- 1Institute of Zoology, Zoological Society of London, London, United Kingdom
- 2School of Geography and the Environment, University of Oxford, Oxford, United Kingdom
- 3School of Geography and Environmental Science, University of Southampton, Hampshire, United Kingdom
In the face of dramatic climate change and human pressure acting on remaining forest areas across tropical, temperate and boreal biomes, there has emerged a coordinated effort to identify and protect forests that are currently considered “intact”. These forests are hypothesized to be more resilient to future abiotic perturbations than fragmented or degraded forests, and therefore, will provide more reliable carbon storage and/or biodiversity services into an uncertain future. Research in the fields of contemporary and paleoecology can offer valuable insights to enhance our ability to assess resilience of forests and whether these would be comparable across forest biomes. Contemporary ecological monitoring has been able to capture processes acting over the short-to-medium term, while paleoecological methods allow us to derive insights of the long-term processes affecting forest dynamics. Recent efforts to both identify intact forests, based on area definitions, and assess vegetation climate sensitivity globally have relied on satellite imagery analysis for the time period 2000–2013. In this paper, we compare these published datasets and do find that on average intact forests in boreal and tropical biomes are less sensitive to temperature and water availability, respectively; however, the patterns are less clear within biomes (e.g., across continents). By taking a longer perspective, through paleoecology, we present several studies that show a range of forest responses to past climatic and human disturbance, suggesting that short-term trends may not be reliable predictors of long-term resilience. We highlight that few contemporary and paleoecology studies have considered forest area when assessing resilience and those that have did find that smaller forest areas exhibited greater dynamism in species composition, which could be a proxy for declining resilience. Climatic conditions in the Anthropocene will be pushing forest systems across biomes into novel climates very rapidly and with current knowledge it is difficult to predict how forests will react in the immediate term, which is the most relevant timeframe for global efforts to reduce carbon emissions.
Introduction
Intact forests (i.e., that have not experienced large-scale anthropogenic disturbance) have been highlighted as being particularly valuable for conservation due to a number of unique attributes and services, such as climate change mitigation, local to regional weather regulation, invaluable biodiversity habitat, clean water provision, support of dependent indigenous cultures as well as wider benefits for human health (Watson et al., 2018). Further, particularly large, contiguous areas of intact ecosystems are posited to be more resilient to changing climate conditions due to their harboring species with higher functional diversity, and therefore, redundancy. A larger, contiguous area allows for greater species dispersal potential as well as greater genetic diversity within extant species (Thompson et al., 2009; Baho et al., 2017). There is already considerable literature showing the negative effects of even moderate levels of disturbance on forest functioning and biodiversity (Gibson et al., 2011; Barlow et al., 2016; Betts et al., 2017), particularly reduced carbon sequestration and loss of species' habitat due to increases in forest edges (Haddad et al., 2015; Qie et al., 2017). At the same time, the impact of disturbance on forest resilience, particularly over larger time scales such as centuries and millennia, is less clear (see Froyd and Willis, 2008; Cole et al., 2014). For example, are there particular types of disturbance that might make a forest ecosystem more resilient over time and is the impact of early Neolithic cultures relevant for the designation of intact forest areas today (Barlow et al., 2012; Willis et al., 2018)? The overall aim of this paper is to discuss and provide a selection of spatial and temporal metrics to inform efforts to assess forest resilience. In particular, we will discuss these methodologies in the context of the intact forest landscape (IFL) metric (Potapov et al., 2017) to explore: (i) how are intact forests responding to disturbance and climate change, (ii) is it possible to distinguish natural variability of a forest ecosystem from increasing variability due to reduced resilience, (iii) are there common mechanisms underpinning resilience across biomes, and (iv) do larger forest patch areas confer resilience?
With increasing anthropogenic pressure, better understanding the dynamics of intact forests is necessary for human development to remain within planetary boundaries (Steffen et al., 2018). Current efforts to identify forest without evidence of contemporary disturbance and that are sufficiently large to maintain the above listed services have relied on remote sensing methods (Potapov et al., 2017). The IFL metric has identified intact forests across all terrestrial biomes (i.e., tropical, temperate, and boreal ecosystems) using a minimum area definition of 500 km2 with a minimum width of 10 km and a minimum corridor width of 2 km, which have not shown any sign of human disturbance since 2000 (Potapov et al., 2017). However, these systems have historically experienced very different levels of human disturbance as well as evolved under very different natural disturbance regimes (Thompson et al., 2009). In theory, intactness is considered to be a core aspect of resilience (Parrish et al., 2003), whereby current conditions can be compared to historical baselines to assess the scale of past disturbance or level of “ecological integrity” (see section on “Baselines”). In practice, the assumption that the same area-based definition of intactness would necessarily correlate with resilience across all forests should be examined. Outlining clear baselines for initial anthropogenic disturbance have proved to be challenging (e.g., Nogué et al., 2017). Paleoecological records have already provided evidence of a long presence of human impacts on forest around the world. For example, evidence of agricultural activity has been dated in New Guinea to 7,000 years ago (e.g., Willis and Birks, 2006) and more examples of early anthropogenic impacts have been found in Amazonia (e.g., McMichael et al., 2012), lowland Central Africa (Tovar et al., 2014) and the Canary Islands (de Nascimento et al., 2009). Therefore, the integration of spatial and temporal methodologies is essential to advance our understanding of the resilience of intact forests.
Competing Frameworks for Resilience
Defining forest resilience has its own challenges. First, it is necessary to decide whether resilience is considered to be a return to an equilibrium state (e.g., engineering resilience) or as a dynamic system that maintains certain functions following a disturbance (e.g., ecological resilience) (Holling, 1973). Carpenter et al. (2001) posited the question “resilience of what, to what?” Are intact forest areas largely valued for their carbon storage and sequestration services, which are supported by highly diverse plant and animal communities, or are they primarily more reliable areas of habitat for endangered and rare species, including refugia for poorly dispersed species, that have the added benefit of being a carbon storage facility? The distinction may seem academic, but the metrics for assessing forest resilience in either scenario could be quite different as well as, potentially, policy approaches for achieving each outcome (Newton, 2016). Existing theory to assess resilience suggest a variety of metrics for predicting whether conditions are approaching a tipping point, and by extension, a likely shift in regime. This requires careful consideration of the temporal scale of analysis, which some have argued should be based on the life-cycle of the slowest relevant variable (e.g., at the century-scale for capturing forest dynamics) (Folke, 2006). However, this shift in temporal scale can lead to a mismatch between the extent of our ecological knowledge and current management policies (Willis et al., 2005).
Assuming a stable state theory, Baho et al. (2017) developed a quantitative framework of resilience, consisting of quantifying measures of resistance, persistence, variability, and recovery that can be estimated using ecosystem-level measures of structural and functional attributes following a disturbance. Resistance relates to the amount of change observed in a chosen metric due to a disturbance. Persistence refers to the length of time species co-exist before going extinct. Variability is expected to be low during a state of stability and increasing as a system approaches a tipping point. While recovery relates to the engineering resilience perspective of returning to a known or desired equilibrium state. Conversely, if considering the resilience of a forest as a dynamic adaptive system, it is unlikely it will “recover” to its original state, but could undergo “renewal” or “regeneration” to a new but similarly functioning system (O‘Neill, 1998). Evidence for taking a more complex view of ecosystem resilience relates to the likelihood an ecosystem in a specific location could have multiple-stable states, due to climate-lags in species response rates, which can also exhibit hysteresis or path dependence (Blonder et al., 2017). Finally, there is a spatial element to resilience, which relates to how connectivity, gradients, ecological lags, and asymmetries contribute to or feedback on a system's response to a disturbance (Cumming, 2011; Allen et al., 2016).
Spatial and Temporal Metrics for Assessing Resilience
Several studies have attempted to estimate the resilience of remaining forest areas using a wide range of metrics; although, largely reliant on a stability-based understanding of resilience, and to our knowledge, none considering the total size of forest area. For instance, relative variability in a response variable has been used as a proxy for forest resilience, such as variability in latent heat fluxes from eddy covariance measures as a predictor of drought vulnerability (Anderegg et al., 2018), climate sensitivity of vegetation using spectral time series of moderate-resolution satellite imagery (Seddon et al., 2016), or establishing a historical range of variation (HRV) to describe a “basin of attraction” for comparison with contemporary responses to disturbance (Seidl et al., 2016). Through establishing an HRV, Seidl et al. (2016) reinforce the understanding that with climate change the range of variability, disturbance regime and rates of recovery will change, thereby defining resilience as a dynamic property of an ecosystem that is important to quantify for informing forest management. Trends in ecosystem responses have been used to assess whether a system is approaching a threshold, such as comparing regional trends in minimum water-use efficiency (WUE) during dry and wet years (Ponce-Campos et al., 2013) or tree-ring growth and isotope signatures to assess changes in community-level productivity (Sangüesa-Barreda et al., 2015; van der Sleen et al., 2015; Brienen et al., 2016).
Beyond temporal trends, spatial patterns may also be indicative of an approaching shift to an alternative state whereby spatial correlation as well as spatial variability may increase. Although, this has mostly been applied in dry environments (Dakos et al., 2011). Other approaches have focused on functional and structural measures of communities to estimate underlying dynamics, which may consider overall species diversity (Hisano et al., 2018), functional redundancy in species presence and their relative abundance (Baho et al., 2017), functional trait composition of the dominant species in a community (Bartlett et al., 2019), whether combinations of functional traits in species are supported by high phylogenetic diversity (Díaz et al., 2013), or discontinuities in the distribution of measures (e.g., animal body mass) that are expected to relate to overall ecological health and resource availability (Nash et al., 2014; Angeler et al., 2016). There are a limited number of metrics that incorporate both spatial and temporal perspectives, although the climate sensitivity metric developed by Seddon et al. (2016) does produce a globally consistent decadal estimate, they do not consider neighborhood effects or contiguousness of vegetation.
All of the metrics listed above, at best, estimate the probability of an ecosystem shifting to an alternative regime, such as a shift in species composition or in life-forms (e.g., to grassland or savannah). Considering both the temporal and spatial scale over which these kinds of shifts could occur, it becomes invaluable to consider a longer temporal scale that paleoecological data can provide. Paleoecological datasets such as fossil pollen and charcoal coupled with statistical modeling are already producing important insights about how forest ecosystem processes vary in time and if observable variability is predictive of an ecosystem approaching a threshold. For example, previous studies, based on a metanalysis of over 200 pollen-records (covering ~20,000 years) have estimated the recovery time between periods of forest cover in the tropics to assess regional forest resilience, offering a binary measure of forest or non-forest (Cole et al., 2014). Other paleoecological resilience metrics have entailed innovative analysis of Holocene pollen, charcoal records, functional traits (e.g., bark thickness, plant height), and also diatoms to explore observable forest responses to past biotic and abiotic drivers (e.g., Seddon et al., 2014; Brussel et al., 2018; van der Sande et al., 2019).
Baselines: Providing Trajectories of Intact Forest to Assess Resilience
The approaches listed above allow the range of observed ecosystem responses to be compared to an ecosystem's ecological history. Ecological history is important for determining the degree of change that has occurred from baseline reference conditions (Willis et al., 2005, 2010; Gillson et al., 2011). Moreover, ecological history has been highlighted as crucial to determine the level of intervention required to restore an ecosystem that has been modified by human impacts to a more “naturally functioning state” (Jackson and Hobbs, 2009). Assessing ecological history was undertaken to inform management of island ecosystems, which included consideration of multiple baselines (Nogué et al., 2017). There are also some examples in the policy literature that have highlighted the importance of identifying baselines. For example, the IUCN's “global standard for the identification of Key Biodiversity Areas” have introduced the concept of ecological integrity (criterion c) (IUCN, 2016), which has been defined as “when [an ecosystem's or species'] dominant ecological characteristics (e.g., elements of composition, structure, function, and ecological processes) occur within their natural ranges of variation and can withstand and recover from most perturbations imposed by natural environmental dynamics or human disruptions” (Parrish et al., 2003, p. 852). This definition essentially combines the concepts of intactness and resilience into one. Operationally, the term involves considering both ecological integrity and intactness of the ecological community, which combined refer to the baseline (or historical benchmark) conditions that support intact species assemblages and ecological processes (IUCN, 2016). Baselines therefore, are relevant to identify in intact forest landscapes. Calder and Shuman (2019) introduced an interesting approach to measure resilience by considering the amount and duration of change from a baseline and the potential to measure the rate of recovery. Using paleoecological datasets from Connecticut and Colorado (USA) the authors suggested that identifying baselines are important to determine recovery, an intrinsic feature of resilience. Trends in recovery rate can also be considered, whereby the ability of a system to recover following disturbances slows before approaching a critical threshold (Veraart et al., 2011). However, the challenge of identifying the accurate baseline applies to nearly any time period (e.g., millennia to decades) and disturbance (e.g., temperature increase, wildfire, and land-use change) (Hansen et al., 2016).
Comparison of Studies and Metrics for Measuring Resilience in Intact Forests
While it is clear the question of forest resilience is incredibly complex, locally driven, likely path dependent, and therefore, difficult to assess at a global level; there are international policy prerogatives to prioritize forest areas for conservation that can help us achieve meaningful climate mitigation and adaptation. Hence, there is a societal need to understand the conditions required to allow for the unhindered persistence of forests across biomes as we enter an uncertain climate regime, the Anthropocene (Malhi et al., 2014). Early efforts may be overly simplistic, namely identifying arbitrarily-sized patches of forest globally and hypothesizing that their size and contiguousness necessarily confers a level of resilience (Potapov et al., 2017), but it is an interesting assumption to explore. Fragmentation of previously large patches of forest have likely resulted in higher carbon emissions already (Maxwell et al., accepted) and the processes by which they would be further disturbed are likely to make them more prone to further degradation (Putz and Romero, 2014).
To explore the robustness of the IFL assumption that area confers resilience, we assess published global contemporary and local paleoecological studies across forest biomes for evidence. We were interested whether IFL areas exhibited lower contemporary climate sensitivity than non-intact forest and whether this metric varied by biome and continent. Then we illustrate different metrics for understanding resilience from paleoecological studies across these biomes. Although, the studies chosen were not intended to be exhaustive, they are helpful in informing the range of responses intact forest landscapes have exhibited over paleoecological time to disturbance and how they compare to ecological or engineering resilience approaches for understanding resilience today.
Analysis of Contemporary Datasets
The analysis we present is based on a contemporary measure of climate sensitivity developed by Seddon et al. (2016) partitioned into intact and non-intact forest areas using the intact forest landscapes (IFL) dataset generated by Potapov et al. (2017) and forest cover dataset produced by Hansen et al. (2013). The IFL dataset also uses the Hansen et al. (2013) forest cover dataset to identify contiguous forest areas, as previously described. They have produced a series of analyses, estimating IFL coverage and loss between 2000 and 2013 and 2013–2016. We used the analysis by Seddon et al. (2016) to explore whether there is evidence that the area-based metric for IFLs are less sensitive to climate anomalies, and therefore, potentially more resilient than non-IFL forest areas. Although with the caveat that Seddon et al. (2016) are quantifying “sensitivity” and not resilience directly. Their approach uses Moderate Resolution Imaging Spectroradiometer (MODIS) Enhanced Vegetation Index (EVI) time series from 2000 to 2013 (Solano et al., 2010) and various climate variables to asses “variability,” under the assumption that greater variability indicates higher sensitivity, potentially lower resilience, and therefore, a higher probability of crossing a threshold to an alternative state. To assess ecosystem sensitivity to short-term climate variability, they developed a new metric, the vegetation sensitivity index (VSI), which compares the relative variance of vegetation productivity (EVI) with three ecologically important MODIS-derived climate variables (air temperature, water availability and cloud-cover) for the months in which EVI and climate are found to be related. They used an autoregressive (AR1) multiple linear regression approach, taking the three climate variables and 1-month lagged vegetation anomalies to identify areas with strong vegetation-climate coupling. Their global VSI is generated from aggregating the EVI sensitivities to each climate variable, weighted by the coefficients from the linear regression modeling. The VSI provides a useful dataset to explore contemporary responses to recent climate anomalies; although, with a monthly canopy response interval, this metric would not be capturing longer term responses. Also, the resolution of the data is 5 km, which would be aggregating the climate response over several landcovers in more fragmented landscapes. While they were assessing the sensitivity of all vegetation, not specifically forest, they found that boreal forest and the wet tropical forests of South America, Southeast Asia and Western Africa were among the most “sensitive.” When they parsed the abiotic drivers, tropical forests were more cloud limited and northern latitudes were more temperature sensitive.
For our comparison we focused on the IFL layers for 2000–2013 as that overlapped with the time period of study for Seddon et al. (2016) and non-intact forest pixels for 2013 from Hansen et al. (2013). The details of our analysis are described in Supplementary Materials. We found that across the tropical biome, IFL areas were less sensitive to water availability than non-IFL areas. Southeast Asian (SEA) IFLs were more sensitive than non-IFLs for all climate sensitivity variables (Figure 1), which according to Potapov et al. (2017) represented 6% of IFL area in 2000 but experienced an almost 15% reduction in IFL area. We also found that this region has considerably less forest area considered intact than non-intact as depicted in the map and histograms in Figure 1. South American (SAM) IFLs exhibited lower sensitivity to water availability and African (AFR) IFLs exhibited lower overall climate sensitivity as well as cloud and water sensitivity than non-IFLs (Figure 1). When comparing intact forests within biomes across sub-regions, we found that SEA IFLs were significantly more sensitive than both SAM and AFR IFLs for overall climate sensitivity, water and cloud sensitivity (Figure S1). SEA and SAM IFLs were similarly sensitive to temperature and both were more sensitive than IFLs in Africa.
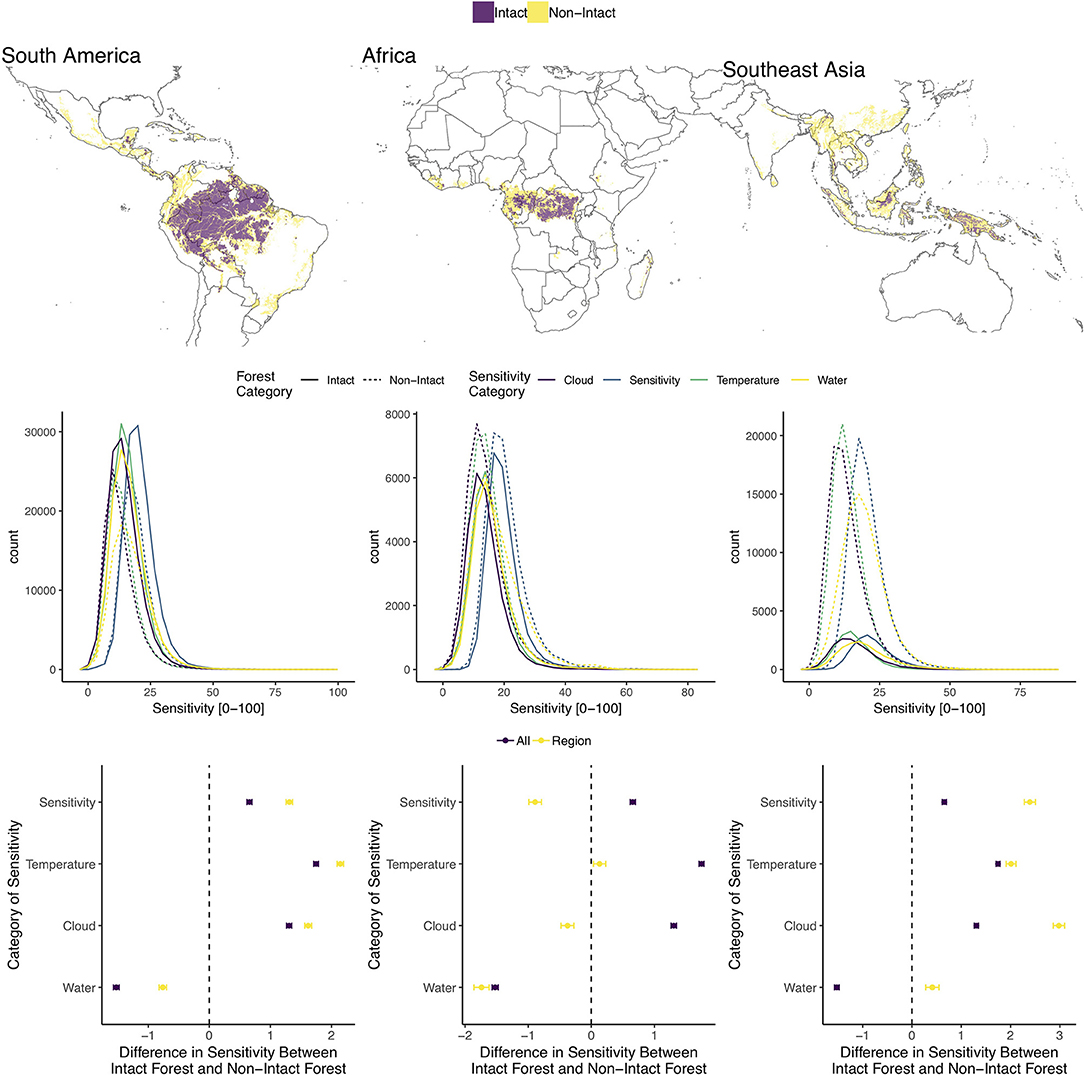
Figure 1. (Top row) Distribution of intact and non-intact pixels for each analyzed continent across the tropics. (Middle row) Histograms of four climate sensitivity metrics for intact (solid) and non-intact (dashed) forests for each continent. (Bottom row) TukeyHSD values, with confidence intervals, comparing climate sensitivity of intact and non-intact forests for each climate sensitivity metric for all (“All”) forest pixels across the tropics and for each continent (“Region”). Negative values indicate intact forest pixels exhibited lower sensitivity for each variable and positive values indicate non-intact forest pixels exhibited lower sensitivity.
Across the boreal biome, IFLs were less sensitive for overall climate and temperature metrics (Figure 2). IFLs in Northern European and Asia (NEA) were less sensitive for all climate metrics than non-IFLs, while the opposite was true for North American (NAM) IFLs. However, NAM IFLs were somewhat less sensitive than NEA IFLs for temperature and cloud metrics, while NEA IFLs were less sensitive to temperature (Figure S1). Potapov et al. (2017) reported that NAM boreal IFL consisted of 24% of global IFL, suffering a 3.3% loss between 2000 and 2013, while NEA boreal IFLs were only 12% of global IFLs and experienced a loss of 4.4%. When contrasting the area of forest in IFLs and non-IFLs across both continents (Figure 2) it is clear that the NEA boreal zone is significantly more fragmented compared to the NAM boreal zone, which exhibits more similar levels of intact and non-intact forest area. We performed the same analysis for temperate and southern boreal forests, although across these biomes very small areas of IFL relative to total forest area remain (Figure S2). Overall, temperate IFLs were more sensitive than non-IFLs with only NEA IFLs being less sensitive to temperature and Oceania (OCE) IFLs being less sensitive to water (Figure S2). Comparisons across biomes are also presented; however the signal was quite complicated.
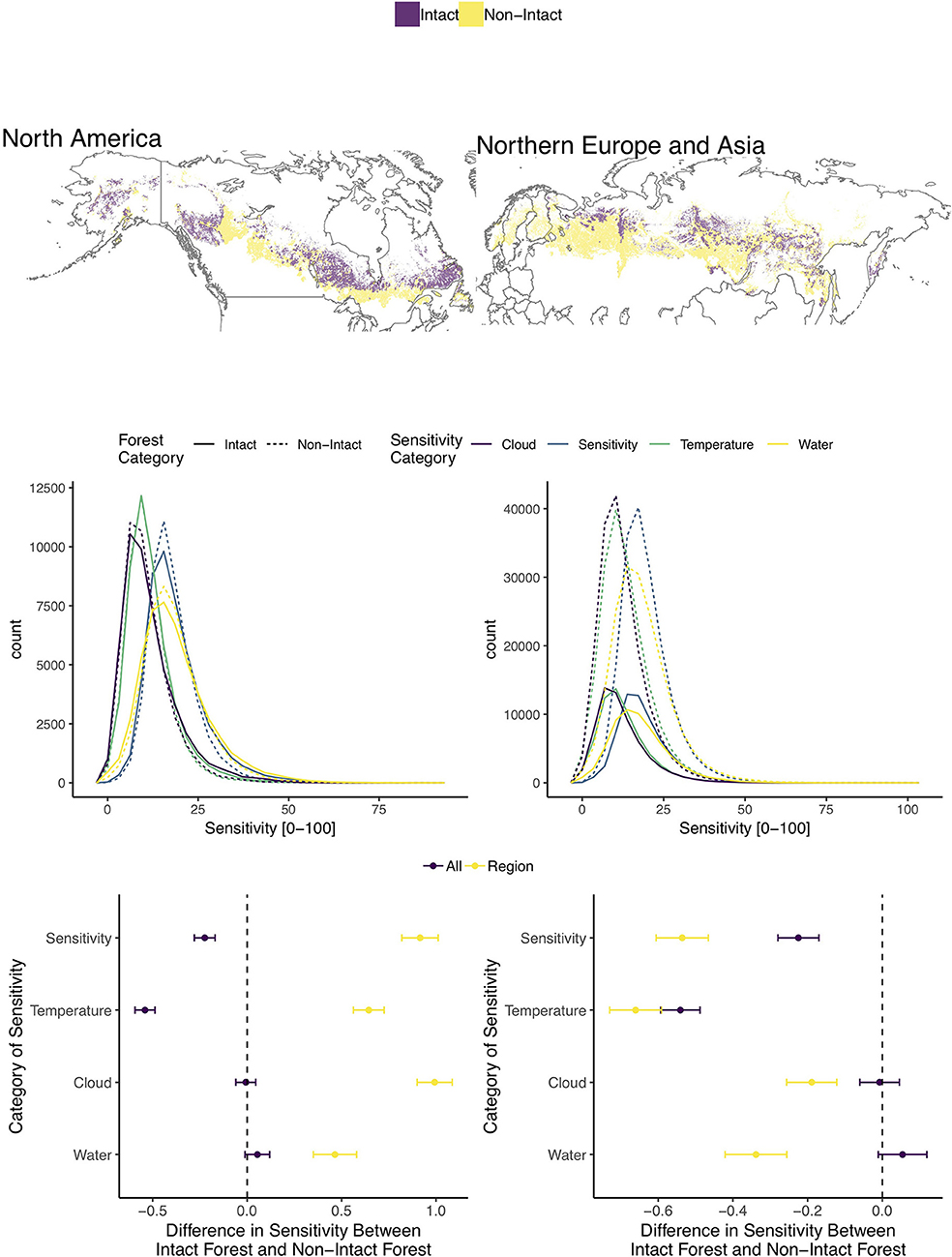
Figure 2. (Top row) Distribution of intact and non-intact pixels for each analyzed continent across the boreal biome. (Middle row) Histograms of four climate sensitivity metrics for intact (solid) and non-intact (dashed) forests for each continent. (Bottom row) TukeyHSD values, with confidence intervals, comparing climate sensitivity of intact and non-intact forests for each climate sensitivity metric for all (“All”) forest pixels across the tropics and for each continent (“Region”). Negative values indicate intact forest pixels exhibited lower sensitivity for each variable and positive values indicate non-intact forest pixels exhibited lower sensitivity.
Identified Paleoecological Studies
Figure 3 presents an overlay of global IFL and non-IFL pixels with the location of several paleoecological studies we discuss as illustrative examples, which are also listed in Table 1. These examples were chosen to highlight the complexity and opportunities that paleoecological approaches can provide in terms of measuring resilience in different landscape configurations and forest systems. These studies use a variety of methods: fossil pollen, charcoal, soil erosion and climate proxies, tree rings, and functional traits. The analysis of these long-term datasets involves statistical analysis from population modeling to rates of change. Studies A and G-I, occurred in a currently fragmented agroforestry landscape that revealed the persistence of forest species over the past 6,000 years (Bhagwat et al., 2012; Nogué et al., 2018). Study B implements paleoecological measures of functional traits and how they may be selected for through changes in disturbance (Brussel et al., 2018). Study C combines lake-sediment charcoal record with fire scars on living spruce trees (Picea) to assess response of this forest system to increasing human pressure since the gold rush (c. 1902) (Gaglioti et al., 2016). Study D presents a study of Nothofagus that revealed a long and stable persistence of this forest system over time (Iglesias et al., 2018). Study E shows a 90,000 year pollen record from an Afromontane site in Cameroon, indicating highly unstable species composition with past climate changes (Lézine et al., 2019). Study F explored the impacts of past human activity on the floristic composition and carbon sequestration of a peatland forest as well as identified “resilience friendly” activities that could effectively conserve and restore this carbon-rich forest system (Hapsari et al., 2018). Finally, study J used population modeling of Symphonia, a Madagascan endemic tree, which revealed rapid oscillations in their metric just before a threshold shift in species composition of the community (Virah-Sawmy et al., 2009).
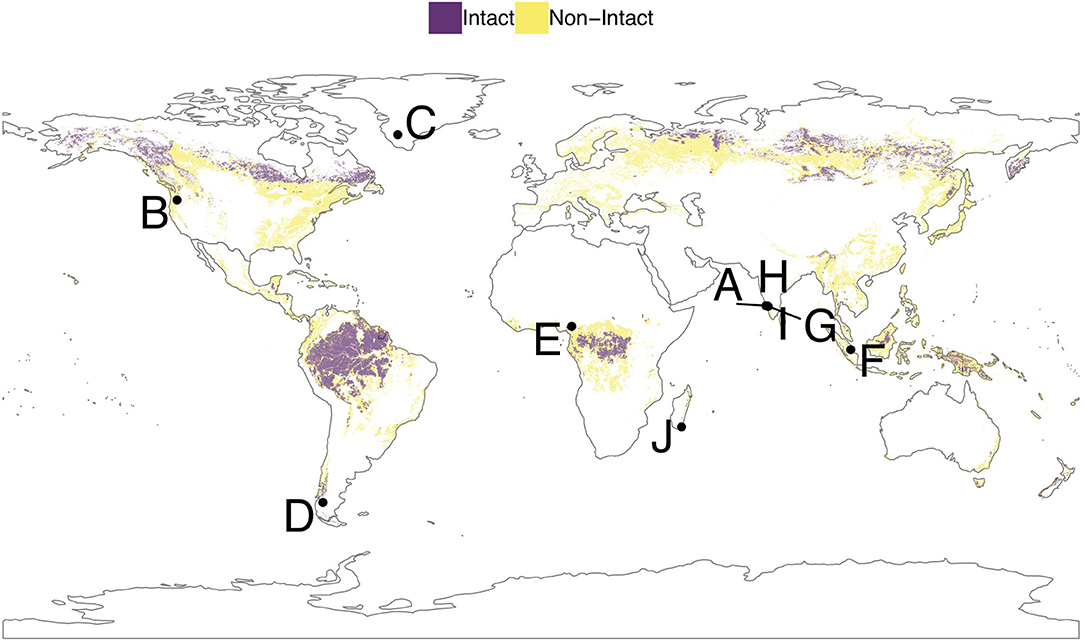
Figure 3. Intact forest landscape (IFL) and non-intact forest pixels with locations of selected paleoecological studies illustrating the range of forest responses to past disturbance. Table 1 lists the details of each study.
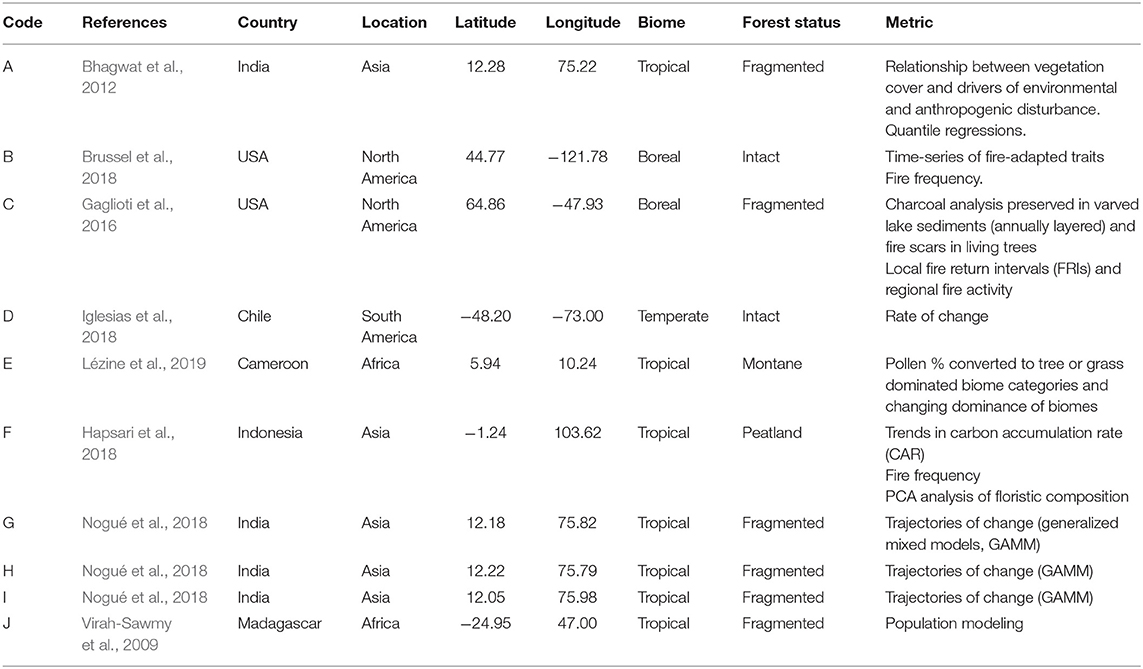
Table 1. List of paleoecological studies from intact forests featured in Figure 1.
Discussion
How Are Intact Forests Responding Currently?
Increasing concentrations of CO2 in the atmosphere combined with changes in temperature, are impacting net ecosystem productivity (NEP) and water use efficiency (WUE) of forests, although the direction of the effect varies by biome. For instance, NEP in northern latitudes appear to be increasing with temperature and the tropics are exhibiting greater sensitivity to CO2 (Fernández-Martínez et al., 2019). There is already contemporary evidence that species composition of forest communities are shifting in response to drying conditions (Fauset et al., 2012) and even with CO2 fertilization, increases in NEP are in areas that are less water limited (Fernández-Martínez et al., 2019). The carbon sink across the tropical forest biome has been relatively neutral since the 1990s, with sequestration rates roughly equaling emissions from deforestation and degradation (Mitchard, 2018). However, there have been spikes in this signal, where the tropics became a net source of carbon during climate extremes (Liu et al., 2017). Trends in carbon uptake of tropical forests in the Amazon are showing signs of slowing as well as enhanced carbon emissions from slowly decaying necromass, which is modeled to be twice the magnitude of the decline in carbon sequestration (Brienen et al., 2016). In addition, there remains considerable uncertainty around the scale of necromass and its vulnerability to increasing fire incidence (Withey et al., 2018). Trends in carbon uptake can vary within the tropics, whereby forests in Africa are more resource limited and smaller, sub-canopy trees can be responsible for 20% of carbon sequestration even though they may only store 11% of stand biomass (Hubau et al., 2019).
Boreal forests are known to be experiencing warming twice the rate of the global average during the twentieth century (Wolken et al., 2011). However, the impact of temperature increase across its range remains unclear (Frégeau et al., 2015; Girardin et al., 2016). Warming is causing increases in the frequency, size and severity of many natural disturbances, including pest outbreaks that are known boreal forest stressors (Millar and Stephenson, 2015) as well as likely increasing rates of soil respiration (German et al., 2012). Although multi-decadal soil warming experiments suggest that increased respiration of microbes may be short-lived with the dominant impact being enhanced root production (Lim et al., 2019). Increasing temperature has been identified as the most likely factor driving increased mortality across North American forests, which has been outpacing the rate of recruitment over the last few decades (van Mantgem et al., 2009). The hotter, and therefore, drier conditions are believed to be interacting with pathogen and insect incidence to create this “novel forest decline” (Wong and Daniels, 2017). Worryingly, suitable climate zones for the boreal biome have been modeled to be moving to higher latitudes one order of magnitude faster than species are expected to be able to migrate (McKenney et al., 2007).
Unlike tropical or temperate systems, old growth boreal systems are not characterized by tall dense stands in equilibrium. Disturbance is a core aspect of this ecosystem, with fire being the dominant driver followed closely by pest incidence and disease. Fire occurs randomly throughout the landscape independent of stand age resulting in a patchy distribution of forest condition. Therefore, it has been argued that this system would be better understood, and therefore, managed at the landscape-scale, whereby a representative distribution of stand ages are conserved to allow for recovery between disturbance events (Kneeshaw et al., 2018). Evidence seems to be growing that management decisions based on the stand-level could be making boreal regions more susceptible to fire and pest damage regardless of biotic factors (Seidl et al., 2016). Our results suggest IFLs in the boreal biome overall show lower sensitivity to temperature anomalies than non-intact forests, suggesting contiguous forest areas could be more resilient for this biome.
What Does the Paleoecological Record Suggest?
Palaeoecology offers a longer temporal perspective that is important for assessing past forest responses; particularly, being able to assess whether a previous regime shift was driven by a climatological (“extrinsic”) or ecological (“intrinsic”) event (Williams et al., 2011; Seddon, 2017). Using examples around the world, the aim of this section is to discuss: stability, population, and fragment dynamics as well as forest responses to disturbances.
The paleoecological literature reveals several records that have displayed stability or persistence over time, even with changes in environmental conditions (e.g., Urrego et al., 2013). Therefore, caution needs to be taken when analyzing contemporary changes in variability. Paleoecological methods for detecting stability or variability can rely on the rate of change in a pollen time-series (Iglesias et al., 2018) as well as turnover in pollen composition (e.g., Birks and Birks, 2008). First, in Patagonia, the Nothofagus (southern beeches) forest was found to have prevailed relatively unchanged for the past 9,800 years until the twentieth century by calculating the rate of change in pollen records, which was equal to zero (Iglesias et al., 2018) (Figure 3, Study D). This stability could be attributed to Nothofagus' resistance to environmental change, its ability to rapidly recover or trade-offs in the plasticity within Nothofagus' species traits. Stability has also been found in the Afromontane forest in the Eastern Arc Mountains of Tanzania for ~48,000 years (Finch et al., 2009) as well as in some tepui mountains in Venezuela (Rull, 2005; Nogué et al., 2009). However, we still do not understand the underlying drivers of this dynamic.
In other regions of the world, paleoecological models have found discernible changes in population dynamics that preceded a tipping point. For instance, in Malagasy littoral forest remnants, authors studied the population dynamics of an endemic tree (Symphonia) (Figure 3, Study J). The results of this case study showed steeper oscillations in the response variance (e.g., species dominance) to a climatic change threshold (Virah-Sawmy et al., 2009), suggesting in this system erratic oscillations provided advance warning of a threshold being reached. At the same time, population models showed that overall, there was coexistence between Symphonia and another local species, Erica, unless the community was located in a nutrient-rich substrate. In this case, competition seems to have promoted a decline in Symphonia, demonstrating that local factors may determine the survival of certain species undergoing abiotic stresses like climate change.
The Western Ghats in India also provides an illustrative example of dynamism in species composition over long-time scales in a fragmented landscape. This region exhibits high levels of forest fragmentation due to heavy pressure to support agricultural activities (Giriraj et al., 2010), which is a common reality across tropical forest regions (Karanth and DeFries, 2010). Using paleoecological data obtained from four small forests fragments (~5 ha) in a coffee (Coffea arabica) agroforestry landscape in Karnataka, India, it was possible to reconstruct 7,500 years of vegetation dynamics (Figure 3, Studies A and G-I). Taking into account regional climatic changes over this same time period (e.g., monsoon activity) and other environmental variables (e.g., anthropogenic fire, and soil erosion), results suggested that fire was responsible for maintaining low tree cover in the landscape over the past 3,500 years. In addition, it appeared that retaining tree cover in this fragmented landscape, was key to maintaining its ecological resilience to subsequent environmental and anthropogenic disturbance (Bhagwat et al., 2012). In addition, by investigating the dynamics of vegetation (tropical forest, cultivated, grasses, and herbaceous taxa) and environmental variables (biomass burning, canopy closure, and habitat specialist trees) over the past 900 years across four of the Western Ghats' fragments, it was found that agricultural activity had not precipitated a collapse of the Western Ghats forests, but instead had enhanced the dynamism of vegetation within and between forest patches. Furthermore, these results are also relevant for conservation as it suggests that small and informally protected-forest patches (e.g., sacred groves) in human-dominated agricultural landscape might sustain high quality tropical forests, temporally and spatially (Bhagwat et al., 2014; Nogué et al., 2018). Although, this heightened dynamism could indicate lower resilience of these fragments to novel climatic conditions.
Finding general patterns and responses to disturbances (e.g., temperature increase and fire) for the boreal forest in the paleoecological record proved to be challenging. Most of the studies are focused on long-term shifts, and the fire regime data are spatially limited and generally of low resolution. Therefore, we discuss major regional temperature-related changes together with local fire responses. First, paleoecological studies of forest responses to the increase of temperature across the Pleistocene-Holocene boundary (Levesque et al., 1997; Hou et al., 2007) suggest different patterns across the boreal biome worldwide (Shuman et al., 2002a,b; Wolken et al., 2011). For example, in Alaska, spruce (Picea) forests have been dominant since the early Holocene; however, during the deglacial period into the early Holocene the woody vegetation was deciduous (e.g., Betula, Salix, and Populus). Second, in addition to changing temperatures, wildfire frequency also seems to control the long-term dynamics of species composition in boreal forests (Carcaillet et al., 2010). However, there is good evidence that in Alaska, climate change drove species composition which in turn drove changes in the fire regime (e.g., Lynch et al., 2002; Higuera et al., 2009). When looking at specific case studies this complexity arises further. Brussel et al. (2018) (Figure 3, Study B) found that fire frequency may have driven selection pressure for fire-adapted traits. This finding is consistent with 14C dated and botanically identified charcoal (Frégeau et al., 2015) together with sedimentary sequences (Carcaillet et al., 2010) showing the maintenance of both fire-prone and less fire-prone tree species over time. At a very local scale, Gaglioti et al. (2016) (Figure 3, Study C) found that even with a 4-fold increase in regional burning following the arrival of gold miners near to Fairbanks, Alaska, the permafrost and watershed of Ace Lake stayed mainly intact. However, these authors worry that the legacy of this heightened fire activity may make this region more susceptible to megafires in the future as temperatures continue to increase. The dynamics described above may suggest the ability of the boreal biome to maintain its structure and function following fire-related disturbances, which could be interpreted as an overall resilience to changes in fire regimes (Carcaillet et al., 2010; Frégeau et al., 2015). However, Johnstone and Chapin (2006) showed that too many fires might also push the composition from evergreen to deciduous forest in the Yukon region.
Can We Distinguish Natural Variability From Variability Approaching a Tipping Point?
If we consider an engineering approach to resilience, how can we discern the natural variability of a dynamic ecosystem from increasing variability due to its approaching a threshold? The danger of using an equilibrium/stable state model of resilience for a complex adaptive system, like a forest ecosystem, is that management to maintain stability may have the effect of reducing resilience through dampening its natural variability (Holling and Meffe, 1996). Also, without considering a comparable baseline of responses to past disturbance, it is very difficult to establish whether contemporarily observed variation is predicting the approach of a system threshold. Being able to combine the temporal-scale of paleoecological studies with short-term and more spatially explicit contemporary ecological studies, is difficult. For one, from the paleoecological record, it is still challenging to analyse species interactions or how vegetation dynamics may have varied spatially in a study landscape. Therefore, being able to differentiate whether more biodiverse vegetation is necessarily more sensitive to recent climate changes (e.g., Willis et al., 2018), or if that “sensitivity” is actually a function of vegetation dynamics within the normal historical range of those ecosystems, remains a challenge.
Southeast Asia is home to more than half of tropical peatland area and 77% of tropical peat volume, storing 68.5 Gt of carbon (Page et al., 2011). Deforestation since 1990 on Southeast Asian peatlands has been dramatic, with only 28% of original peat swamp forest cover remaining (Miettinen et al., 2012). Deforested peatland areas have also been more prone to fire incidence, worsening carbon emissions as well as lower carbon accumulation rates (CAR), which might suggest a shift in regime. A paleoecological study by Hapsari et al. (2018) (Figure 3, Study F) were able to discern a reduction in floristic composition and CAR with human activities during the Malayu Empire 1,200 years ago in Central Sumatra (Indonesia); however, with reduced pressure due to population migration they saw a rapid recovery of both measures within 60–170 years of abandonment. From their findings, they were able to suggest appropriate methods for sustainable use of peatswamp forests as well as appropriate species for reforestation. Unfortunately, CAR across tropical peatlands are expected to slow with increasing temperatures and to become a net source by the end of the century (Gallego-Sala et al., 2018). It is unclear the extent to which restoring peatswamp forest would have a dampening effect on those predictions; however, as evidenced by Hapsari et al. (2018) these systems can recover, and therefore, returning them to a more “intact” state would be advisable.
Proposed Mechanisms for Resilience
There are known and hypothesized physiological limits to abiotic drivers across forest species, such as temperature and water availability. However, photosynthesis appears to be more impacted by drought than by temperature (Cusack et al., 2016). Some groups suggest that there are intrinsic system-level sensitivities to water availability across biomes (Ponce-Campos et al., 2013) with the majority of trees exhibiting relatively narrow hydraulic safety margins (Choat et al., 2012). This would indicate low adaptive capacity to rapid changes in temperature and rainfall regimes, resulting in higher mortality rates for trees more at risk of embolism (Trugman et al., 2018). Predictions for the tropics are that species dominance will shift to those with drought tolerant traits, with concomitant loss of evergreen species, particularly in sites exhibiting increasing drought duration (Aguirre-Gutiérrez et al., 2019; Bartlett et al., 2019). This transition is likely to entail large-scale die-off of the tallest trees in old-growth forest, resulting in a pulse in global carbon emissions (McDowell and Allen, 2015). For instance, heightened mortality has been observed for the largest trees or those with the lowest wood density (Phillips et al., 2010), a dynamic that has been echoed in experimental conditions replicating long-term droughts via through-fall exclusion (TFE) (da Costa et al., 2010). However, in Nothofagus dominated forest in Patagonia, it was the trees exhibiting the most variable growth patterns, not the largest or oldest, that were more likely to die (Suarez et al., 2004). Studies in Latin America have found the smallest and youngest trees exhibiting greater mortality, with concomitant impacts on community composition (Enquist and Enquist, 2011). In addition, a pan-tropical, tree-level study by Johnson et al. (2018) found that climatic conditions, such as mean annual temperature (MAT) and cumulative water deficits (CWD), were better predictors of mortality across size classes, suggesting that size alone was not a reliable predictor.
The primary underlying mechanisms inducing tree mortality seems to be a combination of hydraulic failure and availability of non-structural carbohydrates (NSCs) (Adams et al., 2017; McDowell et al., 2018). How these dynamics manifest at the community-level via competitive or facilitative plant-plant interactions will also determine the resilience of a forest ecosystem to changing climate (Ploughe et al., 2019). For instance, in the Canadian boreal region, Luo and Chen (2015) identified a significant increase in tree mortality over their three decade study and found the dominant drivers appeared to be due to resource competition between individual trees and stand age. Allen et al. (2015) review how tree mortality might be impacted by climate change and identify several worrying drought trends, namely: droughts will be hotter, they will occur everywhere, mortality can be faster with hotter droughts, deaths can outpace growth creating novel forest systems and vapor pressure deficit increases non-linearly with temperature. Worryingly, it is emerging that the compound effects of extreme vapor pressure deficit (VPD) and low soil moisture are occurring with increasing frequency due to reinforcing feedback mechanisms (Zhou et al., 2019).
Another commonality across biomes would be a relationship between forest resilience and biodiversity. Seminal work in grasslands has shown the importance of diverse systems for being able to maximize niche differentiation, thereby enhancing productivity and stability (Tilman et al., 2014). However, this assumes biodiversity measures relate to functional diversity (Cadotte et al., 2011). This recognition has led to the advancement of trait-based diversity assessments, which focus on the functional attributes of individuals based on their morphological, physiological and phenological attributes, how those contribute to performance traits like productivity and their ability to persist in a community through environmental filters (Violle et al., 2007). This has been simulated for the Amazon using a range of plant functional traits, which found with greater biodiversity biomass recovery could be up to 95% of pre-shock levels, granted only after several centuries (Sakschewski et al., 2016). Díaz et al. (2013) proposed a framework assessing specific effect functions (SEF) and specific response functions (SRF), where the former relates to the outcome of a species' functional traits on an ecosystem and the latter relates to the ability of a species to maintain its functions while undergoing environmental change. They add a community-level risk assessment for an ecosystem service by considering the phylogenetic diversity of the SEF, which if high would suggest redundancy of the functional response to environmental change. Much of these assessments are based on community weighted means for each species, and therefore, rely on their relative abundance to relate to a community-level resilience. However with current understanding, it is difficult to predict how important rare species may be for an ecosystem's resilience, as sometimes these species can have a unique combination of functional traits that may disproportionately impact a systems' adaptive capacity during a time of disturbance (Mouillot et al., 2013). Nevertheless, studies have found that forest systems dominated by a small number of species can be more vulnerable to rapid climate changes (Fensham et al., 2015).
Climate change impacts on biodiversity are predicted to be significant, and particularly if species are not able to thermally adapt to new conditions, their loss could be greater than losses due to drought or deforestation (Feeley et al., 2012). CO2 fertilization seems to be beneficial for fast-growing, light-demanding pioneer species (Cusack et al., 2016), which as highlighted above may be more vulnerable to hydraulic failure during drought conditions. One method for assessing the impact of climate change on community-level biodiversity is the community temperature index (CTI), whereby species relative abundance and their known thermal optima are combined to produce an average temperature preference for the community (Stuart-Smith et al., 2015; Gaüzère et al., 2018). With repeat measures in the same site, it can be possible to assess whether more thermophilic species are migrating into a community, suggesting one mode of adaptation to climate change. This dynamic has been found along an Andean transect since 1990, whereby plot-level CTI have been increasing with species migration up-slope. However, these species movements have been halted at the barrier between ecotones (e.g., montane forest to cloud forest) (Fadrique et al., 2018), suggesting physically-imposed limits to species migration. The 90,000 year pollen record from the Afro-montane forest of Cameroon (Figure 3, Study E) showed a highly variable upper limit to the forest zone with a relatively stable lower forest zone (Lézine et al., 2019), also indicating long-term, complex constraints on species dispersal. This dynamic contrasts starkly with the stability the Afromontane forests in Tanzania exhibited over a 48,000-years record (Finch et al., 2009) and further complicates the question of how spatially constrained “natural variability” or a “baseline” for current forest dynamics can be compared.
Do Larger Forest Patch Areas Confer Resilience?
By combining paleoecological and contemporary perspectives, can we answer the question of whether size of forest is positively correlated with resilience? We do know that tropical forests across the world are threatened by fragmentation (e.g., the conversion of large areas of forest into smaller patches) as a result of rapid land use conversion from forest to agriculture (Curtis et al., 2018; Taubert et al., 2018), with extreme predictions resulting in severe fragmentation of half the Amazon Basin (Gomes et al., 2019). We also know that the process of fragmentation has been associated with large-scale biodiversity loss (Laurance et al., 2011; Haddad et al., 2015) and reduction of carbon sequestration (Qie et al., 2017), due to edge effects and habitat loss. However, the debate as to whether several smaller forest fragments vs. the equivalent forest area in one contiguous patch, keeping total habitat area constant, have significantly different biodiversity outcomes is ongoing (Fletcher et al., 2018; Fahrig, 2019). It is not clear the extent to which this can be said of carbon sequestration rates or forest resilience more generally, whereby following habitat fragmentation, vegetation dynamics of intact forests' systems are altered. For example, fragments might display an increase in the frequency and/or amplitude of population, community, and landscape dynamics initially, and then gradually stabilize and approximate the pre-fragmentation conditions (Laurance et al., 2002, 2011). Conversely, fragments may not gradually stabilize toward the pre-fragmentation conditions, and potentially, undergo a regime shift. Unfortunately, more evidence is needed before relying on an area-based definition for predicting the resilience of forest fragments.
Studies from the tropics suggest that forest patches experience exceptionally large variability in vegetation dynamics especially when disturbances associated with shifting cultivation are frequent; yet these records are restricted to relatively short temporal scales (<50 years). In a >30 year project in Amazonian forest fragments, Laurance et al. (2011) found that different fragmented landscapes can diverge to a surprising degree in species composition and dynamics. Understanding how and why the dynamics of fragmented landscapes are altered will improve our ability to predict and manage the consequences of intact forest landscape fragmentation. Our comparison of IFL areas and climate sensitivity suggest that larger contiguous forest patches in the tropics are exhibiting lower sensitivity to water availability, and therefore, may be more resilient in their response to CO2 fertilization. This would be consistent with canopy drought responses, measured as anomalies in EVI, which found shorter recovery times in dense forests compared to secondary and degraded forests over the Amazon for a similar time period to our analysis (Anderson et al., 2018). Additionally, our results identified IFLs in Africa as being less sensitive than both South American and Southeast Asian tropical forest. This seems to be corroborated by analysis of canopy responses to drought over West and Central Africa, using QuickSCAT canopy backscatter data, which found significantly shorter recovery times for the continent compared to similar climate shocks in South America. The authors attributed this observed resilience to the protracted drying trend West and Central Africa has been undergoing since the 1970s (Asefi-Najafabady and Saatchi, 2013).
In boreal systems, the dynamic between fragmentation, fire and timber harvesting are complex and it is difficult to discern whether there are discernible changes with climate change. In principle, due to the boreal region's adaptation to disturbance it is considered to be less impacted by fragmentation (Harper et al., 2015). A 30-years Landsat based analysis of the Canadian boreal region showed lower rates of fragmentation and higher rates of recovery in forests being actively harvested than unmanaged areas driven by fire. However, the authors posit this could relate to harvesting occurring in more productive and less fragmented forests, while more fragmented forest areas could be more susceptible to fire (Hermosilla et al., 2019). Lehsten et al. (2016) focused specifically on the boreal shield and boreal plains to compare fire dynamics within managed and unmanaged systems in a similar ecotone. They found that managed forest areas, particularly fire suppression activity, not only did not reduce the number of large fires but decreased the natural phenomenon of fuel fragmentation, increasing the probability of more severe fires. Finally, fragmentation in the boreal region can also be driven by climate change itself, whereby climate suitability of species may shift so dramatically over the next several decades that populations of vegetation, birds, and mammals could be physically bifurcated at the continental-scale (Murray et al., 2017) or sporadic thawing of permafrost may shift currently forested areas to treeless wetlands (Carpino et al., 2018).
Conclusions
This paper was intended to summarize the range of observable responses of intact forest to environmental change and a selection of contemporary and paleoecological metrics used to assess forest resilience to these changes (see Figure 4). Primarily, resilience metrics seem to fall into three main categories, namely measures of variability, linear trends, and stability of observable forest response such as carbon uptake, species composition, and recovery rate. In general, these metrics do not describe the underlying driver that may be reducing the overall resilience of a forest system, which are included in Figure 4 and have been reviewed at length by Malhi et al. (2014). However, they are, or should be, intended to assess the ability of a forest ecosystem to respond to climate changes, and therefore, will be an aggregated measure at the community level. The analysis we performed and presented was comparing the variability in canopy response to climate anomalies for large, contiguous intact forest areas with non-intact forests across and within biomes. We do not assert that the dataset produced by Seddon et al. (2016) necessarily captures the underlying mechanism that may be causing an increase in discernible vegetation sensitivity to climate anomalies, as there may be “intrinsic” ecosystem dynamics contributing to climate responses of vegetation that are not currently captured by coarse-resolution imagery (e.g., degradation or low adaptive capacity).
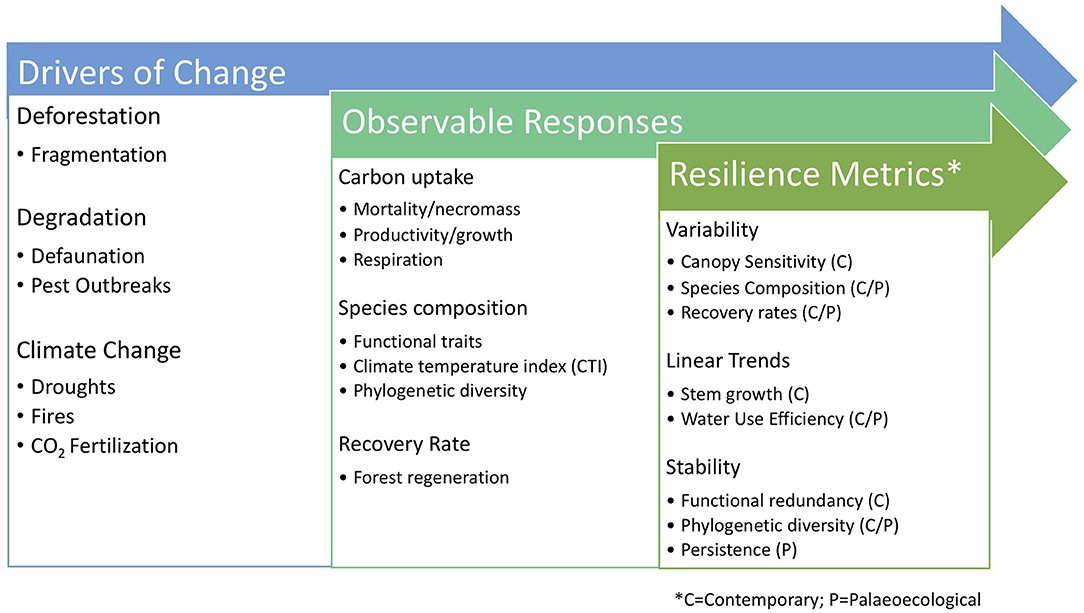
Figure 4. Summary figure of key drivers of change to forests, observable responses, and the existing contemporary and paleoecological metrics to assess the resilience of forest ecosystems to these drivers of change.
By comparing canopy responses within biomes rather than between them, our results suggest that intact forest areas exhibit lower sensitivity to climate drivers that a biome is known to be susceptible to, namely temperature in the boreal region and water availability in the tropics. However, this pattern varied across continents within the same biome. For instance, African forests in the tropics seem to exhibit the lowest canopy sensitivity to climate anomalies and are known to have experienced significant climate variability, which has perhaps selected for more drought resilient traits. If we take exposure to past climate variability as a predictor of future vulnerability, the tropical forests of Southeast Asia, which are dominated by tall trees that have experienced little seasonality historically and which our analysis shows to be exhibiting the highest sensitivity to climate anomalies, would be particularly important for understanding the mechanisms of forest resilience.
Even by combining contemporary and paleoecological perspectives, it remains up for debate how forest resilience will manifest under rapidly accelerating climate change and whether insights can be generalized for biomes or regions. Theory as well as evidence from the literature reveals that resilience is an emergent property of a complex system. Individual tree responses to disturbance are influenced by life histories and functional attributes, and therefore, the ecosystem-level response will, to some extent, be path dependent. At the century to millennium-scale, paleoecology tells us that many intact forests have persisted and been able to adapt to new environmental conditions, which may entail shifts in species, or vegetation structure. However, it is over the short term that many in the forest research community are looking to ensure resilience of forest systems, and particularly, maintain forests as reliable storage of carbon and habitat for threatened biodiversity. A precautionary approach over this time frame, might be to prioritize conservation of large forest areas as they have a higher probability of supporting greater biodiversity and generally have lower opportunity costs to protect. However, with the benefit of hindsight through long-term studies, we can see that past disturbance does enhance aspects of resilience (e.g., recovery rate) and even forest in fragmented landscapes can persist for millennia. With the scale and rapidity of precipitation and temperature changes expected, the immediate future of carbon storage and sequestration services of forest is hard to predict. However, the greater uncertainty seems to be the degree to which the global community is able to dramatically reduce carbon emissions, and by extension, at what concentration of CO2 the atmosphere will eventually stabilize. Ensuring carbon storage in forests cannot be achieved without dramatic reductions in fossil fuel emissions.
Author Contributions
Idea for review developed and analysis performed by AM. AM and SN contributed equally to writing and editing the manuscript.
Funding
AM was supported by NERC grants NE/P001092/1 and NE/P00394X/1 and the MacArthur Foundation.
Conflict of Interest
The authors declare that the research was conducted in the absence of any commercial or financial relationships that could be construed as a potential conflict of interest.
Acknowledgments
We wish to thank Alistair Seddon and co-authors for providing their climate sensitivity data layers for analysis and Jesse Morris and Mary Edwards for invaluable advice regarding current development in boreal forest research.
Supplementary Material
The Supplementary Material for this article can be found online at: https://www.frontiersin.org/articles/10.3389/ffgc.2019.00057/full#supplementary-material
References
Adams, H. D., Zeppel, M. J. B., Anderegg, W. R. L., Hartmann, H., Landhäusser, S. M., Tissue, D. T., et al. (2017). A multi-species synthesis of physiological mechanisms in drought-induced tree mortality. Nat. Ecol. Evol. 1, 1285–1291. doi: 10.1038/s41559-017-0248-x
Aguirre-Gutiérrez, J., Oliveras, I., Rifai, S., Fauset, S., Adu-Bredu, S., Affum-Baffoe, K., et al. (2019). Drier tropical forests are susceptible to functional changes in response to a long-term drought. Ecol. Lett. 22, 855–865. doi: 10.1111/ele.13243
Allen, C. D., Breshears, D. D., and McDowell, N. G. (2015). On underestimation of global vulnerability to tree mortality and forest die-off from hotter drought in the Anthropocene. Ecosphere 6, 1–55. doi: 10.1890/ES15-00203.1
Allen, C. R., Angeler, D. G., Cumming, G. S., Folke, C., Twidwell, D., and Uden, D. R. (2016). Quantifying spatial resilience. J. Appl. Ecol. 53, 625–635. doi: 10.1111/1365-2664.12634
Anderegg, W. R. L., Konings, A. G., Trugman, A. T., Yu, K., Bowling, D. R., Gabbitas, R., et al. (2018). Hydraulic diversity of forests regulates ecosystem resilience during drought. Nature 561, 538–541. doi: 10.1038/s41586-018-0539-7
Anderson, L. O., Neto, G. R., Cunha, A. P., Fonseca, M. G., Moura, Y. M., de Dalagnol, R., et al. (2018). Vulnerability of Amazonian forests to repeated droughts. Philos. Trans. R. Soc. B Biol. Sci. 373:20170411. doi: 10.1098/rstb.2017.0411
Angeler, D. G., Allen, C. R., Barichievy, C., Eason, T., Garmestani, A. S., Graham, N. A. J., et al. (2016). Management applications of discontinuity theory. J. Appl. Ecol. 53, 688–698. doi: 10.1111/1365-2664.12494
Asefi-Najafabady, S., and Saatchi, S. S. (2013). Response of African humid tropical forests to recent rainfall anomalies. Philos. Trans. R. Soc. B Biol. Sci. 368:20120306. doi: 10.1098/rstb.2012.0306
Baho, D. L., Allen, C. R., Garmestani, A., Fried-Petersen, H. B., Renes, S. E., Gunderson, L. H., et al. (2017). A quantitative framework for assessing ecological resilience. Ecol. Soc. 22, 1–17. doi: 10.5751/ES-09427-220317
Barlow, J., Gardner, T. A., Lees, A. C., Parry, L., and Peres, C. A. (2012). How pristine are tropical forests? An ecological perspective on the pre-Columbian human footprint in Amazonia and implications for contemporary conservation. Adv. Environ. Conserv. 151, 45–49. doi: 10.1016/j.biocon.2011.10.013
Barlow, J., Lennox, G. D., Ferreira, J., Berenguer, E., Lees, A. C., Nally, R. M., et al. (2016). Anthropogenic disturbance in tropical forests can double biodiversity loss from deforestation. Nature 535, 144–147. doi: 10.1038/nature18326
Bartlett, M. K., Detto, M., and Pacala, S. W. (2019). Predicting shifts in the functional composition of tropical forests under increased drought and CO2 from trade-offs among plant hydraulic traits. Ecol. Lett. 22, 67–77. doi: 10.1111/ele.13168
Betts, M. G., Wolf, C., Ripple, W. J., Phalan, B., Millers, K. A., Duarte, A., et al. (2017). Global forest loss disproportionately erodes biodiversity in intact landscapes. Nature 547, 441–444. doi: 10.1038/nature23285
Bhagwat, S. A., Nogué, S., and Willis, K. J. (2012). Resilience of an ancient tropical forest landscape to 7,500 years of environmental change. Biol. Conserv. 153, 108–117. doi: 10.1016/j.biocon.2012.05.002
Bhagwat, S. A., Nogué, S., and Willis, K. J. (2014). Cultural drivers of reforestation in tropical forest groves of the Western Ghats of India. For. Ecol. Manag. 329, 393–400. doi: 10.1016/j.foreco.2013.11.017
Birks, H. J. B., and Birks, H. H. (2008). Biological responses to rapid climate change at the Younger Dryas—Holocene transition at Kråkenes, western Norway. Holocene 18, 19–30. doi: 10.1177/0959683607085572
Blonder, B., Moulton, D. E., Blois, J., Enquist, B. J., Graae, B. J., Macias-Fauria, M., et al. (2017). Predictability in community dynamics. Ecol. Lett. 20, 293–306. doi: 10.1111/ele.12736
Brienen, R. J. W., Schöngart, J., and Zuidema, P. A. (2016). “Tree rings in the tropics: insights into the ecology and climate sensitivity of tropical trees,” in Tropical Tree Physiology, eds G. Goldstein and L. S. Santiago (Springer International Publishing). doi: 10.1007/978-3-319-27422-5_20
Brussel, T., Minckley, T. A., Brewer, S. C., and Long, C. J. (2018). Community-level functional interactions with fire track long-term structural development and fire adaptation. J. Veg. Sci. 29, 450–458. doi: 10.1111/jvs.12654
Cadotte, M. W., Carscadden, K., and Mirotchnick, N. (2011). Beyond species: functional diversity and the maintenance of ecological processes and services. J. Appl. Ecol. 48, 1079–1087. doi: 10.1111/j.1365-2664.2011.02048.x
Calder, W. J., and Shuman, B. (2019). Detecting past changes in vegetation resilience in the context of a changing climate. Biol. Lett. 15:20180768. doi: 10.1098/rsbl.2018.0768
Carcaillet, C., Richard, P. J. H., Bergeron, Y., Fréchette, B., and Ali, A. A. (2010). Resilience of the boreal forest in response to Holocene fire-frequency changes assessed by pollen diversity and population dynamics. Int. J. Wildland Fire 19, 1026–1039. doi: 10.1071/WF09097
Carpenter, S., Walker, B., Anderies, J. M., and Abel, N. (2001). From metaphor to measurement: resilience of what to what? Ecosystems 4, 765–781. doi: 10.1007/s10021-001-0045-9
Carpino, O. A., Berg, A. A., Quinton, W. L., and Adams, J. R. (2018). Climate change and permafrost thaw-induced boreal forest loss in northwestern Canada. Environ. Res. Lett. 13:084018. doi: 10.1088/1748-9326/aad74e
Choat, B., Jansen, S., Brodribb, T. J., Cochard, H., Delzon, S., Bhaskar, R., et al. (2012). Global convergence in the vulnerability of forests to drought. Nature 491, 752–755. doi: 10.1038/nature11688
Cole, L. E., Bhagwat, S. A., and Willis, K. J. (2014). Recovery and resilience of tropical forests after disturbance. Nat. Commun. 5:3906. doi: 10.1038/ncomms4906
Cumming, G. S. (2011). Spatial resilience: integrating landscape ecology, resilience, and sustainability. Landsc. Ecol. 26, 899–909. doi: 10.1007/s10980-011-9623-1
Curtis, P. G., Slay, C. M., Harris, N. L., Tyukavina, A., and Hansen, M. C. (2018). Classifying drivers of global forest loss. Science 361, 1108–1111. doi: 10.1126/science.aau3445
Cusack, D. F., Karpman, J., Ashdown, D., Cao, Q., Ciochina, M., Halterman, S., et al. (2016). Global change effects on humid tropical forests: evidence for biogeochemical and biodiversity shifts at an ecosystem scale. Rev. Geophys. 54, 523–610. doi: 10.1002/2015RG000510
da Costa, A. C. L., Galbraith, D., Almeida, S., Portela, B. T. T., da Costa, M., de Athaydes Silva, J. Jr., et al. (2010). Effect of 7 year of experimental drought on vegetation dynamics and biomass storage of an eastern Amazonian rainforest. New Phytol. 187, 579–591. doi: 10.1111/j.1469-8137.2010.03309.x
Dakos, V., Kéfi, S., Rietkerk, M., van Nes, E. H., and Scheffer, M. (2011). Slowing down in spatially patterned ecosystems at the brink of collapse. Am. Nat. 177, E153–E166. doi: 10.1086/659945
de Nascimento, L., Willis, K. J., Fernández-Palacios, J. M., Criado, C., and Whittaker, R. J. (2009). The long-term ecology of the lost forests of La Laguna, Tenerife (Canary Islands). J. Biogeogr. 36, 499–514. doi: 10.1111/j.1365-2699.2008.02012.x
Díaz, S., Purvis, A., Cornelissen, J. H., Mace, G. M., Donoghue, M. J., Ewers, R. M., et al. (2013). Functional traits, the phylogeny of function, and ecosystem service vulnerability. Ecol. Evol. 3, 2958–2975. doi: 10.1002/ece3.601
Enquist, B. J., and Enquist, C. A. F. (2011). Long-term change within a Neotropical forest: assessing differential functional and floristic responses to disturbance and drought. Glob. Change Biol. 17, 1408–1424. doi: 10.1111/j.1365-2486.2010.02326.x
Fadrique, B., Báez, S., Duque, Á., Malizia, A., Blundo, C., Carilla, J., et al. (2018). Widespread but heterogeneous responses of Andean forests to climate change. Nature 564, 207–212. doi: 10.1038/s41586-018-0715-9
Fahrig, L. (2019). Habitat fragmentation: a long and tangled tale. Glob. Ecol. Biogeogr. 28, 33–41. doi: 10.1111/geb.12839
Fauset, S., Baker, T. R., Lewis, S. L., Feldpausch, T. R., Affum-Baffoe, K., Foli, E. G., et al. (2012). Drought-induced shifts in the floristic and functional composition of tropical forests in Ghana. Ecol. Lett. 15, 1120–1129. doi: 10.1111/j.1461-0248.2012.01834.x
Feeley, K. J., Malhi, Y., Zelazowski, P., and Silman, M. R. (2012). The relative importance of deforestation, precipitation change, and temperature sensitivity in determining the future distributions and diversity of Amazonian plant species. Glob. Change Biol. 18, 2636–2647. doi: 10.1111/j.1365-2486.2012.02719.x
Fensham, R. J., Fraser, J., MacDermott, H. J., and Firn, J. (2015). Dominant tree species are at risk from exaggerated drought under climate change. Glob. Change Biol. 21, 3777–3785. doi: 10.1111/gcb.12981
Fernández-Martínez, M., Sardans, J., Chevallier, F., Ciais, P., Obersteiner, M., Vicca, S., et al. (2019). Global trends in carbon sinks and their relationships with CO2 and temperature. Nat. Clim. Change 9, 73–79. doi: 10.1038/s41558-018-0367-7
Finch, J., Leng, M. J., and Marchant, R. (2009). Late Quaternary vegetation dynamics in a biodiversity hotspot, the Uluguru Mountains of Tanzania. Quat. Res. 72, 111–122. doi: 10.1016/j.yqres.2009.02.005
Fletcher, R. J., Didham, R. K., Banks-Leite, C., Barlow, J., Ewers, R. M., Rosindell, J., et al. (2018). Is habitat fragmentation good for biodiversity? Biol. Conserv. 226, 9–15. doi: 10.1016/j.biocon.2018.07.022
Folke, C. (2006). Resilience: the emergence of a perspective for social–ecological systems analyses. Glob. Environ. Change 16, 253–267. doi: 10.1016/j.gloenvcha.2006.04.002
Frégeau, M., Payette, S., and Grondin, P. (2015). Fire history of the central boreal forest in eastern North America reveals stability since the mid-Holocene. Holocene 25, 1912–1922. doi: 10.1177/0959683615591361
Froyd, C. A., and Willis, K. J. (2008). Emerging issues in biodiversity & conservation management: the need for a paleoecological perspective. Quat. Sci. Rev. 27, 1723–1732. doi: 10.1016/j.quascirev.2008.06.006
Gaglioti, B. V., Mann, D. H., Jones, B. M., Wooller, M. J., and Finney, B. P. (2016). High-resolution records detect human-caused changes to the boreal forest wildfire regime in interior Alaska. Holocene 26, 1064–1074. doi: 10.1177/0959683616632893
Gallego-Sala, A. V., Charman, D. J., Brewer, S., Page, S. E., Prentice, I. C., Friedlingstein, P., et al. (2018). Latitudinal limits to the predicted increase of the peatland carbon sink with warming. Nat. Clim. Change 8, 907–913. doi: 10.1038/s41558-018-0271-1
Gaüzère, P., Iversen, L. L., Barnagaud, J.-Y., Svenning, J.-C., and Blonder, B. (2018). Empirical predictability of community responses to climate change. Front. Ecol. Evol. 6:186. doi: 10.3389/fevo.2018.00186
German, D. P., Marcelo, K. R. B., Stone, M. M., and Allison, S. D. (2012). The Michaelis-Menten kinetics of soil extracellular enzymes in response totemperature: a cross-latitudinal study. Glob. Change Biol. 18, 1468–1479. doi: 10.1111/j.1365-2486.2011.02615.x
Gibson, L., Lee, T. M., Koh, L. P., Brook, B. W., Gardner, T. A., Barlow, J., et al. (2011). Primary forests are irreplaceable for sustaining tropical biodiversity. Nature 478, 378–381. doi: 10.1038/nature10425
Gillson, L., Ladle, R. J., and Araújo, M. B. (2011). “Baselines, patterns and process,” in Conservation Biogeography (Chichester, UK: Blackwell Publishing Ltd.), 31–44. doi: 10.1002/9781444390001.ch3
Girardin, M. P., Bouriaud, O., Hogg, E. H., Kurz, W., Zimmermann, N. E., Metsaranta, J. M., et al. (2016). No growth stimulation of Canada's boreal forest under half-century of combined warming and CO2 fertilization. Proc. Natl. Acad. Sci. U.S.A. 113:E8406. doi: 10.1073/pnas.1610156113
Giriraj, A., Murthy, M. S., and Beierkuhnlein, C. (2010). Evaluating forest fragmentation and its tree community composition in the tropical rain forest of Southern Western Ghats (India) from 1973 to 2004. Environ. Monit. Assess. 161, 29–44. doi: 10.1007/s10661-008-0724-5
Gomes, V. H. F., Vieira, I. C. G., Salomão, R. P., and ter Steege, H. (2019). Amazonian tree species threatened by deforestation and climate change. Nat. Clim. Change 9, 547–553. doi: 10.1038/s41558-019-0500-2
Haddad, N. M., Brudvig, L. A., Clobert, J., Davies, K. F., Gonzalez, A., Holt, R. D., et al. (2015). Habitat fragmentation and its lasting impact on Earth's ecosystems. Sci. Adv. 1:e1500052. doi: 10.1126/sciadv.1500052
Hansen, M. C., Potapov, P. V., Moore, R., Hancher, M., Turubanova, S. A., Tyukavina, A., et al. (2013). High-resolution global maps of 21st-century forest cover change. Science 342, 850–853. doi: 10.1126/science.1244693
Hansen, W. D., Chapin, F. S., Naughton, H. T., Rupp, T. S., and Verbyla, D. (2016). Forest-landscape structure mediates effects of a spruce bark beetle (Dendroctonus rufipennis) outbreak on subsequent likelihood of burning in Alaskan boreal forest. For. Ecol. Manage. 369, 38–46. doi: 10.1016/j.foreco.2016.03.036
Hapsari, K. A., Biagioni, S., Jennerjahn, T. C., Reimer, P., Saad, A., Sabiham, S., et al. (2018). Resilience of a peatland in Central Sumatra, Indonesia to past anthropogenic disturbance: improving conservation and restoration designs using palaeoecology. J. Ecol. 106, 2473–2490. doi: 10.1111/1365-2745.13000
Harper, K. A., Macdonald, S. E., Mayerhofer, M. S., Biswas, S. R., Esseen, P.-A., Hylander, K., et al. (2015). Edge influence on vegetation at natural and anthropogenic edges of boreal forests in Canada and Fennoscandia. J. Ecol. 103, 550–562. doi: 10.1111/1365-2745.12398
Hermosilla, T., Wulder, M. A., White, J. C., Coops, N. C., Pickell, P. D., and Bolton, D. K. (2019). Impact of time on interpretations of forest fragmentation: three-decades of fragmentation dynamics over Canada. Remote Sens. Environ. 222, 65–77. doi: 10.1016/j.rse.2018.12.027
Higuera, P. E., Brubaker, L. B., Anderson, P. M., Hu, F. S., and Brown, T. A. (2009). Vegetation mediated the impacts of postglacial climate change on fire regimes in the south-central Brooks Range, Alaska. Ecol. Monogr. 79, 201–219. doi: 10.1890/07-2019.1
Hisano, M., Searle, E. B., and Chen, H. Y. H. (2018). Biodiversity as a solution to mitigate climate change impacts on the functioning of forest ecosystems. Biol. Rev. 93, 439–456. doi: 10.1111/brv.12351
Holling, C. S. (1973). Resilience and Stability of Ecological Systems. Annu. Rev. Ecol. Syst. 4, 1–23. doi: 10.1146/annurev.es.04.110173.000245
Holling, C. S., and Meffe, G. K. (1996). Command and control and the pathology of natural resource management. Conserv. Biol. 10, 328–337. doi: 10.1046/j.1523-1739.1996.10020328.x
Hou, J., Huang, Y., Oswald, W., Foster, D., and Shuman, B. (2007). Centennial-scale compound-specific hydrogen isotope record of Pleistocene – Holocene climate transition from southern New England. Geophys. Res. Lett. 34, 1–5. doi: 10.1029/2007GL030303
Hubau, W., De Mil, T., Van den Bulcke, J., Phillips, O. L., Angoboy Ilondea, B., Van Acker, J., et al. (2019). The persistence of carbon in the African forest understory. Nat. Plants 5, 133–140. doi: 10.1038/s41477-018-0316-5
Iglesias, V., Haberle, S. G., Holz, A., and Whitlock, C. (2018). Holocene dynamics of temperate rainforests in west-central patagonia. Front. Ecol. Evol. 5:177. doi: 10.3389/fevo.2017.00177
IUCN (2016). A Global Standard for the Identification of Key Biodiversity Areas (No. Version 1.0). Gland: IUCN.
Jackson, S. T., and Hobbs, R. J. (2009). Ecological restoration in the light of ecological history. Science 325, 567–568. doi: 10.1126/science.1172977
Johnson, D. J., Needham, J., Xu, C., Massoud, E. C., Davies, S. J., Anderson-Teixeira, K. J., et al. (2018). Climate sensitive size-dependent survival in tropical trees. Nat. Ecol. Evol. 2, 1436–1442. doi: 10.1038/s41559-018-0626-z
Johnstone, J. F., and Chapin, F. S. (2006). Fire interval effects on successional trajectory in boreal forests of Northwest Canada. Ecosystems 9, 268–277. doi: 10.1007/s10021-005-0061-2
Karanth, K. K., and DeFries, R. (2010). Conservation and management in human-dominated landscapes: case studies from India. Conserv. Manage. Hum. Domin. Landsc. Case Stud. India 143, 2865–2869. doi: 10.1016/j.biocon.2010.05.002
Kneeshaw, D., Burton, P. J., De Grandpré, L., Gauthier, S., and Boulanger, Y. (2018). “Is management or conservation of old growth possible in North American boreal forests,” in Ecology and Recovery of Eastern Old-Growth Forests, eds A. M. Barton and W. S. Keeton (Washington, DC: Island Press/Center for Resource Economics), 139–157. doi: 10.5822/978-1-61091-891-6_8
Laurance, W. F., Camargo, J. L. C., Luizão, R. C. C., Laurance, S. G., Pimm, S. L., Bruna, E. M., et al. (2011). The fate of Amazonian forest fragments: a 32-year investigation. Biol. Conserv. 144, 56–67. doi: 10.1016/j.biocon.2010.09.021
Laurance, W. F., Lovejoy, T. E., Vasconcelos, H. L., Bruna, E. M., Didham, R. K., Stouffer, P. C., et al. (2002). Ecosystem decay of amazonian forest fragments: a 22-years investigation. Conserv. Biol. 16, 605–618. doi: 10.1046/j.1523-1739.2002.01025.x
Lehsten, V., de Groot, W., and Sallaba, F. (2016). Fuel fragmentation and fire size distributions in managed and unmanaged boreal forests in the province of Saskatchewan, Canada. For. Ecol. Manage 376, 148–157. doi: 10.1016/j.foreco.2016.06.014
Levesque, A., Cwynar, L., and Walker, I. (1997). Exceptionally steep north – south gradients in lake temperatures during the last deglaciation. Nature 385, 423–426. doi: 10.1038/385423a0
Lézine, A.-M., Izumi, K., Kageyama, M., and Achoundong, G. (2019). A 90,000-year record of Afromontane forest responses to climate change. Science 363, 177–181. doi: 10.1126/science.aav6821
Lim, H., Oren, R., Näsholm, T., Strömgren, M., Lundmark, T., Grip, H., et al. (2019). Boreal forest biomass accumulation is not increased by two decades of soil warming. Nat. Clim. Change 9, 49–52. doi: 10.1038/s41558-018-0373-9
Liu, J., Bowman, K. W., Schimel, D. S., Parazoo, N. C., Jiang, Z., Lee, M., et al. (2017). Contrasting carbon cycle responses of the tropical continents to the 2015–2016 El Niño. Science 358:eaam5690. doi: 10.1126/science.aam5690
Luo, Y., and Chen, H. Y. H. (2015). Climate change-associated tree mortality increases without decreasing water availability. Ecol. Lett. 18, 1207–1215. doi: 10.1111/ele.12500
Lynch, J. A., Clark, J. S., Bigelow, N. H., Edwards, M. E., and Finney, B. P. (2002). Geographic and temporal variations in fire history in boreal ecosystems of Alaska. J. Geophys. Res. Atmosph. 107, 8–1. doi: 10.1029/2001JD000332
Malhi, Y., Gardner, T. A., Goldsmith, G. R., Silman, M. R., and Zelazowski, P. (2014). Tropical forests in the Anthropocene. Annu. Rev. Environ. Resour. 39, 125–159. doi: 10.1146/annurev-environ-030713-155141
Maxwell, S., Evans, T., Watson, J. E. M., Morel, A. C., Grantham, H., Duncan, A., et al. (accepted). Degradation and forgone removals increase the carbon impact of intact forest loss by 626 percent. Sci. Adv.
McDowell, N., Allen, C. D., Anderson-Teixeira, K., Brando, P., Brienen, R., Chambers, J., et al. (2018). Drivers and mechanisms of tree mortality in moist tropical forests. New Phytol. 219, 851–869. doi: 10.1111/nph.15027
McDowell, N. G., and Allen, C. D. (2015). Darcy's law predicts widespread forest mortality under climate warming. Nat. Clim. Change 5, 669–672. doi: 10.1038/nclimate2641
McKenney, D. W., Pedlar, J. H., Lawrence, K., Campbell, K., and Hutchinson, M. F. (2007). Potential impacts of climate change on the distribution of North American Trees. Bio. Sci. 57, 939–948. doi: 10.1641/B571106
McMichael, C. H., Piperno, D. R., Bush, M. B., Silman, M. R., Zimmerman, A. R., Raczka, M. F., et al. (2012). Sparse pre-columbian human habitation in Western Amazonia. Science 336, 1429–1431. doi: 10.1126/science.1219982
Miettinen, J., Shi, C., and Liew, S. C. (2012). Two decades of destruction in Southeast Asia's peat swamp forests. Front. Ecol. Environ. 10, 124–128. doi: 10.1890/100236
Millar, C. I., and Stephenson, N. L. (2015). Temperate forest health in an era of emerging megadisturbance. Science 349, 823–826. doi: 10.1126/science.aaa9933
Mitchard, E. T. A. (2018). The tropical forest carbon cycle and climate change. Nature 559, 527–534. doi: 10.1038/s41586-018-0300-2
Mouillot, D., Bellwood, D. R., Baraloto, C., Chave, J., Galzin, R., Harmelin-Vivien, M., et al. (2013). Rare species support vulnerable functions in high-diversity ecosystems. PLoS Biol. 11:e1001569. doi: 10.1371/journal.pbio.1001569
Murray, D. L., Peers, M. J. L., Majchrzak, Y. N., Wehtje, M., Ferreira, C., Pickles, R. S. A., et al. (2017). Continental divide: predicting climate-mediated fragmentation and biodiversity loss in the boreal forest. PLoS ONE 12:e0176706. doi: 10.1371/journal.pone.0176706
Nash, K. L., Allen, C. R., Angeler, D. G., Barichievy, C., Eason, T., Garmestani, A. S., et al. (2014). Discontinuities, cross-scale patterns, and the organization of ecosystems. Ecology 95, 654–667. doi: 10.1890/13-1315.1
Newton, A. C. (2016). Biodiversity risks of adopting resilience as a policy goal. Conserv. Lett. 9, 369–376. doi: 10.1111/conl.12227
Nogué, S., de Nascimento, L., Froyd, C. A., Wilmshurst, J. M., de Boer, E. J., Coffey, E. E. D., et al. (2017). Island biodiversity conservation needs palaeoecology. Nat. Ecol. Amp. Evol. 1:0181. doi: 10.1038/s41559-017-0181
Nogué, S., Rull, V., Montoya, E., Huber, O., and Vegas-Vilarrúbia, T. (2009). Paleoecology of the guayana highlands (northern South America): holocene pollen record from the Eruoda-tepui, in the Chimantá massif. Palaeogeogr. Palaeoclimatol. Palaeoecol. 281, 165–173. doi: 10.1016/j.palaeo.2009.07.019
Nogué, S., Tovar, C., Bhagwat, S. A., Finsinger, W., and Willis, K. J. (2018). Exploring the ecological history of a tropical agroforestry landscape using fossil pollen and charcoal analysis from four sites in Western Ghats, India. Ecosystems 21, 45–55. doi: 10.1007/s10021-017-0132-1
O‘Neill, R. V. (1998). Recovery in complex ecosystems. J. Aquat. Ecosyst. Stress Recov. 6, 181–187. doi: 10.1023/A:1009996332614
Page, S. E., Rieley, J. O., and Banks, C. J. (2011). Global and regional importance of the tropical peatland carbon pool. Glob. Change Biol. 17, 798–818. doi: 10.1111/j.1365-2486.2010.02279.x
Parrish, J. D., Braun, D. P., and Unnasch, R. S. (2003). Are we conserving what we say we are? Measuring ecological integrity within protected areas. BioScience 53, 851–860. doi: 10.1641/0006-3568(2003)053[0851:AWCWWS]2.0.CO;2
Phillips, O. L., van der Heijden, G., Lewis, S. L., López-González, G., Aragão, L. E. O. C., Lloyd, J., et al. (2010). Drought–mortality relationships for tropical forests. New Phytol. 187, 631–646. doi: 10.1111/j.1469-8137.2010.03359.x
Ploughe, L. W., Jacobs, E. M., Frank, G. S., Greenler, S. M., Smith, M. D., and Dukes, J. S. (2019). Community Response to Extreme Drought (CRED): a framework for drought-induced shifts in plant–plant interactions. New Phytol. 222, 52–69. doi: 10.1111/nph.15595
Ponce-Campos, G. E., Moran, M. S., Huete, A., Zhang, Y., Bresloff, C., Huxman, T. E., et al. (2013). Ecosystem resilience despite large-scale altered hydroclimatic conditions. Nature 494, 349–352. doi: 10.1038/nature11836
Potapov, P., Hansen, M. C., Laestadius, L., Turubanova, S., Yaroshenko, A., Thies, C., et al. (2017). The last frontiers of wilderness: tracking loss of intact forest landscapes from 2000 to 2013. Sci. Adv. 3:e1600821. doi: 10.1126/sciadv.1600821
Putz, F. E., and Romero, C. (2014). Futures of tropical forests (sensu lato). Biotropica 46, 495–505. doi: 10.1111/btp.12124
Qie, L., Lewis, S. L., Sullivan, M. J. P., Lopez-Gonzalez, G., Pickavance, G. C., Sunderland, T., et al. (2017). Long-term carbon sink in Borneo's forests halted by drought and vulnerable to edge effects. Nat. Commun. 8:1966. doi: 10.1038/s41467-017-01997-0
Rull, V. (2005). Vegetation and environmental constancy in the Neotropical Guayana Highlands during the last 6000 years? Rev. Palaeobot. Palynol. 135, 205–222. doi: 10.1016/j.revpalbo.2005.03.008
Sakschewski, B., von Bloh, W., Boit, A., Poorter, L., Peña-Claros, M., Heinke, J., et al. (2016). Resilience of Amazon forests emerges from plant trait diversity. Nat. Clim. Change 6, 1032–1036. doi: 10.1038/nclimate3109
Sangüesa-Barreda, G., Camarero, J. J., Oliva, J., Montes, F., and Gazol, A. (2015). Past logging, drought and pathogens interact and contribute to forest dieback. Agric. For. Meteorol. 208, 85–94. doi: 10.1016/j.agrformet.2015.04.011
Seddon, A. W., Macias-Fauria, M., Long, P. R., Benz, D., and Willis, K. J. (2016). Sensitivity of global terrestrial ecosystems to climate variability. Nature 531, 229–232. doi: 10.1038/nature16986
Seddon, A. W. R. (2017). What do we mean by regime shift? Distinguishing between extrinsic and intrinsic forcing in paleoecological data. Past Glob. Chang. Mag. 25, 94–95. doi: 10.22498/pages.25.2.94
Seddon, A. W. R., Froyd, C. A., Witkowski, A., and Willis, K. J. (2014). A quantitative framework for analysis of regime shifts in a Galápagos coastal lagoon. Ecology 95, 3046–3055. doi: 10.1890/13-1974.1
Seidl, R., Spies, T. A., Peterson, D. L., Stephens, S. L., and Hicke, J. A. (2016). REVIEW: searching for resilience: addressing the impacts of changing disturbance regimes on forest ecosystem services. J. Appl. Ecol. 53, 120–129. doi: 10.1111/1365-2664.12511
Shuman, B., Bartlein, P. J., Logar, N., Newby, P., and Webb, T. III. (2002a). Parallel climate and vegetation responses to the early Holocene collapse of the Laurentide Ice Sheet. Quat. Sci. Rev. 21, 1793–1805. doi: 10.1016/S0277-3791(02)00025-2
Shuman, B., Webb, T. III., Bartlein, P., and Williams, J. (2002b). The anatomy of a climatic oscillation: vegetation change in eastern North America during the Younger Dryas chronozone. Quat. Sci. Rev. 21, 1777–1791. doi: 10.1016/S0277-3791(02)00030-6
Solano, R., Didan, K., Jacobson, A., and Huete, A. (2010). MODIS Vegetation Index C5 User's Guide. Tucson, AZ: University of Arizona.
Steffen, W., Rockström, J., Richardson, K., Lenton, T. M., Folke, C., Liverman, D., et al. (2018). Trajectories of the earth system in the anthropocene. Proc. Natl. Acad. Sci. U.S.A. 115, 8252–8259. doi: 10.1073/pnas.1810141115
Stuart-Smith, R. D., Edgar, G. J., Barrett, N. S., Kininmonth, S. J., and Bates, A. E. (2015). Thermal biases and vulnerability to warming in the world's marine fauna. Nature 528, 88–92. doi: 10.1038/nature16144
Suarez, M. L., Ghermandi, L., and Kitzberger, T. (2004). Factors predisposing episodic drought-induced tree mortality in Nothofagus– site, climatic sensitivity and growth trends. J. Ecol. 92, 954–966. doi: 10.1111/j.1365-2745.2004.00941.x
Taubert, F., Fischer, R., Groeneveld, J., Lehmann, S., Müller, M. S., Rödig, E., et al. (2018). Global patterns of tropical forest fragmentation. Nature 554, 519–522. doi: 10.1038/nature25508
Thompson, I., Mackey, B., McNulty, S., and Mosseler, A. (2009). Forest Resilience, Biodiversity, and Climate Change (No. Technical Series No. 43). Montreal, QC: Secretariat of the Convention on Biological Diversity.
Tilman, D., Isbell, F., and Cowles, J. M. (2014). Biodiversity and ecosystem functioning. Annu. Rev. Ecol. Evol. Syst. 45, 471–493. doi: 10.1146/annurev-ecolsys-120213-091917
Tovar, C., Breman, E., Brncic, T., Harris, D. J., Bailey, R., and Willis, K. J. (2014). Influence of 1,100 years of burning on the central African rainforest. Ecography 37, 1139–1148. doi: 10.1111/ecog.00697
Trugman, A. T., Detto, M., Bartlett, M. K., Medvigy, D., Anderegg, W. R. L., Schwalm, C., et al. (2018). Tree carbon allocation explains forest drought-kill and recovery patterns. Ecol. Lett. 21, 1552–1560. doi: 10.1111/ele.13136
Urrego, D. H., Bush, M. B., Silman, M. R., Niccum, B. A., De La Rosa, P., McMichael, C. H., et al. (2013). Holocene fires, forest stability and human occupation in south-western Amazonia. J. Biogeogr. 40, 521–533. doi: 10.1111/jbi.12016
van der Sande, M. T., Gosling, W., Correa-Metrio, A., Prado-Junior, J., Poorter, L., Oliveira, R. S., et al. (2019). A 7,000-years history of changing plant trait composition in an Amazonian landscape; the role of humans and climate. Ecol. Lett. 22, 925–935. doi: 10.1111/ele.13251
van der Sleen, P., Groenendijk, P., Vlam, M., Anten, N. P. R., Boom, A., Bongers, F., et al. (2015). No growth stimulation of tropical trees by 150 years of CO2 fertilization but water-use efficiency increased. Nat. Geosci. 8, 24–28. doi: 10.1038/ngeo2313
van Mantgem, P. J., Stephenson, N. L., Byrne, J. C., Daniels, L. D., Franklin, J. F., Fulé, P. Z., et al. (2009). Widespread increase of tree mortality rates in the western United States. Science 323, 521–524. doi: 10.1126/science.1165000
Veraart, A. J., Faassen, E. J., Dakos, V., van Nes, E. H., Lürling, M., and Scheffer, M. (2011). Recovery rates reflect distance to a tipping point in a living system. Nature 481, 357–359. doi: 10.1038/nature10723
Violle, C., Navas, M.-L., Vile, D., Kazakou, E., Fortunel, C., Hummel, I., et al. (2007). Let the concept of trait be functional! Oikos 116, 882–892. doi: 10.1111/j.2007.0030-1299.15559.x
Virah-Sawmy, M., Bonsall, M. B., and Willis, K. J. (2009). ‘Tales of Symphonia': extinction dynamics in response to past climate change in Madagascan rainforests. Biol. Lett. 5, 821–825. doi: 10.1098/rsbl.2009.0428
Watson, J. E. M., Evans, T., Venter, O., Williams, B., Tulloch, A., Stewart, C., et al. (2018). The exceptional value of intact forest ecosystems. Nat. Ecol. Evol. 2, 599–610. doi: 10.1038/s41559-018-0490-x
Williams, J. W., Blois, J. L., and Shuman, B. N. (2011). Extrinsic and intrinsic forcing of abrupt ecological change: case studies from the late Quaternary. J. Ecol. 99, 664–677. doi: 10.1111/j.1365-2745.2011.01810.x
Willis, K. J., Bailey, R. M., Bhagwat, S. A., and Birks, H. J. (2010). Biodiversity baselines, thresholds and resilience: testing predictions and assumptions using paleoecological data. Spec. Issue Long-Term Ecol. Res. 25, 583–591. doi: 10.1016/j.tree.2010.07.006
Willis, K. J., and Birks, H. J. B. (2006). What is natural? The need for a long-term perspective in biodiversity conservation. Science 314, 1261–1265. doi: 10.1126/science.1122667
Willis, K. J., Gillson, L., Brncic, T. M., and Figueroa-Rangel, B. L. (2005). Providing baselines for biodiversity measurement. Trends Ecol. Evol. 20, 107–108. doi: 10.1016/j.tree.2004.12.003
Willis, K. J., Jeffers, E. S., and Tovar, C. (2018). What makes a terrestrial ecosystem resilient? Science 359, 988–989. doi: 10.1126/science.aar5439
Withey, K., Berenguer, E., Palmeira Alessandro, F., Espírito-Santo Fernando, D. B., Lennox Gareth, D., Silva Camila, V. J., et al. (2018). Quantifying immediate carbon emissions from El Niño-mediated wildfires in humid tropical forests. Philos. Trans. R. Soc. B Biol. Sci. 373, 20170312. doi: 10.1098/rstb.2017.0312
Wolken, J. M., Hollingsworth, T. N., Rupp, T. S., Chapin, F. S. III., Trainor, S. F., Barrett, T. M., et al. (2011). Evidence and implications of recent and projected climate change in Alaska's forest ecosystems. Ecosphere 2:art124. doi: 10.1890/ES11-00288.1
Wong, C. M., and Daniels, L. D. (2017). Novel forest decline triggered by multiple interactions among climate, an introduced pathogen and bark beetles. Glob. Change Biol. 23, 1926–1941. doi: 10.1111/gcb.13554
Keywords: intact forest landscapes, resilience, tropical forest, boreal forest, paleoecology, climate sensitivity
Citation: Morel AC and Nogué S (2019) Combining Contemporary and Paleoecological Perspectives for Estimating Forest Resilience. Front. For. Glob. Change 2:57. doi: 10.3389/ffgc.2019.00057
Received: 26 March 2019; Accepted: 05 September 2019;
Published: 26 September 2019.
Edited by:
Lucas Cernusak, James Cook University, AustraliaReviewed by:
Bradley Christoffersen, University of Texas Rio Grande Valley Edinburg, United StatesErvan Rutishauser, Smithsonian Tropical Research Institute (SI), United States
Copyright © 2019 Morel and Nogué. This is an open-access article distributed under the terms of the Creative Commons Attribution License (CC BY). The use, distribution or reproduction in other forums is permitted, provided the original author(s) and the copyright owner(s) are credited and that the original publication in this journal is cited, in accordance with accepted academic practice. No use, distribution or reproduction is permitted which does not comply with these terms.
*Correspondence: Alexandra C. Morel, YWxleGFuZHJhLm1vcmVsQGdtYWlsLmNvbQ==