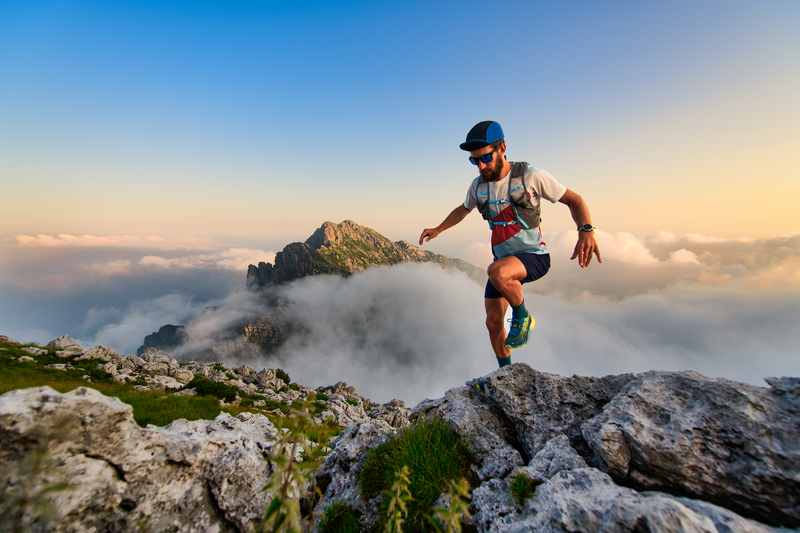
95% of researchers rate our articles as excellent or good
Learn more about the work of our research integrity team to safeguard the quality of each article we publish.
Find out more
ORIGINAL RESEARCH article
Front. For. Glob. Change , 24 June 2021
Sec. Forest Soils
Volume 4 - 2021 | https://doi.org/10.3389/ffgc.2021.629600
The soils beneath the rainforest of Guyana have the potential to hold, and release, large stores of carbon under land use and climate change. Little is known about soil carbon stocks or molecular dynamics in this region. This study therefore aims to elucidate differences in the molecular (lignin and tannin) and bulk soil organic carbon (SOC) stocks in different ‘sub-environments’ along a rainforest-savannah boundary, setting a framework for further investigation into the soil carbon dynamics of the region. Bulk SOC analysis shows that Gleysols have the highest stocks, particularly those under rainforest vegetation (swamp and island forests surrounded by savannah), whereas Plinthosols have significantly lower SOC stocks. Texture and soil water content analysis indicates that predominantly clay soils play a role in high SOC stocks, whilst predominantly sandy soils prevent SOC stocks from accumulating. Clay and sand are present in both Gleysols and Plinthosols, to different extents. Analysis of lignin and tannin in surface soils of the sub-environments reveals clear differences in molecular composition. Heavily degraded lignin signatures in rainforest Gleysols suggests a surrounding physio-chemical environment which promotes their degradation. Conversely, Plinthosols beneath woodland within the savannah have the greatest amount of lignin and tannin products. The presence of the clay mineral kaolinite and iron oxide strengite in these soils indicates a low ability for protection or complexing of organic matter. Therefore, water content and microbial activity may play a more important role in the degradation of lignin and tannin, as well as the SOC stock. With the potential for future deforestation due to land use or climate change, the high lignin degradation of Gleysols indicates a vulnerability to savannah encroachment. Forest Islands isolated from the main forest biome are the most vulnerable to change, and could lose a significant proportion of their SOC stock in a transition to savannah.
Soil organic matter (SOM) is a key component of carbon storage in natural ecosystems. However, pressures from land use change, for example from forest to agriculture, and climate change altering the local weather patterns potentially impacts the amount of carbon stored in SOM through exposing it to greater degradation, increasing CO2 emissions from the soil (Lal, 2004). Amazonian soils store approximately 66.9 Pg C in the top meter, accounting for around 4% of total global carbon stocks (Batjes and Dijkshoorn, 1999). Whilst some areas of the Amazon, which have large potentials to store carbon, have experienced extensive deforestation for agriculture, Guyana’s rainforest remains largely intact. They cover 85% of the land and are bordered by the Rupununi savannah close to Brazil (Palo, 1994). It is therefore likely that soils under rainforest in Guyana hold large carbon stores, however there is less certainty of these stores on the savannah boundary.
In this study we focus on lignin, which is a key component of SOM, constituting about a third to the organic carbon content of the biosphere (Boerjan et al., 2003). Our knowledge of lignin stability and degradation in tropical settings is limited mainly to agricultural systems (Guggenberger et al., 1995; Olk et al., 2002; Filley et al., 2006; Rumpel et al., 2007), whereas little is known about lignin stability and degradation in natural tropical systems. There are some examples of lignin degradation in tropical ecosystems, although these are mainly in wetlands (Bala Krishna Prasad and Ramanathan, 2009; Spencer et al., 2010; DeAngelis et al., 2011).
Soil organic matter stores carbon in complex compounds, for instance lignin, and releases carbon as CO2 primarily through microbial decomposition but also through abiotic factors. In woody plants, such as those found in rainforest and savannah systems (less frequent in the latter), lignin can contribute 30% to mass, whilst non-woody plants, including agricultural crops, show lesser amounts (Adler, 1977). Lignin has previously been considered recalcitrant and therefore preserved in soil due to the complexity and variability in linkages within the lignin biopolymer and its high molecular weight (R. L. Crawford, 1981). However, this recalcitrance has been contested and it is now known that its stability and preservation within soils is largely due to the physio-chemical environment surrounding it (Thevenot et al., 2010). Lignin degradation is fastest under aerobic conditions (Vane and Abbott, 1999; Kiem and Kögel-Knabner, 2003; Dignac et al., 2005; Bahri et al., 2006; Kalbitz et al., 2006; Marschner et al., 2008; Klotzbücher et al., 2011; Dungait et al., 2012), where white rot fungi are one of the most efficient degraders (Gold et al., 1989; Hatakka, 1994; Robertson et al., 2008). Some species of bacteria (Streptomyces and Nocardia) have also been found to degrade lignin (Sørensen, 1962; Antai and Crawford, 1981; Crawford et al., 1983; Godden et al., 1992; Trojanowski, 2001). In addition to biotic degradation, abiotic factors also play a role in the breakdown of lignin, for example through photo-oxidation (Hernes and Benner, 2003; Gallo et al., 2006; Frouz et al., 2011) and leaching (Hernes et al., 2007).
The rainforest and savannah regions in Guyana support a range of different vegetation types. These are likely to affect SOM stocks and cycling through variations in leaf and wood litter chemistry, especially the abundance and type of lignin, tannin and carbohydrates present (Carr et al., 2013). Similarly, climate change may affect the ability of these soils to act as a carbon store. Currently, the seasonality of these ecosystems is divided between two main weather patterns; a coastal ‘Tropical Wet’ (or ‘Tropical Rainforest’) climatic region of two wet and two dry seasons and a ‘Tropical Wet-Dry’ (or ‘Savannah’) climatic region further inland covering the Guyana savannah, where a single wet and dry season prevails, therefore providing a smaller total precipitation than the ‘Tropical Wet’ pattern (Bovolo et al., 2012). However, with climate and land use change there is a potential for these patterns to change toward overall drier and hotter conditions (McSweeney et al., 2010). Hotter conditions are expected to increase the rate at which bacteria can break down organic carbon by heterotrophic respiration, speeding up the rate of chemical reactions (Lloyd and Taylor, 1994; Fenner and Freeman, 2011; Wang et al., 2014). Future alterations in hydrology are less certain than those in temperatures, but hydrology often determines the redox potential of soils, impacting oxic decomposition, with a decrease in microbial decomposition when flooded and intensive degradation during dryer seasons, as microbes utilize moisture remaining from the wet season (Fenner and Freeman, 2011; Bovolo et al., 2012).
Studies that have assessed molecular SOM in natural systems show that vegetation types have differing molecular carbon inputs into soils, which may be either more or less stable and can alter the SOC stock when these vegetation types shift (Guggenberger et al., 1995; Filley et al., 2006; Stewart et al., 2011; Carr et al., 2013). More recent studies have detected that polysaccharides, previously thought of as labile, are a major pyrolysis-GC/MS product in cultivated dystric cambisols, thus actually relatively stable (Gleixner et al., 2002). It has also been suggested that lignin has a faster turnover rate than bulk SOM in some temperate zone grassland and arable soils (Dignac et al., 2005; Heim and Schmidt, 2007). This is contrasted by other studies observing that lignin is not always preferentially degraded (Van Bergen et al., 1997; Kiem and Kögel-Knabner, 2003; Nierop et al., 2003).
In order to better understand the fate of lignin in pristine tropical ecosystems, we have performed a detailed biogeochemical study in a largely natural ecosystem in the Northern Rupununi, Central Guyana. A reconnaissance survey of soils and vegetation over a c. 10 km section of savannah-rainforest boundary was preliminarily undertaken. Following this, a representative section for a detailed transect study of bulk and molecular carbon analysis was chosen, running 2.5 km either side of this boundary. This paper presents an investigation of the molecular characteristics of the SOM and the SOC stock along this boundary. The surface soil is the most critical zone in the profile for carbon dynamics, particularly on the molecular scale, as it is where the majority of dead plant material (above and below ground) is deposited and where most microbial activity occurs (Foster, 1988). Further, lignin and tannin phenols have been shown to be most abundant in the surface soil, decreasing significantly with depth through the profile (Nierop and Filley, 2007; Mason et al., 2009). We therefore semi-quantitatively assess the amount of lignin and tannin products from samples of living plant tissues, freshly deposited litter and surface soil (0–6 cm) and their extents of degradation using thermally assisted hydrolysis and methylation (THM) in the presence of tetramethylammonium hydroxide (TMAH) (Mason et al., 2009).
The aim of the study is to elucidate differences in the composition of molecular SOM and size of the SOC stock under a mosaic of vegetation types on the savannah-rainforest boundary. This baseline data collection and the differences observed in molecular and bulk SOC in the study region provide a framework for further investigation relating to the soil dynamics and their potential alteration through changes in seasonality and impact from climate extreme events (droughts/floods), both linked to climate change. This study provides critical geochemical data across sub-settings of a savannah-forest boundary that is commonly observed in the Amazon system. We present data from soil samples that have been obtained during the end of the dry season 2012, leaving studies that resolve seasonal or multi-annual variability still to be done.
We hypothesize that vegetation will have a dominant effect on the SOM composition and that the soil type will have a strong influence on the degradation state of this SOM. Consistent with other studies (Swain et al., 2010; Angst et al., 2021) we postulate that SOM degradation is inversely related to SOC stock. SOM degradation, defined as the break-down of organic matter in the soil, is controlled by the interplay of climate (precipitation and temperature), vegetation type and soil matrix factors including the physio-chemical environment and microbes. In order to achieve this aim, the objectives of this study were to:
(a) Quantify the surface (0–6 cm) and full profile SOC stock of undisturbed soils across a savannah-rainforest transect.
(b) Identify the molecular chemical composition (lignin and tannin) of vegetation inputs and surface soils across the transect.
(c) Investigate the relationship between the extent of lignin degradation and the SOC stock.
(d) Investigate how variations in soil texture, moisture and vegetation may impact SOC stocks and lignin degradation.
The area of this study is within the Northern Rupununi region of central Guyana. The Rupununi region is situated in the north eastern part of the Takutu Basin – a Mesozoic graben (280 km long and 40 km wide) between parallel faults which form the Pakaraima Mountains to the north and Kanuku Mountains to the south. The study sites are within the tribal lands of Wowetta at 4°01′02.5″N, 59°04′06.0″W (Figure 1). Following a reconnaissance survey of an area covering 10 km along the rainforest-savannah boundary, a transect of the most representative soils and vegetation was identified for sampling. From the boundary, the transect extends 2.5 km into the rainforest and 2.5 km into the savannah. Along this transect the topography undulated through flat areas, small hills or mounds of hardened laterite and wet depressions. The sites along this transect were named Savannah Grassland (short grasses with no trees), Savannah Woodland (clumps of trees forming small woods within the predominantly grass-covered savannah), Savannah Tree (an isolated tree within the grassy savannah), Savannah Swamp (a waterlogged area of grasses, sedges and scattered palm trees which are few in number), Forest Island (an area of rainforest trees surrounded by savannah), Transition zone (dense scrub vegetation directly between the savannah and rainforest proper), Swamp Forest (a waterlogged topographical depression within the rainforest), Pisoplinthic Forest (an area of mixed rainforest tree spp. on a mound of hardened lateritic nodules – pisoplinthic material) and Mixed Forest (an area of level ground with mixed rainforest tree spp.) We use the term ‘sub-environment’ to refer to these sites from this point onward. Similar vegetation types and undulating landscape topography have been found in previous studies of the area (Eden, 1964; Sinha, 1968; Daniel and Hons, 1984; ter Steege, 2000). Although the area of savannah in Guyana is relatively small, its pristine quality provides a unique opportunity to investigate this at a climate sensitive interface (Bovolo et al., 2012).
Figure 1. Location of study sites in Wowetta, Central Guyana. White areas indicate the Northern Rupununi savannah and green areas indicate rainforest. SG, Savannah Grassland; SW, Savannah Woodland; FI, Forest Island; ST, Savannah Tree; SS, Savannah Swamp; T, Transition zone; SF, Swamp Forest; PF, Pisoplinthic Forest; MF, Mixed Forest. Inset: the location of the study areas within Guyana.
Nine sample pits were excavated in February 2012, at the end of the main annual dry season, in order to sample and assess soils in advance of upcoming wet season floods. During sample collection, soil profile descriptions were taken and recorded according to the FAO (IUSS Working Group WRB, 2015). These included soil texture (‘hand texture method’), soil color (Munsell Color chart) structure, boundary depth of each horizon and full sampling depth of the soil profile. Water table depth from the surface was also recorded using measuring tape as soon as the water table had been breached.
Biomass samples (fresh shoot/leaf litter and wood litter) were sampled in triplicate from the surface of the soil pit and the surrounding area (within 0.25 m of the soil pit). Where no litter layer existed, such as in the savannah grassland sites, fresh shoots were sampled. Fresh grass shoots were also sampled in areas of savannah with trees in addition to leaf litter, as the grass itself did not form a litter layer. All soil samples (including for bulk density) were taken in triplicate from 0–6 cm from the surface of each soil profile as well as at the mid-point between the top and bottom of each horizon in every soil profile. Bulk density sampling excluded the pisoplinthic forest (PF), where the pisoplinthic material (hardened aggregates of iron oxide) prevented accurate sampling. Volumetric water content (θ) was calculated using the weight of the pre-dried soil, the weight of oven dry soil, the known volume of bulk density sample and the bulk density (Rowell, 1994). It is important to note that water content was only measured at one point in time, from each triplicate of soil.
All samples for molecular analysis were stored in sealed amber glass jars and stored at – 20°C from the field to the home laboratory to avoid any out of situ degradative changes. An aliquot of all samples was taken in order to conduct pH and molecular organic matter analysis on. Samples were then freeze dried and ground to a fine fraction (<0.25 μm) and passed through a <0.25 μm sieve to verify the particle size. Subsequently, organic soil compounds were extracted using Accelerated Solvent Extraction (ASE).
X-ray diffraction was undertaken on a subset of surface (0–6 cm) soil samples across the transect, covering the range of soil types found (including one sample from the Swamp Forest, Mixed Forest, Transition zone, Savannah Swamp, Savannah Woodland and Savannah Tree soils). Surface samples were selected to correspond to the molecular analysis also conducted at the surface of soil profiles (see Section “THM with TMAH analysis” below), in order to better understand the abiotic factors which may affect the state of molecular degradation. For example, the mineralogy may have consequences for the ability of the soil to protect or complex with organic matter (Gu et al., 1994; Sollins et al., 1996; Arnarson and Keil, 2000; Lützow et al., 2006). X-ray diffraction was carried out using a PANalytical X’Pert Pro Multipurpose Diffractometer (MPD) fitted with an X’Celerator detector, using 500 mg aliquot of the dried, ground and sieved (<0.25 μm) soil samples.
Total Organic Carbon (TOC) concentration was determined using 0.1 g of freeze dried, sieved (to < 0.25 μm) and ground sample. Hydrochloric acid of 4.0 mol/L was added at an amount of 1 mL to each crucible and was drained from the sample for 4 h. The samples were then placed in the oven at 60 to 70°C for 16–24 h. A blank was used with each sample set.
After calculating both TOC concentration and bulk density it was then possible to calculate the weight of organic carbon (t C ha–1) of soils by horizon, using the following formula:
Equation 1 Soil organic carbon stock (SOC):
Where d = depth (cm), Db = bulk density (g cm3), TOC = total organic carbon (%).
Through using TOC concentration as a percentage, the conversion from g per cm3 to t per ha, is avoided, which has otherwise caused confusion and errors in the past (Cannell and Milne, 1995).
Where horizons were missing bulk density data, due to an inability to sample them (e.g., water saturation or depth of horizon), bulk densities were estimated using surrogate values determined by pedotransfer functions or rules (Bouma and Van Lanen, 1987), as used in Batjes and Dijkshoorn (1999). The primary surrogate option was to calculate the mean bulk density of the soil profile and use this value for missing horizons within the profile. The secondary option (if only one bulk density value was available for a profile) was to use a mean soil profile bulk density value of a corresponding soil type and the one available horizon. These surrogate values have been indicated using a ‘∗’ in Table 1 below. The Pisoplinthic Forest soil did not have any similar profiles to use as surrogates, due to the hard lateritic nodules that it consisted of, therefore SOC stock for this profile has not been estimated.
Table 1. Full soil profile TOC%, bulk density and soil SOC stock for each sub-environment, parentheses indicate standard error of triplicates.
Lignin and tannin phenols of vegetation and surface soil were assessed using on-line thermally assisted hydrolysis and methylation (THM) in the presence of unlabeled and 13C-labeled tetramethylammonium hydroxide (TMAH) (Mason et al., 2009). For the standard, 5α-androstane with dichloromethane was used. THM was performed using a pulsed mode open pyrolysis system specifically a CDS 1000 pyroprobe unit (Chemical Data Systems, United States) fitted with a platinum coil and a CDS 1500 valved interface, as previously published (Mason et al., 2009).
The use of 13C-labeled TMAH deconvolutes the contribution of intact lignin phenols [guaiacyl (G), cinnamyl (C), and syringyl (S)] from microbially altered lignin or non-lignin sources including tannins (Filley et al., 1999). For example, the unlabeled thermochemolysis product S6 (3,4,5-trimethoxy benzoic acid methyl ester) is often a composite of syringic acid (lignin-derived) and gallic acid (tannin-derived) with the same mass spectra, however this will have different mass spectra following 13C-labeling (Mason et al., 2009). Thus, the amounts of unlabeled products can be corrected to give the “true” quantities of intact lignin present enabling accurate calculation of the lignin phenol parameters, e.g., lambda (Λ) (Filley et al., 2006; Nierop and Filley, 2007; Mason et al., 2009). Individual lignin phenols were corrected via the calculation of the hydroxyl contents of intact lignin, altered demethylated lignin and non-lignin sources. Source indicators C/G and S/G are used for non-woody and angiosperm/gymnosperm inputs, calculated using lignin phenols as (P18 + G18)/(G4 + G6) and (S4 + S6)/(G4 + G6), respectively (Filley et al., 2006). Ratios of the acid (Ad) and aldehyde (Al) endmembers of the lignin phenols guaiacyl and syringyl, as represented by [Ad/Al]G and [Ad/Al]S (calculated as G6/G4 and S6/S4) indicate the level of oxidation for guaiacyl and syringyl phenols, respectively (Filley et al., 2006). Increasing [Ad/Al]G/S values indicate increasing oxidation, therefore, it is usually expected that these ratios are higher in soils than vegetation (Filley et al., 1999). Lambda (Λ) was calculated as the sum of amounts of the 8 dominant lignin phenols (G4 + G5 + G6 + S4 + S5 + S6 + G18 + P18) (Filley et al., 2006).
In order to determine gallic acid (tannin) and demethylated lignin sources, the % aromatic hydroxyl contents of S6 (1% OH, 2% OH, and 3% OH) were determined using 13C-labeled TMAH. 1% OH refers to intact lignin, 2% OH to microbially demethylated lignin (syringic acid) and 3% OH to gallic acid (tannin derived). Filley et al. (2006) showed how multiple sources such as intact lignin and microbially altered lignin (specifically their methoxyl functionalities) can lead to the formation of identical products. Using previously established equations (Filley et al., 2006), these hydroxyl contents were calculated and then converted into mg/100 mg OC. The unlabeled TMAH thermochemolysis of these samples allowed the identification of S6, and thus the baseline for accurately calculating the 13C % addition.
The soil profiles sampled consist of Plinthosols and Gleysols with a range of sub-classifications and an underlying presence of iron oxide in plinthic form throughout the transect (Figure 2; FAO-ISRIC, 2006). In albic Plinthosols at SG, SW and ST sites, TOC concentration ranges from 0.03 to 1% and pH from 4.3–5.7 with greater acidity and lower SOC in the surface 6 cm. The color of this horizon changes from dark grayish brown in the SG to dark brown/very dark grayish brown beneath SW and ST, likely caused by different vegetation inputs or a persistence of SOM in the latter sites (see Figures 6C,D depicting more degraded lignin phenols which may indicate older SOM and Figure 7 which shows significantly greater gallic acid in SW and ST than SG). Hand texture assessments confirm predominantly sandy textures (sand – sandy clay loam, generally increasing in clay with depth). The SW has a sandy loam surface soil compared to the sand texture of ST and SG, therefore, a slightly high clay content, however the water content, which may therefore also be greater and result in less degradation of organic carbon, does not differ between SW and ST soils. MF is an acric Plinthosol; the surface soil is pH 4.3, has a TOC concentration of 1.1% and a silt loam surface texture grading to clay with depth. PF is a pisoplinthic Plinthosol with a pH of 4.5, a TOC concentration of 2.7% and a mix of pisoplinthic material and coarse sandy texture. Sites FI and SF are both humic Gleysols, with black humified surface horizons that have high TOC concentrations, the FI average being roughly half of SF (6% and 13% respectively). The texture here is predominantly clay, although some sand does exist (e.g., the main profile texture of SF is sandy clay). SS and T soils are plinthic Gleysols with pH of 4.8 and 4.8 respectively and TOC concentration of 2.2 and 2.1% respectively. The Transition zone soil is sandy clay loam to clay with depth, however the SS soil is a sandy loam grading to sand with depth.
Figure 2. Schematic of the sequence of sub-environments sampled along the 5 km transect in Wowetta. SG, Savannah Grassland; SW, Savannah Woodland; FI, Forest Island; ST, Savannah Tree; SS, Savannah Swamp; T, Transition zone; SF, Swamp Forest; PF, Pisoplinthic Forest; MF, Mixed Forest.
The X-ray diffraction results reveal that quartz is present in all the soils tested, including the Gleysols SF and SS, which is also evident from sand detected in the hand texture assessments. The clay mineral kaolinite is present in all samples tested except for the savannah sites ST and SW. The ferric iron phosphate mineral strengite is also present in all the soils tested, except for SF. Anatase, nepheline, and scapolite are also present in small amounts (see Supplementary Material for examples of a savannah and rainforest soils). This mineralogy is unlikely to have a significant effect on the physical protection or complexing of organic carbon, as the quartz, clay mineral kaolinite, ferric iron strengite and other minerals present have a low reactivity (Lindsay, 1979; Haynes, 1982).
The surface 0–6 cm and full profile SOC for each horizon are given in Table 1. The highest surface and full soil profile SOC stocks were measured in humic Gleysols; the Swamp Forest (59 t C ha–1 and 389 t C ha–1, respectively), followed by the Forest Island (31 t C ha–1 and 258 t C ha–1, respectively); these sites also have the highest volumetric water contents of 75 and 67%, respectively (Figure 3). The other sites, consisting of plinthic Gleysols and albic, acric and pisoplinthic Plinthosols, have surface soil averages of approximately 20 t C ha–1 and 41% volumetric water contents. We observe a significant correlation (R2 = 0.9) between surface SOC and surface volumetric water content (Figure 3). The correlation is likely an indication of the texture differences in the soils; with the Gleysols generally consisting of more clay than the Plinthosols, and therefore the ability to hold more water. Similar relationships between soil water content and texture have been evidenced in previous studies (Abrol et al., 1968). Further, the wetter soils occurred at topographical depressions within the landscape where it is likely to accumulate as compared to a slope or hill, therefore topography is also likely to play a role in the greater water content of the Gleysols.
Figure 3. SOC stock at (A) 0–6 cm, (B) total SOC stock of each soil profile (C) average vol. water content for the whole profile of each soil (D) correlation between SOC stock and whole soil profile volumetric water content. SG AP, Savannah Grassland Albic Plinthosol; SW AP, Savannah Woodland Albic Plinthosol; FI HG, Forest Island Humic Gleysol; ST AP, Savannah Tree Albic Plinthosol; SS PG, Savannah Swamp Plinthic Gleysol; T PG, Transition zone Plinthic Gleysol; SF HG, Swamp Forest Humic Gleysol; PF PP, Pisoplinthic Forest Pisoplinthic Plinthosol; MF ACP, Mixed Forest Acric Plinthosol.
Most soils show a decrease in SOC with depth (SG, SS, FI, T, and SF), however some soils show increases in the deepest horizons (SW, ST, and MF). This is most likely due to greater horizon thickness in the deepest horizons, as well as slight increases in bulk density observed in most horizons with depth. In most cases, however, TOC concentration decreases with profile depth.
Thermally assisted hydrolysis and methylation in the presence of TMAH of solvent-extracted powdered soil causes SOM degradation to give a series of oxygenated aromatic products dominated by the methylated guaiacyl (G) and syringyl (S) lignin phenols from soils across the study transect. Figure 4 and Table 2 give the TMAH thermochemolysis products from all of the soils.
Table 2. Compound codes, names, retention times (RT) and characteristic fragment ions (m/z) of the thermochemolysis products detected across the surface soil samples on the study transect.
Figure 4. Partial chromatogram for the total ion current (TIC) of thermochemolysis products from a sample of savannah wood surface soil (methylated lignin and tannin phenols). IS, internal standard. Compound key in Table 2. Full figures for each lignin parameter calculated (Λ, C/G, S/G, [Ad/Al]G and [Ad/Al]S) are given in Supplementary Table 1. Values are given for both 13C-labeled TMAH corrected parameters (indicated with a *) and uncorrected values (without a *).
The distribution of the thermochemolysis products from a savannah woodland soil demonstrate that the most prominent products included G1 (1,2-dimethoxybenzene), G2 (3,4-dimethoxytoluene), G4 (3,4-dimethoxybenzaldehyde), G6 (3,4-dimethoxybenzoic acid methyl ester), S1 (trimethoxybenzene), S6 (3,4,5-benzoic acid methyl ester), P18 (trans 3-(-4-methoxyphenyl)-3-propenoic acid methyl ester), G18 (trans 3-(3,4-dimethoxyphenyl)-3-propanoic acid methyl ester) and the C16:0 fatty acid methyl ester (C16:0 FAME) (Figure 4).
Lambda (Λ) shows the sum of the amounts of the most dominant lignin phenols (see Methods, THM with TMAH analysis). Summed vegetation and surface soil Λ show little variation between sub-environments (Figure 5). The most notable difference is in the surface soil of the Forest Island, with very low Λ (0.1 mg/100 mg OC) as only lignin phenols G6, S6 and G18 were detected in the THM products. The remainder of the main lignin phenols (G4, G5, G6, S4, S5, P18) have therefore been degraded in the soil, demonstrating their lability.
Figure 5. 13C-TMAH corrected Λ values (mg/100 mg OC) for surface soils, and summed inputs = fresh shoot/leaf litter and wood litter across the transect. S.E. bars of 3 replicates shown. SG AP, Savannah Grassland Albic Plinthosol; SW AP, Savannah Woodland Albic Plinthosol; FI HG, Forest Island Humic Gleysol; ST AP, Savannah Tree Albic Plinthosol; SS PG, Savannah Swamp Plinthic Gleysol; T PG, Transition zone Plinthic Gleysol; SF HG, Swamp Forest Humic Gleysol; PF PP, Pisoplinthic Forest Pisoplinthic Plinthosol; MF ACP, Mixed Forest Acric Plinthosol.
Further, lignin phenols (specifically G4 and S4) are also shown to be labile in soils of the Savannah Tree, Savannah Swamp, Swamp Forest and Mixed Forest, as the amounts of these phenols in the soils are lower compared to their respective vegetation inputs (see Supplementary Table 2). However, in the case of the Savannah Tree and Mixed Forest, the significant difference between vegetation and soils does not affect the overall Λ, as this remains similar.
Greatest Λ was seen in the surface soil of the Savannah Woodland site (2.4 mg/100 mg OC), due to high amounts of phenols G6 and G18 (1.1 and 0.6 mg/100 mg OC, respectively). In comparison, all other Λ values of surface soils across the transect were ≤ 1.6 mg/100 mg OC, with the majority between 0.6 and 0.7 mg/100 mg OC.
In the remaining sites (Savannah Tree, Transition zone, Mixed Forest and Pisoplinthic Forest) soil Λ is similar to the respective vegetation Λ, as phenols G6 and G18 are not significantly degraded. This shows that phenols in these soils are less labile, or phenolic replacement from vegetation is high. Further, the Savannah Grassland surface soil has a slightly greater Λ than vegetation inputs (a difference of 0.4 mg/100 mg OC), suggesting preservation of phenols G18 and G6.
Across the savannah-rainforest transect, the sub-environment surface soils differ in woody/non-woody (C/G) composition; a value above 1 is a non-woody signal and below that is a woody signal (Figure 6A). As anticipated, non-woody ratios are predominant in the Savannah Grassland. Non-woody ratios are also predominant in the Savannah Swamp, Transition zone and Mixed Forest areas. In the latter four sites, selective degradation of guaiacyl over cinnamyl is apparent. However, in Savannah Woodland, Savannah Tree, Forest Island, Swamp Forest and Pisoplinthic Forest soils the opposite trend is apparent, emphasizing selective cinnamyl degradation in surface soils. All wood litter and fresh shoot/leaf litter samples have ratios signaling woody and non-woody input respectively, as expected (Nierop and Filley, 2007).
Figure 6. Lignin parameters (A) C/G and (B) S/G of surface soils and vegetation inputs across the transect and oxidation extents of (C) [Ad/Al]G and (D) [Ad/Al]S of surface soils and vegetation inputs in sub-environments across the transect. S.E. bars of 3 replicates are shown. SG AP, Savannah Grassland Albic Plinthosol; SW AP, Savannah Woodland Albic Plinthosol; FI HG, Forest Island Humic Gleysol; ST AP, Savannah Tree Albic Plinthosol; SS PG, Savannah Swamp Plinthic Gleysol; T PG, Transition zone Plinthic Gleysol; SF HG, Swamp Forest Humic Gleysol; PF PP, Pisoplinthic Forest Pisoplinthic Plinthosol; MF ACP, Mixed Forest Acric Plinthosol.
For the S/G ratio, a value above 1 is predominantly a gymnosperm signal, and below that an angiosperm signal. Except for the Mixed Forest soil, all surface soil S/G values are lower than those for vegetation (<1) showing the loss of the angiosperm signals via degradation (Figure 6B). As described in the following section, the Forest Island and Swamp Forest soils show the greatest syringyl degradation.
Increasing [Ad/Al]G/S values indicate increasing oxidation, therefore, it is usually expected that these ratios are higher in soils than vegetation (Supplementary Table 1 and Figures 6C,D; Filley et al., 1999). Oxidation signifies the degradation of lignin phenols. Oxidation ratios [Ad/Al]G/S could not be calculated for the surface soil of the Forest Island, as both G4 and S4 were not detected, suggesting an advanced rate of degradation at this location. The Swamp Forest also has a very degraded soil signature – it has the highest syringyl oxidation level in both surface soil and leaf litter (14.1 and 4.79, respectively). Due to the greater bulk SOC stocks in the Gleysols of the Forest Island and Swamp Forest, it was not expected that lignin would be degraded to this extent. The Savannah Grassland and Pisoplinthic Forest have the lowest guaiacyl oxidation and the Mixed Forest the lowest syringyl oxidation. All other sub-environment surface soils have comparable oxidation levels.
Wood litter in the study area are less oxidized than soils, as expected, and generally have very low values indicating hardwood [Ad/Al]G: 0.52–0.92 and [Ad/Al]S: 0.17–0.75 (Nierop and Filley, 2007). Some surface soils show a similar degree of oxidation to the respective fresh shoot or leaf litter samples, possibly due to rapid polyphenol input from fresh grass shoots, for example the guaiacyl compounds in the soil of the Savannah Grassland (Nierop and Filley, 2007). Similar oxidation degrees between soils and vegetation may also occur due to a high degree of lignin degradation in decomposing leaf litter, such as in the Mixed Forest and Transition zone sites, e.g., [Ad/Al]G 2.31 and 2.24, and [Ad/Al]S 2.44 and 2.31 for soil and leaf litter respectively.
Figure 7 shows the amounts of intact lignin, demethylated lignin and gallic acid in syringyl phenol S6 in wood litter (a), fresh shoots/leaf litter (b) and surface soils (c) across the sampling transect. This syringyl phenol showed the strongest alteration after correction with 13C-labeled TMAH.
Figure 7. Intact lignin, demethylated lignin and gallic acid amounts across the sampling transect (SG-MF) in (A) wood litter, (B) fresh shoot/leaf litter, and (C) surface soils. S.E. bars of 3 replicates shown. SG AP, Savannah Grassland Albic Plinthosol; SW AP, Savannah Woodland Albic Plinthosol; FI HG, Forest Island Humic Gleysol; ST AP, Savannah Tree Albic Plinthosol; SS PG, Savannah Swamp Plinthic Gleysol; T PG, Transition zone Plinthic Gleysol; SF HG, Swamp Forest Humic Gleysol; PF PP, Pisoplinthic Forest Pisoplinthic Plinthosol; MF ACP, Mixed Forest Acric Plinthosol.
It is evident that gallic acid is most abundant in the Savannah Woodland and Tree areas; vegetation input averages are 2.6 mg/100 mg OC and 2.16 mg/100 mg OC respectively, and surface soil averages are 1.48 mg/100 mg OC and 0.47 mg/100 mg OC, respectively (Figures 7A–C). The other sub-environments mostly show much lower amounts (<0.27 mg/100 mg OC). Hättenschwiler and Jørgensen (2010) demonstrated that different plant species can have high variation in foliage and leaf litter chemistry within the same rainforest ecosystem, thus, the differences observed here between sub-environments may be expected.
Our quantitative estimates of SOC stocks (Table 1 and Figures 3A,B) along the transect studied align with measurements from other regions of savannah-rainforest mosaics (Batjes and Dijkshoorn, 1999; Schwartz and Namri, 2002), confirming the small-scale natural heterogeneity of soils along vegetation gradients. For example, Plinthosol average surface soil (0–6 cm) SOC stock is significantly lower than that of the Gleysol average; 9 t C ha–1 compared to 31 t C ha–1. Full soil profile averages of Plinthosols are also lower than those of Gleysols (82 t C ha–1 compared to 227 t C ha–1). This difference in SOC stocks is consistent with surface and full profile Gleysol and Plinthosol SOC stock estimates reported for the Brazilian Amazon (Batjes and Dijkshoorn, 1999), although the scale of carbon burial differs significantly between regions.
For example, when comparing our Gleysol estimates of 128 t C ha–1 at 0–30 cm depth to Batjes and Dijkshoorn’s (1999) study on Amazon Gleysols, they are almost double their average of 70 t C ha–1 (0–30 cm). However, comparing further afield to the Congolese basin, Schwartz and Namri (2002) estimate greater surface (0–10 cm) SOC stocks of 74–109 t C ha–1 in ‘swamp forest’ hydromorphic soils and peat than we present here for Swamp Forest and Forest Island humic Gleysols at an average of 59 t C ha–1 (0–10 cm). Despite these differences, Plinthosols in this study average 38 t C ha–1 at 0–30 cm depth, consistent with previous estimates of 40 t C ha–1 (Batjes and Dijkshoorn, 1999). Schwartz and Namri (2002) show that similar Ferralitic savannah soils could have greater stocks; ranging between 15–30 t C ha–1 at 0–10 cm depth in their Congolese study region. Together these studies from different locations emphasize the broad range and diversity of soil carbon stocks at the small scale. Each study therefore represents one facet of a larger and not yet well constrained carbon puzzle that characterizes forest-savannah boundaries.
Soil organic carbon variation across different soil and vegetation types has been explained in various studies by a textural and hydromorphic effect, where high water content aligns with high SOC stocks through the anoxic conditions and subsequent preservation of carbon (Schwartz and Namri, 2002; Abbott et al., 2013). SOC variation across the transect in Guyana supports this general relationship as humic Gleysols have both the highest SOC stock and volumetric water content. The plinthic Gleysol under Savannah Swamp shows a relatively strong hydromorphic effect on both surface and full soil profile SOC, which are both higher than the Plinthosols. The hydromorphic effect on the plinthic Gleysol in the Transition zone is harder to document, with the surface SOC stock being greater than in the Plinthosol, yet the volumetric water content being similar. The same challenge applies to albic Plinthosols; Savannah Woodland and Savannah Tree soils have higher volumetric water contents than Savannah Grassland, but their surface SOC stocks are similar. As stated in the methods, water content was only measured at one point in time, and therefore an annual average may make this relationship clearer, but was beyond the scope of the study. However, the relationship indicates that texture is likely to be an important factor, as demonstrated by the Gleysols which have a predominantly clay texture likely contributing to greater water content, and Plinthosols which have a predominant texture of sand, and are thus drier. Previous research has also evidenced a relationship between texture and water content (Abrol et al., 1968). Therefore, predominantly clayey soils with greater water contents (swamp savannah and forest sites) show the hydromorphic effect more clearly than those with lower water contents (transition zone and savannah sites). Topography also likely influences water content, as Gleysols were found in depressions within the landscape, as previously noted.
The direct vegetation effect on SOC can be assessed between Savannah Grassland and Savannah with trees, as both contain sandy soils, which are otherwise absent in the forest environments studied. Comparing the two, it may be that the Savannah Woodland and Tree sites have higher vegetation inputs and therefore greater SOC stocks than the Savannah Grassland. However, grasses can have a significant input of SOC through their roots, therefore it is possible that SOC stocks between these different savannah sub-environments may be similar (Lal, 2004; Stahl et al., 2016). The SOC results from the study transect do show that carbon stocks are similar at the surface. We therefore conclude that the vegetation effect in savannah environments is small, at least on the transect analyzed, supporting previous studies in the Congo by Schwartz and Namri (2002). Abiotic factors such as the predominant texture of sand is thus likely to be more influential than vegetation; contributing to the low SOC stocks of these savannah environments through both the poor ability of carbon to be protected and the effect of leaching of carbon easily through sand particles after the wet season flooding.
The textural, hydromorphic and topographical effects on soil carbon carries down from the bulk SOC to the molecular carbon scale. This is evidenced by the complete loss of guaiacyl G4 and syringyl S4 in the Forest Island Gleysol, as well as the highest [Ad/Al]S values in both surface soils and leaf litter of the Swamp Forest site (see Figure 8). Further, the Forest Island has the lowest total lignin phenols (Λ) on the study transect (see Figure 5). The Swamp Forest also has a relatively low Λ compared to other soils on the transect (high lignin oxidation in [Ad/Al]G&S values also result in decreases in Λ values). This high molecular degradation was not initially anticipated due to the high water content and organic matter-rich nature of the soils observed when sampling.
Figure 8. Comparison of lignin phenol degradation ratios [Ad/Al]G&S and Λ against surface 0–6 cm SOC stocks along the study transect, illustrated by a schematic of vegetation types. Forest Island [Ad/Al]G&S and Pisoplinthic Forest SOC stock not determined (n.d.) SG AP, Savannah Grassland Albic Plinthosol; SW AP, Savannah Woodland Albic Plinthosol; FI HG, Forest Island Humic Gleysol; ST AP, Savannah Tree Albic Plinthosol; SS PG, Savannah Swamp Plinthic Gleysol; T PG, Transition zone Plinthic Gleysol; SF HG, Swamp Forest Humic Gleysol; PF PP, Pisoplinthic Forest Pisoplinthic Plinthosol; MF ACP, Mixed Forest Acric Plinthosol.
Figure 8 below demonstrates the difference between SOC stocks and phenolic degradation ([Ad/Al]G&S) and lignin phenol sum (Λ). Where SOC stocks are highest, the degradation extent of lignin guaiacyl and syringyl is high. This is particularly evident with [Ad/Al]S in the Swamp Forest Gleysol and the lack of phenols in the Forest Island Gleysol. Similarly, Savannah Woodland and Savannah Tree plinthosols show decoupling through the reverse trend – low SOC stocks and high amounts of lignin phenols, as well as high tannin phenol amounts (see Figure 7). SOC stocks from Savannah soils through to the Transition soil increase slightly, which is tracked by phenolic degradation. These then decouple again at the Swamp Forest. The decoupling between the degradation of lignin and the SOC stocks contradicts the initial hypothesis stated, that SOM degradation is inversely associated with SOC stock.
Such high degradation of lignin phenols in the Forest Island and Swamp Forest is likely due to the physio-chemical environment and biota surrounding the lignin, which allows for degradation processes to occur, for example the clay texture leading to a greater soil moisture, allowing them to be mineralized by microbes (see Figure 6). The extent of its breakdown suggests that it may be older carbon, although radiocarbon dates would be needed to verify this. The greater clay content of these rainforest soils may hold onto moisture for a longer period than the sandier soils, potentially favoring microbial communities to be active in degrading lignin for more extended periods of time. As previously noted, the volumetric water content was measured from the soil samples collected and therefore only represent one point in time. However, this variable is likely to fluctuate temporally on a seasonal and longer time scale, which will impact microbial activity and rates of organic matter decomposition. Indeed, Bovolo et al. (2012) evidence the seasonality of rainfall more widely across Guyana, showing the two wet and dry seasons of the rainforest, and one wet and dry season of the savannah. In this study it was not possible to assess annual variation in soils and how such seasonality therefore affects SOC, however the effect of weather patterns on soils has been noted by other studies. For example, annual variation in precipitation and short-scale rain events after and between dry periods may break open physically protected OM and activate microbial populations, allowing them to release phenol oxidase for decomposition (Austin et al., 2004; Fenner and Freeman, 2011; Abbott et al., 2013; Schellekens et al., 2015a,b,c). Therefore, lignin which has been previously protected in soil aggregates may become exposed to degradation through wet-dry cycles.
Further, fine-grained soils can protect organic carbon to a greater extent than coarse-grains, as organic matter turnover is governed by accessibility not recalcitrance (Dungait et al., 2012). Incorporation into clay minerals and formation of organo-mineral complexes with the iron oxides present in the soils from underlying plinthic material (Figure 2) may therefore play a role in protecting the SOC. However, significant incorporation into clay minerals is unlikely given that the X-ray diffraction results show that quartz and kaolinite constitute the mineralogy of all the soils sampled along the transect (see Supplementary Figures 1, 2 for examples of a savannah and rainforest soil mineralogy). The presence of the ferric iron phosphate mineral strengite detected in the soils also suggests a low ability for organic matter to complex, as this mineral is known to be stable at low pH in soil (Lindsay, 1979; Haynes, 1982). Conversely, iron oxy-hydroxides (such as hematite) were not detected, which may have otherwise induced more significant organo-mineral complexing (Gu et al., 1994; Sollins et al., 1996; Arnarson and Keil, 2000; Lützow et al., 2006). Therefore, it is likely that the moisture retentive properties of the clay textured soils and their topographical position play a more important role in the degradation of lignin.
The Forest Island lies surrounded by savannah and has much more exposed soil than, for example, the Swamp Forest. Patches of Forest Island on the boundary may therefore undergo more pronounced dehydration during the dry season as well as drying out faster following wet periods, allowing decomposition for a longer portion of the year. This exposure may explain the more significant phenolic depletion in comparison to the Swamp Forest soil, resulting in greater carbon degradation during both the dry and the wet season.
A previous study evidences leaching of dissolved organic matter and humic substances, including lignin, from forest soils into river waters during rainfall events close to the study site at Iwokrama in Central Guyana (Pereira et al., 2014). Leaching is therefore more than likely to account for some forest soil lignin decomposition. This may be the case particularly at Mixed and Pisoplinthic Forest sites, where lignin oxidation is apparent and the water content and SOC stock of the former site is low.
As the transect grades from forest to savannah, the high level of guaiacyl degradation (see Figure 6) in plinthic Gleysols in the Savannah Swamp can also be explained by a texture and hydromorphic effect. The clay texture of this soil means that it retains a higher level of moisture than Plinthosols in other areas of savannah and rainforest. It is likely that this degraded lignin signature is part of old carbon which has built up to an extent, as it also has a higher SOC stock than other savannah soils.
Unique on the study transect, the Savanah Woodland and Savannah Tree soils have significantly high gallic acid contents, which are reflected in the molecular content of the wood and leaf litter inputs. This is likely further influenced by the relatively low soil moisture which may constrain microbial mineralization. The high gallic acid contents, along with high Λ contents, do not have a significant effect on these SOC stocks however, which are some of the lowest on the transect.
The Savannah Grassland acric Plinthosol has a similar Ad/Al oxidation level between the soil and fresh grass shoots, which indicates fresh lignin inputs here (Nierop and Filley, 2007). This results in a similar Λ to the majority of soils on the transect. Grass shoot Λ is significantly lower than other vegetation inputs on the transect however, which constrains the SOC stock. It is likely that grass roots also input significant amounts of carbon into the soil (Stahl et al., 2016), however the low SOC stock suggests that this is lost due to abiotic or biotic factors. Reinforcing this, after the wet season which causes flooding, it is likely that carbon will be leached from the soil profile as the coarse grains of the sandy soil allows for drainage once heavy rains subside. Leaching of molecular compounds and bulk SOC is likely to have a stronger effect on the sandier soils of the savannah compared to those with greater clay contents. Under the exposed short grassland cover, soil may also undergo degradation of lignin phenols via photooxidation, resulting in lower SOC stocks (Hernes and Benner, 2003; Gallo et al., 2006; Frouz et al., 2011). The soil water content for Savannah Grassland, at the time of sampling in the dry season, was 27%, therefore indicating that some microbial mineralization of carbon may also be possible; although this will likely be more constrained than soils with a greater water content (Austin et al., 2004).
This study has revealed that the texture of soils and the water content related to this creates different physio-chemical environments and systems of carbon degradation. In Gleysols, high SOC contents and degraded lignin phenols indicate that carbon may persist and build up over time, being slowly degraded, whereas in Plinthosols, low SOC contents may indicate that carbon does not persist to the same extent. Here, the less degraded lignin phenols indicate fresh lignin inputs (Nierop and Filley, 2007).
As has been discussed above, the various sub-environments along this continuum bear features and mixtures of both carbon cycling systems, which will respond to land use or climate change through altered carbon inputs and soil moisture levels. Climate predictions and El Niño Southern Oscillation (ENSO) effects suggest a shift to higher temperatures (an annual average increase between 0.5–3°C) across northern South America (IPCC, 2013, 2014). More localized models of the Guiana Shield region (partly covering northern Brazil, southern Venezuela, eastern Colombia and the whole of Guyana, Suriname and French Guiana) also indicate increased temperatures (∼2°C) and variable changes in precipitation. These changes likely give rise to longer dry seasons, heavier rainfall events in wet seasons but also increased run off and evapotranspiration rates (Bovolo et al., 2018). Whilst the latter study provides predictions on a more regional scale than the IPCC, there may be further localized differences on and surrounding the transect itself, therefore there are limitations to applying these predictions to our data. In such a scenario, rainforest-dieback is likely to occur, causing a gradual transition to more widespread savannah landscapes and a low carbon input system. Lal (2005), Cramer et al. (2004), Don et al. (2011), and Bonini et al. (2018) have shown that when climate change or forestry activity causes this principal shift in vegetation, SOC reduces. Our study provides evidence that relics of previous Swamp Forests are able to remain as Forest Islands with high SOC stocks, likely due to their resistance, stemming from their position in topographical depressions, clayey and therefore wetter soils. However, the presence of highly degraded lignin phenols in these Forest Island soils reveal their fragility to change if perturbed any further. We therefore consider that the carbon pool of these Forest Islands surrounded by savannah is likely to become more decomposed under warmer and drier conditions. The Savanah Swamp and Transition zone Gleysols illustrate that even in topographical depressions with wetter soils than other savannah sites, SOC stock can be significantly lower; therefore, if the Forest Island degrades to similar vegetation, it may lose some of its SOC stock. The magnitude of a decrease in SOC stocks is difficult to gauge based on the data and due to the yet unknown potential change in soil water content and full understanding of soil carbon dynamics. We therefore suggest further investigation into such dynamics to estimate the potential losses of SOC on this transect.
We find that parent material, topography, texture and hydrological conditions at each sub-environment are very influential upon SOC stock. Clay-textured soils with the highest moisture content (Gleysols) have the highest SOC stocks, particularly those under forest vegetation in depressions (swamp and island forests). The Savannah swamp Gleysol has a substantial carbon stock but it is significantly lower than those of the Swamp Forest and Forest Island. Plinthosols have significantly lower SOC stocks than Gleysols, regardless of savannah or rainforest vegetation. Therefore, soil type is a more accurate indicator of SOC stock than vegetation type.
Analysis of the SOM characteristics in the surface soils of the sub-environments reveals clear differences in molecular composition. The Savannah Woodland Plinthosol has the greatest amount of lignin and tannin products and thus most unaltered plant derived material (although some degradation is apparent). Savannah Grassland and Mixed Forest Plinthosols have lower amounts of lignin and tannin products, although the type of phenols that are present indicate that they are fresher inputs. Despite Forest Island and Swamp Forest Gleysols having the highest SOC stocks, these soils have some of the most degraded lignin and tannin amounts across the transect. The original hypothesis is therefore contradicted by our results, in that SOM degradation is inversely related to SOC stock. The results suggest that the physio-chemical environment of the soil surrounding the lignin phenols dictates their degradation extent: in Gleysols lignin degrades slowly over time, as bulk carbon stocks accumulate; whereas in Plinthosols, we observe fresher inputs of lignin phenols and lower SOC stocks.
Upon deforestation due to land use or climate change, as predicted for northern South America and the Guianas more regionally, the high lignin oxidation and thus molecular carbon degradation of Gleysols indicates a vulnerability to savannah encroachment. Forest Islands isolated from the main forest biome are the most vulnerable to change, and could lose some of their SOC stocks. In order to better elucidate the potential loss of SOC and further understand the soil molecular dynamics, we suggest areas for further exploration below.
This study has set the framework for further investigations into soil carbon dynamics on the rainforest-savannah boundary in Guyana, by assessing both bulk and molecular soil organic carbon. The results have highlighted important areas for future study in order to better understand the dynamics of SOC, which is of significance regarding climate change. We therefore suggest that this consists of:
1. Temporal studies of soil moisture, bulk and molecular SOC in order to better elucidate the relationship between these variables.
2. Radiocarbon dating of SOC in order to clarify its age and better understand turnover dynamics.
3. Analysis of lignin with depth in the soil profiles to gain further information on its fate in soil.
4. Analysis of the soil microbial communities to explore their effect on SOC dynamics.
The raw data supporting the conclusions of this article will be made available by the authors, without undue reservation.
JB planned and carried out the field work, laboratory work, data analysis, and wrote the manuscript. The research plan was conceived by JB, GA, and TW. GA and TW supervised the project and gave suggestions for the final manuscript. All authors contributed to the article and approved the submitted version.
This is a Natural Environment Research Council (NERC) funded research project, with additional funding from the Royal Society Wolfson Research Merit Award. Funding has also been received from UKRI in order to publish this paper as open access.
The authors declare that the research was conducted in the absence of any commercial or financial relationships that could be construed as a potential conflict of interest.
The authors thank the staff at Iwokrama Research Centre, Guyana, and indigenous communities surrounding Wowetta for assistance in the field, B. Bowler and P. Donohoe for assistance with GC–MS analyses as well as C. Jeans for the preparation of the Figures. Thanks also to the Natural Environment Research Council (NERC), the Royal Society Wolfson Research Merit Award held by Tom Wagner and UKRI for funding the project and open access publishing.
The Supplementary Material for this article can be found online at: https://www.frontiersin.org/articles/10.3389/ffgc.2021.629600/full#supplementary-material
Abbott, G. D., Swain, E. Y., Muhammad, A. B., Allton, K., Belyea, L. R., Laing, C. G., et al. (2013). Effect of water-table fluctuations on the degradation of Sphagnum phenols in surficial peats. Geochim. Cosmochim. Acta 106, 177–191.
Abrol, I. P., Khosla, B. K., and Bhumbla, D. R. (1968). Relationship of texture to some important soil moisture constants. Geoderma 2, 33–39. doi: 10.1016/0016-7061(68)90004-9
Angst, G., Mueller, K. E., Nierop, K. G. J., and Simpson, M. J. (2021). Plant - or microbial - derived? A review on the molecular composition of stabilized soil organic matter. Soil Biol. Biochem. 156:108189. doi: 10.1016/j.soilbio.2021.108189
Antai, S. P., and Crawford, D. L. (1981). Degradation of Softwood, Hardwood, and Grass Lignocelluloses by Two Streptomyces Strains. Appl. Environ. Microbiol. 42, 78–380.
Arnarson, T. S., and Keil, R. G. (2000). Mechanisms of pore water organic matter adsorption to montmorillonite. Mar. Chem. 71, 309–320. doi: 10.1016/S0304-4203(00)00059-1
Austin, A., Yahdjian, L., Stark, J., Belnap, J., Porporato, A., Norton, U., et al. (2004). Water pulses and biogeochemical cycles in arid and semiarid ecosystems. Oecologia 141, 221–235.
Bahri, H., Dignac, M. F., Rumpel, C., Rasse, D. P., Chenu, C., and Mariotti, A. (2006). Lignin turnover kinetics in an agricultural soil is monomer specific. Soil Biol. Biochem. 38, 1977–1988.
Bala Krishna Prasad, M., and Ramanathan, A. L. (2009). Organic matter characterization in a tropical estuarine-mangrove ecosystem of India: Preliminary assessment by using stable isotopes and lignin phenols. Estuar. Coast. Shelf Sci. 84, 617–624. doi: 10.1016/j.ecss.2009.07.029
Batjes, N. H., and Dijkshoorn, J. A. (1999). Carbon and nitrogen stocks in the soils of the Amazon Region. Geoderma 89, 273–286.
Boerjan, W., Ralph, J., and Baucher, M. (2003). Lignin Biosynthesis. Annu. Rev. Plant Biol. 54, 519–546.
Bonini, I., Hur Marimon-Junior, B., Matricardi, E., Phillips, O., Petter, F., Oliveira, B., et al. (2018). Collapse of ecosystem carbon stocks due to forest conversion to soybean plantations at the Amazon-Cerrado transition. For. Ecol. Manage. 414, 64–73. doi: 10.1016/j.foreco.2018.01.038
Bouma, J., and Van Lanen, H. (1987). Transfer functions and threshold values: from soil characteristics to land qualities. Wageningen: wageningen university.
Bovolo, C. I., Pereira, R., Parkin, G., Kilsby, C., and Wagner, T. (2012). Fine-scale regional climate patterns in the Guianas, tropical South America, based on observations and reanalysis data. Int. J. Climatol. 32, 1665–1689.
Bovolo, C. I., Wagner, T., Parkin, G., Hein-Griggs, D., Pereira, R., and Jones, R. (2018). The Guiana Shield rainforests-overlooked guardians of South American climate. Environ. Res. Lett. 13:aacf60. doi: 10.1088/1748-9326/aacf60
Cannell, M. G. R., and Milne, R. (1995). Carbon pools and sequestration in forest ecosystems in Britain. Forestry 68, 361–378. doi: 10.1093/forestry/68.4.361
Carr, A. S., Boom, A., Chase, B. M., Meadows, M. E., Roberts, Z. E., Britton, M. N., et al. (2013). Biome-scale characterisation and differentiation of semi-arid and arid zone soil organic matter compositions using pyrolysis-GC/MS analysis. Geoderma 200–201, 189–201.
Cramer, W., Bondeau, A., Schaphoff, S., Lucht, W., Smith, B., and Sitch, S. (2004). Tropical forests and the global carbon cycle: Impacts of atmospheric carbon dioxide, climate change and rate of deforestation. Philosop. Transact. R. Soc. B Biol. Sci. 359, 331–343. doi: 10.1098/rstb.2003.1428
Crawford, D. L., Pometto, A. L., and Crawford, R. L. (1983). Lignin Degradation by Streptomyces viridosporus: Isolation and Characterization of a New Polymeric Lignin Degradation Intermediate. Appl. Environ. Microbiol. 45, 898–904.
Daniel, J. R. K., and Hons, B. A. (1984). Geomorphology of Guyana, an integrated study of natural environments. Guyana: University of Guyana.
DeAngelis, K. M., Allgaier, M., Chavarria, Y., Fortney, J. L., Hugenholtz, P., Simmons, B., et al. (2011). Characterization of Trapped Lignin-Degrading Microbes in Tropical Forest Soil. PLoS One 6:e19306. doi: 10.1371/journal.pone.0019306
Dignac, M. F., Bahri, H., Rumpel, C., Rasse, D. P., Bardoux, G., Balesdent, J., et al. (2005). Carbon-13 natural abundance as a tool to study the dynamics of lignin monomers in soil: an appraisal at the Closeaux experimental field (France). Geoderma 128, 3–17.
Don, A., Schumacher, J., and Freibauer, A. (2011). Impact of tropical land-use change on soil organic carbon stocks – a meta-analysis. Glob. Change Biol. 17, 1658–1670. doi: 10.1111/j.1365-2486.2010.02336.x
Dungait, J. A. J., Hopkins, D. W., Gregory, A. S., and Whitmore, A. P. (2012). Soil organic matter turnover is governed by accessibility not recalcitrance. Glob. Change Biol. 18, 1781–1796.
Eden, J. M. (1964). The Savanna Ecosystem - Northern Rupununi. British Guiana. Montreal: McGill University.
Fenner, N., and Freeman, C. (2011). Drought-induced carbon loss in peatlands. Nat. Geosci. 4, 895–900.
Filley, T. R., Minard, R. D., and Hatcher, P. G. (1999). Tetramethylammonium hydroxide (TMAH) thermochemolysis: proposed mechanisms based upon the application of 13C-labeled TMAH to a synthetic model lignin dimer. Organic Geochem. 30, 607–621.
Filley, T. R., Nierop, K. G. J., and Wang, Y. (2006). The contribution of polyhydroxyl aromatic compounds to tetramethylammonium hydroxide lignin-based proxies. Organic Geochem. 37, 711–727.
Foster, R. C. (1988). Microenvironments of soil microorganisms. Biol. Fertil. Soils 6, 189–203. doi: 10.1007/BF00260816
Frouz, J., Cajthaml, T., and Mudrák, O. (2011). The effect of lignin photodegradation on decomposability of Calamagrostis epigeios grass litter. Biodegradation 22, 1247–1254.
Gallo, M. E., Sinsabaugh, R. L., and Cabaniss, S. E. (2006). The role of ultraviolet radiation in litter decomposition in arid ecosystems. Appl. Soil Ecol. 34, 82–91.
Gleixner, G., Poirier, N., Bol, R., and Balesdent, J. (2002). Molecular dynamics of organic matter in a cultivated soil. Organic Geochem. 33, 357–366.
Godden, B., Ball, A. S., Helvenstein, P., McCarthy, A. J., and Penninckx, M. J. (1992). Towards elucidation of the lignin degradation pathway in actinomycetes. J. Gene. Microbiol. 138, 2441–2448.
Gold, M. H., Wariishi, H., and Valli, K. (1989). Extracellular peroxidases involved in lignin degradation by the white rot: Basidiomycete Phanerochate chrysosporium. ACS Sympos. Ser. 389:ch009.
Gu, B., Schmitt, J., Chen, Z., Liang, L., and McCarthy, J. F. (1994). Adsorption and Desorption of Natural Organic Matter on Iron Oxide: Mechanisms and Models. Environ. Sci. Technol. 28, 38–46. doi: 10.1021/es00050a007
Guggenberger, G., Zech, W., and Thomas, R. J. (1995). Lignin and carbohydrate alteration in particle-size separates of an oxisol under tropical pastures following native savanna. Soil Biol. Biochem. 27, 1629–1638.
Hatakka, A. (1994). Lignin-modifying enzymes from selected white-rot fungi: production and role from in lignin degradation. FEMS Microbiol. Rev. 13, 125–135.
Hättenschwiler, S., and Jørgensen, H. B. (2010). Carbon quality rather than stoichiometry controls litter decomposition in a tropical rain forest. J. Ecol. 98, 754–763. doi: 10.1111/j.1365-2745.2010.01671.x
Haynes, R. J. (1982). Effects of liming on phosphate availability in acid soils - A critical review. Plant Soil 68, 289–308. doi: 10.1007/BF02197935
Heim, A., and Schmidt, M. W. I. (2007). Lignin turnover in arable soil and grassland analysed with two different labelling approaches. Eur. J. Soil Sci. 58, 599–608.
Hernes, P. J., and Benner, R. (2003). Photochemical and microbial degradation of dissolved lignin phenols: Implications for the fate of terrigenous dissolved organic matter in marine environments. J. Geophys. Oceans 108, 1–7.
Hernes, P. J., Robinson, A. C., and Aufdenkampe, A. K. (2007). Fractionation of lignin during leaching and sorption and implications for organic matter “freshness.”. Geophys. Res. Lett. 34:2007GL031017.
IPCC (2013). “Atlas of Global and Regional Climate Projections Supplementary Material RCP4,” in Climate Change The Physical Science Basis Contribution of Working to the Fifth Assessment Report of the Intergovernmental Panel on Climate Change: Vols. 2013 SRC- (Annex, I Group, I), (Geneva: IPCC).
IPCC (2014). “Summary for Policymakers,” in Climate Change Impacts Adaptation and Vulnerability Global and Sectoral Aspects Contribution of Working to the Fifth Assessment Report of the Intergovernmental Panel on Climate Change p pp 5 and 11: Vols. 2014 SRC- (Part, A Group, I I), (Geneva: IPCC).
IUSS Working Group WRB (2015). IUSS World Reference Base for Soil Resources 2014 (updated in 2015). International soil classification system for naming soils and creating legends for soil maps. World Soil Resources Reports No. 106. Qinghai: IUSS Working Group WRB.
Kalbitz, K., Kaiser, K., Bargholz, J., and Dardenne, P. (2006). Lignin degradation controls the production of dissolved organic matter in decomposing foliar litter. Eur. J. Soil Sci. 57, 504–516.
Kiem, R., and Kögel-Knabner, I. (2003). Contribution of lignin and polysaccharides to the refractory carbon pool in C-depleted arable soils. Soil Biol. Biochem. 35, 101–118.
Klotzbücher, T., Filley, T. R., Kaiser, K., and Kalbitz, K. (2011). A study of lignin degradation in leaf and needle litter using 13Clabelled tetramethylammonium hydroxide TMAH thermochemolysis Comparison with CuO oxidation and van Soest methods. Organic Geochem. 42, 1271–1278.
Lal, R. (2004). Soil carbon sequestration to mitigate climate change. Geoderma 123, 1–22. doi: 10.1016/j.geoderma.2004.01.032
Lal, R. (2005). Forest soils and carbon sequestration. For. Ecol. Manage. 220, 242–258. doi: 10.1016/J.FORECO.2005.08.015
Lloyd, J., and Taylor, J. A. (1994). On the Temperature Dependence of Soil Respiration. Funct. Ecol. 8:315. doi: 10.2307/2389824
Lützow, M. V., Kögel-Knabner, I., Ekschmitt, K., Matzner, E., Guggenberger, G., Marschner, B., et al. (2006). Stabilization of organic matter in temperate soils: Mechanisms and their relevance under different soil conditions - A review. Eur. J. Soil Sci. 57, 426–445. doi: 10.1111/j.1365-2389.2006.00809.x
Marschner, B., Brodowski, S., Dreves, A., Gleixner, G., Gude, A., Grootes, P. M., et al. (2008). How relevant is recalcitrance for the stabilization of organic matter in soils? J. Plant Nutrit. Soil Sci. 171, 91–110.
Mason, S. L., Filley, T. R., and Abbott, G. D. (2009). The effect of afforestation on the soil organic carbon (SOC) of a peaty gley soil using on-line thermally assisted hydrolysis and methylation (THM) in the presence of 13C-labelled tetramethylammonium hydroxide (TMAH). J. Analyt. Appl. Pyroly. 85, 417–425.
McSweeney, C., New, M., and Lizcano, G. (2010). UNDP Climate change country profiles-Guyana. Oxford: University of Oxford.
Nierop, K. G. J., and Filley, T. R. (2007). Assessment of lignin and (poly-)phenol transformations in oak (Quercus robur) dominated soils by 13C-TMAH thermochemolysis. Organ. Geochem. 38, 551–565.
Nierop, K. G. J., Naafs, D. F. W., and Verstraten, J. M. (2003). Occurrence and distribution of ester-bound lipids in Dutch coastal dune soils along a pH gradient. Organ. Geochem. 34, 719–729. doi: 10.1016/S0146-6380(03)00042-1
Olk, D. C., Dancel, M. C., Moscoso, E., Jimenez, R. R., and Dayrit, F. M. (2002). Accumulation of lignin residues in organic matter fractions of lowland rice soils: a pyrolysis-gc-ms study. Soil Sci. 167, 590–606.
Palo, M. (1994). “Population and deforestation,” in The Causes of Tropical, eds K. Brown and D. W. Pearce (London: UCl Press).
Pereira, R., Bovolo, C., Spencer, R., Hernes, P., Tipping, E., Vieth-Hillebrand, A., et al. (2014). Mobilization of optically invisible dissolved organic matter in response to rainstorm events in a tropical forest headwater river. Geophys. Res. Lett. 41, 1202–1208.
Robertson, S. A., Mason, S. L., Hack, E., and Abbott, G. D. (2008). A comparison of lignin oxidation, enzymatic activity and fungal growth during white-rot decay of wheat straw. Organ. Geochem. 39, 945–951.
Rumpel, C., González-Pérez, J., Bardoux, G., Largeau, C., Gonzalez-Vila, F. J., and Valentin, C. (2007). Composition and reactivity of morphologically distinct charred materials left after slashandburn practices in agricultural tropical soils. Organ. Geochem. 38, 911–920.
Schellekens, J., Bindler, R., Martínez-Cortizas, A., McClymont, E. L., Abbott, G. D., Biester, H., et al. (2015a). Preferential degradation of polyphenols from Sphagnum - 4-Isopropenylphenol as a proxy for past hydrological conditions in Sphagnum-dominated peat. Geochim. Cosmochim. Acta 150, 74–89. doi: 10.1016/j.gca.2014.12.003
Schellekens, J., Bradley, J. A., Kuyper, T. W., Fraga, I., Pontevedra-Pombal, X., Vidal-Torrado, P., et al. (2015b). The use of plant-specific pyrolysis products as biomarkers in peat deposits. Quatern. Sci. Rev. 123, 254–264. doi: 10.1016/j.quascirev.2015.06.028
Schellekens, J., Buurman, P., Kuyper, T. W., Abbott, G. D., Pontevedra-Pombal, X., and Martínez-Cortizas, A. (2015c). Influence of source vegetation and redox conditions on lignin-based decomposition proxies in graminoid-dominated ombrotrophic peat (Penido Vello, NW Spain). Geoderma 237, 270–282. doi: 10.1016/j.geoderma.2014.09.012
Schwartz, D., and Namri, M. (2002). Mapping the total organic carbon in the soils of the Congo. Glob. Planet. Change 33, 77–93. doi: 10.1016/S0921-8181(02)00063-2
Sinha, N. K. P. (1968). Geomorphic Evolution of the Northern Rupununi Basin, Guyana. Montreal: McGill University.
Sollins, P., Homann, P., and Caldwell, B. A. (1996). Stabilization and destabilization of soil organic matter: Mechanisms and controls. Geoderma 74, 65–105. doi: 10.1016/S0016-7061(96)00036-5
Sørensen, H. (1962). Decomposition of Lignin by Soil Bacteria and Complex Formation between Autoxidized Lignin and Organic Nitrogen Compounds. J. Gene. Microbiol. 27, 21–34.
Spencer, R. G. M., Hernes, P. J., Ruf, R., Baker, A., Dyda, R. Y., Stubbins, A., et al. (2010). Temporal controls on dissolved organic matter and lignin biogeochemistry in a pristine tropical river, Democratic Republic of Congo. J. Geophys. Res. Biogeosci. 115:2009JG001180. doi: 10.1029/2009JG001180
Stahl, C., Freycon, V., Fontaine, S., Dezécache, C., Ponchant, L., Picon-Cochard, C., et al. (2016). Soil carbon stocks after conversion of Amazonian tropical forest to grazed pasture: importance of deep soil layers. Region. Environ. Change 16, 2059–2069. doi: 10.1007/s10113-016-0936-0
Stewart, C., Neff, J., Amatangelo, K., and Vitousek, P. (2011). Vegetation Effects on Soil Organic Matter Chemistry of Aggregate Fractions in a Hawaiian Forest. Ecosystems 14, 382–397.
Swain, E. Y., Perks, M. P., Vanguelova, E. I., and Abbott, G. D. (2010). Carbon stocks and phenolic distributions in peaty gley soils afforested with Sitka spruce (Picea sitchensis)’. Organ. Geochem. 41, 1022–1025.
ter Steege, H. (2000). Legend to the vegetation map of Guyana. Guyana: National Vegetation map of Guyana.
Thevenot, M., Dignac, M. F., and Rumpel, C. (2010). Fate of lignins in soils: A review. Soil Biol. Biochem. 42, 1200–1211.
Trojanowski, J. (2001). Biological degradation of lignin. Int. Biodeteriorat. Biodegrad. 48, 213–218.
Van Bergen, P. F., Bull, I. D., Poulton, P. R., and Evershed, R. P. (1997). Organic geochemical studies of soils from the Rothamsted classical experiments - I. Total lipid extracts, solvent insoluble residues and humic acids from broadbalk wilderness. Organ. Geochem. 26, 117–135. doi: 10.1016/S0146-6380(96)00134-9
Vane, C. H., and Abbott, G. D. (1999). Proxies for land plant biomass: Closed system pyrolysis of some methoxyphenols. Organ. Geochem. 30, 1535–1541.
Keywords: lignin, tannin, thermochemolysis, 13C-labeled TMAH, soil organic carbon, savannah-rainforest boundary, Amazon, climate change
Citation: Black JE, Wagner T and Abbott GD (2021) Assessing Lignin Decomposition and Soil Organic Carbon Contents Across a Tropical Savannah-Rainforest Boundary in Guyana. Front. For. Glob. Change 4:629600. doi: 10.3389/ffgc.2021.629600
Received: 15 November 2020; Accepted: 14 April 2021;
Published: 24 June 2021.
Edited by:
Delphine Derrien, INRA Centre Nancy-Lorraine, FranceReviewed by:
Ingo Schöning, Max Planck Institute for Biogeochemistry, GermanyCopyright © 2021 Black, Wagner and Abbott. This is an open-access article distributed under the terms of the Creative Commons Attribution License (CC BY). The use, distribution or reproduction in other forums is permitted, provided the original author(s) and the copyright owner(s) are credited and that the original publication in this journal is cited, in accordance with accepted academic practice. No use, distribution or reproduction is permitted which does not comply with these terms.
*Correspondence: Jasmine E. Black, amFzbWluZS5lLmJsYWNrMUBnbWFpbC5jb20=
Disclaimer: All claims expressed in this article are solely those of the authors and do not necessarily represent those of their affiliated organizations, or those of the publisher, the editors and the reviewers. Any product that may be evaluated in this article or claim that may be made by its manufacturer is not guaranteed or endorsed by the publisher.
Research integrity at Frontiers
Learn more about the work of our research integrity team to safeguard the quality of each article we publish.