- 1Environmental Studies and Sciences Program, Skidmore College, Saratoga Springs, NY, United States
- 2Department of Biology and School of Environment, McGill University, Montreal, QC, Canada
- 3Lancaster Environment Centre, Lancaster University, Lancaster, United Kingdom
- 4Departamento de Oceanografia, Universidade Federal do Espírito Santo, Vitória, Brazil
- 5Earth System Science Center (CCST), National Institute of Space Research Instituto Nacional de Pesquisas Espaciais (INEP), São José dos Campos, Brazil
- 6Remote Sensing Division (Divisão de Sensoriamento Remoto), National Institute for Space Research (Instituto Nacional de Pesquisas Espaciais), São José dos Campos, Brazil
- 7National Institute for Research in Amazonia (Instituto Nacional de Pesquisas da Amazônia), Manaus, Brazil
- 8The Nature Conservancy, Bogota, Colombia
- 9Asociación para la Conservación de la Cuenca Amazónica, Lima, Peru
- 10Department of Thematic Studies - Environmental Change, Linköping University, Linköping, Sweden
- 11Department of Environmental Science and Policy, George Mason University, Fairfax, VA, United States
- 12National Center for Monitoring and Early Warning of Natural Disasters (CEMADEN), Sáo Paulo, Brazil
- 13School of Environmental Sciences, University of East Anglia, Norwich, United Kingdom
- 14National Geographic Society, Washington, DC, United States
- 15Instituto Centro de Vida (ICV), Cuiabá, Brazil
- 16Coordenação Geral de Observação da Terra, Instituto Nacional de Pesquisas Espaciais (INPE), São Paulo, Brazil
- 17The Amazon Conservation Team – Suriname Program, Paramaribo, Suriname
- 18Department of Ecology, Universidade de Brasília, Brasília, Brazil
- 19Amazon Third Way Project, Universidade Estadual de Campinas, São Paulo, Brazil
- 20Bren School of Environmental Science and Management, University of California, Santa Barbara, Santa Barbara, CA, United States
- 21Coordenação de Zoologia, Museu Paraense Emílio Goeldi, Belém, Brazil
- 22The Nature Conservancy, Brasilia, Brazil
- 23Department of Environmental Sciences, UFRRJ, Federal Rural University of Rio de Janeiro, Institute of Forests, Seropédica, Brazil
- 24World Wide Fund For Nature (WWF), Brasil, Distrito Federal, Brazil
The Amazon Basin is at the center of an intensifying discourse about deforestation, land-use, and global change. To date, climate research in the Basin has overwhelmingly focused on the cycling and storage of carbon (C) and its implications for global climate. Missing, however, is a more comprehensive consideration of other significant biophysical climate feedbacks [i.e., CH4, N2O, black carbon, biogenic volatile organic compounds (BVOCs), aerosols, evapotranspiration, and albedo] and their dynamic responses to both localized (fire, land-use change, infrastructure development, and storms) and global (warming, drying, and some related to El Niño or to warming in the tropical Atlantic) changes. Here, we synthesize the current understanding of (1) sources and fluxes of all major forcing agents, (2) the demonstrated or expected impact of global and local changes on each agent, and (3) the nature, extent, and drivers of anthropogenic change in the Basin. We highlight the large uncertainty in flux magnitude and responses, and their corresponding direct and indirect effects on the regional and global climate system. Despite uncertainty in their responses to change, we conclude that current warming from non-CO2 agents (especially CH4 and N2O) in the Amazon Basin largely offsets—and most likely exceeds—the climate service provided by atmospheric CO2 uptake. We also find that the majority of anthropogenic impacts act to increase the radiative forcing potential of the Basin. Given the large contribution of less-recognized agents (e.g., Amazonian trees alone emit ~3.5% of all global CH4), a continuing focus on a single metric (i.e., C uptake and storage) is incompatible with genuine efforts to understand and manage the biogeochemistry of climate in a rapidly changing Amazon Basin.
Main
Amazonia is the largest swath of tropical rainforest on the planet. A region unto itself, the forest drives a partially self-sustaining regional climate and hydrological system believed to be at increasing risk of sudden collapse (Sampaio et al., 2007; Lovejoy and Nobre, 2018). Far from homogenous, Amazonia incorporates montane to mangrove forests across a range of distinct soils and substrates, integrated by a biogeochemically-diverse riverine network that drives extensive seasonal inundation (Hess et al., 2015). Human impacts across the basin are equally diverse and heterogeneous, reflecting the numerous distinct ecological, political, socioeconomic, and cultural units that fall within its boundaries (Figure 1).
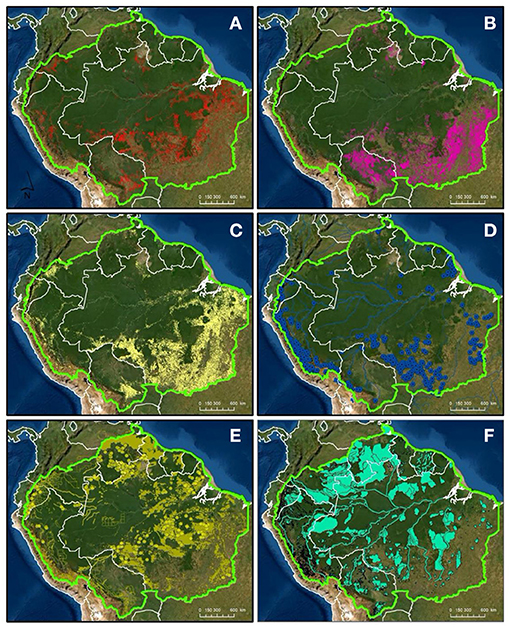
Figure 1. Social and economic drivers of land use in the Amazon: (A) forest loss 2001–2019 (Hansen et al., 2013) (red shading), (B) fires 2001–2019 (RAISG, 2020) (pink shading), (C) agricultural and cattle areas (MAPBIOMAS Version 2.0, 2020) (yellow shading), (D) hydropower and reservoirs (RAISG, 2020) (blue points), (E) oil extraction and mining areas (RAISG, 2020) (yellow shading and points), and (F) fishing and hunting areas (RAISG, 2020) (aqua shading).
After a transient period of reduced deforestation and increased optimism (Davidson et al., 2012), rising agricultural conversion and illegal logging activities are again accelerating Amazonian forest loss (Carvalho et al., 2019). This resurgence has renewed concerns that the region is rapidly approaching a catastrophic “tipping point” (Sampaio et al., 2007; Boers et al., 2017; Lovejoy and Nobre, 2018). Numerous studies catalog the rapid rate of deforestation and forest degradation, the accompanying release of CO2, and the net C balance of the region (e.g., Asner et al., 2012; Gatti et al., 2014; Brienen et al., 2015; Aragão et al., 2018; Bullock et al., 2020; Hubau et al., 2020). While they are the most often discussed, carbon dynamics are just one component of the Amazon Basin's manifold interactions with climate (Figures 2, 3; Supplementary Tables 1, 2). Biogeochemists working across the land-climate subfields widely acknowledge the importance of non-CO2 climate drivers and the need to integrate them in our widening understanding of forested ecosystems' role in regulating global climate. However, despite numerous calls to incorporate these less-recognized agents in forest climate accounting (Bonan, 2008; Jackson et al., 2008; Thompson et al., 2009; Schindler et al., 2020), few studies address the net effect of multiple synergistic or antagonistic climate interacting drivers simultaneously. Resolving the pattern, magnitude, and trade-offs between the full suite of forest–climate interactions, and their response to the nature and extent of anthropogenic change, is the central challenge limiting our understanding of the Amazon's global climate impact.
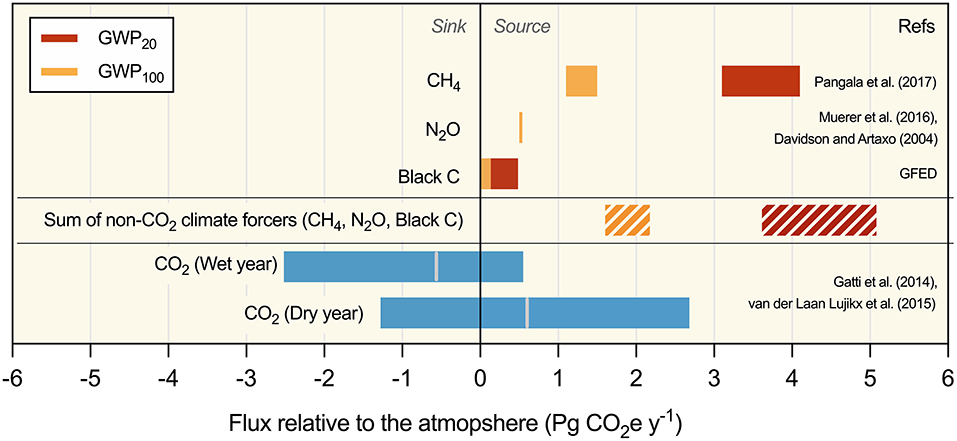
Figure 2. Summary of annual fluxes for primary climate-forcing agents in the Amazon Basin, in Pg CO2-equivalent at two global warming potential (GWP) time horizons (20 and 100 years). For CO2, gray lines indicate means, plus the minimum and maximum uncertainty of five flux estimates for 2010 (anomalously dry year) and 2011 (wet year) that integrate both ecosystem productivity and biomass burning. These are the only years for which large-scale, inversion-based atmospheric C balance measurements for the basin are available. For CH4 and black C, values are means ± 1 SD and for N2O values represent a range. GWP factors are from IPCC AR5 (Myhre et al., 2013, 5) (CH4, N2O) or Bond et al. (2013; black C). There are no Basin-wide estimates of the total annual emission and GWP factors for biogenic volatile organic compounds (BVOCs), aerosols, and ozone. See Supplementary Information for flux calculations.
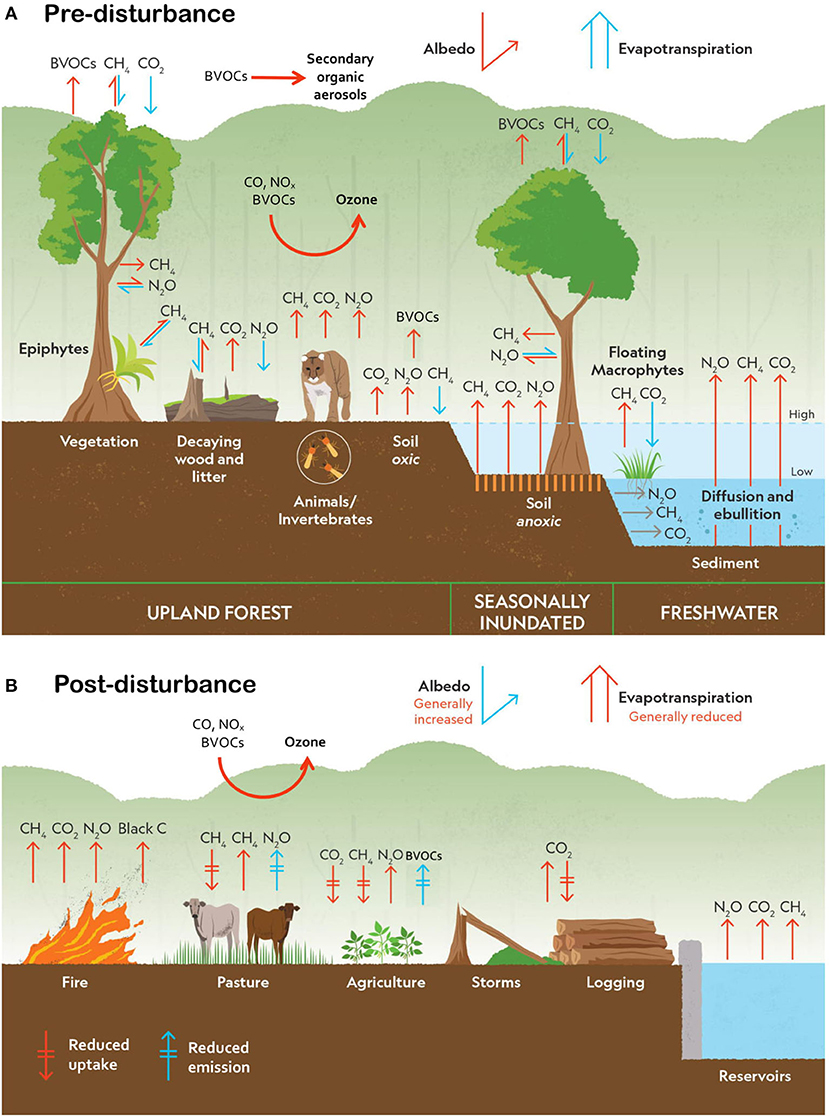
Figure 3. Local-scale (A) pre-disturbance and (B) post-disturbance fluxes, biophysical forcing agents, and their associated short-term radiative forcing impact (positive, red; negative, blue; hashed arrows indicate a reduction in—as opposed to directional change to—a flux following disturbance) from undisturbed upland forests, seasonally inundated forests and wetlands, and freshwater ecosystems of the Amazon Basin. Note that because the magnitude of many of these effects is not well-defined, the arrow length is not proportional to source/sink strength.
Defining a comprehensive radiative balance for the Amazon is a daunting task. Uncertainty in both the magnitude and direction of some forcing agents is high, and existing data across temporal and spatial scales cannot be readily synthesized. Although emission estimates for the major greenhouse gases are well-constrained, other important forcing agents either lack comprehensive Basin-wide emission estimates or have no broad consensus as to their CO2 equivalence [CO2e; e.g., biogenic volatile organic compounds (BVOCs] (Yáñez-Serrano et al., 2020). Further stifling is the reliance on commonly reported CO2e factors strongly dependent on arbitrary time horizons [e.g., 20- vs. 100-year global warming potentials (GWPs); GWPs for CH4 differ by a factor of ~3 (Myhre et al., 2013)]. Finally, because there is currently insufficient information to estimate the full impact of feedback processes (e.g., cloud cover or evapotranspiration response to coupled seasonal warming and drought; Marengo et al., 2018), current land use accounting is primarily focused on direct impacts (Supplementary Table 1). Droughts, related to El Niño (as in 1998 and 2016), or to warming in the tropical Atlantic (2005), or both (2010), impact the physiological response of Amazon forests, decreasing the availability to absorb atmospheric CO2, as well as biodiversity and increasing risk of fires (Marengo et al., 2018). In spite of these substantive challenges, emerging research focused on non-CO2 climate forcers allows us to begin the process of expanding our understanding beyond C. Here, we present a state-of-the-science synthesis of the inter-related climate effects of natural and anthropogenic change agents in the Amazon Basin and their persistent uncertainties with particular consideration given where the pattern and scale of the effect are large but not regularly considered alongside CO2.
Net Climate Forcing at the Basin Scale
Excellent work has been done detailing the biogeochemistry of region and its role in the global climate system (Davidson et al., 2012); however, despite a substantial increase in data and literature addressing various aspects of Amazon Basin's role in regulating global climate, a synthetic examination of the most recent Basin-wide emission estimates for the known climate forcing agents [specifically CO2, CH4, N2O, and black C (BC)] shows (1) high uncertainty in the magnitude of climate-relevant emissions from the Basin; (2) disagreement in the best way to account for their climate forcing relative to CO2 (e.g., choice of GWP factor; Bond et al., 2013), and (3) the critical role that non-CO2 climate forcing agents play in determining the Basin's impact on the global climate system (Figure 2). Even accounting for this large uncertainty, integrating the suite of forcing agents for which data is available leads to the conclusion that the current net biogeochemical effect of the Amazon Basin is most likely to warm the atmosphere; the CO2e from net C uptake is currently smaller than the combined CO2e from N2O, CH4, and BC emissions under most emission scenarios (Figure 2; Table 1; Supplementary Tables 1, 2). This assessment is conservative in that it ignores additional factors such as the indirect climate forcing of BC (Bond et al., 2013), negative radiative forcing from the reflectivity of biogenic aerosols, and the potentially significant but poorly constrained secondary effects of BVOC emissions (Shrivastava et al., 2017) (see Biogenic Volatile Organic Compounds and Black C sections). The only scenarios where the net biogeochemical impact of the basin provides a positive climate service (net uptake of ~0.5–1 Pg CO2e year−1) is when CO2 uptake is considered to be at the highest end of published annual estimates (measured under the most favorable climatic conditions), and the 100-year GWPs are used to calculate CO2e (Table 1). When 20-year GWP values are applied, the net emission is on the order of 1.3–8.2 Pg CO2e year−1; the ~7 Pg CO2e year−1 spread of values across scenarios indicates high uncertainty in these estimates, especially for CO2 (Table 1). Further, because the majority of regional and global anthropogenic impacts are expected to decrease C uptake and increase most non-CO2 forcing agents, we expect this source strength to grow (Table 2). Specific sources of uncertainty for each flux are described below.
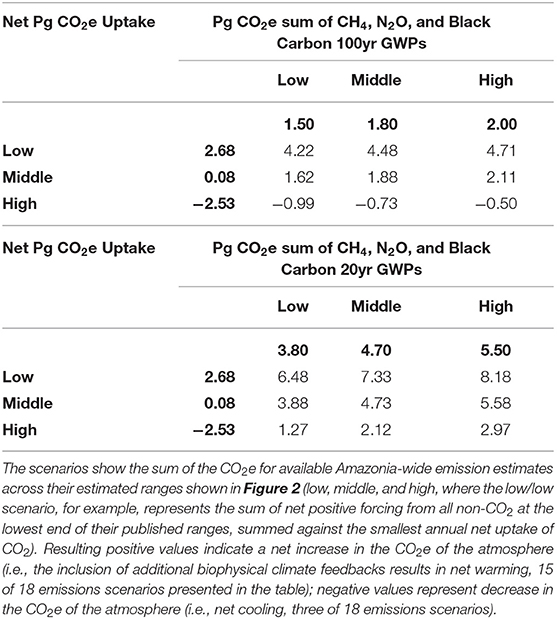
Table 1. Comparison of the published emissions ranges (see Figure 2) for CO2 and non-CO2 climate forcers and their net balance at two global warming potential (GWP) time horizons (20 and 100 years).
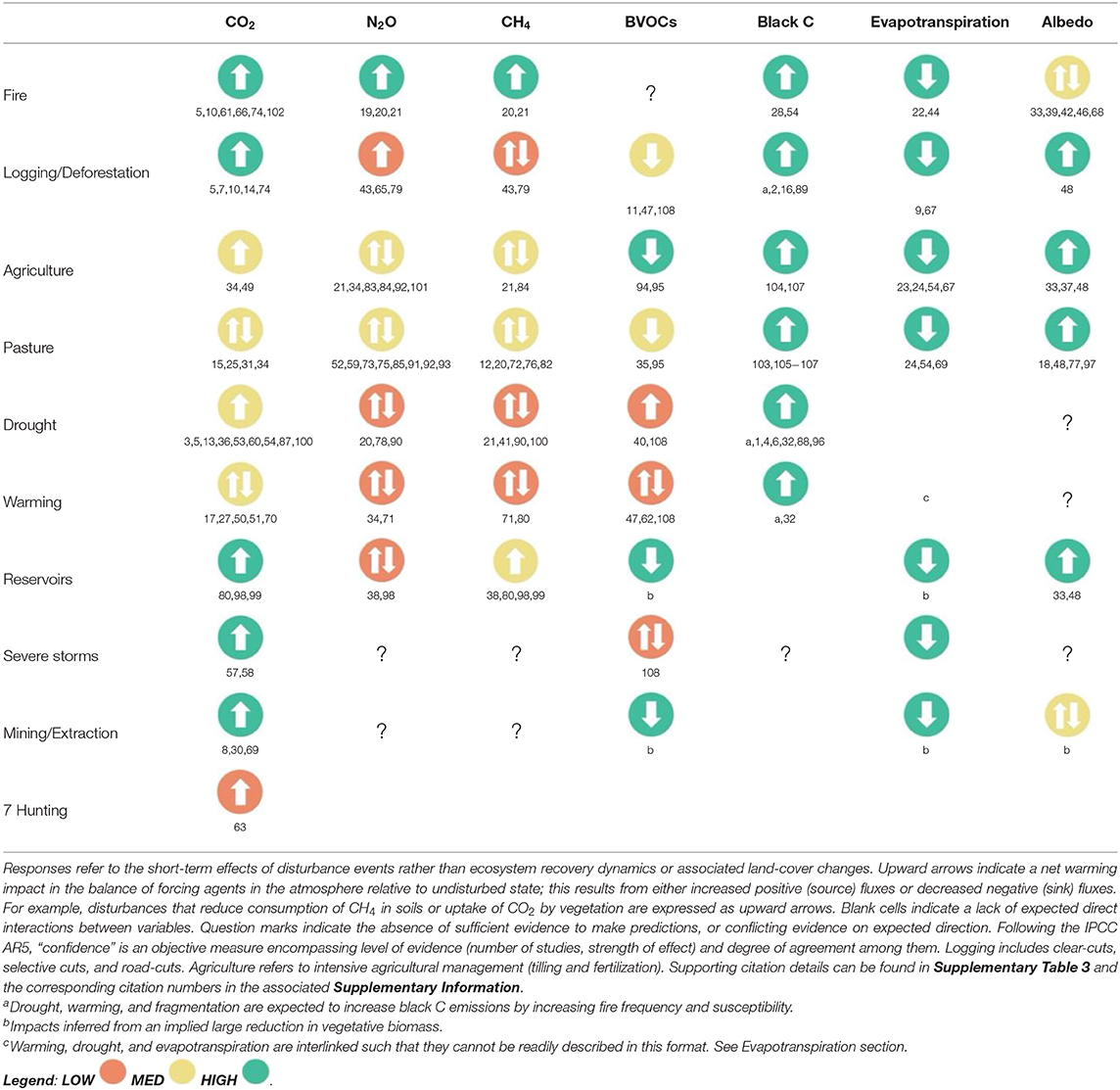
Table 2. Expected direction of response to disturbance for climate forcing agents, associated confidence, and relevant literature citations.
Individual Climate Forcers and Processes
Carbon Dioxide
Net CO2 exchange between Amazonia and the atmosphere reflects the balance of uptake by primary production and weathering, and loss via respiration, decomposition of plant residues in the soils and water, and biomass burning. Because of the size of the total pool (the Amazon rainforest is one of the largest ecosystem C pools on Earth, storing ~150–200 Pg C; Feldpausch et al., 2012), even proportionally small changes in uptake or loss represent large net changes in CO2 exchange with the atmosphere. The largest driver of CO2 emissions from Amazonia is land-use change and forest degradation. When both clearance and regeneration are considered over the 20-year period from 1996 to 2017, these losses amounted to between 4.86 and 5.32 Pg C (Bullock and Woodcock, 2020). A great deal of work has focused on quantifying CO2 fluxes and the relative contribution of different drivers (Supplementary Table 1). However, the best available atmospheric C balance measurements (the gold standard method for net flux estimation) for the basin cover only a 2-year period (Figure 2) and are sensitive to climate and biomass-burning effects, which have strong interannual variability (Pan et al., 2011). Between 1990 and 2007, the basin appeared to be acting as a strong vegetation C sink as CO2 with an estimated uptake of 0.42–0.65 Pg C year−1 (Pan et al., 2011); however, recent work suggests that the magnitude of the C sink has been consistently declining (Brienen et al., 2015; Aragão et al., 2018). Land conversion, drought, and sustained long-term tree mortality (Aleixo I. et al., 2019) have driven a 1/3 reduction in aboveground biomass in the past decade as compared with the 1990s (Brienen et al., 2015; Walker et al., 2020). Aircraft-based air column measurements suggest that the basin as a whole actually lost 0.48 ± 0.18 Pg C year−1 during the drier year of 2010 and was C neutral during the wetter year in 2011 (0.06 ± 0.10 Pg C year−1). Survey data indicate a pronounced response to drought and a long-term average vegetation uptake of just 0.39 ± 0.10 Pg C year−1, far lower than over the preceding decades (Gatti et al., 2014). More concerning still is that from 2010 to 2017, the basin appears to have acted as net source of 0.4 ± 0.2 Pg C year−1, or 0.2 ± 0.2 Pg C year−1 when extreme drought years are excluded from analysis (Gatti et al., 2019), but the results are sensitive to the area considered, and small drought impacts are noted over a more inclusive study area (van der Laan-Luijkx et al., 2015). Indeed, these regional-scale drought events can reduce biomass gains even in areas without observable precipitation deficits (Feldpausch et al., 2016). Taken together, the body of research investigating C flux dynamics in the Amazon Basin provides compelling evidence that one of the earth's largest C sinks is in steep decline and at increasing risk of becoming a regular source of C to the atmosphere.
Virtually all identified anthropogenic changes have increased or are expected to increase CO2 emissions from the Basin; the lone exception is warming, where net impact will depend on the relative magnitude of increases in photosynthesis vs. respiration, as well as the magnitude and variability of associated hydrological changes (Table 2). Major short-term sources include combustion and deforestation associated with conversion to agriculture, extraction industries, or dams. Though fires lit directly for deforestation purposes have historically been the major driver of fire-related emissions, fires carrying through drought-impacted forests are increasingly dominant and expected to increase (Aragão et al., 2018). Longer-term committed (unavoidable) emissions come from current drought- or storm-associated tree mortality, subsequent decay and fire, and the degradation of soil C stocks following land-use change (Table 2). Likewise, this suite of near- and long-term change agents drives more diffuse forest degradation, further reducing future C sink strength (Rappaport et al., 2018).
The soil C pool is particularly vulnerable to loss catalyzed by land-use and climate change, which may also impact soil biodiversity with potential for myriad other downstream biogeochemical effects (Smith et al., 2015). The largest proportion of soil C is stored in the top 50 cm and decreases geographically along a gradient of soil age and evolution from the western to the eastern Amazon (Quesada et al., 2010). Converting forests to high-intensity grazing/introduced pasture, and eventually degraded pasture, decreases soil organic matter storage in the top 30 cm by ~20% with most C either respired as CO2 or eroded to waterways (Fonte et al., 2014; Navarrete et al., 2016). Warmer soil temperatures associated with global change are expected to accelerate soil microbial respiration rates, further increasing C loss (Cusack et al., 2018), though there is some evidence that microbial acclimation may attenuate these temperature-dependent emissions increases (Crowther and Bradford, 2013). Observations from primary forest in central Amazonia suggest a loss of 2.98 Mg C ha−1 (an average of 0.11 Mg C ha−1 year−1) from the top 20 cm of soil between ~1984 and 2012 (Barros and Fearnside, 2019), attributed potentially to warming.
Amazonian aquatic environments are also an important conduit transporting, storing, and processing C between land and the ocean (Davidson et al., 2012; Ward et al., 2017). Along the way, they transform and release terrestrial and aquatic organic matter as CO2 (Raymond et al., 2013). Regional emissions assessments initially concluded outgassing from rivers and wetland represents an annual source of 470 Tg C year−1 (Richey et al., 2002). However, many recent studies have increased the spatial and temporal coverage of measurements to include streams, small rivers, lakes, floodplains in different tributaries and the lower section of the Amazon River (de Fátima F. L. Rasera et al., 2013; Sawakuchi et al., 2017). This expanded assessment increased CO2 emission estimates four-fold to 1.8 Pg C year−1 (Melack, 2016). Carbon and associated nutrients released into the Atlantic Ocean by the Amazon river plume result in a substantial C sink, both from direct sediment deposition and from stimulation of photosynthesis and subsequent associated sediment C deposition (Subramaniam et al., 2008). However, tidal floodplains and areas offshore and along the coastline where freshwater remains unmixed with ocean water could also represent significant sources of CH4 and CO2 (Sawakuchi et al., 2017) and would effectively reduce magnitude of the C sink of the Amazon plume. Across the land to ocean continuum, CO2 (and also CH4) originated by microbial respiration in soil can be transported through groundwater to streams and higher-order rivers (Neu et al., 2011; Call et al., 2018), and labile organic C from riparian zones can also be used for in situ respiration (Richey et al., 2009). Although C originating in wetlands may sustain most of the evasion of CO2 from the Amazon River and its main tributaries (Abril et al., 2014), recent evidence also demonstrates that turnover of lignin and other terrestrially derived macromolecules in the Amazon River could fuel up to 50% of the river's bulk respiration, suggesting that a large fraction of these materials break down in rivers and are evaded as CO2 and do not reach the ocean as previously assumed (Ward et al., 2013). Degradation of these molecules can be intensified by a priming effect in areas where the river receives water from tributaries and wetlands that are rich in labile organic C, creating hotspots of C transformation (Ward et al., 2016).
Non-CO2 Forcers and Processes
Trace Gases
Amazonian terrestrial and freshwater systems support fluxes of a range of climatically significant trace gases, often switching between sink and source in response to pronounced seasonal shifts in inundation or anthropogenic disturbance (Table 2). N2O and CH4 are the best characterized and most significant of these, and it had been believed that for undisturbed ecosystems, the global warming impact of emitted N2O and CH4 (1.3 Pg CO2e year−1) was approximately balanced by that of CO2 taken up by vegetation (Aragão et al., 2014). However, more recent work in the basin suggests CH4 emissions from trees in wooded wetlands actually overwhelm their C sink effect (Pangala et al., 2017; Dalmagro et al., 2019). Upland soils and biomass burning also act as net sources of nitric oxide (NO) and nitrogen dioxide (NO2) to the atmosphere (Bustamante et al., 2009). While not greenhouse gases themselves, these compounds are involved in the formation of radiatively-active ozone and CH4 in the troposphere. A substantial amount of CO is emitted by Amazonian deforestation, particularly from smoldering combustion when the biomass is burned (Fearnside, 1997). This CO removes hydroxyl radicals (OH−) from the atmosphere, thereby extending the average lifetime of the CH4 that these radicals would otherwise remove (Saunois et al., 2019). CO is also converted to CO2, a contribution to global warming that increases the C impact of Amazon Basin deforestation by 2.4–2.8% (Fearnside, 2000). Effects of disturbance and climatic variability on ecosystem trace gas fluxes are mediated predominantly by shifts in soil temperature, moisture (including sediment/soil inundation), gas diffusivity, and nutrient cycling, including increased nutrient inputs (Bustamante et al., 2009).
N2O
Tropical forests are a globally important source of N2O, and Amazon forest emissions range from 1.1 to 6.9 kg N ha−1 year−1 (Abril et al., 2014), with total basin emission estimated at 1.8–1.9 Tg N2O-N year−1 (0.46–0.49 Pg CO2e) (Davidson and Artaxo, 2004). This small range reflects a lack of recent comprehensive synthesis of emissions, rather than high confidence, and does not include emissions from tree stems in upland habitats that have also been recently identified as an important egress pathway for soil-borne N2O (Dalmagro et al., 2019). Inversion models for South America constrain the upper limit of natural soil emissions at around 3 Tg, or 18% of global atmospheric emissions (Saikawa et al., 2013, 2014). While the majority of this source likely derives from microbial production in upland soils, N2O can also be emitted from drying wetland sediments, biomass burning, hydroelectric reservoirs, riverine water columns, and from more recently recognized sources including inundated tree stems, decaying wood, and invertebrate activity (Koschorreck, 2005; Hu et al., 2016; Covey and Megonigal, 2019; Soper et al., 2019).
Disturbances have varied effects on N2O production/consumption in soils and sediments, some of which have reasonable certainty in the direction of their effect, though not magnitude (Table 2, and references therein). Increased concentrations of N2O over the basin are confidently associated with biomass burning. Similarly, soil compaction associated with land clearing and logging increases both soil N2O and NO fluxes by between 30 and 350% (Keller et al., 2005; Meurer et al., 2016). N2O emission from pasture soil varies seasonally, with most emissions occurring during the rainy months (Luizão et al., 1989). After an initial pulse, however, conversion generally reduces long-term soil N2O and NO emissions as a result of declining N availability in aging pasture. Though fertilized and/or tilled agriculture is recognized as a large N2O source globally, several studies find only modest increases when compared with intact forest in Amazonia. Reservoir construction in the Amazon could also increase net N2O fluxes substantially—one study estimated an ~80% increase (Guérin et al., 2008)—but data are present from a just small number of sites, and pre-dam data are limited. Although there are few climate manipulation studies from the global tropics, those that do exist demonstrate that global change factors will have contrasting effects on N2O dynamics in soil. Throughfall exclusion experiments indicate that soil drying reduces N2O emissions in upland forests (Davidson et al., 2008), whereas warming is expected to increase soil enzyme activity, thus enhancing soil N2O emissions from humid tropical forests (Xu-Ri et al., 2012). As with other factors, drying and warming may also modulate N2O emissions by feeding back to fire frequency and severity (Davidson and Artaxo, 2004).
CH4
Soils and sediments can both produce and consume CH4. Well-drained upland soils in the Amazon act predominantly as a net CH4 sink, of 1–3 Tg CH4 year−1 (Bustamante et al., 2009), whereas inundated soils, sediments, and vegetation act as a globally significant net source (Covey and Megonigal, 2019; Saunois et al., 2019). Methane oxidation significantly reduces the diffusive emissions of CH4 from rivers and floodplains (Sawakuchi et al., 2016; Barbosa et al., 2018), and most of the CH4 emissions from these sources may therefore be attributed to bubbles released from wetland sediments that bypass oxidation. Ebullitive fluxes estimates, however, remain highly uncertain due to the restricted spatio-temporal coverage of measurements and the episodic nature of ebullition (Melack et al., 2004). Emissions from vegetation can be both direct (via UV-induced abiotic production) and indirect (as a conduit between sediments and the C atmosphere which bypasses oxidization in the water column, and through microbial activity inside living and deadwood). Abiotic fluxes are likely small (Covey and Megonigal, 2019), but the transport of soil-borne CH4 to the atmosphere by tree stems is the largest CH4 egress pathway in the Basin, contributing ~3.5% of all global CH4 emissions (Pangala et al., 2017; Saunois et al., 2019). Additional, but smaller, contributions come from native animals and livestock, invertebrates, and tank bromeliads (Bloom et al., 2010; Martinson et al., 2010).
Around 20% of the Amazon Basin is seasonally flooded, and recent developments suggest that previous bottom-up regional CH4 emission estimates from these areas are unreliable (Melack et al., 2004). Average fluxes of 195 ± 25 kg CH4 ha−1 year−1 over the eastern part of the Amazon Basin (~1 million km2) have been observed by vertical CH4 mole fraction profiles, likely dominated by seasonal and permanent wetlands (Basso et al., 2016). Extrapolation of inversion results to the whole of the Amazon Basin, using top-down regional estimates of CH4 emissions based on regularly measured atmospheric profiles at four locations, yields a mean total CH4 flux of 43 ± 6 Tg CH4 year−1, which is the equivalent of ~8% of all global CH4 emissions. This estimation agrees well with combinations of floodplain tree emissions (15 ± 2 to 21 ± 3 Tg CH4 year−1) and CH4 emission from other transport pathways, namely, conduit from flooded sediments to the atmosphere via tree stems (21 ± 5 Tg year−1) (Pangala et al., 2017). Hence, CH4 emissions in the basin are larger than previously estimated and contribute ~1.0–4.2 Pg CO2e (Table 1; Figure 1). The lack of integration of these newly recognized fluxes from living tree stems is a critical weakness, limiting our broader understanding of the biogeochemical impacts of Amazonian land conversion.
Of the impacts that may increase the strength of sources in the Basin, wetland warming and reservoir construction could be the most significant. While drying may reduce the area of wetlands and in turn the source strength (Davidson and Artaxo, 2004), recent projections suggest that a 4°C temperature increase in tropical South American wetlands could double already substantial regional CH4 emissions (Zhang et al., 2017). Inundation following dam construction decreases aquatic oxygen levels and increases anoxic organic matter decomposition, releasing significant amounts of CH4 to the atmosphere. This effect is potentially 10 times stronger in tropical systems than for better-studied temperate dams (Barros et al., 2011; Fearnside, 2015). Actual reservoir emissions will vary with the flooded area, river chemistry (which varies markedly across the basin; Ríos-Villamizar et al., 2013), and the extent of pre-clearing, but simulations for 18 planned reservoirs indicate net emissions between 9 and 21 Tg CH4 over the next 100 years (de Faria et al., 2015). Actual emissions may be substantially greater, however, due to downstream evasion associated with water passing through turbines and spillways (Fearnside, 2016).
In addition to direct CH4 production during biomass burning, logging and conversion to agriculture tend to compact soil and reduce the strength of the soil CH4 sink, sometimes shifting upland soils to net sources (Bustamante et al., 2009). Introducing ruminant livestock to the systems further increases source strength, accounting for ~6% of total landscape CH4 emissions in the eastern Basin (Basso et al., 2016). In intact forests by contrast, soil drying associated with climate change is likely to increase the CH4 sink strength, though the magnitude of the effect is uncertain and short-term drought impacts could in fact have the opposite effect (Davidson et al., 2008) (Table 2). Finally, fire also serves as a substantial source of direct emissions, ranging in magnitude from 0.5 to 7.0 Tg CH4 year−1, depending on the severity of the burn season (Saito et al., 2016; Wilson et al., 2016).
Biogenic Volatile Organic Compounds
BVOCs are atmospheric trace gases (e.g., isoprenoids and methanol) produced during plant processes such as growth and communication (Laothawornkitkul et al., 2009), response to heat stress (Peñuelas and Llusià, 2003), or by soils (Bourtsoukidis et al., 2018), litter, and fungi (Yáñez-Serrano et al., 2020). BVOC emissions impact atmospheric reactivity, acting as precursors for the formation and growth of aerosols. Other less-studied positive climate forcing feedbacks include greenhouse effects such as involvement in tropospheric ozone formation, lengthening of the atmospheric lifetime of CH4, increasing CO2 production, and the release of latent heat during water condensation (Peñuelas and Staudt, 2010). A key question, both globally and in the Amazon Basin, is whether increased BVOC emissions have a net cooling or net warming effect (Peñuelas and Llusià, 2003). While some authors have suggested that BVOC emissions are a dominant control on the net forcing balance of forest ecosystems globally (Unger, 2014), others conclude that including more holistic modeling of atmospheric interactions suggests only modest impacts (Scott et al., 2018). Globally, BVOC emissions are large but not well-constrained, with most recent estimates at ~760 Tg C year−1 (Sindelarova et al., 2014). Tropical forests are a significant contributor with emissions from some tropical locations exceeding 1,000 kg ha−1 year−1 (Guenther, 2002). Fluxes vary between Amazon ecoregions (Greenberg et al., 2004), but estimates suggest that 1–6% of net ecosystem productivity (C gain) can be re-emitted as BVOCs (Kuhn et al., 2007).
BVOC emissions are controlled by a diverse set of factors, including plant species and functional type, canopy structure, biotic factors like herbivory, and abiotic variables such as concentrations of other atmospheric gases, nutrient availability, and seasonal changes in temperature, precipitation and soil moisture, solar radiation, leaf area index, and leaf phenology (Peñuelas and Llusià, 2003; Laothawornkitkul et al., 2009; Yáñez-Serrano et al., 2020). Emissions are thus likely to be sensitive to change in both climate and land use in the Amazon Basin. A recent review by Yáñez-Serrano et al. (2020) summarizes these effects in detail. Satellite applications have increased the availability of emissions data and show that BVOCs have decreased by on average 2% per annum in recent years (Bauwens et al., 2016). Although there is significant uncertainty surrounding the impacts of global changes on BVOC production, with studies producing conflicting results, many scenarios suggest an overall increase in emissions (Peñuelas and Llusià, 2003). BVOC production generally increases with temperature, although once temperatures exceed a maximum, isoprene emissions decline sharply, especially in the tropics (Laothawornkitkul et al., 2009). Increases may also be associated with rising CO2 concentrations that stimulate plant productivity or increase N2O (Peñuelas and Llusià, 2003; Laothawornkitkul et al., 2009), but this has not been tested directly in the Amazon (Yáñez-Serrano et al., 2020). Responses to drought stress can be positive or mixed due to differences in leaf physiology, BVOC biochemistry, and study methods (Laothawornkitkul et al., 2009; Yáñez-Serrano et al., 2020). Estimates from the Amazon Cuieiras Biological Reserve show that maximum emissions rates from primary forests occur in the late dry season (Alves et al., 2016), supporting observations that increased production follows warming and drought (Jardine et al., 2015; Pfannerstill et al., 2018). Finally, land-use change that reduces vegetation cover alters emissions. For example, isoprene emission fluxes in Amazonia may decrease substantially due to deforestation or tree mortality (Ganzeveld and Lelieveld, 2004), or where trees are replaced by grasses with a lower emission potential (Peñuelas and Llusià, 2003). This has been shown to result in a net positive radiative forcing in some cases (Scott et al., 2018; IPCC, 2019). Critical current knowledge gaps include regional production rates, species-specific rates, emissions of non-isoprenoid compounds, and responses to factors such as increased photosynthetically active radiation (Yáñez-Serrano et al., 2020). Until the understanding of the basic drivers of emissions at the leaf scale can be applied to estimate regional emissions, and broad agreement on the net forcing impact of BVOC emissions is reached, the climate impact of Amazon BVOCs will remain poorly resolved.
Ozone
Tropospheric ozone (O3) is a highly reactive trace gas that plays a major role in atmospheric chemistry and acts as a greenhouse gas and an air pollutant, which has adverse effects on human health, crops, and vegetation (Cooper et al., 2014; Rowlinson et al., 2019). Due to its short life span of 23 days controlled by temperature, radiation, and precursor emissions from some natural but mostly anthropogenic sources, tropospheric O3's concentration is highly variable in space and time (Cooper et al., 2014). In the Amazon Basin, there is no significant direct source of tropospheric O3. Concentrations largely depend on trace gases such as NOx, CO, and VOCs (Trebs et al., 2012; Martins et al., 2018; Paralovo et al., 2019), and mirroring other interannual regional climate trends, variability in tropospheric ozone in the region is largely regulated by the El Niño-Southern Oscillation (ENSO) cycles (Rowlinson et al., 2019). Originating from biomass burning, urban, and biogenic emissions, these act as important sources of O3 precursors. Their conversion to O3 is regulated to some extent by the exchange between stratosphere and troposphere, as it is efficiently transported by intense convective activity to the upper troposphere, where regional and global circulations disperse them over long distances (Bela et al., 2015; Gerken et al., 2016). While regional smoke, haze plumes from biomass burning, and to some extent NOx from soil are important contributors in the dry-to-wet transition season, biogenic emission of VOCs, particularly from the Amazon rainforest, is deemed important in the wet-to-dry transition season (Bela et al., 2015).
With increased biomass burning, deforestation, high-intensity agricultural land conversion, and rapid urbanization in the Amazon, anthropogenic emissions of O3 precursors are on the rise (Artaxo et al., 2013; Bela et al., 2015), which has substantial impacts on the atmospheric radiation balance, can increase diffuse radiation and boost primary productivity (Sena et al., 2013), and can also reduce surface air quality (Artaxo et al., 2013). Additionally, due to dispersion of the urban anthropogenic plume of O3 precursors, urbanization has the potential to significantly alter chemical composition and surface–atmospheric exchange processes far away from the source especially over pristine Amazon forests where these emissions are relatively low (Trebs et al., 2012; Gerken et al., 2016; Martin et al., 2017). Urban environments may also have a larger impact on the upper troposphere due to high solar radiation and intense convective transport (Alonso et al., 2010). Intense drought as predicted by future climate change scenarios and rapid land-use change in the region will tend to increase O3 concentrations. The strongest sink of O3 is dry deposition, which can occur through stomatal and nonstomatal uptake processes predominantly at night in forest canopy, consequently impacting forest growth. Soil and water surfaces and chemical losses such as reactions with nitric oxide are also O3 sinks (Clifton et al., 2020). Conversion of Amazon rainforests to pastures or other agricultural land may therefore reduce the existing forest O3 sink.
Aerosols
Small particles suspended in the atmosphere ranging from a few nanometers to micrometers, known as aerosols, are another important component of the climate system. They absorb and scatter solar radiation and significantly influence precipitation; because they provide cloud condensation nuclei, aerosols constitute an essential ingredient for cloud formation and development (Shrivastava et al., 2019). Moreover, some particles, known as ice nuclei, can initiate the formation of ice crystals inside clouds, providing a faster growth to precipitable droplet sizes compared with cloud condensation nuclei (Pöschl et al., 2010). The biosphere emits aerosols, which are processed by photochemistry, providing nuclei for cloud formation. This results in precipitation stimulating the formation of aerosol particles, making an intrinsic connection between aerosol and cloud processes, thereby sustaining the hydrological cycle (Pöschl et al., 2010; Pöhlker et al., 2012). The addition of these cloud condensation nuclei, in turn, reduces atmospheric temperature by reflecting sunlight from low clouds (Peñuelas and Staudt, 2010) and can also increase NPP via increases in diffuse radiation (Yáñez-Serrano et al., 2020). A recent analysis suggested biogenic aerosols above the central Amazon accounted for a radiative surface forcing of −20 W m−2, just under half of the overall aerosol effect (Palácios et al., 2020).
Sources of aerosols from the Amazon Basin include both high emissions from biomass burning and low but more consistent production of primary and secondary biological aerosol particles and components (Martin et al., 2010). Primary particles are produced by flora (release of pollen and fungal spores, leaf and soil debris, suspended microbes, and fragments of biological material), and secondary aerosols are formed due to the atmospheric photochemical oxidation and condensation of trace gases including VOCs to low-volatile compounds depositing on preexisting particles or nucleating new particles. In the atmosphere, these particles undergo continuous transformation through photochemical reactions, interactions, and cloud processing; ultimately, these particles deposit on vegetation surfaces through dry deposition, cloud scavenging, and precipitation and advection, transporting these particles out of the Amazon Basin. Recent observations showing higher aerosol particle densities in the upper troposphere seem to indicate aerosol production there could be an important source to the boundary layer (Andreae et al., 2018). In addition to within-Basin sources, long-range transported natural and anthropogenic sources such as Atlantic marine aerosols, African aerosols consisting of Saharan dust, and biomass-burning aerosols from the Sahel region (Pöhlker et al., 2012; Moran-Zuloaga et al., 2018) are more frequent in the wet season (Sena et al., 2018) when enhanced concentrations of aerosol mass, crustal elements (Al, Si, Ti, and Fe) and potassium are observed, providing key nutrients to the Amazonian ecosystem (Martin et al., 2010; Pöhlker et al., 2012).
The Amazon Basin especially in the wet season is used as a laboratory to study pristine continental aerosol particles, which equates to pre-industrial values, against which the effects of human activities globally can be evaluated, including future analysis of anthropogenic influence on cloud formation and precipitation (Martin et al., 2010). With rapid urbanization, fire, deforestation, and land-use change, the region also provides a unique opportunity to investigate how chemical pathways of aerosol formation transition from preindustrial to urban influenced present-day condition (Davidson et al., 2012). Increased aerosol emissions due to these anthropogenic changes directly affect the radiative budget due to surface albedo changes and indirectly by acting as cloud condensation nuclei; changing cloud properties therefore have strong implications to convection, cloud formation, and ratio of direct to diffuse radiation and play a major role in modifying atmospheric composition (Sena et al., 2013). Land-use change from forests to crops to pasture also changes evaporation rates resulting in changes to atmospheric water vapor content and further enhances the effect of deforestation on the radiative balance (Sena et al., 2013). Furthermore, anthropogenic aerosol emissions enhance different pathways of secondary biological aerosol formation in the pristine forest (Shrivastava et al., 2019).
Black Carbon Aerosols
BC is particulate matter > 2.5 μm in diameter, which is formed mainly by incomplete combustion of fossil fuels, biofuels, and biomass, though the term is used to describe compounds with varying definitions. Though mean values are presented in Figure 2, estimates of GWP for BC vary by almost an order of magnitude and reflect the challenge of making direct comparisons with CO2. Around 40% of global open fire emissions come from forest burning in the tropics, and emissions of 0.33 Tg C year−1 of BC have been reported for Latin America. BC emissions from forest degradation and deforestation for South America averaged 0.14 ± 0.09 Tg C year−1 for the years 1997–2016 (Global Fire Emissions Database version 4 GFED4, 2020) (Figure 2). Although the Amazon is certainly a large contributor to this figure, BC is not included in Brazil's Nationally Determined Contribution to the Paris agreement (Azevedo-Ramos and Moutinho, 2018). Biomass-burning aerosols absorb radiation directly (warming the atmosphere) and reduce radiation reaching vegetation but also increase diffuse radiation and cause indirect climate feedbacks that have been shown to increase net primary productivity in the Amazon rainforest by 1.9–2.7% (65–100 Tg C year−1), and up to as much as 615 Tg C year−1 in an extreme fire year (Malavelle et al., 2019; Silva Junior et al., 2019). Other studies show that when including indirect interactions (such as impacts on cloud formation), net radiative forcing is dependent on emission rates of biomass-burning aerosols, having a modest cooling effect at low rates that switches to strong warming as atmospheric loading increases (Liu et al., 2020). As with other forcing agents described here, freshwater is an important factor in the transport and storage of BC. A significant amount of the total BC produced in the Amazon is temporarily stored in intermediate reservoirs along the freshwater continuum and eventually transported to the oceans, where it may potentially be sequestered (Coppola et al., 2018, 2019). Total fluxes of dissolved BC from the Amazon river have been estimated at 1.9–2.7 Tg year−1 (Coppola et al., 2019), most of it relatively modern in origin (Coppola et al., 2019). BC emissions also influence regional hydrology, catalyzing 3% of melting mass loss from nearby Andean glaciers (Magalhães et al., 2019). Widespread drought-intensified burning is likely to increase emissions in the future (Aragão et al., 2018).
Albedo
Albedo is a measure of diffuse reflection of incoming solar radiation. Conversion of forests to pastures or croplands tends to increase albedo, both because relatively dark forest land cover absorbs more radiation than more reflective open areas and as a result of aerosol formation owing to burning for clearance. Along the arc of deforestation, average albedo increases of 2.8% have been observed after land conversion (Loarie et al., 2010). Atmospheric aerosols can be both primary (e.g., BC) and secondary (e.g., secondary organic aerosols formed from BVOCs) and impact the albedo of the atmosphere both directly and via their impact on cloud formation, lifetime, and droplet size (increasing aerosols reduce droplet size but increase cloud cover acting to increase albedo) (IPCC, 2015). BC can reduce the albedo of surfaces after deposition (Ramanathan and Carmichael, 2008). Carbon dioxide equivalences of albedo changes are not standardized and are sensitive to the pattern, timing, and extent of underlying land-cover changes and the time horizon considered. Even considering this uncertainty, it is clear that unlike in higher-latitude forests (Bala et al., 2007), the local albedo-driven cooling effect of land clearance in Amazonia is far smaller than warming driven by increased C emissions and reduced evapotranspiration (Jackson et al., 2008).
Evapotranspiration
Evapotranspiration in the Amazon is a critical driver of local and regional climate, via surface cooling and water recycling, and an important control on vegetation pattern throughout the Basin (Salati et al., 1979; Sampaio et al., 2007; Boers et al., 2017; Lovejoy and Nobre, 2018). Regional changes in precipitation (detailed below) are mirrored by local-scale land-use-driven hydrological changes that in turn influence evapotranspiration. In general, anthropogenic changes that reduce vegetative biomass also reduce evapotranspiration and in turn intensify surface runoff, dry soils, alter moisture transport dynamics, and increase local temperatures (Hayhoe et al., 2011; Dias et al., 2015). The effect of deforestation on soil moisture appears as a minor control on evapotranspiration in the wet season but emerges as an important biophysical driver following the onset of the dry season (Mallick et al., 2016). This seasonal dynamic is complicated by the fact that transpiration is likely an important factor triggering and sustaining the onset of the wet season (Wright et al., 2017). Although secondary and primary forests may have similar rates of evapotranspiration (Sommer et al., 2002), in those portions of the basin with rapid land-use change, dry season reductions in total evapotranspiration are strongly associated with increased local air surface temperatures (Sampaio et al., 2007). The aggregate effect of these local changes can then feedback into regional climate pattern delaying the onset and duration of the dry season (Leite-Filho et al., 2020). Increased dry season lengths also lead to reduced growth in secondary forests, which are in turn more vulnerable to drought stress (Elias et al., 2020), and at a greater risk of fire. Throughfall reduction experiments in mature Amazonian forests suggest primary forests are similarly sensitive (Nepstad et al., 2002). Even modest deforestation-driven reductions in rainfall can reduce convective available potential energy and increase convective inhibition, decreasing latent heat flux and further drying the atmosphere. Initiating cyclical decline, the subsequent reduction in rainfall together with intense warming can then further increase forest dieback. A complex-network analysis of atmospheric water fluxes in the Amazon suggests this self-amplification effect might further reduce Amazonian forest cover by 10–13% (Zemp et al., 2017). A study using the ED-BRAMS (Ecosystem Dynamics 2- Brazilian Regional Atmospheric Modeling System) coupled land–atmosphere model showed that the effects of tree loss on atmospheric moisture and local temperature were greatest along the arc of deforestation. These large changes in modeled local parameters, however, did not translate to greatly reduced precipitation, suggesting significant gaps in our understanding of atmospheric dynamics over the Amazon and/or uncertainties in model estimates of precipitation (Swann et al., 2015).
Nature, Extent, and Underlying Drivers of Anthropogenic Change Agents
Contextualizing the region's changing biogeochemistry is predicated not only on understanding the current state of the biophysical system and its potential responses to change but also on developing an integrated understanding of the social and economic drivers of dynamic resurgent land-use change (Figure 1).
Local: Deforestation and Land-Use Change
Historically, the Brazilian “arc of deforestation” along the southern and southeastern edges of the forest (largely a product of soybean cropping and meat production) was the epicenter of deforestation in the Amazon Basin (Nepstad et al., 2014), while smaller-scale migration-related processes dominated the Andean zone (Steininger et al., 2001), and gold mining was most intense in the northern Basin (Dezécache et al., 2017). In recent years, however, other countries in the region, such as Peru and Bolivia, have also experienced large-scale deforestation. In the case of Peru, the palm oil industry, combined with pressure from artisanal gold mining in the south, has triggered rapid deforestation, whereas the Bolivian Amazon has been more heavily impacted by soybean production (Asner and Tupayachi, 2016; Kalamandeen et al., 2018).
Since 2017, the processes driving deforestation across the region are widely seen as more dynamic and uniquely sensitive to the political decisions of regional governments (Fearnside, 2017; Carvalho et al., 2019). With the signing of the peace agreement in Colombia, even nominally protected areas previously occupied by revolutionary FARC guerrillas are now threatened by cattle grazing (Prem et al., 2019). In the Brazilian Amazon, deforestation rates have increased >60% since 2012 (4,600 km2 year−1) with ~7,420 km2 year−1 lost between 2016 and 2018 (Aguiar et al., 2012). In 2019, the first year of the Bolsonaro presidential administration, 9,762 km2 was deforested, a 30% increase over the previous year (PRODES, 2020). The increasing forest losses are linked to national and state political actions that compromise indigenous land rights, limit monitoring and enforcement, and attempt to inhibit conservation nongovernmental organizations (Ferrante and Fearnside, 2019).
Local: Fire
Both intentionally-set fires associated with land clearing and, increasingly, indirect uncontrolled drought-associated fires occur throughout the Basin and are abundant at forest edges especially in southern Amazonia (Brando et al., 2020). After a general decline compared with the previous decade, the high rate of fire detection in 2019 was strongly linked to a sharp uptick in deforestation, characterized by the highest rates seen since 2008 (Barlow et al., 2020; Cardil et al., 2020). This year was also characterized by exceptionally high fire counts occurring in protected areas (Barlow et al., 2020) and associated with a shift in governance that strongly reduced environmental protections and enforcement (Escobar, 2020). Even in periods of slowed land conversion, gross emissions from fires that carry through drought-affected forests (ignited by human activities such as adjacent secondary slash-and-burn or pasture burning) can offset half the C gained from reduced deforestation (Aragão et al., 2018). Fire incidence in the Brazilian Amazon increased by 36% in the 2015 drought, increasing the total area impacted by all fires to ~800,000 km2 (Aragão et al., 2018). Climatic shifts predicted by Earth system models are likely to amplify fire susceptibility, such that drought-associated fires, a leading cause of forest degradation, may exceed those arising directly from deforestation (Aragão et al., 2014). Model projections suggest an intensification of the fire regime in the southern Amazon could double the burned area to 16% by 2050, but this impact could be reduced substantially by avoiding new deforestation (Brando et al., 2020). Recovery of basal diameter can occur following fire, but canopy openings and drying residual biomass facilitate future fires (Berenguer et al., 2014), and the result is often a stable transition in forest structure and composition, reduced biodiversity, and biomass loss (Davidson et al., 2012).
Local: Agriculture
Historically, deforestation in the Amazon has been dominated by cattle ranching (Richards et al., 2014; Fearnside, 2017), although the impact of soy production has steadily increased (Nepstad et al., 2014). The two practices are interconnected, however, as cattle ranchers in consolidated frontiers sell their land to soy producers and subsequently advance new ranching frontiers deeper in the Basin (Richards et al., 2014).
Land-management practices following deforestation are as important in determining C dynamics and CO2 emissions as land-cover changes (Berenguer et al., 2014; Luyssaert et al., 2014). The use of fire or machinery to remove the plant material after deforestation and prepare the soil (Marin-Spiotta et al., 2009), the introduction of exotic pasture and legume species (Mosquera et al., 2012), the use of fertilizers to increase pasture productivity (Fisher et al., 2007), and increased grazing intensity (Navarrete et al., 2016) are common land-management practices in Amazonia. As outlined above, these impacts can have various and sometimes contrasting net effects on albedo, evapotranspiration, and net fluxes of CO2 and other trace gases (Davidson et al., 2012). The latter are likely highly dependent on management practices and not well-characterized for all systems.
Local: Reservoir Construction
The complex river drainage system of the Amazon Basin is interrupted by more than 190 dams, with an additional 246 hydroelectric reservoirs planned or currently under construction (Lees et al., 2016). These structures create habitat fragmentation that inhibits species migration, changes thermal river profiles, reduces water quality, and alters inundation frequency, timing, and extent across wetland and freshwater ecosystems (Lees et al., 2016), as well as acting as potential sources of trace gases and CO2 (Fearnside and Pueyo, 2012; Fearnside, 2015). In unmodified systems, sediment transport by Amazonian rivers moves organic C and associated nutrients downstream, with some C deposited in floodplain lakes (Sanders et al., 2017). Dams interrupt this natural transport, with consequences for river productivity and fisheries (Forsberg et al., 2017; Latrubesse et al., 2017). Although dams offset emissions from fossil fuel derived electricity, their net climate impact may be greater than the power plants they supplant. An attempt to estimate the potential emissions from future reservoirs suggested that some may emit more than fossil fuel plants for many years (de Faria et al., 2015). However, current data suffer from inadequate spatio-temporal coverage and lag dam construction by several years, rendering regional estimates highly uncertain.
Local: Mining and Oil Extraction
Legal and illegal mining and oil extraction have both direct (deforestation, riparian disturbance, riverine sedimentation, and mercury/oil pollution) and indirect (infrastructure development, illegal hunting, overhunting, and forest fires) effects on Amazon biogeochemistry (Anderson et al., 2019). Between 2005 and 2015, deforestation beyond mining operation lease boundaries was estimated at 11,670 km2, almost a tenth of the total Amazonian forest losses during that period (Sonter et al., 2017). In the Madre de Dios region of the Peruvian Amazon, gold mining accounts for deforestation of ~61 km2 year−1, around half is made up of illegal mining along roads and streams (Asner et al., 2013). Though most have not yet been cleared, registered mining interests cover more than 1,000,000 km2 of Amazonia, including indigenous or strictly protected areas (Ferreira et al., 2014) with plans to open at least two more large areas north of the Amazon River to mining and road building. Oil and gas drilling projects are also widespread, facilitating secondary deforestation in addition to their documented impacts to human health through water and food contamination (Anderson et al., 2019).
Local: Hunting and Overfishing
Animal-mediated shifts in plant community composition can have strong implications for vegetation biomass, and thus C stocks and future uptake rates, but have been explored by only a handful of studies. On land, extirpation of large-bodied frugivores (such as large primates and tapirs) by overhunting impacts forest community composition by replacing large-seeded, high-wood-density tree species with smaller-seeded, low-density species as a result of dispersal limitation. Overharvesting affects up to a third of remaining forest in the Brazilian Amazon, and field data and modeling indicate that eliminating large frugivores reduces aboveground forest biomass by 3–6% on average and up to 38% in certain areas (Peres et al., 2016). Similarly, the widespread overfishing of large frugivorous freshwater fish compromises seed dispersal (Anderson et al., 2011) and may drive similar impacts, though assessment data are very limited. Despite the importance to fishing and the many threats to aquatic biodiversity in the Basin (Anderson et al., 2019), the conservation of freshwater biota is limited compared with terrestrial fauna.
Global: Warming, Drought, and Hydrological Change
The Amazon Basin is characterized by hydrological complexity that is highly susceptible to global change. Freshwater and coastal ecosystems cover over one third of the Amazon Basin and are critically linked to their upland counterparts through riverine and atmospheric water exchanges (Castello et al., 2013). Seasonal processes of flooding and drought modulate ecosystem productivity via water availability and nutrient exchange. They also influence atmospheric chemistry both directly (as a control on evapotranspiration, and BVOCs, CH4, and N2O emissions) and indirectly through feedbacks to fire, tree mortality, and riverine C transport (Barichivich et al., 2018).
Interannual rainfall and river variability in Amazonia can be in part attributed to sea surface temperature variations in the tropical oceans. This is manifested as the extremes of the ENSO in the tropical Pacific, and the meridional SST gradient in the Tropical North Atlantic (TNA). During these warm EN events, convective suppression drives lower rainfall in the Amazon Basin and can be particularly extreme when warming is more pronounced in the eastern Pacific as opposed to more moderate effects when the central Pacific is the focus of warming (Jiménez-Muñoz et al., 2016). Most of the severe droughts in the Amazon region are El Niño-related (e.g., 1998, 2010, 2016; Marengo et al., 2018; Jimenez et al., 2019; Cai et al., 2020), and these events are now occurring at a greater frequency than suggested by natural climate variability (Jimenez et al., 2019). However, in 1963 and 2005, the Amazon was affected by a severe drought that was not El Niño-related. Most of the rainfall anomalies that have happened in southwestern Amazonia are initiated by sea surface temperature anomalies in the TNA, including the recent “megadroughts” in 2005 and 2010 (Marengo et al., 2018; Jimenez et al., 2019).
Projections of an abrupt large-scale shift of the Amazon forest caused by climate change are still dominated by large uncertainties (Lapola et al., 2018). Despite rather low confidence in the 5th Coupled Model Intercomparison Project (CMIP5) ensemble mean projections of precipitation, some consensus can be found in the literature. There is high agreement that annual mean precipitation will decline in Amazonia, which is more pronounced in the eastern and south of the Amazon over the twenty-first century. Small changes in rainfall are projected under a moderate emission scenario. In line with observed historical precipitation trends, dry season length is also expected to expand over southern Amazonia. There is also generally model agreement for an increase in precipitation for the end of the twenty-first century over northwestern Amazonia (i.e., Colombia, Ecuador, and north of Peru) (Boisier et al., 2015).
No long-term unidirectional total rainfall trends have been identified in the Amazon region; however, a longer dry season has been observed in southern Amazonia (Marengo et al., 2018), and the positive precipitation trend observed in northwestern Amazon since 1990 may be a consequence of the intensification of the regional hydrological cycle (Gloor et al., 2013). Furthermore, interannual rainfall variability is intensifying and is reflected in both severe droughts (as in 1998, 2005, 2010, and 2016) and floods (1999, 2009, 2012, and 2014) (Marengo et al., 2016, 2018). Regional warming and reduced dry season precipitation can affect atmospheric moisture from Amazonia to southeastern South America, impacting regional hydrology and reducing stream discharge. Projected annual rainfall changes over the twenty-first century indicate that longer dry seasons will delay the onset of the rainy season and drive drought in eastern and southern Amazonia (Dai, 2013; Fu et al., 2013). Hydrological projections for the Madeira, Tapajós, and Xingu rivers and the Purus basin in western Amazonia suggest that the overlapping effects of decreasing precipitation and increasing temperature will reduce basin discharge (Dalagnol et al., 2017; Sampaio et al., 2019); however, others have suggested that discharge will increase with deforestation (Coe et al., 2009).
The combination of severe droughts and floods stress forests, especially if the flooding regimes of regularly inundated areas are perturbed outside of their natural range (Langerwisch et al., 2013). Amazonian droughts cause tree mortality and reduced biomass accrual (Feldpausch et al., 2016), facilitating fire (Aleixo B. et al., 2019), particularly at forest edges, where the microclimate is drier and hotter than in the continuous forest (Laurance et al., 2016). Large trees are most sensitive to these stressors, which is important because these trees regulate microclimate and forest C stocks. Although intact forests may be resilient to acute droughts, chronic droughts impact regional forest health (Gatti et al., 2014), and floods reduce C uptake in low lying forests (Araújo, 2002). Recent work also suggests a changing climate is driving widespread changes in forest composition, with a distinct shift toward large-statured taxa and dry affiliated genera (Esquivel-Muelbert et al., 2019). The future climate implications of this noted shift will depend on the extent to which these changes reflect a mitigating adaptation to a changing climate.
Although nearly 20% of the Amazon upland forests and ~50% of riparian forests have been affected by land-use change, relatively few studies consider the impact of changes in vegetation cover on the Basin's water balance. Climate change scenarios from the CMIP5 used to model the hydrological and C cycles suggest that an increase in deforestation will intensify both floods and low-flow events (Abe et al., 2018). Hydrologic projections considering tropical forest conversion to pasture and farming result in lower rates of evapotranspiration and higher runoff, which counterbalance climate change effects on streamflow (Guimberteau et al., 2017). The Tocantins River has already seen seasonal flows altered by deforestation, with higher flows in the rainy season and lower flows in the dry season (Costa et al., 2003), and models predict that a similar shift in the Xingu River basin will greatly reduce hydroelectric power generation (Stickler et al., 2013). Hydrological intensification also affects riverine C balance, via outgassing from the Amazon River and the magnitude of export to the Atlantic Ocean, with nonlinear effects to be expected if deforestation is also considered (Langerwisch et al., 2016). Finally, changing hydrology may directly impact the deep C cycle by amplifying upland carbonate weathering in the Andes (Hilton, 2017).
Global: Severe Storms
Extreme precipitation events and convective storms cause blowdowns that are a leading cause of tree mortality in the Basin (Nelson et al., 1994; Negrón-Juárez et al., 2010), with synergistic implications for fire, fragmentation, and evapotranspiration (Marra et al., 2018; Silvério et al., 2019). Basin-wide potential mortality from a single squall in 2005 was estimated at 542 ± 121 million trees, equivalent to 23% of mean annual biomass accumulation (Negrón-Juárez et al., 2010). Biomass recovery can take up to 40 years and can shift community composition toward faster-growing soft-wooded species more vulnerable to future events (Ferrante and Fearnside, 2019). Convective storms, which are currently most common in the northwest region (Negrón-Juárez et al., 2018), are likely to increase in number and intensity with climatic warming (Negrón-Juárez et al., 2010); however, their impacts on future mortality patterns are not well-understood (Negrón-Juárez et al., 2018).
Integrating the Biogeochemistry of Climate in the Amazon Basin
Although much remains to be learned, the general impacts of the dominant change agents on C flux and storage, albedo, and evapotranspiration in the Amazon Basin are comparatively well-resolved. Increasing evidence indicates the importance of non-CO2 trace gas and compound fluxes (especially CH4), though far less is known about their pattern and magnitude (especially in the case of BVOCs). Despite this widespread uncertainty, it is increasingly evident that these non-CO2 forcing agents have at least as large an impact on regional and global climate as C (Table 2). Although they do not always respond synchronously, in many cases, local disturbance and global climatic change are expected to increase the net radiative forcing impact of the Amazon region via multiple pathways (Figure 1B; Table 2).
Given the substantive contribution of these less-recognized forcing agents, the next generation of Amazon studies must integrate a broader suite of climate-forcing agents and their feedbacks. These must explicitly address the combined effects of disturbance on the totality of these processes, and the resulting feedbacks on the local, regional, and global climate system. As with forests more broadly, refining the Amazonian impact on the climate system will require (1) additional empirical data to establish pre-disturbance baselines, including manipulative experiments that explore impacts such as increased temperature and drought, and (2) integrative coupled land–atmosphere models that capture both the complexity of the established forest system and biophysical feedbacks accompanying rapid land-use change. Numerous authors suggest integrating multiple forcing agents into ecosystem service markets and Earth system models (Nobre et al., 2016), but the vast majority of studies in the Amazon focus on a single forcing agent (i.e., C; Supplementary Table 1). Although many studies in the region compare fluxes across hydrological, land-use, or chronological recovery gradients, studies that integrate a range of forcing agents across these gradients are exceedingly rare; no studies have concurrently considered the full suite of forest–climate interactions even at a single site. Even in the case of one well-studied type of land-cover change, deforestation, assessing net effects by considering all forcers simultaneously is extremely challenging. For example, because simulations produce radiative forcing effects that range from −12 to +20% of the CO2-only impact, it remains unclear the extent to which cooling from increased surface albedo offsets radiative forcing feedbacks of lowered BVOC emissions following deforestation (Ward and Mahowald, 2015; Scott et al., 2018). Other authors propose that warming from decreased evapotranspiration more than offsets cooling from increased albedo (Bonan, 2008). Warmer and drier regional climates then feedback to influence uptake of CO2, fire frequency (and thus BC), and exchange of trace gases. A more integrated approach is clearly needed.
The effort to resolve trace gas emission estimates from freshwaters in the basin is another illustrative example of the complexity of just one piece of the challenge ahead. Uncertainty in Amazonian aquatic trace gas budgeting is a result of the paucity of measurements along the aquatic continuum, compounded by the difficulty of estimating the extent of these habitats (Melack, 2016; Ward et al., 2017). Better constraining emission controls and gas-transfer velocities in these aquatic environments are key targets for improving biogeochemical models of the Amazon. These models are necessary to refine emission estimates, which in turn could be used to better predict the climate consequences of anthropogenic impacts. While at the process level these challenges are shared with other geographic areas (e.g., avoiding double counting trace emissions from wetland soils), Amazon riverine systems are characterized by stark biogeochemical heterogeneity that does not have applicable analogs elsewhere (Ríos-Villamizar et al., 2013). These idiosyncrasies—coupled with the rivers' huge geographical extent, the number of ecosystem types they transverse, and the rate of surrounding landscape change—continue to confound our understanding of the Basin's biogeochemical influence on global climate.
Better constraining both terrestrial and aquatic fluxes requires integrating our growing understanding of fine-scale processes with ambitious field measurement campaigns linked to high-resolution top-down atmospheric measurements. Most of the trace-gas balance studies to date have focused on either very small spatial scales (like biometry and eddy covariance), which require a large degree of extrapolation, or on global-scale inversions, which fail to constrain tropical land masses even at the continental scale. Some airborne measurements have provided a regional flux picture in Amazonia by making regular vertical profiles of CO2, CH4, and N2O that are broadly representative of regional scales (~105-106 km2) (D'Amelio et al., 2009; Gatti et al., 2010, 2014; Basso et al., 2016). However, measuring large-scale landscape mosaics is required in order to determine the relevant budget for a region or continent (Chou et al., 2002), and local field data are required to link disturbance and biogeochemical processes to atmospheric observations.
Following a decade of hope for a transition to a sustainable development pattern (Davidson et al., 2012), rapid deforestation and land-use change have returned to the Amazon. This resurgent change refocused popular attention on the fate of the Basin's vast C stocks. Our current understanding of the biogeochemistry of climate in the Amazon, however, suggests that positive forcing from non-CO2 factors plays a large role in the regional and global climate system, now likely dominating the net radiative balance of the Amazon. More important than understanding the status quo of net radiative forcing of this more-diverse set of climate forcers is resolving their coupled responses to the accelerating local and global change agents at work in the basin and applying this understanding to manage the biogeochemistry of climate in the Amazon.
Author Contributions
ZP, FS, and KC collated data. KC and FS led the writing of the manuscript, with text contributed by ABe, ZP, SN, SPan, LB, HC, JMar, PF, and HS. All authors provided valuable feedback on the manuscript, subject matter expertise, contributed to the workshop to formulate the study, and organized by KC, AE, and JB.
Funding
This project was funded as part of the National Geographic Society and Rolex partnership to support a Perpetual Planet. This synthesis began as a multi-day convening hosted by the National Geographic Society in Manaus, Brazil, in 2019, for which we acknowledge the advice of Teagan Blaine. KC and ZP acknowledge generous support from the Deborah and Dexter Senft. ABe was funded by a National Geographic Society research grant. LB, HC, and JMay were funded by FAPESP Grants 2018/14006-4, 2018/14423-4, and 2014/50848-9, respectively. JMay was funded by the National Coordination for High Level Education and Training (CAPES) Grant 88887.136402-00INCT and the National Institute of Science and Technology for Climate Change Phase 2 (CNPq Grant 465501/2014-1). PF was funded by CNPq (311103/2015-4, 429795/2016-5), FAPEAM (708565), INPA (PRJ15.125), and Rede Clima (INPE) FINEP (01.13.0353-00). The author team is grateful for the contribution of two reviewers whose thoughtful comments and suggestions considerably improved the manuscript.
Conflict of Interest
The authors declare that the research was conducted in the absence of any commercial or financial relationships that could be construed as a potential conflict of interest.
Supplementary Material
The Supplementary Material for this article can be found online at: https://www.frontiersin.org/articles/10.3389/ffgc.2021.618401/full#supplementary-material
Supplementary Table 1. Studies reporting observed fluxes of forcing agents between the Amazon basin and the atmosphere.
Supplementary Table 2. List of suggested additional sources concerning conceptual underpinning of biogeochemical cycling and global change in the Amazon Basin.
Supplementary Table 3. Representative studies reporting observed, experimentally derived or modeled responses of forcing agents to disturbance, in the Amazon basin or elsewhere in tropical ecosystems. These sources were used to derive direction and confidence of changes in forcing agents in response to disturbance reported in Table 2.
References
Abe, C. A., Lobo, F. D. L., Dibike, Y. B., Costa, M. P. de F., Dos Santos, V., and Novo, E. M. L. M. (2018). Modelling the effects of historical and future land cover changes on the hydrology of an Amazonian basin. Water 10:932. doi: 10.3390/w10070932
Abril, G., Martinez, J.-M., Artigas, L. F., Moreira-Turcq, P., Benedetti, M. F., Vidal, L., et al. (2014). Amazon River carbon dioxide outgassing fuelled by wetlands. Nature 505, 395–398. doi: 10.1038/nature12797
Aguiar, A. P. D., Ometto, J. P., Nobre, C., Lapola, D. M., Almeida, C., Vieira, I. C., et al. (2012). Modeling the spatial and temporal heterogeneity of deforestation-driven carbon emissions: the INPE-EM framework applied to the Brazilian Amazon. Glob. Change Biol. 18, 3346–3366. doi: 10.1111/j.1365-2486.2012.02782.x
Aleixo, B., Pena, J. L., Heller, L., and Rezende, S. (2019). Infrastructure is a necessary but insufficient condition to eliminate inequalities in access to water: research of a rural community intervention in Northeast Brazil. Sci. Total Environ. 652, 1445–1455. doi: 10.1016/j.scitotenv.2018.10.202
Aleixo, I., Norris, D., Hemerik, L., Barbosa, A., Prata, E., Costa, F., et al. (2019). Amazonian rainforest tree mortality driven by climate and functional traits. Nat. Clim. Change 9, 384–388. doi: 10.1038/s41558-019-0458-0
Alonso, M. F., Longo, K. M., Freitas, S. R., da Fonseca, R. M., Marécal, V., Pirre, M., et al. (2010). An urban emissions inventory for South America and its application in numerical modeling of atmospheric chemical composition at local and regional scales. Atmos. Environ. 44, 5072–5083. doi: 10.1016/j.atmosenv.2010.09.013
Alves, E. G., Jardine, K., Tota, J., Jardine, A., Yãnez-Serrano, A. M., Karl, T., et al. (2016). Seasonality of isoprenoid emissions from a primary rainforest in central Amazonia. Atmospheric Chem. Phys. 16, 3903–3925. doi: 10.5194/acp-16-3903-2016
Anderson, E. P., Osborne, T., Maldonado-Ocampo, J. A., Mills-Novoa, M., Castello, L., Montoya, M., et al. (2019). Energy development reveals blind spots for ecosystem conservation in the Amazon Basin. Front. Ecol. Environ. 17, 521–529. doi: 10.1002/fee.2114
Anderson, J. T., Nuttle, T., Saldaña Rojas, J. S., Pendergast, T. H., and Flecker, A. S. (2011). Extremely long-distance seed dispersal by an overfished Amazonian frugivore. Proc. R. Soc. B Biol. Sci. 278, 3329–3335. doi: 10.1098/rspb.2011.0155
Andreae, M. O., Afchine, A., Albrecht, R., Holanda, B. A., Artaxo, P., Barbosa, H. M. J., et al. (2018). Aerosol characteristics and particle production in the upper troposphere over the Amazon Basin. Atmos Chem Phys 18, 921–961. doi: 10.5194/acp-18-921-2018
Aragão, L. E. O. C., Anderson, L. O., Fonseca, M. G., Rosan, T. M., Vedovato, L. B., Wagner, F. H., et al. (2018). 21st Century drought-related fires counteract the decline of Amazon deforestation carbon emissions. Nat. Commun. 9:536. doi: 10.1038/s41467-017-02771-y
Aragão, L. E. O. C., Poulter, B., Barlow, J. B., Anderson, L. O., Malhi, Y., Saatchi, S., et al. (2014). Environmental change and the carbon balance of Amazonian forests: environmental change in Amazonia. Biol. Rev. 89, 913–931. doi: 10.1111/brv.12088
Araújo, A. C. (2002). Comparative measurements of carbon dioxide fluxes from two nearby towers in a central Amazonian rainforest: the Manaus LBA site. J. Geophys. Res. 107:8090. doi: 10.1029/2001JD000676
Artaxo, P., Rizzo, L. V., Brito, J. F., Barbosa, H. M., Arana, A., Sena, E. T., et al. (2013). Atmospheric aerosols in Amazonia and land use change: from natural biogenic to biomass burning conditions. Faraday Discuss. 165, 203–235. doi: 10.1039/c3fd00052d
Asner, G. P., Clark, J. K., Mascaro, J., Galindo García, G. A., Chadwick, K. D., Navarrete Encinales, D. A., et al. (2012). High-resolution mapping of forest carbon stocks in the Colombian Amazon. Biogeosciences 9, 2683–2696. doi: 10.5194/bg-9-2683-2012
Asner, G. P., Llactayo, W., Tupayachi, R., and Luna, E. R. (2013). Elevated rates of gold mining in the Amazon revealed through high-resolution monitoring. Proc. Natl. Acad. Sci. U. S. A. 110, 18454–18459. doi: 10.1073/pnas.1318271110
Asner, G. P., and Tupayachi, R. (2016). Accelerated losses of protected forests from gold mining in the Peruvian Amazon. Environ. Res. Lett. 12:094004. doi: 10.1088/1748-9326/aa7dab
Azevedo-Ramos, C., and Moutinho, P. (2018). No man's land in the Brazilian Amazon: could undesignated public forests slow Amazon deforestation? Land Use Policy 73, 125–127. doi: 10.1016/j.landusepol.2018.01.005
Bala, G., Caldeira, K., Wickett, M., Phillips, T. J., Lobell, D. B., Delire, C., et al. (2007). Combined climate and carbon-cycle effects of large-scale deforestation. Proc. Natl. Acad. Sci. U. S. A. 104:6550. doi: 10.1073/pnas.0608998104
Barbosa, P. M., Farjalla, V. F., Melack, J. M., Amaral, J. H. F., da Silva, J. S., and Forsberg, B. R. (2018). High rates of methane oxidation in an Amazon floodplain lake. Biogeochemistry 137, 351–365. doi: 10.1007/s10533-018-0425-2
Barichivich, J., Gloor, E., Peylin, P., Brienen, R. J. W., Schöngart, J., Espinoza, J. C., et al. (2018). Recent intensification of Amazon flooding extremes driven by strengthened Walker circulation. Sci. Adv. 4:eaat8785. doi: 10.1126/sciadv.aat8785
Barlow, J., Berenguer, E., Carmenta, R., and França, F. (2020). Clarifying Amazonia's burning crisis. Glob. Change Biol. 26, 319–321. doi: 10.1111/gcb.14872
Barros, H. S., and Fearnside, P. M. (2019). Soil carbon is decreasing under “undisturbed” Amazonian forest. Soil Sci. Soc. Am. J. 83, 1779–1785. doi: 10.2136/sssaj2018.12.0489
Barros, N., Cole, J. J., Tranvik, L. J., Prairie, Y. T., Bastviken, D., Huszar, V. L. M., et al. (2011). Carbon emission from hydroelectric reservoirs linked to reservoir age and latitude. Nat. Geosci. 4, 593–596. doi: 10.1038/ngeo1211
Basso, L. S., Gatti, L. V., Gloor, M., Miller, J. B., Domingues, L. G., Correia, C. S. C., et al. (2016). Seasonality and interannual variability of CH4 fluxes from the eastern Amazon Basin inferred from atmospheric mole fraction profiles. J. Geophys. Res. Atmos. 121, 168–184. doi: 10.1002/2015JD023874
Bauwens, M., Stavrakou, T., Müller, J.-F., De Smedt, I., Van Roozendael, M., van der Werf, G. R., et al. (2016). Nine years of global hydrocarbon emissions based on source inversion of OMI formaldehyde observations. Atmos. Chem. Phys. 16, 10133–10158. doi: 10.5194/acp-16-10133-2016
Bela, M. M., Longo, K. M., Freitas, S. R., Moreira, D. S., Beck, V., Wofsy, S. C., et al. (2015). Ozone production and transport over the Amazon Basin during the dry-to-wet and wet-to-dry transition seasons. Atmospheric Chem. Phys. 15, 757–782. doi: 10.5194/acp-15-757-2015
Berenguer, E., Ferreira, J., Gardner, T. A., Aragão, L. E. O. C., De Camargo, P. B., Cerri, C. E., et al. (2014). A large-scale field assessment of carbon stocks in human-modified tropical forests. Glob. Change Biol. 20, 3713–3726. doi: 10.1111/gcb.12627
Bloom, A. A., Lee-Taylor, J., Madronich, S., Messenger, D. J., Palmer, P. I., Reay, D. S., et al. (2010). Global methane emission estimates from ultraviolet irradiation of terrestrial plant foliage. New Phytol. 187, 417–425. doi: 10.1111/j.1469-8137.2010.03259.x
Boers, N., Marwan, N., Barbosa, H. M. J., and Kurths, J. (2017). A deforestation-induced tipping point for the South American monsoon system. Sci. Rep. 7:41489. doi: 10.1038/srep41489
Boisier, J. P., Ciais, P., Ducharne, A., and Guimberteau, M. (2015). Projected strengthening of Amazonian dry season by constrained climate model simulations. Nat. Clim. Change 5, 656–660. doi: 10.1038/nclimate2658
Bonan, G. B. (2008). Forests and climate change: forcings, feedbacks, and the climate benefits of forests. Science 320, 1444–1449. doi: 10.1126/science.1155121
Bond, T. C., Doherty, S. J., Fahey, D. W., Forster, P. M., Berntsen, T., DeAngelo, B. J., et al. (2013). Bounding the role of black carbon in the climate system: a scientific assessment: Black carbon in climate system. J. Geophys. Res. Atmos. 118, 5380–5552. doi: 10.1002/jgrd.50171
Bourtsoukidis, E., Behrendt, T., Yañez-Serrano, A. M., Hellén, H., Diamantopoulos, E., Catão, E., et al. (2018). Strong sesquiterpene emissions from Amazonian soils. Nat. Commun. 9:2226. doi: 10.1038/s41467-018-04658-y
Brando, P. M., Soares-Filho, B., Rodrigues, L., Assunção, A., Morton, D., Tuchschneider, D., et al. (2020). The gathering firestorm in southern Amazonia. Sci. Adv. 6:eaay1632. doi: 10.1126/sciadv.aay1632
Brienen, R. J. W., Phillips, O. L., Feldpausch, T. R., Gloor, E., Baker, T. R., Lloyd, J., et al. (2015). Long-term decline of the Amazon carbon sink. Nature 519:344. doi: 10.1038/nature14283
Bullock, E. L., and Woodcock, C. E. (2020). Carbon loss and removal due to forest disturbance and regeneration in the Amazon. Sci. Total Environ. 764:142839. doi: 10.1016/j.scitotenv.2020.142839
Bullock, E. L., Woodcock, C. E., Souza, C Jr, and Olofsson, P. (2020). Satellite-based estimates reveal widespread forest degradation in the Amazon. Glob. Change Biol. 26, 2956–2969. doi: 10.1111/gcb.15029
Bustamante, M. M. C., Keller, M., and Silva, D. A. (2009). “Sources and sinks of trace gases in Amazonia and the Cerrado,” in Amazonia and Global Change Geophysical Monograph Series, 337–354. Available online at: https://www.fs.usda.gov/treesearch/pubs/43198 (accessed September 28, 2019). doi: 10.1029/2008GM000733
Cai, W., McPhaden, M. J., Grimm, A. M., Rodrigues, R. R., Taschetto, A. S., Garreaud, R. D., et al. (2020). Climate impacts of the El Niño–Southern Oscillation on South America. Nat. Rev. Earth Environ. 1, 215–231. doi: 10.1038/s43017-020-0040-3
Call, M., Sanders, C. J., Enrich-Prast, A., Sanders, L., Marotta, H., Santos, I. R., et al. (2018). Radon-traced pore-water as a potential source of CO2 and CH4 to receding black and clear water environments in the Amazon Basin. Limnol. Oceanogr. Lett. 3, 375–383. doi: 10.1002/lol2.10089
Cardil, A., de-Miguel, S., Silva, C. A., Reich, P. B., Calkin, D., Brancalion, P. H. S., et al. (2020). Recent deforestation drove the spike in Amazonian fires. Environ. Res. Lett. 15:121003. doi: 10.1088/1748-9326/abcac7
Carvalho, W. D., Mustin, K., Hilário, R. R., Vasconcelos, I. M., Eilers, V., and Fearnside, P. M. (2019). Deforestation control in the Brazilian Amazon: a conservation struggle being lost as agreements and regulations are subverted and bypassed. Perspect. Ecol. Conserv. 17, 122–130. doi: 10.1016/j.pecon.2019.06.002
Castello, L., McGrath, D. G., Hess, L. L., Coe, M. T., Lefebvre, P. A., Petry, P., et al. (2013). The vulnerability of Amazon freshwater ecosystems. Conserv. Lett. 6, 217–229. doi: 10.1111/conl.12008
Chou, W. W., Wofsy, S. C., Harriss, R. C., Lin, J. C., Gerbig, C., and Sachse, G. W. (2002). Net fluxes of CO2 in Amazonia derived from aircraft observations. J. Geophys. Res. Atmospheres 107, ACH 4-1–ACH 4-15. doi: 10.1029/2001JD001295
Clifton, O. E., Fiore, A. M., Massman, W. J., Baublitz, C. B., Coyle, M., Emberson, L., et al. (2020). Dry deposition of ozone over land: processes, measurement, and modeling. Rev. Geophys. 58:e2019RG000670. doi: 10.1029/2019RG000670
Coe, M. T., Costa, M. H., and Soares-Filho, B. S. (2009). The influence of historical and potential future deforestation on the stream flow of the Amazon River – land surface processes and atmospheric feedbacks. J. Hydrol. 369, 165–174. doi: 10.1016/j.jhydrol.2009.02.043
Cooper, O. R., Parrish, D. D., Ziemke, J., Balashov, N. V., Cupeiro, M., Galbally, I. E., et al. (2014). Global distribution and trends of tropospheric ozone: an observation-based review of the Global distribution and trends of tropospheric ozone. Elem. Sci. Anthr. 2:29. doi: 10.12952/journal.elementa.000029
Coppola, A. I., Seidel, M., Ward, N. D., Viviroli, D., Nascimento, G. S., Haghipour, N., et al. (2019). Marked isotopic variability within and between the Amazon River and marine dissolved black carbon pools. Nat. Commun. 10, 1–8. doi: 10.1038/s41467-019-11543-9
Coppola, A. I., Wiedemeier, D. B., Galy, V., Haghipour, N., Hanke, U. M., Nascimento, G. S., et al. (2018). Global-scale evidence for the refractory nature of riverine black carbon. Nat. Geosci. 11, 584–588. doi: 10.1038/s41561-018-0159-8
Costa, M. H., Botta, A., and Cardille, J. A. (2003). Effects of large-scale changes in land cover on the discharge of the Tocantins River, Southeastern Amazonia. J. Hydrol. 283, 206–217. doi: 10.1016/S0022-1694(03)00267-1
Covey, K. R., and Megonigal, J. P. (2019). Methane production and emissions in trees and forests. New Phytol. 222, 35–51. doi: 10.1111/nph.15624
Crowther, T. W., and Bradford, M. A. (2013). Thermal acclimation in widespread heterotrophic soil microbes. Ecol. Lett. 16, 469–477. doi: 10.1111/ele.12069
Cusack, D. F., Markesteijn, L., Condit, R., Lewis, O. T., and Turner, B. L. (2018). Soil carbon stocks across tropical forests of Panama regulated by base cation effects on fine roots. Biogeochemistry 137, 253–266. doi: 10.1007/s10533-017-0416-8
Dai, A. (2013). Increasing drought under global warming in observations and models. Nat. Clim. Change 3, 52–58. doi: 10.1038/nclimate1633
Dalagnol, R., Borma, L. de S., Mateus, P., Rodriguez, D. A., Dalagnol, R., Borma, L. de S., et al. (2017). Assessment of climate change impacts on water resources of the Purus Basin in the southwestern Amazon. Acta Amaz. 47, 213–226. doi: 10.1590/1809-4392201601993
Dalmagro, H. J., Arruda, P. H. Z., de, Vourlitis, G. L., Lathuillière, M. J., Nogueira, J. de S., Couto, E. G., et al. (2019). Radiative forcing of methane fluxes offsets net carbon dioxide uptake for a tropical flooded forest. Glob. Change Biol. 25, 1967–1981. doi: 10.1111/gcb.14615
D'Amelio, M. T. S., Gatti, L. V., Miller, J. B., and Tans, P. (2009). Regional N2O fluxes in Amazonia derived from aircraft vertical profiles. Atmospheric Chem. Phys. 9, 8785–8797. doi: 10.5194/acp-9-8785-2009
Davidson, E. A., and Artaxo, P. (2004). Globally significant changes in biological processes of the Amazon Basin: results of the Large-scale Biosphere–Atmosphere Experiment. Glob. Change Biol. 10, 519–529. doi: 10.1111/j.1529-8817.2003.00779.x
Davidson, E. A., de Araújo, A. C., Artaxo, P., Balch, J. K., Brown, I. F., C. Bustamante, M. M., et al. (2012). The Amazon basin in transition. Nature 481, 321–328. doi: 10.1038/nature10717
Davidson, E. A., Nepstad, D. C., Ishida, F. Y., and Brando, P. M. (2008). Effects of an experimental drought and recovery on soil emissions of carbon dioxide, methane, nitrous oxide, and nitric oxide in a moist tropical forest. Glob. Change Biol. 14, 2582–2590. doi: 10.1111/j.1365-2486.2008.01694.x
de Faria, F., Jaramillo, P., Sawakuchi, H. O., Richey, J. E., and Barros, N. (2015). Estimating greenhouse gas emissions from future Amazonian hydroelectric reservoirs. Environ. Res. Lett. 10:124019. doi: 10.1088/1748-9326/10/12/124019
de Fátima F. L. Rasera, M., Krusche, A. V., Richey, J. E., Ballester, M. V. R., and Victória, R. L. (2013). Spatial and temporal variability of pCO2 and CO2 efflux in seven Amazonian Rivers. Biogeochemistry 116, 241–259. doi: 10.1007/s10533-013-9854-0
Dezécache, C., Faure, E., Gond, V., Salles, J.-M., Vieilledent, G., and Hérault, B. (2017). Gold-rush in a forested El Dorado: deforestation leakages and the need for regional cooperation. Environ. Res. Lett. 12:034013. doi: 10.1088/1748-9326/aa6082
Dias, L. C. P., Macedo, M. N., Costa, M. H., Coe, M. T., and Neill, C. (2015). Effects of land cover change on evapotranspiration and streamflow of small catchments in the Upper Xingu River Basin, Central Brazil. J. Hydrol. Reg. Stud. 4, 108–122. doi: 10.1016/j.ejrh.2015.05.010
Elias, F., Ferreira, J., Lennox, G. D., Berenguer, E., Ferreira, S., Schwartz, G., et al. (2020). Assessing the growth and climate sensitivity of secondary forests in highly deforested Amazonian landscapes. Ecology 101:2954. doi: 10.1002/ecy.2954
Escobar, H. (2020). Deforestation in the Brazilian Amazon is still rising sharply. Science 369:613. doi: 10.1126/science.369.6504.613
Esquivel-Muelbert, A., Baker, T. R., Dexter, K. G., Lewis, S. L., Brienen, R. J. W., Feldpausch, T. R., et al. (2019). Compositional response of Amazon forests to climate change. Glob. Change Biol. 25, 39–56. doi: 10.1111/gcb.14413
Fearnside, P. M. (1997). Greenhouse gases from deforestation in Brazilian Amazonia: net committed emissions. Clim. Change 35, 321–360. doi: 10.1023/A:1005336724350
Fearnside, P. M. (2000). Global warming and tropical land-use change: greenhouse gas emissions from biomass burning, decomposition and soils in forest conversion, shifting cultivation and secondary vegetation. Clim. Change 46, 115–158. doi: 10.1023/A:1005569915357
Fearnside, P. M. (2015). Tropical hydropower in the clean development mechanism: Brazil's Santo Antônio Dam as an example of the need for change. Clim. Change 131, 575–589. doi: 10.1007/s10584-015-1393-3
Fearnside, P. M. (2016). Greenhouse gas emissions from Brazil's Amazonian hydroelectric dams. Environ. Res. Lett. 11:011002. doi: 10.1088/1748-9326/11/1/011002
Fearnside, P. M. (2017). Brazil's Belo Monte Dam: lessons of an Amazonian resource struggle. J. Geogr. Soc. Berl. 148, 167–184. doi: 10.12854/erde-148-46
Fearnside, P. M., and Pueyo, S. (2012). Greenhouse-gas emissions from tropical dams. Nat. Clim. Change 2, 382–384. doi: 10.1038/nclimate1540
Feldpausch, T. R., Lloyd, J., Lewis, S. L., Brienen, R. J. W., Gloor, M., Monteagudo Mendoza, A., et al. (2012). Tree height integrated into pantropical forest biomass estimates. Biogeosciences 9, 3381–3403. doi: 10.5194/bg-9-3381-2012
Feldpausch, T. R., Phillips, O. L., Brienen, R. J. W., Gloor, E., Lloyd, J., Lopez-Gonzalez, G., et al. (2016). Amazon forest response to repeated droughts. Glob. Biogeochem. Cycles 30, 964–982. doi: 10.1002/2015GB005133
Ferrante, L., and Fearnside, P. M. (2019). Brazil's new president and ‘ruralists’ threaten Amazonia's environment, traditional peoples and the global climate. Environ. Conserv. 46, 261–263. doi: 10.1017/S0376892919000213
Ferreira, J., Aragão, L. E. O. C., Barlow, J., Barreto, P., Berenguer, E., Bustamante, M., et al. (2014). Brazil's environmental leadership at risk. Science 346, 706–707. doi: 10.1126/science.1260194
Fisher, M. J., Braz, S. P., Dos, R. S. M., Urquiaga, S., Alves, B. J. R., and Boddey, R. M. (2007). Another dimension to grazing systems: soil carbon. Trop. Grass 41, 65–83. Available online at: https://hdl.handle.net/10568/43186
Fonte, S. J., Nesper, M., Hegglin, D., Velásquez, J. E., Ramirez, B., Rao, I. M., et al. (2014). Pasture degradation impacts soil phosphorus storage via changes to aggregate-associated soil organic matter in highly weathered tropical soils. Soil Biol. Biochem. 68, 150–157. doi: 10.1016/j.soilbio.2013.09.025
Forsberg, B. R., Melack, J. M., Dunne, T., Barthem, R. B., Goulding, M., Paiva, R. C. D., et al. (2017). The potential impact of new Andean dams on Amazon fluvial ecosystems. PLoS ONE 12:e0182254. doi: 10.1371/journal.pone.0182254
Fu, Y., Argus, D. F., Freymueller, J. T., and Heflin, M. B. (2013). Horizontal motion in elastic response to seasonal loading of rain water in the Amazon Basin and monsoon water in Southeast Asia observed by GPS and inferred from GRACE. Geophys. Res. Lett. 40, 6048–6053. doi: 10.1002/2013GL058093
Ganzeveld, L., and Lelieveld, J. (2004). Impact of Amazonian deforestation on atmospheric chemistry. Geophys. Res. Lett. 31:19205. doi: 10.1029/2003GL019205
Gatti, L. V., Gloor, M., Miller, J. B., Doughty, C. E., Malhi, Y., Domingues, L. G., et al. (2014). Drought sensitivity of Amazonian carbon balance revealed by atmospheric measurements. Nature 506, 76–80. doi: 10.1038/nature12957
Gatti, L. V., Miller, J. B., Basso, L. S., Domingues, L. G., Cassol, H. L. G., Marani, L., et al. (2019). Amazon Carbon Balance and its Sensitivity to Climate and Human-Driven Changes. American Geophysical Union. Available online at: http://repositorio.ipen.br/handle/123456789/30979 (accessed May 19, 2020).
Gatti, L. V., Miller, J. B., D'amelio, M. T., aS, Martinewski, A., Basso, L. S., Gloor, M. E., et al. (2010). Vertical profiles of CO2 above eastern Amazonia suggest a net carbon flux to the atmosphere and balanced biosphere between 2000 and 2009. Tellus B Chem. Phys. Meteorol. 62, 581–594. doi: 10.1111/j.1600-0889.2010.00484.x
Gerken, T., Wei, D., Chase, R. J., Fuentes, J. D., Schumacher, C., Machado, L. A., et al. (2016). Downward transport of ozone rich air and implications for atmospheric chemistry in the Amazon rainforest. Atmos. Environ. 124, 64–76. doi: 10.1016/j.atmosenv.2015.11.014
Global Fire Emissions Database version 4 GFED4. (2020). Global Fire Emissions Database version 4 GFED4. Available online at: https://www.geo.vu.nl/~gwerf/GFED/GFED4/tables/GFED4.1s_BC.txt (accessed May 20, 2020).
Gloor, M., Brienen, R. J. W., Galbraith, D., Feldpausch, T. R., Schöngart, J., Guyot, J.-L., et al. (2013). Intensification of the Amazon hydrological cycle over the last two decades. Geophys. Res. Lett. 40, 1729–1733. doi: 10.1002/grl.50377
Greenberg, J. P., Guenther, A. B., Pétron, G., Wiedinmyer, C., Vega, O., Gatti, L. V., et al. (2004). Biogenic VOC emissions from forested Amazonian landscapes. Glob. Change Biol. 10, 651–662. doi: 10.1111/j.1365-2486.2004.00758.x
Guenther, A. (2002). The contribution of reactive carbon emissions from vegetation to the carbon balance of terrestrial ecosystems. Chemosphere 49, 837–844. doi: 10.1016/S0045-6535(02)00384-3
Guérin, F., Abril, G., Tremblay, A., and Delmas, R. (2008). Nitrous oxide emissions from tropical hydroelectric reservoirs. Geophys. Res. Lett. 35:57. doi: 10.1029/2007GL033057
Guimberteau, M., Ciais, P., Ducharne, A., Boisier, J. P., Aguiar, A. P. D., Biemans, H., et al. (2017). Impacts of future deforestation and climate change on the hydrology of the Amazon Basin: a multi-model analysis with a new set of land-cover change scenarios. Hydrol Earth Syst Sci 21, 1455–1475. doi: 10.5194/hess-21-1455-2017
Hansen, M. C., Potapov, P. V., Moore, R., Hancher, M., Turubanova, S. A., Tyukavina, A., et al. (2013). High-resolution global maps of 21st-century forest cover change. Science 342, 850–853. doi: 10.1126/science.1244693
Hayhoe, S. J., Neill, C., Porder, S., Mchorney, R., Lefebvre, P., Coe, M. T., et al. (2011). Conversion to soy on the Amazonian agricultural frontier increases streamflow without affecting stormflow dynamics. Glob. Change Biol. 17, 1821–1833. doi: 10.1111/j.1365-2486.2011.02392.x
Hess, L. L., Melack, J. M., Affonso, A. G., Barbosa, C., Gastil-Buhl, M., and Novo, E. M. L. M. (2015). Wetlands of the Lowland Amazon Basin: Extent, Vegetative Cover, and Dual-season Inundated Area as Mapped with JERS-1 Synthetic Aperture Radar. Wetlands 35, 745–756. doi: 10.1007/s13157-015-0666-y
Hilton, R. G. (2017). Climate regulates the erosional carbon export from the terrestrial biosphere. Geomorphology 277, 118–132. doi: 10.1016/j.geomorph.2016.03.028
Hu, M., Chen, D., and Dahlgren, R. A. (2016). Modeling nitrous oxide emission from rivers: a global assessment. Glob. Change Biol. 22, 3566–3582. doi: 10.1111/gcb.13351
Hubau, W., Lewis, S. L., Phillips, O. L., Affum-Baffoe, K., Beeckman, H., Cuní-Sanchez, A., et al. (2020). Asynchronous carbon sink saturation in African and Amazonian tropical forests. Nature 579, 80–87. doi: 10.1038/s41586-020-2035-0
IPCC (2015). “Climate change 2014: synthesis report,” in Contribution of Working Groups I, II and III to the Fifth Assessment Report of the Intergovernmental Panel on Climate Change, eds Core Writing Team, R. K. Pachauri and L. A. Meyer (Geneva: IPCC), 151.
IPCC (2019). “Summary for Policy Makers, in Climate Change and Land: an IPCC special report on climate change, desertification, land degradation, sustainable land management, food security, and greenhouse gas fluxes in terrestrial ecosystems,” eds P. R. Shukla, J. Skea, E. Calvo Buendia, V. Masson-Delmotte, H.- O. Pörtner, D. C. Roberts, et al., 118.
Jackson, R. B., Randerson, J. T., Canadell, J. G., Anderson, R. G., Avissar, R., Baldocchi, D. D., et al. (2008). Protecting climate with forests. Environ. Res. Lett. 3:044006. doi: 10.1088/1748-9326/3/4/044006
Jardine, K. J., Chambers, J. Q., Holm, J., Jardine, A. B., Fontes, C. G., Zorzanelli, R. F., et al. (2015). Green leaf volatile emissions during high temperature and drought stress in a central Amazon Rainforest. Plants 4, 678–690. doi: 10.3390/plants4030678
Jimenez, J. C., Marengo, J. A., Alves, L. M., Sulca, J. C., Takahashi, K., Ferrett, S., et al. (2019). The role of ENSO flavours and TNA on recent droughts over Amazon forests and the Northeast Brazil region. Int. J. Climatol. doi: 10.1002/joc.6453
Jiménez-Muñoz, J. C., Mattar, C., Barichivich, J., Santamaría-Artigas, A., Takahashi, K., Malhi, Y., et al. (2016). Record-breaking warming and extreme drought in the Amazon rainforest during the course of El Niño 2015–2016. Sci. Rep. 6:33130. doi: 10.1038/srep33130
Kalamandeen, M., Gloor, E., Mitchard, E., Quincey, D., Ziv, G., Spracklen, D., et al. (2018). Pervasive rise of small-scale deforestation in Amazonia. Sci. Rep. 8, 1–10. doi: 10.1038/s41598-018-19358-2
Keller, M., Varner, R., Dias, J. D., Silva, H., Crill, P., de Oliveira, R. C., et al. (2005). Soil–atmosphere exchange of nitrous oxide, nitric oxide, methane, and carbon dioxide in logged and undisturbed forest in the Tapajos National Forest, Brazil. Earth Interact. 9, 1–28. doi: 10.1175/EI125.1
Koschorreck, M. (2005). Nitrogen turnover in drying sediments of an amazon floodplain lake. Microb. Ecol. 49, 567–577. doi: 10.1007/s00248-004-0087-6
Kuhn, U., Andreae, M. O., Ammann, C., Gatti, L. V., Ganzeveld, L., Kruijt, B., et al. (2007). Isoprene and monoterpene ?uxes from Central Amazonian rainforest inferred from tower-based and airborne measurements, and implications on the atmospheric chemistry and the local carbon budget. Atmos. Chem. Phys. 7, 2855–2879. doi: 10.5194/acp-7-2855-2007
Langerwisch, F., Rost, S., Gerten, D., Poulter, B., Rammig, A., and Cramer, W. (2013). Potential effects of climate change on inundation patterns in the Amazon Basin. Hydrol. Earth Syst. Sci. 17, 2247–2262. doi: 10.5194/hess-17-2247-2013
Langerwisch, F., Walz, A., Rammig, A., Tietjen, B., Thonicke, K., and Cramer, W. (2016). Deforestation in Amazonia impacts riverine carbon dynamics. Earth Syst. Dyn. 7:2016. doi: 10.5194/esd-7-953-2016
Laothawornkitkul, J., Taylor, J. E., Paul, N. D., and Hewitt, C. N. (2009). Biogenic volatile organic compounds in the Earth system. New Phytol. 183, 27–51. doi: 10.1111/j.1469-8137.2009.02859.x
Lapola, D. M., Pinho, P., Quesada, C. A., Strassburg, B. B. N., Rammig, A., Kruijt, B., et al. (2018). Limiting the high impacts of Amazon forest dieback with no-regrets science and policy action. Proc. Natl. Acad. Sci. U. S. A. 115:11671. doi: 10.1073/pnas.1721770115
Latrubesse, E. M., Arima, E. Y., Dunne, T., Park, E., Baker, V. R., d'Horta, F. M., et al. (2017). Damming the rivers of the Amazon basin. Nature 546:363. doi: 10.1038/nature22333
Laurance, W. F., Camargo, J. L. C., Fearnside, P. M., Lovejoy, T. E., Williamson, G. B., Mesquita, R. C. G., et al. (2016). “An Amazonian forest and its fragments as a laboratory of global change,” in Interactions Between Biosphere, Atmosphere and Human Land Use in the Amazon Basin Ecological Studies, eds. L. Nagy, B. R. Forsberg, and P. Artaxo (Berlin, Heidelberg: Springer), 407–440. doi: 10.1007/978-3-662-49902-3_17
Lees, A. C., Peres, C. A., Fearnside, P. M., Schneider, M., and Zuanon, J. A. S. (2016). Hydropower and the future of Amazonian biodiversity. Biodivers. Conserv. 25, 451–466. doi: 10.1007/s10531-016-1072-3
Leite-Filho, A. T., Costa, M. H., and Fu, R. (2020). The southern Amazon rainy season: the role of deforestation and its interactions with large-scale mechanisms. Int. J. Climatol. 40, 2328–2341. doi: 10.1002/joc.6335
Liu, L., Cheng, Y., Wang, S., Wei, C., Pöhlker, M., Pöhlker, C., et al. (2020). Impact of biomass burning aerosols on radiation, clouds, and precipitation over the Amazon during the dry season: dependence of aerosol-cloud and aerosol-radiation interactions on aerosol loading. Atmos. Chem. Phys. 20, 13283–13301. doi: 10.5194/acp-20-13283-2020
Loarie, S. R., Lobell, D. B., Asner, G. P., and Field, C. B. (2010). Land-cover and surface water change drive large albedo increases in South America. Earth Interact. 15, 1–16. doi: 10.1175/2010EI342.1
Lovejoy, T. E., and Nobre, C. (2018). Amazon tipping point. Sci. Adv. 4:eaat2340. doi: 10.1126/sciadv.aat2340
Luizão, F., Matson, P., Livingston, G., Luizão, R., and Vitousek, P. (1989). Nitrous oxide flux following tropical land clearing. Glob. Biogeochem. Cycles 3, 281–285. doi: 10.1029/GB003i003p00281
Luyssaert, S., Jammet, M., Stoy, P. C., Estel, S., Pongratz, J., Ceschia, E., et al. (2014). Land management and land-cover change have impacts of similar magnitude on surface temperature. Nat. Clim. Change 4, 389–393. doi: 10.1038/nclimate2196
Magalhães, N. de, Evangelista, H., Condom, T., Rabatel, A., and Ginot, P. (2019). Amazonian biomass burning enhances tropical andean glaciers melting. Sci. Rep. 9:16914. doi: 10.1038/s41598-019-53284-1
Malavelle, F. F., Haywood, J. M., Mercado, L. M., Folberth, G. A., Bellouin, N., Sitch, S., et al. (2019). Studying the impact of biomass burning aerosol radiative and climate effects on the Amazon rainforest productivity with an Earth system model. Atmos. Chem. Phys. 19, 1301–1326. doi: 10.5194/acp-19-1301-2019
Mallick, K., Trebs, I., Boegh, E., Giustarini, L., Schlerf, M., Drewry, D. T., et al. (2016). Canopy-scale biophysical controls of transpiration and evaporation in the Amazon Basin. Hydrol. Earth Syst. Sci. 20, 4237–4264. doi: 10.5194/hess-2015-552
MAPBIOMAS Version 2.0 (2020). MAPBIOMAS Version 2.0. Available online at: https://www.amazoniasocioambiental.org/es/
Marengo, J. A., Souza, C. M., Thonicke, K., Burton, C., Halladay, K., Betts, R. A., et al. (2018). Changes in climate and land use over the Amazon region: current and future variability and trends. Front. Earth Sci. 6:228. doi: 10.3389/feart.2018.00228
Marengo, J. A., Williams, E. R., Alves, L. M., Soares, W. R., and Rodriguez, D. A. (2016). “Extreme seasonal climate variations in the Amazon Basin: droughts and floods,” in Interactions Between Biosphere, Atmosphere and Human Land Use in the Amazon Basin, eds. L. Nagy, B. R. Forsberg, and P. Artaxo (Berlin, Heidelberg: Springer Berlin Heidelberg), 55–76. doi: 10.1007/978-3-662-49902-3_4
Marin-Spiotta, E., Silver, W. L., Swanston, C. W., and Ostertag, R. (2009). Soil organic matter dynamics during 80 years of reforestation of tropical pastures. Glob. Change Biol. 15, 1584–1597. doi: 10.1111/j.1365-2486.2008.01805.x
Marra, D. M., Trumbore, S. E., Higuchi, N., Ribeiro, G. H. P. M., Negrón-Juárez, R. I., Holzwarth, F., et al. (2018). Windthrows control biomass patterns and functional composition of Amazon forests. Glob. Change Biol. 24, 5867–5881. doi: 10.1111/gcb.14457
Martin, S. T., Andreae, M. O., Artaxo, P., Baumgardner, D., Chen, Q., Goldstein, A. H., et al. (2010). Sources and properties of Amazonian aerosol particles. Rev. Geophys. 48:208. doi: 10.1029/2008RG000280
Martin, S. T., Artaxo, P., Machado, L., Manzi, A. O., Souza, R. A. F., de, Schumacher, C., et al. (2017). The Green Ocean Amazon experiment (GoAmazon2014/5) observes pollution affecting gases, aerosols, clouds, and rainfall over the rain forest. Bull. Am. Meteorol. Soc. 98, 981–997. doi: 10.1175/BAMS-D-15-00221.1
Martins, V. S., Novo, E. M., Lyapustin, A., Aragão, L. E., Freitas, S. R., and Barbosa, C. C. (2018). Seasonal and interannual assessment of cloud cover and atmospheric constituents across the Amazon (2000–2015): insights for remote sensing and climate analysis. ISPRS J. Photogramm. Remote Sens. 145, 309–327. doi: 10.1016/j.isprsjprs.2018.05.013
Martinson, G. O., Werner, F. A., Scherber, C., Conrad, R., Corre, M. D., Flessa, H., et al. (2010). Methane emissions from tank bromeliads in neotropical forests. Nat. Geosci. 3, 766–769. doi: 10.1038/ngeo980
Melack, J. M. (2016). “Aquatic Ecosystems,” in Interactions Between Biosphere, Atmosphere and Human Land Use in the Amazon Basin Ecological Studies. eds L. Nagy, B. R. Forsberg, and P. Artaxo (Berlin, Heidelberg: Springer Berlin Heidelberg), 119–148. doi: 10.1007/978-3-662-49902-3_7
Melack, J. M., Hess, L. L., Gastil, M., Forsberg, B. R., Hamilton, S. K., Lima, I. B. T., et al. (2004). Regionalization of methane emissions in the Amazon Basin with microwave remote sensing. Glob. Change Biol. 10, 530–544. doi: 10.1111/j.1365-2486.2004.00763.x
Meurer, K. H. E., Franko, U., Stange, C. F., Rosa, J. D., Madari, B. E., and Jungkunst, H. F. (2016). Direct nitrous oxide (N 2 O) fluxes from soils under different land use in Brazil—a critical review. Environ. Res. Lett. 11:023001. doi: 10.1088/1748-9326/11/2/023001
Moran-Zuloaga, D., Ditas, F., Walter, D., Saturno, J., Brito, J., Carbone, S., et al. (2018). Long-term study on coarse mode aerosols in the Amazon rain forest with the frequent intrusion of Saharan dust plumes. Atmos. Chem. Phys. 18, 10055–10088. doi: 10.5194/acp-18-10055-2018
Mosquera, O., Buurman, P., Ramirez, B. L., and Amezquita, M. C. (2012). Carbon stocks and dynamics under improved tropical pasture and silvopastoral systems in Colombian Amazonia. Geoderma 189–190, 81–86. doi: 10.1016/j.geoderma.2012.04.022
Myhre, G., Shindell, D., Bréon, F.-M., Collins, W., Fuglestvedt, J., Huang, J., et al. (2013). “Anthropogenic and Natural Radiative Forcing,” in Climate Change 2013: The Physical Science Basis. Contribution of Working Group I to the Fifth Assessment Report of the Intergovernmental Panel on Climate Change, eds. T. F. Stocker, D. Qin, M. G-K Plattner, S. K. Tignor, J. Allen, A. Boschung, et al. (Cambridge and New York, NY: Cambridge University Press), 82. Available online at: https://www.ipcc.ch/site/assets/uploads/2018/02/WG1AR5_Chapter08_FINAL.pdf (accessed January 11, 2021).
Navarrete, D., Sitch, S., Aragão, L. E. O. C., and Pedroni, L. (2016). Conversion from forests to pastures in the Colombian Amazon leads to contrasting soil carbon dynamics depending on land management practices. Glob. Change Biol. 22, 3503–3517. doi: 10.1111/gcb.13266
Negrón-Juárez, R. I., Chambers, J. Q., Guimaraes, G., Zeng, H., Raupp, C. F. M., Marra, D. M., et al. (2010). Widespread Amazon forest tree mortality from a single cross-basin squall line event. Geophys. Res. Lett. 37:3733. doi: 10.1029/2010GL043733
Negrón-Juárez, R. I., Holm, J. A., Marra, D. M., Rifai, S. W., Riley, W. J., Chambers, J. Q., et al. (2018). Vulnerability of Amazon forests to storm-driven tree mortality. Environ. Res. Lett. 13:aabe9f. doi: 10.1088/1748-9326/aabe9f
Nelson, B. W., Kapos, V., Adams, J. B., Oliveira, W. J., and Braun, O. P. G. (1994). Forest disturbance by large blowdowns in the Brazilian Amazon. Ecology 75, 853–858. doi: 10.2307/1941742
Nepstad, D., McGrath, D., Stickler, C., Alencar, A., Azevedo, A., Swette, B., et al. (2014). Slowing Amazon deforestation through public policy and interventions in beef and soy supply chains. Science 344, 1118–1123. doi: 10.1126/science.1248525
Nepstad, D. C., Moutinho, P., Dias-Filho, M. B., Davidson, E., Cardinot, G., Markewitz, D., et al. (2002). The effects of partial throughfall exclusion on canopy processes, aboveground production, and biogeochemistry of an Amazon forest. J. Geophys. Res. Atmospheres 107, LBA 53-1. doi: 10.1029/2001JD000360
Neu, V., Neill, C., and Krusche, A. V. (2011). Gaseous and fluvial carbon export from an Amazon forest watershed. Biogeochemistry 105, 133–147. doi: 10.1007/s10533-011-9581-3
Nobre, C. A., Sampaio, G., Borma, L. S., Castilla-Rubio, J. C., Silva, J. S., and Cardoso, M. (2016). Land-use and climate change risks in the Amazon and the need of a novel sustainable development paradigm. Proc. Natl. Acad. Sci. U. S. A. 113:10759. doi: 10.1073/pnas.1605516113
Palácios, R. da S., Morais, F. G., Landulfo, E., Franco, M. A. de M., Kuhnen, I. A., Marques, J. B., et al. (2020). Long term analysis of optical and radiative properties of aerosols in the Amazon Basin. Aerosol Air Qual. Res. 20, 139–154. doi: 10.4209/aaqr.2019.04.0189
Pan, Y., Birdsey, R. A., Fang, J., Houghton, R., Kauppi, P. E., Kurz, W. A., et al. (2011). A large and persistent carbon sink in the world's forests. Science 333, 988–993. doi: 10.1126/science.1201609
Pangala, S. R., Enrich-Prast, A., Basso, L. S., Peixoto, R. B., Bastviken, D., Hornibrook, E. R. C., et al. (2017). Large emissions from floodplain trees close the Amazon methane budget. Nature 552, 230–234. doi: 10.1038/nature24639
Paralovo, S. L., Barbosa, C. G., Carneiro, I. P., Kurzlop, P., Borillo, G. C., Schiochet, M. F. C., et al. (2019). Observations of particulate matter, NO2, SO2, O3, H2S and selected VOCs at a semi-urban environment in the Amazon region. Sci. Total Environ. 650, 996–1006. doi: 10.1016/j.scitotenv.2018.09.073
Peñuelas, J., and Llusià, J. (2003). BVOCs: plant defense against climate warming? Trends Plant Sci. 8, 105–109. doi: 10.1016/S1360-1385(03)00008-6
Peñuelas, J., and Staudt, M. (2010). BVOCs and global change. Trends Plant Sci. 15, 133–144. doi: 10.1016/j.tplants.2009.12.005
Peres, C. A., Emilio, T., Schietti, J., Desmoulière, S. J. M., and Levi, T. (2016). Dispersal limitation induces long-term biomass collapse in overhunted Amazonian forests. Proc. Natl. Acad. Sci. U. S. A. 113, 892–897. doi: 10.1073/pnas.1516525113
Pfannerstill, E. Y., Nölscher, A. C., Yáñez-Serrano, A. M., Bourtsoukidis, E., Keßel, S., Janssen, R. H. H., et al. (2018). Total OH reactivity changes over the Amazon rainforest during an El Niño Event. Front. For. Glob. Change 1:12. doi: 10.3389/ffgc.2018.00012
Pöhlker, C., Wiedemann, K. T., Sinha, B., Shiraiwa, M., Gunthe, S. S., Smith, M., et al. (2012). Biogenic potassium salt particles as seeds for secondary organic aerosol in the Amazon. Science 337, 1075–1078. doi: 10.1126/science.1223264
Pöschl, U., Martin, S. T., Sinha, B., Chen, Q., Gunthe, S. S., Huffman, J. A., et al. (2010). Rainforest aerosols as biogenic nuclei of clouds and precipitation in the Amazon. Science 329, 1513–1516. doi: 10.1126/science.1191056
Prem, M., Saavedra, S., and Vargas, J. F. (2019). End-of-Conflict Deforestation: Evidence From Colombia's Peace Agreement. Rochester, NY: Social Science Research Network. Available online at: https://papers.ssrn.com/abstract=3306715 (accessed October 10, 2019).
PRODES (2020). Coordenação-Geral de Observação da Terra. Available online at: http://www.obt.inpe.br/OBT/assuntos/programas/amazonia/prodes (accessed June 2, 2020).
Quesada, C. A., Lloyd, J., Schwarz, M., Patiño, S., Baker, T. R., Czimczik, C., et al. (2010). Variations in chemical and physical properties of Amazon forest soils in relation to their genesis. Biogeosciences 7, 1515–1541. doi: 10.5194/bg-7-1515-2010
RAISG (2020). Available online at: https://www.amazoniasocioambiental.org/es/ (accessed January 11, 2021).
Ramanathan, V., and Carmichael, G. (2008). Global and regional climate changes due to black carbon. Nat. Geosci. 1, 221–227. doi: 10.1038/ngeo156
Randerson, J. T., van der Werf, G. R., Giglio, L., Collatz, G. J., and Kasibhatla, P. S. (2018). Global Fire Emissions Database, Version 4, (GFEDv4). Oak Ridge, TN: ORNL DAAC.
Rappaport, D. I., Morton, D. C., Longo, M., Keller, M., Dubayah, R., and dos-Santos, M. N. (2018). Quantifying long-term changes in carbon stocks and forest structure from Amazon forest degradation. Environ. Res. Lett. 13:065013. doi: 10.1088/1748-9326/aac331
Raymond, P. A., Hartmann, J., Lauerwald, R., Sobek, S., McDonald, C., Hoover, M., et al. (2013). Global carbon dioxide emissions from inland waters. Nature 503, 355–359. doi: 10.1038/nature12760
Richards, P. D., Walker, R. T., and Arima, E. Y. (2014). Spatially complex land change: the indirect effect of Brazil's agricultural sector on land use in Amazonia. Glob. Environ. Change 29, 1–9. doi: 10.1016/j.gloenvcha.2014.06.011
Richey, J. E., Krusche, A. V., Johnson, M. S., da Cunha, H. B., and Ballester, M. V. (2009). “The role of rivers in the regional carbon balance,” in Amazonia and Global Change, ed. M. Keller (Washington, DC: American Geophysical Union). Available online at: https://ui.adsabs.harvard.edu/abs/2009GMS.186.489R/abstract (accessed November 13, 2019). doi: 10.1029/2008GM000734
Richey, J. E., Melack, J. M., Aufdenkampe, A. K., Ballester, V. M., and Hess, L. L. (2002). Outgassing from Amazonian rivers and wetlands as a large tropical source of atmospheric CO2. Nature 416, 617–620. doi: 10.1038/416617a
Ríos-Villamizar, E. A., Piedade, M. T. F., Da Costa, J. G., Adeney, J. M., and Junk, W. J. (2013). Chemistry of different Amazonian water types for river classification: a preliminary review. Water Soc. 178, 17–28. doi: 10.2495/WS130021
Rowlinson, M. J., Rap, A., Arnold, S. R., Pope, R. J., Chipperfield, M. P., McNorton, J., et al. (2019). Impact of El Niño–Southern Oscillation on the interannual variability of methane and tropospheric ozone. Atmos. Chem. Phys. 19, 8669–8686. doi: 10.5194/acp-19-8669-2019
Saikawa, E., Prinn, R. G., Dlugokencky, E., Ishijima, K., Dutton, G. S., Hall, B. D., et al. (2014). Global and regional emissions estimates for N2O. Atmospheric Chem. Phys. 14, 4617–4641. doi: 10.5194/acp-14-4617-2014
Saikawa, E., Schlosser, C. A., and Prinn, R. G. (2013). Global modeling of soil nitrous oxide emissions from natural processes. Glob. Biogeochem. Cycles 27, 972–989. doi: 10.1002/gbc.20087
Saito, M., Kim, H.-S., Ito, A., Yokota, T., and Maksyutov, S. (2016). Enhanced methane emissions during Amazonian drought by biomass burning. PLoS ONE 11:e0166039. doi: 10.1371/journal.pone.0166039
Salati, E., Dall'Olio, A., Matsui, E., and Gat, J. R. (1979). Recycling of water in the Amazon Basin: an isotopic study. Water Resour. Res. 15, 1250–1258. doi: 10.1029/WR015i005p01250
Sampaio, G., Borma, L. S., Cardoso, M., Alves, L. M., von Randow, C., Rodriguez, D. A., et al. (2019). “Assessing the Possible Impacts of a 4 °C or Higher Warming in Amazonia,” in Climate Change Risks in Brazil, eds. C. A. Nobre, J. A. Marengo, and W. R. Soares (Cham: Springer International Publishing), 201–218. doi: 10.1007/978-3-319-92881-4_8
Sampaio, G., Nobre, C., Costa, M. H., Satyamurty, P., Soares-Filho, B. S., and Cardoso, M. (2007). Regional climate change over eastern Amazonia caused by pasture and soybean cropland expansion. Geophys. Res. Lett. 34:30612. doi: 10.1029/2007GL030612
Sanders, L. M., Taffs, K. H., Stokes, D. J., Sanders, C. J., Smoak, J. M., Enrich-Prast, A., et al. (2017). Carbon accumulation in Amazonian floodplain lakes: a significant component of Amazon budgets? Limnol. Oceanogr. Lett. 2, 29–35. doi: 10.1002/lol2.10034
Saunois, M., Stavert, A. R., Poulter, B., Bousquet, P., Canadell, J. G., Jackson, R. B., et al. (2019). The Global Methane Budget 2000-2017. Earth Syst. Sci. Data Discuss.
Sawakuchi, H. O., Bastviken, D., Sawakuchi, A. O., Ward, N. D., Borges, C. D., Tsai, S. M., et al. (2016). Oxidative mitigation of aquatic methane emissions in large Amazonian rivers. Glob. Change Biol. 22, 1075–1085. doi: 10.1111/gcb.13169
Sawakuchi, H. O., Neu, V., Ward, N. D., Barros, M. de L. C., Valerio, A. M., Gagne-Maynard, W., et al. (2017). Carbon Dioxide Emissions along the Lower Amazon River. Front. Mar. Sci. 4:76. doi: 10.3389/fmars.2017.00076
Schindler, T., Mander, Ü., Machacova, K., Espenberg, M., Krasnov, D., Escuer-Gatius, J., et al. (2020). Short-term flooding increases CH4 and N2O emissions from trees in a riparian forest soil-stem continuum. Sci. Rep. 10:3204. doi: 10.1038/s41598-020-60058-7
Scott, C. E., Monks, S. A., Spracklen, D. V., Arnold, S. R., Forster, P. M., Rap, A., et al. (2018). Impact on short-lived climate forcers increases projected warming due to deforestation. Nat. Commun. 9, 1–9. doi: 10.1038/s41467-017-02412-4
Sena, E. T., Artaxo, P., and Correia, A. L. (2013). Spatial variability of the direct radiative forcing of biomass burning aerosols and the effects of land use change in Amazonia. Atmos Chem Phys 13, 1261–1275. doi: 10.5194/acp-13-1261-2013
Sena, E. T., Dias, M. S., Carvalho, L. M. V., and Dias, P. S. (2018). Reduced wet-season length detected by satellite retrievals of cloudiness over Brazilian Amazonia: a new methodology. J. Clim. 31, 9941–9964. doi: 10.1175/JCLI-D-17-0702.1
Shrivastava, M., Andreae, M. O., Artaxo, P., Barbosa, H. M., Berg, L. K., Brito, J., et al. (2019). Urban pollution greatly enhances formation of natural aerosols over the Amazon rainforest. Nat. Commun. 10, 1–12. doi: 10.1038/s41467-019-08909-4
Shrivastava, M., Cappa, C. D., Fan, J., Goldstein, A. H., Guenther, A. B., Jimenez, J. L., et al. (2017). Recent advances in understanding secondary organic aerosol: Implications for global climate forcing. Rev. Geophys. 55, 509–559. doi: 10.1002/2016RG000540
Silva Junior, C. H. L., Anderson, L. O., Silva, A. L., Almeida, C. T., Dalagnol, R., Pletsch, M. A. J. S., et al. (2019). Fire Responses to the 2010 and 2015/2016 Amazonian Droughts. Front. Earth Sci. 7:97. doi: 10.3389/feart.2019.00097
Silvério, D. V., Brando, P. M., Bustamante, M. M. C., Putz, F. E., Marra, D. M., Levick, S. R., et al. (2019). Fire, fragmentation, and windstorms: A recipe for tropical forest degradation. J. Ecol. 107, 656–667. doi: 10.1111/1365-2745.13076
Sindelarova, K., Granier, C., Bouarar, I., Guenther, A., Tilmes, S., Stavrakou, T., et al. (2014). Global data set of biogenic VOC emissions calculated by the MEGAN model over the last 30 years. Atmos. Chem. Phys. 14, 9317–9341. doi: 10.5194/acp-14-9317-2014
Smith, P., Cotrufo, M. F., Rumpel, C., Paustian, K., Kuikman, P. J., Elliott, J. A., et al. (2015). Biogeochemical cycles and biodiversity as key drivers of ecosystem services provided by soils. Soil 1, 665–685. doi: 10.5194/soil-1-665-2015
Sommer, R., Sá, T. D. de A., Vielhauer, K., Araújo, A. C., de, Fölster, H., and Vlek, P. L. G. (2002). Transpiration and canopy conductance of secondary vegetation in the eastern Amazon. Agric. For. Meteorol. 112, 103–121. doi: 10.1016/S0168-1923(02)00044-8
Sonter, L. J., Herrera, D., Barrett, D. J., Galford, G. L., Moran, C. J., and Soares-Filho, B. S. (2017). Mining drives extensive deforestation in the Brazilian Amazon. Nat. Commun. 8, 1–7. doi: 10.1038/s41467-017-00557-w
Soper, F. M., Sullivan, B. W., Osborne, B. B., Shaw, A. N., Philippot, L., and Cleveland, C. C. (2019). Leaf-cutter ants engineer large nitrous oxide hot spots in tropical forests. Proc. R. Soc. B Biol. Sci. 286:20182504. doi: 10.1098/rspb.2018.2504
Steininger, M. K., Tucker, C. J., Townshend, J. R. G., Killeen, T. J., Desch, A., Bell, V., et al. (2001). Tropical deforestation in the Bolivian Amazon. Environ. Conserv. 28, 127–134. doi: 10.1017/S0376892901000133
Stickler, C. M., Coe, M. T., Costa, M. H., Nepstad, D. C., McGrath, D. G., Dias, L. C. P., et al. (2013). Dependence of hydropower energy generation on forests in the Amazon Basin at local and regional scales. Proc. Natl. Acad. Sci. U. S. A. 110, 9601–9606. doi: 10.1073/pnas.1215331110
Subramaniam, A., Yager, P. L., Carpenter, E. J., Mahaffey, C., Björkman, K., Cooley, S., et al. (2008). Amazon River enhances diazotrophy and carbon sequestration in the tropical North Atlantic Ocean. Proc. Natl. Acad. Sci. U. S. A. 105, 10460–10465. doi: 10.1073/pnas.0710279105
Swann, A. L. S., Longo, M., Knox, R. G., Lee, E., and Moorcroft, P. R. (2015). Future deforestation in the Amazon and consequences for South American climate. Agric. For. Meteorol. 214–215, 12–24. doi: 10.1016/j.agrformet.2015.07.006
Thompson, I., Mackey, B., McNulty, S., and Mosseler, A. (2009). Forest Resilience, Biodiversity, and Climate Change. Available online at: https://www.fs.usda.gov/treesearch/pubs/36775 (accessed September 26, 2019).
Trebs, I., Mayol-Bracero, O. L., Pauliquevis, T., Kuhn, U., Sander, R., Ganzeveld, L., et al. (2012). Impact of the Manaus urban plume on trace gas mixing ratios near the surface in the Amazon Basin: implications for the NO-NO2-O3 photostationary state and peroxy radical levels. J. Geophys. Res. Atmospheres 117:16386. doi: 10.1029/2011JD016386
Unger, N. (2014). Human land-use-driven reduction of forest volatiles cools global climate. Nat. Clim. Change 4, 907–910. doi: 10.1038/nclimate2347
van der Laan-Luijkx, I. T., van der Velde, I. R., Krol, M. C., Gatti, L. V., Domingues, L. G., Correia, C. S. C., et al. (2015). Response of the Amazon carbon balance to the 2010 drought derived with CarbonTracker South America: Amazon Carbon Cycle During the 2010 Drought. Glob. Biogeochem. Cycles 29, 1092–1108. doi: 10.1002/2014GB005082
Walker, W. S., Gorelik, S. R., Baccini, A., Aragon-Osejo, J. L., Josse, C., Meyer, C., et al. (2020). The role of forest conversion, degradation, and disturbance in the carbon dynamics of Amazon indigenous territories and protected areas. Proc. Natl. Acad. Sci. U. S. A. 117, 3015–3025. doi: 10.1073/pnas.1913321117
Ward, D. S., and Mahowald, N. M. (2015). Local sources of global climate forcing from different categories of land use activities. Earth Syst. Dyn. 6, 175–194. doi: 10.5194/esd-6-175-2015
Ward, N. D., Bianchi, T. S., Medeiros, P. M., Seidel, M., Richey, J. E., Keil, R. G., et al. (2017). Where carbon goes when water flows: carbon cycling across the aquatic continuum. Front. Mar. Sci. 4:7. doi: 10.3389/fmars.2017.00007
Ward, N. D., Bianchi, T. S., Sawakuchi, H. O., Gagne-Maynard, W., Cunha, A. C., Brito, D. C., et al. (2016). The reactivity of plant-derived organic matter and the potential importance of priming effects along the lower Amazon River. J. Geophys. Res. Biogeosciences 121, 1522–1539. doi: 10.1002/2016JG003342
Ward, N. D., Keil, R. G., Medeiros, P. M., Brito, D. C., Cunha, A. C., Dittmar, T., et al. (2013). Degradation of terrestrially derived macromolecules in the Amazon River. Nat. Geosci. 6, 530–533. doi: 10.1038/ngeo1817
Wilson, C., Gloor, M., Gatti, L. V., Miller, J. B., Monks, S. A., McNorton, J., et al. (2016). Contribution of regional sources to atmospheric methane over the Amazon Basin in 2010 and 2011: regional CH4 Sources In the Amazon Basin. Glob. Biogeochem. Cycles 30, 400–420. doi: 10.1002/2015GB005300
Wright, J. S., Fu, R., Worden, J. R., Chakraborty, S., Clinton, N. E., Risi, C., et al. (2017). Rainforest-initiated wet season onset over the southern Amazon. Proc. Natl. Acad. Sci. U. S. A. 114, 8481–8486. doi: 10.1073/pnas.1621516114
Xu-Ri Prentice, I. C., Spahni, R., and Niu, H. S. (2012). Modelling terrestrial nitrous oxide emissions and implications for climate feedback. New Phytol. 196, 472–488. doi: 10.1111/j.1469-8137.2012.04269.x
Yáñez-Serrano, A. M., Bourtsoukidis, E., Alves, E. G., Bauwens, M., Stavrakou, T., Llusià, J., et al. (2020). Amazonian biogenic volatile organic compounds under global change. Glob. Change Biol. 26, 4722–4751. doi: 10.1111/gcb.15185
Zemp, D. C., Schleussner, C.-F., Barbosa, H. M. J., Hirota, M., Montade, V., Sampaio, G., et al. (2017). Self-amplified Amazon forest loss due to vegetation-atmosphere feedbacks. Nat. Commun. 8, 1–10. doi: 10.1038/ncomms14681
Keywords: methane, nitrous oxide, climate change, black carbon, biogenic VOC emission, land use - land cover change
Citation: Covey K, Soper F, Pangala S, Bernardino A, Pagliaro Z, Basso L, Cassol H, Fearnside P, Navarrete D, Novoa S, Sawakuchi H, Lovejoy T, Marengo J, Peres CA, Baillie J, Bernasconi P, Camargo J, Freitas C, Hoffman B, Nardoto GB, Nobre I, Mayorga J, Mesquita R, Pavan S, Pinto F, Rocha F, de Assis Mello R, Thuault A, Bahl AA and Elmore A (2021) Carbon and Beyond: The Biogeochemistry of Climate in a Rapidly Changing Amazon. Front. For. Glob. Change 4:618401. doi: 10.3389/ffgc.2021.618401
Received: 16 October 2020; Accepted: 12 February 2021;
Published: 11 March 2021.
Edited by:
Dylan Craven, Universidad Mayor, ChileReviewed by:
Wu Sun, Carnegie Institution for Science (CIS), United StatesAna Maria Yáñez-Serrano, Ecological and Forestry Applications Research Center (CREAF), Spain
Copyright © 2021 Covey, Soper, Pangala, Bernardino, Pagliaro, Basso, Cassol, Fearnside, Navarrete, Novoa, Sawakuchi, Lovejoy, Marengo, Peres, Baillie, Bernasconi, Camargo, Freitas, Hoffman, Nardoto, Nobre, Mayorga, Mesquita, Pavan, Pinto, Rocha, de Assis Mello, Thuault, Bahl and Elmore. This is an open-access article distributed under the terms of the Creative Commons Attribution License (CC BY). The use, distribution or reproduction in other forums is permitted, provided the original author(s) and the copyright owner(s) are credited and that the original publication in this journal is cited, in accordance with accepted academic practice. No use, distribution or reproduction is permitted which does not comply with these terms.
*Correspondence: Kristofer Covey, a2NvdmV5QHNraWRtb3JlLmVkdQ==