- 1Department of Biology, University of Mississippi, Oxford, MS, United States
- 2Department of Environmental Systems Science, ETH Zürich, Zurich, Switzerland
- 3Department of Biology, Boston University, Boston, MA, United States
- 4Department of Biology, West Virginia University, Morgantown, WV, United States
- 5University of Brasília, Brasília, Brazil
- 6Soil and Water Sciences Department, University of Florida, Gainesville, FL, United States
- 7Biodiversity Research Center, Academia Sinica, Taipei, Taiwan
- 8Biologia Vegetal Department, University of Campinas, Campinas, Brazil
- 9Department of Entomology and Plant Pathology, University of Arkansas, Fayetteville, AR, United States
- 10Department of Biology, Duke University, Durham, NC, United States
Introductions and invasions by fungi, especially pathogens and mycorrhizal fungi, are widespread and potentially highly consequential for native ecosystems, but may also offer opportunities for linking microbial traits to their ecosystem functions. In particular, treating ectomycorrhizal (EM) invasions, i.e., co-invasions by EM fungi and their EM host plants, as natural experiments may offer a powerful approach for testing how microbial traits influence ecosystem functions. Forests dominated by EM symbiosis have unique biogeochemistry whereby the secretions of EM plants and fungi affect carbon (C) and nutrient cycling; moreover, particular lineages of EM fungi have unique functional traits. EM invasions may therefore alter the biogeochemistry of the native ecosystems they invade, especially nitrogen (N) and C cycling. By identifying “response traits” that favor the success of fungi in introductions and invasions (e.g., spore dispersal and germination) and their correlations with “effect traits” (e.g., nutrient-cycling enzymes) that can alter N and C cycling (and affect other coupled elemental cycles), one may be able to predict the functional consequences for ecosystems of fungal invasions using biogeochemistry models that incorporate fungal traits. Here, we review what is already known about how EM fungal community composition, traits, and ecosystem functions differ between native and exotic populations, focusing on the example of EM fungi associated with species of Pinus introduced from the Northern into the Southern Hemisphere. We develop hypotheses on how effects of introduced and invasive EM fungi may depend on interactions between soil N availability in the exotic range and EM fungal traits. We discuss how such hypotheses could be tested by utilizing Pinus introductions and invasions as a model system, especially when combined with controlled laboratory experiments. Finally, we illustrate how ecosystem modeling can be used to link fungal traits to their consequences for ecosystem N and C cycling in the context of biological invasions, and we highlight exciting avenues for future directions in understanding EM invasion.
Introduction
Invasive exotic species may greatly modify local biodiversity and ecosystem services worldwide (Vilà et al., 2011; Pyšek et al., 2012). Specifically, invasive species have the potential to (1) reduce biodiversity, (2) disrupt important co-evolved mutualisms and species coexistence (Bever et al., 2010), (3) alter ecosystem processes and the potential of ecosystem to provide services (Jackson et al., 2002), and (4) produce alternative ecosystem states that are resistant to ecological restoration (Suding et al., 2004). It is thus critical to understand the eco-evolutionary dynamics of invasions, and their consequences for biodiversity and ecosystem functions (Pecl et al., 2017; Bonebrake et al., 2018).
The roles of fungi in invasions have been relatively overlooked compared to the attention that plants and animals have received, despite the enormous scale of the occurrence and potential impacts of fungi (Desprez-Loustau et al., 2007; Gladieux et al., 2015). The well-known exceptions involve invasive pathogens, such as Dutch elm disease (Ophiostoma spp.) (Brasier and Buck, 2001) and chestnut blight (Cryphonectria parasitica) (Anagnostakis, 2001; Dutech et al., 2012), which have caused dieback of host trees in their exotic range. Non-pathogenic fungi such as mycorrhizal fungi associated with plant roots have been introduced extensively around the globe (Schwartz et al., 2006; Vellinga et al., 2009). In the case of ectomycorrhizal (EM) fungi, these introductions have typically been co-introductions with their host plants (Vellinga et al., 2009), and have frequently resulted in co-invasion by these fungi and their host plants into native ecosystems (Nuñez and Dickie, 2014), and occasionally separate invasion by the fungi via colonization of novel hosts among the native flora of the exotic range (Vellinga et al., 2009; Dickie et al., 2017). Although recent studies have begun to elucidate the consequences of some of these EM fungal invasions for the population dynamics of their invasive host plants (Nuñez et al., 2009; Hayward et al., 2015), we still understand very little about their consequences for the population and evolutionary dynamics of the fungi themselves, or for native communities and ecosystem functions. Here, we argue that introductions and invasions of non-pathogenic fungi such as EM fungi present opportunities for answering fundamental questions about biological invasions, as well as questions on the functional consequences of microbial composition, diversity, and traits.
Although ecologists have for decades been exploring links between diversity and function in ecological communities (Tilman et al., 1997; Loreau et al., 2001; Hooper et al., 2005), microbial ecologists increasingly highlight the value of microbial functional traits, rather than species composition or diversity per se, to predict ecosystem function (Nielsen et al., 2011; Crowther et al., 2014; Fierer et al., 2014; Krause et al., 2014; Talbot et al., 2014; Treseder and Lennon, 2015) and EM fungal invasions may offer a powerful way to test how soil fungal traits can explain critical ecosystem functions of microbial communities. In particular, the response-and-effect trait framework (Lavorel and Garnier, 2002; Suding et al., 2008) advocates identifying two groups of traits in communities: traits that determine the response of the community to environmental change (so-called “response traits,” often related to fecundity, regeneration, or dispersal), and traits that determine the effect of the community on ecosystem functions (so-called “effect traits,” often related to nutrient cycling or storage). Although this response-effect trait framework was largely developed around plant communities, it is hypothesized to apply well to soil fungi (Koide et al., 2014; Treseder and Lennon, 2015). To predict the ecosystem consequences of shifts in fungal community composition and diversity during biological invasions, we need to ask specifically how “response traits” that may favor success during introductions and invasions (e.g., traits related to spore dispersal and germination, or stress tolerance) may be linked to “effect traits” (e.g., production of unique nutrient-cycling enzymes) that may determine their impacts on native communities and ecosystem processes. For example, there may be tradeoffs among species in stress tolerance (affecting ability to survive transport during introduction) to the quality of litter produced [affecting resulting contributions to stable soil carbon (C)] (Crowther et al., 2015). Such tradeoffs may lead to introduced, exotic EM fungal communities having different suites of functional traits compared to those in their native source communities.
Rapid evolution is increasingly being recognized as a key driving force of ecological processes (Thompson, 2013; Hendry, 2016), but may be particularly important when microbial species are introduced into novel environments. Invaders may experience or exert particularly strong direct selection pressures (Palumbi, 2001; Colautti and Lau, 2015), not only due to a lack of coevolutionary history with species encountered in the introduced range (Callaway et al., 2005), but also owing to their escape from the evolutionary constraint of community complexity in their native environment (de Mazancourt et al., 2008; Strauss, 2014). Indeed, traits of invasive fungal pathogens, such as virulence and host compatibility, have been demonstrated to evolve rapidly in exotic populations, despite moderate levels of genetic diversity (Gladieux et al., 2015). Trait evolution in introduced and invading populations of mutualistic fungi (such as EM fungi), however, has not yet been demonstrated, even though such evolutionary shifts could substantially alter effects of these fungi on invaded communities and ecosystems. Studies of evolution in these exotic mycorrhizal fungal communities could teach us a great deal about fungal population biology and plant-microbe coevolution.
Co-introduction of Pines and EM Fungi Results in Unique, Low-Diversity Exotic Assemblages of EM Fungi
Pines (plant species in the genus Pinus) are largely native to the Northern Hemisphere, but exotic pine plantations now cover more than 5 million ha south of the Equator (Simberloff et al., 2010; Figure 1). After early attempts to establish pine plantations in the Southern Hemisphere in the 1800s and early 1900s failed, it was quickly discovered that pines, which are obligately associated with symbiotic EM fungi, cannot survive without soil inoculum from previously established nurseries or pine forests (Richardson et al., 1994). The introduction and inoculation process, in most cases, has greatly reduced diversity in the EM fungal community associated with pines, and only rarely are exotic pines in the Southern Hemisphere colonized by EM fungi native to their new locale, i.e., by EM fungi from non-pine host trees in the exotic range (Vellinga et al., 2009; Dickie et al., 2017). The diversity and composition of EM fungi found in exotic pine plantations is likely influenced by the local history of European colonization and commerce with Northern Hemisphere countries, as well as response traits of the fungi themselves that determine which taxa survive the ecological filtering of the introduction process (Dunstan et al., 1998), such as tolerance of desiccation during transport, tolerance of novel abiotic conditions, ability to initially colonize from spores, and ability to use novel species of Pinus as hosts.
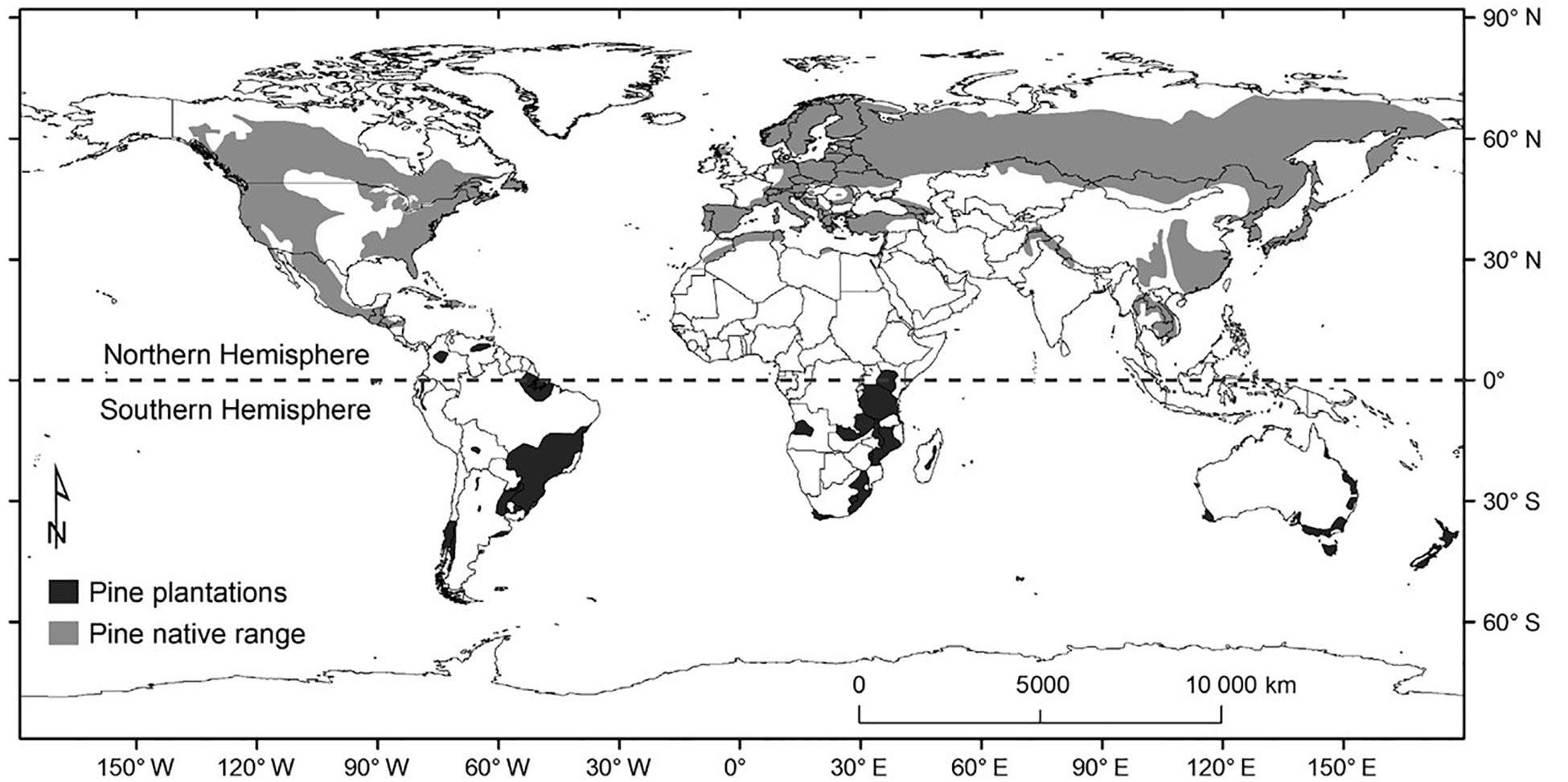
Figure 1. Native range of pines, and distribution of pine plantations in the Southern Hemisphere [map from Lantschner et al. (2017), used with permission from John Wiley and Sons (RightsLink License # 4847761041822)].
As a result, pine plantations in the Southern Hemisphere possess significantly impoverished assemblages of EM fungi, often fewer than 20 species (and as few as four, or even one for pine trees at the invasion front) at a single site (Chu-Chou and Grace, 1988; Walbert et al., 2010; Hynson et al., 2013; Hayward et al., 2015; Policelli et al., 2018), compared to native and managed populations of those same pines in the Northern Hemisphere, where they often associate with 100 or more species of EM fungi (Horton and Bruns, 2001). Thus, the enormous expansion of pine plantation forestry in the Southern Hemisphere has resulted in the global spread of a limited number of highly successful EM fungi species across the entire planet, far beyond their original native ranges (Figure 2). Moreover, the EM fungi that are co-introduced with exotic pines often represent fungal lineages novel to their new habitat. For example, conversion of grasslands to pine plantations may result in a shift in dominance among soil fungi from arbuscular mycorrhizal (AM) fungi (subphylum Glomeromycotina) to EM fungi (predominantly Basidiomycota), but pine-specific EM fungal lineages such as the Suilloid genera Suillus and Rhizopogon may still be novel when the recipient community is a non-pine EM-dominated forest, such as the Eucalyptus forests of southeastern Australia.
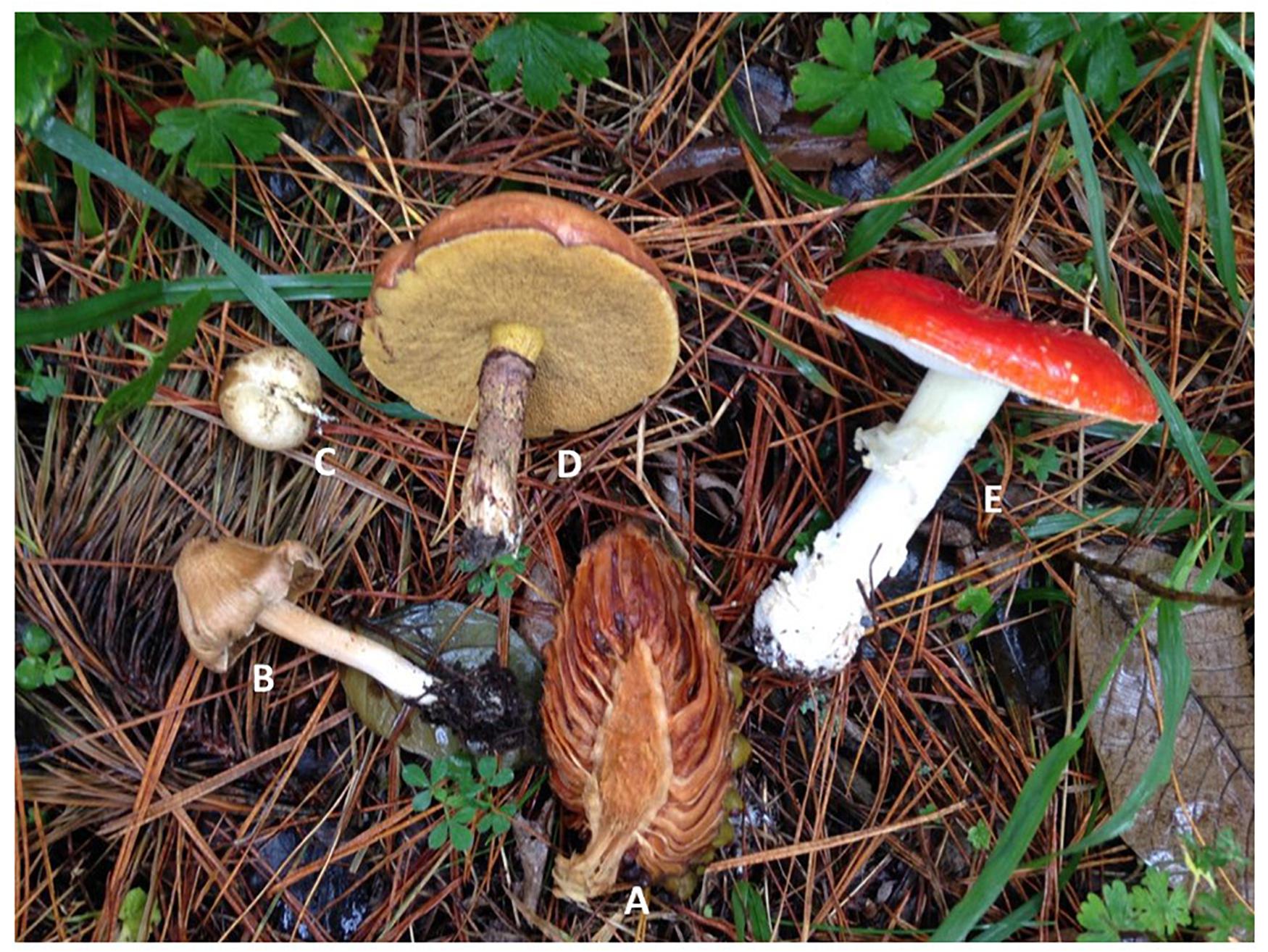
Figure 2. Ectomycorrhizal partners commonly co-occurring in exotic pine forests in Australia. (A) cone (split in half) of a common host plant, Pinus radiata, and four ectomycorrhizal fungi: (B) Inocybe sindonia, (C) Rhizopogon pseudoroseolus, (D) Suillus luteus, and (E) Amanita muscaria. Photo by R. Vilgalys, Blue Mountains near Sydney, Australia.
With the aid of particular EM fungi, some pine species often invade from plantations into nearby native habitats, while a lack of suitable EM fungal inoculum outside plantations hinders pine invasions at some sites (Nuñez et al., 2009). Specifically, it has been shown that certain lineages of EM fungi that facilitate early succession of pines in their native range, especially Suilloid fungi in the genera Suillus and Rhizopogon, seem uniquely suited to facilitate invasion of pines out of plantations in their exotic range (Hayward et al., 2015; Urcelay et al., 2017; Policelli et al., 2018). While in the native range Suilloid fungi typically represent less than 5% of observations of EM fungi on roots (Danielson, 1984; Gardes and Bruns, 1996), in the invasive range pines are sometimes able to invade out of plantations with no other fungi besides Suilloid species (Hayward et al., 2015; Policelli et al., 2018). These taxa have particular suites of response traits that are associated with their success during invasions: they produce abundant spores, which are effectively dispersed through biotic and abiotic vectors and which persist in soil and readily colonize invading pine seedlings outside of forests/plantations (Ashkannejhad and Horton, 2006; Urcelay et al., 2017; Policelli et al., 2018). In contrast, many EM fungi colonize plant roots mainly via mycelial growth from existing colonized roots, and as a result, they are rarely found on the roots of individual seedlings colonizing new habitat zones away from established plantations or forests (Horton and Bruns, 2001).
Within the species-poor fungal communities of pine plantations and invasions, fruiting body (“mushroom”) production by EM fungi is also dominated by a very small number of species, which often fruit much more abundantly than in their home range. A well-known example is Suillus luteus, which was estimated to produce hundreds of kilograms of dry mass per hectare in Pinus radiata plantations of Ecuador (Chapela et al., 2001), far exceeding the total fruiting biomass in many native pine habitats of the Northern Hemisphere. Indeed, S. luteus is one of the most abundant exotic EM mushrooms found under pines across the Southern Hemisphere (Nuñez et al., 2009). These instances of exceptional dominance may result from some combination of enemy release, competitive release due to reduced diversity of co-occurring EM fungi, plastic responses to novel environments, or rapid evolution. To our knowledge, none of these mechanisms have been tested. Regardless, the introduction and subsequent invasion of particular low-diversity suites of EM fungi may lead to cascading consequences at multiple scales, due to the effect traits of those particular fungi (Figure 3).
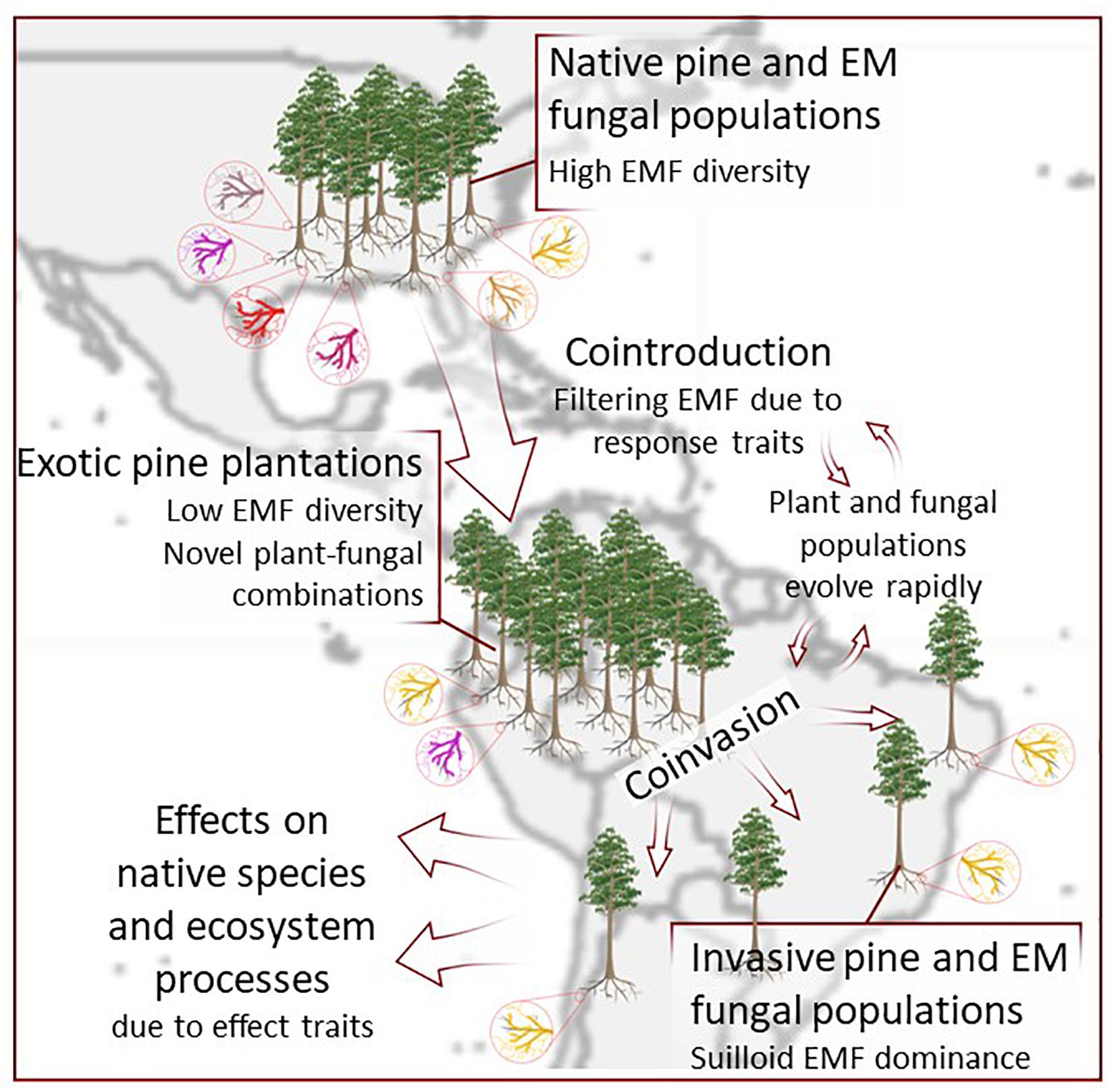
Figure 3. Introduction of pines and ectomycorrhizal fungi (EMF) leads to reduction of EMF diversity in exotic plantations compared to the native range, and novel combinations of pines and fungi from the Northern Hemisphere. Co-invasion out of plantations into native habitats leads to dominance of Suilloid EMF. Effect traits of EMF in plantations and invaded areas may drive effects for native species and ecosystems, and those effects may be modified by rapid evolution.
When pines and EM fungi are co-introduced and subsequently a subset co-invade into native habitats, the plants and EM fungi will affect each other’s population dynamics, including effects on invasion dynamics (Nuñez and Dickie, 2014; Dickie et al., 2017) and on populations of native species (Brewer et al., 2018). In addition, introduced and invading pines and EM fungi may each have direct effects on ecosystem functions, such as the coupled cycling of C, nitrogen (N), and phosphorus (P) (Townsend et al., 2011). Net ecosystem consequences of pine-EM fungal introductions and invasions have been explored (Scholes and Nowicki, 1998; Wilcke and Lilienfein, 2002; Simberloff et al., 2010; Dickie et al., 2014), but not yet using approaches that allow distinguishing direct effects of the pines themselves from effects of the co-introduced EM fungi. Here, we focus on the substantial consequences that plant-EM fungal co-introductions and co-invasions may have for ecosystem processes and show how these large-scale natural experiments can be opportunities to link fungal traits to their cascading consequences for ecosystem biogeochemical cycling.
Potential Effects of Ectomycorrhizal Introductions and Invasions on Biogeochemical Cycling
The cycling of C and nutrients such as N and P are often intimately coupled in ecosystems (Townsend et al., 2011), and previous work has demonstrated substantial effects of pine plantations and invasions on various soil and ecosystem functions, including C, N, and P cycling (Scholes and Nowicki, 1998; Simberloff et al., 2010). For example, the conversion of cerrado vegetation in Brazil to Pinus caribaea plantation initially resulted in increased storage of C and N in soil organic layers, increased N availability, increased C storage in aboveground and belowground standing biomass, and no changes in P storage (Lilienfein et al., 2000, 2001; Wilcke and Lilienfein, 2002; Lilienfein and Wilcke, 2003). Studies of invading P. nigra in New Zealand have also demonstrated increases in C storage in aboveground plant biomass, paralleling the Brazilian cerrado studies, but P. nigra invasion of grassland vegetation seems to cause a net loss in C from the mineral soil, as well as release of recalcitrant P (Dickie et al., 2011), echoing results from other studies of pine introductions across New Zealand and Australia (Scholes and Nowicki, 1998). P. contorta invasion in New Zealand has also increased P availability in soils, along with increased concentrations of nitrate-N (Dickie et al., 2014). Grassland afforestation with coniferous trees has generally increased organic matter mineralization and organic P availability in soils (Chen et al., 2008). Thus, studies to date are relatively consistent in showing that pine introductions initially result in increased litter inputs, aboveground C storage, and soil N and P availability, while effects on decomposition and soil C storage are not consistent, being largely dependent on the type of ecosystem being invaded.
Variation in effects on decay by EM plant-fungal invasion may be explained by the context-dependency of decomposition activities by EM fungi and the free-living saprotrophic microbes that they support. EM-dominated forests may differ from AM-dominated forests in their nutrient economies, especially for C and N cycling (Orwin et al., 2011; Averill et al., 2014, 2018; Sulman et al., 2017; Craig et al., 2018; Liese et al., 2018; Cotrufo et al., 2019; Zak et al., 2019) and also for P cycling (Rosling et al., 2016). As less is known for P, we focus more here on potential consequences for C and N cycling. If true, introduction and invasion of EM plants and fungi into AM-dominated ecosystems has the potential to transform C and nutrient cycling in those systems in ways not predicted simply by changes in the plant species alone. However, the few studies to date have sometimes come to very different conclusions, such as whether EM-dominated forests store more or less C in soil organic matter (SOM) compared to AM-dominated forests. A key point of agreement, however, is that many EM fungi have (and AM fungi generally do not have) abilities to degrade SOM and take up organic N and P (Read and Perez-Moreno, 2003; Smith et al., 2018; Zak et al., 2019), potentially outcompeting and slowing decomposition by free-living saprobic fungi. Some field surveys and modeling studies suggest that this ability results in increased storage of C and slower N mineralization in EM forests (Orwin et al., 2011; Averill et al., 2014; Sulman et al., 2017), the so-called “N-mining” or “Slow-decay” hypothesis. This hypothesis represents an extension of what has been called the “Gadgil effect,” wherein EM roots were observed to suppress litter decomposition, a phenomenon originally observed in an exotic P. radiata plantation in New Zealand (Gadgil and Gadgil, 1971). In contrast, other studies (Craig et al., 2018) have found support for the idea that more rapid decomposition rates in AM forests result in enhanced production of mineral-protected microbial residues in mineral soils and thus increased C storage in AM forests, the so-called “Microbial Efficiency-Matrix Stabilization” or “MEMS” hypothesis. Attempts to reconcile these opposing conclusions have highlighted three key factors: (1) these processes are likely context-dependent, with the importance of EM-mediated soil N-mining declining with increased mineral N availability (Averill et al., 2018); (2) the two processes may predominate in different organic matter fractions and soil layers, with EM effects concentrated in particulate (as opposed to mineral-associated) organic matter (Cotrufo et al., 2019) and thus in more organic surface horizons (Craig et al., 2018); and (3) different phylogenetic lineages of EM fungi likely vary substantially in their effect traits such as degradative abilities, responses to soil N availability, and competitive effects on free-living saprobes (Zak et al., 2019).
Intimately linked with the unique biogeochemical capabilities of EM fungi are the quantity and chemical diversity of the secretions (such as degradative enzymes) produced by EM fungi and their forest tree host plants, which may represent key effect traits that have consequences for C and nutrient cycling in systems where these organisms are introduced. First, root secretions produced by different forest plant species may favor different mycorrhizal fungi and saprophytes (Ali and Jackson, 1988; Sun and Fries, 1992), which may contribute to the enrichment of specific EM fungi at plantation and invasion sites. For example, the flavonoid and phenolglycoside from Eucalyptus root exudates favor growth of S. bovinus but not Paxillus involutus (Jones et al., 2004), which could consequently amplify effects of secretions by the selected EM fungi.
Another potentially important effect trait of invasive EM fungi is their affinity or aversion to soil mineral N. Indeed, some Suillus species exhibit relatively high protease and peptidase activity compared to other EM fungi, and some polyphenol oxidase/peroxidase activity (Talbot and Treseder, 2010; Talbot et al., 2015), suggesting that they may compete with free-living saprobes in decomposition of SOM. However, Suilloid fungi (e.g., Suillus and Rhizopogon spp.) grow relatively poorly under low C:N conditions (Hatakeyama and Ohmasa, 2004) and are more sensitive to changes in C:N ratio than other EM fungi (Fransson et al., 2007). As a result, it may be that Suilloid fungi especially thrive and compete with free-living saprobes, suppressing the decomposition of soil C by free-living saprobes and stimulating productivity of their co-invading host plants, when exotic soil mineral N availability is low relative to soil C (i.e., high soil C:N) (Figure 4). In contrast, some other EM fungi commonly co-introduced with trees, such as P. involutus (Lilleskov et al., 2002), Thelephora terrestris (Lilleskov et al., 2002; Cox et al., 2010) and Scleroderma citrinum (van der Linde et al., 2018) appear to be nitrophilic, specialized to thrive under low C:N conditions or responding positively to atmospheric N deposition, and having more limited capabilities to access N in organic matter.
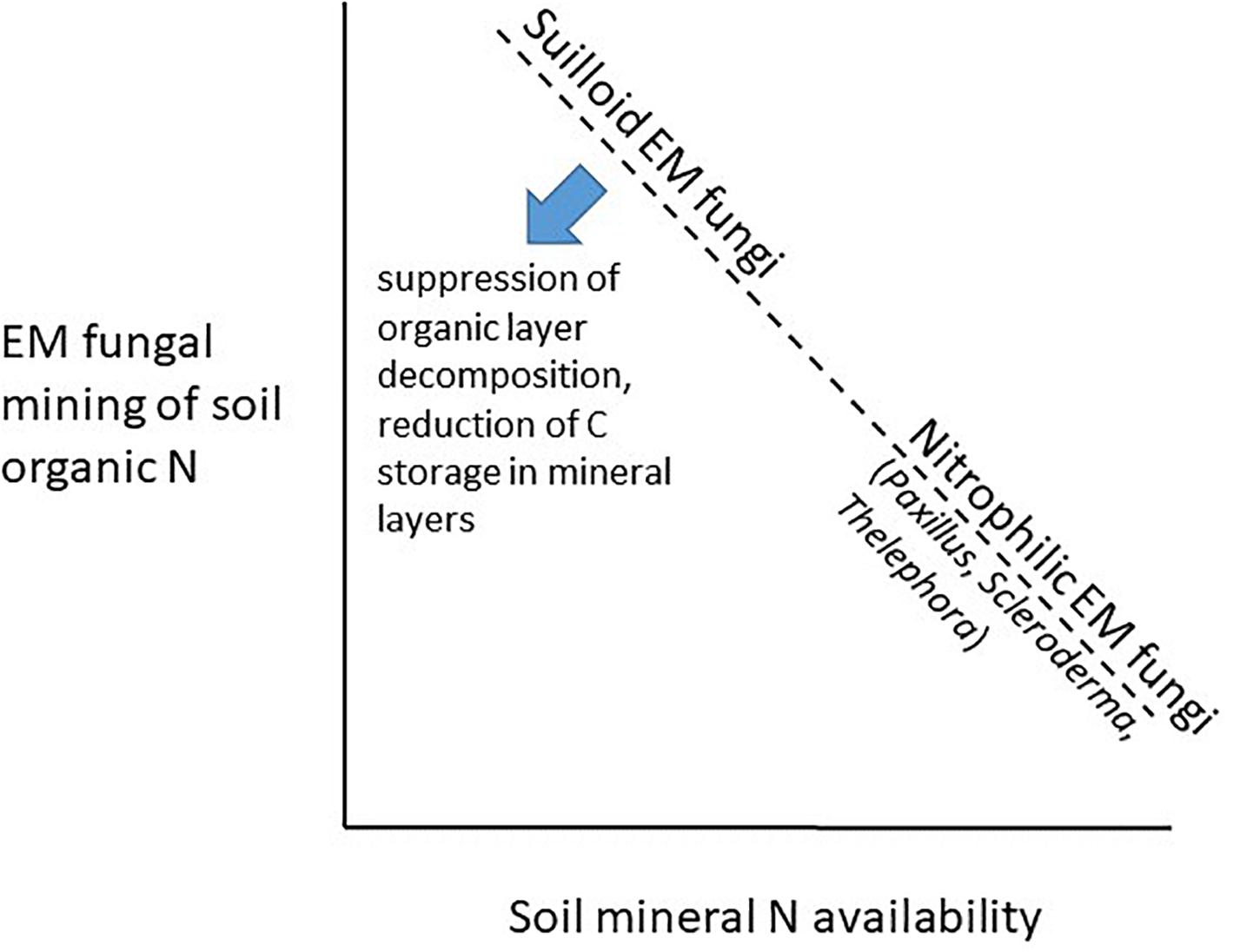
Figure 4. Hypothesized trade-off between soil organic N decomposition capability (an effect trait) and tolerance to high soil mineral N (i.e., being “nitrophilic,” a “response trait”), resulting in stronger predicted “Gadgil effect” of EM fungal suppression of decomposition by free-living saprobes (in surface organic soil layers) at sites where Suilloid EM fungi are favored by conditions of low soil mineral N availability, along with reduced C storage in mineral layers due to reduced production of mineral protected microbial residues.
These previous studies lead us to hypothesize that EM fungi may have invasion-biogeochemistry trait “syndromes” where species trade off soil organic N acquisition capabilities for tolerance to high soil mineral N (i.e., being “nitrophilic”). If so, soil conditions in the exotic range may interact with the response trait of nitrophobicity to alter the composition of the EM fungal community during introduction and invasion, which may in turn drive effects of the EM fungi on C and N cycling, due to the effect trait of organic N acquisition ability. As a result, the Slow-decay hypothesis would predict the “Gadgil effect” of EM fungal suppression of decomposition by free-living saprobes to especially predominate in surface organic soil layers and in particulate organic matter, at sites where Suilloid EM fungi are favored by conditions of low soil mineral N availability, however, the MEMS hypothesis would additionally predict reduced C storage in underlying mineral soils due to reduced production of mineral-protected microbial residues (Figure 4). The balance between these two processes happening in different soil layers would determine the net effect of introduced and invasive EM fungi on soil C and N cycling.
Compared with N cycling in temperate forest soils, less is known about EM fungal control over P cycling, although there is evidence that EM and AM associations may differ in their consequences for P dynamics in soils (Jansa et al., 2011; Rosling et al., 2016), with EM fungi capable of short-circuiting the soil P cycle by facilitating direct access by plants to organic P (bypassing saprobic organisms; Carleton and Read, 1991). As EM fungal species and genotypes differ in their P uptake patterns and efficiency (Jansa et al., 2011), changes in EM fungal species composition, richness, and diversity might also have impacts on P uptake efficiency for the plant hosts (Cairney, 2011). For example, P uptake efficiency has been found to decrease as a consequence of decreased EM fungal diversity under drought conditions (Köhler et al., 2018). EM fungal contributions to host P supply vary according to the relative ability of EM fungi to enhance host P acquisition, the availability of P in its different forms, edaphic conditions, and host P status (Cairney, 2011). When soil P is limiting, reductions in EM fungal hyphal growth and biomass might further negatively impact P acquisition by forest tree hosts (Teste et al., 2016). EM fungal species able to reach adsorbed P sources via abundant external mycelium growth and soil exploration capacity may have a competitive advantage in limited P conditions (Köhler et al., 2018). The extent and mechanisms to which EM fungal invasions may influence P acquisition by tree hosts in forest ecosystems, however, remains largely speculative. Even when P limitations could be counterbalanced by morphological and physiological responses of the plant hosts (Vance et al., 2003), replacing one mycorrhizal community by another might strongly affect soil P cycling with unknown consequences for the ecosystem.
Although EM fungal species in their native range may be classified based on their traits, such as nitrophobicity and ability to access organic N or P, rapid evolution of introduced and invading populations of EM fungi may modify their traits and resulting effects on ecosystem functions. For example, in species-rich native communities, stabilizing selection on foraging niches may prevent expansion of foraging niches. But if invading species experience a loss of competitors in their ecological guild, this stabilizing selection may be relaxed (Ackerly, 2003), resulting in evolution of expanded resource foraging niches in the exotic range (de Mazancourt et al., 2008). Moreover, admixture in the introduced range among historically isolated populations may provide novel genetic material that facilitates rapid adaptive evolution (Colautti and Lau, 2015). For EM fungi, novel selection pressures may include reduced diversity of natural enemies, reduced diversity of competing EM fungi, novel microbial enemies or competitors, different pine host species than in their native population, and novel soil properties. Some combination of these selection pressures may modify the effect traits of EM fungi, modifying their consequences for communities and ecosystem functions in recipient exotic habitats (Figure 3).
Using Plant-Fungal Introductions and Invasions to Test Hypotheses on the Ecosystem Consequences of EM Fungal Traits
Widespread biological introductions of plants and associated microbial communities, such as pines and their EM fungi, present an opportunity to answer a fundamental question in microbial ecology: To what extent do the traits of soil fungi predict their effects on C, N, and P cycling in novel ecosystems? A key challenge in addressing this question on the consequences of plant-fungal co-introductions and co-invasions will be separating the direct effects of the plants themselves, which may be substantial, from effects of the fungi per se. We suggest that this challenge can be addressed using a combination of field studies, laboratory experiments, and ecosystem modeling to incorporate fungal traits. Here, we briefly describe key characteristics of ideal field and laboratory studies, and then focus on illustrating the utility of ecosystem modeling, presenting new modeling results. In all three approaches, to test the combined predictions of the Slow-decay and MEMS hypotheses, the ideal approach would measure ecosystem properties and putative EM fungal effect traits across observed or manipulated soil mineral N availability gradients, in both organic and mineral soil layers, and in both nitrophilic and nitrophobic EM fungal species.
Field studies would ideally utilize replicated gradients spanning pine plantation/forest through pine invasion into pine-free habitats, with sites chosen to create a factorial natural experiment including:
• Exotic sites spanning a gradient (natural or generated by anthropogenic sources) from relatively high to relatively low soil mineral N availability.
• Exotic sites that vary in diversity and composition of introduced EM fungi.
• Exotic sites that vary in the native habitat displaced by plantations and invasions, including both EM-dominated habitats (such as Eucalyptus forest in Australia; Nothofagus forest in southern South America; or dipterocarp forest in south and south-east Asia) and AM-dominated habitats (such as páramo, puna, or other open high mountain vegetation and AM-dominated forests in South America).
• Northern Hemisphere native pine habitats, plantations, and sites where pines are encroaching into adjacent native habitats (such as P. elliottii encroaching into wet P. palustris savanna).
• Northern Hemisphere native vs. introduced pine habitats (e.g., P. sylvestris in its native range in Europe vs. exotic range in North America).
The factorial combinations of soil mineral N availability and EM fungal composition could allow testing of the prediction of a stronger “Gadgil effect” in surface organic soil layers at sites where Suilloid EM fungi dominate, and reduced production of mineral-protected microbial residues and C storage in mineral soils at those sites. In general, comparison of plantation and invasion sites that vary in EM fungal diversity and composition would facilitate exploration of the effects of EM fungal traits, holding the presence of pines constant. Sites where EM-dominated habitats are being invaded by introduced EM fungi would potentially reveal effects of pines per se and/or effects of particular lineages of EM fungi (when comparing sites varying in EM fungal composition), holding the presence of EM fungi constant across the sites compared. Pine invasions into AM-dominated conifer habitats, such as Araucaria forest, would hold constant the presence of conifers (although different conifer species may have important trait differences). Inclusion of native pine sites in the Northern Hemisphere would allow comparison with high EM fungal diversity sites and would serve as a baseline for asking how the functions of EM fungal communities change when diversity is reduced and/or particular lineages are lost.
Inferences from observational field studies would be correlative, so ideally they would be combined with manipulative laboratory experiments. These experiments could include nitrophilic and nitrophobic EM fungal species observed to dominate at different exotic field sites, synthesized on pine hosts (Riffle, 1973; Richter and Bruhn, 1989; Dunabeitia et al., 1996; Baxter and Dighton, 2001), and grown in mineral vs. organic soils with high or low mineral N availability. In both laboratory and field studies, C, N, and P pools and cycling processes would be measured and linked with EM fungal traits and plant traits. EM fungal traits would include expression of key fungal functional genes controlling soil C, N, and P cycling (Table 1). Plant traits would include root morphology, turnover, and foraging patterns. This approach would allow determination of the genome-wide molecular function of the specific fungal species that dominate soil nutrient cycling, and their consequences for C, N, and P cycling. Such results could also inform a new generation of ecosystem models that incorporate variation in key microbial traits.
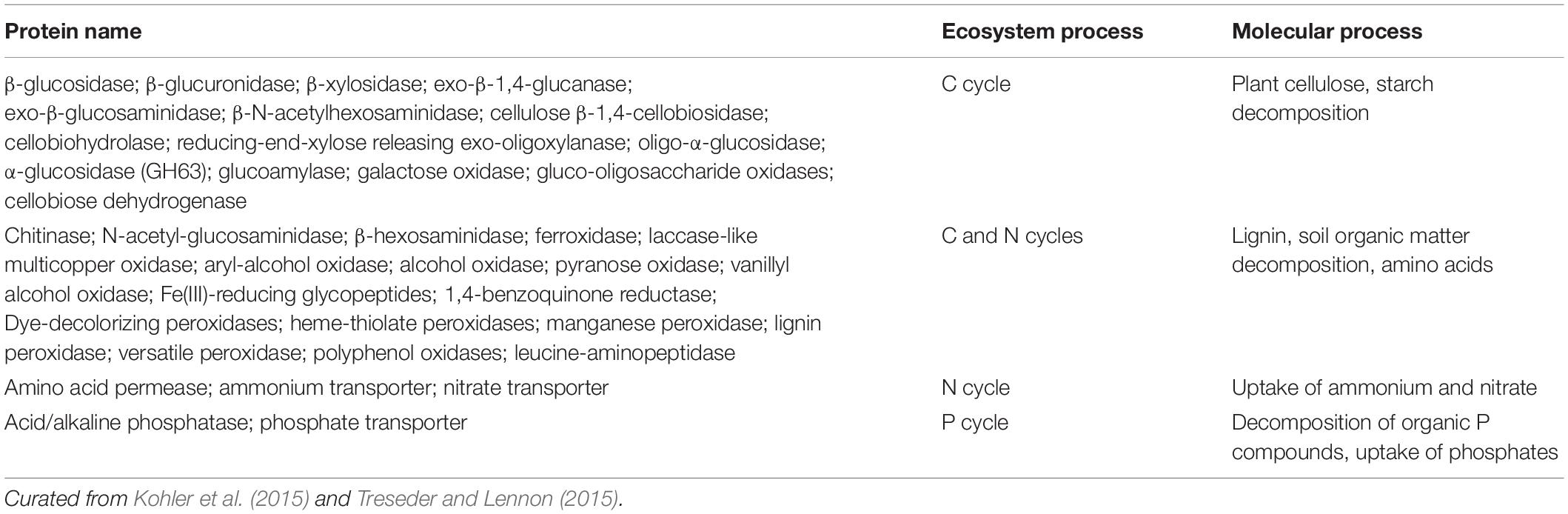
Table 1. Fungal proteins involved in C, N, and P cycling that could be targeted in field and laboratory studies.
Ecosystem Modeling as a Tool for Linking Microbial Traits to Ecosystem Function
Despite evidence that microorganisms vary widely in key traits [e.g., C use efficiency (CUE), organic acid production, enzyme production] (Zak et al., 2019), the current generation of ecosystem biogeochemistry models mostly assumes that all soil microbes are the same; i.e., most models have a single microbial class and those that include microbial diversity are poorly parameterized due to data limitations (see Wang et al., 2013; Sulman et al., 2014; Wieder et al., 2015). Moreover, the latter are extremely sensitive to estimates of these traits (Allison et al., 2010), such that their lack of diversity in microbial traits calls into question their ability to predict the biogeochemical consequences of major shifts in geographic range that are occurring in dominant microbial communities. Empirical data from field and laboratory experiments could inform ecosystem modeling to predict how: (1) shifts in EM introductions and invasions and associated changes in functional traits of fungi, and (2) competitive suppression of saprobic fungi by EM fungi, alter C and N cycling from their characteristic state in native habitats. While this proposed modeling exercise is ambitious, it is feasible, as demonstrated by a recent success in aquatic ecosystems, which integrated transcriptomics data into larger biogeochemical models (Coles et al., 2017).
For example, new mycorrhizal functions have been added to the “Fixation and Uptake of Nitrogen” (FUN) model, which optimally allocates C to the mycorrhizal strategy (i.e., AM, EM, or non-mycorrhizal) that has the greatest nutrient return on plant C investment (Brzostek et al., 2014; Shi et al., 2016). The FUN model has recently been coupled with a microbial decomposition model, CORPSE (Sulman et al., 2014, 2017; Figure 5). There is also now a version of the FUN model that incorporates P (Allen et al., 2020), but it has not yet been fully coupled with CORPSE. The FUN-CORPSE model takes into account plant root traits, such as differences in fine root morphology between AM and EM systems, when calculating the size of the rhizosphere. The model also considers variation in root turnover rates, as the calculated size of the rhizosphere varies as a function of fine root production and mortality. Variation in root foraging traits is not considered explicitly, but is incorporated implicitly because AM roots are modeled as better at scavenging in high N environments and EM roots are better at mining in low N environments. Soil mineralogy is considered to some degree in the model, as clay content is modeled as a factor that controls the protection of SOM.
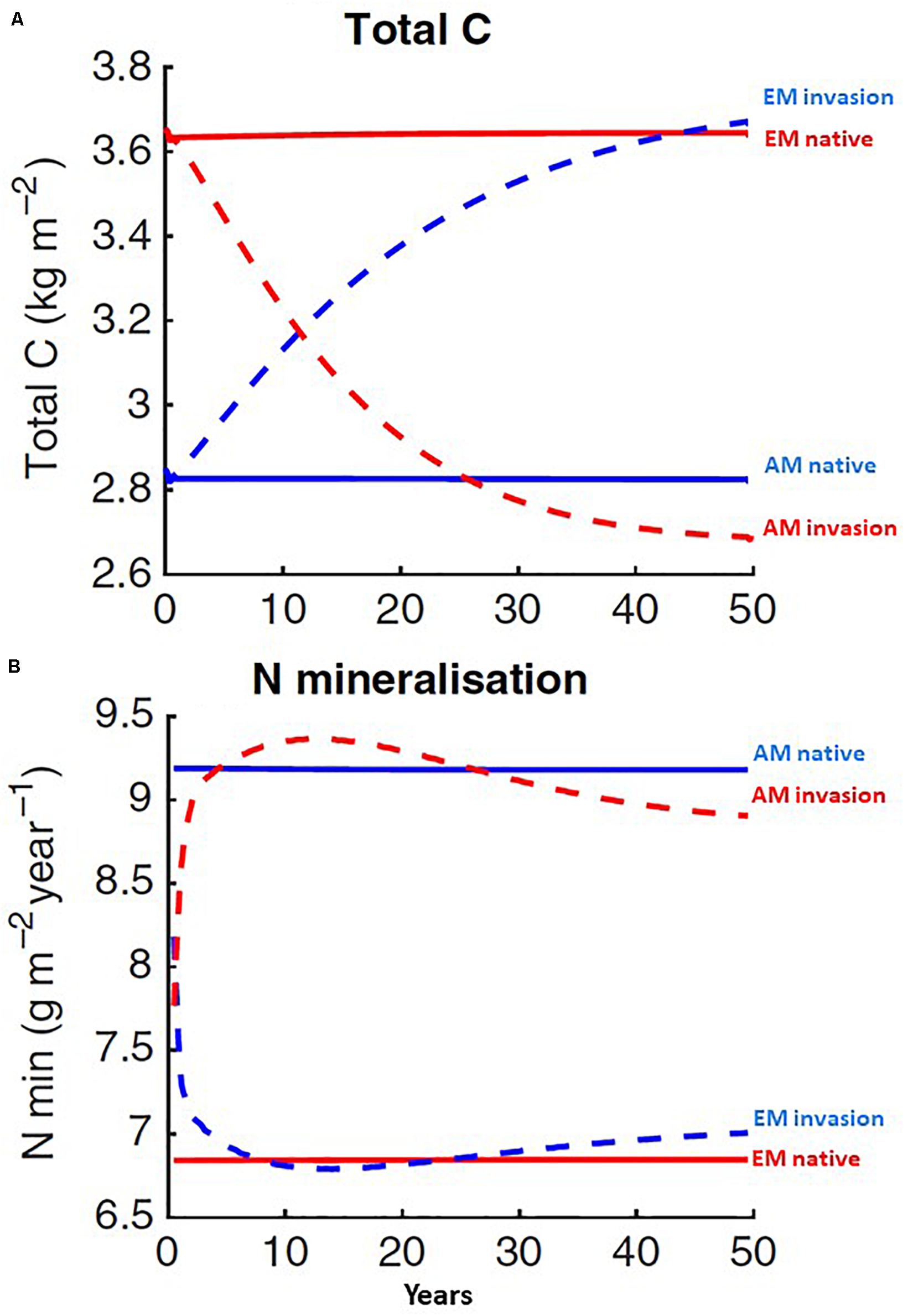
Figure 5. FUN optimally allocates C to gain N through roots and symbionts and CORPSE predicts the impacts of the C on soil processes. EM or AM invasion leads to sustained shifts in the trajectories of (A) C and (B) N cycling over time. Figure created using data from Sulman et al. (2017).
The coupled FUN-CORPSE model has been extensively validated and has been able to predict root exudation and the resulting stimulation of C and N mineralization in the rhizosphere across 45 forested plots in the USA that varied in the abundance of AM and EM trees. Importantly, the coupled model linked litter and substrate chemistry feedbacks on microbial CUE with the ability of plant-associated microbes to prime the decomposition of SOM from mineral-bound and energetically protected pools (Sulman et al., 2017). FUN and CORPSE are coupled into larger terrestrial biosphere models (e.g., Community Land Model, Geophysical Fluid Dynamics Lab Model).
FUN-CORPSE has been used to examine the extent to which AM or EM dominance may alter soil C and N cycling (Sulman et al., 2017). In contrast to AM invasion, EM invasion enhanced soil C storage and reduced the availability of inorganic N to plants (Figure 5). This simulation suggests that the conversion of AM systems to EM systems may alter ecosystem-level C and N cycling, as predicted by the Slow-decay hypothesis. However, FUN currently assumes that all fungi have the same traits, which omits important trait diversity among EM fungi and saprobes, preventing a test of our prediction that C storage and N cycling will be altered at exotic sites where Suilloid EM fungi are favored by conditions of low soil mineral N availability. This limitation could be addressed by integrating phylogenetic variation in effect traits (such as CUE and organic N decomposition ability) among EM fungi, and variation in the allocation of plant C to different enzyme systems, across invasion chronosequences, informed by data generated from field and laboratory studies as described above.
Specifically, it would be feasible to integrate empirical data into the model using stepwise increases in complexity, by leveraging empirical linkages between soil processes (i.e., enzyme activities, N mineralization) and “omics” (e.g., metagenomics, metatranscriptomics, metabolomics) data. For example, one could use the relative abundances of transcripts that code for growth vs. energy expenditure in EM fungi to parametrize CUE, the enzymes that degrade SOM, and the fraction of assimilated C expended on enzyme synthesis. To provide an additional estimate of CUE in fungal species, one could use metagenomic data on fungal communities to classify gene abundance based on those that encode for metabolic processes, resource acquisition (Table 1), and growth using Kbase, a novel computational – omics platform (as in Saifuddin et al., 2019). To complement and calibrate these omics approaches, one could also perform laboratory incubations with subsets of soils from each site in which isotopically labeled substrates (e.g., glucose, glycine) are added to provide an additional empirical estimate of CUE and microbial turnover. In addition, one could add functional diversity of EM fungi, AM fungi, and saprobes to the model using data on metabolic dominance data coupled with transcript data to parameterize differences between these functional groups in their energy expenditures on enzyme systems that carry out the molecular processes outlined in Table 1.
Conclusion
Despite the substantial negative ecological consequences of the world’s most widespread biological introductions, we suggest that some of them – such as pines and their symbiotic EM fungi in the Southern Hemisphere – can be leveraged as model systems for understanding how the functional traits of soil microbial communities affect coupled soil C and nutrient cycling in ecosystems. Accomplishing this objective would require using an integrative approach – including ecosystem modeling informed by field studies and laboratory experimentation – with the goal of connecting the genes and functional traits of soil fungi to their above- and belowground functional consequences in ecosystem models. The resulting outcomes could significantly improve the predictive ability of ecosystem models of C and N cycling by integrating the functional traits of soil microbial assemblages.
Author Contributions
EBr, JH, JR, and RV designed the figures. All authors contributed to the article and approved the submitted version.
Funding
JH was supported by the United States National Science Foundation award 1953299. CA was supported by the Ambizione Grant No. PZ00P3_17990 from the Swiss National Science Foundation. JB, CA, EBr, H-LL, NP, and RV were supported by the United States Department of Energy (DOE) Systems Biology of Microbiomes in Nutrient Cycling award (DE-FOA-0002059). JB was supported by a DOE Bioimaging Research award (DE-SC0012704). LN and EBu were supported by the Brazilian CNPq award (Grant No. 441561/2016-0) and Brazilian FAPESP award (Grant No. 2016-50481-3). RV and JAR were funded by the United States National Science Foundation award DEB 1554181.
Conflict of Interest
The authors declare that the research was conducted in the absence of any commercial or financial relationships that could be construed as a potential conflict of interest.
The handling editor declared a past co-authorship with one of the authors CA.
References
Ackerly, D. D. (2003). Community assembly, niche conservatism, and adaptive evolution in changing environments. Int. J. Plant Sci. 164, S165– S184.
Ali, N. A., and Jackson, R. M. (1988). Effects of plant roots and their exudates on germination of spores of ectomycorrhizal fungi. Trans. Br. Mycol. Soc. 91, 253–260. doi: 10.1016/s0007-1536(88)80212-2
Allen, K. E., Fisher, J. B., Phillips, R. P., Powers, J., and Brzostek, E. R. (2020). Modeling the carbon cost of plant nitrogen and phosphorus uptake across temperate and tropical forests. Front. For. Glob. Change 3:43. doi: 10.3389/ffgc.2020.00043
Allison, S. D., Wallenstein, M. D., and Bradford, M. A. (2010). Soil-carbon response to warming dependent on microbial physiology. Nat. Geosci. 3, 336–340. doi: 10.1038/ngeo846
Anagnostakis, S. L. (2001). The effect of multiple importations of pests and pathogens on a native tree. Biol. Invasions 3, 245–254.
Ashkannejhad, S., and Horton, T. R. (2006). Ectomycorrhizal ecology under primary succession on coastal sand dunes: interactions involving Pinus contorta, suilloid fungi and deer. New Phytol. 169, 345–354. doi: 10.1111/j.1469-8137.2005.01593.x
Averill, C., Dietze, M. C., and Bhatnagar, J. M. (2018). Continental-scale nitrogen pollution is shifting forest mycorrhizal associations and soil carbon stocks. Glob. Change Biol. 24, 4544–4553. doi: 10.1111/gcb.14368
Averill, C., Turner, B. L., and Finzi, A. C. (2014). Mycorrhiza-mediated competition between plants and decomposers drives soil carbon storage. Nature 505, 543–545. doi: 10.1038/nature12901
Baxter, J. W., and Dighton, J. (2001). Ectomycorrhizal diversity alters growth and nutrient acquisition of grey birch (Betula populifolia) seedlings in host–symbiont culture conditions. New Phytol. 152, 139–149. doi: 10.1046/j.0028-646x.2001.00245.x
Bever, J. D., Dickie, I. A., Facelli, E., Facelli, J. M., Klironomos, J., Moora, M., et al. (2010). Rooting theories of plant community ecology in microbial interactions. Trends Ecol. Evol. 25, 468–478. doi: 10.1016/j.tree.2010.05.004
Bonebrake, T. C., Brown, C. J., Bell, J. D., Blanchard, J. L., Chauvenet, A., Champion, C., et al. (2018). Managing consequences of climate-driven species redistribution requires integration of ecology, conservation and social science. Biol. Rev. Camb. Philos. Soc. 93, 284–305. doi: 10.1111/brv.12344
Brasier, C. M., and Buck, K. W. (2001). Rapid evolutionary changes in a globally invading fungal pathogen (Dutch elm disease). Biol. Invasions 3, 223–233. doi: 10.1023/A:1015248819864
Brewer, J. S., Souza, F. M., Callaway, R. M., and Durigan, G. (2018). Impact of invasive slash pine (Pinus elliottii) on groundcover vegetation at home and abroad. Biol. Invasions 20, 2807–2820. doi: 10.1007/s10530-018-1734-z
Brzostek, E. R., Fisher, J. B., and Phillips, R. P. (2014). Modeling the carbon cost of plant nitrogen acquisition: mycorrhizal trade-offs and multipath resistance uptake improve predictions of retranslocation. J. Geophys. Res. Biogeosci. 119, 1684–1697. doi: 10.1002/2014jg002660
Cairney, J. W. (2011). Ectomycorrhizal fungi: the symbiotic route to the root for phosphorus in forest soils. Plant Soil 344, 51–71. doi: 10.1007/s11104-011-0731-0
Callaway, R. M., Hierro, J. L., and Thorpe, A. S. (2005). “Evolutionary trajectories in plant and soil microbial communities: centaurea invasions and the geographic mosaic of coevolution,” in Exotic Species Invasions: Insights into Ecology, Evolution and Biogeography, eds D. F. Sax, S. D. Gaines, and J. J. Stachowicz (Sunderland, MA: Sinauer), 341–363.
Carleton, T. J., and Read, D. J. (1991). Ectomycorrhizas and nutrient transfer in conifer-feather moss ecosystems. Can. J. Bot. 69, 778–785. doi: 10.1139/b91-101
Chapela, I. H., Osher, L. J., Horton, T. R., and Henn, M. R. (2001). Ectomycorrhizal fungi introduced with exotic pine plantations induce soil carbon depletion. Soil Biol. Biochem. 33, 1733–1740. doi: 10.1016/s0038-0717(01)00098-0
Chen, C. R., Condron, L. M., and Xu, Z. H. (2008). Impacts of grassland afforestation with coniferous trees on soil phosphorus dynamics and associated microbial processes: a review. For. Ecol. Manag. 255, 396–409. doi: 10.1016/j.foreco.2007.10.040
Chu-Chou, M., and Grace, L. J. (1988). Mycorrhizal fungi of radiata pine in different forests of the north and south islands in New Zealand. Soil Biol. Biochem. 20, 883–886. doi: 10.1016/0038-0717(88)90098-3
Colautti, R. I., and Lau, J. A. (2015). Contemporary evolution during invasion: evidence for differentiation, natural selection, and local adaptation. Mol. Ecol. 24, 1999–2017. doi: 10.1111/mec.13162
Coles, V. J., Stukel, M. R., Brooks, M. T., Burd, A., Crump, B. C., Moran, M. A., et al. (2017). Ocean biogeochemistry modeled with emergent trait-based genomics. Science 358, 1149–1154. doi: 10.1126/science.aan5712
Cotrufo, M. F., Ranalli, M. G., Haddix, M. L., Six, J., and Lugato, E. (2019). Soil carbon storage informed by particulate and mineral-associated organic matter. Nat. Geosci. 12, 989–994. doi: 10.1038/s41561-019-0484-6
Cox, F., Barsoum, N., Lilleskov, E. A., and Bidartondo, M. I. (2010). Nitrogen availability is a primary determinant of conifer mycorrhizas across complex environmental gradients. Ecol. Lett. 13, 1103–1113. doi: 10.1111/j.1461-0248.2010.01494.x
Craig, M. E., Turner, B. L., Liang, C., Clay, K., Johnson, D. J., and Phillips, R. P. (2018). Tree mycorrhizal type predicts within-site variability in the storage and distribution of soil organic matter. Glob. Change Biol. 24, 3317–3330. doi: 10.1111/gcb.14132
Crowther, T. W., Maynard, D. S., Crowther, T. R., Peccia, J., Smith, J. R., and Bradford, M. A. (2014). Untangling the fungal niche: the trait-based approach. Front. Microbiol. 5:579. doi: 10.3389/fmicb.2014.00579
Crowther, T. W., Sokol, N. W., Oldfield, E. E., Maynard, D. S., Thomas, S. M., and Bradford, M. A. (2015). Environmental stress response limits microbial necromass contributions to soil organic carbon. Soil Biol. Biochem. 85, 153–161. doi: 10.1016/j.soilbio.2015.03.002
Danielson, R. M. (1984). Ectomycorrhizal associations in jack pine stands in northeastern Alberta. Can. J. Bot. 62, 932–939. doi: 10.1139/b84-132
de Mazancourt, C., Johnson, E., and Barraclough, T. G. (2008). Biodiversity inhibits species’ evolutionary responses to changing environments. Ecol. Lett. 11, 380–388. doi: 10.1111/j.1461-0248.2008.01152.x
Desprez-Loustau, M.-L., Robin, C., Buée, M., Courtecuisse, R., Garbaye, J., Suffert, F., et al. (2007). The fungal dimension of biological invasions. Trends Ecol. Evol. 22, 472–480. doi: 10.1016/j.tree.2007.04.005
Dickie, I. A., Bufford, J. L., Cobb, R. C., Desprez-Loustau, M.-L., Grelet, G., Hulme, P. E., et al. (2017). The emerging science of linked plant-fungal invasions. New Phytol. 215, 1314–1332. doi: 10.1111/nph.14657
Dickie, I. A., St John, M. G., Yeates, G. W., Morse, C. W., Bonner, K. I., Orwin, K., et al. (2014). Belowground legacies of Pinus contorta invasion and removal result in multiple mechanisms of invasional meltdown. AoB Plants 6:plu056.
Dickie, I. A., Yeates, G. W., St. John, M. G., Stevenson, B. A., Scott, J. T., Rillig, M. C., et al. (2011). Ecosystem service and biodiversity trade-offs in two woody successions. J. Appl. Ecol. 48, 926–934. doi: 10.1111/j.1365-2664.2011.01980.x
Dunabeitia, M. K., Hormilla, S., Salcedo, I., and Pena, J. I. (1996). Ectomycorrhizae synthesized between Pinus radiata and eight fungi associated with Pinus spp. Mycologia 88, 897–908. doi: 10.2307/3761052
Dunstan, W. A., Dell, B., and Malajczuk, N. (1998). The diversity of ectomycorrhizal fungi associated with introduced Pinus spp. in the Southern Hemisphere, with particular reference to Western Australia. Mycorrhiza 8, 71–79. doi: 10.1007/s005720050215
Dutech, C., Barrès, B., Bridier, J., Robin, C., Milgroom, M. G., and Ravigné, V. (2012). The chestnut blight fungus world tour: successive introduction events from diverse origins in an invasive plant fungal pathogen. Mol. Ecol. 21, 3931–3946. doi: 10.1111/j.1365-294x.2012.05575.x
Fierer, N., Barberán, A., and Laughlin, D. C. (2014). Seeing the forest for the genes: using metagenomics to infer the aggregated traits of microbial communities. Front. Microbiol. 5:614. doi: 10.3389/fmicb.2014.00614
Fransson, P. M. A., Anderson, I. C., and Alexander, I. J. (2007). Ectomycorrhizal fungi in culture respond differently to increased carbon availability. FEMS Microbiol. Ecol. 61, 246–257. doi: 10.1111/j.1574-6941.2007.00343.x
Gadgil, R. L., and Gadgil, P. D. (1971). Mycorrhiza and litter decomposition. Nature 233:133. doi: 10.1038/233133a0
Gardes, M., and Bruns, T. D. (1996). Community structure of ectomycorrhizal fungi in a Pinus muricata forest: above- and below-ground views. Can. J. Bot. 74, 1572–1583. doi: 10.1139/b96-190
Gladieux, P., Feurtey, A., Hood, M. E., Snirc, A., Clavel, J., Dutech, C., et al. (2015). The population biology of fungal invasions. Mol. Ecol. 24, 1969–1986. doi: 10.1111/mec.13028
Hatakeyama, T., and Ohmasa, M. (2004). Mycelial growth of strains of the genera Suillus and Boletinus in media with a wide range of concentrations of carbon and nitrogen sources. Mycoscience 45, 169–176. doi: 10.1007/s10267-003-0169-1
Hayward, J., Horton, T. R., Pauchard, A., and Nuñez, M. A. (2015). A single ectomycorrhizal fungal species can enable a Pinus invasion. Ecology 96, 1438–1444. doi: 10.1890/14-1100.1
Hooper, D. U., Chapin, F. S. III, Ewel, J. J., Hector, A., Inchausti, P., Lavorel, S., et al. (2005). Effects of biodiversity on ecosystem functioning: a consensus of current knowledge. Ecol. Monogr. 75, 3–35.
Horton, T. R., and Bruns, T. D. (2001). The molecular revolution in ectomycorrhizal ecology: peeking into the black-box. Mol. Ecol. 10, 1855–1871. doi: 10.1046/j.0962-1083.2001.01333.x
Hynson, N. A., Vincent, S. F., Perry, B. A., and Treseder, K. K. (2013). Identities and distributions of the co-invading ectomycorrhizal fungal symbionts of exotic pines in the Hawaiian Islands. Biol. Invasions 15, 2373–2385. doi: 10.1007/s10530-013-0458-3
Jackson, R. B., Banner, J. L., Jobbágy, E. G., Pockman, W. T., and Wall, D. H. (2002). Ecosystem carbon loss with woody plant invasion of grasslands. Nature 418, 623–626. doi: 10.1038/nature00910
Jansa, J., Finlay, R., Wallander, H., Smith, F. A., and Smith, S. E. (2011). “Role of mycorrhizal symbioses in phosphorus cycling,” in Phosphorus in Action, eds E. Bünemann, A. Oberson, and E. Frossard (Berlin: Springer), 137–168. doi: 10.1007/978-3-642-15271-9_6
Jones, D. L., Hodge, A., and Kuzyakov, Y. (2004). Plant and mycorrhizal regulation of rhizodeposition. New Phytol. 163, 459–480. doi: 10.1111/j.1469-8137.2004.01130.x
Kohler, A., Kuo, A., Nagy, L. G., Morin, E., Barry, K. W., Buscot, F., et al. (2015). Convergent losses of decay mechanisms and rapid turnover of symbiosis genes in mycorrhizal mutualists. Nat. Genet. 47, 410–415. doi: 10.1038/ng.3223
Köhler, J., Yang, N., Pena, R., Raghavan, V., Polle, A., and Meier, I. C. (2018). Ectomycorrhizal fungal diversity increases phosphorus uptake efficiency of European beech. New Phytol. 220, 1200–1210. doi: 10.1111/nph.15208
Koide, R. T., Fernandez, C., and Malcolm, G. (2014). Determining place and process: functional traits of ectomycorrhizal fungi that affect both community structure and ecosystem function. New Phytol. 201, 433–439. doi: 10.1111/nph.12538
Krause, S., Le Roux, X., Niklaus, P. A., Van Bodegom, P. M., Lennon, J. T., Bertilsson, S., et al. (2014). Trait-based approaches for understanding microbial biodiversity and ecosystem functioning. Front. Microbiol. 5:251. doi: 10.3389/fmicb.2014.00251
Lantschner, M. V., Atkinson, T. H., Corley, J. C., and Liebhold, A. M. (2017). Predicting North American scolytinae invasions in the Southern hemisphere. Ecol. Appl. 27, 66–77. doi: 10.1002/eap.1451
Lavorel, S., and Garnier, E. (2002). Predicting changes in community composition and ecosystem functioning from plant traits: revisiting the Holy Grail. Funct. Ecol. 16, 545–556. doi: 10.1046/j.1365-2435.2002.00664.x
Liese, R., Lübbe, T., Albers, N. W., and Meier, I. C. (2018). The mycorrhizal type governs root exudation and nitrogen uptake of temperate tree species. Tree Physiol. 38, 83–95. doi: 10.1093/treephys/tpx131
Lilienfein, J., and Wilcke, W. (2003). Element storage in native, agri-, and silvicultural ecosystems of the Brazilian savanna. Plant Soil 254, 425–442.
Lilienfein, J., Wilcke, W., Angelo Ayarza, M., Vilela, L., do Carmo Lima, S., and Zech, W. (2000). Soil acidification in Pinus caribaea forests on Brazilian savanna Oxisols. For. Ecol. Manag. 128, 145–157. doi: 10.1016/s0378-1127(99)00143-7
Lilienfein, J., Wilcke, W., Thomas, R., Vilela, L., Lima, S., do, C., et al. (2001). Effects of Pinus caribaea forests on the C, N, P, and S status of Brazilian savanna Oxisols. For. Ecol. Manag. 147, 171–182. doi: 10.1016/s0378-1127(00)00472-2
Lilleskov, E. A., Fahey, T. J., Horton, T. R., and Lovett, G. M. (2002). Belowground ectomycorrhizal fungal community change over a nitrogen deposition gradient in Alaska. Ecology 83, 104–115. doi: 10.1890/0012-9658(2002)083[0104:befcco]2.0.co;2
Loreau, M., Naeem, S., Inchausti, P., Bengtsson, J., Grime, J. P., Hector, A., et al. (2001). Biodiversity and ecosystem functioning: current knowledge and future challenges. Science 294, 804–808. doi: 10.1126/science.1064088
Nielsen, U. N., Ayres, E., Wall, D. H., and Bardgett, R. D. (2011). Soil biodiversity and carbon cycling: a review and synthesis of studies examining diversity–function relationships. Eur. J. Soil Sci. 62, 105–116. doi: 10.1111/j.1365-2389.2010.01314.x
Nuñez, M. A., and Dickie, I. A. (2014). Invasive belowground mutualists of woody plants. Biol. Invasions 16, 645–661. doi: 10.1007/s10530-013-0612-y
Nuñez, M. A., Horton, T. R., and Simberloff, D. (2009). Lack of belowground mutualisms hinders Pinaceae invasions. Ecology 90, 2352–2359. doi: 10.1890/08-2139.1
Orwin, K. H., Kirschbaum, M. U. F., St John, M. G., and Dickie, I. A. (2011). Organic nutrient uptake by mycorrhizal fungi enhances ecosystem carbon storage: a model-based assessment. Ecol. Lett. 14, 493–502. doi: 10.1111/j.1461-0248.2011.01611.x
Palumbi, S. R. (2001). Humans as the world’s greatest evolutionary force. Science 293, 1786–1790. doi: 10.1126/science.293.5536.1786
Pecl, G. T., Araújo, M. B., Bell, J. D., Blanchard, J., Bonebrake, T. C., Chen, I.-C., et al. (2017). Biodiversity redistribution under climate change: impacts on ecosystems and human well-being. Science 355:eaai9214.
Policelli, N., Bruns, T. D., Vilgalys, R., and Nuñez, M. A. (2018). Suilloid fungi as global drivers of pine invasions. New Phytol. 222, 714–725. doi: 10.1111/nph.15660
Pyšek, P., Jarošík, V., Hulme, P. E., Pergl, J., Hejda, M., Schaffner, U., et al. (2012). A global assessment of invasive plant impacts on resident species, communities and ecosystems: the interaction of impact measures, invading species’ traits and environment. Glob. Change Biol. 18, 1725–1737. doi: 10.1111/j.1365-2486.2011.02636.x
Read, D. J., and Perez-Moreno, J. (2003). Mycorrhizas and nutrient cycling in ecosystems–a journey towards relevance? New Phytol. 157, 475–492. doi: 10.1046/j.1469-8137.2003.00704.x
Richardson, D. M., Williams, P. A., and Hobbs, R. J. (1994). Pine invasions in the Southern hemisphere: determinants of spread and invadability. J. Biogeogr. 21, 511–527.
Richter, D. L., and Bruhn, J. N. (1989). Pinus resinosa ectomycorrhizae: seven host-fungus combinations synthesized in pure culture. Symbiosis 7, 211–228.
Riffle, J. W. (1973). Pure culture synthesis of ectomycorrhizae on Pinus ponderosa with species of amanita, Suillus, and Lactarius. For. Sci. 19, 242–250.
Rosling, A., Midgley, M. G., Cheeke, T., Urbina, H., Fransson, P., and Phillips, R. P. (2016). Phosphorus cycling in deciduous forest soil differs between stands dominated by ecto- and Arbuscular mycorrhizal trees. New Phytol. 209, 1184–1195. doi: 10.1111/nph.13720
Saifuddin, M., Bhatnagar, J. M., Segrè, D., and Finzi, A. C. (2019). Microbial carbon use efficiency predicted from genome-scale metabolic models. Nat. Commun. 10:3568.
Scholes, M. C., and Nowicki, T. E. (1998). “Effects of pines on soil properties and processes,” in ‘Ecology and Biogeography of Pinus’, ed. D. M. Richardson (Cambridge: Cambridge University Press), 341–353.
Schwartz, M. W., Hoeksema, J. D., Gehring, C. A., Johnson, N. C., Klironomos, J. N., Abbott, L. K., et al. (2006). Global movement of mycorrhizal fungus inoculum: promise and possible consequences. Ecol. Lett. 9, 501–515. doi: 10.1111/j.1461-0248.2006.00910.x
Shi, M., Fisher, J. B., Brzostek, E. R., and Phillips, R. P. (2016). Carbon cost of plant nitrogen acquisition: global carbon cycle impact from an improved plant nitrogen cycle in the community land model. Glob. Change Biol. 22, 1299–1314. doi: 10.1111/gcb.13131
Simberloff, D., Nunez, M. A., Ledgard, N. J., Pauchard, A., Richardson, D. M., Sarasola, M., et al. (2010). Spread and impact of introduced conifers in South America: lessons from other southern hemisphere regions. Austral Ecol. 35, 489–504. doi: 10.1111/j.1442-9993.2009.02058.x
Smith, S. E., Anderson, I. C., and Smith, F. A. (2018). Mycorrhizal associations and phosphorus acquisition: from cells to ecosystems. Ann. Plant Rev. Online 48, 409–439. doi: 10.1002/9781119312994.apr0529
Strauss, S. Y. (2014). Ecological and evolutionary responses in complex communities: implications for invasions and eco-evolutionary feedbacks. Oikos 123, 257–266. doi: 10.1111/j.1600-0706.2013.01093.x
Suding, K. N., Gross, K. L., and Houseman, G. R. (2004). Alternative states and positive feedbacks in restoration ecology. Trends Ecol. Evol. 19, 46–53. doi: 10.1016/j.tree.2003.10.005
Suding, K. N., Lavorel, S., Chapin, F. S., Cornelissen, J. H. C., Diaz, S., Garnier, E., et al. (2008). Scaling environmental change through the community-level: a trait-based response-and-effect framework for plants. Glob. Change Biol. 14, 1125–1140. doi: 10.1111/j.1365-2486.2008.01557.x
Sulman, B. N., Brzostek, E. R., Medici, C., Shevliakova, E., Menge, D. N. L., and Phillips, R. P. (2017). Feedbacks between plant N demand and rhizosphere priming depend on type of mycorrhizal association. Ecol. Lett. 20, 1043–1053. doi: 10.1111/ele.12802
Sulman, B. N., Phillips, R. P., Oishi, A. C., Shevliakova, E., and Pacala, S. W. (2014). Microbe-driven turnover offsets mineral-mediated storage of soil carbon under elevated CO2. Nat. Clim. Change 4, 1099–1102. doi: 10.1038/nclimate2436
Sun, Y.-P., and Fries, N. (1992). The effect of tree-root exudates on the growth rate of ectomycorrhizal and saprotrophic fungi. Mycorrhiza 1, 63–69. doi: 10.1007/bf00206138
Talbot, J. M., Bruns, T. D., Taylor, J. W., Smith, D. P., Branco, S., Glassman, S. I., et al. (2014). Endemism and functional convergence across the North American soil mycobiome. Proc. Natl. Acad. Sci. U.S.A. 111, 6341–6346. doi: 10.1073/pnas.1402584111
Talbot, J. M., Martin, F., Kohler, A., Henrissat, B., and Peay, K. G. (2015). Functional guild classification predicts the enzymatic role of fungi in litter and soil biogeochemistry. Soil Biol. Biochem. 88, 441–456. doi: 10.1016/j.soilbio.2015.05.006
Talbot, J. M., and Treseder, K. K. (2010). Controls over mycorrhizal uptake of organic nitrogen. Pedobiologia 53, 169–179. doi: 10.1016/j.pedobi.2009.12.001
Teste, F. P., Laliberté, E., Lambers, H., Auer, Y., Kramer, S., and Kandeler, E. (2016). Mycorrhizal fungal biomass and scavenging declines in phosphorus-impoverished soils during ecosystem retrogression. Soil Biol. Biochem. 92, 119–132. doi: 10.1016/j.soilbio.2015.09.021
Tilman, D., Knops, J., Wedin, D., Reich, P., Ritchie, M., and Siemann, E. (1997). The influence of functional diversity and composition on ecosystem processes. Science 277, 1300–1302. doi: 10.1126/science.277.5330.1300
Townsend, A. R., Cleveland, C. C., Houlton, B. Z., Alden, C. B., and White, J. W. (2011). Multi-element regulation of the tropical forest carbon cycle. Front. Ecol. Environ. 9, 9–17. doi: 10.1890/100047
Treseder, K. K., and Lennon, J. T. (2015). Fungal traits that drive ecosystem dynamics on land. Microbiol. Mol. Biol. Rev. 79, 243–262. doi: 10.1128/mmbr.00001-15
Urcelay, C., Longo, S., Geml, J., Tecco, P. A., and Nouhra, E. (2017). Co-invasive exotic pines and their ectomycorrhizal symbionts show capabilities for wide distance and altitudinal range expansion. Fungal Ecol. 25, 50–58. doi: 10.1016/j.funeco.2016.11.002
van der Linde, S., Suz, L. M., Orme, C. D. L., Cox, F., Andreae, H., Asi, E., et al. (2018). Environment and host as large-scale controls of ectomycorrhizal fungi. Nature 558, 243–248.
Vance, C. P., Uhde-Stone, C., and Allan, D. L. (2003). Phosphorus acquisition and use: critical adaptations by plants for securing a nonrenewable resource. New Phytol. 157, 423–447.
Vellinga, E. C., Wolfe, B. E., and Pringle, A. (2009). Global patterns of ectomycorrhizal introductions. New Phytol. 181, 960–973.
Vilà, M., Espinar, J. L., Hejda, M., Hulme, P. E., Jarošík, V., Maron, J. L., et al. (2011). Ecological impacts of invasive alien plants: a meta-analysis of their effects on species, communities and ecosystems. Ecol. Lett. 14, 702–708.
Walbert, K., Ramsfield, T. D., Ridgway, H. J., and Jones, E. E. (2010). Ectomycorrhizal species associated with Pinus radiata in New Zealand including novel associations determined by molecular analysis. Mycorrhiza 20, 209–215.
Wang, G., Post, W. M., and Mayes, M. A. (2013). Development of microbial-enzyme-mediated decomposition model parameters through steady-state and dynamic analyses. Ecol. Appl. 23, 255–272.
Wieder, W. R., Grandy, A. S., Kallenbach, C. M., Taylor, P. G., and Bonan, G. B. (2015). Representing life in the Earth system with soil microbial functional traits in the MIMICS model. Geosci. Model. Dev. 8, 1789–1808.
Wilcke, W., and Lilienfein, J. (2002). Biogeochemical consequences of the transformation of native Cerrado into Pinus caribaea plantations in Brazil. Plant Soil 238, 175–189.
Keywords: ectomycorrhizal fungi, Pinus (pine), invasive species, introduced species impacts, carbon and nitrogen cycling
Citation: Hoeksema JD, Averill C, Bhatnagar JM, Brzostek E, Buscardo E, Chen K-H, Liao H-L, Nagy L, Policelli N, Ridgeway J, Rojas JA and Vilgalys R (2020) Ectomycorrhizal Plant-Fungal Co-invasions as Natural Experiments for Connecting Plant and Fungal Traits to Their Ecosystem Consequences. Front. For. Glob. Change 3:84. doi: 10.3389/ffgc.2020.00084
Received: 28 February 2020; Accepted: 16 June 2020;
Published: 14 July 2020.
Edited by:
Peyton Smith, Texas A&M University, United StatesReviewed by:
Jörg Luster, Swiss Federal Institute for Forest, Snow and Landscape Research (WSL), SwitzerlandJunwei Luan, International Centre for Bamboo and Rattan, China
Copyright © 2020 Hoeksema, Averill, Bhatnagar, Brzostek, Buscardo, Chen, Liao, Nagy, Policelli, Ridgeway, Rojas and Vilgalys. This is an open-access article distributed under the terms of the Creative Commons Attribution License (CC BY). The use, distribution or reproduction in other forums is permitted, provided the original author(s) and the copyright owner(s) are credited and that the original publication in this journal is cited, in accordance with accepted academic practice. No use, distribution or reproduction is permitted which does not comply with these terms.
*Correspondence: Jason D. Hoeksema, aG9la3NlbWFAb2xlbWlzcy5lZHU=