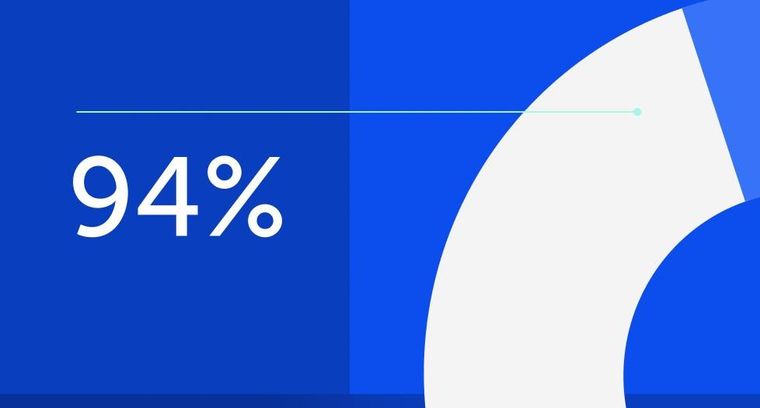
94% of researchers rate our articles as excellent or good
Learn more about the work of our research integrity team to safeguard the quality of each article we publish.
Find out more
ORIGINAL RESEARCH article
Front. For. Glob. Change, 02 July 2019
Sec. Tropical Forests
Volume 2 - 2019 | https://doi.org/10.3389/ffgc.2019.00034
This article is part of the Research TopicIntact ForestsView all 18 articles
A correction has been applied to this article in:
Corrigendum: The Legacy of Pre–Columbian Fire on the Pine–Oak Forests of Upland Guatemala
Mountain tropical forests of the Southern Maya Area (Pacific Chiapas and Guatemala, El Salvador, and Northern Honduras) predominantly comprise pine and oak formations, which form intricate mosaics and complex successional interactions following large–scale fire. These forests have been transformed by the peoples of the Maya civilization through practices of horticulture, agriculture, and architectural developments over thousands of years. Anthropogenic impacts and the extent of early human interaction with these upland forests is currently poorly understood. In this study we identify: (i) the natural baseline vegetation of the region; (ii) when human impact and agrarian practices began in the Maya uplands; and (iii) what impacts the Maya had on forest structure, composition, and successional regeneration. Past vegetation, anthropogenic use of fire, and faunal abundance were reconstructed using proxy analysis of fossil pollen, macroscopic charcoal, microscopic charcoal, and dung fungal spores (Sporormiella). Three phases of forest succession were identified from 4000B.C.E. to 1522CE that broadly overlap with the well–defined archaeological periods of (i) the Archaic (10,000–2000B.C.E.); (ii) Pre–Classic (2000B.C.E.−100C.E.); (iii) Terminal Pre–Classic (100–250C.E.); (iv) Classic (250–950C.E.); and (v) Post–Classic (950–1522C.E.). These results also include the earliest evidence for agriculture within the Southern Maya Area through presence of peppers (Capsicum) from 3850B.C.E. and the rise of maize cultivation (Zea mays) from 970B.C.E. Persistent high intensity burning driven by agricultural practices and lime production during the Late–Pre-Classic (400–100B.C.E.) to Classic Period resulted in a compositional change of forest structure c.150B.C.E. from oak (Quercus) dominated forests to pine (Pinus) dominated forests. The legacy of Pre–Columbian anthropogenically driven fire in these mountain tropical forests demonstrates the resilience and thresholds for fire driven succession. These findings are particularly relevant for addressing current land use and management strategies involving agriculture, fire, and forest management in the mountain tropical forests of the Southern Maya Area.
Unlike the Amazon or boreal regions, Central America does not have large tracts of intact forests (Watson et al., 2018), and further reductions in the extent of intact forests are a concern. Potapov et al. (2017), for example, determined that forest cover declined by 13.3% in Guatemala between 2000 and 2013C.E. Mountain tropical forests (MTF) are important for the provision of ecosystem services, particularly biodiversity and water (Martínez et al., 2009), and loss of intactness has serious implications for these ecosystem services. Given the current high international interest in the effects of human actions that cause degradation of forests and subsequent loss of ecological function, the development of long–term ecological data will provide insight on how forests were influenced by anthropogenic and natural factors during pre–history and thereby help inform potential future responses to similar actions and/or occurrences. For Central America such long–term ecological datasets are scarce and are rarely considered in modern conservation discussions (Jeffers et al., 2015). In particular, the Middle to Late Holocene (6000–2000B.C.E.) vegetation history of the Central American uplands (>1,000 m.a.s.l.) is not well-documented but is essential to our understanding of early human impacts and prehistoric land use across the Maya Area (Neff et al., 2006). Filling these temporal and spatial gaps in knowledge is essential for understanding the function and protection of intact forests in this region and more generally.
The Maya occupied three separate areas: the Southern Uplands, and the Central, and Northern Lowlands (<1,000 m) (Figure 1). Our research area lies within the Southern Uplands, which includes the highlands of Guatemala and adjacent Chiapas (Coe and Houston, 1966). There are altitudinal gradients in temperature and precipitation, with annual mean temperatures ranging between 14 and 25°C and annual rainfall ranging between 900 and 3,700 mm per year (Kappelle, 2006). The vegetation inhabiting this upland area typically comprises tropical and subtropical mixed deciduous and coniferous forests, known as mountain tropical forest (MTF) formations, which start in the Sierra Madre de Chiapas (Southern Mexico) and extend down to Northern Nicaragua (Dinerstein et al., 2017).
Figure 1. Topographic map of Central America depicting (i) Maya regions; (ii) independent climate proxies (blue diamonds); (iii) previous vegetation reconstructions in the Maya Area (green diamonds); (iv) location of archaeological complex Chinkultic (black triangle); and (v) location of Cenote Kail (red circle). Dark gray shading represents elevation >1,000 m.a.s.l. (see Supplementary Information for sites).
Early human populations across Central America are suggested to have increasingly interacted with their surrounding environment from 6000B.C.E., aided by progressively more favorable climatic conditions (Turner and Miksicek, 1984; Colunga–GarcíaMarín and Zizumbo–Villarreal, 2004; Ford and Nigh, 2009). In palaeoecology records from Maya sites, anthropogenic impacts to vegetation are typically inferred from the: (i) presence of known cultigens, such as Capsicum (peppers), Cucurbitaceae (gourds), Maranta arundinacea (arrowroot), Phaseolus (beans), and Zea mays (Maize) (White, 1999); (ii) presence of “weedy taxa,” such as, Amarathaceae, Compositae, and Polygonum (Dull, 2004a; Franco-Gaviria et al., 2018); (iii) reductions in all or select arboreal taxa, such as Quercus (Dull, 2004a,b, 2007; Velez et al., 2011); and (iv) increases in local and regional burning (e.g., Dull, 2004a,b, 2007; Anderson and Wahl, 2016).
Archaic (10,000–2000B.C.E.) anthropogenic impacts and the extent of early human interaction with the upland forests of the Maya Area are currently poorly understood. There have been 11 palaeopalynological studies conducted in the southern Maya Area (Figures 1, 2), and only five of these reconstructions have been undertaken in the uplands (Dull, 2004a; Caffrey et al., 2011; Velez et al., 2011; Franco-Gaviria et al., 2018). Interpretations of fossil pollen and charcoal records from Laguna Verde (El Salvador) and Lago Amatitlan (Guatemala) identify extensive human alteration of the upland vegetation between 2550 and 625B.C.E. (Dull, 2004a; Velez et al., 2011). Likewise, records from Lake San Lorenzo and Lake Esmeralda (Chiapas) provide evidence that anthropogenic and climatic impacts drove vegetation changes from c.450B.C.E. (Franco-Gaviria et al., 2018). In contrast, palynological data from Miqul Meadow (Guatemala) indicates that climate is the sole driver of vegetation change (Caffrey et al., 2011). These records are mostly low in resolution (more than 200 years between samples: e.g., Laguna Verde and Miqul Meadow) and poorly chronologically constrained (e.g., Miqul Meadow), limitations that prevent conclusive assessment of questions related to the timing of events. In order to reconstruct the impacts of disturbance events in MTF and forest succession, palaeoecological datasets must be sampled at a resolution higher than that of the rate of succession, which is up to 200 years in MTF (Kappelle, 2006). In addition, many of these studies rely on geographically distant proxy records to infer the impacts of climate on vegetation dynamics (e.g., La Yeguada, Panama: (Bush et al., 1992) and Peten Itza, Guatemala: Islebe et al., 1996). Given the spatial heterogeneity in precipitation patterns across Central America through time (e.g., Metcalfe et al., 2015), it is critical to include local palaeoclimate records for comparison with vegetation reconstructions.
Figure 2. Summary of reported vegetation drivers and impacts to the vegetation from the Maya Area during the Pre–Columbian Holocene, as reported by authors from palynological reconstructions (see Supplementary Information for sites).
Currently the arboreal canopy of MTF predominantly comprise a combination of coniferous forest taxa (e.g., Pinus and Abies) and mixed hardwood forest (MHWF) taxa (e.g., Quercus, Alnus, and Liquidambar), which are well-adapted to variable climatic conditions and natural fires (Corrales et al., 2015). These MTF are a combination of pine forests (PF), pine–oak forests (POF), mountain–pine–oak forests (MPOF), oak forests (OF), pine–oak–Liquidambar forests (POLF), mountain rain forests (MRF), and cloud forests (CF) (Kappelle, 2006). These typically overlap in floristic composition but vary in species abundance (Miranda, 1952; Breedlove, 1981; Kappelle, 2006; Figueroa-Rangel et al., 2008, 2010, 2012). POF form intricate mosaics and complex successional interactions, especially at higher elevations, which extend up into the broad–leaved evergreen CF (Rzedowski, 2006). Altitudinally, MPOF in Chiapas are found above 1,500 m, while POF have an extensive range from 500 to 3,400 m.a.s.l., with strong turnovers between species along altitudinal gradients (Kappelle, 2006). There are over 150 species of Pinus and Quercus that can be found across the uplands of Guatemala (Muller, 1942; Kappelle, 2006). Only 47% of forest cover (1990–2000C.E.) remains, and it is highly fragmented due to high human population densities and land modification for subsistence farming (Corrales et al., 2015).
Drivers of POF dynamics have been previously investigated in upland Guatemala (Velez et al., 2011), neighboring Chiapas (Domínguez-Vázquez and Islebe, 2008), Pacific Mexico (Figueroa-Rangel et al., 2008, 2010, 2012) and Costa Rica (Islebe and Hooghiemstra, 1997), detailing the climatic and anthropogenic mechanisms that contribute toward changes in POF composition through time (Kappelle, 2006). In these studies fire has been identified as the dominant driver of structural and successive turnover within POF systems. However, there is disagreement as to what factors are driving these fires, changes in burning are controlled by complex interactions of fire, fuel load, climate, and humans (Cochrane and Barber, 2009; Bowman et al., 2011; Anderson and Wahl, 2016). While it is not possible wholly to differentiate between anthropogenic and naturally occurring fires (Anderson and Wahl, 2016), inferences can be made by combining multiple lines of evidence such as fossil charcoal with the presence of known agricultural grains (e.g., Zea mays) (Dull, 2004a,b, 2007), or with climatic proxy data, to infer known shifts in precipitation, which can impact fuel loads and ignitions (Bowman et al., 2011).
Research conducted on the Pacific coast of Mexico (Figueroa-Rangel et al., 2008, 2010, 2012) and in Costa Rica (Islebe and Hooghiemstra, 1997) suggests that fire within POF are primarily climatically driven; whereas palynological work in Guatemala and Chiapas (Domínguez-Vázquez and Islebe, 2008; Velez et al., 2011) indicates a more intertwined relationship of climate and people. Other factors considered to affect the structure and successional regeneration of POF are overall climate (temperature and precipitation), soil (type, nutrient availability), and anthropogenic activities (timber extraction and agriculture) (Kappelle, 2006). In addition, herbivory can cause deviations in successional pathways through sapling browsing and seed dispersal (Baker et al., 2016;Arroyo-Rodríguez et al., 2017).
It has been suggested that without further disturbances natural recovery will return a fallow field to a POF within c.80 years (Figueroa-Rangel et al., 2008). Sustained low–intensity and long-duration human disturbance leads to a deviation from this natural sequence resulting in slowed recovery, and more intensive anthropogenic or climatic disturbances can reverse or reset recovery times (Kappelle, 2006). However, these are theoretical timelines and to date there is very little evidence on recovery rates from different types of disturbances (fire, human, climate) in this region, nor on how this varies according to altitude.
This study seeks to identify (i) the natural baseline vegetation of the region; (ii) when human impact and agrarian practices began in the Maya uplands; and (iii) what impacts the Maya had on forest structure, composition, and successional regeneration. To address the potential impacts of anthropogenic influences and herbivory upon the biota of upland Guatemala, a proxy reconstruction of changes in vegetation, burning, and animal populations from 4000B.C.E. to 1522C.E. was developed using fossil pollen, macroscopic charcoal (>150 μm), microscopic charcoal (<150 μm), and fossil dung fungal spore (Sporormiella), from Cenote Kail, a lake situated in the uplands of the Southern Maya Area. These records were then compared with local and regional climatic archives and information on human population dynamics collected from nearby archaeological sites.
Cenote Kail (150 m diameter) is located within the uplands of the Southern Maya Area (N16°00′00.0″W91°33′14.4, 1,534 m.a.s.l.) and situated 28 km away from the well-documented archaeological complex Chinkultic (Ball, 1980; Figure 1). This city was established sometime between 50B.C.E and 75C.E. and occupied until 300–350C.E. The city was then abandoned between 350 and 700C.E. before being occupied again from 700 to 1,250C.E. (Ball, 1980). The lake is presently surrounded by a coniferous forest mosaic best described as POF or MPOF. Vegetation is distributed between densely populated mixed deciduous and coniferous forested patches, and large open shrub/grasslands.
In 2015 a 545 cm–long composite sediment core, with overlapping sections, was extracted from Cenote Kail using a Livingstone piston corer (Livingstone, 1955). Forty-six samples (1g wet weight) were extracted at 10 cm intervals for biological proxy analysis of macroscopic charcoal (>150 μm), microscopic charcoal (<150 μm), pollen, and coprophilous fungal spores (Sporormiella).
An age depth model was constructed using 38 calibrated radiocarbon dates obtained from charcoal and terrestrial leaf fragments, which represent a single event or from one to two seasons of growth (Table 1). Samples were pre–treated using standard acid–base–acid protocols (Abbott and Stafford, 1996). Radiocarbon dates were generated at the W.M. Keck Carbon Cycle Accelerator Mass Spectrometry Laboratory at the University of California, Irvine. The IntCal13 radiocarbon dataset (Reimer et al., 2013) was used to calibrate the measured radiocarbon dates, and OxCal (v.4.3) was used to construct an age–depth model applying a Bayesian approach (Ramsey, 2009). Outliers were identified using the general outlier model implementing an outlier probability of 0.05 (Ramsey, 2008). Sedimentation rates were calculated using this age-depth model.
Fossil pollen was used to reconstruct the abundance and composition of past vegetation dynamics. Fossil pollen extraction and preparation followed standard palynological procedures applying the Oxford Long–Term Ecology Laboratory (OxLEL) protocol (OxLEL, 2016). Silicone oil was used as the mounting agent to allow for the rotation of grains, easing identification. Samples were spiked with known concentrations of an exotic marker, Lycopodium spores (batch No. 20848 or 9666), to calculate pollen accumulation rates. Pollen influx was calculated using pollen accumulation rates and sedimentation rate (Bennett and Willis, 2001). Counting and identification of pollen grains were conducted at 400x and 1000x magnification. For each level a minimum of 300 terrestrial pollen grains were counted (Data Sheet 1). Morphological identification was achieved using (i) pollen databases (APSA, 2007; Bush and Weng, 2007; Martin and Harvey, 2017); (ii) published plates: (Roubik and Moreno, 1991; Willard et al., 2004); and (iii) botanical reference materials from the OxLEL reference collection. In order to interpret the relative composition of the forest, coniferous and mixed—hard—wood forest (MHWF) canopy taxa were compared as a ratio. The abundance of Sporormiella spores was used to indicate herbivorous animal presence and abundance. Sporormiella spores were counted and morphologically identified on the same slides (Davis and Shafer, 2006; Baker et al., 2016).
Macroscopic fossil charcoal fragments (150 μm), were used to infer past occurrences of local fires where local is taken to represent burning within a 10 km radius of the catchment area (Gavin et al., 2003; Lynch et al., 2004; Higuera et al., 2007, 2011; Peters and Higuera, 2007; Anderson and Wahl, 2016). All fragments over 150 μm in the 1 g samples were counted at 10x magnification.
Microscopic charcoal (<150 μm), representing a regional signal of up to 100 km (see Clark, 1988), were also counted on the same slides, applying the point counting method at 400x magnification (Clark, 1982). Microscopic charcoal counts were recorded until a minimum of 50 Lycopodium spores and 200 fields of view were encountered for each level to allow for influx of microscopic charcoal (cm2 per year) to be calculated.
Pollen counts were converted to percentages, while Sporormiella, macroscopic, and microscopic charcoal are presented as annual influx (Maher, 1981; Bennett, 1994; Bennett and Willis, 2001; Whitlock and Larsen, 2002; Baker et al., 2016). To identify discrete zones in the resulting palynological diagrams, constrained hierarchical clustering upon the palynological assemblage was applied following the broken stick model (Bennett, 1996).
Statistical analysis and presentation of data were performed using packages Vegan (Oksanen et al., 2015) and Rioja (Juggins et al., 2009) in base R (R. Core Team, 2012). Before preforming all ordination analyses, the percentage data were square–root transformed to normalize the distribution and for variance stabilization (Bennett and Willis, 2001; Legendre and Legendre, 2012). A square root transform was chosen because it can be applied to data sets containing zero values. Detrended Correspondence Analysis (DCA) was conducted upon the palynological assemblage data to check if it was appropriate to apply a linear or unimodal ordination method (Ter Braak and Prentice, 1988). The site scores for the first axis of the DCA were then extracted to calculate the species turnover. Next, a Principal Component Analysis (PCA) was used to infer similarities between samples and the change in trajectories of composition of taxa through time, applying a singular value decomposition of the centered, but not scaled, data matrix. Finally, a Canonical Correspondence Analysis (CCA) was performed to quantify the relationship between environmental variables (fire and herbivory) and the palynological assemblage data. Ellipses representing the discrete Zones were calculated using standard parameterization (cos(theta + d/2), cos(theta – d/2)), where cos(d) is the correlation of the parameters (see Murdoch and Chow, 1996).
The age–depth model indicates that the sediment sequence (545–105 cm) continuously (i.e., without hiatus) spans 4000B.C.E. to 1522C.E. (Figure 3). The general outlier model (see Ramsey, 2008) identified six dates as outliers and thus were removed from the overall age–depth model (Table 1). The overall model agreement index was high (96.7), indicating there is very little variance between the modeled ages and the observational data. The sedimentation rate is on average 1.2 mm per year.
Three statistically significant Zones were identified using the broken stick model (Figures 4, 5). Seventy–six taxa were recognized in the palynological sequence extracted from Cenote Kail (see Supplementary Information). Throughout this sequence Pinus, Quercus and Morella cerifera dominate the arboreal component while Compositae and Poaceae are the most abundant herbaceous taxa (Figure 5). Temporal spacing between samples is as follows: (i) Zone 1 represents an average spacing of 130 years spanning 1800 years (with a range of 68–415 years); (ii) Zone 2 represents an average spacing of 137 years spanning 2300 years (with a range of 53–192 years); and, (iii) Zone 3 represents an average of 90 years spanning 1400 years (with a range of 68–160 years).
Figure 4. Canonical correspondence analysis of the palaeopalynological data set ordinated against independent environmental indicators of local fire (macroscopic charcoal), regional fire (microscopic charcoal), and herbivory (Sporormiella) (A). Principal component analysis of the palaeopalynological data set (B). Zones are derived from the broken stick model and are represented by ellipses at a confidence of 95%. Zone 1 = green triangles; Zone 2 = yellow squares; Zone 3 = blue circles.
Figure 5. Palynological percentage diagram of taxa appearing in an abundance >2%; forest structure; coniferous to hardwood ratio; pollen influx; macroscopic and microscopic influx; DCA axis 1; and Occupation of Chinkultic (dark bands). The palynological data are expressed as a percentage of total land pollen.
Results from the CCA show that microscopic and macroscopic charcoal are significant environmental variables most associated with Zones 2 and 3, while Sporormiella is most associated with Zone 1 and is not statistically significant (Figure 4A). The PCA displays a distinct gradient and several associations between taxa and samples (Figure 4B). The first axis represents 20.2% of the variation, while axis two represents 10.5% of the variation. The arch between samples suggests that there is only one clear gradient. When the independently calculated palynological Zones are superimposed upon these quadrants, the top, and bottom right quadrants are most associated with Zone 1, the top left quadrant is most associated with Zone 2 and the bottom left quadrant is most associated with Zone 3 (Figure 4B). The first taxonomic association comprises canopy taxa Quercus, understory taxa Leguminosae, Myrica, Ericaceae, and the herbaceous and agrarian taxa Capsicum, which is most associated with Zone 1. The second taxonomic association comprises of understory taxa Juniperus and Terminalia, herbaceous taxa Polygalaceae, Apiaceae, Compositae Aphelandra, and agrarian taxa Capsicum most associated with Zone 2. The third taxonomic association comprises canopy taxa Pinus, Alnus, and Liquidambar together with understory taxa Morella cerifera, and herbaceous taxa Poaceae, and Campanulaceae, which is most associated with Zone 3 (Figure 4B).
Zone 1 (545.75–421 cm, 14 samples, 4000–2200B.C.E.) spans c.1800 years concurrent with the last 2000 years of the Archaic Period (10,000–2000B.C.E.) (Figure 5). This Zone is predominantly defined by POF taxa Quercus (25.9%) and Pinus (11%) alongside herbaceous taxa Compositae (27.6%). Between 4000 and 3300 B.C.E. there is evidence for a decline in canopy taxa (from 61.3 to 37.3% of the total pollen sum), particularly Quercus (18–9.7%). In contrast, Pinus became abundant during this period, rising from 4000B.C.E. (2.3%) to 3300B.C.E. (16.7%). Myrica and Alnus are mostly present throughout this Zone, peaking at 3100B.C.E. (15.3%). Brosimum (0–4.3%), Anacardiaceae (0.3–4.3%), Leguminosae (0–6.3%), Morella cerifera, and Rubiaceae (0–9%) are present in low abundance and on average decrease from 4000 to 2200B.C.E. The ratio of coniferous to MHWF is on average 30:70. The coniferous to MHWF ratio changes from 25:75 to 66:34 between 3700 and 3300B.C.E. Compositae abundance increases between 4000 and 3200B.C.E. (17.3–35.3%) and peaks at 2900B.C.E. (53.3%) and 2700B.C.E. (47.3%). Amaranthaceae is present at 10.3% from 4000B.C.E. but subsequently declines leading up to 3700B.C.E. (1.3%). Capsicum appears and increases from 3850 to 3300 B.C.E. (0.3–5.3%), and Polygalaceae follows a similar trend, peaking at 3300B.C.E. (4.3%). Poaceae is stable and in low abundance throughout the record (2.3–5.6%). Pollen influx is low, decreasing from 4000 to 3300B.C.E. (965–344 grains x103 cm2 per year) and increasing after 2300B.C.E. (1,919 grains x104 cm2 per year). Sporomiella abundance is relatively high and stable (2.6–10.5%), falling below 4% abundance at 3100B.C.E. (2.6%), 2700B.C.E. (2.9%), and 2320B.C.E. (3.8%); there is a sustained high abundance of >10% between 2600 and 2500B.C.E. (10.2–13.3%). Macroscopic and microscopic charcoal are relatively low, decreasing between 4000 and 3000B.C.E. (macroscopic charcoal: 0.44–0.32 particles cm2 per year; and, microscopic charcoal: 552–86 particles cm2yr1) then increasing through to 2300B.C.E (macroscopic: 1.2 particles cm2 per year; and, microscopic: 770 particles cm2 per year).
Zone 2 (412−263.5cm, 17 samples, 2200B.C.E−100C.E.) spans 2100 years, including the Early, Middle, and Late Pre–Classic Periods (2000–B.C.E.−100C.E.) and is defined by arboreal taxa Quercus, Pinus and Morella cerifera and herbaceous taxa Compositae, Poaceae, and Zea mays (Figure 5). Quercus continues to dominate the arboreal component (23%) but decreases between 1550 and 970B.C.E. (34–6%). After 970B.C.E. (6%) Quercus recovers until 550B.C.E. (43%) before decreasing rapidly by 350C.E. (17.3%) and stabilizing by 100C.E. (13%). Pinus continues to be persistently present and in stable abundance with slight increases between 1550 and 650B.C.E. (4.6–17.7%) and a larger increase from 340B.C.E.−100C.E. (6.3–28.3%). The low ratio of coniferous to MHWF taxa indicates relatively abundant MHWF, particularly Quercus (47:63), with a turnover to more coniferous taxa between 1150 and 970B.C.E. (17:83–74:26) and 230B.C.E.−50C.E. (4:6–7:3). Morella cerifera first substantially enters the record from 970B.C.E. (10.3%) but does not establish until 230B.C.E. where it rises to the second most abundant forest taxa through to 100C.E. (19.7%). Prior to the arrival of Zea mays (c.1000B.C.E.), Amaranthaceae abundance briefly increases c.1150B.C.E. (5.3%). The rise of Zea mays from 970B.C.E. (8.6%) peaks at 930B.C.E. (17%) and is abundant until 650B.C.E. (4.6%). Polygalaceae re–establishes between 1350 and 750B.C.E. (4–3.3%). Poaceae begins to increase from 1150B.C.E (2%) through to 100C.E. (11.5%), while Compositae remains the dominant herbaceous taxa (33.1%). Pollen influx is high overall during this Zone, peaking at 1150B.C.E. (175 × 104 grains cm2 per year), with the exception of lower values at 970B.C.E. (577 × 103 grains cm2 per year) and again between 650 and 550B.C.E. (810–781 × 103 grains cm2 per year) and 340–130B.C.E. (881– 98 × 103 grains cm2 per year). Macroscopic charcoal rises substantially after 1150B.C.E. (1.25 particles cm2 per year) until 50C.E. (24.9 particles cm2 per year). Microscopic charcoal follows a similar trend; however, it begins to increase from the beginning of this Zone (1,163 particles cm2 per year) and peaks at 970B.C.E. (4,004 particles cm2 per year), increasing again from 650 B.C.E. to 50C.E. (911–5,660 particles cm2 per year). Sporormiella continues to be high in abundance and remains stable (0.6–8.8%) but on average is lower (4.4%) than in Zone 1 (7.2%). There are particularly high abundances of Sporormiella from 2100B.C.E. (7.4%) to 1750B.C.E. (8.8%), 930B.C.E (6.5%), and at 550B.C.E. (7.7%).
Zone 3 (254.5–114 cm, 15 samples, 100–1,522C.E.) encompasses c.1400 years and is defined by the arboreal components: Pinus, Quercus, Morella cerifera, and Liquidambar, and herbaceous components: Compositae and Poaceae (Figure 5). This zone is representative of the Terminal Preclassic (150–250C.E.), Classic (250–950C.E.) and Post–Classic Periods (950–1,522C.E.). Quercus and Morella cerifera decline between 200 and 1,070C.E. (30–4.7%) while Pinus increases (21.3–64%). Liquidambar establishes and rises from 1070C.E. (0.7%) through to 1522C.E. (9.3%). Of the remaining MHWF canopy taxa, Alnus increases after 850.C.E. (1.3%) through to 1150C.E. (6.3%) and then again from 1200 to 1,522.E. (0.7–6.3%). The coniferous to MHWF ratio increases between 100 and 1,522C.E. (78:22) in favor of coniferous taxa. Compositae remains in high abundance (22.9%) but decreases after 850C.E. (45.3%) through to 1522C.E. (7.7%). Poaceae increases from 200 to 650C.E. (15.7–28%). Pollen influx decreases from 200C.E. through to 1522C.E. (10 to 20 × 104 grains cm2 per year). Sporormiella abundance increases from 70B.C.E. (0.9%) through to 200C.E. (7.1%) then decreases until the end of this Zone (4.1%). Overall Sporormiella abundance is comparatively lower (3.3%) than in Zone 2 (4.4%). Influx of macroscopic and microscopic charcoal decreases from 200 to 1000C.E. (macroscopic charcoal: 0.44–0.32 particles cm2 per year; and, microscopic charcoal: 552–86 particles cm2 per year).
This palaeoenvironmental sequence represents the vegetation dynamics of the Maya uplands of Guatemala from 4000B.C.E. to 1522C.E. Our data indicate that the natural baseline of this region is best described as OF to MPOF. This deciduous coniferous mosaic of oak dominated forests largely persisted from 4000 to 230B.C.E. after which, the vegetation assemblage deviates away from the natural baseline toward pine dominated forests. Deviation from the natural baseline was attributed to extensive and prolonged anthropogenic settlement and activities surrounding agrarian practices and use of fire for architectural developments (Anderson and Wahl, 2016). While our record suggests that this region has been predominantly forested through time, there is clear evidence for compositional changes in flora as a direct result of anthropogenic activities, particularly between 3700 and 3300B.C.E. and from 1000B.C.E.−1522C.E. (Figure 5). For example, the transition from MPOF in Zone 1 (4000–2200B.C.E.) through to PFs in Zone 3 (100–1522C.E.) is reflected in the taxonomic associations of the PCA and the environmental drivers presented in the CCA (Figure 4).
Archaeological records have widely found that village farming became firmly established across the wider Maya Area after c.1800B.C.E. (Neff et al., 2006). This is reflected in the interpretations of the reconstructed palynological assemblages for both the Maya lowlands and the upland areas (Figure 2). Disturbance driven by anthropogenic activities, such as: (i) agricultural practices (e.g., Dull, 2004a,b, 2007); (ii) rearing livestock (Lovell, 1985); (iii) timber extraction (e.g., Dull, 2004a,b, 2007; Velez et al., 2011); and (iv) lime production (Anderson and Wahl, 2016), can initiate or maintain local vegetation succession impacting: (i) forest composition, (ii) structure, and (iii) regeneration (González-Espinosa et al., 1991).
Archaic populations from the Maya Area combined agriculture (e.g., pepper, beans, maize, squash, and chili) with hunting and gathering from as early as 5200B.C.E. (Pope et al., 2001). Evidence for agriculture from Centote Kail is first indicated by vegetation changes from 4000B.C.E. The observed changes include (i) a decline in canopy and understory taxa; (ii) increases in weedy disturbance taxa; (iii) a very small increase in local and regional burning; (iv) and the presence of cultivated taxa such as Capsicum (White, 1999), between c.3850 and 3300B.C.E. or Zea maysbetween c.970 and 550B.C.E. (Figure 5). A mixture of traditional hunter–gather practices combined with limited agriculture is exemplified by the abundance and variety of edible fruits and nuts (e.g., Brosimum, Myrica, and Anacardiaceae) and high abundance of fauna as indicated by the influx of Sporormiella.
Little is known about the Pre–Columbian human habitation of the upland Maya Areas, particularly during the Archaic, due to a scant archaeological record (MacNeish, 1982; Clark and Cheetham, 2002; Lohse et al., 2006; Lohse, 2009). Evidence from Cenote Kail suggests that people were manipulating the uplands of Guatemala from at least c.4000B.C.E. and practicing agriculture from c.3850B.C.E. This is the earliest palynological evidence for agriculture in the Southern Maya Area, preceding evidence from lowland Pacific Guatemala and from El Salvador, which all indicate agrarian practices established from c.3500B.C.E. (Dull, 2004a; Neff et al., 2006). Based on this evidence, we hypothesize that agriculture in the Southern Maya Area started in the uplands and spread to the lowlands, driven by increasingly favorable climatic conditions in the lowlands during the Holocene Thermal Maximum (6000–3000BC.E.) (Ford and Nigh, 2009). Human populations dispersed with the expansion of the lowland forests (Rosenmeier et al., 2002; Hillesheim et al., 2005; Neff et al., 2006; Wahl et al., 2006, 2014; Bush et al., 2009; Mueller et al., 2009; Escobar et al., 2012), increasingly interacting with the tropical forest ecosystem and gaining ethnobotanical knowledge (Ford and Nigh, 2009).
The start of the Pre–Classic (2000B.C.E.) is marked by the first appearance of state level settlements and an increased reliance on domesticated crops, particularly Zea mays (Neff et al., 2006). Settlements and agriculture were concentrated around water bodies, such as Cenotes, which provided reliable access to fresh water for sustenance and agriculture (Lucero et al., 2014). Although pollen records have been used to document the spread of maize agriculture in the Maya Area, most of these records are from the lowlands (Figure 1). Consequently, our understanding of the initial arrival of maize in the uplands of Central America is poor. Results from Cenote Kail are filling this gap. For example, traditional Zea mays agriculture is evident from 970B.C.E. in the Cenote Kail record. This is late compared to the uplands of El Salvador, c.2500B.C.E. (Dull, 2004a); however, the relative palynological abundance of Zea mays found in Cenote Kail, suggests that the expanse of agriculture in the Guatemala highlands was much greater. Zea mays agriculture is typically associated with Milpa (e.g., Dull, 2004a,b, 2007), which entails a 5–10 year cycle between periods of cultivation and fallow (Cowgill, 1962). Intensification of milpa cycling due to increasing human populations commonly leads to the depletion of nutrients in the soil (Ford and Nigh, 2009). We suggest that after 550B.C.E the agricultural settlement surrounding Cenote Kail was abandoned in favor of more productive soils in the lowlands.
By c.350B.C.E. large pyramids were being built across the Maya Area including the establishment of Chinkultic c.50B.C.E. (Ball, 1980). These pyramids were typically covered in plaster for architectural as well as decorative purposes (Anderson and Wahl, 2016). The production of this lime plaster involved the burning of powdered limestone (Oates, 2008). Monuments built during the Pre–Classic period were covered in this plaster (Hansen, 2001, 2012; Anderson and Wahl, 2016). Hansen (2012) reports that floor thickness alone could exceed 13cm. Anderson and Wahl (2016) explore the amount of fuel required to produce sufficient lime to meet the demands of this monument building and the impact that this might have had on the forest environment. They calculate 192 km2 of forest would have been required for burning to create enough plaster for the construction of El Mirador in the central Maya Area. While Chinkultic is significantly smaller than El Mirador, the required plaster to create the complex of temples and ball courts would have been extensive. Agriculture in the Guatemala highlands culminated c.400 years before the Chinkultic settlement was established (Ball, 1980). Therefore, we suggest that the large increase of macroscopic and microscopic charcoal from c.200B.C.E. in Cenote Kail might relate to the production of lime plaster at Chinkultic, coinciding with its founding. The extraction of wood from the surrounding forests for burning is reflected in the structure of these POF as well as patterns for local and regional burning.
Relatively equal abundances of canopy, understory, and herbaceous taxa from 4000 to 3700B.C.E. indicate a diverse and stratified forest structure comprising at least three vegetative levels. The high diversity of taxa, particularly in the understory (e.g., Leguminosae, Anacardiaceae, Myrica, and Rubiaceae), suggests a relatively low and open canopy allowing light to penetrate to the forest floor (Bush, 2000). This structure is typical of middle succession in mixed POF after a large clearance event (Peterson and Reich, 2001).
Weedy disturbance taxa (e.g., Amaranthaceae), reductions in canopy taxa (e.g., Quercus) and the creation of more open habitats exemplify anthropogenic disturbance prior to the agrarian establishment of Zea mays (e.g., Dull, 2004a,b, 2007). Anthropogenic impacts, as indicated by agricultural grains and reductions in forest taxa in Zone 1 occur at Cenote Kail between c.4000 and 3300B.C.E. Disturbance to the natural vegetation baseline was likely caused by settlement and agrarian practices directly surrounding Cenote Kail. After 3300B.C.E. cultivation ceases and arboreal taxa (understory and canopy) re–establish within c.100 years (3200–3100B.C.E.). This follows the expected recovery time of c.80 years for POF (Kappelle, 2006; Figueroa-Rangel et al., 2008). By 2600B.C.E. the mature structure of the POF are well-established and persist in relative equilibrium until further anthropogenic disturbance at 1150C.E. Deforestation, agrarian cultivar, and increased regional burning surrounding Cenote Kail all coincide at c.1000B.C.E. (Figure 5), and are attributed to the expansion and development of the Pre–Classic Maya (e.g., Neff et al., 2006). Increases in local and regional burning from the onset of Pre–Classic agriculture marks the sustained decline and eventual transition of POF to Pine dominated forests.
Plant community composition after burning is often explained by: (i) the sprouting ability of dominant species, (ii) the ability of subdominant species to increase in numbers, and (iii) the failure of invasive species to become established (Elliott et al., 1999, McDonald et al., 2003). Many species of oak rapidly sprout from their root collar after burning (e.g., Quercus insignis, Q. skinneri), dominating early successional stands (Barnes and Van Lear, 1998; Kirby and Watkins, 2015). However, several species of pine have also adapted to fire through the development of thick bark, serotiny, rapid growth, and sprouting, including Pinus teocote and P. pseudostrobus (Richardson, 2000; Rodríguez-Trejo and Fulé, 2003). The early successional formation of oak dominance is evident between 970 and 550B.C.E. (Figure 5). As the forest becomes more established, oak abundance typically diminishes under faster-growing conifers (Sheffer, 2012).
Extraction of wood, sustained land clearance, and most importantly burning has changed the structure of the POF from oak-dominated to pine-dominated (Figure 5). Additionally, herbivores, such as the white–tailed deer (Odocoileus virginianus) may also have impacted forest composition and regeneration through selective browsing on young saplings and individual species (Vera, 2000; Kirby and Watkins, 2015). We suggest that the coppice systems created by the Maya are particularly vulnerable to browsing from medium and large sized herbivores (Joys et al., 2004). Pines have been found to be preferentially browsed compared with other arboreal taxa (Blair and Brunett, 1980). While overall herbivore abundance decreases through time, their browsing impacts upon forest structure may have been particularly important to recovery after prolonged disturbance (Joys et al., 2004).
The pine-dominated forests become increasingly established between 150B.C.E. and 1070C.E. (Figure 5). When coniferous forests form closed stands, they change the environment beneath them. The most substantial changes involve the greater uptake of soil water and decrease of light reaching the ground (Jucker et al., 2014). The combination of these factors makes it almost impossible for other arboreal taxa to establish and grow (Kappelle, 2006). For example, most species of oak suffer increased reproductive failure under closed canopy conditions (Jucker et al., 2014), relying on the dispersal of acorns to forest edges or clearings through animal transport (Lopez-Barrera, 2003; Kappelle, 2006). Large herbivores, such as the Baird tapir (Tapirus bairdii), are important to the structure and diversity of recovering POF due to their role as long distance seed dispersers, ingesting whole seeds and dropping them intact with their feces (Bodmer, 1991; Rodrigues et al., 1993; Fragoso, 1997; Olmos, 1997; Lawton, 2000). The reduction of herbivore abundance during the transition of oak dominated POF to pine dominated POF at Cenote Kail (c.220B.C.E.) is likely to have contributed to the established rise in pine. Coniferous forest stands remain dominant until they are removed through felling or die of disease, insect attack or old age (Jones, 1974). The transition from pine dominated coniferous forests to MHWF is gradual and relies upon the breakup of the coniferous forest canopy to allow for secondary canopy taxa to rise through (Jones, 1974). The establishment of Quercus, Liquidambar and Alnus at Cenote Kail after c.850C.E. demonstrates this final transition back to MHWF dominance (Figure 5).
Results from this study indicate that fire has been the most important driver of vegetative change in this ecosystem throughout the last c.6000 years, particularly during the Pre–Classic and Classic periods (2000B.C.E.−950C.E.). Fire driven change from MHWF (oak–dominated) to coniferous forests (pine–dominated) has previously been attributed to climate driven aridity (e.g. Figueroa-Rangel et al., 2008, 2010, 2012); however, our study suggests that anthropogenic activity is the most likely source of burning and overall vegetative change. The predominantly anthropogenic signal for burning represented in Cenote Kail is inferred through the combined evidence of: (i) agricultural practices, (ii) reduction of MHWF taxa, and (iii) rapid increase of burning coinciding with the establishment and expansion of nearby Maya temples and settlements (e.g., Chinkultic).
Terrestrial hydroclimatic reconstructions from the upland Maya Area suggest that only modest changes in precipitation amounts occurred over the last several millennia. Climatic evidence from Lago Amatitlan indicates lower lake levels from 250B.C.E. to 125C.E. and 875 to 1375C.E., which has been attributed to a decline in water level resulting from either a drier climate and/or reforestation after anthropogenic abandonment (Velez et al., 2011). Also, evidence from San Lorenzo (Chiapas) indicates generally wetter conditions from c.1400–700B.C.E., 500–850B.C.E., and c.1200–1522C.E. interrupted by periods of drought from c.700–500B.C.E. and c.850–1200C.E. (Franco-Gaviria et al., 2018). These records suggest that although climate may have played an abetting role in driving forest dynamics, the timing of these hydroclimatic changes do not reflect the forest and fire dynamics reconstructed from Cenote Kail. Our findings suggest that anthropogenic activities revolving around agriculture and architectural developments have initiated and maintained successive regeneration of vegetation from mixed oak dominated forests to pine dominated forests. However, further work investigating past hydroclimate changes for this region will be required to fully understand the role of climate as an independent driver of the vegetation surrounding Cenote Kail.
The sedimentary sequence from Cenote Kail was continuously deposited from 4000B.C.E. to 1522C.E. and provides a record of past changes in vegetation and human impacts. We have found that POF within the Southern Maya area were transformed by Pre–Columbian human populations through practices of agriculture and architectural developments over thousands of years extending back into the Archaic Period (Betz, 1997; Piperno and Pearsall, 1998; Smith, 1998; Dull, 2004a; Neff et al., 2006). Three successional phases can be discerned following a combination of natural and anthropogenically modified successional pathways. The Archaic period is defined by light anthropogenic disturbance, centered around some land clearance for agriculture. Zea mays cultivation is prevalent from 970 to 550B.C.E., after which time sedentary agriculture does not appear to be widely practiced. Herbivorous animals, such as deer and tapir, are likely to have played an important role in forest recovery after disturbance; however, discerning their individual impacts would require further research. Persistent high intensity burning for lime production during the Late–Pre-Classic to Classic Period are suggested to have resulted in a turnover of forest structure c.150B.C.E. from oak-dominated POF to pine-dominated POF. Evidence for the fragmentation, degradation and subsequent recovery of these MTF over the past c.6000 years provides a valuable comparison for the present–day anthropogenic activities that are driving current changes in this region. To protect the remaining intact fragments of these MTF and to encourage the recovery of areas that have suffered past compositional or structural shifts, fire needs to be carefully managed.
WH conceived the presented idea with input from SN, KW, and GP. WH conducted all palaeoecological lab work, statistical analysis, and age-depth modeling. SN and KW verified the analytical methods. NS and BS conducted the field work. NS carried out the radiocarbon dating. KW, SN, NS, and GP helped supervise the project. WH drafted the final manuscript. All authors discussed the results contributing to the final manuscript.
The authors declare that the research was conducted in the absence of any commercial or financial relationships that could be construed as a potential conflict of interest.
The authors would like to thank both reviews and the editor for their thoughtful, constructive, and insightful comments. This research was funded by the Natural Environment Research Council of the United Kingdom NE/L002612/1, US National Science Foundation (EAR−1502989) and St. Edmund Hall, University of Oxford. Gillian Petrokofsky is funded by a European Commission LIFE+ grant.
The Supplementary Material for this article can be found online at: https://www.frontiersin.org/articles/10.3389/ffgc.2019.00034/full#supplementary-material
Supplementary Table 1. Sites represented on Figures 1 and 2.
Supplementary Table 2. Images of palynological taxa at 400x magnification identified from Cenote Kail.
Data Sheet 1. Raw Palaeoecological Data.
Abbott, M. B., and Stafford, T. W. (1996). Radiocarbon geochemistry of modern and ancient Arctic lake systems, Baffin Island, Canada. Quaternary Res. 45, 300–311. doi: 10.1006/qres.1996.0031
Anderson, L., and Wahl, D. (2016). Two Holocene paleofire records from Peten, Guatemala: implications for natural fire regime and prehispanic Maya land use. Glob. Planet. Change 138, 82–92. doi: 10.1016/j.gloplacha.2015.09.012
APSA (2007). The Australasian Pollen and Spore Atlas V1.0. Canberra, ACT: Australian National University. Available online at: http://apsa.anu.edu.au/ (accessed June 13, 2018).
Arroyo-Rodríguez, V., Melo, F. P., Martínez-Ramos, M., Bongers, F., Chazdon, R. L., Meave, J. A., et al. (2017). Multiple successional pathways in human-modified tropical landscapes: new insights from forest succession, forest fragmentation and landscape ecology research. Biol. Rev. 92, 326–340. doi: 10.1111/brv.12231
Baker, A. G., Cornelissen, P., Bhagwat, S. A., Vera, F. W., and Willis, K. J. (2016). Quantification of population sizes of large herbivores and their long-term functional role in ecosystems using dung fungal spores. Methods Ecol. Evolu. 7, 1273–1281. doi: 10.1111/2041-210X.12580
Ball, J. W. (1980). The Archaeological Ceramics of Chinkultic, Chiapas, Mexico. Provo: New World Archaeological Foundation, 43.
Barnes, T. A., and Van Lear, D. H. (1998). Prescribed fire effects on advanced regeneration in mixed hardwood stands. South. J. Appl. Forest. 22, 138–142.
Bennett, K. D. (1994). Confidence intervals for age estimates and deposition times in late–Quaternary sediment sequences. Holocene 4, 337–348. doi: 10.1177/095968369400400401
Bennett, K. D. (1996). Determination of the number of zones in a biostratigraphical sequence. N. Phytol. 132, 155–170. doi: 10.1111/j.1469-8137.1996.tb04521.x
Bennett, K. D., and Willis, K. J. (2001). “Pollen,” in Tracking Environmental Change Using Lake Sediements. Volume 3: Terrestrial, Algal, and Siliceous Indicators, eds J. P. Smol, H. J. Birks, and W. M. Last (Springer), 5–32.
Blair, R. M., and Brunett, L. E. (1980). Seasonal browse selection by deer in a southern pine–hardwood habitat. J. Wildl. Manage. 44, 79–88. doi: 10.2307/3808353
Bodmer, R. E. (1991). Strategies of seed dispersal and seed predation in Amazonian ungulates. Biotropica 23, 255–261. doi: 10.2307/2388202
Bowman, D. M., Balch, J., Artaxo, P., Bond, W. J., Cochrane, M. A., D'antonio, C. M., et al. (2011). The human dimension of fire regimes on Earth. J. Biogeogr. 38, 2223–2236. doi: 10.1111/j.1365-2699.2011.02595.x
Breedlove, D. E. (1981). Flora of Chiapas, Part 1: Introduction to the Flora of Chiapas. San Francisco, CA: The California Academy of Sciences.
Bush, M. B. (2000). Deriving response matrices from Central American modern pollen rain. Quaternary Res. 54, 132–143. doi: 10.1006/qres.2000.2138
Bush, M. B., Correa-Metrio, A. Y., Hodell, D. A., Brenner, M., Anselmetti, F. S., Ariztegui, D., et al. (2009). “Re-evaluation of climate change in lowland Central America during the Last Glacial Maximum using new sediment cores from Lake Petén Itzá, Guatemala,” in Past Climate Variability in South America and Surrounding Regions, Developments in Paleoenvironmental Research, eds F. Vimeux, F. Sylvestre, and M. Khodri (Dordrecht: Springer), 113–128.
Bush, M. B., Piperno, D. R., Colinvaux, P. A., De Oliveira, P. E., Krissek, L. A., Miller, M. C., et al. (1992). A 14 300-yr paleoecological profile of a lowland tropical lake in Panama. Ecol. Monogr. 62, 251–275.
Bush, M. B., and Weng, C. (2007). Introducing a new (freeware) tool for palynology. J. Biogeogr. 34, 377–380. doi: 10.1111/j.1365-2699.2006.01645.x
Caffrey, M. A., Taylor, M. J., and Sullivan, D. G. (2011). A 12,000–year record of vegetation and climate change from the Sierra de Los Cuchumatanes, Guatemala. J. Latin Am. Geogr. 10, 129–151. doi: 10.1353/lag.2011.0041
Clark, J. E., and Cheetham, D. (2002). “Mesoamerica's tribal foundations,” in The Archaeology of Tribal Societies, ed W. A. Parkinson (International Monographs in Prehistory), 278–339. doi: 10.2307/j.ctv8bt29z.18
Clark, J. S. (1988). Particle motion and the theory of charcoal analysis: source area, transport, deposition, and sampling. Quaternary Res. 30, 67–80. doi: 10.1016/0033-5894(88)90088-9
Clark, R. L. (1982). Point count estimation of charcoal in pollen preparations and thin sections of sediments. Pollen et Spores. 24, 523–535.
Cochrane, M. A., and Barber, C. P. (2009). Climate change, human land use and future fires in the Amazon. Glob. Chang. Biol. 15, 601–612. doi: 10.1111/j.1365-2486.2008.01786.x
Colunga–GarcíaMarín, P., and Zizumbo–Villarreal, D. (2004). Domestication of plants in Maya LowlandsDomesticacion de plantas en las tierras bajas Mayas. Econ. Bot. 58, 101–S110. doi: 10.1663/0013-0001(2004)58[S101:DOPIML]2.0.CO;2
Corrales, L., Bouroncle, C., and Zamora, J. C. (2015). “An overview of forest biomes and ecoregions of Central America,” in Climate Change Impacts on Tropical Forests in Central America: An Ecosystem Service Perspective, ed Chiabai (Routledge), 33–54.
Cowgill, U. M. (1962). An agricultural study of the southern Maya lowlands. Am. Anthropol. 64, 273–286. doi: 10.1525/aa.1962.64.2.02a00030
Davis, O. K., and Shafer, D. S. (2006). Sporormiella fungal spores, a palynological means of detecting herbivore density. Palaeogeogr. Palaeoclimatol. Palaeoecol. 237, 40–50. doi: 10.1016/j.palaeo.2005.11.028
Dinerstein, E., Olson, D., Joshi, A., Vynne, C., Burgess, N. D., Wikramanayake, E., et al. (2017). An ecoregion–based approach to protecting half the terrestrial realm. BioScience 67, 534–545. doi: 10.1093/biosci/bix014
Domínguez-Vázquez, G., and Islebe, G. A. (2008). Protracted drought during the late Holocene in the Lacandon rain forest, Mexico. Veg. History Archaeobotany 17, 327–333.
Dull, R. A. (2004a). An 8000–year record of vegetation, climate, and human disturbance from the Sierra de Apaneca, El Salvador. Quaternary Res. 61, 159–167. doi: 10.1016/j.yqres.2004.01.002
Dull, R. A. (2004b). A Holocene record of Neotropical savanna dynamics from El Salvador. J. Paleolimnol., 32, 219–231. doi: 10.1023/B:JOPL.0000042906.46791.9c
Dull, R. A. (2007). Evidence for forest clearance, agriculture, and human-induced erosion in precolumbian el salvador. Ann. Assoc. Am. Geogr. 97, 127–141. doi: 10.1111/j.1467-8306.2007.00527.x
Elliott, K. J., Hendrick, R. L., Major, A. E., Vose, J. M., and Swank, W. T. (1999). Vegetation dynamics after a prescribed fire in the southern Appalachians. For. Ecol. Manage. 114, 199–213. doi: 10.1016/S0378-1127(98)00351-X
Escobar, J., Hodell, D. A., Brenner, M., Curtis, J. H., Gilli, A., Mueller, A. D., et al. (2012). A~ 43–ka record of paleoenvironmental change in the Central American lowlands inferred from stable isotopes of lacustrine ostracods. Quat. Sci. Rev., 37, 92–104. doi: 10.1016/j.quascirev.2012.01.020
Figueroa-Rangel, B. L., Willis, K. J., and Olvera-Vargas, M. (2008). 4200 years of pine-dominated upland forest dynamics in west-central Mexico: human or natural legacy. Ecology 89, 1893–1907. doi: 10.1890/07-0830.1
Figueroa-Rangel, B. L., Willis, K. J., and Olvera-Vargas, M. (2010). Cloud forest dynamics in the Mexican neotropics during the last 1300 years. Glob. Chang. Biol. 16, 1689–1704. doi: 10.1111/j.1365-2486.2009.02024.x
Figueroa-Rangel, B. L., Willis, K. J., and Olvera-Vargas, M. (2012). Late–Holocene successional dynamics in a transitional forest of west–central Mexico. Holocene 22, 143–153. doi: 10.1177/0959683611414929
Ford, A., and Nigh, R. (2009). Origins of the Maya forest garden: Maya resource management. J. Ethnobiol. 29, 213–236. doi: 10.2993/0278-0771-29.2.213
Fragoso, J. M. (1997). Tapir-generated seed shadows: scale-dependent patchiness in the Amazon rain forest. J. Ecol. 519–529.
Franco-Gaviria, F., Correa-Metrio, A., Cordero-Oviedo, C., López-Pérez, M., Cárdenes-Sand,í, G. M., and Romero, F. M. (2018). Effects of late Holocene climate variability and anthropogenic stressors on the vegetation of the Maya highlands. Quat. Sci. Rev. 189, 76–90. doi: 10.1016/j.quascirev.2018.04.004
Gavin, D. G., Brubaker, L. B., and Lertzman, K. P. (2003). An 1800–year record of the spatial and temporal distribution of fire from the west coast of Vancouver Island, Canada. Can. J. For. Res. 33, 573–586. doi: 10.1139/x02-196
González-Espinosa, M., Quintana-Ascencio, P. F., Ramírez-Marcial, N., and Gaytán-Guzmán, P. (1991). Secondary succession in disturbed Pinus-Quercus forests in the highlands of Chiapas, Mexico. J. Veg. Sci. 2, 351–360. doi: 10.2307/3235927
Hansen, R. D. (2001). “The first cities: the beginnings of urbanization and state formation in the Maya Lowlands,” in Maya: Divine Kings of the Rain Forest, ed N. Grube (Verlag: Konemann Press), 50–65.
Hansen, R. D. (2012). “The beginning of the end: conspicuous consumption and environmental impact of the Preclassic lowland Maya,” in An Archaeological Legacy: Essays in Honor of Ray T. Matheny, eds D. G. Matheny, J. C. Janetski, and G. Nielson (Provo: Brigham Young University), 243–291.
Higuera, P. E., Gavin, D. G., Bartlein, P. J., and Hallett, D. J. (2011). Peak detection in sediment–charcoal records: impacts of alternative data analysis methods on fire–history interpretations. Int. J. Wildland Fire 19, 996–1014. doi: 10.1071/WF09134
Higuera, P. E., Peters, M. E., Brubaker, L. B., and Gavin, D. G. (2007). Understanding the origin and analysis of sediment–charcoal records with a simulation model. Quaternary Sci. Rev. 26, 1790–1809. doi: 10.1016/j.quascirev.2007.03.010
Hillesheim, M. B., Hodell, D. A., Leyden, B. W., Brenner, M., Curtis, J. H., Anselmetti, F. S., et al. (2005). Climate change in lowland Central America during the late deglacial and early Holocene. J. Quaternary Sci. 20, 363–376. doi: 10.1002/jqs.924
Islebe, G. A., and Hooghiemstra, H. (1997). Vegetation and climate history of montane Costa Rica since the last glacial. Quat. Sci. Rev. 16, 589–604. doi: 10.1016/S0277-3791(96)00051-0
Islebe, G. A., Hooghiemstra, H., Brenner, M., and Curtis, J. H. (1996). A Holocene vegetation history from lowland Guatemala. Holocene 6, 265–271. doi: 10.1177/095968369600600302
Jeffers, E. S., Nogué, S., and Willis, K. J. (2015). The role of palaeoecological records in assessing ecosystem services. Quat. Sci. Rev. 112, 17–32. doi: 10.1016/j.quascirev.2014.12.018
Jones, J. R. (1974). “Silviculture of southwestern mixed conifers and aspen: the status of our knowledge,” in Research Paper RM−122. Fort Collins, CO: US Department of Agriculture, Forest Service, Rocky Mountain Forest and Range Experiment Station. 44 p, 122. doi: 10.5962/bhl.title.98880
Joys, A. C., Fuller, R. J., and Dolman, P. M. (2004). Influences of deer browsing, coppice history, and standard trees on the growth and development of vegetation structure in coppiced woods in lowland England. Forest Ecol. Manage. 202, 23–37. doi: 10.1016/j.foreco.2004.06.035
Jucker, T., Bouriaud, O., Avacaritei, D., Dănilă, I., Duduman, G., Valladares, F., et al. (2014). Competition for light and water play contrasting roles in driving diversity–productivity relationships in Iberian forests. J. Ecol. 102, 1202–1213. doi: 10.1111/1365-2745.12276
Juggins, S. (2009). Package ‘Rioja’. Available online at: https://cran.r-project.org/web/packages/rioja/index.html
Kappelle, M. (2006). “Neotropical montane oak forests: overview and outlook,” in Ecology and Conservation of Neotropical Montane Oak Forests (Berlin; Heidelberg: Springer), 449–467. doi: 10.1007/3-540-28909-7_34
Kirby, K., and Watkins, C. (2015). Europe's Changing Woods and Forests: from Wildwood to Managed Landscapes. Wallingford, UK: CABI. doi: 10.1079/9781780643373.0000
Lawton, R. O. (2000). Baird's Tapir. Monteverde: Ecology and Conservation of a Tropical Cloud Forest. New York, NY: Oxford University Press.
Livingstone, D. A. (1955). A lightweight piston sampler for lake deposits. Ecology 36, 137–139. doi: 10.2307/1931439
Lohse, J. C. (2009). “The archaic to preclassic transition in Belize: what we know and why we don't know more. research reports in Belizean archaeology,” in Archaeological Investigations in the Eastern Maya Lowlands: Papers of the 2008 Belize Archaeology Symposium, eds J. Morris, S. Jones, J. Awe, G. Thompson, and C. Helmke, 141–150.
Lohse, J. C., Awe, J., Griffith, C., Rosenswig, R., and Valdez, F. (2006). Preceramic occupations in belize: updating the paleoindian and archaic record. Latin Am. Antiquity 17, 209–226. doi: 10.2307/25063047
Lopez-Barrera, F. (2003). Edge Effects in a Forest Mosaic: Implications for Oak Regeneration in the Highlands of Chiapas, Mexico (Ph.D. Thesis). University of Edinburgh, Edinburgh.
Lovell, W. G. (1985). Conquest and Survival in Colonial Guatemala, A Historical Geography of the Cuchumatán Highlands, 1500–1821. McGill-Queen's University Press.
Lucero, L. J., Fedick, S. L., Dunning, N. P., Lentz, D. L., and Scarborough, V. L. (2014). 3 water and landscape: ancient maya settlement decisions. Archeol. Papers Am. Anthropol. Assoc. 24, 30–42. doi: 10.1111/apaa.12027
Lynch, J. A., Clark, J. S., and Stocks, B. J. (2004). Charcoal production, dispersal, and deposition from the Fort Providence experimental fire: interpreting fire regimes from charcoal records in boreal forests. Can. J. For. Res. 34, 1642–1656. doi: 10.1139/x04-071
MacNeish, R. S. (1982). Third Annual Report of the Belize Archaic Archaeological Reconnaissance. Robert, S. Peabody Foundation for Archaeology, Andover.
Maher, L. J. (1981). Statistics for microfossil concentration measurements employing samples spiked with marker grains. Rev. Palaeobot. Palynol. 32, 153–191. doi: 10.1016/0034-6667(81)90002-6
Martin, A. C., and Harvey, W. J. (2017). The Global Pollen Project: a new tool for pollen identification and the dissemination of physical reference collections. Methods Ecol. Evolu. 8, 892–897. doi: 10.1111/2041-210X.12752
Martínez, M. L., Pérez-Maqueo, O., Vázquez, G., Castillo-Campos, G., García-Franco, J., Mehltreter, K., et al. (2009). Effects of land use change on biodiversity and ecosystem services in tropical montane cloud forests of Mexico. For. Ecol. Manage. 258, 1856–1863. doi: 10.1016/j.foreco.2009.02.023
McDonald, R. I., Peet, R. K., and Urban, D. L. (2003). Spatial pattern of Quercus regeneration limitation and Acer rubrum invasion in a Piedmont forest. J. Veg. Sci. 14, 441–450. doi: 10.1111/j.1654-1103.2003.tb02170.x
Metcalfe, S. E., Barron, J. A., and Davies, S. J. (2015). The Holocene history of the North American Monsoon:‘known knowns' and ‘known unknowns' in understanding its spatial and temporal complexity. Quat. Sci. Rev. 120, 1–27. doi: 10.1016/j.quascirev.2015.04.004
Miranda, F. (1952). La Vegetación de Chiapas, Primera Parte. Ediciones Del Gobierno Del Estado Seccion Autografica Departmento De Prensa Y Turismo.
Mueller, A. D., Islebe, G. A., Hillesheim, M. B., Grzesik, D. A., Anselmetti, F. S., Ariztegui, D., et al. (2009). Climate drying and associated forest decline in the lowlands of northern Guatemala during the late Holocene. Quaternary Res. 71, 133–141. doi: 10.1016/j.yqres.2008.10.002
Muller, C. H. (1942). The Central American Species of Quercus(No. 477). US Government Printing Office. doi: 10.5962/bhl.title.65496
Murdoch, D. J., and Chow, E. D. (1996). A graphical display of large correlation matrices. Am. Statistic. 50, 178–180.
Neff, H., Pearsall, D. M., Jones, J. G., Arroyo, B., Collins, S. K., and Freidel, D. E. (2006). Early Maya adaptive patterns: Mid–late Holocene paleoenvironmental evidence from Pacific Guatemala. Latin Am. Antiquity 17, 287–315. doi: 10.2307/25063054
Oates, J. A. (2008). Lime and Limestone: Chemistry and Technology, Production and Uses. New York, NY: John Wiley & Sons.
Oksanen, J., Blanchet, F. G., Kindt, R., Legendre, P., Minchin, P. R., O'hara, R. B., et al. (2015). Package ‘Vegan.’
Olmos, F. A. (1997). “Tapirs as seed dispersers and predators,” in Tapirs-Status Survey and Conservation Action Plan, eds D. M. Brooks, R. E. Bodmer, and S. Matola (Gland and Cambridge, UK: Tapir Specialist Group IUCN/SSC, IUCN), 164.
OxLEL (2016). Pollen Preparation Procedure. Oxford Long–Term Ecology Lab. Protocols. Available online at: https://oxlel.zoo.ox.ac.uk/wp – content/uploads/2016/10/OxLEL– Fossil – pollen – extraction – protocol.pdf (accessed June 13, 2018).
Peters, M. E., and Higuera, P. E. (2007). Quantifying the source area of macroscopic charcoal with a particle dispersal model. Quaternary Res. 67, 304–310. doi: 10.1016/j.yqres.2006.10.004
Peterson, D. W., and Reich, P. B. (2001). Prescribed fire in oak savanna: fire frequency effects on stand structure and dynamics. Ecol. Appl. 11, 914–927. doi: 10.1890/1051-0761(2001)011[0914:PFIOSF]2.0.CO;2
Piperno, D. R., and Pearsall, D. M. (1998). The Origins of Agriculture in the Lowland Neotropics. San Diego, CA: Academic Press.
Pope, K. O., Pohl, M. E., Jones, J. G., Lentz, D. L., Von Nagy, C., Vega, F. J., et al. (2001). Origin and environmental setting of ancient agriculture in the lowlands of Mesoamerica. Science 292, 1370–1373.
Potapov, P., Hansen, M. C., Laestadius, L., Turubanova, S., Yaroshenko, A., Thies, C., et al. (2017). The last frontiers of wilderness: tracking loss of intact forest landscapes from 2000 to 2013. Sci. Adv. 3:e1600821. doi: 10.1126/sciadv.1600821
Ramsey, C. B. (2008). Deposition models for chronological records. Quaternary Sci. Rev. 27, 42–60. doi: 10.1016/j.quascirev.2007.01.019
Ramsey, C. B. (2009). Bayesian analysis of radiocarbon dates. Radiocarbon 51, 337–360. doi: 10.1017/S0033822200033865
R. Core Team (2012). R: A Language and Environment for Statistical Computing. Vienna: R Foundation for Statistical Computing.
Reimer, P. J., Bard, E., Bayliss, A., Beck, J. W., Blackwell, P. G., Ramsey, C. B., et al. (2013). IntCal13 and Marine13 radiocarbon age calibration curves 0–50,000 years cal BP. Radiocarbon 55, 1869–1887. doi: 10.2458/azu_js_rc.55.16947
Richardson, D. M. (ed.). (2000). Ecology and Biogeography of Pinus. Cambridge: Cambridge University Press.
Rodrigues, M., Olmos, F., and Galetti, M. (1993). Seed dispersal by tapir in southeastern Brazil. Mammalia 57, 460–461.
Rodríguez-Trejo, D. A., and Fulé, P. Z. (2003). Fire ecology of Mexican pines and a fire management proposal. Int. J. Wildland Fire 12, 23–37. doi: 10.1071/WF02040
Rosenmeier, M. F., Hodell, D. A., Brenner, M., and Curtis, J. H. (2002). A 4000–year lacustrine record of environmental change in the Southern Maya Lowlands, Peten, Guatemala. Quat. Res. 57, 183–190. doi: 10.1006/qres.2001.2305
Roubik, D. W., and Moreno, P. (1991). Pollen and spores of Barro Colorado Island [Panama]. 36:268. doi: 10.2307/4110734
Rzedowski, J. (2006). Vegetación de México. 1ra. Edición Digital, Comisión Nacional para el Conocimiento y Uso de la Biodiversidad, México, 504 p.
Sheffer, E. (2012). A review of the development of Mediterranean pine–oak ecosystems after land abandonment and afforestation: are they novel ecosystems? Ann. For. Sci., 69, 429–443. doi: 10.1007/s13595-011-0181-0
Smith, B. D. (1998). Between foraging and farming. Science 279, 1651–1652. doi: 10.1126/science.279.5357.1651
Ter Braak, C. J., and Prentice, I. C. (1988). A theory of gradient analysis. Adv. Ecol. Res. 18, 271–317. doi: 10.1016/S0065-2504(08)60183-X
Turner, B. L., and Miksicek, C. H. (1984). Economic plant species associated with prehistoric agriculture in the Maya lowlands. Econ. Bot. 38, 179–193. doi: 10.1007/BF02858831
Velez, M. I., Curtis, J. H., Brenner, M., Escobar, J., Leyden, B. W., and Popenoe de Hatch, M. (2011). Environmental and cultural changes in highland Guatemala inferred from Lake Amatitlán sediments. Geoarchaeology 26, 346–364. doi: 10.1002/gea.20352
Vera, F. W. M. (2000). Grazing Ecology and Forest History. Wallingford, UK: CABI publishing. doi: 10.1079/9780851994420.0000
Wahl, D., Byrne, R., and Anderson, L. (2014). An 8700 year paleoclimate reconstruction from the southern Maya lowlands. Quat. Sci. Rev. 103, 19–25. doi: 10.1016/j.quascirev.2014.08.004
Wahl, D., Byrne, R., Schreiner, T., and Hansen, R. (2006). Holocene vegetation change in the northern Peten and its implications for Maya prehistory. Quaternary Res. 65, 380–389. doi: 10.1016/j.yqres.2005.10.004
Watson, J. E., Evans, T., Venter, O., Williams, B., Tulloch, A., Stewart, C., et al. (2018). The exceptional value of intact forest ecosystems. Nat. Ecol. Evolu. 2, 599–610. doi: 10.1038/s41559-018-0490-x
Whitlock, C., and Larsen, C. (2002). “Charcoal as a fire proxy,” in Tracking Environmental Change Using Lake Sediments, Developments in Paleoenvironmental Research, Vol 3. eds J. P. Smol, H. J. B. Birks, W. M. Last, R. S. Bradley, and K. Alverson (Dordrecht: Springer), 75–97.
Keywords: fire, pine-oak forest, Zea mais, early agriculture, Capsicum, Sporormiella, palaeoecocology, Maya
Citation: Harvey WJ, Nogué S, Stansell N, Petrokofsky G, Steinman B and Willis KJ (2019) The Legacy of Pre–Columbian Fire on the Pine–Oak Forests of Upland Guatemala. Front. For. Glob. Change 2:34. doi: 10.3389/ffgc.2019.00034
Received: 13 December 2018; Accepted: 10 June 2019;
Published: 02 July 2019.
Edited by:
Tom Evans, Wildlife Conservation Society, United StatesReviewed by:
Tammo Reichgelt, Lamont Doherty Earth Observatory (LDEO), United StatesCopyright © 2019 Harvey, Nogué, Stansell, Petrokofsky, Steinman and Willis. This is an open-access article distributed under the terms of the Creative Commons Attribution License (CC BY). The use, distribution or reproduction in other forums is permitted, provided the original author(s) and the copyright owner(s) are credited and that the original publication in this journal is cited, in accordance with accepted academic practice. No use, distribution or reproduction is permitted which does not comply with these terms.
*Correspondence: William J. Harvey, d2lsbGlhbS5oYXJ2ZXlAc2VoLm94LmFjLnVr
Disclaimer: All claims expressed in this article are solely those of the authors and do not necessarily represent those of their affiliated organizations, or those of the publisher, the editors and the reviewers. Any product that may be evaluated in this article or claim that may be made by its manufacturer is not guaranteed or endorsed by the publisher.
Research integrity at Frontiers
Learn more about the work of our research integrity team to safeguard the quality of each article we publish.