- 1Department of Forestry and Environmental Resources, North Carolina State University, Raleigh, NC, United States
- 2Department of Ecosystem Science and Management, Texas A&M University, College Station, TX, United States
Loss of coastal wetlands is occurring at an increasingly rapid rate due to drainage of these wetlands for alternative land-uses, which also threatens carbon (C) storage in these C-rich ecosystems. Wetland drainage results in water table drawdown and increased peat aeration, which enhances decomposition of previously stabilized peat and changes stable C isotope profiles. The effect of water table drawdown on the pool size and δ13C signature of plant C, soil organic C (SOC), and microbial biomass C (MBC) across a range of organic and mineral soils has not previously been reported in coastal freshwater forested wetlands. To this end, litter, roots, and soils were collected from organic and mineral soil horizons in two coastal freshwater forested wetlands in North Carolina with different hydrological regimes: (1) a natural bottomland hardwood forest (natural); and (2) a ditched and drained, intensively-managed wetland for loblolly pine silviculture (managed). We found that hydrology and soil horizon, and to a lesser degree micro-topography, was important in shaping observed differences in size and 13C signature of soil and microbial pools between the natural and managed wetland. The natural wetland had higher SOC and MBC concentrations in the litter, surface organic, and mineral horizons compared to the managed wetland. In the managed wetland, 13C of SOC was enriched across most of the soil profile (Oa and mineral soil horizons) compared to the natural wetland, suggesting enhanced decomposition and incorporation of microbially-derived inputs into stable SOC pools. Root C concentration decreased with soil depth, while root 13C signature became enriched with soil depth. In the litter and Oe horizon of the natural wetland, MBC was higher and 13C of MBC and SOC was enriched in hummocks compared to hollows. The 13C of MBC and SOC tended to be enriched in upper soil horizons and depleted in lower soil horizons, particularly in the managed wetland. We conclude that drainage of these coastal wetlands has enhanced the breakdown of previously stabilized C and has the potential to alter regional C storage, feedback to climate warming, and ecosystem response to changing environmental conditions.
Introduction
Globally, wetlands store large quantities of carbon (C) in belowground pools over a small land area (Johnson and Kern, 2003; Lal, 2005; Yu et al., 2011), providing a critical role in mitigating atmospheric greenhouse gas concentrations through long-term sequestration in soils. The ability of forested wetlands to store large amounts of atmospheric C stems largely from high organic matter inputs and reduced soil organic C (SOC) degradation in saturated soils (Trettin et al., 1996; Yu et al., 2006; Miao, 2013). Coastal freshwater forested wetlands along the southeastern US are particularly important to regional C storage (Johnson and Kern, 2003), and provide myriad ecosystem and economic services (Tiner, 1984; Richardson, 1994; Jansson et al., 1998; Mitsch and Gosselink, 2000; Snodgrass et al., 2000; Hinton et al., 2013; Tanentzap et al., 2014). As such, the value of wetlands to regional and global ecosystem health is undeniable. Unfortunately, these wetlands are currently under accelerated threat from sea level rise, increased frequency/intensity of extreme storm events and an array of anthropogenic disturbances, most notably drainage for alternative land-uses (Dahl, 2011), threatening the C storage capacity of these ecosystems.
It has been estimated that 87–89 million hectares (ha) of wetlands existed in the conterminous United States prior to European settlement, approximately half of which were lost by the 1950's (Tiner, 1984; Dahl, 1990). Drainage has further reduced the extent of freshwater forested wetlands in the conterminous US (~9.7% managed since the mid-1970s; Dahl et al., 1991; Dahl, 2011) and around the world (Gorham, 1991; Minkkinen and Laine, 1998; Holden et al., 2004). Wetland drainage occurs for various reasons, including agricultural and silvicultral purposes, urbanization, and expansion of coastal communities. Between 2004 and 2009, drainage for loblolly pine (Pinus taeda L.) silviculture accounted for a majority (38%) of total freshwater forested wetlands converted in the southeastern US compared to development (26%), agriculture (13%), sea level rise (4%), and other upland land uses (19%) (Dahl, 2011). Pine silviculture in the southeastern US is instrumental in providing wood, paper, and energy supplies for the US and internationally, thus earning the label “wood basket of the US” (Fox, 2000; Fox et al., 2004). Thus, advanced loblolly pine silviculture is of great importance to local and regional economies, but the drainage of natural forested wetlands has poorly quantified environmental costs in terms of loss of C (Armentano and Menges, 1986) and climate change impacts that could alter long-term stability of coastal regions worldwide.
Hydrology is a key regulating factor of wetland primary productivity and decomposition processes (Day and Megonigal, 1993; Kasimir et al., 2018), greenhouse gas emissions (Fenner and Freeman, 2011; Dai et al., 2012; Miao, 2013; Kasimir et al., 2018), and C storage potential (Yu et al., 2006). Microorganisms are also a key component of the SOC cycle, controlling organic matter decomposition, nutrient mineralization and immobilization, and SOC stabilization. Rates of aerobic microbial activity, decomposition, and soil respiration are typically lower in saturated soils (Freeman et al., 2004; Fenner and Freeman, 2011; Miao, 2013; Minick et al., 2016). Microbial biomass, which correlates with the potential for SOC decomposition (Neely et al., 1991), also tends to be lower in saturated soils compared to unsaturated soils (Poret-Peterson et al., 2007). Wetland drainage results in drawdown of the water table, enhancing soil aeration and stimulating breakdown of previously stable SOC (Gorham, 1991; Trettin et al., 1996; Fenner and Freeman, 2011; Kasimir et al., 2018). This results in loss of SOC as increased (aerobic) heterotrophic respiration releases C to the atmosphere as CO2. Therefore, it is generally considered that wetland drainage leads to losses of SOC (Gorham, 1991; Trettin et al., 1996; Trettin and Jurgensen, 2003), but this has not always been observed (Minkkinen and Laine, 1998; Toberman et al., 2008, 2010) and depends on time since drainage and post-drainage land use (Minkkinen and Laine, 1998; Noormets et al., 2010) or other edaphic factors such as pH and concentration of phenolic compounds (Toberman et al., 2008, 2010). For instance, more intense disturbance after drainage of forested wetlands in the southeastern US has resulted in large losses of SOC (Trettin et al., 1996) compared to less intense disturbances (Noormets et al., 2010). Given that drainage changes the vertical distribution of water in the soil profile, with clear potential to influence wetland SOC storage, analysis of SOC cycling as a function of land-use change is necessary to understand long-term ecosystem C storage potential.
Stable C isotope profiles of SOC pools provides a robust indicator of the degree of microbial processing of SOC and is a widely used tool in soil ecological research, integrating across several biogeochemical process (Ehleringer et al., 2000). For instance, the length of previous exposure to altered environmental conditions (such as changes in hydrology after wetland drainage; Minkkinen and Laine, 1998; Noormets et al., 2010) can greatly affect organic matter decomposition rates and hence its isotopic signature (Melillo et al., 1989). Aerated soils typically display 13C enrichment of SOC with increasing soil depth (O'brien and Stout, 1978; Natelhoffer and Fry, 1988; Melillo et al., 1989; Wang et al., 2015). Enrichment of SOC with soil depth may result from numerous independent or interacting processes including: (1) breakdown of 13C-depleted C compounds by microbes during decomposition; (2) isotopic fractionation (discrimination) by soil microbes, in which the light C isotope (12C) is preferentially utilized during metabolism and respiration of SOC while the heavy carbon isotope (13C) remains in the residual SOC pool; (3) incorporation of microbially-derived, 13C-enriched compounds into SOC pools; and (4) via inputs of 13C-depleted CO2 to the atmosphere as fossil fuels are burned (the Suess effect; Werth and Kuzyakov, 2010; Lerch et al., 2011; Keeling et al., 2017; Minick et al., 2017a). Soil microbial biomass C also tends to be enriched in 13C compared to bulk soil (Werth and Kuzyakov, 2008) and mounting evidence suggests a much greater contribution of microbial biomass C to stable C pools than previously considered (Schmidt et al., 2011). This indicates that 13C enrichment of SOC with depth results from a greater degree of decomposition and reflects contributions of 13C-enriched microbial products and necromass to SOC, partially explaining the observed enrichment of SOC pools with soil depth (Natelhoffer and Fry, 1988; Werth and Kuzyakov, 2008; Schmidt et al., 2011). By contrast, under saturated or anaerobic soil conditions, breakdown of organic matter is suppressed and therefore should result in different patterns of SOC 13C natural abundance with soil depth as organic material is preserved in a less decomposed state (von Fischer and Tieszen, 1995; Esmeijer-Liu et al., 2012; Hobbie et al., 2017). For instance, Krüger et al. (2014) found that depth profiles of 13C were uniform in peatland soils, although some enrichment from surface peat soils to deeper peat soils is not completely unexpected as fresh plant material is slowly decomposed over long periods of time (Hobbie et al., 2017). Suppressed breakdown of SOC in wetlands should lead to 13C of SOC being more similar to that of plant-derived organic materials, particularly of non-soluble, 13C-depleted, recalcitrant C compounds such as lignin (Benner et al., 1987; von Fischer and Tieszen, 1995; Bowling et al., 2008; Segnini et al., 2013). Microbial stable C isotope composition should also provide an indicator of the type of C compounds soil microorganisms are metabolizing (Bowling et al., 2008; Coyle et al., 2009; Werth and Kuzyakov, 2010), as well as reflect the balance between the 13C of immobilized C substrates and respired CO2. Unfortunately, 13C analysis of microbial biomass C and SOC in wetlands have rarely been studied simultaneously or under different land-use regimes. Thus, it is unclear how hydrology affects 13C profiles of soil and microbial C pools in managed vs. natural wetland systems, leaving a major gap in our knowledge of how land-use change alters wetland SOC stabilization.
Finally, hummock and hollow micro-topography is a common feature of wetlands, and has been shown to influence SOC cycling due to effects on soil aeration through interactions with fluctuations in the water table (Miao et al., 2017), which has important implications for wetland C biogeochemistry and C cycling processes (Keiluweit et al., 2017). Similarly, in intensively managed pine plantations of the lower coastal plain the soil is bedded into rows to increase soil aeration and reduce competition, increasing tree seeding survival and growth (Fox et al., 2007). Bedding of pine plantations creates more spatially uniform micro-topography, compared to a natural forested wetland, with alternating high (bed) and low (interbed) zones, which can also influence soil C and nutrient cycling (Minick et al., 2015, 2017b).
The objective of this study was to characterize the effects of intensive management on SOC cycling and storage in coastal forested wetlands of the US Southeast. We hypothesized that: (1) the lower water table and improved aeration in the managed wetland would promote decomposition of existing SOC and greater microbial biomass; (2) the enhanced breakdown of SOC in the managed wetland would result in the enrichment of SOC 13C as 13C enriched microbial products are incorporated into the SOC pool; and (3) the δ13C of microbial biomass will reflect the signature of the substrate, i.e., be more enriched in the managed wetland. To this end, plant litter, roots and soils were sampled from two micro-topographic positions and numerous organic and mineral soil horizons (e.g., depth) in a natural and managed forested wetland along the lower coastal plain of North Carolina to quantify the C concentration and 13C isotopic signature of vegetation, SOC and microbial pools.
Methods
Site Description
The natural forested wetland was located at Alligator River National Wildlife Refuge (ARNWR) in Dare County, North Carolina (35°47′N, 75°54′W), with the Alligator River to the west, Albemarle Sound to the north, and the Croatan Sound to the east (Figure 1). The refuge was established in 1984 and is characterized by a diverse assemblage of pocosin (wetland fed by rainwater or groundwater, acidic peat and mineral soils, oligotrophic, woody vegetation) wetland types and swamp forests (Allen et al., 2011). ARNWR has a network of roads and canals, but in general contains vast expanses of minimally disturbed forested- and shrub-wetlands. Thirteen plots were established in a 4 km2 area in the middle of a bottomland hardwood forest surrounding a 35 m eddy covariance flux tower (US-NC4 in the AmeriFlux database; Miao, 2013). Of the 13 plots (7 m radius), the five central plots were utilized for this study (Figure 1). Over-story plant species composition was predominantly composed of black gum (Nyssa sylvatica), swamp tupelo (Nyssa biflora), bald cypress (Taxodium distichum), with occasional red maple (Acer rubrum), sweet gum (Liquidambar styraciflua), white cedar (Chamaecyparis thyoides), and loblolly pine (Pinus taeda). The understory was predominantly fetterbush (Lyonia lucida), bitter gallberry (Ilex albra), red bay (Persea borbonia), and sweet bay (Magnolia virginiana) (Table 1). The mean annual temperature and precipitation from an adjacent meteorological station (Manteo AP, NC, 35°55′N, 75°42′W, National Climatic Data Center) for the period 1981–2010 were 16.9°C and 1,270 mm, respectively. These wetlands are characterized by a hydroperiod that operates over short time scales and is driven primarily by variation in precipitation. Soils are classified as a Pungo series histosols (very poorly drained dystic thermic typic Haplosaprist) with a deep, highly decomposed muck layer overlain by a shallow, less decomposed peat layer and underlain by highly reduced mineral sediments of Pleistocene origin (Riggs, 1996). Ground elevation is <1 m above sea level. Periodic in situ measurements of redox potential indicate that standing water is relatively aerated (Eh = 175–260 mV), while surface soils of hummocks are more aerated (Eh = 320 mV) than hollows (Eh = 120–150 mV) compared to deeper organic soils (Eh = 50–90 mV). Micrometeorological variables (precipitation, water table depth) were measured at a micro-met station established at the center of the study site from January 1, 2016 to December 31, 2016. Both precipitation and water table data were collected at 30-min intervals. Water table depth was measured with a pressure water level data logger (Infinities, Port Orange, FL, USA). Precipitation was measured with a tipping bucket rain gauge (TE-525, Texas Electronics, TX, USA).
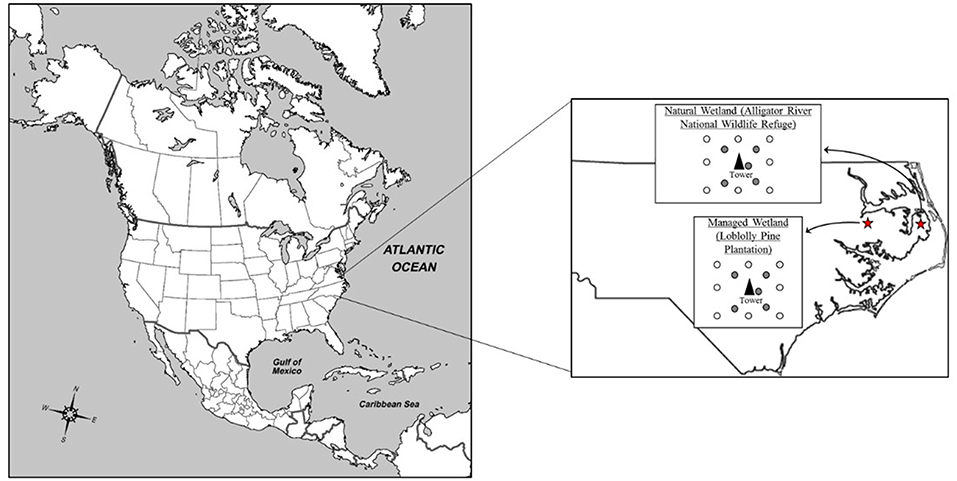
Figure 1. Location of field sites in eastern North Carolina, USA. The natural wetland is located in the Alligator River National Wildlife Refuge, Dare County, NC, while the managed wetland is currently in loblolly pine silviculture and is located in Washington County, NC. Both sites are equipped with a centrally located eddy flux tower, with 13 plots surrounding the tower. Of those thirteen plots, five were utilized for this study (gray shaded circles).
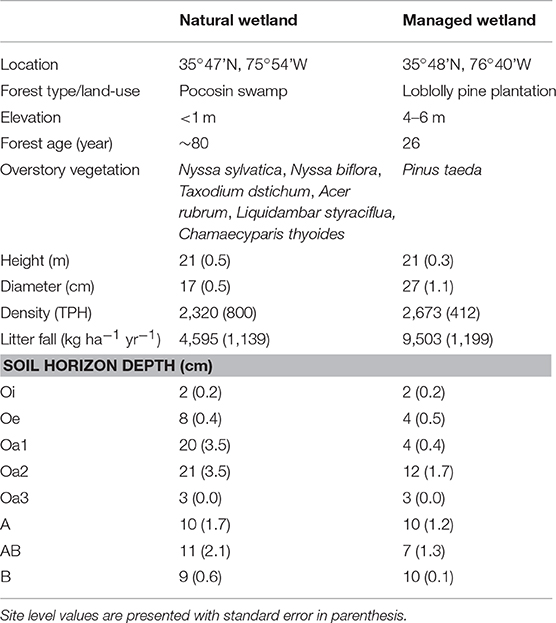
Table 1. Site characteristics, including forest age, dominant overstory vegetation, tree height (m), diameter at breast height (>5 cm), tree density (trees per hectare >2.5 cm; TPH), average annual litter fall (2015–2016), and depth of soil horizons (cm).
The managed wetland was an intensively managed loblolly pine plantation located in Washington County, NC (35°48'N, 76°40'W) within the lower coastal plain mixed forest province (Noormets et al., 2010, 2012; Sun et al., 2010; Figure 1). In the late 1800's/early 1900's, the area was clearcut and ditched for agriculture. Since the mid-1960's, this site has been owned and operated by Weyerhaeuser NR Company for commercial timber production and is currently in its fourth rotation of loblolly pine. The pine plantation watersheds are managed with a network of parallel ditches (0.9–1.3 m deep; 90 m spacing) and more widely spaced roadside canals. Drainage lowers the height of the water table, and improves site access and tree productivity by reducing stresses caused by excessive soil water, especially during winter months. The current loblolly pine stand is also part of the Ameriflux core sites (US-NC2 in the Ameriflux database) and was planted in 1992, on bedded rows, with 2-year-old half sibling seedlings after clear-cutting the previous mature pine plantation. Planting spacing was 1.5 by 4.5 m. Bedding is common best management practice along the southeastern lower coastal plain (Fox et al., 2007) in order to increase growth and survival of seedlings. Bedding creates unique micro-topography in managed pine forests, resulting in high (bed) and low (interbed; space between beds) micro-topographic locations, which influence SOC stabilization (Minick et al., 2017b) and nitrogen (N) cycling (Minick et al., 2014). Thirteen plots were established in the mature loblolly pine stand surrounding a 28 m eddy flux tower. Of these 13 plots (7 m radius), five central plots were utilized for this study (Figure 1). The understory was primarily composed of young red maple (A. rubrum) and other non-woody plants such as devil's walking stick (Aralia spinosa), pokeweed (Phytolacca americana), beautyberry (Callicarpa americana), giant cane (Arundinaria macrosperma), and meadow grass (Poa spp.) (Domec et al., 2015). The histic-mineral soil at this site is classified as a Belhaven series histosol (loamy, mixed, dystic, thermic Terric Haplosaprists) with a highly decomposed organic matter layer underlain by loamy marine sediments. Belhaven and Pungo soil series are typically found within the same soil map of this region, with Belhaven series soils having somewhat higher permeability due to the loamy soil texture (USDA Soil Survey Staff, 2008). The soils are very poorly drained with a ground elevation of 4–6 m above sea level. The long-term (1945–2010) average annual precipitation was 1290 ± 199 mm, evenly distributed throughout the year. Long term mean annual temperature averaged 15.5°C, with a monthly high temperature occurring in July (26.6°C), and a monthly low occurring in January (6.4°C). Micrometeorological variables (precipitation, water table depth) were measured at a micro-met station established at the center of the study site from January 1, 2016 to December 31, 2016. Water table depth was measured hourly with a pressure water level data logger (Infinities, Port Orange, FL, USA). Precipitation was measured continuously by two tipping bucket rain gages (TE-525, CSI; Onset1 Data Logging Rain Gauge, Onset Computer Corporation, USA), and one backup manual rain gage (Forestry Suppliers Inc., USA).
Litterfall and Fresh Litter
For both sites, average annual litterfall (Table 1) was quantified over numerous collection periods in 2015 and 2016 from three litter baskets (2,122 cm2) located within each of the five plots. After collection, litter was dried at 50°C until constant weight and the dry weight of litter was recorded.
In 2016, fresh litter was collected for C concentration and 13C isotopic signature. This litter was collected from young trees or from newly fallen branches with litter still attached. Fresh litter was dried at 50°C until constant weight and then ground to a fine powder in a Wiley mill (Thomas Scientific, Swedesboro, NJ, USA). Ground fresh litter samples were analyzed in duplicate for C concentration and δ13C isotopic signature on a Picarro G2201-i Cavity Ring-Down Spectroscopy (CRDS) isotopic CO2/CH4 analyzer (Picarro Inc., Sunnyvale, CA, USA) interfaced with a Picarro Combustion Module (Picarro Inc., Sunnyvale, CA, USA). For δ13C analysis, certified standards were used to develop a standard curve from the expected and measured δ13C values (R2 > 0.999). These standards included USGS 40 (L-glutamic acid) (δ13C = −26.39‰; USGS Reston Stable Isotope Laboratory, Reston, VA, USA), protein (δ13C = −26.98‰; Elemental Microanalysis Ltd, Okehampton, UK), urea (δ13C = −48.63‰; Elemental Microanalysis Ltd, Okehampton, UK), atropine (δ13C = −18.96‰; Costech Analytical Technologies, Inc, Valencia, CA, USA), and acetanilide (δ13C = −28.10‰; Costech Analytical Technologies, Inc, Valencia, CA, USA). For C concentration, atropine standards were weighed out over a range of C concentrations that encompassed the expected C concentrations of the unknown samples. A standard curve for C concentration was also developed from the expected and measured C concentration of the atropine standards (R2 > 0.99). All unknown sample C concentration and δ13C values were adjusted using the linear equation derived from the appropriate standard curve. Soil, root, and microbial biomass C samples were analyzed using the same procedure (see below).
Soil Sample Collection and Processing
Soils at both the natural and managed forested wetlands were sampled in early 2016. A single time point measurement such as this is not uncommon in studies where the objective is to capturte forest management (or vegetation) effects on soil C pools (see Krüger et al., 2014; Hobbie et al., 2017, for examples of other studies which measured SOC pools at wetland sites) and not necessarily seasonal dynamics. The SOC pool is typically quite stable while microbial biomass can vary seasonally as microbial activity is sensitive to changes in temperature and moisture (see Miao, 2013; Miao et al., 2017). Our results highlight clear differences though between the two wetland sites in MBC and δ13C of MBC and therefore provide a reasonable index for comparing the effects of forest management on microbial biomass.
In the natural wetland, two soil samples were collected from high (hummock) and low (hollow) micro-topographic positions in early 2016 within each of the five plots. First, surface litter (Oi horizon) was collected within a 0.25 × 0.25 m2 quadrant. In the natural wetland, rooting density is very high at the surface (Domec et al., 2015) rendering traditional coring methods ineffective. Therefore, after surface litter was collected a saw was used to cut a 0.25 × 0.25 m2 monolith (Oe horizon) down to ~8 cm depth. Below the Oe horizon was an approximate 20 cm deep space containing very large coarse roots and water and/or air. This was particularly evident in hummocks. Coarse roots in this region (root mat) were also cut within the 0.25 × 0.25 m2 quadrant and collected for analysis. Finally, the organic (Oa horizon) and mineral (A, AB, and B) soil horizons below the root mat were sampled with a Macaulay auger. The Oa horizon samples were subdivided into three sub-horizons: Oa1, which was directly below the coarse root mat but still contained a large amount of coarse and fine roots; Oa2, which comprised the bulk of the soil profile below Oa1, but was more homogenous with little root biomass; and Oa3, which was the 3 cm portion above the mineral A horizon. In some cases, the Oa3 horizon may be better characterized as an OaA as some vertical mixing of the upper organics and lower mineral soil had occurred. Mineral soil samples were collected from the A, AB, and the top 10 cm of B mineral horizons.
In the managed wetland, two soil samples were collected from high (bed) and low (interbed) micro-topographic positions in early 2016 from each of five plots. A dense surface rooting zone was not present at the managed wetland as was at the natural wetland. Therefore, a 5 cm diameter by 1.5 m long PVC corer was used to sample organic and mineral soils. Surface litter samples were first collected from a 0.25 × 0.25 m2 quadrant. Organic (Oe and Oa) and mineral (A, AB, and B) soil horizons were then sampled beneath the collected litter using the PVC corer and subdivided into the same horizons as the natural wetland: Oe, Oa1, Oa2, Oa3, A, AB, and top 10 cm of B mineral horizons.
Root and Soil Characteristics
Live and dead roots were removed from the samples and soil was removed from roots by rinsing in distilled water (dH2O). Roots were then dried to constant mass and ground to a fine powder in a Wiley mill. Ground root samples were analyzed in duplicate for C concentration and δ13C isotopic signature on a CRDS isotope analyzer. After roots were removed, the remaining fresh soil from each of the two samples per plot and horizon were composited and homogenized. Soil pH was measured on fresh soil samples with a glass electrode in a 1:2 mixture (by mass) of soil and dH2O. Gravimetric soil water content was determined by drying a fresh subsample for 24 h at 105°C.
After microbial biomass analysis (see below), the remaining soil was dried at 70°C. Air dried soils were homogenized by passing through a 2 mm sieve. Soil organic matter content was measured by loss on ignition at 600°C for 4 h. A subsample of the dried, homogenized soil was ground to a fine powder before C concentration and δ13C isotopic signature on a CRDS isotope analyzer.
Soil Microbial Biomass C
The chloroform fumigation extraction (CFE) method was adapted from Vance et al. (1987) in order to estimate MBC and δ13C. Briefly, one subsample of fresh soil (~2 g dry weight each) was placed in a 50 mL beaker in a vacuum desiccator to be fumigated. Another subsample was placed into an extraction bottle for immediate extraction in 0.5 M K2SO4 by shaking for 1 h and subsequently filtering through Whatman #2 filter paper to remove soil particles. The samples in the desiccator were fumigated with ethanol-free chloroform (CHCl3) and allowed to incubate under vacuum for 4 d. After the 4 d incubation, fumigated samples were extracted similar to that of unfumigated samples. Filtered 0.5 M K2SO4 extracts were frozen at −20°C until further analysis. Upon thawing, extracts were dried at 60°C in a ventilated drying oven and then ground to a fine powder with mortar and pestle. Ground samples were analyzed for C concentration and δ13C isotopic signature on a CRDS isotope analyzer. Microbial biomass C was determined using the following equation:
where the chloroform-labile pool (EC) is the difference between C in the fumigated and non-fumigated extracts, and kEC (extractable portion of MBC after fumigation) was estimated as 0.45 (Joergensen, 1996).
δ13C of MBC was estimated as the δ13C of the C extracted from the fumigated soil sample in excess of that extracted from the non-fumigated soil sample using the following equation:
where Cf and Cnf is the concentration (mg kg−1 soil) of C extracted from the fumigated and non-fumigated soil samples, respectively, and δ13Cf and δ13Cnf is the 13C natural abundance (%) of the fumigated and non-fumigated soil samples, respectively.
Statistical Analysis
All data were analyzed using repeated-measures ANOVA (PROC MIXED package). Each of the two sites and two micro-topographical sampling locations per site were treated separately as treatments (Trt) and analyzed as fixed effects, with soil horizon (Hrz) as the repeated measure (Littell et al., 2000; Schabenberger and Pierce, 2001; Sainju and Singh, 2008). Soil horizons (or soil depths) in a soil profile are spatially correlated due to lateral and horizontal movement of organic and mineral soil materials. Therefore, we chose to treat soil horizon as a repeated measure for modeling this correlation in space (Littell et al., 2000; Schabenger and Pierce, 2001). Data were log transformed where necessary to meet assumptions of ANOVA. All data were plotted as means of raw data. If significant main effects or interactions were identified in the repeated-measures ANOVA (P < 0.05), then differences were tested using least square means post-hoc analysis with a Tukey's adjustment. Regression analysis (PROC REG) was used to relate soil C pools, isotopic signature, and soil water content. All statistical analysis were performed using SAS 9.4 software (SAS Institute, Cary, NC, USA).
Results
Tree biomass and annual litterfall was ~40 and 50% higher, respectively, in the managed compared to natural wetland (Table 1). On average, organic horizons were deeper in the natural wetland (54 cm to mineral soil) compared to the managed wetland (25 cm to mineral soil; Table 1). Water table depth was different between the two sites, with the natural wetland having continuous water table depths above zero cm and completely flooded at numerous time periods throughout 2016 (Figure 2A). Alternatively, water table depth in the managed wetland was never above zero cm and was punctuated by seasonally deep water table depths (Figure 2B). Water table depth was clearly influenced by precipitation for both sites, with large precipitation events leading to quick decreases in water table depth followed by a gradual increase (Figures 2A,B). Annual cumulative precipitation for 2016 was 1,126 and 1,444 mm in the natural and managed wetlands, respectively. Similar temporal patterns of precipitation and fluctuations in water table depth were found for each site, indicating that hydrologic regimes across the region were similar during this study (Figures 2A,B).
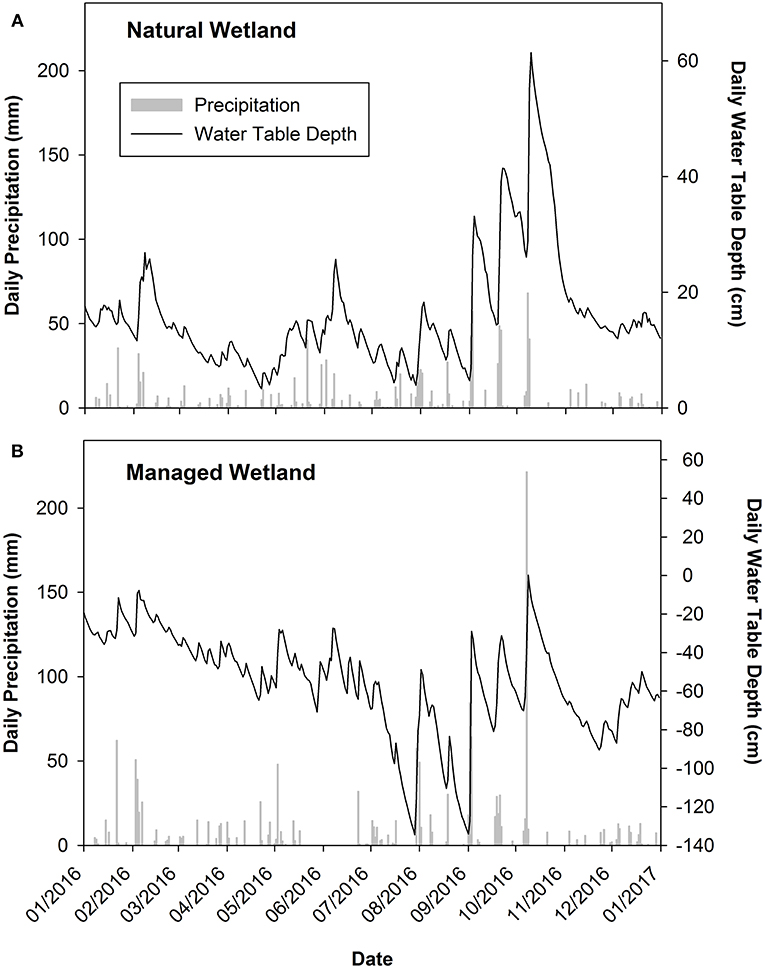
Figure 2. Seasonal dynamics of precipitation (daily cumulative) and water table depth (daily average) in 2016 from the (A) natural wetland and (B) managed wetland located in coastal North Carolina. In the natural wetland, the average height of hummocks was 12.8 cm, hollows was −3.8 cm, and the site average was 8.7 cm, relative to the elevation of the water level data logger location at the ground micrometeorological station (Miao et al., 2017). Similar information for the managed wetland micro-topography is not available.
The natural wetland was highly acidic, ranging from 4.2 to 4.9, with minimal differences between soil horizons (Tables 2, 3). The managed wetland was also acidic, ranging from 4.0 to 5.6, with differences between soil horizons primarily driven by higher pH in litter and B horizons (Table 3). Litter and B horizon pH were higher in the managed wetland compared to the natural wetland. Soil organic matter content decreased moving down the soil profile for both the natural and managed wetland (Tables 2, 3). Soil organic matter content was similar between sites and sampling locations in the litter and organic horizons, but was approximately two times higher in the mineral horizons (A, AB, and B) of the natural wetland compared to the managed wetland. Soil water content ranged from 20 to 90% and generally decreased with depth (Table 3). In the natural wetland, soil water content was higher in all soil horizons compared to the managed wetland (Table 3). Not surprisingly, hollows in the natural wetland had higher water content in the litter and Oe horizon compared to that of the hummocks (Table 3).

Table 2. Results (F-values and significance) of repeated-measures ANOVA testing effects of all site (e.g., natural and managed wetland) and sampling location (e.g., hummock, hollow, bed, or interbed) combinations (Trt), soil horizons (Hrz), and interactions for soil pH, soil organic matter (SOM), soil water content (SWC), soil organic C (SOC) and microbial biomass C (MBC) pools, 13C isotopic signature of each pool, and difference between 13C signature of each pool.
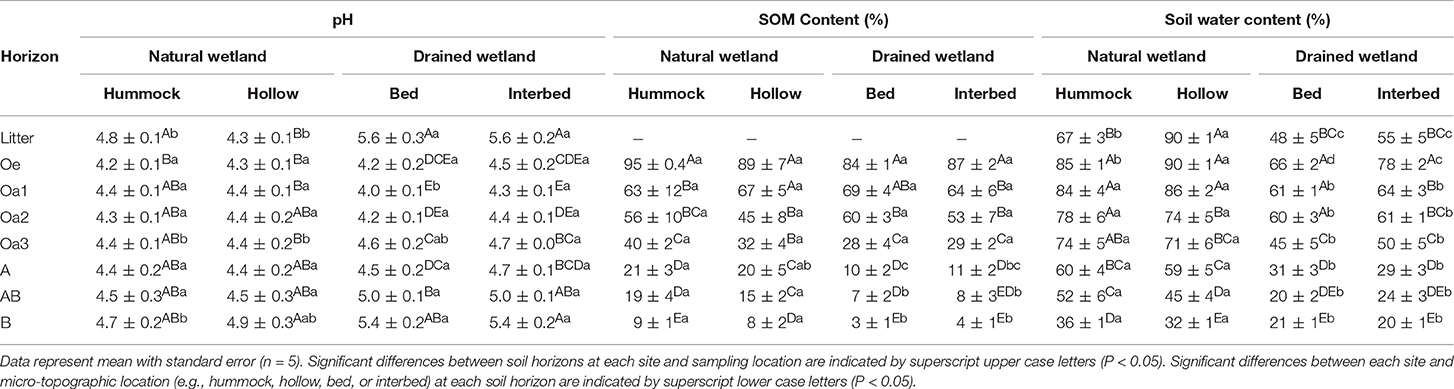
Table 3. Soil pH, organic matter content (%), and water content (%) for samples collected from organic and mineral horizons from a natural coastal freshwater forested wetland (Natural Wetland) and an intensively managed loblolly pine plantation (Managed Wetland) in coastal North Carolina, USA.
Soil organic C concentration ranged from 7.6 to 516.0 g C kg soil−1 (Figure 3A) and was influenced by micro-topography and soil horizon (Table 2). A clear decline in SOC concentration occurred with depth at both sites and micro-topographic positions (Figure 3A). In the litter, Oe, A, AB, and B horizons, both hummocks and hollows in the natural wetland had higher SOC concentration compared to the managed wetland (Figure 3A). Microbial biomass C also declined with depth (Figure 3B) and was influenced by drainage, microtopography, and soil horizon (Table 2 and Figure 3B). In the litter horizon, MBC was similar between the hummock of the natural wetland and the bed and interbeds of the managed wetland, but lower in the hollow of the natural wetland (Figure 3B). Interestingly, MBC in the Oe horizon in the hummock of the natural wetland was two to three times higher than other micro-topographic positions in the natural or managed wetland (Figure 3B). The managed wetland tended to have higher MBC in the Oa2 horizon compared to the natural wetland, while the natural wetland had higher MBC in the AB mineral horizon (Figure 3B). Finally, no difference in site or micro-topography was found for root C concentrations, although a significant effect of horizon was found (Table 2), evident as a decrease in root C concentration with depth (Figure 3C).
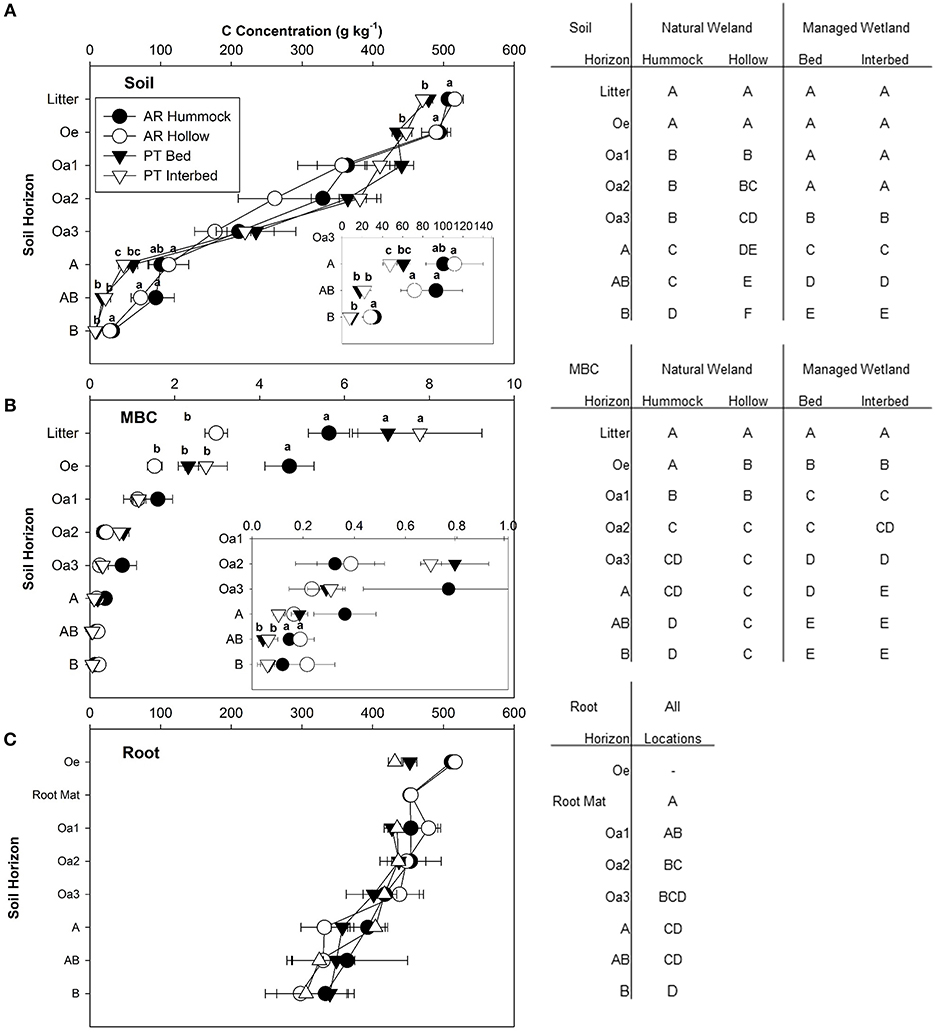
Figure 3. Concentration of C in soils (A), microbial biomass C (MBC) (B), and roots (live + dead) (C) for each horizon sampled at the natural and managed wetlands. Insets were provided to help visualize differences at lower concentrations of soil and microbial biomass C found in deeper soil horizons. Data represent means with standard error (n = 5). Significant differences between field sites and micro-topographical zones are indicated by lower case letters within each figure (P < 0.05). Significant differences between soil horizons are indicated by upper case letters in the tables beside each figure (P < 0.05).
Surface litter (Oi horizon) δ13C ranged from −30.7 to −30.3‰ (Figure 4A). Soil δ13C ranged from −30.0 to −26.2‰ across all sites and micro-topographical locations (Figure 4A). Differences in soil δ13C between site, micro-topography, and soil horizon were observed below the litter layer and continued down to the B mineral horizon (Table 2 and Figure 4A). In the Oe horizon, 13C of hummocks was enriched (−29.1 ± 0.29‰) compared to hollows of the natural wetland (−29.7 ± 0.14‰) and interbeds of the managed wetland (−29.9 ± 0.07‰; Figure 4A). In the Oa1 horizon of the managed wetland, the beds were more enriched in 13C than the hollows of the natural wetland. Beyond the Oa1 horizon, both the beds and interbeds of the managed wetland were more enriched in 13C down to the deepest mineral horizon sampled. This pattern became more apparent with depth, as the δ13C signature of soils continued to become enriched in the managed wetland, while no change in δ13C of soils was found with depth past the Oa1 horizon in the natural wetland (Figure 4A). The greatest differences between sites were in the A and AB horizons, with the δ13C of the natural wetland ~-28.9‰ and that of the managed wetland ~-26.5‰.
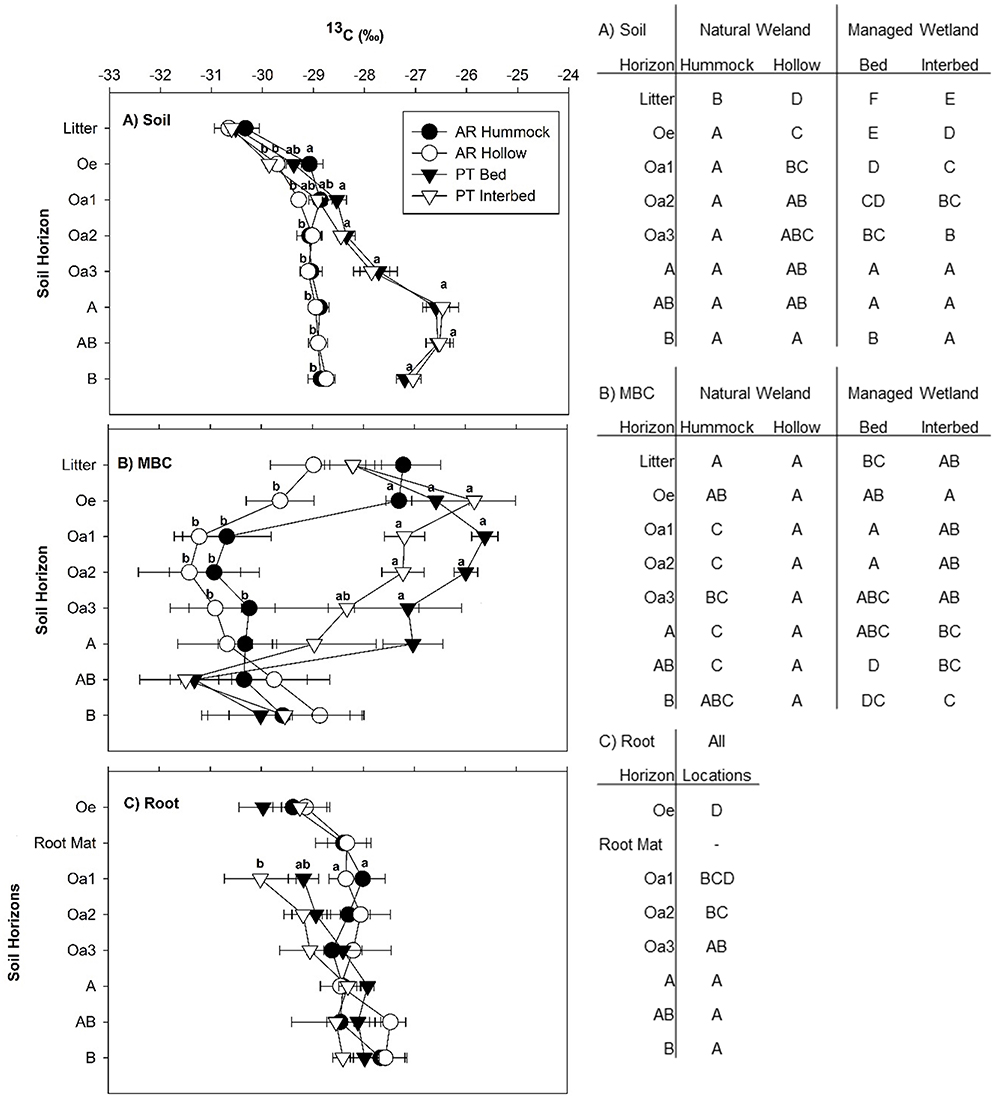
Figure 4. The δ13C of soil organic C (A), microbial biomass C (MBC) (B), and roots (live + dead) (C) measured in each soil horizon sampled at the natural and managed wetlands. Data represent mean with standard error (n = 5). Significant differences between field sites and micro-topographical zones are indicated by lower case letters within each figure (P < 0.05). Significant differences between soil horizons are indicated by upper case letters in the tables beside each figure (P < 0.05).
For δ13C of MBC, a high degree of variability was found but differences due to site, micro-topography, and soil horizon were observed (Table 2). In the Oe horizon, 13C of MBC was depleted in the hollows of the natural wetland compared to all other sites and micro-topographic combinations. In both the Oa1 and the Oa2, 13C of MBC in the hummocks and hollows of the natural wetland were highly depleted compared to that of the bed and interbed of the managed wetland (Figure 4B). After the Oa2 horizon, the δ13C of MBC in the managed wetland became more depleted while little difference between soil horizons was found for the natural wetland, except that the MBC in litter and Oe horizons of hummocks tended to be enriched in 13C compared to all other depths.
The δ13C isotopic signature was also determined for fresh litter from four major tree species in the natural wetland, loblolly pines in the managed wetland, and live plus dead roots from both sites. For the natural wetland, δ13C of fresh litter ranged from −31.6‰ in Nyssa to −28.3‰ in Taxodium (Table 4). The δ13C value of loblolly pine litter was −30.0 ± 0.01‰ and −31.4 ± 0.04‰ from the natural and managed wetland, respectively (Table 4). The δ13C of roots ranged from −30.7‰ in Oa1 to −27.1‰ in the B horizon (Figure 4C). Root δ13C exhibited an overall difference between site and micro-topography (Table 2). Across all horizons, δ13C of roots was different between hummocks (−28.4 ± 0.18‰) and hollows (−28.2 ± 0.17‰) of the natural wetland compared to beds (−29.0 ± 0.17‰) of the managed wetland. Across horizons, a similar trend was observed in 13C of roots between sites and micro-topographic positions, with the most depleted 13C values in the Oe horizon (−29.4 ± 0.23‰) compared to Oa2 horizon (−28.6 ± 0.23‰) and the most enriched values in the A (−28.3 ± 0.16‰), AB (−28.1 ± 0.24‰), and B (−27.9 ± 0.17‰) mineral horizons (Figure 4C).
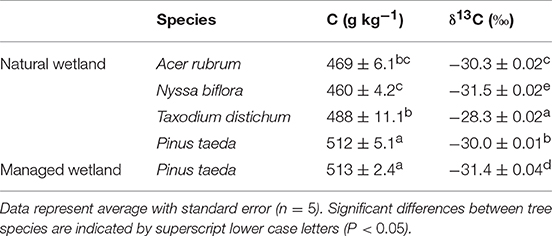
Table 4. The C concentration and δ13C of fresh litter for major tree species in the natural and managed wetland.
The differences in δ13C between SOC, MBC, and root C pools were calculated. A positive value indicates enrichment of 13C in the first pool compared to the second pool, while a negative value indicates depletion. For the difference between SOC and MBC, an interaction between site, micro-topography, and horizon was observed (Table 2). In the litter and Oe horizons, MBC tended to be enriched in the heavy isotope compared to SOC (Figure 5A). In both the Oa1 and Oa2 horizons, MBC was depleted in the heavy isotope compared to SOC in the natural wetland, but enriched in the managed wetland (Figure 5A). At both sites, MBC was depleted in the heavy isotope compared to SOC in the mineral horizons (Figure 5A).
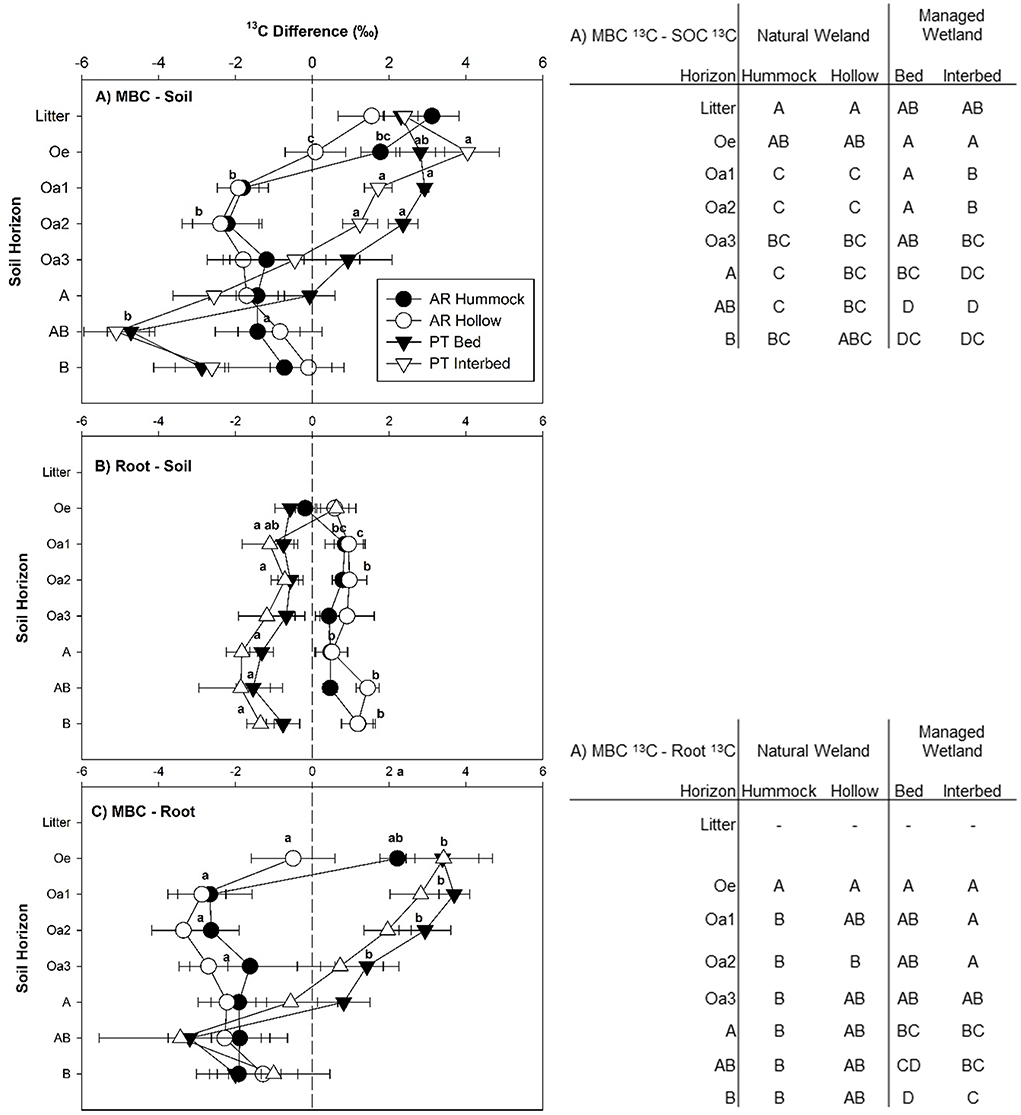
Figure 5. Difference in δ13C signature between soil and microbial biomass C (MBC) (A), soil and root (B), and MBC and roots (C) for each horizon sampled at the natural and managed wetland. A positive value indicates enrichment of 13C in the first pool compared to the second pool, while a negative value indicates depletion in the first pool compared to the second pool. Data represent mean with standard error (n = 5). Significant differences between field sites and micro-topographical zones are indicated by lower case letters within each figure (P < 0.05). Significant differences between soil horizons are indicated by upper case letters in the tables beside each figure (P < 0.05).
Root-C in the natural wetland was enriched in 13C compared to SOC while the opposite was true for the managed wetland (Figure 5B). Furthermore, an overall difference was found for each site by micro-topography combination (Table 2), with the difference in δ13C between SOC and root-C being more positive in hummocks (0.6 ± 0.20‰) and hollows (0.9 ± 0.18‰) of the natural wetland compared to beds (−0.9 ± 0.18‰) and interbeds (−1.1 ± 0.18‰) of the managed wetland (Figure 5B). The difference between the δ13C signature of root-C and MBC was also influenced by horizon (Table 2), but most strongly in the managed wetland (Figure 5C). In the managed wetland, MBC was enriched in the heavy isotope compared to root-C from the Oe horizon down to the Oa3 horizon, but was only enriched in the hummock of the Oe horizon in the natural wetland (Figure 5C). Otherwise, MBC was depleted in the heavy isotope compared to root-C in the natural wetland and in the mineral horizons of the managed wetland.
Strong relationships were found between SOC concentration, isotopic signature, and soil water content (Figures 6, 7), particularly in the managed wetland. Overall, correlations were higher in the managed wetland site and lower in the natural wetland, especially that of the hummocks in the natural wetland. SOC was positively correlated with MBC, with a much stronger relationship in the managed wetland (Figure 6A). SOC content exhibited a negative relationship with SOC δ13C (Figure 6B), with 13C becoming more depleted as SOC concentration increased. In the managed wetland, SOC concentration was positively related with MBC δ13C (Figure 6C), although no relationship was found in the natural wetland. Finally, the difference between SOC and MBC δ13C was positively correlated to the SOC concentration (Figure 6D). Interestingly, in the managed wetland site MBC tended to be depleted in 13C compared to SOC at concentrations ranging from 0 to ~200 g kg−1, but became increasingly enriched compared to SOC at concentrations >200 g kg−1, up to ~500 g kg−1. No relationship was found between SOC δ13C and MBC δ13C in the natural wetland, and only a weak negative relationship existed for beds [Adj. r2 = 0.15; F(1, 339) = 5.6; P = 0.02] and interbeds [Adj. r2 = 0.24; F(1, 35) = 10.7; P = 0.002] of the managed wetland.
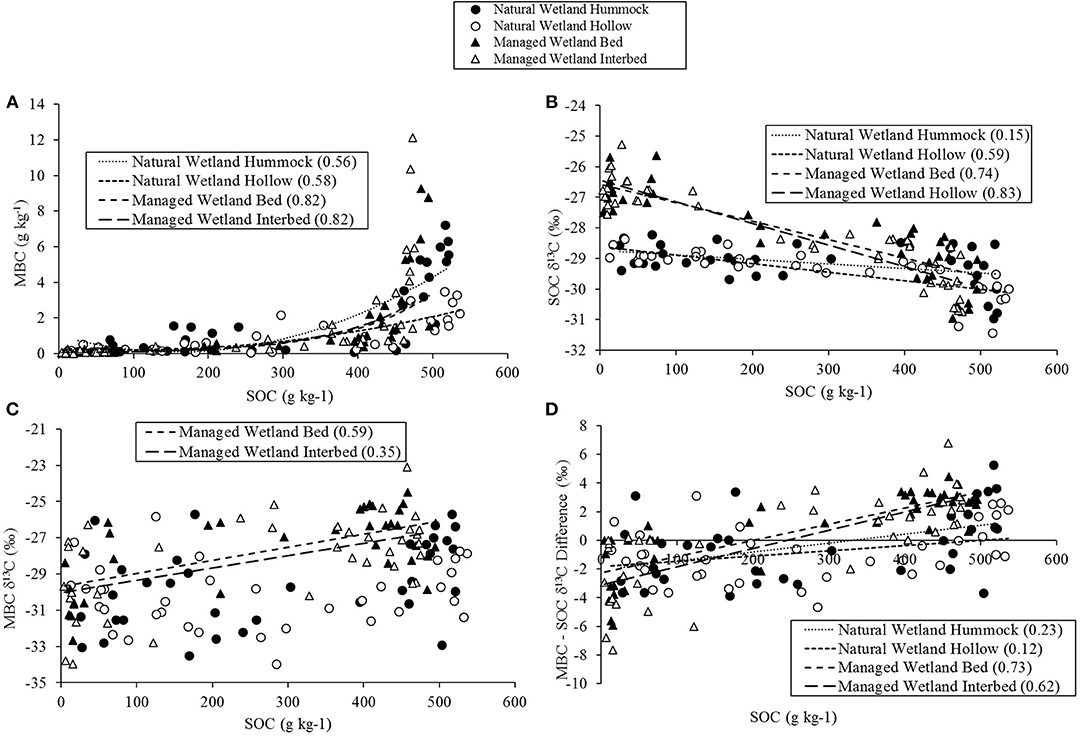
Figure 6. Regression analysis comparing relationships across all soil horizons between soil organic carbon (SOC) and microbial biomass C (MBC) pool size (A), δ13C of SOC (B), δ13C of MBC (C), and (D) the difference in δ13C between MBC and SOC (positive value indicates MBC is enriched in 13C compared to SOC and negative value indicates MBC is depleted in 13C compared to SOC). Adjusted R2- values are presented in parenthesis for any significant correlation found for the four site by micro-topographic location combinations.
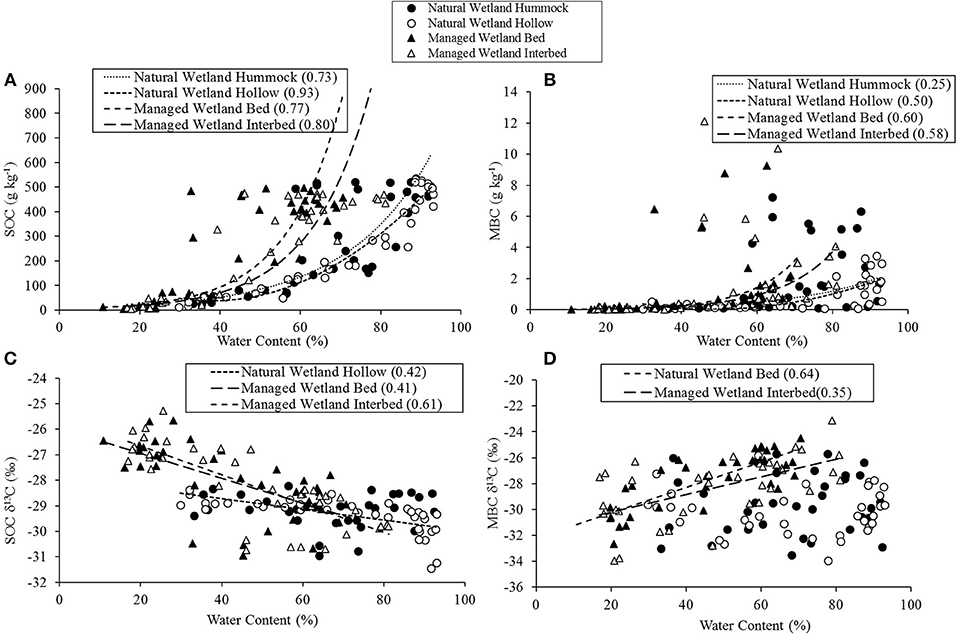
Figure 7. Regression analysis comparing relationships across all soil horizons between soil water content and soil organic carbon (SOC) content (A), microbial biomass C (MBC) (B), δ13C of SOC (C), and δ13C of MBC (D). Adjusted R2-values are presented in parenthesis for any significant correlation found for the four site by micro-topographic location combinations.
Soil water content, expressed as a percentage of total soil, exhibited a positive, exponential relationship with SOC concentration at both sites and micro-topographical positions (Figure 7A). Likewise, soil water content and MBC also had a positive relationship (Figure 7B), but to a lesser extent than that with SOC. A negative correlation was found between soil water content and SOC δ13C, except for that of hummocks in the natural wetland, where no relationship was identified (Figure 7C). Soil water content and MBC δ13C were positively correlated in the managed wetland, while no relationship was found at either micro-topographical position in the natural wetland (Figure 7D). Soil water content also was highly positively correlated with soil organic matter content, across all sites and micro-topographic positions [Adj. r2 = 0.81; F(1, 128) = 534.9; P < 0.0001]. The relationship was even stronger when sites and micro-topographic positions were separated, as indicated by analysis for hummocks [Adj. r2 = 0.88; F(1, 27) = 211.2; P < 0.0001] and hollows [Adj. r2 = 0.92; F(1, 29) = 330.6; P < 0.0001] of the natural wetland, and beds [Adj. r2 = 0.83; F(1, 33) = 170.0; P < 0.0001] and interbeds [Adj. r2 = 0.88; F(1, 33) = 258.0; P < 0.0001] of the managed wetland.
Discussion
Land-use change in coastal wetlands disrupts processes that enhance C storage in these C-rich ecosystems, potentially resulting in release of greenhouse gases from the soil to the atmosphere. In order to predict changes in the SOC balance of drained wetlands, an understanding of how SOC and microbial pools respond is necessary. We hypothesized that drainage would enhance decomposition of existing SOC as increased soil aeration due to water table drawdown would be more favorable to microbial activity, conferring changes in the SOC and MBC pool size and δ13C isotopic signature. As a result of the hypothesized enhancement of microbial activity under drained conditions, enrichment of SOC 13C was expected as new microbial products and biomass (formed under the more favorable decomposition conditions) were incorporated into the SOC pool (Schmidt et al., 2011). This was evident by the 13C of SOC in the managed wetland being more enriched in Oa and mineral horizons, but not in the litter or Oe horizon. By contrast, 13C of SOC in the Oe horizon was enriched in the hummocks of the natural wetland compared to the hollows and the interbed of the managed wetland, although no other micro-topographical effects on SOC pool size or isotopic signature were detected. Furthermore, 13C of MBC in the Oe horizon of hummocks in the natural wetland was the same as that of the managed wetland, indicating the potential contribution of microbial biomass to the SOC pool and its influence on the 13C signature of SOC. Litter and root 13C were more similar to that of SOC with depth in the natural wetland, indicating slowed decomposition and preservation of plant-derived organic matter under more saturated conditions found in the natural wetland. It is important to note that in this study live and dead roots were not separated, but the decrease in root C concentration and enrichment of root 13C with depth at both sites suggest an increasing contribution of dead, partially decomposed roots to the total live + dead root pool with soil depth. Interestingly, MBC in the natural wetland was similar to or greater than that of the managed wetland in the litter and Oe horizon, respectively, with micro-topography as a clear source of spatial heterogeneity. These results provide evidence of the important role hydrology and micro-topography play in controlling SOC patterns in coastal freshwater forested wetlands, and shed light on how drainage of freshwater wetlands in coastal regions alter soil and microbial carbon pools and storage.
Concentration of SOC and MBC decreased rapidly with depth at both wetland sites regardless of micro-topographical position, similar to what has been found in tropical and temperate wetlands (Bernal and Mitsch, 2008; Krüger et al., 2014), as well as in loblolly pine plantations of the lower coastal plains of North Carolina (Minick et al., 2017b). We found that MBC was positively correlated with SOC, as more C can support a larger microbial population (Poret-Peterson et al., 2007), with the greatest degree of variability in MBC in the litter layer. For MBC, concentrations ranged from 0.04 g kg−1 to 7.23 g kg−1 in the natural wetland and from 0.01 to 12.13 g kg−1 in the managed wetland. Our wide ranging values for MBC span litter to organic to mineral soils and are in agreement of soils from other wetland sites (0.9 and 6.0 g kg−1; Groffman et al., 1996; Rokosch et al., 2009), organic soils from upland hardwood forest spodosols (7.7–10.8 g kg−1; Fisk et al., 2015), mineral soils from coastal North Carolina (0.04–0.56 g kg−1; Li et al., 2004; Zhang et al., 2018), forest floor from loblolly pine plantations (8.1–13.7 g kg−1; Allen and Schlesinger, 2004), and mineral soils from loblolly pine plantations across the southeastern US (0.05–0.73 g kg−1; Lee and Jose, 2003; Rifai et al., 2010). In the natural wetland, higher MBC was found in the litter (Oi) and surface organic horizon (Oe) of hummocks compared to hollows. Furthermore, MBC in litter and Oe horizons from hummocks of the natural wetland were similar or higher, respectively, compared to the managed wetland. This suggests that hummocks are biogeochemical hotspots in natural wetlands, acting as localized zones of intense plant and microbial activity with large and biologically active C pools (Kim and Verma, 1992; Belyea, 1996; Belyea and Clymo, 2001; Miao et al., 2017; Minick et al., 2018). These results indicate a robust separation of organic and mineral soil horizons with distinct chemical and biological properties, reflecting the type of C within the soil profile and its chemical properties (Trettin and Jurgensen, 2003).
In support of our second hypothesis, the 13C signature of SOC changed minimally with depth in the natural wetland, but became increasingly enriched with depth at the managed wetland, similar to that observed in upland ecosystems (Wang et al., 2015). This is not necessarily surprising, as hydrologic conditions in wetland soils tend to maintain SOC in a form more closely resembling that of original plant-derived organic matter, whereas less saturated soils have faster decomposition rates which process soil organic matter into amorphous organic materials. von Fischer and Tieszen (1995) and Krüger et al. (2014) found minimal or inconsistent effects of soil depth (down to 60 cm) on the 13C isotopic enrichment of SOC with soil depth in anaerobic soils of forested wetlands in Puerto Rico, while Hobbie et al. (2017) found consistent enrichment of 13C of peat up to ~100 cm depth in a northern forested bog. The slight increase in δ13C below the Oe horizon in the natural wetland suggests some degree of decomposition (Alewell et al., 2011), which was also evident by the lack of fibrous material upon visual inspection of the Oa compared to Oe soil horizons. Further, no differences in δ13C between hummocks and hollows were found below the Oe horizon of the natural wetland, suggesting that these deeper soil horizons were more frequently submerged than the hummock Oe horizon which promotes leaching of water soluble, 13C-enriched organic compounds (e.g., carbohydrates) and retention of lower quality, non-extractable 13C-depleted organic compounds (e.g., lignin, phenol; Lerch et al., 2011). In the managed wetland, 13C enrichment of SOC with depth suggests enhanced decomposition (Natelhoffer and Fry, 1988; von Fischer and Tieszen, 1995) and incorporation of 13C-enriched microbial biomass into SOC (von Fischer and Tieszen, 1995; Schmidt et al., 2011). As SOC concentration increased, 13C of SOC became more depleted, reflecting the presence of 13C-depleted plant-derived chemical constituents of higher C concentration in a less decomposed state (Hobbie et al., 2017). Alternatively, SOC of more decomposed, lower C substrates were 13C-enriched indicating that this C was of a different nature than that of less decomposed, higher C substrates (Hobbie et al., 2017). Enrichment of 13C SOC with depth may also reflect the assimilation of 13C-depleted CO2 into new plant biomass and deposition of this organic matter to surface soils as atmospheric CO2 has become more depleted in 13C over time due to continued burning of fossil fuels (Keeling et al., 2017). In the natural wetland, no difference was found between the δ13C of the Oe surface horizon (~2–10 cm depth, Table 1) and any other surficial soil horizon in either hummocks (above the water table) or hollows (below the water table), suggesting that the decline in δ13C of atmospheric CO2 has not influenced observed patterns of δ13C of SOC with depth at this wetland site. In the managed wetland, the change in δ13C of surface litter to the mineral soil was ~-4‰, while the average change in δ13C of atmospheric CO2 since pre-industrial times (1880's) was −2‰(Keeling et al., 2017). Therefore, this only accounts for ~2‰ of this difference, leaving the remaining 2‰ difference as a result of biological processes during decomposition.
Dominant vegetation type, the associated water-use efficiency (WUE), and water availability have also been shown to impact stable C isotope composition of plant biomass with potential impacts to SOC (Farquhar and Richards, 1984; Ni and Pallardy, 1991). For instance, Peri et al. (2012) suggested that a negative relationship between SOC 13C and water availability resulted from a decline in water-use efficiency at wetter sites promoting depletion of 13C in plant biomass and soil. Alternatively, under drought stress or at drier sites, trees reduce stomatal aperture in order to lessen water loss during photosynthesis, with the effect of reducing photosynthetic 13C isotopic discrimination and enriching 13C of plant biomass and potentially that of SOC (Farquhar and Richards, 1984; Gebrekirstos et al., 2011; Werner et al., 2012). The δ13C values of deciduous and pine tree litter from a temperate forest ranged from −27‰ (Pinus taeda and Pinus echinata) to −30‰ (Nyssa sylvatica) (Garten and Taylor, 1992), although their analyses were of tree species growing along water stressed ridge tops which exhibited higher water-use efficiency and 13C-enriched biomass compared to lower elevation, non-water stressed environments. In the current study, loblolly pine litter from the managed wetland was depleted in 13C (−31.4 ± 0.04‰) compared to that of the natural wetland (−30.0 ± 0.01‰; Table 3), indicating that these pines have lower water-use efficiency as a result of high water availability in comparison to those measured in Garten and Taylor (1992). Furthermore, differences in bulk root 13C between sites were minimal and, as such, 13C of plant tissue does not explain the patterns of 13C enrichment of SOC at the managed wetland. Therefore, the major driver of the observed patterns of SOC 13C with soil depth between the managed and natural wetland is likely the effect of drainage on decomposition dynamics and not nuanced differences between plant species or WUE effects on plant biomass 13C.
The δ13C signature of MBC varied minimally between soil horizons at both wetland sites, but was influenced by drainage and micro-topography. In the organic horizons, 13C of MBC was enriched in the managed wetland compared to the natural wetland, with one major exception. In the natural wetland, 13C of MBC in the Oe horizon from the hummock was similar to that of the managed wetland, but enriched compared to hollows of the natural wetland. The 13C of MBC was also depleted in the mineral horizons compared to the organic horizons of the managed wetland and similar to that of the natural wetland. This may reflect the saturation of mineral horizons of the managed wetland due to a relatively high water table even under drained conditions, given the depths of mineral soils sampled (Table 1) are below the water table numerous times throughout year (Figure 2). We also found that 13C of MBC is generally enriched compared to SOC at high SOC concentrations (primarily in the managed wetland) when SOC more closely resembles litter (or is litter). But as SOC concentration decreased, MBC tended to be depleted in 13C compared to SOC. For example, SOC with concentrations below 200 g kg−1 in the managed wetland (which were associated with mineral soil horizons) were enriched in 13C compared to MBC, while SOC with concentrations above 200 g kg−1 (which were associated with organic soil horizons) were depleted in 13C compared to MBC. By comparison, in the natural wetland, MBC tended to be 13C-depleted compared to SOC for all soil horizons except the Oe. Previous studies have found that 13C of microbial biomass is typically enriched compared to the bulk SOC pool (Dijkstra et al., 2006; Bernal and Mitsch, 2008; Werth and Kuzyakov, 2010; Lerch et al., 2011), but results from our study only partially agree with these observations. The 13C-enrichment of MBC compared to SOC indicates microbes are degrading 13C-enriched biochemical constituents under non-saturated or aerobic conditions (Bowling et al., 2008). Furthermore, the 13C of MBC is expected to parallel that of SOC (Dijkstra et al., 2006), but little to no relationship was found between MBC 13C and SOC 13C in our study. Therefore, these results do not support our third hypothesis, in that 13C of MBC did not necessarily reflect the 13C of SOC. As noted, Dijkstra et al. (2006) found a strong positive correlation between 13C of MBC and SOC in A horizon mineral soils which were characterized by a much smaller range of SOC concentrations (1.4–4.4%) and wider range of 13C (−3.0 to −24.5‰) compared to our study. We are not aware of any studies which compared MBC isotope composition across soil horizons in wetland soils or soils spanning such a large range of SOC concentrations. Therefore, our results shed new light into the pattern of MBC and SOC with soil depth in natural and managed wetland ecosystems and over a wide range of SOC and MBC concentrations, but narrow range of 13C.
Only a few studies have reported depleted 13C signatures of microbial biomass in comparison to SOC (Blair et al., 1985; Wick et al., 2003; Werth and Kuzyakov, 2010). Werth and Kuzyakov (2010) reported that the chloroform-fumigation extraction procedure resulted in an enriched microbial 13C signature compared to bulk soil while the chloroform-fumigation incubation procedure resulted in a depleted microbial 13C signature compared to bulk SOC. The current study utilized the chloroform-fumigation extraction procedure and measured a shift from an enriched to depleted MBC 13C signature with depth at both sites, indicating that these results were not likely due to experimental artifacts. The 13C-depletion of MBC compared to SOC with soil depth likely indicates: (1) microbes have shifted substrate use to more 13C-depleted biochemical constituents under saturated or anaerobic conditions (von Fischer and Tieszen, 1995; Bowling et al., 2008; Werth and Kuzyakov, 2008), and as previously mentioned, leaching of 13C-enriched compounds (e.g., sucrose, starch; Bowling et al., 2008; Lerch et al., 2011) under saturated conditions could be one explanation for the observed patterns of SOC 13C and MBC 13C in the natural wetland; and/or (2) potential shifts in microbial community structure (Boschker et al., 1999).
A change in the microbial community structure could also confer changes in microbial extracellular enzyme production and substrate use (Lerch et al., 2011; Singh et al., 2018), and therefore drive observed patterns of MBC 13C under different environmental conditions found in coastal forests (Boschker et al., 1999; Wang et al., 2015). Soil organic C is also a complex mixture of organic compound classes, which exhibit a range of 13C (Bowling et al., 2008), and therefore changes in the microbial community structure and/or enzyme capacity of the soil microbial community could alter microbial C substrate use and δ13C of microbial biomass. Unpublished data from the natural wetland indicate that fruiting bodies from various fungal groups (e.g., basidiomycetes, saprotrophic fungi) are enriched in 13C compared to SOC, which has been found in numerous other studies (Gleixner et al., 1993; Hobbie et al., 1999), but a shift to a bacterial dominated microbial community is likely under saturated conditions like those found in deeper soil horizons. Dijkstra et al. (2006) and others (Gleixner et al., 1993; Hobbie et al., 1999; Kohzu et al., 1999) have all suggested that various fungal groups (e.g., mycorrhizal, basidiomycetes, saprophytic fungi) are enriched compared to the SOC pool, while some bacterial groups (as measured by PLFA) can be either enriched or depleted compared to the decomposing substrate (Blair et al., 1985; Boschker et al., 1999; Wick et al., 2003; Lerch et al., 2011). Therefore, a shift to a more bacteria-dominated microbial community may also explain the observed shift in MBC to depletion in 13C compared to SOC under saturated conditions. This shift also likely occurs higher up in the soil profile in the natural wetland due to the higher water table, as indicated by the more depleted MBC in the upper soil horizons of the natural wetland compared to the managed wetland.
In conclusion, we found that hydrology, and to a lesser degree micro-topography, was important in shaping the size and stable C isotopic signature of soil and microbial C pools in these natural and managed coastal freshwater forested wetlands. We have highlighted that within the natural wetland, characterized by hummock-hollow micro-topography, surface soils of hummocks are a highly active zone of microbial soil C processing, comparable to or greater than that of drained wetlands. Our data suggest that management of wetlands for loblolly pine production leads to more advanced breakdown of SOC, although the effects of this on the total ecosystem C balance remains unknown. The decreased saturation of soils in drained wetlands likely leads to more processing of organic matter compared to the natural wetland (Kasimir et al., 2018), and therefore a greater contribution of microbes to the soil organic matter pool over time, resulting in the observed enrichment of 13C in SOC with depth. It is possible that enhanced breakdown of SOC in managed wetlands may be counterbalanced by the growth of highly productive loblolly pine trees and enhanced above- (e.g., litterfall) and below-ground (e.g., root productivity and turnover) inputs (Noormets et al., 2015), but this will require future studies to characterize all C pools in the critical zone of these ecosystems (Minkkinen and Laine, 1998). Taken together, our results suggest that saturated soils in natural forested wetland ecosystems along the lower coastal plains of the southeastern US influence C isotopic composition across the soil profile by slowing decomposition of plant-derived C compounds, and alter the relationships between soil chemical and biological properties that are typically observed in non-wetland ecosystems (Dijkstra et al., 2006; Bernal and Mitsch, 2008; Werth and Kuzyakov, 2010). As coastal wetlands are rapidly being altered by humans, and simultaneous threatened by climate change and sea level rise, understanding the relationships between organic C inputs, microbial community composition and function, as influenced by hydrology and management, is fundamental to our capacity to project impacts on regional-to-global SOC cycling and storage. Essential to this challenge will be to expand such studies to other similar sites across the region to increase confidence in the conclusions and practical application of these results.
Author Contributions
All authors contributed to the conception and design of the study. KM wrote the first draft of the manuscript. KM and XL collected the samples from the field. KM performed laboratory analysis. BM performed the water table analysis. All authors contributed to manuscript revision and approved the submitted version.
Conflict of Interest Statement
The authors declare that the research was conducted in the absence of any commercial or financial relationships that could be construed as a potential conflict of interest.
Acknowledgments
We thank the two reviewers whose comments greatly improved this manuscript. Support for this project was provided by: US DOE National Institute of Climatic Change Research (08-SC-NICCR-1072), the USDA Forest Service Eastern Forest Environmental Threat Assessment Center (13-JV-11330110-081), US DOE Terrestrial Ecosystems Program (11-DE-SC0006700), USDA NIFA Carbon Cycle Science (2014-67003-22068), and the Ameriflux Management Project Core Site (M1900080). Significant in-kind operational support was provided by the U.S. Fish and Wildlife Service, Alligator River National Wildlife Refuge.
References
Alewell, C., Giesler, R., Klaminder, J., Leifeld, J., and Rollog, M. (2011). Stable carbon isotopes as indicators for environmental change in palsa peats. Biogeosciences 8, 1769–1778. doi: 10.5194/bg-8-1769-2011
Allen, A., and Schlesinger, W. (2004). Nutrient limitations to soil microbial biomass and activity in loblolly pine forests. Soil Biol. Biochem. 36, 581–589. doi: 10.1016/j.soilbio.2003.12.002
Allen, T., Wang, Y., Gore, B., Swords, J., and Newcomb, D. (2011). “Coastal wetland mapping using time series SAR imagery and LiDAR: alligator river national wildlife refuge, North Carolina,” in Proceedings, Pecora 18 symposium, Herndon, VA, 14–17.
Armentano, T., and Menges, E. (1986). Patterns of change in the carbon balance of organic soil-wetlands of the temperate zone. J. Ecol. 74, 755–774. doi: 10.2307/2260396
Belyea, L. R. (1996). Separating the effects of litter quality and microenvironment on decomposition rates in a patterned peatland. Oikos 77, 529–539. doi: 10.2307/3545942
Belyea, L. R., and Clymo, R. S. (2001). Feedback control of the rate of peat formation. Proc. Biol. Sci. R. Soc. 268, 1315–1321. doi: 10.1098/rspb.2001.1665
Benner, R., Marilyn, L., Fogel, E., Sprague, K., and Hodson, R. E. (1987). Depletion of 13C in lignin and its implications for stable carbon isotope studies. Nature 329, 708–710. doi: 10.1038/329708a0
Bernal, B., and Mitsch, W. J. (2008). A comparison of soil carbon pools and profiles in wetlands in Costa Rica and Ohio. Ecol. Eng. 34, 311–323. doi: 10.1016/j.ecoleng.2008.09.005
Blair, N., Leu, A., Munoz, E., Olsen, J., Kwong, E., and Marais, D. (1985). Carbon isotopic fractionation in heterotrophic microbial metabolism. Appl. Environ. Microbiol. 50, 996–1001.
Boschker, H. T. S., De Brouwer, J. F. C., and Cappenberg, T. E. (1999). The contribution of macrophyte-derived organic matter to microbial biomass in salt-marsh sediments: Stable carbon isotope analysis of microbial biomarkers. Limnol. Oceanogr. 44, 309–319. doi: 10.4319/lo.1999.44.2.0309
Bowling, D. R., Pataki, D. E., and Randerson, J. T. (2008). Carbon isotopes in terrestrial ecosystem pools and CO2 fluxes. New Phytol. 178, 24–40. doi: 10.1111/j.1469-8137.2007.02342.x
Coyle, J. S., Dijkstra, P., Doucett, R. R., Schwartz, E., Hart, S. C., and Hungate, B. A. (2009). Relationships between C and N availability, substrate age, and natural abundance 13C and 15N signatures of soil microbial biomass in a semiarid climate. Soil Biol. Biochem. 41, 1605–1611. doi: 10.1016/j.soilbio.2009.04.022
Dahl, T. E. (1990). Wetland Losses in the United States 1780's to 1980's. U.S. Department of the Interior. Fish and Wildlife Service, Washington, D.C. 21.
Dahl, T. E. (2011). Status and Trends of Wetlands in the Conterminous United States 2004–2009. U.S. Department of Interior, Fish and Wildlife Service, Washington, DC.
Dahl, T. E., Johnson, C. E., and Frayer, W. (1991). Wetlands, Status and Trends in the Conterminous United States Mid-1970's to Mid-1980's. U.S. Department of Interior, Fish and Wildlife Service, Washington, DC.
Dai, Z., Trettin, C. C., Li, C., Li, H., Sun, G., and Amatya, D. M. (2012). Effect of assessment scale on spatial and temporal variations in CH4, CO2, and N2O fluxes in a forested wetland. Water Air Soil Pollut. 223, 253–265. doi: 10.1007/s11270-011-0855-0
Day, F. P., and Megonigal, J. P. (1993). The relationship between variable hydroperiod, production allocation, and belowground organic turnover in forested wetlands. Wetlands 13, 115–121. doi: 10.1007/BF03160871
Dijkstra, P., Ishizu, A., Doucett, R., Hart, S. C., Schwartz, E., Menyailo, O. V., et al. (2006). 13C and 15N natural abundance of the soil microbial biomass. Soil Biol. Biochem. 38, 3257–3266. doi: 10.1016/j.soilbio.2006.04.005
Domec, J. C., King, J. S., Ward, E., Oishi, A. C., Palmroth, S., Radecki, A., et al. (2015). Conversion of natural forests to managed forest plantations decreases tree resistance to prolonged droughts. Forest Ecol. Manage. 355, 58–71. doi: 10.1016/j.foreco.2015.04.012
Ehleringer, J. R., Buchmann, N., and Flanagan, L. B. (2000). Carbon isotope ratios in belowground carbon cycle processes. Ecol. Appl. 10, 412–422. doi: 10.1890/1051-0761(2000)010[0412:CIRIBC]2.0.CO;2
Esmeijer-Liu, A. J., Kürschner, W. M., Lotter, A. F., Verhoeven, J. T., and Goslar, T. (2012). Stable carbon and nitrogen isotopes in a peat profile are influenced by early stage diagenesis and changes in atmospheric CO2 and N deposition. Water Air Soil Pollut. 223, 2007–2022. doi: 10.1007/s11270-011-1001-8
Farquhar, G., and Richards, R. (1984). Isotopic composition of plant carbon correlates with water-use efficiency of wheat genotypes. Funct. Plant Biol. 11, 539–552. doi: 10.1071/PP9840539
Fenner, N., and Freeman, C. (2011). Drought-induced carbon loss in peatlands. Nat. Geosci. 4, 895–900. doi: 10.1038/ngeo1323
Fisk, M., Santangelo, S., and Minick, K. (2015). Carbon mineralization is promoted by phosphorus and reduced by nitrogen addition in the organic horizon of northern hardwood forests. Soil Biol. Biochem. 81, 212–218. doi: 10.1016/j.soilbio.2014.11.022
Fox, T. R. (2000). Sustained productivity in intensively managed forest plantations. Forest Ecol. Manage. 138, 187–202. doi: 10.1016/S0378-1127(00)00396-0
Fox, T. R., Jokela, E. J., and Allen, H. (2004). “The evolution of pine plantation silviculture in the southern United States,” in Gen. Tech. Rep. SRS 75. Asheville, NC: US Department of Agriculture, Forest Service, Southern Research Station. Chapter 8. p. 63–82.
Fox, T. R., Jokela, E. J., and Allen, H. L. (2007). The development of pine plantation silviculture in the Southern United States. J Forest 105, 337–347. doi: 10.1093/jof/105.7.337
Freeman, C., Ostle, N., Fenner, N., and Kang, H. (2004). A regulatory role for phenol oxidase during decomposition in peatlands. Soil Biol. Biochem. 36, 1663–1667. doi: 10.1016/j.soilbio.2004.07.012
Garten, C., and Taylor, G. (1992). Foliar δ13C within a temperate deciduous forest: spatial, temporal, and species sources of variation. Oecologia 90, 1–7. doi: 10.1007/BF00317801
Gebrekirstos, A., van Noordwijk, M., Neufeldt, H., and Mitlöhner, R. (2011). Relationships of stable carbon isotopes, plant water potential and growth: an approach to asses water use efficiency and growth strategies of dry land agroforestry species. Trees 25, 95–102. doi: 10.1007/s00468-010-0467-0
Gleixner, G., Danier, H. J., Werner, R. A., and Schmidt, H. L. (1993). Correlations between the 13C content of primary and secondary plant products in different cell compartments and that in decomposing basidiomycetes. Plant Physiol. 102, 1287–1290. doi: 10.1104/pp.102.4.1287
Gorham, E. (1991). Northern peatlands: role in the carbon cycle and probable responses to climatic warming. Ecol. Appl. 1, 182–195. doi: 10.2307/1941811
Groffman, P. M., Hanson, G. C., Kiviat, E., and Stevens, G. (1996). Variation in microbial biomass and activity in four different wetland types. Soil Sci. Soc. Am. J. 60, 622–629. doi: 10.2136/sssaj1996.03615995006000020041x
Hinton, J. W., Chamberlain, M. J., and Rabon, D. R. (2013). Red wolf (Canis rufus) recovery: a review with suggestions for future research. Animals 3, 722–744. doi: 10.3390/ani3030722
Hobbie, E. A., Chen, J., Hanson, P. J., Iversen, C. M., McFarlane, K. J., Thorp, N. R., et al. (2017). Long-term carbon and nitrogen dynamics at SPRUCE revealed through stable isotopes in peat profiles. Biogeosciences 14, 2481–2494. doi: 10.5194/bg-14-2481-2017
Hobbie, E. A., Macko, S. A., and Shugart, H. H. (1999). Insights into nitrogen and carbon dynamics of ectomycorrhizal and saprotrophic fungi from isotopic evidence. Oecologia 118, 353–360. doi: 10.1007/s004420050736
Holden, J., Chapman, P., and Labadz, J. (2004). Artificial drainage of peatlands: hydrological and hydrochemical process and wetland restoration. Progr. Phys. Geogr. 28, 95–123. doi: 10.1191/0309133304pp403ra
Jansson, Å., Folke, C., and Langaas, S. (1998). Quantifying the nitrogen retention capacity of natural wetlands in the large-scale drainage basin of the Baltic Sea. Landscape Ecol. 13, 249–262. doi: 10.1023/A:1008020506036
Joergensen, R. G. (1996). The fumigation-extraction method to estimate soil microbial biomass: calibration of the kEC value. Soil Biol. Biochem. 28, 25–31. doi: 10.1016/0038-0717(95)00102-6
Johnson, M. G., and Kern, J. S. (2003). “Quantifying the organic carbon held in forested soils of the United States and Puerto Rico,” in The Potential of U.S. Forest Soils to Sequester Carbon and Mitigate the Greenhouse Effect, eds J. M. Kimble, L. S. Heath, R. A. Birdsey, R. Lal (Boca Raton, FL: CRC Press), 47–72.
Kasimir, Å., He, H., Coria, J., and Nordén, A. (2018). Land use of drained peatlands: Greenhouse gas fluxes, plant production, and economics. Global Change Biol. 24, 3302–3316. doi: 10.1111/gcb.13931
Keeling, R. F., Graven, H. D., Welp, L. R., Resplandy, L., Bi, J., Piper, S. C., et al. (2017). Atmospheric evidence for a global secular increase in carbon isotopic discrimination of land photosynthesis. Proc. Natl Acad. Sci. USA. 114, 10361–10366. doi: 10.1073/pnas.1619240114
Keiluweit, M., Wanzek, T., MKleber, M., Nico, P., and Fendorf, S. (2017). Anaerobic microsites have an unaccounted role in soil carbon stabilization. Nat. Commun. 8, 1–10. doi: 10.1038/s41467-017-01406-6
Kim, J., and Verma, S. B. (1992). Soil surface CO2 flux in a Minnesota peatland. Biogeochemistry 18, 37–51. doi: 10.1007/BF00000425
Kohzu, A., Yoshioka, T., Ando, T., Takahashi, M., Koba, K., and Wada, E. (1999). Natural 13C and 15N abundance of field-collected fungi and their ecological implications. New Phytol. 144, 323–330. doi: 10.1046/j.1469-8137.1999.00508.x
Krüger, J., Leifeld, J., and Alewell, C. (2014). Degradation changes stable carbon isotope depth profiles in palsa peatlands. Biogeosciences 11, 3369–3380. doi: 10.5194/bg-11-3369-2014
Lal, R. (2005). Forest soils and carbon sequestration. Forest Ecol. Manage. 220, 242–258. doi: 10.1016/j.foreco.2005.08.015
Lee, K., and Jose, S. (2003). Soil respiration, fine root production, and microbial biomass in cottonwood and loblolly pine plantations along a nitrogen fertilization gradient. Forest Ecol. Manage. 185, 263–273. doi: 10.1016/S0378-1127(03)00164-6
Lerch, T. Z., Nunan, N., Dignac, M.-F., Chenu, C., and Mariotti, A. (2011). Variations in microbial isotopic fractionation during soil organic matter decomposition. Biogeochemistry 106, 5–21. doi: 10.1007/s10533-010-9432-7
Li, Q., Allen, H. L., and Wollum, I. I. A. G. (2004). Microbial biomass and bacterial functional diversity in forest soils: effects of organic matter removal, compaction, and vegetation control. Soil Biol. Biochem. 36, 571–579. doi: 10.1016/j.soilbio.2003.12.001
Littell, R. C., Pendergast, J., and Natarajan, R. (2000). Modelling covariance structure in the analysis of repeated measures data. Stat. Med. 19, 1793–1819. doi: 10.1002/1097-0258(20000715)19:13<1793::AID-SIM482>3.0.CO;2-Q
Melillo, J. M., Aber, J. D., Linkins, A. E., Ricca, A., Fry, B., and Nadelhoffer, K. J. (1989). “Carbon and nitrogen dynamics along the decay continuum: plant litter to soil organic matter,” in Ecology of Arable Land, eds M. Bergstrom and L. Bergstrom (Clarholm: Kluwer Academic Publishers), 53–62.
Miao, G. (2013). A Multi-Scale Study on Respiratory Processes in a Lower Coastal Plain Forested Wetland in the Southeastern United States. Dissertation, North Carolina State University.
Miao, G., Noormets, A., Domec, J.-C., Fuentes, M., Trettin, C. C., Sun, G., et al. (2017). Hydrology and microtopography control carbon dynamics in wetlands: implications in partitioning ecosystem respiration in a coastal plain forested wetland. Agric. For. Meteorol. 247, 343–355. doi: 10.1016/j.agrformet.2017.08.022
Minick, K. J., Fisk, M. C., and Groffman, P. M. (2017a). Soil Ca alters processes contributing to C and N retention in the Oa/A horizon of a northern hardwood forest. Biogeochemistry 132, 343–357. doi: 10.1007/s10533-017-0307-z
Minick, K. J., Kelley, A. M., Miao, G., Li, X., Noormets, A., Mitra, B., et al. (2018). Microtopography alters hydrology, phenol oxidase activity and nutrient availability in organic soils of a coastal freshwater forested wetland. Wetlands 1–11. doi: 10.1007/s13157-018-1107-5
Minick, K. J., Leggett, Z. H., Sucre, E. B., Fox, T. R., and Strahm, B. D. (2017b). Soil and aggregate-associated carbon in a young loblolly pine plantation: influence of bioenergy intercropping. Soil Sci. 182, 233–240. doi: 10.1097/SS.0000000000000215
Minick, K. J., Pandey, C., Fox, T. R., and Subedi, S. (2016). Dissimilatory nitrate reduction to ammonium and N2O flux: effect of soil redox potential and N fertilization in loblolly pine forests. Biol. Fertil. Soils 52, 1–14. doi: 10.1007/s00374-016-1098-4
Minick, K. J., Strahm, B. D., Fox, T. R., Sucre, E. B., and Leggett, Z. H. (2015). Microbial nitrogen cycling response to forest-based bioenergy production. Ecol. Appl. 25, 2366–2381. doi: 10.1890/14-1745.1
Minick, K. J., Strahm, B. D., Fox, T. R., Sucre, E. B., Leggett, Z. H., and Zerpa, J. L. (2014). Switchgrass intercropping reduces soil inorganic nitrogen in a young loblolly pine plantation located in coastal North Carolina. For. Ecol. Manage. 319:161–168. doi: 10.1016/j.foreco.2014.02.013
Minkkinen, K., and Laine, J. (1998). Long-term effect of forest drainage on the peat carbon stores of pine mires in Finland. Canad. J. Forest Res. 28, 1267–1275. doi: 10.1139/x98-104
Mitsch, W. J., and Gosselink, J. G. (2000). The value of wetlands: importance of scale and landscape setting. Ecol. Econom. 35, 25–33. doi: 10.1016/S0921-8009(00)00165-8
Natelhoffer, K. J., and Fry, B. (1988). Controls on natural nitrogen-15 and carbon-13 abundances in forest soil organic matter. Soil Sci. Soc. Am. J. 52, 1633–1640. doi: 10.2136/sssaj1988.03615995005200060024x
Neely, C. L., Beare, M. H., Hargrove, W. L., and Coleman, D. C. (1991). Relationships between fungal and bacterial substrate-induced respiration, biomass and plant residue decomposition. Soil Biol. Biochem. 23, 947–954. doi: 10.1016/0038-0717(91)90175-J
Ni, B., and Pallardy, S. G. (1991). Response of gas exchange to water stress in seedlings of woody angiosperms. Tree Physiol. 8, 1–9. doi: 10.1093/treephys/8.1.1
Noormets, A., Epron, D., Domec, J. C., McNulty, S. G., Fox, T. R., Sun, G., et al. (2015). Effects of forest management on productivity and carbon sequestration: a review and hypothesis. Forest Ecol. Manage. 355, 124–140. doi: 10.1016/j.foreco.2015.05.019
Noormets, A., Gavazzi, M. J., McNulty, S. G., Domec, J. C., Sun, G., King, J. S., et al. (2010). Response of carbon fluxes to drought in a coastal plain loblolly pine forest. Global Change Biol. 16, 272–287. doi: 10.1111/j.1365-2486.2009.01928.x
Noormets, A., McNulty, S. G., Domec, J.-C., Gavazzi, M., Sun, G., and King, J. S. (2012). The role of harvest residue in rotation cycle carbon balance in loblolly pine plantations. Respiration partitioning approach. Glob. Change Biol. 18, 3186–3201. doi: 10.1111/j.1365-2486.2012.02776.x
O'brien, B. J., and Stout, J. D. (1978). Movement and turnover of soil organic matter as indicated by carbon isotope measurements. Soil Biol. Biochem. 10, 309–317. doi: 10.1016/0038-0717(78)90028-7
Peri, P. L., Ladd, B., Pepper, D. A., Bonser, S. P., Laffan, S. W., and Amelung, W. (2012). Carbon (δ13C) and nitrogen (δ15N) stable isotope composition in plant and soil in southern patagonia's native forests. Global Change Biol. 18, 311–321. doi: 10.1111/j.1365-2486.2011.02494.x
Poret-Peterson, A. T., Ji, B., Engelhaupt, E., and Gulledge, J. (2007). Soil microbial biomass along a hydrologic gradient in a subsiding coastal bottomland forest: implications for future subsidence and sea-level rise. Soil Biol. Biochem. 39, 641–645. doi: 10.1016/j.soilbio.2006.09.016
Richardson, C. J. (1994). Ecological functions and human values in wetlands: a framework for assessing forestry impacts. Wetlands 14, 1–9. doi: 10.1007/BF03160616
Rifai, S. W., Markewitz, D., and Borders, B. (2010). Twenty years of intensive fertilization and competing vegetation suppression in loblolly pine plantations: impacts on soil C, N, and microbial biomass. Soil Biol. Biochem. 42, 713–723. doi: 10.1016/j.soilbio.2010.01.004
Riggs, S. R. (1996). Sediment evolution and habitat function of organic-rich muds within the Albemarle estuarine system, North Carolina. Estuaries 19:169–185. doi: 10.2307/1352223
Rokosch, A. E., Bouchard, V., Fennessy, S., and Dick, R. (2009). The use of soil parameters as indicators of quality in forested depressional wetlands. Wetlands 29, 666–677. doi: 10.1672/08-150.1
Sainju, U. M., and Singh, B. P. (2008). Nitrogen storage with cover crops and nitrogen fertilization in tilled and nontilled soils. Agron. J. 100, 619–627. doi: 10.2134/agronj2007.0236
Schabenberger, O., and Pierce, F. J. (2001). Contemporary Statistical Models for the Plant and Soil Sciences. Boca Raton, FL: CRC Press. doi: 10.1201/9781420040197
Schmidt, M. W., Torn, M, S., Abiven, S., Dittmar, T., Guggenberger, G., Janssens, I. A., et al. (2011). Persistence of soil organic matter as an ecosystem property. Nature 478:49. doi: 10.1038/nature10386
Segnini, A., de Souza, A. A., Novotny, E. H., Bonagamba, T. J., Posadas, A., Quiroz, R., et al. (2013). Characterization of peatland soils from the high Andes through 13C nuclear magnetic resonance spectroscopy. Soil Sci. Soc. Am. J. 77, 673–679. doi: 10.2136/sssaj2012.0291
Singh, B., Minick, K, J., Strickland, M. S., Wickings, K. G., Crippen, T. L., Tarone, A. M., et al. (2018). Temporal and spatial impact of human cadaver decomposition on soil bacterial and arthropod community structure and function. Front. Microbiol. 8:2616. doi: 10.3389/fmicb.2017.02616
Snodgrass, J. W., Komoroski, M. J., Bryan, A. L., and Burger, J. (2000). Relationships among isolated wetland size, hydroperiod, and amphibian species richness: implications for wetland regulations. Conserv. Biol. 14, 414–419. doi: 10.1046/j.1523-1739.2000.99161.x
Sun, G., Noormets, A., Gavazzi, M. J., McNulty, S. G., Chen, J., Domec, J. C., et al. (2010). Energy and water balance of two contrasting loblolly pine plantations on the lower coastal plain of North Carolina, USA. For. Ecol. Manag. 259, 1299–1310. doi: 10.1016/j.foreco.2009.09.016
Tanentzap, A. J., Szkokan-Emilson, E. J., Kielstra, B. W., Arts, M. T., Yan, N. D., and Gunn, J. M. (2014). Forests fuel fish growth in freshwater deltas. Nat. Commun. 5:4077. doi: 10.1038/ncomms5077
Tiner, R. W. Jr. (1984). Wetlands of the United States: Current Status and Recent Trends. U.S. Department of Interior, Fish and Wildlife Service, Washington, DC.
Toberman, H., Freeman, C., Artz, R., Evans, C., and Fenner, N. (2008). Impeded drainage stimulates extracellular phenol oxidase activity in riparian peat cores. Soil Use Manage. 24, 357–365. doi: 10.1111/j.1475-2743.2008.00174.x
Toberman, H., Laiho, R., Evans, C., Artz, R., Fenner, N., Straková, P., et al. (2010). Long-term drainage for forestry inhibits extracellular phenol oxidase activity in Finnish boreal mire peat. Eur. J. Soil Sci. 61, 950–957. doi: 10.1111/j.1365-2389.2010.01292.x
Trettin, C. C., Davidian, M., Jurgensen, M. F., and Lea, R. (1996). Organic matter decomposition following harvesting and site preparation of a forested wetland. Soil Sci. Soc. Am. J. 60, 1994–2003. doi: 10.2136/sssaj1996.03615995006000060053x
Trettin, C. C., and Jurgensen, M. F. (2003). “Carbon cycling in wetland forest soils,” in The Potential of U.S. Forest Soils to Sequester Carbon and Mitigate the Greenhouse Effect, eds J. M. Kimble, L. S. Heath, R. A. Birdsey, and R. Lal (Boca Raton, FL: CRC Press), 311–331.
USDA Soil Survey Staff (2008). Natural Resources Conservation Service. United States Department of Agriculture. Official Soil Series Descriptions Available online at: https://soilseries.sc.egov.usda.gov/OSD_Docs/B/BELHAVEN.html
Vance, E. D., Brookes, P. C., and Jenkinson, D. S. (1987). An extraction method for measuring soil microbial biomass-C. Soil Biol. Biochem. 19, 703–707. doi: 10.1016/0038-0717(87)90052-6
von Fischer, J. C., and Tieszen, L. L. (1995). Carbon isotope characterization of vegetation and soil organic matter in subtropical forests in Luquillo, Puerto Rico. Biotropica 27, 138–148. doi: 10.2307/2388989
Wang, G., Jia, Y., and Li, W. (2015). Effects of environmental and biotic factors on carbon isotopic fractionation during decomposition of soil organic matter. Sci. Rep. 5, 1–11. doi: 10.1038/srep11043
Werner, C., Schnyder, H., Cuntz, M., Keitel, C., Zeeman, M. J., Dawson, T. E., et al. (2012). Progress and challenges in using stable isotopes to trace plant carbon and water relations across scales. Biogeosciences 9, 3083–3111. doi: 10.5194/bg-9-3083-2012
Werth, M., and Kuzyakov, Y. (2008). Root-derived carbon in soil respiration and microbial biomass determined by 14C and 13C. Soil Biol. Biochem. 40, 625–637. doi: 10.1016/j.soilbio.2007.09.022
Werth, M., and Kuzyakov, Y. (2010). 13C fractionation at the root–microorganisms–soil interface: A review and outlook for partitioning studies. Soil Biol. Biochem. 42, 1372–1384. doi: 10.1016/j.soilbio.2010.04.009
Wick, L. Y., Pasche, N., Bernasconi, S. M., Pelz, O., and Harms, H. (2003). Characterization of multiple-substrate utilization by anthracene-degrading mycobacterium frederiksbergense LB501T. Appl. Environ. Microbiol. 69, 6133–6142. doi: 10.1128/AEM.69.10.6133-6142.2003
Yu, K., Faulkner, S. P., and Patrick, W. H. (2006). Redox potential characterization and soil greenhouse gas concentration across a hydrological gradient in a Gulf coast forest. Chemosphere 62, 905–914. doi: 10.1016/j.chemosphere.2005.05.033
Yu, Z., Beilman, D., Frolking, S., MacDonald, G. M., Roulet, N. T., Camill, P., et al. (2011). Peatlands and their role in the global carbon cycle. Eos Trans. Am. Geophys. Union 92, 97–98. doi: 10.1029/2011EO120001
Keywords: wetland drainage, microbial biomass carbon, loblolly pine silviculture, microtopography, δ13C, soil organic carbon, land-use change
Citation: Minick KJ, Mitra B, Li X, Noormets A and King JS (2019) Water Table Drawdown Alters Soil and Microbial Carbon Pool Size and Isotope Composition in Coastal Freshwater Forested Wetlands. Front. For. Glob. Change 2:7. doi: 10.3389/ffgc.2019.00007
Received: 14 January 2019; Accepted: 25 March 2019;
Published: 17 April 2019.
Edited by:
Salli F. Dymond, University of Minnesota Duluth, United StatesReviewed by:
Matthew Vadeboncoeur, University of New Hampshire, United StatesCourtney M. Siegert, Mississippi State University, United States
Copyright © 2019 Minick, Mitra, Li, Noormets and King. This is an open-access article distributed under the terms of the Creative Commons Attribution License (CC BY). The use, distribution or reproduction in other forums is permitted, provided the original author(s) and the copyright owner(s) are credited and that the original publication in this journal is cited, in accordance with accepted academic practice. No use, distribution or reproduction is permitted which does not comply with these terms.
*Correspondence: Kevan J. Minick, a2ptaW5pY2tAbmNzdS5lZHU=