- 1Institute of Soil Science and Soil Conservation, Justus-Liebig University Giessen, Giessen, Germany
- 2Research Centre Jülich, Institute of Bio- and Geosciences, Agrosphere (IBG-3), Jülich, Germany
- 3Faculty of Environment and Natural Resources, Chair of Soil Ecology, University of Freiburg, Freiburg im Breisgau, Germany
Phosphorus (P) is preferentially bound to colloids in soil. On the one hand, colloids may facilitate soil P leaching leading to a decrease of plant available P, but on the other hand they can carry P to plant roots, thus supporting the P uptake of plants. We tested the magnitude and the kinetics of P delivery by colloids into a P sink mimicking plant roots using the Diffusive Gradients in Thin-Films (DGT) technique. Colloids were extracted with water from three forest soils differing in parent material using a method based on dispersion and sedimentation. Freeze-dried colloids, the respective bulk soil, and the colloid-free extraction residue were sterilized and mixed with quartz sand and silt to an equal P basis. The mixtures were wetted and the diffusive fluxes of P into the DGTs were measured under sterile, water unsaturated conditions. The colloids extracted from a P-poor sandy podzolic soil were highly enriched in iron and organic matter compared to the bulk soil and delivered more P at a higher rate into the sink compared to bulk soil and the colloid-free soil extraction residue. However, colloidal P delivery into the sink was smaller than P release and transport from the bulk soil developed on dolomite rock, and with no difference for a soil with intermediate phosphorus-stocks developed from gneiss. Our results provide evidence that both the mobility of colloids and their P binding strength control their contribution to the plant available P-pool of soils. Overall, our findings highlight the relevance of colloids for P delivery to plant roots.
Introduction
Intact forest ecosystems are of extraordinary relevance for biodiversity, the functioning of biogeochemical cycles, landscape water balance and human health (Watson et al., 2018). Phosphorus (P) is an essential element for all living organisms (Elser et al., 2007) and a major limiting factor for the productivity of forest ecosystems, as well as an important driver for soil and ecosystem development (Vitousek et al., 2010; Lang et al., 2017). Recent studies indicate that forests may lose their ability to recycle P due to climate change and enhanced global nitrogen (N) input (Jonard et al., 2015). Understanding the functioning of forest ecosystems and their nutrient cycles is therefore of utmost importance for their protection and sustainable use.
Natural soil colloids (diameter <1,000 nm) embody the smallest particulate phase in soils. Colloids bind larger amounts of P through their high specific surface area compared to other soil components (de Jonge et al., 2004). These particles are formed through weathering, the formation of secondary minerals and the incomplete degradation of organic material (e.g., Missong et al., 2016). Due to their small size, colloids are subject to Brownian motion so that they diffuse through the soil solution, whereby smaller particles are more strongly influenced by the Brownian motion and diffuse faster than large colloids (e.g., Molnar et al., 2019).
The importance of colloids for P-binding and -transport in agricultural soils, which receive P-containing fertilizers, has been recognized for quite some time (e.g., Haygarth et al., 1997; Jacobsen et al., 1997; de Jonge et al., 2004; Heathwaite et al., 2005; Ilg et al., 2005; Jiang et al., 2015; Li et al., 2020). More recently, the importance of colloids for P-binding and –transport was also shown for unfertilized forest soils (Bol et al., 2016; Gottselig et al., 2017a,b, 2020; Missong et al., 2018a,b; Wang et al., 2020). These studies revealed that the P-content of colloids of acidic forest soils was up to 16 times larger than the P-contents of the corresponding bulk soils (Missong et al., 2018b). They also showed that up to 91% of the P leached from forest topsoils was not truly dissolved, but bound to colloids (Missong et al., 2018a). Determined by field flow fractionation, these colloids could be subdivided into three size classes of particles smaller than 25 nm rich in organic carbon (C), particles between 25 and 240 nm composed mainly of organic C, iron (Fe), silicon (Si), and aluminum (Al), and finally particles between 240 and 500 nm with an important contribution of phyllosilicates (Missong et al., 2018a). In calcareous forest soils, calcium (Ca) was the primary metallic element in water-dispersible colloids (WDC) (Wang et al., 2020).
The availability of P bound to colloids for plant uptake is crucial for evaluating the relevance of particle-facilitated P-leaching and redistribution for P-cycling in ecosystems. If the P bound to colloids would be well-accessible for plants, then transport of P from intensely rooted topsoils into deep subsoils or out of the ecosystem together with colloids would have severe consequences for the P supply to the vegetation and the stability of ecosystems. Montalvo et al. (2015a) demonstrated that colloids from a volcanic soil (Andosol) could also act as P-carriers supporting the diffusional transport of P to plant roots and the subsequent P-uptake of plants in hydroponic systems, while no such effect was observed for particles derived from a strongly weathered tropical soil (Oxisol). Based on their finding that ortho-P and hexamethaphosphate bound to Fe-oxide colloids were well-accessible for spinach grown in nutrient solution, Bollyn et al. (2017) emphasized the potential role of pedogenic Fe-oxides for the P-availability in soils and the possibility of using synthetic Fe-oxides as nanofertilizer facilitating P transport to roots. Therefore, an important question is whether colloids from more widely distributed soils of temperate climate zones, like Cambisols, could also support the diffusional P transport to roots and whether this process does also occur in porous media under water-unsaturated moisture conditions. Unsaturated porous media provide many opportunities for attachment of colloids to water-solid interfaces and water-air interfaces or their entrapment in thin water films (Kretzschmar et al., 1999; Flury and Aramrak, 2017). Hence, the enhancement of diffusional colloidal P transport to roots or similar P sinks might be less relevant in water-unsaturated porous media, like most terrestrial soils, than in hydroponic systems. Indeed, Bollyn et al. (2019) observed no additional fertilizer effect of P bound to Fe-oxide nanoparticles compared to soluble KH2PO4 when added to strongly weathered tropical soils. They argued that the much smaller diffusion coefficient of colloids compared to ortho-phosphate in combination with its lower bioavailability were responsible for this lacking additional fertilizer effect in relation to ortho-phosphate. Nevertheless, the experiments of Bollyn et al. (2019) demonstrated that P bound to Fe-oxides increased plant growth and P uptake relative to a zero P control, indicating that it can be used by plants at least partly.
We examined the delivery of P bound to natural soil colloids from forest soils to artificial P sinks in comparison to P-delivery from colloid-free soil and bulk soil. To this end, we extracted colloids from three forest soils differing in parent material and soil P stock with water. For each of the three soils, the colloids, the colloid-free extraction residue and the respective bulk soil were mixed with quartz silt and sand and P-delivery into artificial P-sinks was quantified at a moisture content equal to 90% water-holding capacity using the Diffusive Gradients in Thin-Films technique (DGT) (Zhang et al., 1998). DGT is based on the continuous depletion of P in the soil solution around the sampler. Reduced P concentrations are then compensated by desorption of P from the soil solid phase. DGT therefore measures the P fraction that can be released into soil water and transported to a sink after soil solution depletion, thus simulating the diffusive uptake of P by plant roots (Kruse et al., 2015). For soils in which P uptake by plants is limited by diffusion, DGT is a good predictor of plant-available P (Degryse et al., 2009), since DGT mainly extracts plant-available P as shown by 33P isotope dilution studies (Six et al., 2012; Mason et al., 2013). Considering that the mobility of WDC by diffusion and the lability of colloid-bound P are important drivers for their role in ecosystems, the DGT technique provides valuable insights into the behavior of WDC at the root-soil interface. We hypothesized that: (1) colloids deliver more P at a faster rate into the P-sink than bulk soil and colloid-free residual soil, and that (2) soil properties control the magnitude and the kinetics of colloid-facilitated P-delivery into a nutrient sink according to their impact on colloid mobility, the partitioning of P between WDC and non-dispersible soil material and the P binding strength of colloids. Thus, soil properties control the potential relevance of colloids for plant nutrition.
Materials and Methods
Forest Sites
Disturbed soil samples from A-horizons and the O/A-transition horizon were collected from three mature beech forest sites in Germany in a sampling campaign in summer 2017. All sites were similar with regard to their forest stand characteristics (tree species composition and stand age), while they differed in the source material of soil formation (Table 1) and therefore in their soil P-stock and -availability (Lang et al., 2016, 2017; Prietzel et al., 2016). The soil sampled at the Mitterfels site (MIT) developed on paragneiss and had the highest P content (Tables 1, 2), followed by the sample from the calcareous site Mangfall (MAN) located in the dolomite alps the with a slightly smaller P content, while the sample from the sandy podzolic soil of the site Lüss (LUE) had a much smaller P content (Tables 1, 2). For further information regarding the sites visit https://www.ecosystem-nutrition.uni-freiburg.de/.
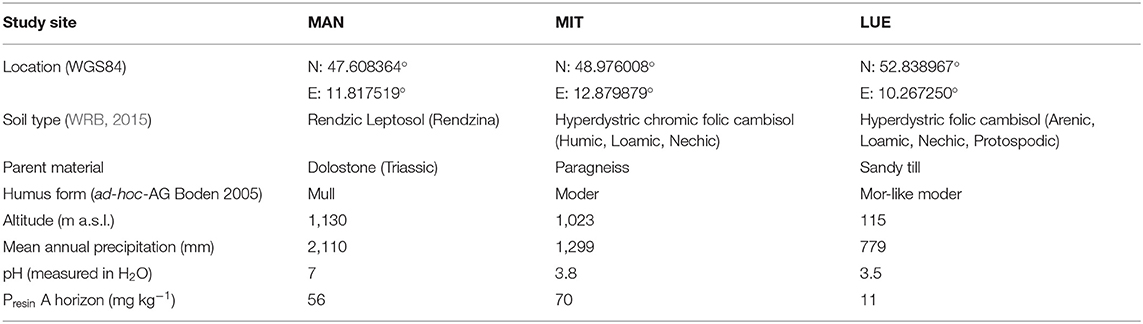
Table 1. Characteristics of the study sites Mangfall (MAN), Mitterfels (MIT) and Lüss (LUE) (Prietzel et al., 2016; Lang et al., 2017).
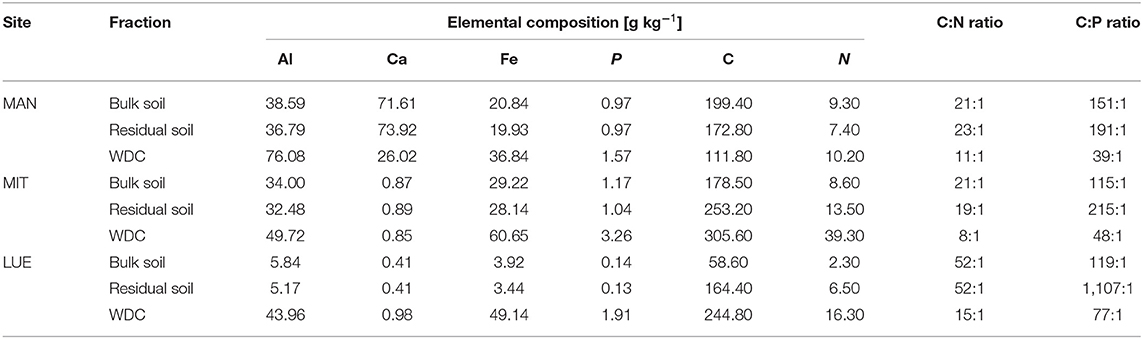
Table 2. Elemental composition of P-sources of the study sites Mangfall (MAN), Mitterfels (MIT), and Lüss (LUE) after microwave-assisted extraction and ICP-OES and TOC/TN measurements in [g kg−1], including C:N and C:P ratios.
Extraction and Preparation of Water-Dispersible Colloids
Water-dispersible colloids (WDC) were extracted from topsoil samples from the MAN, MIT and LUE sites following an extraction routine based on suspension in water with subsequent sedimentation and centrifugation steps developed by Séquaris and Lewandowski (2003) and Missong et al. (2016). The centrifugation times for the separation of colloids were determined according to Hathaway (1956) to separate the WDC fraction <700 nm. First 0.2 L demineralized water was added to 100 g sample material and shaken on an overhead shaker for 6 h. Subsequently 0.6 L demineralized water was added, the suspension was left to settle for 20 min and the supernatant was pipetted off. The supernatant was centrifuged at 4,000 g for 4 min and the supernatant was removed by pipette. The supernatant after centrifugation was centrifuged again at 14,000 g for 90 min and the supernatant discarded, thus we potentially lost the smallest WDC. The amount of P bound to WDC lost with the supernatants was quantified through separation from dissolved P using dialysis tubes (Spectrum™ Labs Spectra/Por™ 7, Repligen Corporation, Rancho Dominguez, USA) with subsequent colorimetric measurements (Van Veldhoven and Mannaerts, 1987). The sedimented portion after this process step formed the WDC P-source, while the sedimented soil components after shaking and sedimentation, as well as the sedimented soil components after centrifugation at 4,000 g formed the colloid-free residual soil fraction. The WDC suspensions obtained by extraction were shock-frozen in 45 mL PE bottles using liquid nitrogen. The caps of the PE bottles were then removed and replaced with fine-pored filters (GE WhatmanTM SG81, GE Healthcare Bio-Sciences, Marlborough, USA) for freeze-drying (Christ Beta 1–8 LSCplus, Martin Christ Gefriertrocknungsanlagen GmbH, Osterode am Harz, Germany). The dried WDC powders were subsequently transferred into 1 L PE bottles and homogenized for 6 h using an overhead shaker.
Bulk soil and residual soil (residue of the WDC-extraction) were dried in a drying cabinet at 40°C. After drying, bulk soil, residual soil and WDC were sterilized with a 60Co source as described by Dalkmann et al. (2014) to prevent microbial cycling of P. Total C and -N contents of the P-sources were measured using an vario MAX cube (Elementar Analysensysteme GmbH, Langenselbold, Germany). Aliquots (0.5 g) of the three different P-source materials were digested using 5 mL 69% HNO3, 3 mL 30% H2O2 (both ROTIPURAN® Supra, Carl Roth GmbH + Co. KG, Karlsruhe, Germany) and 5 mL H2O by microwave-assisted extraction (Öztan and Düring, 2012) and the P-contents were determined by inductively coupled plasma optical emission spectrometry (ICP-OES) (Agilent 720 ICP-OES ES, Agilent Technologies Inc., Santa Clara, USA) as specified in DIN ISO 22036:2009-06 at the element-specific wavelength of 213.618 nm. Particle size distributions of WDC were obtained by Nanoparticle Tracking Analysis (NTA). Three replicates of WDC suspension from every site were analyzed using the laser unit NanoSight LM 14 (Malvern Panalytical Ltd., Malvern, United Kingdom) combined with the microscope NanoSight LM 10 (Malvern Panalytical Ltd., Malvern, United Kingdom). Images were evaluated by the NTA 3.0 software (Malvern Panalytical Ltd., Malvern, United Kingdom) (Supplementary Material 1).
Test System
A mixture of 60 mass-% quartz sand and 40 mass-% quartz silt (20.5 g in total, Gebrüder Dorfner GmbH & Co., Hirschau, Germany) were filled into 60 mL screw-cap beakers made of polycarbonate (Thermo Fisher Scientific, Waltham, Massachusetts, USA). The particle size composition of the quartz mixture determined according to DIN 19683/2 was 1.0% medium sand, 60.4% fine sand, 20.0% coarse silt, 12.3% medium silt, 4.1% fine silt, and 2.2% clay. After autoclaving, the beakers were opened in a sterile environment and the equivalent of 1 mg P of a P-source [bulk soil, residual soil or WDC of the sites MAN, MIT, and LUE, as well as an ortho-phosphate solution (Certipur®, Merck KGaA, Darmstadt, Germany) as a positive control] was filled into a screw-cap beaker. The screw-cap beakers were closed again and mixed for 6 h on an overhead shaker (Heidolph REAX 2, Heidolph Instruments GmbH & CO. KG, Schwabach, Germany). The screw-cap beakers were then opened again in a sterile environment and autoclaved water was added with an air cushion pipette until 90% of the water holding capacity was reached. The water holding capacity of the porous media including the individual soil fractions was determined in preliminary tests according to Alef (1998, p. 106). The screw cap beakers were then closed for 24 h. During this time, an equilibrium of P between the added water and the soil solid phase was established. The screw-cap beakers were opened again in the sterile environment and one DGT [LSLP-NP Loaded DGT device for P and metals (B) in soil, DGT Research, Quernmore, Great Britain] was inserted in each beaker and gently pressed into the substrate. The screw cap beakers were closed and the DGTs were removed after 24, 48, 72, 120, and 168 h, with three DGTs per treatment removed after 48 h. The ambient temperature during the experiment was kept constant at 22 ± 0.3°C. The semi-permeable filter membranes were rinsed with demineralized water afterwards to stop the mass transport into the device. The DGTs were opened at the groove of the housing and the diffusion layers together with the semipermeable membrane, as well as the ferrihydrite gels were transferred to 2 mL Safelock tubes (Eppendorf AG, Hamburg, Germany) and stored at 4°C until further analysis. The ferrihydrite gels were than eluted with 0.25 M H2SO4 as described by Zhang et al. (1998). The P-concentrations of the eluates were determined using the method of Van Veldhoven and Mannaerts (1987) after formation of phosphomolybdate complexes with malachite green and measured at 630 nm by a UV/Vis photometer (T80 Spectrophotometer, PG Instruments Ltd., Lutterworth, Great Britain) (Supplementary Material 2). Additionally the diffusion layers of the disassembled DGTs after 168 h exposure time to the P-sources were rinsed with water and Al, Ca, Fe, and P concentrations in the gels were determined using ICP-OES after microwave-assisted digestion similar to the P-sources, using 5 mL 69% HNO3, 3 mL 30% H2O2, and 5 mL H2O for each gel. Due to the harsh extractant used and the high temperatures, the resulting concentrations can be interpreted as total elemental concentrations.
Statistical Analysis
The statistical evaluation of the measurements after 48 h was performed with R (version 3.5.1, The R Foundation for Statistical Computing, Vienna, Austria) and RStudio (version 1.1.456, RStudio Inc., Boston, USA). The software packages used included lme4 (v. 1.1.21), lsmeans (v. 2.30.0) multcomp (v. 1.4.10), and multcompView (v. 0.1.7). The script can be found in the Supplementary Material 3. The cumulated amount of P in the sinks delivered from the bulk soil, residual soil, or WDC of the sites after 48 h were investigated by pairwise comparisons using the t-test statistics after testing of homogeneity of variances (Bartlett, 1937). The mean values of the pairwise comparisons were adjusted using the false discovery rate (FDR) (Benjamini and Hochberg, 1994). The kinetics of P accumulation in the DGTs after 24, 48, 72, 120, and 168 h were calculated and evaluated with SigmaPlot Version 12.0 (Systat Software GmbH, Erkrath, Germany). The transport of P from the P-source into the sink of the DGT device is controlled by two kinetic processes, the desorption of P from the solid phase (WDC, bulk soil or residual soil) into the aqueous phase and the diffusion of dissolved P (and colloidal P) through the aqueous phase into the DGT. Both kinetic processes result in a decreasing amount of P delivered to the DGTs per unit time with increasing duration of the experiment. We therefore decided to describe the delivery of P into the DGTs by means of an empirical first order law of velocity model that lumps the desorption rate constant and the diffusion rate constant into one effective parameter (Atkins and de Paula, 2013, p. 840):
With A0 as the total amount of P delivered into the P-sinks up to t = ∞ [μg], p as the release and transport rate of P [h−1] and t as the time of measurement [h]. We assume that the number of five points of time of our experiments would not have allowed the estimation of desorption rate constants and diffusion rate constants independently.
Results
Elemental Composition of Soils and Their Fractions
The largest Al- and Ca-concentrations were found for MAN soil (Table 2), while MIT soil showed the largest Fe-concentrations. The WDC of all three sites were enriched in the elements Al, Fe, and P compared to the bulk soil and the residual soil (WDC > bulk soil > residual soil). Most striking is the much stronger enrichment of Fe (WDC/bulk soil ratio of 13:1) and P (WDC/bulk soil ratio of 14:1) in the WDC of the sandy site LUE compared to the MAN soil (Fe ratio of 1.8:1, P ratio of 1.6:1) and MIT soil (Fe ratio of 2.1:1, P ratio of 2.8:1). Moreover, WDC had smaller C:N and C:P ratios than bulk soil and residual soil. While C was enriched in the WDC fraction of MIT and LUE in comparison to the bulk soil, a depletion of C was found for MAN. Analysis of the supernatants after centrifugation at 14,000 g revealed that 3.3% (LUE), 2.9% (MIT), and 0.6% (MAN) of WDC bound P got discarded during WDC extraction.
WDC Size Distribution
The mean size of the WDC particles was similar for the three sites with an average of 195 nm for MAN (median 188 nm), 188 nm for MIT (median 180 nm), and 195 nm for LUE (median 173 nm). However, the size distribution of particles differed between sites (Figure 1). The WDC size distribution was characterized by three main peaks, one around 50 nm particle size, one around 100–150 nm particle size and one around 200–250 nm particle size. The share of the largest size fraction of the particles decreased in the order MAN > MIT > LUE. The WDC of LUE contained the largest concentration of the medium-sized collodial particles. Data from NTA can be found in the Supplementary Material 1.
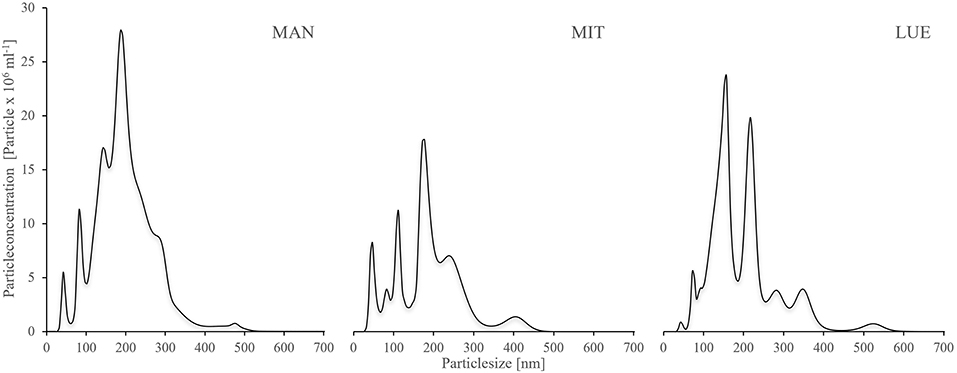
Figure 1. Size distribution of the WDC extracted from the topsoils of the sites Mangfall (MAN), Mitterfels (MIT), and Lüss (LUE), determined using Nanoparticle Tracking Analysis (NTA). NTA is capable of measuring natural colloids >50 nm, thus smaller particles were not detected. Size distribution showed three main peaks across all three sites, one around 50 nm, one between 100–150 nm and one around 200–250 nm. Most larger particles were found at MAN, followed by MIT and LUE. A tabular list of particle sizes and particle concentrations is given in the Supplementary Material 1.
Delivery of P Into the DGTs
The largest amounts of P were found in the DGTs in contact with the ortho-phosphate—solution of the positive control (24 h: 37.3 μg; 48 h: 51.2 μg, 47.7 μg, 44.6 μg; 72 h: 40.18 μg; 120 h: 60.1 μg; and 168 h: 63.0 μg). The regression models of the P-supply into the DGTs and the statistical evaluation of the DGT measurements after 48 h revealed differences in the supply of P from the bulk soils, WDC and residual soils of the different sites into the DGTs (MAN < MIT < LUE) (Figure 2 and Table 3). Furthermore, the order of the rates of P–release and –transport from the three P-sources into the DGTs varied between sites. While P-release and –transport increased in the order WDC < residual soil < bulk soil for MAN, the order was residual soil < bulk soil = WDC for MIT, and residual soil < bulk soil < WDC for LUE. Multiple pairwise comparisons of the cumulated P quantities in the DGTs after 48 h between the P-sources showed no significant differences (p < 0.05) at the MAN site. However, significant differences between residual soil on the one hand and bulk soil and WDC on the other hand at the MIT site, and significantly different rates of P-release and -transport between all fractions at the LUE site (Table 3) were observed. The WDC of the P-rich, calcareous MAN site supplied the smallest P quantities in the experiment both over 48 h and the entire (168 h) measurement period. In contrast, the largest P quantities from P-sources derived from soil were delivered by the WDC of the LUE site, which after 48 h supplied on average 74 times the quantity of P as the WDC of the MAN site (Table 3). In addition to the highest cumulated P quantities, the regression model for the P-release and –transport from the WDC of the LUE site into the DGTs indicated no decrease in the P-replenishment rate over the experimental time span of 168 h (Figure 2). Furthermore, when disassembling the DGTs in contact with the WDC of the LUE site a dark brown suspension between diffusion gel and filter membrane and a coloration of the diffusion gel (Figure 3), as well as a dark brown coloration of the nutrient sinks themselves (Figure 4) were recognized. These colorations also occurred to a small extent in the gel layers and nutrient sinks that were in contact with the WDC fraction of the MIT site, while they were absent in gel layers and nutrient sinks that were in contact with the WDC fraction of the MAN site. The elemental analyzes of the diffusion layers after 168 h exposure time to the P-sources by ICP-OES after microwave-assisted extraction revealed that gels used for bulk soil and the colloid-free soil residuals from the calcareous site MAN contained the largest amount of Ca in the experimental setup, while no Ca was traceable in the gels in contact with WDC from this site (Table 4). Furthermore, Al and Fe were absent in the diffusion layer in contact with WDCs extracted from MAN. In contrast, the highest amounts of Al and Fe were detected in the colored gel of the WDC from the LUE site, while the WDCs from the Fe-rich MIT site contained in comparison only marginal amounts of Fe in the gel.
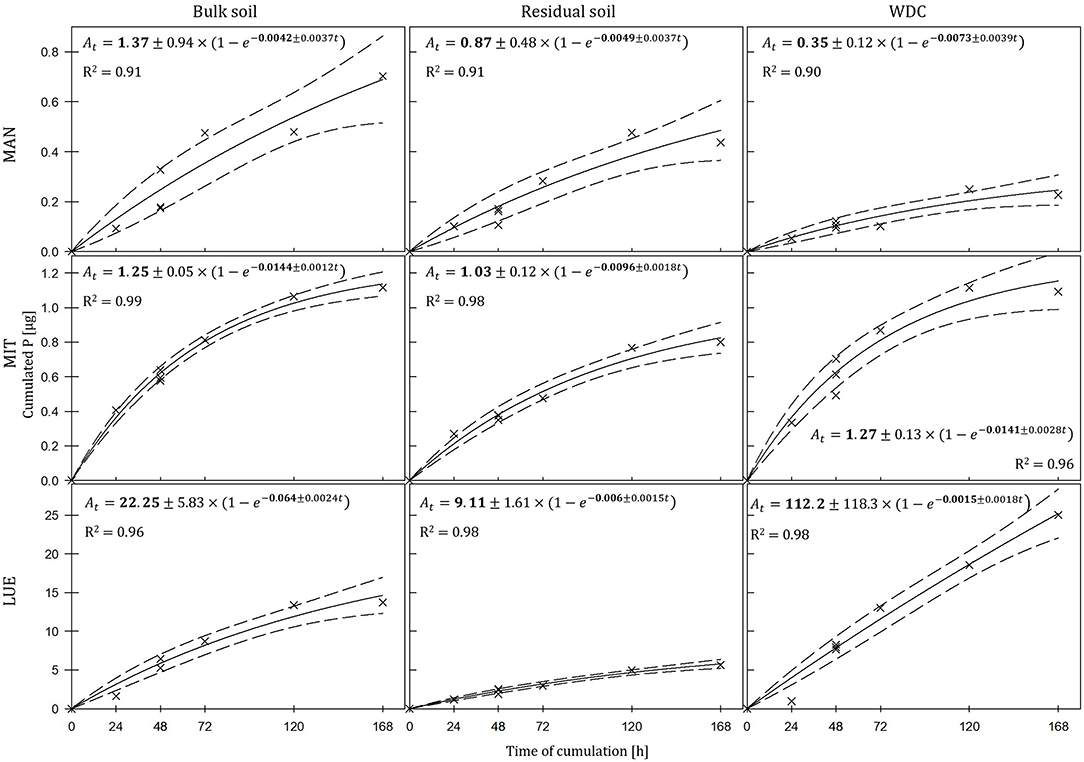
Figure 2. Kinetics of P-delivery by bulk soil, residual soil, and WDC of the sites Mangfall (MAN), Mitterfels (MIT), and Lüss (LUE). P quantities cumulated in DGTs after 24, 48, 72, 120, and 168 h are displayed with crosshairs, the regression models with lines and the corresponding 95% confidence intervals with dashed lines. Note the different scaling of y-axes for the three sites. The estimated parameters are shown in bold ± their standard error.
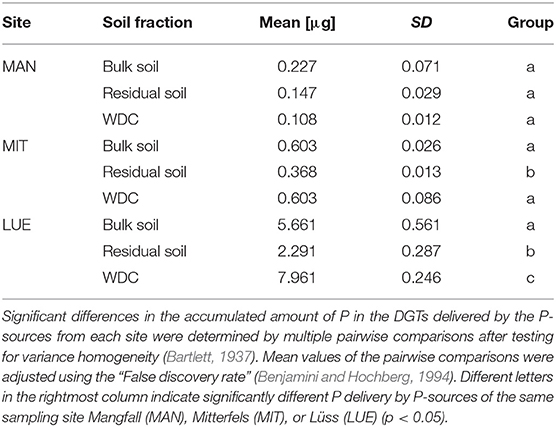
Table 3. Mean values and standard deviations of P delivered into the DGTs within 48 h by different P-sources (n = 3).
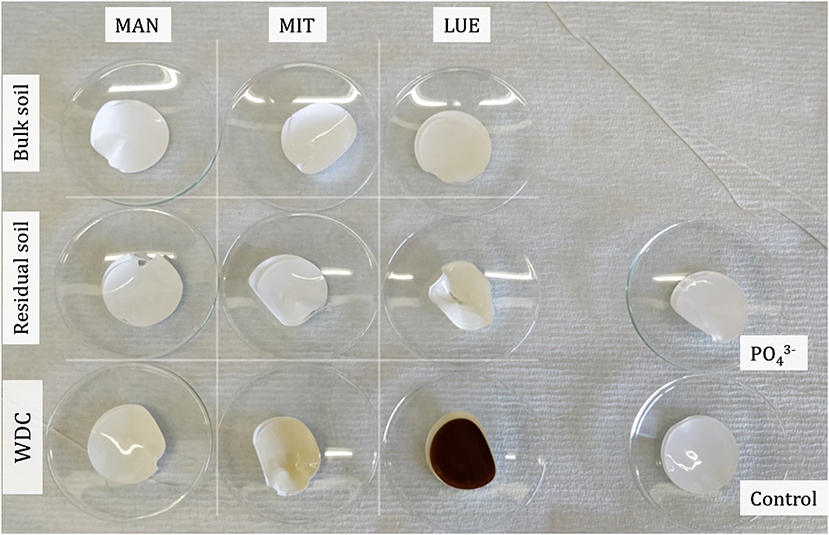
Figure 3. Diffusion gels and filter membranes of the disassembled DGTs after 168 h accumulation time of all P-sources and sites. The diffusion gel of the WDC from the site Lüss (LUE) showed a strong coloration due to the diffusion of WDC into the gel.
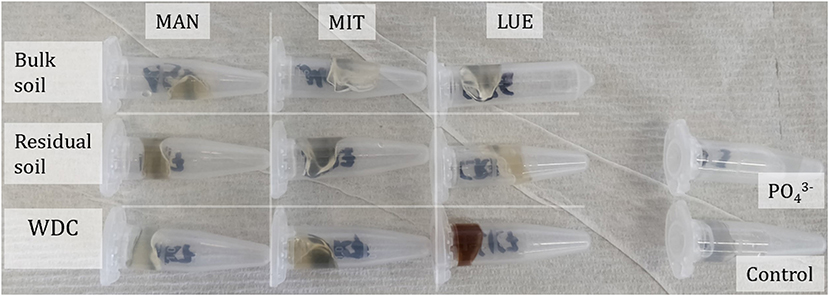
Figure 4. Ferrihydrite gel nutrient sinks of the disassembled DGTs after 168 h accumulation time of all soil fractions and sites following their extraction with sulfuric acid. The nutrient sink of WDC of the site Lüss (LUE) showed a strong coloration due to the diffusion of WDC into the sink.
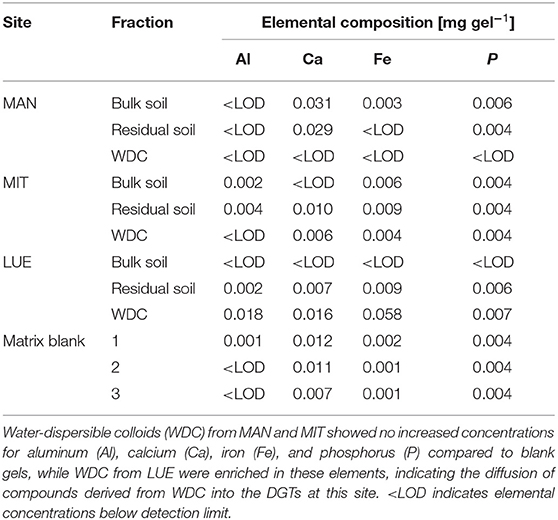
Table 4. Elemental composition of diffusion layer gels after 168 h in contact with the P-sources of the sites Mangfall (MAN), Mitterfels (MIT), and Lüss (LUE) in mg gel−1.
Discussion
P-Release From the Solid Phase of the Different Soils and Soil Fractions
The delivery of P to the DGTs by different P-sources reflected the speciation, binding, and solubility of P in the soils. Prietzel et al. (2016) demonstrated with X-ray absorption near-edge structure spectroscopy (XANES) that in the Ah horizon of the calcareous MAN site, P is present mainly in organic form (either bound to Ca, most likely as precipitate of Ca and inositol hexakisphosphate or as unsorbed organic P), as well as in inorganic form bound to Al-(hydr)oxides. These P-forms show low solubility (precipitates) or desorbability [Al-(hydr)oxides] at the given pH-value of 7.0, resulting in marginal amounts of P transported into the sinks compared to MIT and LUE. The sandy soil of the LUE site features a low sorption capacity for inorganic P (Lang et al., 2017). In line with this limited P sorption capacity, a large fraction of water-soluble P of most likely microbial origin was detected for the Ae horizon at LUE (Lang et al., 2017). Both, the limited P sorption capacity of the soil material and the large fraction of water-soluble P likely promoted the delivery of P by the LUE bulk soil in the DGTs.
Delivery of P Bound to WDC Into the DGTs
Water-dispersible colloids from the P-poor LUE site delivered significantly more P into the DGTs than the bulk soil or the colloid-free residual P-source. The almost linear increase in cumulated P over time from the LUE WDC indicated the immediate compensation of the depleted P concentration in the pore water surrounding the DGTs. This phenomenon was not observed for any other soil fraction at the other sites. Noteworthy, the amount of P cumulated over the measurement period of 168 h in the DGTs in contact with the LUE WDC exceeded the P-capacity of the DGTs of 6.7 μg (Zhang et al., 1998). Although P can be sorbed to the ferrihydrite P-sink above this P-capacity, the ferrihydrite binding sites were already loaded to such extent that P-species were likely not immediately removed from the gel surrounding the ferrihydrite so that the rate of P-accumulation in the DGTs should have decreased over time (Davison and Zhang, 2012). Considering the distinctly increased Fe and P content in the WDC fraction compared to the bulk soil at the site LUE, the measured P flux into the DGT, as well as the coloration of diffusion gel and nutrient sink inside the DGTs (Figures 3, 4), the intensive P delivery by LUE WDC could have been caused by mobile OM-Al(Fe)-P complexes (Gerke, 2010) dissolved organically-bound P (e.g., phosphate esters) and mobile OM-Al- and Fe-containing WDC (Jiang et al., 2017). This assumption is supported by the analysis of the diffusion gels using ICP-OES, showing the diffusion of Al, Ca, and Fe into the samplers. Because the gels were rinsed thoroughly before digestion, we assume that most measured complexes, molecules, and WDC have diffused into the gels and were not just attached to its surface. Since the diffusion layer and the nutrient sink use the same type of gel, P-containing compounds that diffused into the diffusion layer may have also diffused into the nutrient sink over time. It must be stated that the use of the colorimetric method might exclude a part of organic P transported into the DGT. The digestion of the gels with 0.25 M H2SO4 and the malachite green method however likely released most P bound to DOM (Baldwin, 1998), OM-Al/Fe complexes (Gerke, 2010), and colloids (Stainton, 1980) through hydrolysis and quantified it in addition to ortho-phosphate. Nevertheless, we likely underestimated the total amount of P translocated into the sinks.
The potential of the DGT technique to measure the transport of colloids into a nutrient sink depends on whether these compounds can diffuse through the filter membrane and the diffusive gel layer during the time of the experiment (Davison and Zhang, 2012). The polyethersulfone filter membrane used in the DGTs has a pore width of 450 nm and should be permeable for most WDC in our experiment. While (Zhang and Davison, 1999) stated the average pore size of polyacrylamide gels cross-linked with agarose derivative used for diffusive gel layers with radii >5 nm, van der Veeken et al. (2008) demonstrated the diffusion of latex particles with radii up to 129 nm into these gels. However, diffusion coefficients (Dc) strongly decrease with increasing spherical diameter of the particle according to the Stokes-Einstein equation. As a result, WDC particles or large molecules diffuse much slower than ions like ortho-phosphate. For example, Dc in water is 9.5 10−11 m2 s−1 for a spherical particle of 5 nm, 5.95 10−12 m2 s−1 for 80 nm, and 2.4 10−12 m2 s−1 for 200 nm diameter (T = 295.15 K, Supplementary Material 4). We used particle Dc to estimate the distance they could travel due to Brownian motion based on their “mean squared displacement.” The square root of this estimate indicated that very small particles of 5 nm diameter could have moved 10 mm on average during the 168 h of the experiment, much more than the thickness of the diffusion layer of 0.8 mm. Further calculations, now assuming an equal mix of 5, 80, and 200 nm sized colloids and a density of 2 g cm−3 for all particles estimate colloid concentrations to be in the order of 1.7–5.1 10−8 mol l−1. These concentrations could have been large enough to cause a potential diffusion of all added 5 nm colloids and even 21% of the added 80 nm diameter colloids across the diffusion layer into the DGT sink during the 168 h of our experiment (Supplementary Material 4). Hence, it appeared possible that the diffusion of mobile colloids was an important reason for the fast and constant delivery of P into the DGTs in contact with the LUE WDC.
Unfortunately the used particle tracking analysis is not able to quantify natural colloids <50 nm properly due to technical restrains (Gallego-Urrea et al., 2011). Therefore, a larger portion of particles smaller than 50 nm might have been present in the WDC of LUE than indicated by the NTA data of Figure 1. Overall, the results for the LUE site supported our hypothesis that colloids supply P more efficiently into a P sink than bulk soil or colloid-free residual soil.
Montalvo et al. (2015a,b) demonstrated that colloids and nanoparticles carry P to root surfaces, thereby contributing to the P supply and uptake of plants in hydroponic systems and under water-saturated soil conditions. However, especially in most agricultural soils except Hydragic Anthrosols (WRB, 2015, “paddy rice soils”), water-saturated conditions occur rarely and if, then only for very limited periods of time. Under more common unsaturated conditions the mobility of colloids and nanoparticles in porous media like soils is strongly reduced by interception of particles at water-air interfaces or in thin water films (e.g., Kretzschmar et al., 1999; Flury and Aramrak, 2017). The results of our experiment for the LUE site indicated that colloids can likely carry P to a sink also under water-unsaturated conditions.
Soil pH, WDC Composition, and P-Speciation as Drivers for WDC Mobility and P-Delivery
While the WDC from LUE delivered P at a quick rate, the results for MAN and MIT did not show increased P supply into the DGTs compared to the bulk soil. This suggested that soil properties, as well as the WDC properties controlled their mobility and the binding strength of WDC-P. Since the lowest P quantities were delivered by WDC from the calcareous site MAN and neither coloration of the DGT diffusive gels were visible nor Al, Ca, or Fe were detectable after digestion by ICP-OES, we assumed that the extracted MAN WDC lack OM-Fe-/Al-P complexes and small P-bearing colloids that contributed to the P-flux into the sinks at LUE. Wang et al. (2020) showed that in the Oh and Ah horizons of the MAN site, P was mainly bound to WDC >240 nm rich in Al and Si, which were likely formed from phyllosilicates (Missong et al., 2018a). These larger WDC were not able to diffuse into the sinks of the DGTs due to their size. Two reasons, high Ca2+ concentrations and a circumneutral pH value of the soil might limited the mobility of the small WDC from MAN and the P-release into the dissolved phase from these particles. Large concentrations of Ca2+ released from the MAN soil derived from dolomite rock might have promoted the flocculation of these clay mineral particles in the porous media. Flocculated WDC would not diffuse in the soil solution or with a much lower rate than single particles because of their larger hydrodynamic radius and mass (Banchio et al., 1999). Furthermore, flocculated WDC may have been retained in the pore space, which in turn may have changed the permeability of the porous medium (Molnar et al., 2019). Moreover, the pH value of 7.0 of the MAN bulk soil was closer to the point of zero charge of many soil components compared to the pH values of the acidic forest soils in MIT and LUE, which may have led to aggregation of the MAN WDC. Aggregation has been demonstrated for example for titanium nanoparticles in porous media at pH values close to the point of zero charge of the nanoparticles (Dunphy Guzman et al., 2006). Contrary to MAN, the WDC from the acidic forest soils of MIT and LUE were likely better dispersed at pH values much lower than the particles point of zero charge. The strong binding of (dissolved) organic matter to Fe- and Al-(hydr)oxides at low pH values (Kaiser et al., 1997) can promote the mobilization of particles (Kretzschmar et al., 1995; Philippe and Schaumann, 2014; Cheng and Saiers, 2015), thus enhancing also the mobility of P bound to these particles. The (weak) podzolic features of the LUE soil would support the theory of diffusion of OM-Fe/Al-P complexes into the DGTs. Missong et al. (2018b) found indications of a translocation of WDC-bound P from the topsoil to the Bsv horizon of the LUE soil. Similarly, Wood et al. (1984), Turner et al. (2012), Celi et al. (2013), and Wu et al. (2014) reported translocation of P during podzolization. However, according to Lang et al. (2017) podzolization was not promoting P transport to greater soil depths at the LUE site.
Although comparable amounts of Al and Fe were added with the WDC from the MIT soil as with WDC from the LUE soil to the quartz sand and silt mixture, 10 times less Fe was found in the diffusion layer of the DGT in contact with MIT colloids, and no Al was detectable. The larger Fe contents of the MIT WDC potentially reflected a stronger cementation of individual colloids, which might have reduced their mobility.
Conclusions
Our study demonstrates the enhanced delivery of P by colloids compared to bulk soil and colloid-free soil extraction residue into DGTs in a porous medium under unsaturated conditions. This underpins the relevance of natural colloids for the supply of soil P to plants. Therefore, leaching of these colloids from intensely rooted topsoils to deep subsoils with few roots, or the export of these particles with surface runoff or interflow can decrease the plant availability of P in terrestrial ecosystems. The magnitude and kinetics of colloid-facilitated P delivery likely depend on the mobility of colloids and the strength of the binding of P to the colloids. Building up on previous work on this issue we could confirm that the mobilization of P by and from mobile colloids plays a crucial role for DGT extraction of P.
Data Availability Statement
The original contributions presented in the study are included in the article/Supplementary Material, further inquiries can be directed to the corresponding author/s.
Author Contributions
RB, EK, and JS formulated the hypotheses. AK and JS conceived the experiment. RB, EK, and FL gave feedback regarding the concept and theory in all phases of the study. AK, BB, PR, and JS sampled the soils. AK, BB, and PR carried out the experiments. AK performed statistical analyses and wrote a first draft of the manuscript. All authors discussed the results and contributed to the final manuscript.
Funding
This study was funded in the framework of the Priority Program SPP 1685 Ecosystem Nutrition-Forest Strategies for Limited Phosphorus Resource of the Deutsche Forschungsgemeinschaft (DFG SI 1106/8-1, 2).
Conflict of Interest
The authors declare that the research was conducted in the absence of any commercial or financial relationships that could be construed as a potential conflict of interest.
The reviewer ES declared a past co-authorship with one of the author RB to the handling Editor.
Acknowledgments
Thanks to Astrid Jäger, Diedrich Steffens, and Caroline Löw for their comments that helped to improve the experimental design. We also thank Elke Müller, Elke Schneidenwind, and Ann-Kathrin Nimführ for their technical assistance in the laboratory, as well as Jan Wolff for the provision of resin-P data for the topsoil at the Mangfall (MAN) site. We gratefully acknowledge the help of Daniel F. Kaiser, Sebastian Loeppmann, Marius Schmitt, and Jaane Krüger during the sampling of soils. Without the help of Daniel F. Kaiser and Linda Vogt we never would have been able to extract many kilograms of soil for the isolation of colloids. Big thanks to Jaane Krüger also for organizing and administrating our collaboration.
Supplementary Material
The Supplementary Material for this article can be found online at: https://www.frontiersin.org/articles/10.3389/ffgc.2020.577364/full#supplementary-material
Abbreviations
Al, aluminum; C, carbon; Ca, calcium; Co, cobalt; DGT, Diffusive Gradients in Thin-Films; DOM, dissolved organic matter; FDR, false discovery rate; Fe, iron; ICP-OES, inductively coupled plasma optical emission spectrometry; LUE, Lüss; MAN, Mangfall; MIT, Mitterfels; NTA, nanoparticle tracking analysis; OM, organic matter; P, phosphorus; Si, silicon; WDC, water-dispersible colloids.
References
Alef, K., (ed.). (1998). Methods in Applied Soil Microbiology and Biochemistry. London: Academic Press.
Atkins, P. W., and de Paula, J. (2013). Physikalische Chemie (5. Auflage). Weinheim: WileyVCH Verlag GmbH and Co. KGaA.
Baldwin, D. S. (1998). Reactive “organic” phosphorus revisited. Water Res. 32, 2265–2270. doi: 10.1016/S0043-1354(97)00474-0
Banchio, A. J., Nägele, G., and Bergenholtz, J. (1999). Viscoelasticity and generalized Stokes–Einstein relations of colloidal dispersions. J. Chem. Phys. 111, 8721–8740. doi: 10.1063/1.480212
Bartlett, M. S. (1937). Properties of sufficiency and statistical tests. Proc. R. Soc. 160, 268–282. doi: 10.1098/rspa.1937.0109
Benjamini, Y., and Hochberg, Y. (1994). Controlling the false discovery rate: a practical and powerful approach to multiple testing. J. R. Stat. Soc. Ser. B 57, 289–300.
Bol, R., Julich, D., Brödlin, D., Siemens, J., Kaiser, K., Dippold, M. A., et al. (2016). Dissolved and colloidal phosphorus fluxes in forest ecosystems-an almost blind spot in ecosystem research. J. Plant Nutr. Soil Sci. 179, 425–438. doi: 10.1002/jpln.201600079
Bollyn, J., Castelein, L., and Smolders, E. (2019). Fate and bioavailability of phosphorus loaded to iron oxyhydroxide nanoparticles added to weathered soils. Plant Soil 438, 297–311. doi: 10.1007/s11104-019-04008-x
Bollyn, J., Faes, J., Fritzsche, A., and Smolders, E. (2017). Colloidal-bound polyphosphates and organic phosphates are bioavailable: a nutrient solution study. J. Agric. Food Chem. 65, 6762–6770. doi: 10.1021/acs.jafc.7b01483
Celi, L., Cerli, C., Turner, B. L., Santoni, S., and Bonifacio, E. (2013). Biogeochemical cycling of soil phosphorus during natural revegetation of Pinus sylvestris on disused sand quarries in Northwestern Russia. Plant Soil 367, 121–134. doi: 10.1007/s11104-013-1627-y
Cheng, T., and Saiers, J. E. (2015). Effects of dissolved organic matter on the co-transport of mineral colloids and sorptive contaminants. J. Contam. Hydrol. 177–178, 148–157. doi: 10.1016/j.jconhyd.2015.04.005
Dalkmann, P., Siebe, C., Amelung, W., Schloter, M., and Siemens, J. (2014). Does long-term irrigation with untreated wastewater accelerate the dissipation of pharmaceuticals in soil? Environ. Sci. Technol. 48, 4963–4970. doi: 10.1021/es501180x
Davison, W., and Zhang, H. (2012). Progress in understanding the use of diffusive gradients in thin films (DGT) – back to basics. Environ. Chem. 9, 1–13. doi: 10.1071/EN11084
de Jonge, L. W., Moldrup, P., Rubaek, G. H., Schelde, K., and Djurhuus, J. (2004). Particle leaching and particle-facilitated transport of phosphorus at field scale. Vadose Zone J. 3, 462–470. doi: 10.2113/3.2.462
Degryse, F., Smolders, E., Zhang, H., and Davison, W. (2009). Predicting availability of mineral elements to plants with the DGT technique: a review of experimental data and interpretation by modelling. Environ. Chem. 6, 198–218. doi: 10.1071/EN09010
Dunphy Guzman, K. A., Finnegan, M. P., and Banfield, J. F. (2006). Influence of surface potential on aggregation and transport of titania nanoparticles. Environ. Sci. Technol. 40, 7688–7693. doi: 10.1021/es060847g
Elser, J. J., Bracken, M. E. S., Cleland, E. E., Gruner, D. S., Harpole, W. S., Hillebrand, H., et al. (2007). Global analysis of nitrogen and phosphorus limitation of primary producers in freshwater, marine and terrestrial ecosystems. Ecol. Lett. 10, 1135–1142. doi: 10.1111/j.1461-0248.2007.01113.x
Flury, M., and Aramrak, S. (2017). Role of air-water interfaces in colloid transport in porous media: a review: air-water interfaces and colloid transport. Water Resour. Res. 53, 5247–5275. doi: 10.1002/2017WR020597
Gallego-Urrea, J. A., Tuoriniemi, J., and Hassellöv, M. (2011). Applications of particle-tracking analysis to the determination of size distributions and concentrations of nanoparticles in environmental, biological and food samples. TrAC Trends Analyt. Chem. 30, 473–483. doi: 10.1016/j.trac.2011.01.005
Gerke, J. (2010). Humic (organic matter)-Al(Fe)-phosphate complexes: an underestimated phosphate form in soils and source of plant-available phosphate. Soil Sci. 175, 417–425. doi: 10.1097/SS.0b013e3181f1b4dd
Gottselig, N., Amelung, W., Kirchner, J. W., Bol, R., Eugster, W., Granger, S. J., et al. (2017a). Elemental composition of natural nanoparticles and fine colloids in european forest stream waters and their role as phosphorus carriers: colloids in european forest streams. Glob. Biogeochem. Cycles 31, 1592–1607. doi: 10.1002/2017GB005657
Gottselig, N., Nischwitz, V., Meyn, T., Amelung, W., Bol, R., Halle, C., et al. (2017b). Phosphorus binding to nanoparticles and colloids in forest stream waters. Vadose Zone J. 16, 1–12. doi: 10.2136/vzj2016.07.0064
Gottselig, N., Sohrt, J., Uhlig, D., Nischwitz, V., Weiler, M., and Amelung, W. (2020). Groundwater controls on colloidal transport in forest stream waters. Sci. Total Environ. 717:134638. doi: 10.1016/j.scitotenv.2019.134638
Hathaway, J. C. (1956). Procedure for clay mineral analyses used in the sedimentary petrology laboratory of the U.S. geological survey. Clay Min. 3, 8–13. doi: 10.1180/claymin.1956.003.15.05
Haygarth, P. M., Warwick, M. S., and House, W. A. (1997). Size distribution of colloidal molybdate reactive phosphorus in river waters and soil solution. Water Res. 31, 439–448. doi: 10.1016/S0043-1354(96)00270-9
Heathwaite, L., Haygarth, P., Matthews, R., Preedy, N., and Butler, P. (2005). Evaluating colloidal phosphorus delivery to surface waters from diffuse agricultural sources. J. Environ. Qual. 34, 287–298. doi: 10.2134/jeq2005.0287a
Ilg, K., Siemens, J., and Kaupenjohann, M. (2005). Colloidal and dissolved phosphorus in sandy soils as affected by phosphorus saturation. J. Environ. Qual. 34, 926–935. doi: 10.2134/jeq2004.0101
Jacobsen, O. H., Moldrup, P., Larsen, C., Konnerup, L., and Petersen, L. W. (1997). Particle transport in macropores of undisturbed soil columns. J. Hydrol. 196, 185–203. doi: 10.1016/S0022-1694(96)03291-X
Jiang, X., Bol, R., Cade-Menun, B. J., Nischwitz, V., Willbold, S., Bauke, S. L., et al. (2017). Colloid-bound and dissolved phosphorus species in topsoil water extracts along a grassland transect from Cambisol to Stagnosol. Biogeosciences 14, 1153–1164. doi: 10.5194/bg-14-1153-2017
Jiang, X., Bol, R., Nischwitz, V., Siebers, N., Willbold, S., Vereecken, H., et al. (2015). Phosphorus containing water dispersible nanoparticles in arable soil. J. Environ. Qual. 44, 1772–1781. doi: 10.2134/jeq2015.02.0085
Jonard, M., Fürst, A., Verstraeten, A., Thimonier, A., Timmermann, V., Potočić, N., et al. (2015). Tree mineral nutrition is deteriorating in Europe. Glob. Change Biol. 21, 418–430. doi: 10.1111/gcb.12657
Kaiser, K., Guggenberger, G., Haumaier, L., and Zech, W. (1997). Dissolved organic matter sorption on sub soils and minerals studied by 13C-NMR and DRIFT spectroscopy. Eur. J. Soil Sci. 48, 301–310. doi: 10.1111/j.1365-2389.1997.tb00550.x
Kretzschmar, R., Borkovec, M., Grolimund, D., and Elimelech, M. (1999). Mobile subsurface colloids and their role in contaminant transport. Adv. Agron. 66, 121-193. doi: 10.1016/s0065-2113(08)60427-7
Kretzschmar, R., Robarge, W. P., and Amoozegar, A. (1995). Influence of natural organic matter on colloid transport through saprolite. Water Resour. Res. 31, 435–445. doi: 10.1029/94WR02676
Kruse, J., Abraham, M., Amelung, W., Baum, C., Bol, R., Kühn, O., et al. (2015). Innovative methods in soil phosphorus research: a review. J. Plant Nutr. Soil Sci. 178, 43–88. doi: 10.1002/jpln.201400327
Lang, F., Bauhus, J., Frossard, E., George, E., Kaiser, K., Kaupenjohann, M., et al. (2016). Phosphorus in forest ecosystems: new insights from an ecosystem nutrition perspective. J. Plant Nutr. Soil Sci. 179, 129–135. doi: 10.1002/jpln.201500541
Lang, F., Krüger, J., Amelung, W., Willbold, S., Frossard, E., Bünemann, E. K., et al. (2017). Soil phosphorus supply controls P nutrition strategies of beech forest ecosystems in central Europe. Biogeochemistry 136, 5–29. doi: 10.1007/s10533-017-0375-0
Li, F., Liang, X., Li, H., Jin, Y., Jin, J., He, M., et al. (2020). Enhanced soil aggregate stability limits colloidal phosphorus loss potentials in agricultural systems. Environ. Sci. Eur. 32:17. doi: 10.1186/s12302-020-0299-5
Mason, S. D., McLaughlin, M. J., Johnston, C., and McNeill, A. (2013). Soil test measures of available P (Colwell, resin and DGT) compared with plant P uptake using isotope dilution. Plant Soil 373, 711–722. doi: 10.1007/s11104-013-1833-7
Missong, A., Bol, R., Nischwitz, V., Krüger, J., Lang, F., Siemens, J., et al. (2018a). Phosphorus in water dispersible-colloids of forest soil profiles. Plant Soil 427, 71–86. doi: 10.1007/s11104-017-3430-7
Missong, A., Bol, R., Willbold, S., Siemens, J., and Klumpp, E. (2016). Phosphorus forms in forest soil colloids as revealed by liquid-state 31 P-NMR. J. Plant Nutr. Soil Sci. 179, 159–167. doi: 10.1002/jpln.201500119
Missong, A., Holzmann, S., Bol, R., Nischwitz, V., Puhlmann, H., v. Wilpert, K., et al. (2018b). Leaching of natural colloids from forest topsoils and their relevance for phosphorus mobility. Sci. Total Environ. 634, 305–315. doi: 10.1016/j.scitotenv.2018.03.265
Molnar, I. L., Pensini, E., Asad, M. A., Mitchell, C. A., Nitsche, L. C., Pyrak-Nolte, L. J., et al. (2019). Colloid transport in porous media: a review of classical mechanisms and emerging topics. Transp. Porous Med. 130, 129–156. doi: 10.1007/s11242-019-01270-6
Montalvo, D., Degryse, F., and McLaughlin, M. J. (2015a). Natural colloidal P and its contribution to plant P uptake. Environ. Sci. Technol. 49, 3427–3434. doi: 10.1021/es504643f
Montalvo, D., McLaughlin, M. J., and Degryse, F. (2015b). Efficacy of hydroxyapatite nanoparticles as phosphorus fertilizer in andisols and oxisols. Soil Sci. Soc. Am J. 79, 551–558. doi: 10.2136/sssaj2014.09.0373
Öztan, S., and Düring, R.-A. (2012). Microwave assisted EDTA extraction—determination of pseudo total contents of distinct trace elements in solid environmental matrices. Talanta 99, 594–602. doi: 10.1016/j.talanta.2012.06.042
Philippe, A., and Schaumann, G. E. (2014). Interactions of dissolved organic matter with natural and engineered inorganic colloids: a review. Environ. Sci. Technol. 48, 8946–8962. doi: 10.1021/es502342r
Prietzel, J., Klysubun, W., and Werner, F. (2016). Speciation of phosphorus in temperate zone forest soils as assessed by combined wet-chemical fractionation and XANES spectroscopy. J. Plant Nutr. Soil Sci. 179, 168–185. doi: 10.1002/jpln.201500472
Séquaris, J.-M., and Lewandowski, H. (2003). Physicochemical characterization of potential colloids from agricultural topsoils. Colloids Surfaces A 217, 93–99. doi: 10.1016/S0927-7757(02)00563-0
Six, L., Pypers, P., Degryse, F., Smolders, E., and Merckx, R. (2012). The performance of DGT versus conventional soil phosphorus tests in tropical soils - an isotope dilution study. Plant Soil 359, 267–279. doi: 10.1007/s11104-012-1192-9
Stainton, M. P. (1980). Errors in molybdenum blue methods for determining orthophosphate in freshwater. Can. J. Fish. Aquat. Sci. 37, 472–478. doi: 10.1139/f80-061
Turner, B. L., Condron, L. M., Wells, A., and Andersen, K. M. (2012). Soil nutrient dynamics during podzol development under lowland temperate rain forest in New Zealand. Catena 97, 50–62. doi: 10.1016/j.catena.2012.05.007
van der Veeken, P. L. R., Pinheiro, J. P., and van Leeuwen, H. P. (2008). Metal speciation by DGT/DET in colloidal complex systems. Environ. Sci. Technol. 42, 8835–8840. doi: 10.1021/es801654s
Van Veldhoven, P. P., and Mannaerts, G. P. (1987). Inorganic and organic phosphate measurements in the nanomolar range. Analyt. Biochem. 161, 45–48. doi: 10.1016/0003-2697(87)90649-X
Vitousek, P. M., Porder, S., Houlton, B. Z., and Chadwick, O. A. (2010). Terrestrial phosphorus limitation: mechanisms, implications, and nitrogen–phosphorus interactions. Ecol. Appl. 20, 5–15. doi: 10.1890/08-0127.1
Wang, L., Missong, A., Amelung, W., Willbold, S., Prietzel, J., and Klumpp, E. (2020). Dissolved and colloidal phosphorus affect P cycling in calcareous forest soils. Geoderma 375:114507. doi: 10.1016/j.geoderma.2020.114507
Watson, J. E. M., Evans, T., Venter, O., Williams, B., Tulloch, A., Stewart, C., et al. (2018). The exceptional value of intact forest ecosystems. Nat. Ecol. Evol. 2, 599–610. doi: 10.1038/s41559-018-0490-x
Wood, T., Bormann, F. H., and Voigt, G. K. (1984). Phosphorus cycling in a northern hardwood forest: biological and chemical control. Science 223, 391–393. doi: 10.1126/science.223.4634.391
WRB (2015). World Reference Base for Soil Resources 2014, Update 2015. International soil classification system for naming soils and creating legends for soil maps. World soil resources reports. FAO, Rome.
Wu, Y., Prietzel, J., Zhou, J., Bing, H., Luo, J., Yu, D., et al. (2014). Soil phosphorus bioavailability assessed by XANES and Hedley sequential fractionation technique in a glacier foreland chronosequence in Gongga Mountain, Southwestern China. Sci. China Earth Sci. 57, 1860–1868. doi: 10.1007/s11430-013-4741-z
Zhang, H., and Davison, W. (1999). Diffusional characteristics of hydrogels used in DGT and DET techniques. Analyt. Chim. Acta 398, 329–340. doi: 10.1016/S0003-2670(99)00458-4
Keywords: plant nutrition, ecosystem nutrition, colloid-facilitated transport, cambisol, beech, DGT technique, phosphorus, soil
Citation: Konrad A, Billiy B, Regenbogen P, Bol R, Lang F, Klumpp E and Siemens J (2021) Forest Soil Colloids Enhance Delivery of Phosphorus Into a Diffusive Gradient in Thin Films (DGT) Sink. Front. For. Glob. Change 3:577364. doi: 10.3389/ffgc.2020.577364
Received: 29 June 2020; Accepted: 22 December 2020;
Published: 20 January 2021.
Edited by:
Jennifer L. Soong, Colorado State University, United StatesCopyright © 2021 Konrad, Billiy, Regenbogen, Bol, Lang, Klumpp and Siemens. This is an open-access article distributed under the terms of the Creative Commons Attribution License (CC BY). The use, distribution or reproduction in other forums is permitted, provided the original author(s) and the copyright owner(s) are credited and that the original publication in this journal is cited, in accordance with accepted academic practice. No use, distribution or reproduction is permitted which does not comply with these terms.
*Correspondence: Alexander Konrad, YWxleGFuZGVyLmtvbnJhZCYjeDAwMDQwO3Vtd2VsdC51bmktZ2llc3Nlbi5kZQ==