robinson, 2001; Yoneyama et al., 2001). For instance, Roupala montana (Proteaceae), a common species found in the Cerrado, is more effective in acquiring NH4+ related to NO3–. This species tends to have higher foliar δ15N than other species of the same biome (Bustamante et al., 2004), as NH4+ tends to be more 15N-enriched than NO3– (Högberg, 1997; Houlton et al., 2007). In our data set, there are 44 specimens of R. montana, most of them from savannas of Central Brazil, and a minor part from savannas encroached on the Amazon region. The median δ15N of R. montana was 5.6‰ (25th percentile = 4.3‰; 75th percentile = 6.5‰), while the overall foliar δ15N median of plants found in savannas was −0.6‰ (25th percentile = −1.6‰; 75th percentile = 0.6‰; Supplementary Data Sheet 1).
Another example of taxonomy influencing foliar δ15N is in the fixers grouping. Taxonomy determines which species of Fabaceae family can establish symbiotic relations with bacteria of the genus Rhizobium, which fix atmospheric N in exchange for carbohydrates (Sprent, 1995). As the atmospheric N has a δ15N of 0‰, and the fractionation between the Rhizobium and the plant is almost negligible (Högberg, 1997), species that rely mainly on BNF potentially have a lower δ15N in comparison with plants relying on the soil as the unique N source (Virginia and Delwiche, 1982). Although Fabaceae is an important family in tropical biomes, often specimens do not rely on BNF as a source of N (Vitousek et al., 2002).
There was a large range of δ15N values in our data set. However, the median δ15N in sampling sites with DOF (including forests of the Amazon and Atlantic Forest biome) was equal to 4.6‰ (25th percentile = 1.8‰; 75th percentile = 7.1‰; Supplementary Data Sheet 1), which is well above the overall median for foliar δ15N, supporting the hypothesis of low and facultative BNF in tropical forests (Hedin et al., 2009; Barron et al., 2011; Nardoto et al., 2014). Most of the negative or near-zero δ15N values were observed in the savanna’s sites, with a median equal to = −0.6‰ (25th percentile = −1.2‰; 75th percentile = 0.1‰; Supplementary Data Sheet 1). Despite many specimens with negative foliar δ15N in sites with savannas vegetation, it is not possible to assess the role of BNF since near-zero δ15N values in the savannas can be derived from BNF as well as the association of legumes with mycorrhizal fungi in N-limited systems, where depleted δ15N compounds are delivered to the plant (Bustamante et al., 2004).
Due to the importance of water availability in driving foliar δ13C values by the photosynthetic discrimination against 13CO2 (Lloyd and Farquhar, 1994; Diefendorf et al., 2010; Cornwell et al., 2018), we hypothesized that climatic and soil conditions would be more important than taxonomy (Fyllas et al., 2009; Cornwell et al., 2018). This hypothesis was confirmed since sampling site explained a major share of the variance, with taxonomy representing a lower but representative percentage (Figure 2). Taxonomy also exerts influence on foliar δ13C by modulating environmental seasonal changes, such as water availability, mainly through leaf adjustments like stomatal conductance and photosynthetic rates (Read and Farquhar, 1991; Comstock and Ehleringer, 1992). As explained by Cernusak et al. (2013), water availability seems to be a key control of foliar δ13C; however, the degree of response to this variable depends on how each species will counteract the environmental forcing with leaf adjustments.
Strictly speaking about the taxonomic levels and their role on functional leaf traits, our study is in line with Fyllas et al. (2009); Asner and Martin (2016), and Oliveras et al. (2020), who found a higher proportion of the C and N variance explained by the botanical family compared with genus and species. For δ13C, while we observed that species was the more constrained factor, Fyllas et al. (2009) reported that genus was the dominant genetic level at a local scale for Amazonian sites. To the best of our knowledge, this is the first study reporting the partitioning of variance for foliar δ15N, therefore restricting any comparison with previously published studies, especially those under similar geographic conditions to ours.
The Role of Fabaceae in the Nitrogen Cycle
Taxonomy was the most important factor in explaining foliar N variance (∼50%) for N2-fixers, while the variance explained by taxonomy and residual was similar for Fabaceae non-fixers. This trend is in line with previous studies conducted in tropical regions (Fyllas et al., 2009; Asner and Martin, 2016). An iconic example of such control is the higher foliar N concentration found in the Fabaceae family, especially those able to establish an association with N-fixing organisms (Figures 3, 6), a trend already observed by many authors (McKey, 1994; Vitousek et al., 2002; Townsend et al., 2007; Fyllas et al., 2009; Nasto et al., 2014; Adams et al., 2016; Taylor and Ostrowsky, 2019). However, the interpretation of this result is still unknown.
McKey (1994) proposed that the high foliar N concentration in Fabaceae evolved before the association with N-fixing organisms, the so-called “N-demanding lifestyle” of Fabaceae. In this sense, Wolf et al. (2017) defined the term “hardwired” (i.e., having an inherent pattern) for this N-rich lifestyle and found that in fact Fabaceae are not hardwired, but instead, this family appears to have a high N plasticity, responding to site N availability and rhizobia colonization. Our results indeed show a large variability in foliar N concentration of Fabaceae specimens (Figure 3), suggesting high plasticity, as proposed by Wolf et al. (2017). The ability of specimens in this botanical family in assimilating nitrate efficiently via the nitrate reductase enzyme was also postulated (Smirnoff and Stewart, 1985). On the other hand, foliar N concentration of non-fixers was also higher than that of non-Fabaceae, favoring the hypothesis that the Fabaceae family is indeed hardwired to have an N-demanding lifestyle.
If a higher N concentration is hardwired in Fabaceae, most of the variance of foliar N in this family would be explained by taxonomy. Therefore, the variance partitioning for foliar N had a comparable weight for taxonomy and site control, regardless of the Fabaceae group (fixers and non-fixers), with no dominant contribution from either of these factors on the variance (Figure 7 and Supplementary Table 2). However, it seems that site characteristics are driving potential associations with root symbionts, which likely increase even more the foliar N contents of fixers in these species (Figure 6). Further research on this topic is needed to mechanistically partition the effects of root symbionts.
Another topic less discussed is the fact that the foliar C:N ratio in fixers was also lower than in non-fixers, which in turn was lower than in non-Fabaceae (Figure 6). This is an ecologically key functional trait of Fabaceae in their role to regulate C accumulation mainly in tropical systems (Davidson et al., 2007; Siddique et al., 2008; Russell and Raich, 2012; Batterman et al., 2013), besides the fact that the LES links N and C acquisition by photosynthesis (Wright et al., 2004). Lower C:N ratios are also important in accelerating litter decomposition, therefore enhancing N cycling (Melillo et al., 1982). As expected, foliar δ15N in fixers was lower than in non-fixers (Figure 6), which can be attributed to the relatively low isotopic fractionation of BNF (Högberg, 1997). In addition, a large number of non-Fabaceae families presented lower values of foliar δ15N relative to Fabaceae (overall median; Figure 5), which could explain the similar results between non-Fabaceae and Fabaceae fixers (Figure 6).
Could Wood Density Predict Foliar Properties?
Based on the LES, we expected that trees with low density would have a higher foliar N content than high-density trees (Wright et al., 2004; Chave et al., 2009). Therefore, in the successional continuum of tropical forests, fast-growing, low-density trees would invest more on nutrients and less on structural C, while slow-growing, high-density trees would invest less on nutrients and more on structural C (Poorter et al., 2004; Wright et al., 2007). However, only foliar C concentration fully followed the expected trend, where higher C was found in high-density trees (Figure 8). The fact that Wright et al. (2007) could not have found a correlation between wood density and specific leaf area, one of the pillars of the LES, and neither could we find a correlation between wood density and foliar N, reinforces the idea that a convergence between leaf and wood traits, if it exists, is more complex than we initially thought.
On the other hand, the increase of foliar C with wood density was expected since wood density is defined as the dry mass of wood per volume of fresh wood. The wood C concentration in tropical forests is larger in relation to other elements, but it is not very variable. For example, Elias and Potvin (2003) found a wood C variability of 45–50% among 32 species of tropical trees in Panama. Vieira et al. (2011) found the same range of variability in the coastal Atlantic Forest in southeast Brazil. As the variability in wood density is much higher than the wood C content, high-density trees tend to have higher C concentration than low-density trees simply because the latter has more mass packed per unit of volume than the former. This connection between wood C and density also explains why most of the variance of foliar C is explained by taxonomy (Figure 2), since most of the variance of wood density is highly connected with taxonomy (Chave et al., 2006, 2009).
Finally, we also expected that a correlation between wood density and foliar δ13C would arise, first because foliar δ13C is correlated with wood δ13C (Lins et al., 2016), and second because low-density, light-demanding trees have higher growth rates compared with high-density trees, shade-tolerant trees (Chave et al., 2009; Wright et al., 2010). Therefore, it is fair to suppose that shade-tolerant trees have lower water limitation related to light-demanding trees, which tend to be taller and more exposed to the sunlight (Bucci et al., 2004; Wright et al., 2010). Moreover, lower water limitation leads to more negative foliar δ13C values (Farquhar and Sharkey, 1982). Such light effect was clearly shown in a subtropical forest of China (Ehleringer et al., 1986) and forests along an Andes-to-Amazon elevation gradient (Martin et al., 2020). Although differences were not statistically significant, we observed an opposite trend, with foliar δ13C values of high-density trees, on average, less negative than low-density trees (data not shown). Therefore, our initial hypothesis was rejected. Perhaps because as concluded by Wright et al. (2003), there are few species at both ends of the light-demanding/shade-tolerant spectrum, but instead, most of the trees in a tropical forest stand have intermediate light requirements.
Conclusion
In this paper, we tested how taxonomy and local effects modulate the variance of canopy foliar traits, including the two rainforests (Amazon and Atlantic) and the most iconic savanna of Brazil (Cerrado). Therefore, we extended variance partitioning of canopy foliar traits beyond the wet vegetation types of the tropics included across our sampling sites. Overall, we found that the variance of δ13C and δ15N was explained by different proportions of random variables rather than the elemental composition of C and N. The weight of sampling site in explaining the variance of C and N stable isotopes was more accentuated compared with the elemental composition. On the other hand, taxonomy seems to be more important for foliar C, N, and C:N ratio. This is especially true for the Fabaceae family, which independently of fixing N2 or not had higher N foliar concentration in comparison with other families. Even among Fabaceae, those genera capable of establishing symbiotic associations with fixing bacteria had higher N foliar concentration than non-fixing genera. However, there are still several open questions. For instance, there were a dozen families that had higher N foliar concentrations compared with Fabaceae. Is there also a type of taxonomic control in these families? The answer to this type of question would be important to simulate how families with low trait plasticity would respond to increases in temperature and changes in precipitation patterns in comparison with families with high trait plasticity. These findings would contribute to model future tropical vegetation and its biodiversity under a scenario of global changes, and the feedbacks of those changes on the climate and nutrient cycling.
Data Availability Statement
The original contributions presented in the study are included in the article/Supplementary Material, further inquiries can be directed to the corresponding author/s.
Author Contributions
LAM, GBN, and PBC idealized the manuscript. SAV and NH worked on trees taxonomy. ALA-F, TFG, SRML, EM, and AS built the dataset and worked on statistical tests. LAM did the primary writing with the contribution of all co-authors. All authors contributed to the article and approved the submitted version.
Conflict of Interest
The authors declare that the research was conducted in the absence of any commercial or financial relationships that could be construed as a potential conflict of interest.
Supplementary Material
The Supplementary Material for this article can be found online at: https://www.frontiersin.org/articles/10.3389/ffgc.2021.662801/full#supplementary-material
Footnotes
References
Adams, M. A., Turnbull, T. L., Sprent, J. I., and Buchmann, N. (2016). Legumes are different: leaf nitrogen, photosynthesis, and water use efficiency. Proc. Natl. Acad. Sci. U. S. A. 113, 4098–4103. doi: 10.1073/pnas.1523936113
Amundson, R., Austin, A. T., Schuur, E. A. G., Yoo, K., Matzek, V., Kendall, C., et al. (2003). Global patterns of the isotopic composition of soil and plant nitrogen. Glob. Biogeochem. Cy. 17:1031. doi: 10.1029/2002GB001903
Anderegg, L. D. L., Berner, L. T., Badgley, G., Sethi, M. L., Law, B. E., and HilleRisLambers, J. (2018). Within-species patterns challenge our understanding of the leaf economics spectrum. Ecol. Lett. 21, 734–744. doi: 10.1111/ele.12945
Antonelli, A., and Sanmartín, I. (2011). Why are there so many plant species in the Neotropics? Taxon 60, 403–414. doi: 10.1002/tax.602010
Antonelli, A., Zizka, A., Carvalho, F. A., Scharn, R., Bacon, C. D., Silvestro, D., et al. (2018). Amazonia is the primary source of neotropical biodiversity. Proc. Natl. Acad. Sci. U. S. A. 115, 6034–6039. doi: 10.1073/pnas.1713819115
Asner, G. P., and Martin, R. E. (2016). Convergent elevation trends in canopy chemical traits of tropical forests. Glob. Change Biol. 22, 2216–2227. doi: 10.1111/gcb.13164
Baker, T. R., Phillips, O. L., Malhi, Y., Almeida, S., Arroyo, L., Di Fiore, A., et al. (2004). Variation in wood density determines spatial patterns in Amazonian forest biomass. Glob. Change Biol. 10, 545–562. doi: 10.1111/j.1529-8817.2003.00751.x
Barron, A. R., Purves, D. W., and Hedin, L. O. (2011). Facultative nitrogen fixation by canopy legumes in a lowland tropical forest. Oecologia 165, 511–520. doi: 10.1007/s00442-010-1838-3
Bartón, K. (2020). MuMIn: Multi-Model Inference. R Package Version 1.43.17. Available online at: https://cran.r-project.org/web/packages/MuMIn/index.html (accessed May 14, 2021).
Basu, S., Ghosh, S., and Sanyal, P. (2019). Spatial heterogeneity in the relationship between precipitation and carbon isotopic discrimination in C3 plants: inferences from a global compilation. Glob. Planet. Change 176, 121–131. doi: 10.1016/j.gloplacha.2019.02.002
Batterman, S. A., Hedin, L. O., van Breugel, M., Ransijn, J., Craven, D. J., and Hall, J. S. (2013). Key role of symbiotic dinitrogen fixation in tropical forest secondary succession. Nature 502, 224–227. doi: 10.1038/nature12525
Boddey, R. M., Peoples, M. B., Palmer, B., and Dart, P. J. (2000). Use of the 15N natural abundance technique to quantify biological nitrogen fixation by woody perennials. Nutr. Cycl. Agroecosys. 57, 235–270. doi: 10.1023/A:1009890514844
Bucci, S. J., Goldstein, G., Meinzer, F. C., Scholz, F. G., Franco, A. C., and Bustamante, M. (2004). Functional convergence in hydraulic architecture and water relations of tropical savanna trees: from leaf to whole plant. Tree Physiol. 24, 891–899. doi: 10.1093/treephys/24.8.891
Bustamante, M. M. C., Martinelli, L. A., Silva, D. A., Camargo, P. B., Klink, C. A., Domingues, T. F., et al. (2004). 15N natural abundance in woody plants and soils of central Brazilian savannas (Cerrado). Ecol. Appl. 14, 200–213. doi: 10.1890/01-6013
Cardinale, B. J., Duffy, J. E., Gonzalez, A., Hooper, D. U., Perrings, C., Venail, P., et al. (2012). Biodiversity loss and it impact on humanity. Nature 486, 59–67. doi: 10.1038/nature11148
Carvalho, G. (2020). Flora: Tools for Interacting with the Brazilian Flora 2020. R Package Version 0.3.4. Available online at: https://cran.r-project.org/web/packages/flora/index.html (accessed May 7, 2021).
Cernusak, L. A., Ubierna, N., Winter, K., Holtum, J. A. M., Marshall, J. D., and Farquhar, G. D. (2013). Environmental and physiological determinants of carbon isotope discrimination in terrestrial plants. New Phytol. 200, 950–965. doi: 10.1111/nph.12423
Chalk, P., and Smith, C. (2020). On inorganic N uptake by vascular plants: can 15N tracer techniques resolve the NH4+ versus NO3- “preference” conundrum? Eur. J. Soil Sci. doi: 10.1111/ejss.13069
Chapin, F. S. I. I. I., Autumn, K., and Pugnaire, F. (1993). Evolution of suits of traits in response to environmental stress. Am. Nat. 142, S78–S92. doi: 10.1086/285524
Chave, J., Coomes, D., Jansen, S., Lewis, S. L., Swenson, N. G., and Zanne, A. E. (2009). Towards a worldwide wood economics spectrum. Ecol. Lett. 12, 351–366. doi: 10.1111/j.1461-0248.2009.01285.x
Chave, J., Muller-Landau, H. C., Baker, T. R., Easdale, T. A., Steege, H. T., and Webb, C. O. (2006). Regional and phylogenetic variations of wood density across 2456 neotropical tree species. Ecol. Appl. 16, 2356–2367. doi: 10.1890/1051-0761(2006)016[2356:rapvow]2.0.co;2
Cheng, H., Sinha, A., Cruz, F. W., Wang, X., Edwards, R. L., d’Horta, F. M., et al. (2013). Climate change patterns in Amazonia and biodiversity. Nat. Commun. 4:1411. doi: 10.1038/ncomms2415
Cleveland, C. C., Townsend, A. R., Taylor, P., Alvarez-Clare, S., Bustamante, M. M. C., Chuyong, G., et al. (2011). Relationships among net primary productivity, nutrients and climate in tropical rain forest: a pan-tropical analysis. Ecol. Lett. 14, 939–947. doi: 10.1111/j.1461-0248.2011.01658.x
Comstock, J. P., and Ehleringer, J. R. (1992). Correlating genetic variation in carbon isotopic composition with complex climatic gradients. Proc. Natl. Acad. Sci. U. S. A. 89, 7747–7751. doi: 10.1073/pnas.89.16.7747
Cornelissen, J. H. C., Lavorel, S., Garnier, E., Díaz, S., Buchmann, N., Gurvich, D. E., et al. (2003). A handbook of protocols for standardised and easy measurement of plant functional traits worldwide. Aust. J. Bot. 51, 335–380. doi: 10.1071/BT02124
Cornwell, W. K., Wright, I. J., Turner, J., Maire, V., Barbour, M. M., Cernusak, L. A., et al. (2018). Climate and soils together regulate photosynthetic carbon isotope discrimination within C3 plants worldwide. Glob. Ecol. Biogeogr. 27, 1056–1067. doi: 10.1111/geb.12764
Craig, H. (1953). The geochemistry of the stable carbon isotopes. Geochim. Cosmochim. Acta 3, 53–92. doi: 10.1016/0016-7037(53)90001-5
Craine, J. M., Brookshire, E. N. J., Cramer, M. D., Hasselquist, N. J., Koba, K., Marin-Spiotta, E., et al. (2015). Ecological interpretations of nitrogen isotope ratios of terrestrial plants and soils. Plant Soil 396, 1–26. doi: 10.1007/s11104-015-2542-1
Craine, J. M., Elmore, A. J., Aidar, M. P. M., Bustamante, M., Dawson, T. E., Hobbie, E. A., et al. (2009). Global patterns of foliar nitrogen isotopes and their relationships with climate, mycorrhizal fungi, foliar nutrient concentrations, and nitrogen availability. New Phytol. 183, 980–992. doi: 10.1111/j.1469-8137.2009.02917.x
Davidson, E. A., de Carvalho, C. J. R., Figueira, A. M., Ishida, F. Y., Ometto, J. P. H. B., Nardoto, G. B., et al. (2007). Recuperation of nitrogen cycling in Amazonian forests following agricultural abandonment. Nature 447, 995–998. doi: 10.1038/nature05900
Delignette-Muller, M., and Dutang, C. (2015). fitdistrplus: an R package for fitting distributions. J. Stat. Softw. 64, 1–34. doi: 10.18637/jss.v064.i04
Diefendorf, A. F., Mueller, K. E., Wing, S. L., Koch, P. L., and Freeman, K. H. (2010). Global patterns in leaf 13C discrimination and implications for studies of past and future climate. Proc. Natl. Acad. Sci. U. S. A. 107, 5738–5743. doi: 10.1073/pnas.0910513107
Ehleringer, J. R., Field, C. B., Lin, Z., and Kuo, C. (1986). Leaf carbon isotope and mineral composition in subtropical plants along an irradiance cline. Oecologia 70, 520–526. doi: 10.1007/BF00379898
Elias, M., and Potvin, C. (2003). Assessing inter- and intra-specific variation in trunk carbon concentration for 32 neotropical tree species. Can. J. For. Res. 33, 1039–1045. doi: 10.1139/X03-018
Farquhar, G. D., Ehleringer, J. R., and Hubick, K. T. (1989). Carbon isotope discrimination and photosynthesis. Annu. Rev. Plant Physiol. Plant Mol. Biol. 40, 503–537. doi: 10.1146/annurev.pp.40.060189.002443
Farquhar, G. D., and Sharkey, T. D. (1982). Stomatal conductance and photosynthesis. Ann. Rev. Plant. Physiol. 33, 317–345. doi: 10.1146/annurev.pp.33.060182.001533
Fyllas, N. M., Patiño, S., Baker, T. R., Nardoto, G. B., Martinelli, L. A., Quesada, C. A., et al. (2009). Basin-wide variations in foliar properties of Amazonian forest: phylogeny, soils and climate. Biogeosciences 6, 2677–2708. doi: 10.1023/A:1015798428743
Hedin, L. O., Brookshire, E. N. J., Menge, D. N. L., and Barron, A. R. (2009). The nitrogen paradox in tropical forest ecosystems. Annu. Rev. Ecol. Syst. 40, 613–635. doi: 10.1146/annurev.ecolsys.37.091305.110246
Högberg, P. (1997). 15N natural abundance in soil-plant systems. New Phytol. 137, 179–203. doi: 10.1046/j.1469-8137.1997.00808.x
Hooper, D. U., Chapin, F. S. I. I. I., Ewel, J. J., Hector, A., Inchaust, P., Lavorel, S., et al. (2005). Effects of biodiversity on ecosystem functioning: a consensus of current knowledge. Ecol. Monogr. 75, 3–35. doi: 10.1890/04-0922
Houlton, B. Z., Sigman, D. M., Schuur, E. A. G., and Hedin, L. O. (2007). A climate-driven switch in plant nitrogen acquisition within tropical forest communities. Proc. Natl. Acad. Sci. U. S. A. 104, 8902–8906. doi: 10.1073/pnas.0609935104
Hughes, C. E., Pennington, R. T., and Antonelli, A. (2013). Neotropical plant evolution: assembling the big picture. Bot. J. Linn. Soc. 171, 1–18. doi: 10.1111/boj.12006
Hulshof, C. M., and Spasojevic, M. J. (2020). The edaphic control of plant diversity. Global Ecol. Biogeogr. 29, 1634–1650. doi: 10.1111/geb.13151
Kuznetsova, A., Brockhoff, P. B., and Christensen, R. H. B. (2017). lmerTest package: tests in linear mixed effects models. J. Stat. Softw. 82, 1–26. doi: 10.18637/jss.v082.i13
Ledo, R. M. D., and Colli, G. R. (2017). The historical connections between the Amazon and the Atlantic Forest revisited. J. Biogeogr. 44, 2551–2563. doi: 10.1111/jbi.13049
Lenth, R. V., Buerkner, P., Herve, M., Love, J., Riebl, H., and Singmann, H. (2021). Emmeans: Estimated Marginal Means, Aka Least-Squares Means. R Package Version 1.6.0. Available online at: https://cran.r-project.org/web/packages/emmeans/index.html (accessed May 17, 2021).
Lins, S. R. M., Coletta, L. D., Ravagnani, E. C., Gragnani, J. G., Mazzi, E. A., and Martinelli, L. A. (2016). Stable carbon composition of vegetation and soils across an altitudinal range in the coastal Atlantic forest of Brazil. Trees 30, 1315–1329. doi: 10.1007/s00468-016-1368-7
Lloyd, J., and Farquhar, G. D. (1994). 13C discrimination during CO2 assimilation by the terrestrial biosphere. Oecologia 99, 201–215. doi: 10.1007/BF00627732
Martin, E. R., Asner, G. P., Bentley, L. P., Shenkin, A., Salinas, N., Huaypar, K. Q., et al. (2020). Covariance of sun and shade leaf traits along a tropical forest elevation gradient. Front. Plant Sci. 10:1810. doi: 10.3389/fpls.2019.01810
Martinelli, L. A., Nardoto, G. B., Soltangheisi, A., Reis, C. R. G., Abdalla-Filho, A. L., Camargo, P. B., et al. (2020). Determining ecosystem functioning in Brazilian biomes through foliar carbon and nitrogen concentrations and stable isotope ratios. Biogeochemistry 154, 405–423. doi: 10.1007/s10533-020-00714-2
Martinelli, L. A., Piccolo, M. C., Townsend, A. R., Vitousek, P. M., Cuevas, E., McDowell, W., et al. (1999). Nitrogen stable isotopic composition of leaves and soil: tropical versus temperate forests. Biogeochemistry 46, 45–65. doi: 10.1023/A:1006100128782
McKey, D. (1994). “Legumes and nitrogen: the evolutionary ecology of a nitrogen-demanding lifestyle,” in Advances in Legume Systematics – Part 5: The Nitrogen Factor, eds J. I. Sprent and D. McKey (Kew: Royal Botanic Gardens), 211–228.
Melillo, J. M., Aber, J. D., and Muratore, J. F. (1982). Nitrogen and lignin control of hardwood leaf litter decomposition dynamics. Ecology 63, 621–626. doi: 10.2307/1936780
Meseguer, A. S., Antoine, P. O., Fouquet, A., Delsuc, F., and Condamine, F. L. (2020). The role of the Neotropics as a source of world tetrapod biodiversity. Glob. Ecol. Biogeogr. 29, 1565–1578. doi: 10.1111/geb.13141
Nakagawa, S., Johnson, P. C. D., and Schielzeth, H. (2017). The coefficient of determination R2 and intra-class correlation coefficient from generalized linear mixed-effects models revised and expanded. J. R. Soc. Interface 14:20170213. doi: 10.1098/rsif.2017.0213
Nardoto, G. B., Quesada, C. A., Patiño, S., Saiz, G., Baker, T. R., Schwarz, M., et al. (2014). Basin-wide variations in Amazon forest nitrogen cycling characteristics as inferred from plant and soil 15N:14N measurements. Plant. Ecol. Divers. 7, 173–187. doi: 10.1080/17550874.2013.807524
Nasto, M. K., Alvarez-Clare, S., Lekberg, Y., Sullivan, B. W., Townsend, A. R., and Cleveland, C. C. (2014). Interactions among nitrogen fixation and soil phosphorus acquisition strategies in lowland tropical rain forests. Ecol. Lett. 17, 1282–1289. doi: 10.1111/ele.12335
Oelmann, Y., Buchmann, N., Gleixner, G., Habekost, M., Roscher, C., Rosenkranz, S., et al. (2011). Plant diversity effects on aboveground and belowground N pools in temperate grassland ecosystems: development in the first 5 years after establishment. Glob. Biogeochem. Cy. 25:GB2014. doi: 10.1029/2010GB003869
Oliveira, L. Z., Uller, H. F., Klitzke, A. R., Eleotério, J. R., and Vibrans, A. C. (2019). Towards the fulfillment of a knowledge gap: wood densities for species of the subtropical Atlantic forest. Data 4:104. doi: 10.3390/data4030104
Oliveras, I., Bentley, L., Fyllas, N. M., Gvozdevaite, A., Shenkin, A. F., Peprah, T., et al. (2020). The influence of taxonomy and environment on leaf trait variation along tropical abiotic gradients. Front. For. Glob. Change 3:18. doi: 10.3389/ffgc.2020.00018
Ometto, J. P. H. B., Ehleringer, J. R., Domingues, T. F., Berry, J. A., Ishida, F. Y., Mazzi, E., et al. (2006). The stable carbon and nitrogen isotopic composition of vegetation in tropical forests of the Amazon Basin, Brazil. Biogeochemistry 79, 251–274. doi: 10.1007/s10533-006-9008-8
Pardo, L. H., Templer, P. H., Goodale, C. L., Duke, S., Groffman, P. M., Adams, M. B., et al. (2006). Regional assessment of N saturation using foliar and root δ15N. Biogeochemistry 80, 143–171. doi: 10.1007/s10533-006-9015-9
Pennington, R. T., Lavin, M., and Oliveira-Filho, A. (2009). Woody plant diversity, Evolution, and ecology in the tropics: perspectives from seasonally dry tropical forests. Annu. Rev. Ecol. Evol. Syst. 40, 437–457. doi: 10.1146/annurev.ecolsys.110308.120327
Poorter, L., van de Plassche, M., Willems, S., and Boot, R. G. A. (2004). Leaf traits and herbivory rates of tropical tree species differing in successional status. Plant Biol. 6, 746–754. doi: 10.1055/s-2004-821269
Rangel, T. F., Edwards, N. R., Holden, P. B., Diniz-Filho, J. A., Gosling, W. D., Coelho, M. T. P., et al. (2018). Modeling the ecology and evolution of biodiversity: biogeographical cradles, museums, and graves. Science 361:eaar5452. doi: 10.1126/science.aar5452
Read, J., and Farquhar, G. (1991). Comparative studies in Nothofagus (Fagaceae). I. Leaf carbon isotope discrimination. Funct. Ecol. 5, 684–695. doi: 10.2307/2389489
Robinson, D. (2001). δ15N as an integrator of the nitrogen cycle. Trends Ecol. Evol. 16, 153–162. doi: 10.1016/S0169-5347(00)02098-X
Rull, V. (2011). Neotropical biodiversity: timing and potential drivers. Trends Ecol. Evol. 26, 508–513. doi: 10.1016/j.tree.2011.05.011
Russell, A. E., and Raich, J. W. (2012). Rapidly growing tropical trees mobilize remarkable amounts of nitrogen, in ways that differ surprisingly among species. Proc. Natl. Acad. Sci. U. S. A. 109, 10398–10402. doi: 10.1073/pnas.1204157109
Schuur, E. A. G., and Matson, P. A. (2001). Net primary productivity and nutrient cycling across a mesic to wet precipitation gradient in Hawaiian montane forest. Oecologia 128, 431–442. doi: 10.1007/s004420100671
Sena-Souza, J. P., Houlton, B. Z., Martinelli, L. A., and Nardoto, G. B. (2020). Reconstructing continental-scale variation in soil δ15N: a machine learning approach in South America. Ecosphere 11:e03223. doi: 10.1002/ecs2.3223
Siddique, I., Engel, V. L., Parrotta, J. A., Lamb, D., Nardoto, G. B., Ometto, J. P. H. B., et al. (2008). Dominance of legume trees alters nutrient relations in mixed species forest restoration plantings within seven years. Biogeochemistry 88, 89–101. doi: 10.1007/s10533-008-9196-5
Smirnoff, N., and Stewart, G. R. (1985). Nitrate assimilation and translocation by higher plants: comparative physiology and ecological consequences. Physiol. Plant 64, 133–140. doi: 10.1111/j.1399-3054.1985.tb02326.x
Sprent, J. I. (1995). Legume trees and shrubs in the tropics: N2 fixation in perspective. Soil Biol. Biochem. 27, 401–407. doi: 10.1016/0038-0717(95)98610-Z
Swenson, N. G., and Enquist, B. J. (2007). Ecological and evolutionary determinants of a key plant functional trait: wood density and its community-wide variation across latitude and elevation. Am. J. Bot. 94, 451–459. doi: 10.3732/ajb.94.3.451
Taylor, B. N., and Ostrowsky, L. R. (2019). Nitrogen-fixing and non-fixing trees differ in leaf chemistry and defence but not herbivory in a lowland Costa Rican rain forest. J. Trop. Ecol. 35, 270–270. doi: 10.1017/S0266467419000233
Tedersoo, L., Laanisto, L., Rahimlou, S., Toussaint, A., Hallikma, T., and Pärtel, M. (2018). Global database of plants with root-symbiotic nitrogen fixation: NodDB. J. Veg. Sci. 29, 560–568. doi: 10.1111/jvs.12627
Tilman, D., Isbell, F., and Cowles, J. M. (2014). Biodiversity and ecosystem functioning. Annu. Rev. Ecol. Evol. Syst. 45, 471–493. doi: 10.1146/annurev-ecolsys-120213-091917
Townsend, A. R., Asner, G. P., and Cleveland, C. C. (2008). The biogeochemical heterogeneity of tropical forests. Trends Ecol. Evol. 23, 424–431. doi: 10.1016/j.tree.2008.04.009
Townsend, A. R., Cleveland, C. C., Asner, G. P., and Bustamante, M. M. C. (2007). Controls over foliar N:P ratios in tropical rain forests. Ecology 88, 107–118. doi: 10.1890/0012-9658(2007)88[107:cofnri]2.0.co;2
Ulloa, U. C., Acevedo-Rodríguez, P., Beck, S., Belgrano, M. J., Bernal, R., Berry, P. E., et al. (2017). An integrated assessment of the vascular plant species of the Americas. Science 358, 1614–1617. doi: 10.1126/science.aao0398
Valentine, A. J., Benedito, V. A., and Kang, Y. (2018). Legume nitrogen fixation and soil abiotic stress: from physiology to genomics and beyond. Annu. Plant Rev. Online 42, 207–248. doi: 10.1002/9781119312994.apr0456
Vasseur, F., Violle, C., Enquist, B. J., Granier, C., and Vile, D. (2012). A common genetic basis to the origin of the leaf economics spectrum and metabolic scaling allometry. Ecol. Lett. 15, 1149–1157. doi: 10.1111/j.1461-0248.2012.01839.x
Vieira, S. A., Alves, L. F., Duarte-Neto, P. J., Martins, S. C., Veiga, L. G., Scaranello, M. A., et al. (2011). Stocks of carbon and nitrogen and partitioning between above- and belowground pools in the Brazilian coastal Atlantic Forest elevation range. Ecol. Evol. 1, 421–434. doi: 10.1002/ece3.41
Virginia, R. A., and Delwiche, C. C. (1982). Natural 15N abundance of presumed N2-fixing and non-N2-fixing plants from selected ecosystems. Oecologia 54, 317–325. doi: 10.1007/BF00380000
Vitousek, P. M., Cassman, K., Cleveland, C., Crews, T., Field, C. B., Grimm, N. B., et al. (2002). Towards an ecological understanding of biological nitrogen fixation. Biogeochemistry 57, 1–45. doi: 10.1023/A:1015798428743
Vitousek, P. M., and Sanford, R. L. Jr. (1986). Nutrient cycling in moist tropical forest. Annu. Rev. Ecol. Syst. 17, 137–167. doi: 10.1146/annurev.es.17.110186.001033
Westoby, M., Falster, D. S., Moles, A. T., Vesk, P. A., and Wright, I. J. (2002). Plant ecological strategies: some leading dimensions of variation between species. Annu. Rev. Ecol. Evol. Syst. 33, 125–159. doi: 10.1146/annurev.ecolsys.33.010802.150452
Wolf, A. A., Funk, J. L., and Menge, D. N. L. (2017). The symbionts made me do it: legumes are not hardwired for high nitrogen concentrations but incorporate more nitrogen when inoculated. New Phytol. 213, 690–699. doi: 10.1111/nph.14303
Wright, I. J., Ackerly, D. D., Bongers, F., Harms, K. E., Ibarra-Manriquez, G., Martinez-Ramos, M., et al. (2007). Relationships among ecologically important dimensions of plant trait variation in seven neotropical forests. Ann. Bot. 99, 1003–1015. doi: 10.1093/aob/mcl066
Wright, I. J., Reich, P. B., Westoby, M., Ackerly, D. D., Baruch, Z., Bongers, F., et al. (2004). The worldwide leaf economics spectrum. Nature 428, 821–827. doi: 10.1038/nature02403
Wright, S. J., Kitajima, K., Kraft, N. J. B., Reich, P. B., Wright, I. J., Bunker, D. E., et al. (2010). Functional traits and the growth-mortality trade-off in tropical trees. Ecology 91, 3664–3674. doi: 10.1890/09-2335.1
Wright, S. J., Muller-Landau, H. C., Condit, R., and Hubbell, S. P. (2003). Gap-dependent recruitment realized vital rates, and size distributions of tropical trees. Ecology 84, 3174–3185. doi: 10.1890/02-0038
Keywords: Neotropics, variance, site, taxonomy, Fabaceae, wood density
Citation: Martinelli LA, Abdalla-Filho AL, Gomes TF, Lins SRM, Mariano E, Soltangheisi A, de Camargo PB, Vieira SA, Higuchi N and Nardoto GB (2021) Partitioning of Environmental and Taxonomic Controls on Brazilian Foliar Content of Carbon and Nitrogen and Stable Isotopes. Front. For. Glob. Change 4:662801. doi: 10.3389/ffgc.2021.662801
Received: 01 February 2021; Accepted: 08 June 2021;
Published: 12 July 2021.
Edited by:
Michelle Y. Wong, Cary Institute of Ecosystem Studies, United StatesReviewed by:
Zachary E. Kayler, University of Idaho, United StatesErika J. Foster, Purdue University, United States
Copyright © 2021 Martinelli, Abdalla-Filho, Gomes, Lins, Mariano, Soltangheisi, de Camargo, Vieira, Higuchi and Nardoto. This is an open-access article distributed under the terms of the Creative Commons Attribution License (CC BY). The use, distribution or reproduction in other forums is permitted, provided the original author(s) and the copyright owner(s) are credited and that the original publication in this journal is cited, in accordance with accepted academic practice. No use, distribution or reproduction is permitted which does not comply with these terms.
*Correspondence: Luiz A. Martinelli, bWFydGluZWxsaUBjZW5hLnVzcC5icg==