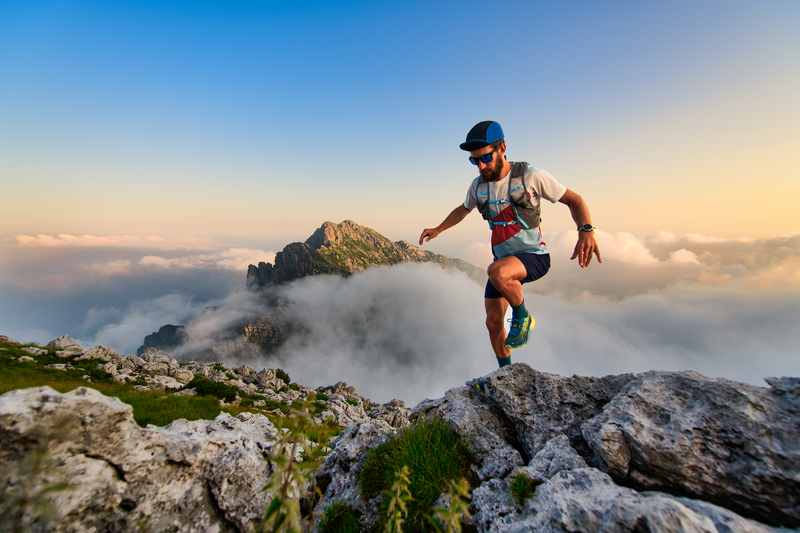
95% of researchers rate our articles as excellent or good
Learn more about the work of our research integrity team to safeguard the quality of each article we publish.
Find out more
MINI REVIEW article
Front. For. Glob. Change , 20 August 2019
Sec. Forests and the Atmosphere
Volume 2 - 2019 | https://doi.org/10.3389/ffgc.2019.00045
This article is part of the Research Topic Interactions between Ozone Pollution and Forest Ecosystems View all 9 articles
The correct integration of the detoxification processes in a risk assessment model for ozone damage on plants remains challenging. In particular, the intracellular compartmentation of antioxidant metabolites could play a role, since each compartment presents its own sensitivity to ROS and metabolite set. For each compartment, we tried to provide both qualitative and quantitative information on the metabolites present as well as the putative transporters implied. When they are known, the modifications caused by O3 or oxidative stress are presented. Clearly, under O3 exposure, integrative data which would allow to improve predictive models for O3 risk assessment are missing.
Ozone (O3) is a phytotoxic air pollutant known to negatively impact crop and forest productivity (Wittig et al., 2009; Jolivet et al., 2016; Li et al., 2017). In order to determine the critical level above which O3 damage on plants occurs, the PODY (Phytotoxic Ozone Dose above a threshold flux of Y nmol.m−2.s−1) was derived from flux-based methods to improve dose-response relationships including the detoxifying capacity of leaf tissues (Musselman et al., 2006; Dizengremel et al., 2009). Flux-based models use a range of cut-off thresholds (Y) indicative of varying detoxification capacities. However, the considerable uncertainties to determine the contribution of each metabolite to the cellular antioxidant potential make the estimation of the threshold difficult to assess. This difficulty can be explained by the differences in concentrations and redox status of these metabolites between compartments. Here, we resumed recent highlights on the spatial distribution of the main defense metabolites to decipher their possible roles in response to O3 and point out which importance the occurrence of this compartmentation might have in estimating the detoxification threshold.
Different classes of molecules serve as antioxidants amongst which ascorbate (AsA), glutathione (GSH), and phenolic compounds as flavonols are considered as the most ubiquitous according to their presence in several cell compartments (Foyer and Noctor, 2011; Figure 1), including vacuole and the extracellular space (Zhao and Dixon, 2009; Agati et al., 2012). Sugars such as sucrose, RFOs (raffinose family oligosaccharides) and fructans are known to directly quench ROS in different organelles and thus contribute to antioxidant defense (Keunen et al., 2013). Another type are liphophilic antioxidants, such as α-tocopherol or carotenoids located in organelle membranes particularly in plastids (Das and Roychoudhury, 2014). Subcellular compartments also possess various enzymes able either to act as ROS scavengers or to support regeneration of the reduced form of the antioxidants (Noctor et al., 2018). The first group includes enzymes trapping the superoxide ion (such as superoxide dismutase, SODs) and hydrogen peroxide with catalases (specifically located in the peroxisome) or several peroxidases (AsA, GSH, or thioredoxin dependent) whose cell location has already been detailed in several reviews (Rouhier and Jacquot, 2005; Dos Santos and Rey, 2006; Mhamdi et al., 2010b; Rahantaniaina et al., 2013; Noctor et al., 2018). The second group, responsible for the regeneration of antioxidants, is ascribed to the close redox coupling of AsA and GSH pools in vivo (HAF as Halliwell Asada Foyer cycle). The presence of dehydroascorbate reductase (DHAR) seems to be sufficient to consider the presence and the functioning of the HAF cycle within a specific cell compartment (Figure 1; Rahantaniaina et al., 2013). It is also important to consider that the HAF cycle functioning needs to be linked with a correct enzyme regeneration through NAD(P)H reducing power, e.g., under O3 stress (Dizengremel et al., 2008; Dghim et al., 2013), in cytosol as well as in organelles. In fine, detoxification results from the combined actions of all these mechanisms, which therefore explains the difficulty to choose key parameter(s) in modeling the detoxifying capacity of leaf tissues.
Figure 1. Compartmentation of plant oxidative system. This non-exhaustive figure summarizes current knowledge from plant subcellular localization of antioxidants in non-stress conditions. In view of the multiplicity of all enzyme isoforms, the figure only mentions the presence of the HAF cycle in each compartment where the dehydroascorbate reductase (DHAR) has been characterized. AsA, total ascorbate; GSH, total glutathione; GSSG, glutathione disulphide; GS-conj, glutathione conjugates; HAF, Halliwell-Asada-Foyer; MRP, Multidrug Resistance associated Protein; CRT, Chloroquine Resistance Transporter; PHT, phosphate transporter; POX, phenol peroxidases; ROS, reactive oxygen species. See the text for references.
One major issue when considering the total pool of antioxidants is the difficulty to evaluate it as a whole, taking into account the diversity of the metabolites it contains. Indeed, the methods and kits used are disputable (Noctor et al., 2016). For example, in order to take into account the total antioxidant capacity in plants, some authors used the FRAP assay (ferric reducing ability of plasma given in micromoles of Fe2+ per gram in dry matter). However, the significance of the FRAP test is questionable since it takes antioxidants into consideration that do not necessarily react to the oxidative load generated by O3 (Severino et al., 2007). To solve this issue, researches focused on specific metabolites considered as cue to explain differences in O3 tolerance. AsA is one of the main examples of a potential factor in O3 tolerance (Burkey et al., 2000; Conklin and Barth, 2004) at least for some species. To support this fact, studies with AsA deficient mutants and clones (Conklin et al., 1996, 2000; Veljovic-Jovanovic et al., 2002) showed that low concentrations of AsA in the plant tissue limit O3 tolerance. However, in numerous examples and particularly in ligneous species (e.g., Populus genotypes or Quercus sp.), differences in AsA content were not sufficient to explain the differences in O3 tolerance (Dusart et al., 2019; Pellegrini et al., 2019). Other antioxidants such as GSH and/or phenolic compounds could play a more important role in some species or genotypes. In addition, changes at the leaf scale could hide more subtle changes at the scale of a cell compartment that is crucial for cell homeostasis. The redox state of each metabolite also varies, as some compartments differ from others with lower reduction states. Thus, the apoplast and vacuole were endowed with a lower level of reduction for AsA and GSH (Noctor and Foyer, 2016). In this respect, both the antioxidant content and the ability of cell compartments to regulate the redox levels of the molecules have to be considered.
The different cell compartments are not equal in terms of antioxidant concentrations (Zechmann, 2017) and redox state (Foyer and Noctor, 2016) and the main differences between cell compartments are discussed in this section. We also highlight some specific features related to the transport of these metabolites through intracellular membranes, which also contributes to the extent of antioxidant content and redox status in each compartment.
The apoplast comprises the cell wall and the fluid in the intercellular spaces as such constitutes the first barrier encountered by O3 after entering the leaf through the stomata (Laisk et al., 1989). AsA contained in the apoplastic fluid was often considered as the first line of defense against O3 and, consequently, its content may increase with the beginning of the exposure (Riikonen et al., 2009). In this context, it has been integrated in different models (Polle et al., 1995; Ranieri et al., 1996; Plöchl et al., 2000; Burkey and Eason, 2002; Conklin and Barth, 2004; Tuzet et al., 2011). In fact, in many species, the importance of apoplastic AsA regarding O3 tolerance is still unclear (D'Haese et al., 2005; Booker et al., 2012; Dai et al., 2018). For example investigated poplar clones showed no relation between apoplastic AsA content and O3 sensitivity of clones (Van Hove et al., 2001; Di Baccio et al., 2008). Moreover, the efficiency of apoplast detoxification by AsA depends on the export of the oxidized form to the cytoplasm where it then has to be regenerated (Luwe and Heber, 1995). Finally, despite an efficient transmembrane exchange between apoplast and symplasm, the AsA concentrations (around 0.2–1.5 mM) in the apoplast are 10–30 times lower than in the cytosol (Moldau et al., 1997; Plöchl et al., 2000; Van Hove et al., 2001) with higher oxidation rates (Booker et al., 2012). The transport proteins responsible for DHA uptake and ascorbate efflux have not yet been identified, even though the ascorbate efflux mechanism possibly occurs via an anion channel (Smirnoff, 2018). It should also be considered that the presence of ascorbate oxidase in the apoplast could maintain the AsA pool in a more oxidized state than the intracellular pool (Smirnoff, 2018).
There are furthermore other metabolites with antioxidant activity being present in the apoplast. Considering GSH, its content in the apoplast has been found to be very low (Zechmann, 2014), limiting its ability to scavenge ROS or contribute to ASA regeneration. Apoplastic GSH is therefore expected to be rather involved in signaling than in detoxification (Zechmann et al., 2008). Phenolic compounds (Grace, 2007) may be widespread in the apoplastic fluid of plants and in some cases were shown to increase in response to O3 (Langebartels et al., 1991; Eckey-Kaltenbach et al., 1993; Booker et al., 2012). However this increase was considered to have little effectiveness in scavenging O3 in Arabidopsis (Booker et al., 2012). Cell wall bound phenolics may also scavenge ROS (Vreeburg and Fry, 2007) but their contribution to O3 detoxification is yet unknown. In the cell wall of foliar and stem cells, O3 exposure resulted in an increase in lignin biosynthesis in a dose-dependent manner suggesting a role in detoxification (Cabane et al., 2012). The contribution of phenolics and more widely secondary metabolites in apoplastic O3 detoxification has not been sufficiently studied so far and should be further investigated especially because of their species-specific diversity.
Cytosol is an important determinant in the antioxidant capacity of the cell, acting as a hub for the production/transportation to other cell compartments and between compartments (Zechmann, 2017). Using labeling techniques (Zechmann, 2011), showed that the highest concentration of AsA was found in this compartment (Figure 1). Moreover, isoenzymes related to HAF cycle localized in the cytosol seem to play an important role in O3 tolerance (Yoshida et al., 2006; Di Baccio et al., 2008; Mhamdi et al., 2010a; Rahantaniaina et al., 2017; Dusart et al., 2019). A good example of the cytosol interface is the GSH biosynthesis taking place both in cytosol and chloroplast (Rausch et al., 2007). Interestingly, although chloroplasts synthesize GSH, this metabolite is also readily taken up by intact chloroplasts (Foyer et al., 2001). For the other compartments, unable to carry out GSH biosynthesis, transport from cytosol must also be efficient (Rausch et al., 2007). While these carriers are far from being characterized, their regulation under oxidative stress is fully unknown.
In vacuole, the AsA concentration is often considered not to exceed 2 mM, making it the lowest within the plant cells (Figure 1; Zechmann, 2017). However, its level was 2- and 4-times increased during drought and high light stress, respectively, which represents the strongest increase among all subcellular compartments (Rautenkranz et al., 1994; Zechmann, 2017). Changes in AsA concentration under O3 are not known. This raises the question whether vacuolar AsA could significantly contribute to cell defense against oxidative stress. When facing stress conditions, a large amount of H2O2 is transported and accumulated in the vacuole, which might act as a sink for ROS (Michalak, 2006; Koffler et al., 2014). It is expected that the increase in vacuolar AsA contributes to delocalize the detoxification of H2O2 and thus to avoid cytosol redox imbalance. AsA seems to contribute to ROS scavenging in the vacuole, either directly or by coupling with phenolics and phenol oxidase. In fact, vacuoles are also well-known to hold large amounts of polyphenols, especially anthocyanins, flavan-3-ol monomers, proanthocyanidins, and glycosylated flavonols. Vacuolar flavonols have been suggested to contribute to H2O2 detoxification in this compartment, by giving electrons to phenol peroxidases (POX) (Sakihama et al., 2002). Furthermore, it has been shown that vacuolar AsA can reduce phenoxy radicals produced by POX and then regenerate phenolic compounds (Takahama and Oniki, 1997). Therefore, an alternative AsA/phenolics/POX mechanism might efficiently contribute to the cellular defense arsenal against ROS, jointly to the “classic” HAF cycle. On this point, none or very little free GSH (lower than 0.5 mM) has ever been identified in the vacuole, limiting the potential regeneration of ascorbate by this way in this compartment (Zechmann et al., 2008; Zechmann, 2014). In addition, DHAR was reported in the vacuole (Zhang et al., 2015) but HAF cycle does not seem to be functioning due to the lack of glutathione reductase isoform in this compartment, therefore leading to glutathione disulphide (GSSG) accumulation (Queval et al., 2011). In any case, oxidized AsA (DHA and monodehydroascorbate) can cross the tonoplast to be regenerated in the cytoplasm (Rautenkranz et al., 1994). In case of oxidative stress, vacuoles also act as a sink for oxidized GSH or GSH conjugates formed in the cytosol and transferred to the vacuole via the action of one or more ABC transporters of the MRP (Multidrug Resistance associated Protein) subclass (Queval et al., 2011; Koffler et al., 2014). Despite extensive investigation of tonoplast transporters, the role of MRPs in the transport of GSSG to the vacuole is less clear (Bachhawat et al., 2013) as well as the final fate of this oxidized form in the vacuole.
Within the chloroplast, the electron transport chain is, in addition to its role as major energy producer, one of the main sites of endogenous ROS generation (Asada, 2006; Tripathy and Oelmüller, 2012). Being triggered and increased by apoplastic ROS, the chloroplastic ROS function as amplifiers of signals from outer cell compartments to the nucleus where they modify the nuclear gene expression (Shapiguzov et al., 2012; Foyer and Noctor, 2016; Kleine and Leister, 2016). The presence of AsA (10 mM) and GSH (1 mM) has been previously reported in the chloroplast where they represent a significant part of the cell antioxidant pool (Figure 1; Queval et al., 2011; Zechmann, 2011). Interestingly, abiotic stresses lead to a major increase in AsA and GSH contents in the chloroplast (Heyneke et al., 2013). Furthermore, although lacking under normal conditions, both antioxidants also accumulated inside the thylakoid lumen under stress, thus, demonstrating the particular dependence of the chloroplast on these antioxidants when facing harmful abiotic stress (Heyneke et al., 2013). Concerning AsA and GSH transport, chloroplast and cytosol are tightly connected by the presence of many transporters. AsA uptake by chloroplasts is mediated by a member of a phosphate transporter family, named PHT4;4 (Fernie and Tóth, 2015; Miyaji et al., 2015). An active uptake of cytosolic GSH also occurred across the chloroplast envelope (Noctor et al., 2002), even though the molecular identity of the transporter(s) is still unknown (Bachhawat et al., 2013). In addition, three proteins belonging to the CRT (Chloroquine Resistance Transporter)-like transporter family were found to be chloroplastic and responsible for glutathione efflux from the chloroplast to the cytosol in Arabidopsis (Bachhawat et al., 2013).
At the same time, choroplasts contain large amounts of flavonoid-like ROS defense agents, which they are able to biosynthesize (Hernández et al., 2009; Pollastri and Tattini, 2011). Under severe light, flavonoids complete the scavenging role of the most abundant lipid-soluble antioxidants group(s), the carotenoids (and tocopherols), in chloroplasts (DellaPenna and Pogson, 2006). Due to their ability to remodel lipid membranes, flavonoids might preserve the integrity of the chloroplast envelope and therefore prevent oxidative stress-caused damage (Agati et al., 2012).
An endogenous production of ROS takes place in mitochondria essentially at the level of the complexes I, II, and III of the respiratory chain which must not be neglected even though the contribution of this organelle to oxidative stress is rather low (Apel and Hirt, 2004; Rhoads et al., 2006; Bettini et al., 2008; Waszczak et al., 2018). Mitochondria are well-supplied with antioxidants and the enzymes of the HAF cycle are present (Jiménez et al., 1997; Foyer et al., 2001; Foyer and Noctor, 2011). Similar as chloroplast, a concentration of 10 mM AsA was determined in mitochondria (Zechmann, 2011; Zechmann et al., 2011). Considering that the last step of ascorbate biosynthesis can take place in the intermembrane space in contact with complex 1 (Millar et al., 2003), it is now seen that DHA is transported in the matrix to subsequently be reduced by the HAF cycle (Navrot et al., 2007). In this context, a high concentration of GSH (between 7 and 15 mM) has been determined in this organelle (Zechmann et al., 2008; Queval et al., 2011; Zechmann, 2014), despite it is considered to be devoid of GSH synthesis pathway. This implies an uptake of GSH from the cytosol assumed by different transporters (Chen and Lash, 1998). In addition, there is no work mentioning the accumulation of phenols in the mitochondria, these compounds been known as inhibitors of the respiratory activity (Demos et al., 1975). Finally, in spite of a quite large panel of antioxidants, the mitochondria have been pointed out as being more sensitive to O3 than chloroplasts (Pellinen et al., 1999). Nevertheless, there is no consensus to validate this difference of sensitivity between organelles (Sutinen et al., 1990).
Due to H2O2 production driven by photorespiration, the peroxisome is also provided with antioxidant systems (Corpas et al., 2019). To minimize H2O2 accumulation in this compartment, a significant catalase activity has been observed. In addition, the presence of DHAR isoform suggests a functioning HAF cycle (Jiménez et al., 1997) in the peroxisome. A high concentration of AsA (23 mM) was determined in the peroxisome (Zechmann, 2017) while GSH was also detected but at low level (4 mM) (Zechmann et al., 2008). O3 increased catalase activity and enhanced the number of peroxisome in tolerant birch leaves (Oksanen et al., 2004). The increase of peroxisome number could be a response to an enhanced requirement for detoxification as photorespiration decreased (Booker et al., 1997; Bagard et al., 2008).
This article points out the great diversity of antioxidative systems, scattered in the different cellular compartments of leaves. The data so far published suggest that this diversity must be taken into account in O3 risk assessment. However, under O3, there is a lack of information regarding changes in the concentrations of the different antioxidants in each compartment under ozone treatment. As mentioned in previous works, and considering its occurrence in different cell compartments, it is obvious that the HAF cycle has a prominent role in cell detoxification. In addition, phenolic compounds in cell wall, vacuole and chloroplasts might also play a protective role. Subcellular immunocytochemical localization could allow a more precise identification of the respective contribution of each compartment to the global defense system. The next step would be to get an integrative scheme allowing to improve the modeling for the participation of detoxification to risk assessment. Recently, an attempt to integrate the vacuole in an H2O2 metabolism under oxidative constraint model appeared to be promising, especially since transporters were considered (Tuzet et al., 2019). Indeed, the transport of antioxidants between compartments during oxidative stress should be also studied to better understand the role of compartmentation.
All authors listed have made a substantial, direct and intellectual contribution to the work, and approved it for publication.
This laboratory was supported by the French National Research Agency through the Laboratory of Excellence ARBRE (ANR-12-LABXARBRE-01).
The authors declare that the research was conducted in the absence of any commercial or financial relationships that could be construed as a potential conflict of interest.
Agati, G., Azzarello, E., Pollastri, S., and Tattini, M. (2012). Flavonoids as antioxidants in plants: location and functional significance. Plant Sci. 196, 67–76. doi: 10.1016/j.plantsci.2012.07.014
Apel, K., and Hirt, H. (2004). Reactive oxygen species: metabolism, oxidative stress, and signal transduction. Annu. Rev. Plant Biol. 55, 373–399. doi: 10.1146/annurev.arplant.55.031903.141701
Asada, K. (2006). Production and scavenging of reactive oxygen species in chloroplasts and their functions. Plant Physiol. 141, 391–396. doi: 10.1104/pp.106.082040
Bachhawat, A. K., Thakur, A., Kaur, J., and Zulkifli, M. (2013). Glutathione transporters. Biochim. Biophys. Acta BBA-Gen. Subj. 1830, 3154–3164. doi: 10.1016/j.bbagen.2012.11.018
Bagard, M., Le Thiec, D., Delacote, E., Hasenfratz-Sauder, M.-P., Banvoy, J., Gérard, J., et al. (2008). Ozone-induced changes in photosynthesis and photorespiration of hybrid poplar in relation to the developmental stage of the leaves. Physiol. Plant. 134, 559–574. doi: 10.1111/j.1399-3054.2008.01160.x
Bettini, P., Cosi, E., Bindi, D., and Buiatti, M. (2008). Reactive oxygen species metabolism in plants: production, detoxification and signaling in stress response. Plant Stress 2, 28–39.
Booker, F. L., Burkey, K. O., and Jones, A. M. (2012). Re-evaluating the role of ascorbic acid and phenolic glycosides in ozone scavenging in the leaf apoplast of Arabidopsis thaliana L. Plant Cell Environ. 35, 1456–1466. doi: 10.1111/j.1365-3040.2012.02502.x
Booker, F. L., Reid, C. D., Brunschon-Harti, S., Fiscus, E. L., and Miller, J. E. (1997). Photosynthesis and photorespiration in soybean [Glycine max (L.) Merr.] chronically exposed to elevated carbon dioxide and ozone. J. Exp. Bot. 48, 1843–1852.
Burkey, K. O., and Eason, G. (2002). Ozone tolerance in snap bean is associated with elevated ascorbic acid in the leaf apoplast. Physiol. Plant. 114, 387–394. doi: 10.1034/j.1399-3054.2002.1140308.x
Burkey, K. O., Wei, C., Eason, G., Ghosh, P., and Fenner, G. P. (2000). Antioxidant metabolite levels in ozone-sensitive and tolerant genotypes of snap bean. Physiol. Plant. 110, 195–200. doi: 10.1034/j.1399-3054.2000.110208.x
Cabane, M., Afif, D., and Hawkins, S. (2012). “Chapter 7–lignins and abiotic stresses,” in Advances in Botanical Research Lignins, Vol. 61, eds L. Jouanin and C. Lapierre (Burlington: Academic Press), 219–262.
Chen, Z., and Lash, L. H. (1998). Evidence for mitochondrial uptake of glutathione by dicarboxylate and 2-oxoglutarate carriers. J. Pharmacol. Exp. Ther. 285, 608–618.
Conklin, P. L., and Barth, C. (2004). Ascorbic acid, a familiar small molecule intertwined in the response of plants to ozone, pathogens, and the onset of senescence. Plant Cell Environ. 27, 959–970. doi: 10.1111/j.1365-3040.2004.01203.x
Conklin, P. L., Saracco, S. A., Norris, S. R., and Last, R. L. (2000). Identification of ascorbic acid-deficient Arabidopsis thaliana mutants. Genetics 154, 847–856.
Conklin, P. L., Williams, E. H., and Last, R. L. (1996). Environmental stress sensitivity of an ascorbic acid-deficient Arabidopsis mutant. Proc. Natl. Acad. Sci. U.S.A. 93, 9970–9974. doi: 10.1073/pnas.93.18.9970
Corpas, F. J., del Río, L. A., and Palma, J. M. (2019). Impact of nitric oxide (NO) on the ROS metabolism of peroxisomes. Plants 8:37. doi: 10.3390/plants8020037
Dai, L., Feng, Z., Pan, X., Xu, Y., Li, P., Lefohn, A. S., et al. (2018). Increase of apoplastic ascorbate induced by ozone is insufficient to remove the negative effects in tobacco, soybean and poplar. Environ. Pollut. 245, 380–388. doi: 10.1016/j.envpol.2018.11.030
Das, K., and Roychoudhury, A. (2014). Reactive oxygen species (ROS) and response of antioxidants as ROS-scavengers during environmental stress in plants. Front. Environ. Sci. 2:53. doi: 10.3389/fenvs.2014.00053
DellaPenna, D., and Pogson, B. J. (2006). Vitamin synthesis in plants: tocopherols and carotenoids. Annu. Rev. Plant Biol. 57, 711–738. doi: 10.1146/annurev.arplant.56.032604.144301
Demos, E. K., Woolwine, M., Wilson, R. H., and McMillan, C. (1975). The effects of ten phenolics compoundds on hypocotyl growth and mitochondrial metabolism of mung bean. Am. J. Bot. 62, 97–102. doi: 10.1002/j.1537-2197.1975.tb12343.x
Dghim, A. A., Dumont, J., Hasenfratz-Sauder, M.-P., Dizengremel, P., Le Thiec, D., and Jolivet, Y. (2013). Capacity for NADPH regeneration in the leaves of two poplar genotypes differing in ozone sensitivity. Physiol. Plant. 148, 36–50. doi: 10.1111/j.1399-3054.2012.01686.x
D'Haese, D., Vandermeiren, K., Asard, H. A. N., and Horemans, N. (2005). Other factors than apoplastic ascorbate contribute to the differential ozone tolerance of two clones of Trifolium repens L. Plant Cell Environ. 28, 623–632. doi: 10.1111/j.1365-3040.2005.01308.x
Di Baccio, D., Castagna, A., Paoletti, E., Sebastiani, L., and Ranieri, A. (2008). Could the differences in O3 sensitivity between two poplar clones be related to a difference in antioxidant defense and secondary metabolic response to O3 influx? Tree Physiol. 28, 1761–1772. doi: 10.1093/treephys/28.12.1761
Dizengremel, P., Le Thiec, D., Bagard, M., and Jolivet, Y. (2008). Ozone risk assessment for plants: central role of metabolism-dependent changes in reducing power. Environ. Pollut. 156, 11–15. doi: 10.1016/j.envpol.2007.12.024
Dizengremel, P., Thiec, D. L., Hasenfratz-Sauder, M.-P., Vaultier, M.-N., Bagard, M., and Jolivet, Y. (2009). Metabolic-dependent changes in plant cell redox power after ozone exposure. Plant Biol. 11, 35–42. doi: 10.1111/j.1438-8677.2009.00261.x
Dos Santos, C. V., and Rey, P. (2006). Plant thioredoxins are key actors in the oxidative stress response. Trends Plant Sci. 11, 329–334. doi: 10.1016/j.tplants.2006.05.005
Dusart, N., Gérard, J., Le Thiec, D., Collignon, C., Jolivet, Y., and Vaultier, M.-N. (2019). Integrated analysis of the detoxification responses of two Euramerican poplar genotypes exposed to ozone and water deficit: focus on the ascorbate-glutathione cycle. Sci. Total Environ. 651, 2365–2379. doi: 10.1016/j.scitotenv.2018.09.367
Eckey-Kaltenbach, H., Heller, W., Sonnenbichler, J., Zetl, I., Schäfer, W., Ernst, D., et al. (1993). Oxidative stress and plant secondary metabolism: 6f two Euramerican poplar geno Phytochemistry 34, 687–691. doi: 10.1016/0031-9422(93)85340-W
Fernie, A. R., and Tóth, S. Z. (2015). Identification of the elusive chloroplast ascorbate transporter extends the substrate specificity of the PHT family. Mol. Plant 8, 674–676. doi: 10.1016/j.molp.2015.02.006
Foyer, C. H., and Noctor, G. (2011). Ascorbate and glutathione: the heart of the redox hub. Plant Physiol. 155, 2–18. doi: 10.1104/pp.110.167569
Foyer, C. H., and Noctor, G. (2016). Stress-triggered redox signalling: what's in pROSpect? Plant Cell Environ. 39, 951–964. doi: 10.1111/pce.12621
Foyer, C. H., Theodoulou, F. L., and Delrot, S. (2001). The functions of inter-and intracellular glutathione transport systems in plants. Trends Plant Sci. 6, 486–492. doi: 10.1016/S1360-1385(01)02086-6
Grace, S. C. (2007). “Phenolics as antioxidants,” in Antioxidants and Reactive Oxygen Species in Plants, ed N. Smirnoff (Oxford: Blackwell Publishing Ltd, 141–168.
Hernández, I., Alegre, L., Van Breusegem, F., and Munné-Bosch, S. (2009). How relevant are flavonoids as antioxidants in plants? Trends Plant Sci. 14, 125–132. doi: 10.1016/j.tplants.2008.12.003
Heyneke, E., Luschin-Ebengreuth, N., Krajcer, I., Wolkinger, V., Müller, M., and Zechmann, B. (2013). Dynamic compartment specific changes in glutathione and ascorbate levels in Arabidopsis plants exposed to different light intensities. BMC Plant Biol. 13:104. doi: 10.1186/1471-2229-13-104
Jiménez, A., Hernández, J. A., del Rio, L. A., and Sevilla, F. (1997). Evidence for the presence of the ascorbate-glutathione cycle in mitochondria and peroxisomes of pea leaves. Plant Physiol. 114, 275–284. doi: 10.1104/pp.114.1.275
Jolivet, Y., Bagard, M., Caban, M., Vaultier, M.-N., Gandin, A., Afif, D., et al. (2016). Deciphering the ozone-induced changes in cellular processes: a prerequisite for ozone risk assessment at the tree and forest levels. Ann. For. Sci. 73, 923–943. doi: 10.1007/s13595-016-0580-3
Keunen, E. L. S., Peshev, D., Vangronsveld, J., Van Den Ende, W. I. M., and Cuypers, A. N. N. (2013). Plant sugars are crucial players in the oxidative challenge during abiotic stress: extending the traditional concept. Plant Cell Environ. 36, 1242–1255. doi: 10.1111/pce.12061
Kleine, T., and Leister, D. (2016). Retrograde signaling: organelles go networking. Biochim. Biophys. Acta BBA-Bioenerg. 1857, 1313–1325. doi: 10.1016/j.bbabio.2016.03.017
Koffler, B. E., Luschin-Ebengreuth, N., Stabentheiner, E., Müller, M., and Zechmann, B. (2014). Compartment specific response of antioxidants to drought stress in Arabidopsis. Plant Sci. 227, 133–144. doi: 10.1016/j.plantsci.2014.08.002
Laisk, A., Kull, O., and Moldau, H. (1989). Ozone concentration in leaf intercellular air spaces is close to zero. Plant Physiol. 90, 1163–1167. doi: 10.1104/pp.90.3.1163
Langebartels, C., Kerner, K., Leonardi, S., Schraudner, M., Trost, M., Heller, W., et al. (1991). Biochemical Plant Responses to Ozone. Plant Physiol. 95:8. doi: 10.1104/pp.95.3.882
Li, P., Feng, Z., Catalayud, V., Yuan, X., Xu, Y., and Paoletti, E. (2017). A meta-analysis on growth, physiological, and biochemical responses of woody species to ground-level ozone highlights the role of plant functional types. Plant Cell Environ. 40, 2369–2380. doi: 10.1111/pce.13043
Luwe, M., and Heber, U. (1995). Ozone detoxification in the apoplasm and symplasm of spinach, broad bean and beech leaves at ambient and elevated concentrations of ozone in air. Planta 197, 448–455. doi: 10.1007/BF00196666
Mhamdi, A., Hager, J., Chaouch, S., Queval, G., Han, Y., Taconnat, L., et al. (2010a). Arabidopsis glutathione reductase1 plays a crucial role in leaf responses to intracellular hydrogen peroxide and in ensuring appropriate gene expression through both salicylic acid and jasmonic acid signaling pathways. Plant Physiol. 153, 1144–1160. doi: 10.1104/pp.110.153767
Mhamdi, A., Queval, G., Chaouch, S., Vanderauwera, S., Breusegem, F. V., and Noctor, G. (2010b). Catalase function in plants: a focus on Arabidopsis mutants as stress-mimic models. J. Exp. Bot. 61, 4197–4220. doi: 10.1093/jxb/erq282
Michalak, A. (2006). Phenolic compounds and their antioxidant activity in plants growing under heavy metal stress. Pol. J. Environ. Stud. 15, 523–530.
Millar, A. H., Mittova, V., Kiddle, G., Heazlewood, J. L., Bartoli, C. G., Theodoulou, F. L., et al. (2003). Control of ascorbate synthesis by respiration and its implications for stress responses. Plant Physiol. 133, 443–447. doi: 10.1104/pp.103.028399
Miyaji, T., Kuromori, T., Takeuchi, Y., Yamaji, N., Yokosho, K., Shimazawa, A., et al. (2015). AtPHT4; 4 is a chloroplast-localized ascorbate transporter in Arabidopsis. Nat. Commun. 6:5928. doi: 10.1038/ncomms6928
Moldau, H., Padu, E., and Bichele, I. (1997). Quantification of ozone decay and requirement for ascorbate in Phaseolus vulgaris L. mesophyll cell walls. Phyton Ann. Rei. Bot. 37, 175–180.
Musselman, R. C., Lefohn, A. S., Massman, W. J., and Heath, R. L. (2006). A critical review and analysis of the use of exposure-and flux-based ozone indices for predicting vegetation effects. Atmos. Environ. 40, 1869–1888. doi: 10.1016/j.atmosenv.2005.10.064
Navrot, N., Rouhier, N., Gelhaye, E., and Jacquot, J.-P. (2007). Reactive oxygen species generation and antioxidant systems in plant mitochondria. Physiol. Plant. 129, 185–195. doi: 10.1111/j.1399-3054.2006.00777.x
Noctor, G., and Foyer, C. H. (2016). Intracellular redox compartmentation and ROS-related communication in regulation and signaling. Plant Physiol. 171, 1581–1592. doi: 10.1104/pp.16.00346
Noctor, G., Mhamdi, A., and Foyer, C. H. (2016). Oxidative stress and antioxidative systems: recipes for successful data collection and interpretation. Plant Cell Environ. 39, 1140–1160. doi: 10.1111/pce.12726
Noctor, G., Reichheld, J.-P., and Foyer, C. H. (2018). ROS-related redox regulation and signaling in plants. Semin. Cell Dev. Biol. 80, 3–12. doi: 10.1016/j.semcdb.2017.07.013
Noctor, G., Veljovic-Jovanovic, S., Driscoll, S., Novitskaya, L., and Foyer, C. H. (2002). Drought and oxidative load in the leaves of C3 plants: a predominant role for photorespiration? Ann. Bot. 89, 841–850. doi: 10.1093/aob/mcf096
Oksanen, E., Häikiö, E., Sober, J., and Karnosky, D. F. (2004). Ozone-induced H2O2 accumulation in field-grown aspen and birch is linked to foliar ultrastructure and peroxisomal activity. New Phytol. 161, 791–799. doi: 10.1111/j.1469-8137.2003.00981.x
Pellegrini, E., Hoshika, Y., Dusart, N., Cotrozzi, L., Gérard, J., Nali, C., et al. (2019). Antioxidative responses of three oak species under ozone and water stress conditions. Sci. Total Environ. 647, 390–399. doi: 10.1016/j.scitotenv.2018.07.413
Pellinen, R., Palva, T., and Kangasjärvi, J. (1999). Subcellular localization of ozone-induced hydrogen peroxide production in birch (Betula pendula) leaf cells. Plant J. 20, 349–356. doi: 10.1046/j.1365-313X.1999.00613.x
Plöchl, M., Lyons, T., Ollerenshaw, J., and Barnes, J. (2000). Simulating ozone detoxification in the leaf apoplast through the direct reaction with ascorbate. Planta 210, 454–467. doi: 10.1007/PL00008153
Pollastri, S., and Tattini, M. (2011). Flavonols: old compounds for old roles. Ann. Bot. 108, 1225–1233. doi: 10.1093/aob/mcr234
Polle, A., Wieser, G., and Havranek, W. M. (1995). Quantification of ozone influx and apoplastic ascorbate content in needles of Norway spruce trees (Picea abies L., Karst) at high altitude. Plant Cell Environ. 18, 681–688. doi: 10.1111/j.1365-3040.1995.tb00569.x
Queval, G., Jaillard, D., Zechmann, B., and Noctor, G. (2011). Increased intracellular H2O2 availability preferentially drives glutathione accumulation in vacuoles and chloroplasts. Plant Cell Environ. 34, 21–32. doi: 10.1111/j.1365-3040.2010.02222.x
Rahantaniaina, M.-S., Li, S., Chatel-Innocenti, G., Tuzet, A., Issakidis-Bourguet, E., Mhamdi, A., et al. (2017). Cytosolic and chloroplastic DHARs cooperate in oxidative stress-driven activation of the salicylic acid pathway. Plant Physiol. 174, 956–971. doi: 10.1104/pp.17.00317
Rahantaniaina, M.-S., Tuzet, A., Mhamdi, A., and Noctor, G. (2013). Missing links in understanding redox signaling via thiol/disulfide modulation: how is glutathione oxidized in plants? Front. Plant Sci. 4:477. doi: 10.3389/fpls.2013.00477
Ranieri, A., D'urso, G., Nali, C., Lorenzini, G., and Soldatini, G. F. (1996). Ozone stimulates apoplastic antioxidant systems in pumpkin leaves. Physiol. Plant. 97, 381–387. doi: 10.1034/j.1399-3054.1996.970224.x
Rausch, T., Gromes, R., Liedschulte, V., Müller, I., Bogs, J., Galovic, V., et al. (2007). Novel insight into the regulation of GSH biosynthesis in higher plants. Plant Biol. 9, 565–572. doi: 10.1055/s-2007-965580
Rautenkranz, A. A., Li, L., Machler, F., Martinoia, E., and Oertli, J. J. (1994). Transport of ascorbic and dehydroascorbic acids across protoplast and vacuole membranes isolated from barley (Hordeum vulgare L. cv Gerbel) leaves. Plant Physiol. 106, 187–193. doi: 10.1104/pp.106.1.187
Rhoads, D. M., Umbach, A. L., Subbaiah, C. C., and Siedow, J. N. (2006). Mitochondrial reactive oxygen species. Contribution to oxidative stress and interorganellar signaling. Plant Physiol. 141, 357–366. doi: 10.1104/pp.106.079129
Riikonen, J., Mäenpä, M., Alavillamo, M., Silfver, T., and Oksanen, E. (2009). Interactive effect of elevated temperature and O3 on antioxidant capacity and gas exchange in Betula pendula saplings. Planta 230, 419–427. doi: 10.1007/s00425-009-0957-8
Rouhier, N., and Jacquot, J.-P. (2005). The plant multigenic family of thiol peroxidases. Free Radic. Biol. Med. 38, 1413–1421. doi: 10.1016/j.freeradbiomed.2004.07.037
Sakihama, Y., Cohen, M. F., Grace, S. C., and Yamasaki, H. (2002). Plant phenolic antioxidant and prooxidant activities: phenolics-induced oxidative damage mediated by metals in plants. Toxicology 177, 67–80. doi: 10.1016/S0300-483X(02)00196-8
Severino, J. F., Stich, K., and Soja, G. (2007). Ozone stress and antioxidant substances in Trifolium repens and Centaurea jacea leaves. Environ. Pollut. 146, 707–714. doi: 10.1016/j.envpol.2006.04.006
Shapiguzov, A., Vainonen, J., Wrzaczek, M., and Kangasjärvi, J. (2012). ROS-talk–how the apoplast, the chloroplast, and the nucleus get the message through. Front. Plant Sci. 3:292. doi: 10.3389/fpls.2012.00292
Smirnoff, N. (2018). Ascorbic acid metabolism and functions: a comparison of plants and mammals. Free Radic. Biol. Med. 122, 116–129. doi: 10.1016/j.freeradbiomed.2018.03.033
Sutinen, S., Skarby, L., Wallin, G., and Sellden, G. (1990). Long-term exposure of Norway spruce, Picea abies (L.) Karst., to ozone in open top chambers. II. Effects on the ultrastructure of needles. New Phytol. 115, 345–355. doi: 10.1111/j.1469-8137.1990.tb00461.x
Takahama, U., and Oniki, T. (1997). A peroxidase/phenolics/ascorbate system can scavenge hydrogen peroxide in plant cells. Physiol. Plant. 101, 845–852. doi: 10.1111/j.1399-3054.1997.tb01072.x
Tripathy, B. C., and Oelmüller, R. (2012). Reactive oxygen species generation and signaling in plants. Plant Signal. Behav. 7, 1621–1633. doi: 10.4161/psb.22455
Tuzet, A., Perrier, A., Loubet, B., and Cellier, P. (2011). Modelling ozone deposition fluxes: the relative roles of deposition and detoxification processes. Agric. For. Meteorol. 151, 480–492. doi: 10.1016/j.agrformet.2010.12.004
Tuzet, A., Rahantaniaina, M.-S., and Noctor, G. (2019). Analyzing the function of catalase and the ascorbate–glutathione pathway in H2O2 processing: insights from anexperimentally constrained kinetic model. Antioxid. Redox Signal. 30, 1238–1268. doi: 10.1089/ars.2018.7601
Van Hove, L. W. A., Bossen, M. E., San Gabino, B. G., and Sgreva, C. (2001). The ability of apoplastic ascorbate to protect poplar leaves against ambient ozone concentrations: a quantitative approach. Environ. Pollut. 114, 371–382. doi: 10.1016/S0269-7491(00)00237-2
Veljovic-Jovanovic, S., Noctor, G., and Foyer, C. H. (2002). Are leaf hydrogen peroxide concentrations commonly overestimated? The potential influence of artefactual interference by tissue phenolics and ascorbate. Plant Physiol. Biochem. 40, 501–507. doi: 10.1016/S0981-9428(02)01417-1
Vreeburg, R. A. M., and Fry, S. C. (2007). “Reactive oxygen species in cell walls,” in Antioxidants and Reactive Oxygen Species in Plants, ed N. Smirnoff (Oxford: Blackwell Publishing Ltd, 215–249.
Waszczak, C., Carmody, M., and Kangasjarvi, J. (2018). Reactive oxygen species in plant signaling. Annu. Rev. Plant Biol. 69, 209–236. doi: 10.1146/annurev-arplant-042817-040322
Wittig, V. E., Ainsworth, E. A., Naidu, S. L., Karnosky, D. F., and Long, S. P. (2009). Quantifying the impact of current and future tropospheric ozone on tree biomass, growth, physiology and biochemistry: a quantitative meta-analysis. Glob. Change Biol. 15, 396–424. doi: 10.1111/j.1365-2486.2008.01774.x
Yoshida, S., Tamaoki, M., Shikano, T., Nakajima, N., Ogawa, D., Ioki, M., et al. (2006). Cytosolic dehydroascorbate reductase is important for ozone tolerance in Arabidopsis thaliana. Plant Cell Physiol. 47, 304–308. doi: 10.1093/pcp/pci246
Zechmann, B. (2011). Subcellular distribution of ascorbate in plants. Plant Signal. Behav. 6, 360–363. doi: 10.4161/psb.6.3.14342
Zechmann, B. (2014). Compartment-specific importance of glutathione during abiotic and biotic stress. Front. Plant Sci. 5:566. doi: 10.3389/fpls.2014.00566
Zechmann, B. (2017). Compartment-specific importance of ascorbate during environmental stress in plants. Antioxid. Redox Signal. 29, 1488–1501. doi: 10.1089/ars.2017.7232
Zechmann, B., Mauch, F., Sticher, L., and Müller, M. (2008). Subcellular immunocytochemical analysis detects the highest concentrations of glutathione in mitochondria and not in plastids. J. Exp. Bot. 59, 4017–4027. doi: 10.1093/jxb/ern243
Zechmann, B., Stumpe, M., and Mauch, F. (2011). Immunocytochemical determination of the subcellular distribution of ascorbate in plants. Planta 233, 1–12. doi: 10.1007/s00425-010-1275-x
Zhang, Y.-J., Wang, W., Yang, H.-L., Li, Y., Kang, X.-Y., Wang, X.-R., et al. (2015). Molecular properties and functional divergence of the dehydroascorbate reductase gene family in lower and higher plants. PLoS ONE 10:e0145038. doi: 10.1371/journal.pone.0145038
Keywords: ozone, ROS, antioxidants, cellular compartmentation, risk assessment models
Citation: Dusart N, Gandin A, Vaultier M-N, Joffe R, Cabané M, Dizengremel P and Jolivet Y (2019) Importance of Detoxification Processes in Ozone Risk Assessment: Need to Integrate the Cellular Compartmentation of Antioxidants? Front. For. Glob. Change 2:45. doi: 10.3389/ffgc.2019.00045
Received: 12 June 2019; Accepted: 05 August 2019;
Published: 20 August 2019.
Edited by:
Elena Paoletti, Italian National Research Council (CNR), ItalyReviewed by:
Marcela Regina Engela, Institute of Botany São Paulo, BrazilCopyright © 2019 Dusart, Gandin, Vaultier, Joffe, Cabané, Dizengremel and Jolivet. This is an open-access article distributed under the terms of the Creative Commons Attribution License (CC BY). The use, distribution or reproduction in other forums is permitted, provided the original author(s) and the copyright owner(s) are credited and that the original publication in this journal is cited, in accordance with accepted academic practice. No use, distribution or reproduction is permitted which does not comply with these terms.
*Correspondence: Yves Jolivet, eXZlcy5qb2xpdmV0QHVuaXYtbG9ycmFpbmUuZnI=
Disclaimer: All claims expressed in this article are solely those of the authors and do not necessarily represent those of their affiliated organizations, or those of the publisher, the editors and the reviewers. Any product that may be evaluated in this article or claim that may be made by its manufacturer is not guaranteed or endorsed by the publisher.
Research integrity at Frontiers
Learn more about the work of our research integrity team to safeguard the quality of each article we publish.