- 1Department of Psychology, Neuroscience and Behaviour, McMaster University, Hamilton, ON, Canada
- 2Department of Biology, McMaster University, Hamilton, ON, Canada
- 3Department of Integrative Biology, University of Guelph, Guelph, ON, Canada
The round goby (Neogobius melanostomus) is the fastest spreading invasive fish in the Laurentian Great Lakes, and concurrent invasions are occurring globally. To inform management and mitigation strategies, metabolic rate of adult round goby has been rigorously quantified, but metabolism of the juvenile life stages represents an important knowledge gap. Here, we measured routine and maximum metabolic rates (RMR and MMR) of early juvenile round goby (mass 0.007–0.07 g, mean 0.02 g; total length 6.6–19.4 mm, mean 13.7 mm), ~100× smaller than any round goby measured previously. To understand how metabolism may be affected by a warming climate, we reared round goby at several ecologically relevant temperatures (15°C, 19°C, and 23°C) and a projected surface temperature under climate change scenarios (27°C). We tested early juveniles at two separate and distinct ages (6–7 weeks and 12–14 weeks post-hatch). Growth was highest at 23°C compared to 15°C and 27°C, but this pattern only emerged after 12–14 weeks of development. Routine metabolic rates increased over 2-fold between fish reared at 15°C vs. 27°C (Q10 = 1.98), but we found no clear difference in our estimation of the “scope for activity” (i.e., maximum—routine metabolic rate) across temperatures, suggesting a wide thermal performance curve. We also found that the bioenergetics models previously developed for adults do not effectively translate to juveniles; the current models underestimated observed RMR values by ~20–60% depending on temperature. Overall, data from this study can be used to improve bioenergetic modeling in the round goby by considering these earlier life stages and fill an important gap of knowledge in our understanding of an ecologically invasive species.
1 Introduction
The spread of invasive species and a changing global climate represent two major environmental threats (1–3, 7). Invasive species cause harm by disrupting ecological niches, competing with and consuming native species, and even driving local species extinctions (4–7). Invasive fresh- and marine-water fishes often grow and reproduce at faster rates when compared to native fish species (8), and consume a more generalist diet (9), contributing to their competitiveness and pervasiveness. The ecological impacts of invasive species may be exacerbated by climate change, as invasive species may be tolerant of larger temperature ranges relative to native fish species (10, 11). For example, in the northwestern United States, warming water temperatures have been proposed to have driven the decline of native bull trout (Salvelinus confluentus) populations, while simultaneously contributing to the range-expansion of invasive brown trout (Salmo trutta) (7).
One invasive species that may particularly benefit from a warming climate is the round goby (Neogobius melanostomus). This is a benthic teleost fish that is native to the Black and Caspian Seas of Eurasia (12). The round goby has inadvertently been introduced across the Northern Hemisphere by humans (e.g., via ballast water of transoceanic ships) (13) and has since rapidly spread throughout all five Laurentian (North American) Great Lakes, the Baltic Sea, and several rivers and lakes across Europe and North America (12, 14). In the North American Great Lakes, the round goby has had the fastest range expansion compared to any other invasive fish species (12). The round goby establishment presents many ecological problems. For example, the round goby outcompetes native fishes for food and shelter, but also consumes the eggs of other fishes, posing ecological and economic concerns for sport and commercially important fish species (12, 15). Round goby prey upon benthic invertebrates, which presents a way for harmful once-sequestered contaminants to travel up trophic levels to species that consume round goby (16). For example, mercury levels in smallmouth bass (Micropterus dolomieu) bioaccumulated following the invasion of the round goby (17), and round goby are also thought to spread botulism to birds (18, 19). Controlling the spread of the round goby into new environments, and managing populations that are already established, is important for the health of aquatic ecosystems and native fish populations.
Water temperatures are expected to rise globally, and in at least some of the Great Lakes (e.g., Lake Ontario and Lake Erie), the average lake-wide summertime surface water temperatures are projected to reach as high as 27°C by the turn of the century (2071–2100), though shallow inshore areas may get even warmer than this (20). Warming water temperatures may provide an advantage to the invasive round goby over native fishes, as they have been shown to exhibit a broad range of thermal tolerances (−1 to 30°C) (21) and experience warmer temperatures in their native range compared to many of the habitats in which they are invasive. For example, the southern Caspian Sea has summer surface water temperatures that are considerably higher between 25 to 29°C (22), compared to the Great Lakes summer surface water temperatures (~15 to 23°C) (23). Nevertheless, our understanding of the thermal physiology of round goby is primarily limited to adult stages. A bioenergetics model has previously been developed for adult round goby (mass ≥ 2 g) (24), with methods identified to quantify the likelihood and capacity for natural dispersion (25–27). However, these modeling studies are limited by a lack of empirical knowledge of early life stages that could be primarily responsible for the invasion success of this species (28). This presents a large gap in our knowledge of an incredibly successful and damaging invasive species.
One of the most important variables used for bioenergetic modeling is metabolic rate, which is indicative of overall energy flux through an animal. Respirometry is a common method used to measure metabolism in fishes and other organisms and typically involves measuring the rate of oxygen consumption (MO2) as a proxy for metabolic rates (29–32). Measures of metabolic rate can inform many aspects of life history, such as growth rates, reproduction rates, capacity for dispersal and range expansion, etc. (33, 34). In fishes, metabolic rate is highly sensitive to temperature, although this sensitivity can depend on the developmental stage of the organism (29, 31, 35). Thus, metabolic data for juvenile round goby would be valuable for improving current bioenergetics models, ultimately expanding our capacity to predict the impact of juvenile round goby on food web dynamics, patterns of growth, fitness, and dispersal (36–38).
This study aimed to measure metabolic performance in juvenile round goby at two separate ages and four ecologically relevant water temperatures. Three of our temperatures (15, 19, and 23°C) are currently observed in the Laurentian Great Lakes during breeding months, and one temperature (27°) is a projected estimate of future water temperature because of climate change (23). We note, however, that round goby likely currently experience this high temperature in parts of their native range (i.e., the Caspian Sea) (22). We measured routine (RMR) and maximum metabolic rates (MMR) of early juvenile round goby to provide an estimate of their “scope for activity”. RMR represents the typical “day-to-day” metabolic rate, consolidating the minimum energy requirements to sustain life (i.e., standard metabolic rate, SMR), but allows for occasional activity (39). In contrast, MMR is the maximum rate of energy expenditure, setting the limit for aerobic activities (35). In fish, the measures of metabolic rate (e.g., SMR, RMR, and MMR) are expected to change with water temperature and life stage (29, 32, 35, 40). The difference between the maximum and standard metabolic rate [MMR–SMR; i.e., the “aerobic scope” (AS)] provides a range for the capacity of oxygen transport (and by proxy, metabolism) above the animal's baseline energy expenditure in which all aerobic activities (e.g., locomotion, reproduction, dispersal, etc.) occur and has been linked to animal fitness and performance (36, 37, 41, 42). Calculating aerobic scope requires accurate measures of the standard metabolic rate (SMR), which can be difficult to accurately measure in many experimental settings (31, 39). As an alternative, we assessed an estimation of the “scope for activity,” calculated as the difference between MMR and RMR (25, 43, 44). While estimating the scope for activity by subtracting RMR from MMR underestimates the oxygen transport for an animal's activities beyond its vital function, it may be more ecologically relevant and comparable to the natural conditions experienced by organisms in the wild compared to the aerobic scope (43), as fish rarely exist under SMR conditions (39). Finally, the temperature sensitivity coefficient, Q10, was calculated to measure how sensitive metabolism is to changes in temperature at different ages (45). Quantifying possible changes to metabolic rate due to climate change and rising water temperatures is important in predicting how round goby populations may respond in ecological settings, informing management and mitigation strategies (46).
2 Materials and methods
2.1 Fish collection, husbandry, and acclimation
Round goby eggs were raised in the laboratory (McMaster University; Hamilton, Ontario, Canada) between July and October 2023, and early life stage juveniles that hatched from these clutches were used in this study. We focused on two cohorts. The first cohort was collected as eggs from the field in July 2023 (LaSalle Marina, Lake Ontario; Hamilton, Ontario, Canada; 43.300463°N, 79.846205°W). We placed thirty artificial nests (constructed from modified square PVC pipes, 20 cm length × 13 cm width × 6 cm height) at several near-shore locations in LaSalle Marina at a depth of 0.7–1.0 m, when water temperatures were between ~19–21°C. The inner walls of the nests were lined with removable acetate sheets, which enabled us to carefully remove eggs from the nest after adult round goby females spawned on the walls or ceiling. Once eggs were detected with a guarding male they were transported back to the laboratory in aerated coolers. Once in the laboratory the eggs were placed in small holding aquaria (20 L; 40.6 cm length × 20.3 cm width × 25.4 cm height) with a strong air supply and allowed to develop at water temperatures of ~19°C (room temperature). The first cohort began hatching August 8th, 2023, after about 13–15 days in the lab and were reared until they were 12–14 weeks old (post-hatch) before use in experiments. The second cohort of eggs was from wild-caught pairs of round goby collected from the same field site (in June 2023) that spawned in the laboratory in artificial nests identical to the ones used in our field collections. The second cohort of eggs was removed from the breeding tanks and placed in holding tanks to develop (room temperature water, ~19°C). This second cohort of egg clutches began to hatch October 18th, 2023, 16–18 days post-fertilization (dpf), and were 6–7 weeks old (post-hatch) at the time of experimentation. Thus, all the young used in the study hatched from eggs that developed completely or partly in the laboratory (described above). The rearing conditions of both cohorts are summarized in Table 1 of the Supplementary material.
Once hatched, the young were reared for one additional week in standard holding tanks (20L; 40.6 cm length × 20.3 cm width × 25.4 cm height) held at room temperature (~19°C) before being moved to the acclimation tanks (the same dimensions as the holding tanks, as described above). All hatching, holding, and acclimation tanks were filled with dechlorinated reservoir water and kept at room temperature. All juveniles were fed twice daily with live brine shrimp nauplii (Titan Animal®, Montreal, Quebec, Canada), and were kept on a constant 16h:8h on-off light schedule throughout their development and during our experiments.
Approximately 1 week after hatching (~21 dpf for cohort 1; ~25 dpf for cohort 2) we began the acclimation phase of our experiment. Juvenile round goby were randomly selected from the development tanks and placed into eight 20 L acclimation tanks (same dimensions as above) each containing ~50 young. Water temperature was then adjusted to one of four temperature treatments (with 2 tanks per temperature): 15°C, 19°C, 23°C, and 27°C, by raising or lowering temperatures from 19°C by 1°C every 12 h. Once the target temperature was reached, fish were maintained at these temperatures for the duration of our experiments. Younger juveniles were exposed to their target temperature treatments for 5–6 weeks at the time of experimentation, and older juveniles were exposed for 11–13 weeks. The 15°C and 19°C tank temperature was maintained using an external water chiller (Active Aqua®, California, USA), while 23°C and 27°C tanks used small submersible 50-Watt water heaters (Aqueon®, Wisconsin, USA) to maintain temperature. Small glass vials (which were identical to the respirometers in size and shape), and halved clay pots were placed in each holding tank to provide enrichment, shelter, and to acclimatize the fish to the equipment used in the experiments (see below). The juveniles were frequently observed taking refuge within the vials and under the clay pots.
Water quality checks for pH, nitrate, nitrite, and ammonia were completed weekly throughout the study using API® Freshwater Master Test Kit. Water temperature was checked twice a day, and we conducted water changes once per week. All subjects used in our study were monitored at a minimum of twice per day throughout their development to ensure they did not exhibit signs of stress or illness. All our experimental protocols were approved by the Animal Research Ethics Board at McMaster University (AUP # 22-04-11) and adhere to the guidelines set by the Canadian Council on Animal Care.
2.2 Measuring metabolic rates using respirometry
All metabolic rate trials were conducted in a separate 20 L experimental tank. The sides of the experimental tank were covered with opaque blue vinyl to reduce stress and ensure that the fish could not see the experimenter during trials. Before each trial the experimental tank was filled with 16 L of cold municipal tap water treated with SeaChem® Prime and left to vigorously aerate for 24 h. Tap water was used instead of aged reservoir water to minimize the amount of bacterial respiration within the experimental tank. The experimental tank water temperature was then set to the desired temperature (i.e., to match the temperature of the acclimation tank) using an external water chiller (for 15°C and 19°C; Active Aqua®, California, USA) or using submersible aquarium heaters (for 23°C and 27°C; Aqueon®, Wisconsin, USA). The water chiller pump (and not the chiller itself) remained on during the 23°C or 27°C experiments to ensure similar flow conditions in all trials, and to circulate water within the tank to ensure a uniform temperature. During the trials, the tank temperature was continually measured by two Pt100 temperature probes (PyroScience GmbH®, Aachen, Germany) placed near the respirometry chambers, and never varied more than ±1°C from the set temperature. Temperature data was recorded every 3 seconds via two 4-channel FireSting®-O2 optical oxygen and temp meters (model: FSO2-C4; PyroScience GmbH®, henceforth the “oxygen meter”).
Routine metabolic rate (RMR) and maximum metabolic rate (MMR) experiments were conducted on the same fish and on the same day, with RMR typically commencing c. 12:00pm (~5 h after the lights had turned on) and MMR commencing approximately 30 min after RMR finished. Fish were fasted for 24 h before we began trials to ensure a post-absorptive state. Our respirometry experiments were conducted in OXVIAL4 (PyroScience GmbH) closed respirometry chambers (~1.5 cm diameter × ~4.8 cm height, volume = 5 mL) connected to the oxygen meter and placed in these experimental tanks. These respirometers employ contactless optode oxygen sensors to record the levels of O2 within the chambers over time and relay oxygen consumption data via the oxygen meter to Pyro Workbench software. Each respirometry chamber was re-calibrated prior to every experiment to the appropriate temperature (as measured by the Pt100 probe connected to the same oxygen meter), using 100% air-saturated water. Air saturation (% DO, dissolved oxygen percentage) within each chamber was above 95% DO prior to the start of each trial.
At the start of an RMR trial, seven juvenile round goby were haphazardly selected from a single acclimation temperature and using a small net were gently placed in a small container filled with aerated experimental tank water. A 5 mL transfer pipette was modified to help place goby into the respirometer chambers. The pipette was cut at the 1.5 mL mark and again halfway down the bulb, and these long ends were removed. The remaining middle segment was fitted to the small container at the bulb side, while the other end could be inserted approximately halfway into the respirometer chamber. Each individual juvenile was then gently moved into a respirometry chamber (n = 1 fish per chamber, with 7 chambers total) by inverting the container and allowing the fish to slowly sink into the chamber on their own volition. The chamber was sealed underwater and visually inspected to ensure there were no air bubbles. An eighth chamber was ran empty to record background microbial respiration and served as our control. The rate of oxygen consumption inside the control chamber was calculated over the entire measurement period (beginning once the first fish-containing chamber was sealed until the final chamber was unsealed). The rate of oxygen consumption within the control chamber was subsequently subtracted from the oxygen consumption rates of each of the seven chambers containing fish to account for the oxygen consumed by microbes.
Round goby in the laboratory and field often take refuge under rocks or artificial shelter (47), and the presence of shelter can reduce stress in fish during respirometry experiments (48). We therefore laid our respirometers horizontally to give these bottom-dwelling fish more space to move around, and we embedded the chambers in gravel substrate, also placing artificial shelters constructed from small clay pots over each chamber. The pots covered approximately half of the length of the respirometry chambers, and each individual received their own shelter. This allowed the fish to remain far back in the chambers and completely under the shelter or move to the front of the chamber in a less sheltered area. Oxygen (in % DO) and temperature (°C) data from the chambers was recorded to a computer via the oxygen meter and logged using Pyro Workbench software in 5- and 3-second intervals, respectively, and monitored/visualized in real-time. Oxygen uptake was measured for 60 min, or until % DO within a chamber dropped below 70%, whichever came first. Once either endpoint was reached, the entire chamber and the associated shelter were promptly removed from the tank to minimize potential disturbances to other fish. Outside of the tank, the fish were carefully removed from the chambers and placed in their own Falcon tube containing aerated experimental tank water until they were ready for use in MMR trials. These tubes were kept in a water bath to maintain experimental temperature conditions, and each fish was held in these tubes for at least 30 min to recover.
To elicit MMR, we subjected fish to an exhaustive chase followed by air exposure. This protocol was based on the method described in Behrens et al. (49), which was used to elicit MMR in adult round goby. Fish were added individually to a circular arena (10.5 cm diameter × 7.5 cm height) and chased for 3 min in a circle using a metal rod. We regularly alternated the motion of the rod in clockwise and counterclockwise directions to ensure the fish was actively swimming against the current. Afterwards the fish was carefully netted and air exposed for 30 seconds. This step was changed from the Behrens et al. (49) protocol (wherein a 1-min air exposure time was used), as in pilot studies not all juveniles survived the full minute of air exposure. After air exposure, fish were placed back into the respirometry chambers. This process of reintroducing the fish into the respirometer after air exposure and sealing the chamber never took longer than 30 seconds (and typically took ~15 seconds). Oxygen consumption was then measured for a minimum of 30 min.
2.3 Mass and length measurements
Following MMR trials, the juvenile round goby were removed from the chambers and euthanized via benzocaine overdose (>250 mg L−1). Immediately afterwards their body length and mass were measured. Each sacrificed fish was photographed using an iPhone® camera (Model A2111) with a reference scale bar included in each photo. ImageJ software was then used on the digital images to determine their total body lengths, rounded to the nearest 0.1 mm. Body mass was measured on a scale accurate to 0.0001 g (Mettler-Toledo® Model: AB 204-S), and each fish was independently measured three times after being carefully blotted dry. The average of three mass replicates were used in any subsequent calculations. The average mass and length measurement for each temperature and/or age is summarized in Table 1.
2.4 Data management and calculations
All data was recorded using Pyro Workbench (version 1.4.7.2305) software. Oxygen units were first converted from DO% to mg O2 L−1 in Pyro Data Inspector (version 1.4.7.2305). The first 15 min of data were censured to reduce the possible effects of handling stress. A slope was calculated using the initial measurement from 15 min post-addition to the final measurement at either 60 min, or when the chamber dropped below 70% DO, whichever came first. Metabolic rate (mg O2 s−1) was then calculated using the equation:
where ΔCf is the rate at which oxygen concentration changed over time (in mg O2 L−1 s−1) in a fish-containing respirometer; ΔCc is the rate of background respiration (i.e., from the control chambers; mg O2 L−1 s−1); Vr is the volume of the respirometer (0.005 L); Vf is the volume of the fish (which is equal to its mass, assuming equal density in water) (50). Since oxygen decreased over time, the absolute value was taken to express a positive metabolic rate. Metabolic rate units were converted from mg O2 s−1 to μmol O2 h−1 to better match previously published metabolic rates in young fish (32).
To calculate MMR, we calculated slopes of 1-min-long intervals over the first 15 min post-chase using a rolling regression model as described by Little et al. (51). We only considered slopes in which R2 ≥ 0.85 and took the largest-magnitude slope within these 15 min as the maximum rate of oxygen consumption. A scope for activity (μmol O2 h−1) was estimated for each fish by subtracting their measured RMR from their corresponding MMR.
The Q10 temperature coefficient was calculated to describe the temperature sensitivity of metabolism. However, larger fish are expected to have greater absolute metabolic rates, and rearing temperature may influence the size of the fish. Therefore, to account for potential differences in mass due to rearing temperatures skewing our Q10 values, we first followed the methods employed in other studies to control for these mass effects (24, 52) and performed an allometric correction on observed metabolic rates:
where Rcor is the allometrically-corrected adjusted measure (RMR or MMR), Robs is the observed measure value, Mavg is the overall average mass (~21.8 mg), Mobs is the mass of the individual fish, and β is the empirically determined allometric mass-scaling exponent from our linear mixed effect model for each measure (the logMb coefficient from Table 2). We only used these allometrically corrected values in our calculation for Q10, and all other analyses (including the depictions of our data) use uncorrected metabolic rate values. We calculated Q10 for RMR and MMR using the median of our allometrically-corrected metabolic rates between 15°C and 27°C, according to the following equation:
where R1, cor and R2, cor are the median corrected metabolic rate at 15°C (T1) and 27°C (T2), respectively. To calculate a Q10 for the scope for activity, we first corrected it by subtracting MMRcor – RMRcor (and not via Equation 2). The median of this corrected scope for activity value was then used in Equation 3 above, again between 15°C and 27°C.
2.5 Statistical analyses
All statistical tests were performed in R version 4.3.2 (53). For all analyses we used a significance level of α = 0.05. In total, we performed experiments on 139 fish, although our analysis only includes 99. We only included the individual in our analysis if we had data for both RMR and MMR trials, as the scope for activity requires both measures. We examined fish for signs of stress and abnormal swimming behavior (e.g., refusal to swim during the chase protocol) and excluded those exhibiting these signs (n = 6). Fish were also excluded if they displayed any physical abnormalities (n = 1). Unresponsive fish (e.g., those that were idle, but the operculum was moving) during any stage but before euthanasia were also excluded (n = 9). Fish mortalities at any stage (post-RMR, during recovery, pre/post-chase protocol, post-MMR but prior to euthanasia) were also excluded (n = 10). Significant data loss (either during RMR or MMR, and due to computer/equipment error) resulted in those chambers being excluded (n = 4). Experimenter error resulted in two fish being removed (n = 2). In one case, there was no R2 for the 1-min MMR slopes that was >0.85 and this fish too was removed (n = 1). Finally, we excluded fish if their RMR was inexplicably higher than MMR (n = 7).
Mass and length data for fish at every temperature and age were assessed for normality first using a Shapiro-Wilks test (assumed normally distributed if p > 0.05), followed by visually assessing the quantile-quantile (Q-Q) test. The Shapiro-Wilks test determined that most of our data was normally distributed (W > 0.88, p > 0.20), except for mass from the 23°C younger round goby (W = 0.7, p < 0.001), and length from the 15°C older goby (W = 0.88, p = 0.04). Neither a log10 nor square-root transformation on either set of data resulted in a normal distribution, but an assessment of the Q-Q plot revealed a few outliers were driving this failure to achieve normality (1 for mass, 2 for length). Thus, we assumed that these measures were normally distributed as well. A one- or two-way ANOVA was subsequently performed on both mass and length measurements using temperature and age as covariates, followed by a Tukey HSD post-hoc test.
Empirically determined metabolic scaling coefficients are important for accurate bioenergetics modeling. Linear mixed effect models (lme4 package) (54) were used to assess how log10-transformed mass, temperature, and age affected metabolic rate. All metabolic variables were first log10-transformed. All models initially included log10-transformed mass as a covariate, plus temperature and age (and including a temperature × age factor) as fixed effects. The specific respirometry chamber itself was included to account for random effects and the possibility of equipment variability. The most parsimonious model was selected using Akaike Information Criterion (AIC), and the model with the lowest AIC value was considered to be the best fit for the data (lmerTest package) (55). A significance level of α = 0.05 was chosen to determine if a factor had a clear effect on the model. The temperature × age factor was not statistically significant for any models and was removed. As temperature and age were both included as fixed factors, all models generated are relative to younger early juveniles reared at 15°C (Table 2).
3 Results
3.1 Juvenile body measurements across temperatures
In younger fish (6–7 weeks old), body mass did not vary across temperature treatments (one-way ANOVA; F = 1.29, p = 0.29), but there was a strong effect of temperature in the older juveniles (12–14-week-old juveniles ANOVA; F = 11.74, p < 0.001; Figure 1A). Among older individuals, body mass generally increased with temperature before appearing to collapse at 27°C; we found no difference between the masses of older juveniles reared at 15°C compared to 27°C (Tukey-HSD: p = 0.66; Figure 1A). There was no clear difference in growth between 6 and 12 weeks in fish reared at 15°C or 27°C (p = 0.70, p = 0.40 respectively; Figure 1A), while body size more than doubled at the intermediate temperatures (216% increase at 19°C, p < 0.0001; and a 281% increase at 23°C, p < 0.0001). Overall, body mass was affected by an interaction between age and rearing temperature (two-way ANOVA; age: F = 64.20, p < 0.001; temperature: F = 11.41, p < 0.001; age × temperature: F = 7.98 p < 0.001; Figure 1A).
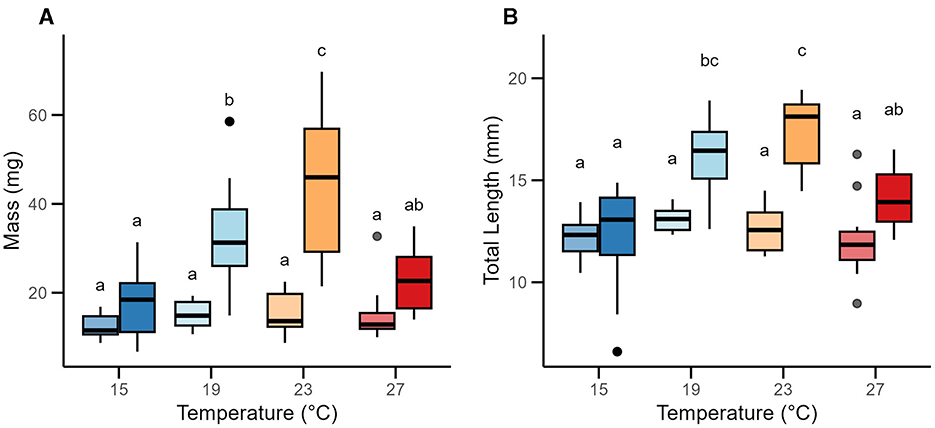
Figure 1. Juvenile growth at two timepoints under rearing temperatures. The box plots depict the median and first and third quartiles. Whiskers display the interquartile range of the data, and solid dots represent outliers. Younger juveniles (6–7 weeks) appear on the left side of each temperature condition, represented in lighter coloring, with older juveniles (12–14 weeks) on the right (darker coloring). Sample sizes for each temperature and age condition can be found in Table 1. (A) Mass measurements of juveniles by temperature treatment and age. Mass measurements (wet weight) of each goby were taken immediately after the MMR trial concluded. The average of the three replicates were used in subsequent analyses. (B) Length measurements of juveniles by temperature treatment and age. After mass measurements, juveniles were photographed with a reference scale bar included in the photo. ImageJ was used to measure their lengths relative to the scale bar. All lengths were rounded to the nearest 0.1 mm to account for uncertainty in the measurements.
Patterns of total length were similar to body mass (Figure 1B). Overall, there was a clear effect of temperature and age (and an interaction between temperature and age) on early juveniles' total lengths (two-way ANOVA; age: F = 49.11, p < 0.0001; temperature: F = 14.43, p < 0.0001; age × temperature: F = 7.33, p < 0.001; Figure 1B). There was no clear difference in body length in the younger juveniles across temperatures regimes (one-way ANOVA: F = 1.45, p = 0.24), but a marked difference in body length of the older cohort across rearing temperatures (F = 15.54, p < 0.0001). The older fish reared at 23°C were on average 22%−40% longer than fish reared at 27°C or 15°C, respectively (23–27°C: p < 0.01, 23–15°C: p < 0.0001), but were similar in size to the 19°C juveniles (p = 0.48). Generally, total body length appeared to increase rapidly between 15°C and 19°C (p < 0.0001), and then plateaued between 19°C and 23°C (p = 0.48), and was suppressed between 23°C and 27°C (p < 0.01; Figure 1B).
3.2 Allometric scaling of metabolic rate
Unsurprisingly, larger fish had greater absolute routine metabolic rates (RMR) which scaled near-isometrically [log10(mass): β = 0.91 ± 0.15; t = 6.125, p < 0.0001], and generally increased with water temperature (temperature effects: 19°C = 0.27 ± 0.06, t = 4.245, p < 0.0001, 23°C = 0.22 ± 0.08, t = 2.933, p < 0.01, 27°C = 0.37 ± 0.07, t = 5.532, p < 0.0001; Table 2, Figure 2). The most parsimonious model for log10 RMR (AIC = 1.6) was:
where Mb is the mass of the fish, and T19, T23, and T27 are the respective 19, 23, and 27°C conditions. The intercept (relative to a 15°C, younger juvenile) is 0.83 (± 0.30) (t = 2.789, p < 0.01), and ε is the error term (though our error term, ε, was not significant in any models of metabolism). When adjusted to the average body mass of 21.8 mg, juvenile fish reared in warmer temperatures (19°C, 23°C, and 27°C) had higher RMR than fish reared at 15°C (for all pairwise comparisons; t-ratio ≤ −2.83, p < 0.05). Even though fish reared at 27°C had the highest RMR, there was no clear difference between these fish and the fish reared in 19°C or 23°C rearing conditions (19°C−27°C: t-ratio = −1.73, p = 0.52, 23°C−27°C: t-ratio = −1.88, p = 0.24, 19°C−23°C: t-ratio = 0.76, p = 0.87). The overall Q10 of juvenile round goby RMR between 15°C and 27°C was 1.96. Interestingly, while the mass and temperature terms had clear effects, the age term did not (age: −0.11 ± 0.06; t = −1.831, p = 0.07; Table 2).
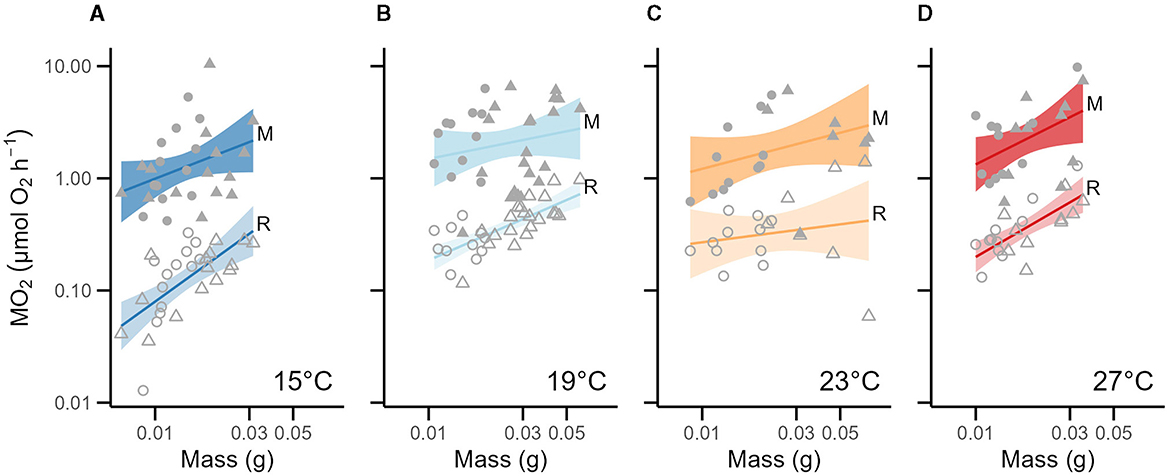
Figure 2. Absolute metabolic rates across temperatures as a function of mass. For each graph, the x-axis represents mass on a log10 scale, and the y-axis is the absolute metabolic rate on a log10 scale. Across all temperatures, MMR appears as solid dots with a solid simple regression line (95%-CI for the regression line is shown; top line), RMR appears as hollow dots (95%-CI for the simple regression line is shown in a lighter color; bottom line). The 6–7-week-old cohort is represented in circles, and the 12–14-week-old cohort is represented in triangles. The same fish were used in the RMR and MMR measurements. Note that we depict a simple regression line through the data for a given temperature acclimation, and the equation of the linear mixed-effect model from Table 2 is not shown. Panels show the absolute metabolic rates at (A) 15°C (n = 28). (B) 19°C (n = 32). (C) 23°C (n = 18). (D) 27°C (n = 21).
The maximum metabolic rate (MMR) of juvenile round goby was largely temperature insensitive, and the most parsimonious model (AIC = 63.0) did not include temperature as a factor. The Q10 of juvenile round goby MMR between 15°C and 27°C was 1.74, however, we found clear effects of mass and age (Table 2). Larger fish had higher MMRs, and this relationship scaled with negative allometry [log10(mass): β = 0.82 ± 0.18, t = 4.579, p < 0.0001; Table 2, Figure 2], though older juveniles of similar size are predicted to have lower MMRs than younger juveniles (age: −0.19 ± 0.08; t = −2.428, p < 0.05). For example, an averaged-mass juvenile (21.8 mg) at 6–7 weeks was predicted to have a maximum metabolic rate that is ~1.55 × higher than a same-sized juvenile at 12–14 weeks (t-ratio = 2.371, p < 0.05). The model which was the best fit for the observed MMR data was:
where Aolder is a conditional age factor that applied to the older cohort of fish only (i.e., this term is zero for the younger juveniles; Table 2).
Similar to the model for MMR, our estimation of the scope for activity (SA) (AIC = 132.7) was affected by the same factors in the linear mixed-effect model. Like MMR, the scope for activity was greater in larger individuals and exhibited a negative-allometric relationship with body mass [log10 (mass): β = 0.69 ± 0.26; t = 2.679, p < 0.01; Table 2, Figure 3]. The scope for activity was predicted to be greater in younger juveniles that were similarly sized to older juveniles (age: −0.23 ± 0.11; t = −2.066, p < 0.05; Table 2). Our model which was the best fit of the data was:
This model suggests that, like MMR, the scope for activity (SA) of juvenile round goby is largely insensitive to the temperatures we measured. The Q10 of the scope for activity of juvenile round goby between 15°C and 27°C was 1.72 but was clearly dependent on the size (i.e., mass) and age of the fish. Older individuals were had a lower estimated scope for activity relative to younger fish only if they had a similar mass; and indeed, when the average mass between both age cohorts was used, younger juveniles' scope for activity was estimated to be 70% higher than in older juveniles (t-ratio = 2.018, p < 0.05).
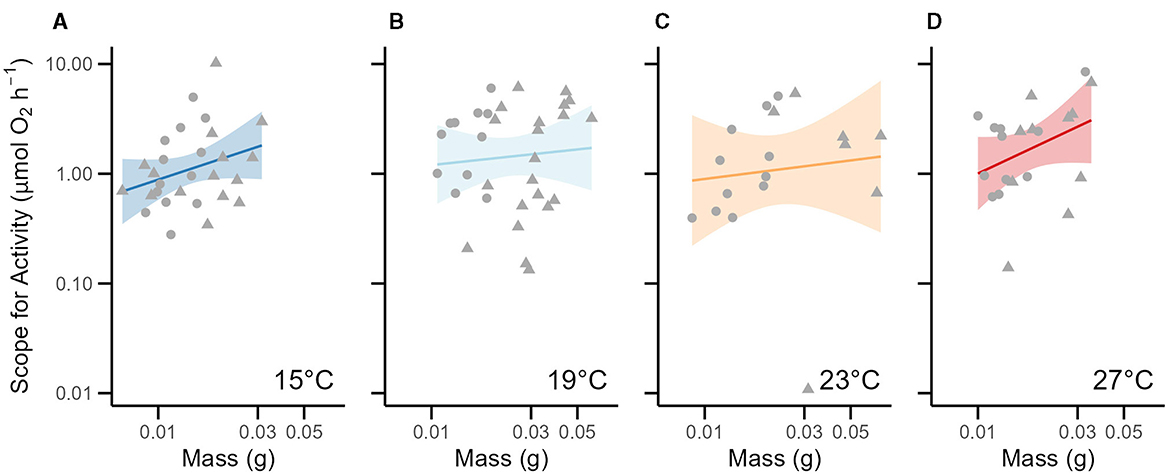
Figure 3. Relative scope for activity across temperatures as a function of mass. For each graph, the x-axis represents mass on a log10 scale, and the y-axis is the absolute scope for activity on a log10 scale. Across all temperatures, the estimated scope for activity (MMR – RMR) appears as solid dots with a solid simple regression line (95%-CI is shown). The 6–7-week-old cohort is represented in circles, and the 12–14-week-old cohort is represented in triangles. Note that we depict a simple regression line through the data for a given temperature acclimation, and the equation of the linear mixed-effect model from Table 2 is not shown. Panels show the relative scope for activity at (A) 15°C (n = 28). (B) 19°C (n = 32). (C) 23°C (n = 18). (D) 27°C (n = 21).
4 Discussion
Our measures of metabolism suggest that round goby have a high capacity to cope with warming water temperatures. Routine metabolism increased with warming temperatures as expected, but our assessment of the scope for activity, an important organismal metabolic trait often linked to fitness, was consistent across all temperature treatments. In contrast, our measurements of growth showed substantial inhibition of growth at high temperatures and indicate a thermal optimum near 23°C, supporting previous observations of maximized feeding in adults at these intermediate temperatures (24). Our results will facilitate the refinement of bioenergetic models to include early life stages, which will allow for a more accurate representation of the cost of this invasive species in ecological settings.
Bioenergetic modeling is useful way to consolidate various measures of animal physiology (e.g., metabolic rates, consumption rates, etc.) to better understand how energy and materials (e.g., nutrients and contaminants) move throughout the food web (24). While bioenergetic models can be used to infer larger-scale ecological impacts of species on processes such as competition, predator-prey dynamics, and growth rates, they can also predict the impact that environmental perturbations, such as climate change, will have on species (56). Existing bioenergetic models created for the round goby (24), which utilized fish ~2 g and above, a size class 100-fold larger than the average mass of early juveniles used in this study (~0.02 g), substantially underestimated our observed RMR values. When comparing our mean RMR at each temperature to their model predictions (but using our average mass), our observed RMR is at a minimum 128% higher at 15°C, and up to 263% higher at 19°C. Our results of much higher RMRs are consistent with our broad expectations of high mass-specific metabolism in juvenile fishes (32). Developing fish tend to have faster metabolic rates, driven by the weight-dependent nature of metabolism, and the costs of rapid development in larval and juvenile life stages. For example, growth rates in larval and young juvenile Atlantic cod (Gadus morhua) fishes can exceed more than 10-fold that of adult conspecifics (32). Lee and Johnson (24) showed that smaller, younger round goby that weigh less consume more, and we propose this is likely to compensate for their increased metabolic costs required to fuel their rapid growth rate. Our study lends credence to the idea that estimates of metabolism of earlier life stages are needed to create more robust energetic models, as extrapolating estimates gleaned from adult stages underestimate the early-life energetic costs in larval and juvenile fish. In invasive fish like the round goby, underestimating these early-life costs might potentially downplay the ecological impact of this life stage.
The effects of temperature in our study varied depending on which trait was being measured. Routine metabolic rate increased dramatically with temperature, with 27°C-reared fish having metabolic rates that were ~232% higher than 15°C-reared fish. However, there was no clear significant effect of temperature on MMR nor on scope for activity. The estimated scope for activity was maintained regardless of rearing temperature, suggesting that juvenile round goby have a broad, flat thermal performance curve and adopt a “thermal generalist” strategy, which fits the current theory about what makes a good invader (11, 57). Even though we found clear effects of temperature on RMR the much higher and more variable values of MMR likely statistically obscured any impact of temperature on the estimated scope for activity. Even though RMR is temperature sensitive, implying that background activities may be greatly influenced by temperature (though this requires further exploration), our results suggest that the capacity of juvenile round goby to perform high intensity aerobic activities (such as exhaustive swimming or digestion of large meals) (58) is not hindered at elevated temperatures. This stability of thermal performance across a wide temperature range may provide an advantage in the face of climate change for the invasive round goby over native fishes that live in the same habitats. For example, smallmouth bass (Micropterus dolomieu) in North America or European perch (Perca fluviatilis) in parts of Europe experience a decline in aerobic scope above ~20°C (59, 60). Climate change is predicted to limit the geographical range of several native fish species, such as lake trout (Salvelinus namaycush), lake whitefish (Coregonus clupeaformis), slimy sculpin (Cottus cognatus), and others (61). This phenomenon is coupled with competition from the now well-established round goby (and other invasive species) in the Great Lakes and pushes native fish populations to be at even greater peril.
The ability to maintain aerobic performance across a wide temperature range might also explain how the round goby has been able to successfully invade and thrive in many freshwater environments globally across a wide breadth of latitudes. Performance seems to be largely temperature insensitive in adults (52, 62), and here we show a similar pattern in juveniles. Vivó-Pons et al. (75) assessed the functional distinctiveness of the round goby compared to native fishes in the Baltic Sea, in part to determine where functional niches overlap (and intraspecific competition is likely). We propose something similar be developed for the Great Lakes basin, with a greater focus on the thermal biology and temperature niches of native fishes compared to round goby, to predict how warming water temperatures might affect different fish populations.
Despite differences in mass-specific metabolic rate, our findings are generally consistent with previous work on adult round goby. For example, while we estimated the scope for activity (MMR–RMR), the aerobic scope (MMR–SMR) in adults was measured and found to be consistent between 15°C−25°C, with a final increase at 28°C (52). A different pattern was observed for growth, the 12–14-week-old fish in our study showed a clear growth optimum at 23°C and the greater relative growth was observed at intermediate water temperatures (19°C, 23°C). The negative effects of extreme temperatures were especially pronounced at the higher temperatures, as the average body mass of 27°C-reared fish was 48% lighter than fish reared at 23°C, and they were on average 18% shorter. The decline in growth observed in fish held at 27°C might be explained by higher routine energy costs (i.e., RMR), reduced appetite, or decreased digestive efficiency outweighing the rate of energy assimilation, which would all subsequently diminish the net amount of energy available for processes such as growth (63–66). Our findings largely complement the data shown in Lee and Johnson's (24) bioenergetics model, which show that feeding was maximized between 23°C−26°C, but then declined sharply at warmer temperatures. Together, the results imply that temperatures between 19°C and 23°C may be optimal for growth in these species, but animal performance may be resilient across a wider range of temperatures. Based on these data, and because body condition and body size have been linked to fitness and overwinter survival in the past, we speculate that juvenile round goby born near-shore and/or later in the summer when water temperatures are typically warmer, may have a survival advantage compared to those fish that hatched earlier in the season or in deeper, cooler waters (67, 78). However, this advantage may be lost if water temperatures exceed 27°C, which is what projections from climate change models predict the average summertime surface water temperatures will warm to in the North American Great Lakes by 2071–2100 (23).
A limitation of our study for conservation management is that our findings were obtained in a well-controlled laboratory setting. We know little about how our measures of metabolism compare to metabolism in the real world, and to date very few studies have investigated the integration of physiological measures in the laboratory vs. the field (68). In the field, multiple environmental factors typically act in concert to influence metabolism (e.g., temperature, salinity, and oxygen availability) (31). Another limitation in the design of our study was that the closed respirometry did not allow for a longer acclimation time after the fish were introduced into the respirometer. This meant we had a constrained window to measure RMR, which could have led to overestimates of RMR. Although the fish had access to shelter (which we added to reduce stress and encourage recovery) (48), the respirometers were placed in gravel substrate to mimic their natural condition for these benthic fish, and we censured the first 15 min of the trial, these measures may not have been enough to cause the stress of handling to subside completely. Despite these limitations, our results certainly add to a growing body of literature that suggest that aerobic scope (in our case the scope for activity) is less thermally sensitive than other important fitness-linked traits such as growth (69, 70). There is still much unknown about the thermal physiology of this early life stage of fishes in general and round goby in particular, so more targeted experiments investigating the role of elevated rearing temperatures on early juvenile's performance, physiology, and fitness (e.g., CTmax/CTmin, LT50, Tpref) are still needed. Future studies should incorporate field observations and validate our laboratory findings. Such studies will require technological advances that facilitate field monitoring of tiny fish, but once these are developed a natural first step will be to compare our controlled laboratory measures of metabolism with measures from the field that take into account the environmental variability and stressors not captured in a controlled setting (50, 59, 71). Having ecologically valid information will allow us to better understand how climate change might further the pervasiveness and competitive edge that round goby have over native fish species, globally.
Our findings might be applied to management strategies for controlling the spread of round goby. The role of temperature in shaping metabolic performance is clear (31, 33, 72, 73). Our findings show that juvenile round goby have metabolic resilience to warming water temperatures. As water temperatures rise, round goby will be capable of greater range expansions and knowledge of the juvenile round goby's response to climate change could help prepare resource managers come up with practical approaches to limiting the ecological impact of this invasive species (46, 74). Future work should explore how round goby juveniles compare to the juveniles of native species in terms of their metabolic resilience to environmental stressors (68, 75).
We found that younger juveniles had an estimated scope for activity that was nearly ~70% higher than their older-juvenile counterparts. While we expect that younger juveniles would have a greater metabolism to support a faster growth rate, this may also be a result in part to the round goby's bipartite life cycle. While adult round goby are benthic and usually sedentary, early-stage juveniles exhibit a diel vertical migration through the pelagic zone (13, 28). Diel vertical migration in juvenile round goby is characterized by the movement up the water column at night and down during the day (28, 76). Considering round goby lack swim bladders to regulate buoyancy (12), this vertical migration behavior is likely highly energetically demanding. Juveniles found undergoing diel vertical migration were 7–8 mm in length (28), similar to the younger cohort of juveniles we measured here (~12 mm). In younger and more pelagic early juvenile round goby, a large scope for activity may be needed to support their energetic needs and ascend the water column. In addition, their scope for activity might need to be consistent across a wide range of temperatures to perform this daily migration activity of transitioning from deeper, colder waters to warmer, surface warmers. Notably, despite their small size, early juvenile round goby possesses 40–80% of the swimming capacity of adults (77) and taken together with our demonstratation of the metabolic performance of this life stage, we speculate that dispersal by juvenile round goby contributes greatly to the invasion success of this species. There is still much unknown about diel vertical migration behavior in early life-stage juvenile round goby, such as potential benefits received and energetic costs incurred, and these represents a remaining large gap in our understanding of this species.
Given our results, we emphasize that care should be taken when attempting to extrapolate data from bioenergetics models based on one life stage and applying it to other life stages. Metabolic homogeneity should not be assumed across various life histories, owing to potentially different lifestyles, behaviors, energy stores, and growth patterns. These life history differences may be especially pronounced between larval, juvenile, and adult stages (32). To this end, we suggest that an updated bioenergetics model for the round goby be developed that incorporates data from all life stages. This will allow for a more accurate representation of the energetic consequences of this invasive species across a range of ecological settings.
Data availability statement
The original contributions presented in the study are included in the article/Supplementary material, further inquiries can be directed to the corresponding author.
Ethics statement
The animal study was approved by Animal Research Ethics Board (AREB) at McMaster University (AUP# 22-04-11). The study was conducted in accordance with the local legislation and institutional requirements.
Author contributions
HH: Conceptualization, Data curation, Formal analysis, Investigation, Methodology, Visualization, Writing – original draft, Writing – review & editing. SZ: Conceptualization, Data curation, Investigation, Methodology, Writing – review & editing. WT: Methodology, Writing – review & editing. AT: Methodology, Writing – review & editing. SB: Conceptualization, Funding acquisition, Methodology, Project administration, Resources, Supervision, Writing – review & editing.
Funding
The author(s) declare financial support was received for the research, authorship, and/or publication of this article. This work was supported by Natural Sciences and Engineering Research Council of Canada (NSERC) Discovery grant (RGPIN-2016-05772) and a Fishers and Oceans Canada grant provided to SB.
Acknowledgments
We thank Dr. G. R. Scott for his guidance and advice throughout the project. Thank you to Ryan Hodgson for helping on the initial set-up of the experiments and assisting with experiments. Thank you to Reyad Malakh, David Benbassat, and Harrison Caruana for your assistance running trials. Lastly, thank you to the entire ABEL team at McMaster University for your continued support, feedback, and making sure the fish were always well fed.
Conflict of interest
The authors declare that the research was conducted in the absence of any commercial or financial relationships that could be construed as a potential conflict of interest.
Publisher's note
All claims expressed in this article are solely those of the authors and do not necessarily represent those of their affiliated organizations, or those of the publisher, the editors and the reviewers. Any product that may be evaluated in this article, or claim that may be made by its manufacturer, is not guaranteed or endorsed by the publisher.
Supplementary material
The Supplementary Material for this article can be found online at: https://www.frontiersin.org/articles/10.3389/frish.2024.1489837/full#supplementary-material
References
1. Early R, Bradley BA, Dukes JS, Lawler JJ, Olden JD, Blumenthal DM, et al. Global threats from invasive alien species in the twenty-first century and national response capacities. Nat Commun. (2016) 7:12485. doi: 10.1038/ncomms12485
2. Radchuk V, Reed T, Teplitsky C, van de Pol M, Charmantier A, Hassall C, et al. Adaptive responses of animals to climate change are most likely insufficient. Nat Commun. (2019) 10:3109. doi: 10.1038/s41467-019-10924-4
3. Thomas CD, Cameron A, Green RE, Bakkenes M, Beaumont LJ, Collingham YC, et al. Extinction risk from climate change. Nature. (2004) 427:145–8. doi: 10.1038/nature02121
4. Mooney HA, Cleland EE. The evolutionary impact of invasive species. PNAS. (2001) 98:5446–51. doi: 10.1073/pnas.091093398
5. Mollot G, Pantel JH, Romanuk TN. Chapter Two – The effects of invasive species on the decline in species richness: a global meta-analysis. Adv Ecol Res. (2017) 56:61–83. doi: 10.1016/bs.aecr.2016.10.002
6. Havel JE, Kovalenko KE, Thomaz SM, Amalfitano S, Kats LB. Aquatic invasive species: challenges for the future. Hydrobiologica. (2015) 750:147–70. doi: 10.1007/s10750-014-2166-0
7. Bell DA, Kovach RP, Muhlfeld CC, Al-Chokhachy R, Cline TJ, Whited DC, et al. Climate change and expanding invasive species drive widespread declines of native trout in the norther Rocky Mountains, USA. Sci Adv. (2021) 7:eabj5471. doi: 10.1126/sciadv.abj5471
8. Olden JD, Poff NL, Bestgen KR. Life-history strategies predict fish invasions and extirpations in the Colorado river basin. Ecol Monogr. (2006) 76:25–40. doi: 10.1890/05-0330
9. Romanuk TN, Zhou Y, Brose U, Berlow EL, Williams RJ, Martinez ND. Predicting invasion success in complex ecological networks. Phil Trans R Soc B. (2009) 364:1743–54. doi: 10.1098/rstb.2008.0286
10. Sorte CJB, Ibáñez I, Blumenthal DM, Molinari NA, Miller LP, Grosholz ED, et al. Poised to prosper? A cross-system comparison of climate change effects on native and non-native species performance. Ecol Lett. (2012) 16:261–70. doi: 10.1111/ele.12017
11. Bates AE, McKelvie CM, Sorte CJB, Morley SA, Jones NAR, Mondon JA, et al. Geographical range, heat tolerance and invasion success in aquatic species. Proc Royal Soc B. (2013) 280:20131958. doi: 10.1098/rspb.2013.1958
12. Kornis MS, Mercado-Silva N, Vander Zanden MJ. Twenty years of invasion: a review of round goby Neogobius melanostomus biology, spread, and ecological implications. J Fish Biol. (2012) 80:235–85. doi: 10.1111/j.1095-8649.2011.03157.x
13. Hayden TA, Miner JG. Rapid dispersal and establishment of a benthic Ponto-Caspian goby in Lake Erie: diel vertical migration of early juvenile round goby. Biol Invasions. (2009) 11:1767–76. doi: 10.1007/s10530-008-9356-5
14. Astorg L, Charette C, Windle MJS, Derry AM. Two decades since first invasion: revisiting round goby impacts on nearshore aquatic communities in the Upper St. Lawrence River. J Great Lakes Res. (2022) 48:581–92. doi: 10.1016/j.jglr.2022.01.017
15. Balshine S, Verma A, Chant V, Theysmeyer T. Competitive interactions between round gobies and logperch. J Great Lakes Res. (2005) 31:68–77. doi: 10.1016/S0380-1330(05)70238-0
16. McCallum ES, Marentette JR, Schiller C, Jindal S, Empringham K, Marsh-Rollo S, et al. Diet and foraging of Round Goby (Neogobius melanostomus) in a contaminated harbour. Aquat Ecosyst Health Manage. (2017) 23:252–64. doi: 10.1080/14634988.2016.1254468
17. Hogan LS, Marschall E, Folt C, Stein RA. How non-native species in Lake Erie influence trophic transfer of mercury and lead to top predators. J Great Lakes Res. (2007) 33:46–61. doi: 10.3394/0380-1330(2007)33[46:HNSILE]2.0.CO;2
18. Essian DA, Chipault JG, Lafrancois BM, Leonard JBK. Gut content analysis of Lake Michigan waterbirds in years with avian botulism type E mortality, 2010–2012. J Great Lakes Res. (2016) 42:1118–28. doi: 10.1016/j.jglr.2016.07.027
19. Kenow KP, Houdek SC, Fara LJ, Gray BR, Lubinski BR, Heard DJ, et al. Distribution and foraging patterns of common loons on Lake Michigan with implications for exposure to type E avian botulism. J Great Lake Res. (2018) 44:497–513. doi: 10.1016/j.jglr.2018.02.004
20. Trumpickas J, Shuter BJ, Minns CK, Cyr H. Characterizing patterns of nearshore water temperature variation in the North American Great Lakes and assessing sensitivities to climate change. J Great Lakes Res. (2015) 41:53–64. doi: 10.1016/j.jglr.2014.11.024
21. Moskal'kova KI. Ecological and morphophysiological prerequisites to range extension in the round goby Neogobius melanostomus under conditions of anthropogenic pollution. J Ichthyol. (1996) 36:584–590.
22. Kashkooli OB, Ghadami M, Amini M, Modarres R. Spatiotemporal variation of the southern Caspian Sea surface temperature during 1982-2016. J Mar Syst. (2019) 193:126–36. doi: 10.1016/j.jmarsys.2019.02.006
23. Trumpickas J, Shuter BJ, Minns CK. Forecasting impacts of climate change on Great Lakes surface water. J Great Lakes Res. (2009) 35:454–63. doi: 10.1016/j.jglr.2009.04.005
24. Lee VA, Johnson TB. Development of a bioenergetics model for the round goby (Neogobius melanostomus). J Great Lakes Res. (2005) 31:125–34. doi: 10.1016/S0380-1330(05)70244-6
25. Tierney KB, Kasurak AV, Zielinski BS, Higgs DM. Swimming performance and invasion potential of the round goby. Environ Biol Fishes. (2011) 92:491–502. doi: 10.1007/s10641-011-9867-2
26. Wolfe RK, Marsden JE. Tagging methods for the round goby (Neogobius melanostomus). J Great Lakes Res. (1998) 24:731–5. doi: 10.1016/S0380-1330(98)70857-3
27. Ray WJ, Corkum LD. Habitat and site affinity of the round goby. J Great Lakes Res. (2001) 27:329–34. doi: 10.1016/S0380-1330(01)70648-X
28. Hensler SR, Jude DJ. Diel vertical migration of round goby larvae in the great lakes. J Great Lakes Res. (2007) 33:295–302. doi: 10.3394/0380-1330(2007)33[295:DVMORG]2.0.CO;2
29. Fry FEJ. The effect of environmental factors on the physiology of fish. In:Hoar WS, Randall DJ., , editor. Fish Physiology, New York, NY: Academic Press, Inc. (1971). p. 1–98. doi: 10.1016/S1546-5098(08)60146-6
30. Lefevre S, Bayley M, McKenzie DJ. Measuring oxygen uptake in fishes with bimodal respiration. J Fish Biol. (2015) 88:206–31. doi: 10.1111/jfb.12698
31. Chabot D, McKenzie DJ, Craig JF. Metabolic rate in fishes: definitions, methods and significance for conservation physiology. J Fish Biol. (2016) 88:1–9. doi: 10.1111/jfb.12873
32. Peck MA, Moyano M. Measuring respiration rates in marine fish larvae: challenges and advances. J Fish Biol. (2016) 88:173–205. doi: 10.1111/jfb.12810
33. Brown JH, Gillooly JF, Allen AP, Savage VM, West GB. Toward a metabolic theory of ecology. Ecology. (2004) 85:1771–89. doi: 10.1890/03-9000
34. Goossens S, Wybouw N, Van Leeuwen T, Bonte D. The physiology of movement. Mov Ecol. (2020) 8:5. doi: 10.1186/s40462-020-0192-2
35. Norin T, Clark TD. Measurement and relevance of maximum metabolic rate in fishes. J Fish Biol. (2016) 88:122–51. doi: 10.1111/jfb.12796
36. Claireaux G, Lefrançois C. Linking environmental variability and fish performance: integration through the concept of scope for activity. Phil Trans R Soc B. (2007) 362:2031–2041. doi: 10.1098/rstb.2007.2099
37. Cucco A, Sinerchia M, Lefraçois C, Magni P, Ghezzo M, Umgiesser G, et al. A metabolic scope based model of fish response to environmental changes. Ecol Modell. (2012) 237:132–41. doi: 10.1016/j.ecolmodel.2012.04.019
38. Marras S, Killen SS, Lindström J, McKenzie DJ, Steffensen JF, Domenici P. Fish swimming in schools save energy regardless of their spatial position. Behav Ecol Sociobiol. (2015) 69:219–26. doi: 10.1007/s00265-014-1834-4
39. Chabot D, Steffensen JF, Farrell AP. The determination of standard metabolic rate in fishes. J Fish Biol. (2016) 88:81–121. doi: 10.1111/jfb.12845
40. Flynn EE, Todgham AE. Thermal windows and metabolic performance curves in a developing Antarctic fish. J Comp Physiol B. (2018) 188:271–82. doi: 10.1007/s00360-017-1124-3
41. Pörtner HO, Farrell AP. Physiology and climate change. Science. (2008) 322:690–2. doi: 10.1126/science.1163156
42. Marcek BJ, Humston R, Fabrizio MC, Shen J, Brill RW. Modeling the distribution of Atlantic Croaker and Spot in a dynamic seascape using metabolic scope. Estuaries Coast. (2024) 47:258–75. doi: 10.1007/s12237-023-01240-8
43. Wieser W. Developmental and metabolic constraints of the scope for activity in young rainbow trout (Salmo gairdneri). J Exp Biol. (1985) 118:133–42. doi: 10.1242/jeb.118.1.133
44. Reidy SP, Kerr SR, Nelson JA. Aerobic and anaerobic swimming performance of individual atlantic cod. J Exp Biol. (2000) 203:347–57. doi: 10.1242/jeb.203.2.347
45. Mundim KC, Baraldi S, Machado HG, Vieira FMC. Temperature coefficient (Q10) and its applications in biological systems: beyond the Arrhenius theory. Ecol Modell. (2020) 431:109127. doi: 10.1016/j.ecolmodel.2020.109127
46. Lagos ME, White CR, Marshall DJ. Do invasive species live faster? Mass-specific metabolic rate depends on growth form and invasion status. Funk Ecol. (2017) 31:2080–8086. doi: 10.1111/1365-2435.12913
47. McCallum ES, Gulas ST, Balshine S. Accurate resource assessment requires experience in a territorial fish. Anim Behav. (2017) 123:249–57. doi: 10.1016/j.anbehav.2016.10.032
48. Millidine KJ, Armstrong JD, Metcalfe NB. Presence of shelter reduces maintenance metabolism of juvenile salmon. Funct Ecol. (2006) 20:839–45. doi: 10.1111/j.1365-2435.2006.01166.x
49. Behrens JW, von Friesen LW, Brodin T, Ericsson P, Hirsch PE, Persson A, et al. Personality- and size-related metabolic performance in invasive round goby (Neogobius melanostomus). Physiol Behav. (2020) 215:112777. doi: 10.1016/j.physbeh.2019.112777
50. Clark TD, Sandblom E, Jutfelt F. Aerobic scope measurements of fishes in an era of climate change: respirometry, relevance and recommendations. J Exp Biol. (2013) 216:2771–82. doi: 10.1242/jeb.084251
51. Little AG, Dressler T, Kraskura K, Hardison E, Hendriks B, Prystay T, et al. Maxed out: optimizing accuracy, precision, and power for field measures of maximum metabolic rate in fishes. Physiol Biochem Zool. (2020) 93:243–54. doi: 10.1086/708673
52. Christensen EA, Norin T, Tabak I, van Deurs M, Behrens JW. Effects of temperature on physiological performance and behavioural thermoregulation in an invasive fish, the round goby. J Exp Biol. (2021) 224:jeb237669. doi: 10.1242/jeb.237669
53. R Core Team. R: A Language and Environment for Statistical Computing. R Foundation for Statistical Computing, Vienna, Austria. (2023). Available at: https://www.R-project.org/ (accessed September 2, 2024).
54. Bates D, Mächler M, Bolker B, Walker S. Fitting Linear Mixed-Effects Models Using lme4. J Stat Softw. (2015) 67:1–48. doi: 10.18637/jss.v067.i01
55. Kuznetsova A, Brockhoff PB, Christensen RHB. lmerTest package: tests in linear mixed effects models. J Stat Softw. (2017) 82:1–26. doi: 10.18637/jss.v082.i13
56. Jørgensen C, Enberg K, Mangel M. Modelling and interpreting fish bioenergetics: a role for behaviour, life-history traits and survival trade-offs. J Fish Biol. (2016) 88:389–402. doi: 10.1111/jfb.12834
57. Kelley AL. The role thermal physiology plays in species invasion. Conserv Physiol. (2014) 2:cou045. doi: 10.1093/conphys/cou045
58. Holt RE, Jørgensen C. Climate change in fish: effects of respiratory constraints on optimal life history and behaviour. Biol Lett. (2015) 11:20141032. doi: 10.1098/rsbl.2014.1032
59. Brownscombe JW, Smith K, Bzonek PA. Accelerometer-based swimming metabolism of smallmouth bass (Micropterus dolomieu). J Fish Biol. (2024) 105:254–64. doi: 10.1111/jfb.15774
60. Jensen DL, Overgaard J, Wang T, Gesser H, Malte H. Temperature effects on aerobic scope and cardiac performance of European perch (Perca fluviatilis). J Therm Biol. (2017) 68:162–9. doi: 10.1016/j.jtherbio.2017.04.006
61. Meisner DJ, Goodier JL, Regier HA, Shuter BJ, Christie WJ. An assessment of the effects of climate warming on Great Lakes basin fishes. J Great Lakes Res. (1987) 13:340–52. doi: 10.1016/S0380-1330(87)71656-6
62. Healy TM, Schulte PM. Thermal acclimation is not necessary to maintain a wide thermal breadth of aerobic scope in the common killifish (Fundulus heteroclitus). Physiol Biochem Zool. (2012) 85:107–19. doi: 10.1086/664584
63. Pimentel MS, Faleiro F, Diniz M, Machado J, Pousão-Ferreira P, Peck MA, et al. Oxidative stress and digestive enzyme activity of flatfish larvae in a changing ocean. PLoS ONE. (2015) 10:e0134082. doi: 10.1371/journal.pone.0134082
64. Dawood MAO. Nutritional immunity of fish intestines: important insights for sustainable aquaculture. Rev Aquacult. (2020) 13:642–63. doi: 10.1111/raq.12492
65. Brownscombe JW, Raby GD, Murchie KJ, Danylchuk AJ, Cooke SJ. An energetics-performance framework for wild fishes. J Fish Biol. (2022) 101:4–12. doi: 10.1111/jfb.15066
66. Mugwanya M, Dawood MAO, Kimera F, Sewilam H. Anthropogenic temperature fluctuations and their effect on aquaculture: a comprehensive review. Aquacult Fish. (2022) 7:223–43. doi: 10.1016/j.aaf.2021.12.005
67. Pangle KL, Sutton TM, Kinnunen RE, Hoff MH. Overwinter survival of juvenile lake herring in relation to body size, physiological condition, energy stores, and food ration. TAFS. (2004) 133:1235–46. doi: 10.1577/T03-127.1
68. Turko AJ, Firth BL, Craig PM, Eliason EJ, Raby GD, Borowiec BG. Physiological differences between wild and captive animals: a century-old dilemma. J Exp Biol. (2023) 226:jeb246037. doi: 10.1242/jeb.246037
69. Schulte PM. The effects of temperature on aerobic metabolism: towards a mechanistic understanding of the responses of ectotherms to a changing environment. J Exp Biol. (2015) 218:1856–66. doi: 10.1242/jeb.118851
70. Little AG, Loughland I, Seebacher F. What do warming waters mean for fish physiology and fisheries? J Fish Biol. (2020) 97:328–40. doi: 10.1111/jfb.14402
71. Metcalfe JD, Wright S, Tudorache C, Wilson RP. Recent advances in telemetry for estimating the energy metabolism of wild fishes. J Fish Biol. (2016) 88:284–97. doi: 10.1111/jfb.12804
72. Clarke A, Johnston NM. Scaling of metabolic rate with body mass and temperature in teleost fish. J Anim Ecol. (1999) 68:893–905. doi: 10.1046/j.1365-2656.1999.00337.x
73. Agarwal D, Shanmugam SA, Kathirvelpandian A, Eswaran S, Rather MA, Rakkannan G. Unraveling the impacts of climate change on fish physiology: a focus on temperature and salinity dynamics. J Appl Ichthyol. (2024) 2024:5782274. doi: 10.1155/2024/5782274
74. Sorensen PW. Introduction to the biology and control of invasive fishes and a special issue on this topic. Fishes. (2021) 6:69. doi: 10.3390/fishes6040069
75. Vivó-Pons A, Wallin-Kihlberg I, Olsson J, Ljungberg P, Behrens J, Lindegren M. The devil is in the details: exploring how functionally distinct round goby is among native fish in the Baltic Sea. NeoBiota. (2023) 89:161–86. doi: 10.3897/neobiota.89.110203
76. Behrenfeld MJ, Gaube P, Penna AD, O'Malley RT, Burt WJ, Hu Y, et al. Global satellite-observed daily vertical migrations of ocean animals. Nature. (2019) 576:257–61. doi: 10.1038/s41586-019-1796-9
77. Chow E, Zarini S, Coffield O, Chippindale Q, Balshine S. Swimming capacity and behaviour of juvenile round goby (Neogobius melanostomus). Ecol Freshwater Fish. (2024) 2024:e12810. doi: 10.1111/eff.12810
Keywords: ecophysiology, climate change, routine metabolic rate, maximum metabolic rate, scope for activity, invasive species, Great Lakes, Neogobius melanostomus
Citation: Herron HA, Zarini S, Thompson WA, Turko AJ and Balshine S (2025) Temperature- and ontogeny-dependent metabolism in invasive early juvenile round goby (Neogobius melanostomus). Front. Fish Sci. 2:1489837. doi: 10.3389/frish.2024.1489837
Received: 02 September 2024; Accepted: 10 December 2024;
Published: 07 January 2025.
Edited by:
Paolo Domenici, National Research Council (CNR), ItalyReviewed by:
Leobardo Manuel Gómez-Oliván, Universidad Autónoma del Estado de México, MexicoChristel Lefrancois, Environnement et Sociétés (LIENSs), France
Copyright © 2025 Herron, Zarini, Thompson, Turko and Balshine. This is an open-access article distributed under the terms of the Creative Commons Attribution License (CC BY). The use, distribution or reproduction in other forums is permitted, provided the original author(s) and the copyright owner(s) are credited and that the original publication in this journal is cited, in accordance with accepted academic practice. No use, distribution or reproduction is permitted which does not comply with these terms.
*Correspondence: Hunter A. Herron, aGVycm9uaEBtY21hc3Rlci5jYQ==