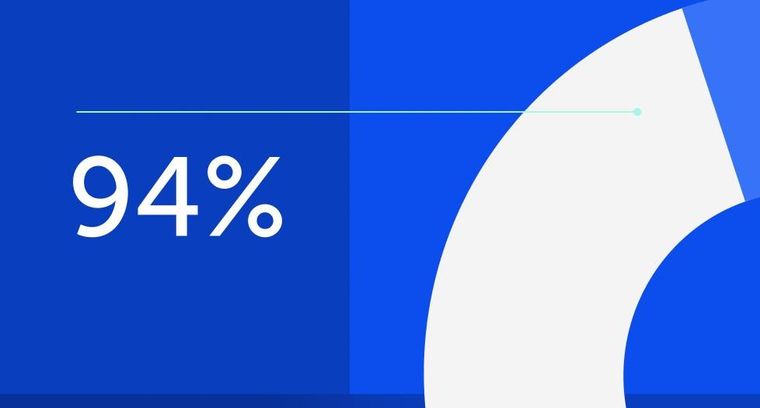
94% of researchers rate our articles as excellent or good
Learn more about the work of our research integrity team to safeguard the quality of each article we publish.
Find out more
ORIGINAL RESEARCH article
Front. Ethol., 20 March 2025
Sec. Adaptation and Evolution
Volume 4 - 2025 | https://doi.org/10.3389/fetho.2025.1520935
This article is part of the Research TopicEthology Today: Learning from the Past, Mapping the FutureView all articles
Some birds scratch their heads by moving their foot ventrally underneath their wing and others do so by moving their foot over their wing. Two competing hypotheses have been proposed to explain the distribution of these patterns. The phylogenetic hypothesis proposes that the underwing route is a novel pattern evolved in birds as the evolution of wings meant that the foot did not have to move over the front leg as is it does in quadrupeds. Consequently, the overwing route is an atavism reflecting the tetrapod ancestry of birds. The biomechanical hypothesis proposes that body morphology or environmental context determines which pattern is most effective and so explains variation across species. Earlier attempts to test these hypotheses were limited by relatively small, taxonomically biased samples of birds that did not take phylogenetic relationships into account and with few morphological traits explicitly compared. The present study includes data for 1157 species from 92% of avian families and expands the number of morphological traits compared. The most plausible ancestral state, at least for Neoaves, was overwing scratching, turning the original phylogenetic hypothesis on its head. It is also clear from the analyses that head scratching pattern is a highly labile evolutionary trait that, in some orders, repeatedly switches between over and under wing patterns. Moreover, while some morphological traits biased the likely scratching pattern used in some clades, the biomechanical hypothesis failed to predict the pattern of scratching across all birds. The most likely explanation is that the two forms of scratching are independently evolved behavior patterns and that a yet to be determined reason can switch between patterns in different lineages.
Scratching the head using a hind foot is a reflex-like action that is widespread among vertebrate tetrapods (Stein, 1983; Llinás, 2001). When a quadrupedal tetrapod, such as a dog or a cat, scratches its head, it typically raises the hind foot up and over the shoulder to contact its head (Sherrington, 1906; Kuhta and Smith, 1990). This trajectory of the foot over the shoulder is not unique to mammals, as it has also been described in reptiles (Reese, 1923; Schall and Sarni, 1987; Field and Stein, 1997; Earhart and Stein, 2000; Berkowitz, 2002) and amphibians (Schaeffer, 1911; Fukson et al., 1980; Giszter et al., 1989), suggesting that this movement pattern is a shared primitive trait. Moreover, in both reptiles and mammals it has been found that the placement of the foot and its repetitive up and down oscillating movement is controlled by neural circuits in the spinal cord (Huang et al., 1970; Mortin and Stein, 1989; Stein et al., 1995; Garcia et al., 2020). However, like many complex reflex-like actions that involve multiple body segments, there needs to be sequential or simultaneous coordination among those segments (Teitelbaum and Pellis, 1992; Pellis, 1996). In the case of head scratching, the foot is raised to a particular location in space, where oscillating movements begin, but then to achieve contact on the head, the animal makes a combination of torso, neck and head movements, indicating that supra-spinal neural circuits are also involved (Pellis, 2010). Within this broader tetrapod context, birds pose an interesting evolutionary puzzle.
When scratching, they can reach their heads with their feet in one of two ways: they can move their foot along the midline of their ventrum or they can move their foot laterally and over their wing. The former is referred to as the underwing or direct method, while the latter is the overwing or indirect method (Simmons, 1961, 1974). A clearly observable difference between the two is not only the trajectory of the foot, but also that when scratching overwing, the wing is dropped down, whereas when scratching underwing, the wing remains in place, flush against the body (Figures 1a; Simmons, 1961, 1974; Pellis, 1983, 2010). Whether scratching underwing or overwing, contact can start on any location of the head and then shifted over the head and beak by movements of the head and neck (Pellis, 1983, 2010, 2011). That is, the two methods achieve the same functional goal, but do so through a different combination of head and body movements. As the two methods are functionally equivalent, the puzzle is why some species preferentially use underwing and some overwing to scratch their heads. Two competing hypotheses have been proposed.
Figure 1. Scratching method in birds. A cormorant (Phalacrocorax varius, Suliformes) (a) and a purple-crowned lorikeet (Parvipsitta porphyrocephala, Psittaciformes) (b) are shown scratching their heads with the underwing method. An azure kingfisher (Ceyx azureus, Coraciiformes) (c) and a western whistler (Pachycephala fuliginosa, Passeriformes) (d) are shown scratching their heads with the overwing method. Two of the most recently proposed phylogenetic relationships among bird orders by Kimball et al. (2019) and Stiller et al. (2024) are shown in panel (e) Color circles at the tip of the tree branches show the scratching method for each order. Yellow = underwing, purple = overwing. The question mark indicates that the scratching methods is unknown. Photographs for a and b were provided by one of the authors (ANI), while those for c and d were kindly provided by Mike S. Y. Lee.
Lorenz proposed a phylogenetic hypothesis (Lorenz, 1950, 1958), which reflected the prevalent perspective in ethology at the time that behavior patterns are markers of phylogenetic relationships (Lorenz, 1941; Tinbergen, 1959; Van Tests, 1965). This hypothesis proposes that with the evolution of wings, the obstruction of the shoulder and forelimb was eliminated, allowing birds to use a direct method to reach the head. This, however, leaves the problem of why some birds scratch overwing. According to the phylogenetic hypothesis, the conserved, ancestral over the wing pattern that is re-expressed in some species (Lorenz, 1950; Simmons, 1957; Brown, 1975) is, an atavism (Dumont and Robertson, 1986; Kavanau, 1990).
However, as reports of head scratching accumulated, it became apparent that some bird species use both methods of scratching, and in some cases, the same individual bird can shift from one pattern to the other (Nice and Schantz, 1959a, b; Simmons, 1961; Brereton and Immelmann, 1962; Simmons, 1974; Burtt and Hailman, 1978). Also, at the time, it seemed that all birds scratched underwing when they are young, regardless of which pattern is used in adulthood (Burtt and Hailman, 1978; Medicus, 1992). Finally, some species that spend long periods of time in flight, such as frigate birds (Fregatta spp.) and swallows (Hirundinidae) scratch their heads underwing when in flight even though they scratch overwing when perched (Kramer, 1964; Burtt et al., 1988). Together, these, and related observations, led to an alternative hypothesis, that the type of head scratching implemented depends on “the bird’s posture, balance, and center of gravity at any given moment” (Blumberg, 2017, p. 3). That is, the method of head scratching used arises not because of ancestral or derived motor patterns, but because of the biases arising from biomechanical constraints (Blumberg, 2005; Burtt et al., 1988). This “biomechanical hypothesis” is in direct opposition to the phylogenetic hypothesis.
Neither hypothesis has been adequately tested, with authors supporting one hypothesis or the other using singular examples to illustrate the explanatory power of the preferred hypothesis (e.g., Blumberg, 2005; Brown, 1975). In a previous study, we used a relatively large sample of 391 species to conduct a more comprehensive test of predictions derived from the phylogenetic hypothesis, and a smaller sample of 35 species to test a prediction derived from the biomechanical hypothesis (Pellis et al., 2014). As predicted by the phylogenetic hypothesis underwing scratching is prevalent among the earliest branches of the avian tree, such as Paleognathae (e.g., ostrich, emu) and Galloanserae (e.g., pheasants, ducks), with overwing scratching appearing sporadically, and independently, in later evolved branches, such as Passeriformes (e.g., sparrows, finches) and Psittaciformes (e.g., cockatoos, parrots), but curiously, overwing scratching seemed more prevalent than the occasional atavism would predict (see Figure 4.3 in Pellis et al., 2014). A major source of anatomical variation that has implications for the posture adopted, and so the biomechanical forces experienced when raising a leg, is the relative length of the leg, particularly the tarsometatarsus (Stoessel et al., 2013). The absolute and relative length of the tarsometatarsus was not, however, associated with whether a species scratched overwing or underwing (Pellis et al., 2014). However, there were limitations in this earlier study.
First, both the number of species and the number of families represented were limited for species outside of the parrot-songbird clade (Psittacopasserae). This may have biased the overall incidence of overwing scratching, which tends to be more prevalent in this clade. Second, among the Passeriformes, the species represented were mostly from North America. As families from the Southern hemisphere diverged earlier than those from the Northern hemisphere in this order (Raikow and Bledsoe, 2000; Payevsky, 2014; Selvatti et al., 2015), the phylogenetic pattern of emergence of head scratching methods may have been misrepresented. Third, the limited number of species in some clades meant that we had to make order and family level comparisons, so limiting the ability to detect within clade transitions between the two methods of scratching, weakening the strength of the inferences made about the phylogeny of head scratching. Last, the species sampled to test the biomechanical hypothesis was insufficient to convincingly assess for the presence of possible correlations.
Here we present a much larger dataset that encompasses 1157 species from 92% of families of all birds.
With this updated data set, two predictions from the phylogenetic hypothesis and one from the biomechanical hypothesis could be tested. First, given the greater representation from non-Passeriformes and non-Psittaciformes orders, the ancestral pattern of scratching for the class was re-evaluated. The phylogenetic hypothesis posits that birds evolved underwing scratching once wings replaced forelegs. If this is so, it not only predicts that underwing scratching is the ancestral pattern in birds, but also that scratching overwing should sporadically emerge in more recently derived lineages as an atavism (Lorenz, 1950; Brown, 1975). Second, the expanded sampling of the Passeriformes and Psittaciformes, allows for a more detailed analysis to identify which lineages contain species that have reverted to the supposed pre-bird pattern of overwing scratching. If the phylogenetic hypothesis is correct, as appears to be the case for birds as a class (Pellis et al., 2014), the ancestral pattern in Psittacopasserae will be underwing scratching with a sporadic emergence of overwing scratching in latter branches. Third, if body morphology truly has no role to play in determining scratching pattern, then, in no order/family should any morphological factor influence the scratching pattern used. The expanded range of morphological traits measured and compared in the present study tested this prediction. Overall, these analyses illustrate how advances in phylogenetics (e.g., Prum et al., 2015), enlarged morphological (e.g., Tobias et al., 2022) and behavioral (present compilation) databases can be combined to give new life to old ethological questions. In this way, the classical ethology emphasis on the importance of describing behavior and placing it within a phylogenetic context (Lorenz, 1973; Tinbergen, 1963) can be revived without necessarily being committed to classical ethology explanations, some of which have proven to be deficient (e.g., Hinde, 1956; Lehrman, 1953).
To ensure as large a data set as possible, the scratching method for different bird species were obtained from variety of sources. This includes personal observations of birds both in the wild and in captivity (S.M.P and V.C.P), and where possible, were recorded on film or video (Pellis, 1983, 2010, 2011), as well as in the literature, including a systematic search of Birds of the World (Billerman et al., 2022). Additionally, we used videos and pictures of birds available in different websites. This includes the Internet Bird Collection and the Macaulay Library (https://www.macaulaylibrary.org), Wikiaves (https://www.wikiaves.com.br), Google Images, Flickr, Alamy, YouTube, Twitter, and Instagram, as well as video clips extracted from nature documentaries (see Supplementary Table 1). Given that different sources were used to derive our data set, we used a simple set of postural criteria that could be reliably applied across species regardless of the source. To determine whether a bird scratched overwing or underwing, if the leg was positioned laterally and the ipsilateral wing dropped downward from its resting position flush against the body when the foot was in contact with the head, the scratch was classified as overwing, whereas if the leg was positioned ventrally along the body and the wing remained in its resting position, flush against the body, when the foot was in contact with the head, then it was classified as underwing. As these constitute the standard set of postural criteria that have been used to document types of head scratching for decades (e.g., Nice and Schantz, 1959a, b; Simmons, 1961, 1974), we could be confident in the classification of species that we extracted from the literature. Similarly, as some of the data for some species were derived from single photographs of when the foot was in contact with the head, these postural criteria could be readily ascertained. For species we observed with the naked eye, these criteria were also readily identified whether the bird was large and relatively slow, such as goose, or small and relatively quick, such as a finch. For species which were recorded on video, either available on-line or from our own efforts, the sequence of movements could be watched in real time or slow motion, confirming the presence of these postural criteria. Moreover, the cases for which video records were available, we confirmed that all parts of the head could be contacted with both types of scratching (see Introduction).
For about 50% of the current sample, the pattern determined for a given species was corroborated by multiple reports from the literature, from photographs and video clips available online, from nature documentaries on television and by our own observations, and for some species we could determine that multiple scratches were made by the same individual bird (see columns E and G in Supplementary Table 1, respectively). In a few species, both methods of scratching have been reported (see Pellis et al., 2014 for a review of the literature and the Supplementary Table for updated data), but this co-occurrence could be present in two ways. First, many individual members of the species may scratch consistently with one method, but some individuals may scratch with the alternate method (e.g., kea, Nestor notabilis). Second, the same individual from a given species may scratch using both methods when perched (e.g., this has been reported for a few species of North American Passeriformes, Brereton and Immelmann, 1962, Supplementary Table 1). Consequently, in cases in which only a single head scratch from a single bird is available, the possibility that different members of the same species or even the same individual can scratch using both methods cannot be discounted, weakening the conclusions that can be drawn for some clades (see Discussion). However, we draw the reader’s attention to cases in which we have observed from dozens to hundreds of head scratching across many individuals, with all members of those species consistently scratching using only one method only (Pellis, 1983, 2010, 2011; Pellis and Pellis, 1982). For example, the silver gull (Larus novaehollandiae) is now represented by our personal observations from multiple sites over different locations in Australia (e.g., Queensland, Victoria), separated over several decades and including dozens of individual birds, with many individuals observed to scratch their heads multiple times. In all cases, individuals of this species have only been observed to scratch underwing. Thus, while it is still possible that some members of this species can also scratch overwing, it is reasonable to consider underwing scratching as typical of this species. Similarly, while the number of bouts of scratching were not specified, McKinney (1965) reports that, for over a dozen species of Anas, all species scratch underwing, a conclusion we have corroborated with observations of some of the same species and additional ones, including those from closely related genera (Supplementary Table 1). Consistently observed use of only one type of scratching method in closely related species increases the confidence in drawing the conclusion that a particular method is typical of those species and clades. That cases of individuals from the same species using both methods are rarely reported, suggests that, for most species, one method or the other is typically used, but given that, without more detailed experimental studies inducing scratching in more species, it cannot be discounted that at least on some occasions an individual from a given species can use the alternative scratching pattern. Therefore, representing species in this study as scratching with one method or the other, we are only inferring that this is the typical, not the exclusive, pattern for those species. As noted above, confidence in how typical one method of scratching may be for a given species varies with the data available (Supplementary Table 1).
Only a few cases of scratching while flying have been reported in the literature. However, from the cases that have been reported and from those that we have observed, scratching in flight only occurs underwing, irrespective of what pattern is used when the birds are perched. Thus, for the present study, what needs explaining is why, when perched, do different species preferentially use one method of scratching rather than the other? And so, for this reason, comparisons are only made for birds scratching when perched. Nonetheless, to make all data available for other researchers for future use, where available, how birds scratched when in flight are included in Supplementary Table 1. Similarly, it should be noted that when we found reports of, or observed immature birds scratching their heads, we included them in the Supplementary Table but given that head scratching method can change with age in some species (see Introduction), only head scratches by adults were used for species comparisons. Because we collated data from many different sources, it is not possible to provide a systematically reported sample size of observations for each species. For example, in most of the literature from which we extracted information on the head scratching of species, the number of observations is not usually given. Similarly, for many on-line sources of photographs and videoclips, if more than one scratch is present it usually cannot be inferred whether it is the same individual that is the performer. It is only in rare instances when multiple consecutive scratches on the same videoclip were seen performed by the same individual that the number of scratches for an individual could be unambiguously determined. Still, we provide the number of reports for each species and when available, the number of observations (Supplementary Table 1).
We collected data for a total of 1157 species of birds, more than 10% of all bird’s species. This includes data for 36 of the 38 orders, and 230 of the 250 families in the Clements taxonomy, and all species were named matching that taxonomy (Clements and Principe, 2000, Clements et al., 2022). We always searched pictures/videos first in the Macaulay Library because it is a reliable source for species identification and contains almost all bird species in the world. In the case of two monotypic families, the Magellanic plover (Pluvianellus socialis; Pluvianellidae) and the Plains-wanderer (Pedionomus torquatus; Pedionomidae), the scratching method was obtained from pictures sent directly to us and not available to the public (see Supplementary Table 1). Given the number of total species of birds from our sources are, by necessity, limited, the number of species that could be sampled from a family or order varied. For example, in hummingbirds (Family Trochilidae), we systematically searched scratching method for all species and managed to record the scratching method for 114 out of 365 species. In contrast, for Psittaciformes, photos, videos or personal observations could not be obtained for many species, but we could ensure that least one species from each genus was sampled, leaving only two genera not represented in our data set. Finally, for the speciose Passeriformes, species from 18 families and many genera could not be included in the data set, although the number of species and families included, especially from the Southern Hemisphere, greatly exceeded that of our earlier study (Pellis et al., 2014). That is, given the limitations in the data available, we did our best to obtain as an exhaustive and representative sample as possible.
Of course, the limitations in sampling within some orders and families may have limited our ability to assess relative differences in lability between the two methods of scratching in earlier and later branches in the avian tree. There are two considerations to bear in mind. First, videos/photos of head scratching behavior are relatively rare and many pelagic, nocturnal, and cryptically behaving species are not going to be observable, so it is not possible to sample widely in some clades. Nonetheless, as data become available in the future, some of our current analyses can be repeated to determine if including additional species changes the results. Second, ancestral state reconstruction is more dependent on sampling different clades across a tree, especially early branches, that can greatly influence tree topology (Salisbury and Kim, 2001; Li et al., 2008). Indeed, changes in topology can often affect the conclusions drawn, especially if it results in differing numbers of evolutionary changes (Iwaniuk, 2004). Since, in the present study, early branches are well represented, the topology of the trees used are likely reasonable representations of the distribution of scratching methods across the class. But it should be noted that ancestral state reconstructions are estimates and, indeed, those estimates can vary across different prior models (see below), so interpreting those reconstructions should be done cautiously.
For the phylogenetic trees, we used a subset of the Aves v1.2 tree from the package clootl in R (R Core Team, 2022; McTavish et al., 2024; Miller and McTavish, 2024), which unites phylogenetic estimates for 9,239 species from 262 phylogenies using the Open Tree synthesis algorithm. Importantly, this includes the most recent backbone of major avian clades (Figure 1e, Stiller et al., 2024) as well as the most up to date trees for species relationships within clades. In our case, we obtained a tree for 1157 species that used 186 phylogenies. Using this phylogenetic tree, we modeled the evolution of scratching method in birds using the functions fitMk from the R package phytools (Revell, 2012), which fits an extended time-continuous Markov (Mk) model for discrete character evolution (Lewis, 2001). For species that have both modes of scratching, each mode was assigned a probability of 0.5 at the tip. We compared the fit of two different models, a one-parameter equal rates (ER) model, in which a single rate is estimated for all possible transitions and an all-rates different matrix (ARD) model, in which all possible transitions between states receive distinct values. The best fitting model was chosen based on the Akaike Information Criteria (AIC) score. For all birds, within each model (ER or ARD), we used three different root priors, a “flat” root prior in which all states have equal probabilities occurring at the root (Schluter et al., 1997), a “fitzjohn” root prior, which implements the prior distribution of FitzJohn et al. (2009) and an “estimated” prior, which finds the stationary distribution of state frequencies and sets that as the prior (Revell, 2012). Using the best fitting model, we obtained marginal ancestral states using both maximum likelihood and stochastic mapping using the functions ancr and make.simmap, respectively, in the R package phytools (Revell, 2012), for each of the three priors. The two approaches differ in that maximum likelihood estimates of the ancestral state only at the nodes, while stochastic mapping generates a range of character histories or stochastic maps and allows changes to occur on the branches (Revell, 2013). The stochastic maps can then be summarized to get an estimate of ancestral states at each node (Revell, 2013; Revell and Harmon, 2022). All figures show the maximum likelihood estimates at the nodes, while the number of transitions between the two scratching modes were obtained from the stochastic mapping.
While all birds have a rigid, box-like body (Bellairs and Jenkin, 1960), there is considerable variation in body size, in the length and shape of birds’ wings (e.g., Rayner, 1988; Lockwood et al., 1998), with geographic and other intraspecific variation in wing length among adults (e.g., Hamilton, 1961; James, 1982; Copete et al., 1999) and across age (e.g., Stewart, 1963; Alatalo et al., 1984), head size and shape (Burtt, 1983), as well as the length and shape of the bill (e.g., Clayton and Cotgreave, 1994; Barbosa, 1996), and most critically for body posture and balance (Pellis et al., 2014), leg length and morphology (Zeffer and Lindhe Norberg, 2003; Stoessel et al., 2013). Therefore, in the present study, we used several morphological traits that are available for almost all species (see Tobias et al., 2022 for the AVONET database) to evaluate the impact of body morphology on scratching method used.
To assess which morphological character best predicts scratching method, we used phylogenetic logistic regression analyses with the function phyloglm (logistic_IG10 method, 1000 independent bootstrap replicates, and p-values computed using Wald tests) from the package phytools (Revell, 2012), which fits the phylogenetic logistic regression described in Ives and Garland (2010). Because phyloglm only accepts binary characters, we omitted the 25 species which scratch with both methods from this analysis. The morphological data was standardized and centered by using z-scores for comparisons. Because all morphological measurements correlate with mass and with each other, which can lead to high collinearity, we used a model with six of the morphological measurements available in the AVONET data base; body mass, beak length (nares), beak width, tarsometatarsus length, hand wing index (HWI) and tail length. We tested the levels of collinearity between variables by calculating variance inflation factors (VIF) using the vif function from the car package (Fox and Weisberg, 2019) in R. VIF values were calculated from logistic regressions identical to the PGLM models but with the phylogenetic component removed, as the meaning of VIF within the context of phylogenetic regressions is unclear. All variables in all the models had VIF values below 5. Forest plots were obtained using the plot.model function from the package sjPlot (Lüdecke, 2024).
Additionally, we tested for evolutionary covariation between scratching method and body size using the threshold model (Felsenstein, 2012; Revell, 2014), implemented in the function threshBayes of the R package phytools (Revell, 2012). Again, the 25 species with both scratching methods were omitted from this analysis. The threshold model can be used to test for evolutionary covariation between continuous (body mass) and discrete (scratching method) traits. Under the threshold model, a discrete character evolves as a function of an underlying and continuously varying attribute called ‘liability’. Once ‘liability’ crosses a value, the state of the discrete character changes in value (Felsenstein 2012; Revell, 2014). We ran 106 generations, with a burn-in of 200000, sampling every 100 generations. We also used this method to test for evolutionary covariation between scratching method and foot use during feeding (Gutiérrez-Ibáñez et al., 2023) within parrots.
To assess if there were differences in body mass or relative size of any of the morphological variables between the two methods of scratching, we ran a phylogenetic generalized least squares (PGLS) analysis to account for phylogenetic relatedness (Garland and Ives, 2000). PGLS allows the covariance matrix to be modified to accommodate the degree to which trait evolution deviates from Brownian motion, through a measure of phylogenetic correlation, λ (Pagel, 1999). Our PGLS analyses and maximum likelihood estimates of λ were performed using the ape (Paradis et al., 2004) and nlme (Pinheiro et al., 2017) packages in R. For differences in mass, we ran a simple model equivalent to a pANOVA, while for differences in relative size, we ran models equivalent to a pANCOVA with the morphological variable (e.g. beak length) as the predicted variable, body mass as the predictor and scratching methods as a categorical covariate. Figures were prepared in R (Core Team, 2022) using different functions in the package Phytools and ggplot2. Figures were then imported into Adobe Illustrator for additional adjustments.
In the ancestral state reconstruction, the best fitting model was always ARD across the three priors (Table 1). For the three root priors, the number and rate of transitions between overwing and underwing patterns were similar (Table 1), but the estimated ancestral state for all birds and some of the basal nodes within birds differed among them (Table 1; Figures 2a–c). For the “estimated” prior - both stochastic mapping and maximum likelihood estimates - yielded a high probability (> 94%) that the last common ancestor of all birds scratched underwing (Table 1; Figure 2a). In contrast, with a “flat” prior, the last common ancestor of all birds has a chance of less than 40% to have scratched underwing (Table 1; Figure 2b), and with the “Fitzjhon” prior, this probability went down to less than 8% (Table 1; Figure 2c). In other words, we found that depending on the prior used, the last common ancestor of all birds is recovered as underwing or overwing. The discrepancy between priors is similar for the last common ancestor of the Neognathae (Neoaves + Galloanserae, Table 1; Figure 2) but less for the last common ancestor of all Neoaves, which is estimated as having used an overwing scratching method with a probability of 70% to 97%. Further, all other deep nodes within Neoaves are estimated across priors as having used an overwing scratching method (Figure 2). This means that, within Neoaves, the overwing scratching method is the most likely ancestral state and underwing scratching has evolved independently many times. This is also reflected in the number and rate of transitions in our evolutionary models. For all priors, the rate of transitions from underwing to overwing scratching is 20 to 50 times lower than the rate from overwing to underwing (Table 1). Similarly, the average number of transitions from underwing to overwing scratching is less than three while the number of transitions from overwing to underwing scratching is at least 55 (Table 1).
Table 1. List of different Markov (Mk) models (ER, equal rates; ARD, all rates different) and their fits across three different root priors (see Methods).
Figure 2. Ancestral state reconstruction of scratching methods for all birds using maximum likelihood. Ancestral state reconstructions of scratching method for all birds with three different priors, an “estimated” prior (a), a “flat” prior (b) and one using the method of FitzJohn et al. (2019) (c) (see Methods for details). The color bars at the tips indicate the scratching method for each of the 1157 species. The pie charts at the nodes represent posterior probabilities of each scratching method, based on the best-defined model for each prior. Large pie charts at the nodes indicate that the proportion of neither methods was higher than 95%. Yellow = underwing, purple = overwing, teal= both methods.
With respect to body size and morphology, although there is an overlap in body size, species that scratch underwing generally have larger body sizes than species that scratch overwing (Figures 3a, b). This was supported by three different tests. First, a phylogenetic ANOVA revealed a significant difference in mass between scratching methods (F2,1155 = 3.49, p= 0.03, λ =0.996). Second, under the threshold model, we found an evolutionary covariation between body size and scratching method (r = 0.206). Finally, a logistic regression model (phyloglm), with scratching method as the predicted variable and six different morphological measurements (see Methods) as the predictors, show mass (Table 2; Figures 3a, b) and hand wing index (Figure 3c) as significant predictors of scratching method across all birds, with mass having the largest effect (Table 2). Other morphological features, including tarsus length and tail length were not significant predictors of scratching method across all birds (Table 2; Figures 3d–f).
Figure 3. Scratching method and morphology across birds. A boxplot of the log10 of body mass (g) for birds that scratch underwing, overwing or with both methods (a). Density plot showing the distribution of body mass for the different scratching methods across birds (b). Forest plot showing the standardized effect size (± 95% CI) for different predictors in a phylogenetic logistic regression model predicting scratching method. Effect sizes are expressed as odds ratios (c). Blue shows positive effects, red shows negative ones. Scatterplot showing the log10-transformed length of the tarsus (mm) plotted as a function of the body mass (g) for all species in this study (d). Scatterplot showing the log10-transformed length of the tail (mm) plotted as a function of the body mass (g) (e). Scatterplot showing Hand-Wing Index (HWI) plotted as a function of the body mass (g) for all species in this study (f). Yellow = underwing scratching, purple = overwing scratching, teal= both methods. Morphological data from Tobias et al. (2022).
Table 2. Results of phylogenetic logistic regression analyses with scratching method as the predicted variable and six different morphological characters as the predictors for all birds as well as Psittaciformes, Caprimulgiformes (Strisores), Charadriiformes and Passeriformes.
Detailed analyses of four avian orders which exhibit the most transitions between the two types of scratching reveal that ancestry tends to be the most predictive of type of scratch used. The analyses outlined below also demonstrate that in some orders, but not all, morphological variables can influence the most likely scratching method used and which variables are significant predictors varies across groups.
An ancestral state reconstruction reveals a complex evolutionary history for scratching methods within parrots (Figure 4a). While the ancestral state reconstruction for all birds recovered overwing as the ancestral state for all parrots (Figures 2, 4a), underwing scratching is ubiquitous among parrots. Of the 134 species for which we have data, 85 species scratch underwing (63%) while only 48 (35%) scratch overwing and one species uses both methods. Because most deep nodes have less than a 95% probability of having one scratching method or the other, it is not possible to estimate how many times underwing scratching evolved within parrots. However, given that some of the species that scratch underwing are nested well within clades that scratch overwing, it is likely that the transition from overwing to underwing has happened at least five times within parrots. Interestingly, our ancestral state reconstruction suggests that independently of when underwing scratching evolved in parrots, in at least three instances overwing scratching evolved de novo from an underwing scratching ancestor (Figure 4a).
Figure 4. Scratching method and morphology in Psittaciformes. Ancestral state reconstruction of scratching method for 134 species of the order Psittaciformes (Parrots) (a). Prior was set to overwing following the results of the best fitted models for all birds (Figure 2). The grey and green color bars at the tips show if each species can bring their foot to their beak (data from Gutiérrez-Ibáñez et al., 2023). The other bars indicate the scratching method for each of the 134 species. Yellow = underwing scratching, purple = overwing scratching, teal= both methods. The pie charts at each node represent posterior probabilities of each scratching method. Large pie charts indicate that the proportion of neither method was higher than 95%. Bar plot showing the proportion of species within each scratching method that are capable or not of bringing their foot to their beak while feeding (b). Density plot showing the distribution of body mass for the different scratching methods across parrots (c). Violin plot of the log10 of body mass (g) for parrots that scratch underwing or overwing (d). Forest plot showing the standardized effect size (± 95% CI) for different predictors in a phylogenetic logistic regression model predicting scratching method within parrots (e). Effect sizes are expressed as odds ratios. Blue dots/bars show positive effects, red shows negative ones. Scatterplot showing the log10-transformed length of the tarsus (mm) plotted as a function of the body mass (g) for 134 species of parrots (f). Scatterplot showing the log10-transformed length of the beak (mm) plotted as a function of the body mass (g) for parrots (g). Morphological data from Tobias et al. (2022) and the silhouette images were either made by the authors or are from phylopic.org, courtesy of Ferran Sayol, Andy Wilson and Michael Keesey and are in the public domain (as is also the case for Figures 5-7).
Unlike most other birds, many parrots can bring their foot to their beak during feeding (Gutiérrez-Ibáñez et al., 2023). Therefore, we tested if this foot to beak movement, which involves the foot travelling ventrally along the body’s midline as in underwing scratching, is correlated with underwing scratching. Under the threshold model, we found an evolutionary covariation between foot-to-beak movement and scratching method (r = -0.467). More specifically, most of the species that can bring their foot to their beak scratch underwing, a small percentage that do not do so (12.8%) also scratch underwing. Conversely, 40.8% of species that scratch overwing are also capable of bringing their foot to their beak (Figure 4b), suggesting that the correlation between foot-to-head movements during feeding and the method used to scratch the head is not always consistent. Our results, however, do show that in parrots, morphology can have some influence in scratching method used. A logistic regression shows both mass and tarsometatarsus length are significant predictors of scratching behavior (Table 2; Figures 4c–g). Under the threshold model, we also found an evolutionary covariation between body size and scratching method in parrots (r = 0.503). Therefore, as with birds in general (Figure 3), parrots that scratch underwing generally have larger body sizes than species that scratch overwing although there is some overlap in body mass between the two scratching methods exist (Figures 4c, d). Additionally, parrots that scratch overwing have relatively longer metatarsi than those that scratch underwing (Figure 4e). Within parrots no other morphological trait was related to scratching behavior (Table 2; Figures 4f, g).
We found that within Passeriformes, the largest radiation of birds accounting for more than half of all bird species (Tudge, 2008), most scratch overwing and that this is the ancestral state for this order (Figure 5a). Within the order, we found at least 30 independent transitions to underwing scratching. These transitions were not distributed uniformly across the passerine phylogeny. For example, we found no instances of underwing scratching in suboscines nor in the infraorder Corvides, a large radiation of 29 families with more than 800 species (e.g., Jønsson et al., 2011, 2017). On the other hand, we found multiple independent changes to underwing scratching in the superfamilies Passerida and Sylviida (Figure 5a). Regarding morphology, a logistic regression showed that beak width, hand wing index and tarsus length were significant predictors of scratching behavior (Table 2; Figure 5d), but unlike for parrots and birds in general, mass was not a significant predictor. Also, passerines have the opposite correlation regarding tarsometatarsus length than that present in parrots: passerines that scratch underwing tend to have longer tarsometatarsi than those that scratch overwing (Figure 5c). Although these morphological traits were significant predictors, it should be noted that the effect sizes for these traits were small, accounting for only around 5% of the variance.
Figure 5. Scratching method and morphology in Passeriformes. Ancestral state reconstruction of scratching method for 449 species of the order Passeriformes (a). Prior was set to overwing following the results of the best fitted models for all birds (Figure 2). The bars at the tips indicate the scratching method for each of the 449 species. Yellow = underwing scratching, purple = overwing scratching, teal = both methods. The pie charts at each node represent posterior probabilities of each scratching method. Forest plot showing the standardized effect size (± 95% CI) for different predictors in a phylogenetic logistic regression model predicting scratching method within songbirds (b). Effect sizes are expressed as odds ratios. Blue dots/bars show positive effects, red shows negative ones. Scatterplot showing the log10-transformed length of the tarsus (mm) plotted as a function of the body mass (g) for 449 species of Passeriformes (c). Scatterplot showing Hand-Wing Index plotted as a function of the body mass (g) for Passeriformes (d).
In the order Caprimulgiformes, which includes hummingbirds (family Trochilidae), as well swifts (Apodidae), nightjars (Caprimulgidae) and several others, we found at least seven independent transitions from overwing, the ancestral state for this clade, to underwing (Figure 6a). This includes six among hummingbirds and one in the oilbird (Steatornis caripensis; Steatornithidae). Regarding morphology, a logistic regression showed that beak length and mass are significant predictors of scratching behavior among Caprimulgiformes (Table 2; Figure 6b). In this case, Caprimulgiformes with larger body size and relatively long beaks are more likely to scratch underwing (Figures 6c, d). Note that this relationship is retained even if the sword-tailed hummingbird (Ensifera ensifera), an extreme example of an elongated beak relative to body size, is excluded.
Figure 6. Scratching method and morphology in Caprimulgiformes (Strisores). Ancestral state reconstructions of scratching method for 118 species of the order Caprimulgiformes (nightjars, nighthawks, hummingbirds and allies) (a). Prior was set to overwing following the results of the best fitted models for all birds (Figure 2). The bars at the tips indicate the scratching method for each of the 118 species. The pie charts at each node represent posterior probabilities of each scratching method. Forest plot showing the standardized effect size (± 95% CI) for different predictors in a phylogenetic logistic regression model predicting scratching method within Caprimulgiformes (b). Effect sizes are expressed as odds ratios. Blue dots/bars show positive effects, red shows negative ones. Scatterplot showing the log10-transformed length of the beak (mm) plotted as a function of the body mass (g) for 118 species of Caprimulgiformes (c). Scatterplot showing the log10-transformed length of the tail (mm) plotted as a function of the body mass (g) for Caprimulgiformes (d). Yellow = underwing scratching, purple = overwing scratching, teal = both methods.
In shorebirds (order Charadriiformes), we found three transitions to underwing scratching from an overwing scratching ancestor (Figure 7a). This includes two independent instances in the suborder Charadrii, one in the family Burhinidae (stone-curlews) and one in the Egyptian plover (Pluvianus aegyptius, Pluvianidae). The other is a single switch to underwing scratching in the last common ancestor of the other two suborders: Lari and Scalopaci. Regarding morphology, unlike other orders and birds in general, a logistic regression revealed no significant morphological trait predictors of scratching behavior, including no relationship with body mass (Table 2; Figures 7b–d).
Figure 7. Scratching method and morphology in Charadriiformes. Ancestral state reconstructions of scratching method for 98 species of the order Charadriiformes (shorebirds) (a). Prior was set to overwing following the results of the best fitted models for all birds (Figure 2). The bars at the tips indicate the scratching method for each of the 98 species. The pie charts at each node represent posterior probabilities of each scratching method. Forest plot showing the standardized effect size (± 95% CI) for different predictors in a phylogenetic logistic regression model predicting scratching method within shorebirds (a). Effect sizes are expressed as odds ratios. Blue dots/bars show positive effects, red shows negative ones. Scatterplot showing the log10-transformed length of the beak (mm) plotted as a function of the body mass (g) for 98 species of shorebirds (c). Scatterplot showing the log10-transformed length of the tarsus (mm) plotted as a function of the body mass (g) for Charadriiformes (d). Yellow = underwing scratching, purple = overwing scratching, teal = both methods.
The greatly enlarged data set used in this paper identified some significant effects of body morphology on the method of head scratching used, thus providing some support for the biomechanical hypothesis. However, even when detected, the morphological contribution was relatively small. Four conclusions can be drawn from the analyses of morphological traits. First, only mass was a significant predictor across clades, but that relationship did not necessarily apply within all orders. Second, the other morphological traits that did have an effect were not consistent across orders; some morphological predictors were idiosyncratic to a particular order or had opposing effects in different orders. For example, in Psittaciformes, longer tarsometatarsi were significantly associated with a greater likelihood of scratching overwing (Figure 4e; Table 2), but in Passeriformes, were significantly associated with a greater likelihood of scratching underwing (Figure 5c; Table 2). Third, whether the effects were across the class or limited to specific orders, the effect sizes for all morphological features were small (Table 2). In other words, even when morphological traits were associated with the increased likelihood of one scratching pattern over the other, those traits accounted for a small percentage of the interspecific variation in scratching pattern within an order. Fourth, for some orders, such as the Charadriiformes, that have considerable variation in both scratching patterns used and in body morphology, no significant influence of any morphological feature was found (Figure 7; Table 2). The data therefore do not support the hypothesis that biomechanical factors related to body morphology accounts for the type of scratching method used (Blumberg, 2005, 2017). In some lineages, some morphological traits may bias which method is more likely to be used, but these biases are far from sufficient to account for the distribution of the scratching method used across all birds (Figure 2; Table 1).
We also note that part of the rationale for the biomechanical hypothesis hinges on variability in head scratching behavior within species (Blumberg, 2005, 2017). First, while it is true that some species that scratch overwing when perched, scratch underwing when in flight (Kramer, 1964; Burtt et al., 1988), scratching overwing during flight would require dropping the ipsilateral wing to allow passage of the foot over it, which is incompatible with the aerodynamics of flight. Indeed, when perched, swallows have only been observed to scratch overwing and gulls only underwing (Supplementary Table 1), even though both use the underwing method during flight. This is why we focused on perched birds for the present study, so that the scratching behavior is not impeded by the requirements of staying aloft. Further, even in the context of being perched, only 25 species in our dataset exhibited variability among and within individuals in the method used. Second, developmental transitions from underwing as nestlings to overwing as adults occur (Burtt and Hailman, 1978) and, in at least one species, the reverse pattern occurs (see below). Despite these developmental changes in behavior pattern, the adult pattern appears to be invariable in most species. The developmental change might be associated with biomechanical constraints, but there are few developmental studies available, and they provide little kinematic information from which to draw conclusions about developmental changes in postural support, balance and center of gravity that could account for age-related changes in preferred scratching method. We cannot discount the biomechanical hypothesis entirely with respect to the development of head scratching behavior. However, intraspecific variation is low and seems an insufficient rationale to support the biomechanical hypothesis as an explanation for the evolution of head scratching behavior patterns across all birds.
Ability to use the foot/leg in a particular way is also not consistent with the biomechanical hypothesis. Many birds can reach, and grasp food items held in the beak (Gutiérrez-Ibáñez et al., 2023), and do so by a ventral approach, with the foot traveling parallel to the midline (Pellis, 1983, 2011). Yet when such birds scratch their heads, some scratch overwing (Pellis, 1983) and some scratch underwing (Pellis, 2011). Among Psittaciformes, an order in which many can reach for food in the beak, and some can even bring food up to the beak (Gutiérrez-Ibáñez et al., 2023), we did find a correlation between foot use and with head scratching underwing, but it is important to note that several species that can coordinate beak and foot movements to manipulate objects scratch overwing (Figure 4a). The findings with parrots that foot use in food handling can bias the type of scratching method points to another contributing factor as to what scratching method is used. Behavioral biases may arise not only from morphology, but also from how behavioral systems are organized (Bowers, 2017; Burghardt and Bowers, 2017). An behavioral system directly related to scratching method may be preening (Ten Cate, 1985). Nonetheless, while both the roles of morphology and behavioral organization need further study, our present findings suggest that phylogeny explains more of the distribution of scratching methods across the avian class than do these factors.
With a smaller and under sampled data set (391 species), we had previously concluded that underwing scratching was the likely ancestral state (Pellis et al., 2014), which is consistent with the original version of the phylogenetic hypothesis (Lorenz, 1950; Brown, 1975). However, with a much larger data set (1157 species), that sampled scratching behavior in a great majority of avian families (>90%), our present ancestral reconstruction does not provide a clear result in this regard (Figure 2). Depending on the prior used in our MK models, the predicted ancestral state was either overwing, underwing, or an almost equal chance of either pattern of scratching being ancestral (Figure 2; Table 1). Even though our ancestral state reconstruction provides support for both scratching methods as the ancestral state for all birds, the most parsimonious assumption would be that the ancestral state is underwing scratching. This is because all paleognaths (ratites) and Galloanserae (waterfowl and gamefowl), which are the two earliest divergent branches of birds, scratch underwing (Figure 1e, Figure 2). That said, it should be noted that all extant paleognatha have a unique combination of ecologies and morphology when compared to other birds, including larger average body size, terrestrial niches, and are either flightless or have poor flying abilities. Thus, the morphology of paleognaths likely does not reflect that of ancestral birds, which were more diverse in body size and ecology and the majority appeared to have been volant (Wang et al., 2022; Widrig and Field, 2022). Still, that not a single incidence of overwing scratching has been reported in Galloanserae would suggest that the same is true for paleognaths, irrespective of morphological considerations. Consequently, the possibility of underwing scratching being the ancestral state, as predicted by the classical phylogenetic hypothesis, cannot be discounted.
The lack of resolution could be due to an unavoidable problem with ancestral state reconstruction, in which accuracy is diminished at deeper nodes, especially in the absence of fossil evidence (e.g., Cunningham et al., 1998; Cunningham, 1999; Keating, 2023; Gearty et al., 2024). Nonetheless, it is also possible that given the widespread occurrence of both scratching methods across avian phylogeny, this uncertainty is not an artefact but may reflect the true ancestral state: both methods were present in early birds. Moreover, given that our analyses found that overwing was more likely to be the ancestral state in Neognathae, and almost certain to be the ancestral state in Neoaves (Figure 2), the presence of overwing is likely deep in the phylogeny of birds.
The present findings, while generally more supportive of the phylogenetic than the morphological hypothesis, are not fully consistent with the traditional framing of the phylogenetic hypothesis. But before exploring the consequences of our findings on the modifications to the hypothesis needed, another criticism that has been leveled against the phylogenetic hypothesis can be re-evaluated.
It would be expected that ancestral patterns emerge earlier ontogenetically than derived patterns (Medicus, 1992). The few developmental studies available show that species that scratch overwing precede that method with underwing scratching (Nice and Schantz, 1959a, b; Hailman, 1960; Berger, 1966). If overwing scratching is supposed to reflect the ancestral pattern of scratching by quadrupedal tetrapods (Lorenz, 1958), then the developmental transitions reported contradict this expectation (Medicus, 1992). The current findings do not unequivocally identify underwing scratching as the ancestral avian pattern, raising the possibility that both types of scratching were present in ancestral birds (Figure 2). If so, then it would not necessarily be the case that overwing should developmentally precede underwing. Rather, it could go in either direction. Indeed, hatchlings from the long-billed curlew (Numenius americanus) scratch overwing (Forsythe, 1973) and based on seven photographs taken from the web of different adults scratching their head, we found that the adult pattern is to scratch underwing (Supplementary Table 1). Thus, the long-billed curlew shows that a developmental transition from overwing to underwing is possible. But given that our findings have revealed that phylogenetically transitions from overwing to underwing scratching are more common than switches from underwing to overwing scratching (Figure 2), it would seem more likely that most developmental studies would find switches from overwing to underwing. Regardless of the frequency of the different directions of switching, the developmental data do not contradict a phylogenetic hypothesis for the origins and evolutionary changes in methods of head scratching.
According to the original phylogenetic hypothesis, ancestral birds switched from overwing to underwing because the wing is held flush against the body, thereby eliminating the problem of the foreleg as an obstacle as is the case in four-legged vertebrates. The purportedly “rare” cases of overwing scratching were therefore considered an atavism in which some species reverted to using the ancestral quadrupedal pattern (Lorenz, 1950; Brown, 1975). The present findings contradict both assumptions. First, as already noted, overwing scratching is either co-ancestral with underwing scratching or at least its origins are deep in avian ancestry. Second, overwing scratching is much more prevalent across birds than expected for a rare atavism, and, especially for most orders of Neoaves, the most likely switches are from overwing to underwing, the exact opposite of what is expected from the traditional phylogenetic hypothesis (Figure 2). Indeed, only in a few cases is there strong evidence for switches from underwing to overwing scratching (e.g., see Figure 4 for parrots). These findings greatly undermine the original phylogenetic hypothesis. Thus, while our findings show that phylogeny may account for most of the pattern of distribution of the two methods of head scratching, a revised conceptual framework is needed to understand how phylogeny accounts for the distribution present in birds.
What needs to be explained is why ancestral state reconstructions are ambiguous in determining the ancestral state of scratching (Figure 2). Consider a dog scratching its chest instead of its head. To gain access to these ventral regions of its upper body, its foot travels along the midline of its ventrum, not over its shoulder - a pattern we have seen repeatedly in many species of mammals (Komorowska and Pellis, 2004; Pellis, 2010; Pellis et al., 2014). Moreover, this may not be limited to mammalian quadrupeds, as studies have shown that the hindfeet of turtles can follow more than one trajectory to reach the rostral areas of their bodies (Mortin and Stein, 1989). That is, in a quadruped, there is not a single pattern of scratching with the hind foot, rather, there at least two – one to access the lateral and dorsal areas of the neck and head and one to access the ventral portions of the chest, throat and jaw. Moreover, detailed kinematic analysis of head scratching in both birds and mammals show that the two ways to access the head with the foot are truly bimodal (Pellis, 1983, 2010, 2011) – there are no intermediate trajectories, either the foot travels ventrally, close to the mid-line or laterally on the side of the body. If there are two, distinct motor patterns, then birds inherited both methods of reaching the head from their quadruped ancestors, in which case, the underwing method is not a novel innovation of ancestral birds.
In birds, the need to follow the lateral route, over the shoulder to gain access to the dorsal areas of the head would have been eliminated once the forelimb became a wing that is flush against the body. In this case, all areas of the head and neck could be accessed via the ventral route, given their short, rigid body and long, flexible neck (Bellairs and Jenkin, 1960). Whether the overwing or underwing method is used, the bird raises its foot to a level about halfway up or higher on the horizontal axis of its upper body and begins to make oscillatory scratching movements. Head and neck movements are then used to make foot-head contact, with transitions in the area being scratched involving further head and neck movements (Pellis, 1983, 2010, 2011). That is, the flexible neck provides the birds with the means to access their foot with their heads, irrespective of its final location or how it traveled there. However, the overwing method involves the bird making more leg and body/wing movements than is the case for the underwing method (Pellis, 1983, 2010, 2011; Simmons, 1961), and so potentially more difficult to execute than underwing scratching. Consistent with this possibility, it should also be noted that, in species that use the foot to grasp items held in the bill, the foot travels directly along a ventral path, and, as in underwing scratching, there are minimal compensatory postural changes involved, and this is the case whether the species scratches underwing or overwing (Pellis, 1983, 2011).
Our modified phylogenetic hypothesis is that birds inherited two independent methods for scratching the head, thereby causing the ambiguity in identifying an ancestral state (Figure 2). This new hypothesis raises two additional questions. First, how can the two scratching methods both be retained, yet in most species only one is enacted, and second, why do some lineages exhibit back and forth switching between the two methods over evolutionary time?
If the two patterns of scratching are independent, then one way to ensure priority for one over the other would be for one of them to be inhibited. How this can be achieved is illustrated at the proximate level of analysis. Many infantile reflexes disappear from the behavioral repertoire with maturation (Peiper, 1963). That these infantile reflexes are not eliminated from the repertoire but instead are inhibited by suppression of brainstem neural circuits by maturing circuits in the forebrain is made evident by their re-emergence following neurological damage to the forebrain in adulthood (Teitelbaum, 1967; Kolb and Whishaw, 2015). At an ultimate level of analysis, one can imagine that if a behavior pattern becomes non-adaptive, a genetic variant in the population that can inhibit its expression through a neural mechanism would have an advantage. Such an inhibition mechanism would also allow future evolutionary changes in which if such a behavior pattern was again advantageous, a genetic variant that inhibited the inhibitory gene, would disinhibit the behavior, without the need to re-evolve the behavior pattern de novo. There are a few examples of evolutionary patterns suggesting such inhibition and disinhibition in vertebrates (e.g., Foster and Baker, 2019), but by far the most compelling cases are for some invertebrate clades in which there are detailed analyses of both the kinematics of the behavior patterns, their underling neural circuitry and their phylogenetic distribution (Paul, 2007; Katz, 2011). How common such processes are across clades remains to be determined. but hypothetically, it may be useful for re-conceptualizing the phylogenetic hypothesis of avian head scratching. Because of this uncertainty, the scenario we suggest for how the two types of scratching has evolved in birds is highly speculative, but it may be a useful avenue to consider and to guide future research on the phylogeny of avian head scratching.
In some cases, the switching between scratching patterns may arise from morphological changes that affect the biomechanics of scratching rendering one method easier to execute than the other. This would be consistent with overwing scratching being ancestral in most lineages and then subsequent switches to underwing. Given the additional compensatory movements associated with overwing scratching (see above), morphological changes may make the overwing scratch method increasingly inefficient, leading to its inhibition and the disinhibition of underwing scratching. Our data show, however, that morphology only explains a small fraction of the variation in the method of scratching most likely used. In part, the morphological traits that we used (Table 2 and see Methods), may not adequately capture the biomechanical influences present. Future studies should consider the articular surfaces of the leg joints or the morphology of the synsacrum, as these likely reflect degrees of freedom in movement. Moreover, while any one trait may have little to no effect, the combined effects of articular joints, synsacrum and leg length could affect the center of mass (e.g., Hertel and Campbell, 2007; Andrada et al., 2015; Macaulay et al., 2023), which would affect balance and so a variety of motor actions, including the switch between overwing and underwing scratching methods. More refined measurements of body morphology and postural balance may reveal that a greater proportion of the variation in the distribution of type of scratching method used is explained by biomechanical influences. Nonetheless, given that so few individual birds can perform both scratching patterns (Figure 2; Supplementary Table 1) and that we even found that some morphological traits that can have a direct influence on postural support, such as leg length, either have no effect on the scratching pattern used or opposite effects in different clades (Figures 4, 5, 7), leads us to suspect that body morphology and biomechanics will not provide a complete explanation for the phylogenetic distribution of the two types of head scratching. Nonetheless, there are some limitations in our data that cannot completely discount a larger involvement for biomechanical factors. The biomechanical hypothesis predicts a high incidence of species/individuals using both scratching methods, a prediction not supported by our data (Figure 2). However, our data were limited by many species only being represented by one bird scratching once or even a few individuals scratching once (Supplementary Table 1). The presence of both methods may require observing many individuals scratching many times each. To test this prediction fully will require detailed studies of bird species from several key nodes of the avian tree.
Perhaps the changes in brain size and brain organization that may accompany diversification (Iwaniuk and Hurd, 2005) have inadvertent behavioral consequences (De Winter, 2005). That is, the more evolutionary transitions, the greater the likelihood that neural reorganization leads to altering the circuits in scratching pattern inhibition. If so, then branches of the avian tree that have undergone more rapid evolutionary changes would also be the branches that are more likely to show switching between methods of scratching. Indeed, Passeriformes and Psittaciformes are among the most speciose and rapidly evolving avian clades (Hackett et al., 2008; Phillimore and Price, 2008; Jetz et al., 2012) and are the clades providing the most examples of switches from overwing to underwing and, especially for parrots, which also show many switches back again from underwing to overwing (Figures 4, 5). What our data suggest is that a major component of the explanation for the distribution of head scratching likely involves altering patterns of inhibition and disinhibition over evolutionary time, supporting a revised, neural-based, phylogenetic hypothesis.
The puzzle as to why some birds scratch underwing and others overwing may best be resolved as the differential evolution of two, independently derived patterns from quadruped ancestors. That species-typical behavior patterns within lineages may appear, disappear and reappear through a process of inhibition and disinhibition has likely been underestimated in vertebrates (Kavanau, 1990). Such patterns of evolutionary change are well documented in some invertebrate lineages (Katz, 2011; Paul, 2007) and may also be more common than thought in vertebrates. The situation for the pattern of scratching in birds also suggests that not all switches in behavior across lineages may best be accounted for by adaptive changes. The emergence of some traits may reflect broader changes in neural organization, rather than specific adaptations in a particular lineage (De Winter, 2005).
The original contributions presented in the study are publicly available. This data can be found here: Figshare, https://doi.org/10.6084/m9.figshare.28550588.v2.
The animal study was approved by Animal Welfare Committee of the University of Lethbridge. The study was conducted in accordance with the local legislation and institutional requirements.
CG-I: Conceptualization, Data curation, Formal Analysis, Investigation, Methodology, Visualization, Writing – original draft, Writing – review & editing. VP: Conceptualization, Data curation, Investigation, Writing – original draft, Writing – review & editing. AI: Conceptualization, Data curation, Formal Analysis, Funding acquisition, Investigation, Methodology, Supervision, Writing – original draft, Writing – review & editing. SP: Conceptualization, Data curation, Funding acquisition, Investigation, Supervision, Writing – original draft, Writing – review & editing.
The author(s) declare that financial support was received for the research and/or publication of this article. Natural Sciences and Engineering Research Council of Canada Discovery grants: PM (grant numbers 2018-03706 and 2024-03978) and IN (grant number 2020-04899).
We would like to thank Ricardo Matus and Yvette Pauligk for providing pictures of scratching for two species of birds and Mike S. Y. Lee for providing some of the pictures that we used for Figure 1. The research was supported by grants from the Natural Sciences and Engineering Council of Canada to IN (2020-04899) and PM (grant numbers 2018-03706 and 2024-03978).
The authors declare that the research was conducted in the absence of any commercial or financial relationships that could be construed as a potential conflict of interest.
The author(s) declared that they were an editorial board member of Frontiers, at the time of submission. This had no impact on the peer review process and the final decision.
The author(s) declare that no Generative AI was used in the creation of this manuscript.
All claims expressed in this article are solely those of the authors and do not necessarily represent those of their affiliated organizations, or those of the publisher, the editors and the reviewers. Any product that may be evaluated in this article, or claim that may be made by its manufacturer, is not guaranteed or endorsed by the publisher.
The Supplementary Material for this article can be found online at: https://www.frontiersin.org/articles/10.3389/fetho.2025.1520935/full#supplementary-material
Alatalo R. V., Gustafsson L., Lundkrg A. (1984). Why young passerine birds have shorter wings than older birds? Ibis 126, 410–415. doi: 10.1111/j.1474-919X.1984.tb00264.x
Andrada E., Haase D., Sutedja Y., Nyakatura J. A., Kilbourne B. M., Denzler J., et al. (2015). Mixed gaits in small avian terrestrial locomotion. Sci. Rep. 5, 13636. doi: 10.1038/srep13636
Barbosa A. (1996). Relationship between bill morphology and preening behaviour in waders. Ethol. Ecol. Evol. 8, 291-296. doi: 10.1080/08927014.1996.9522919
Bellairs A. A., Jenkin C. R. (1960). “The skeleton of birds,” in Biology and comparative physiology of birds, vol. 1 . Ed. Marshall J. A.. (New York: Academic Press), 241–300. doi: 10.1016/B978-1-4832-3142-6.50012-4
Berkowitz A. (2002). Both shared and specialized spinal circuitry for scratching and swimming in turtles. J. Comp. Physiol. A 188, 225–234. doi: 10.1007/s00359-002-0297-7
Billerman S. M., Keeney B. K., Rodewald P. G., Schulenberg T. S. (Eds.) (2022). Birds of the world (Ithaca, NY: Cornell Laboratory of Ornithology). Available at: https://birdsoftheworld.org/bow/home (Accessed October 07, 2024).
Blumberg M. S. (2017). Development evolving: The origins and meanings of instinct. Wiley Interdiscip. Rev. Cogn. Sci. 8, e1371. doi: 10.1002/wcs.1371
Bowers R. I. (2017). “Behavior systems,” in Encyclopedia of animal cognition and behavior. Eds. Vonk J., Shackelford T. K. (Springer, Berlin, Germany), 1–8. doi: 10.1007/978-3-319-47829-6_1232-1
Brereton J. l. G., Immelmann K. (1962). Head-scratching in the psittaciformes. Ibis 104, 167–175. doi: 10.1111/j.1474-919X.1962.tb08643.x
Burghardt G. M., Bowers R. I. (2017). “From instinct to behavior systems: an integrated approach to ethological psychology,” in APA handbook of comparative psychology: Vol. 1. Basic concepts, methods, neural substrate, and behavior. Ed. Call J. (American Psychological Association, Washington, D. C), 333–364. doi: 10.1037/0000011-017
Burtt E. H. Jr. (1983). Head-scratching method in Galapagos finches unaffected by variation in cranial morphology. Wilson Bull. 95, 158–160.
Burtt E. H. Jr., Bitterbaum J., Hailman J. P. (1988). Head-scratching method in swallows depends on behavioral context. Wilson Bull. 100, 679–682.
Burtt E. H. Jr., Hailman J. P. (1978). Head-scratching among North American wood warblers (Parulidae). Ibis 120, 153–170. doi: 10.1111/j.1474-919X.1978.tb06772.x
Clayton D. H., Cotgreave P. (1994). Relationship of bill morphology to grooming behaviour in birds. Anim. Behav. 47, 195-201. doi: 10.1006/qnbe.1994.1022
Clements J. F., Schulenberg T., Iliff M. J., Fredericks T., Gerbracht J. A., Lepage D., et al. (2022). Downloadable Checklist Clements Checklist. Available online at: https://www.birds.cornell.edu/clementschecklist/download (Accessed October 07, 2024).
Copete J. L., Mariné R., Bigas D., Martínez-Vilalta A. (1999). Differences in wing shape between sedentary and migratory reed buntings Emberiza schoeniclus. Bird Study 46, 100–103. doi: 10.1080/00063659909461119
Cunningham C. W. (1999). Some limitations of ancestral character-state reconstruction when testing evolutionary hypotheses. Syst. Biol. 48, 665–674. doi: 10.1080/106351599260238
Cunningham C. W., Omland K. E., Oakley T. H. (1998). Reconstructing ancestral character states: A critical reappraisal. TREE. 13, 361–366. doi: 10.1016/s0169-5347(98)01382-2
De Winter W. (2005). “Convergent evolution in brain ‘shape’ and locomotion in primates,” in Shaping primate evolution. Eds. Anapol F., German R. Z., Jablonski N. J. (Cambridge UK: Cambridge University Press), 2016–2225. doi: 10.1017/CBO9780511542336.013
Dumont J. P. C., Robertson R. M. (1986). Neural circuits: An evolutionary perspective. Science 233, 849–853.53. doi: 10.1126/science.233.4766.849
Earhart G. M., Stein P. S. G. (2000). Step swim and scratch motor patterns in the turtle. J. Neurophysiol. 84, 2181–2190. doi: 10.1152/jn.2000.84.5.2181
Felsenstein J. (2012). A comparative method for both discrete and continuous characters using the threshold model. Am. Nat. 179, 145–156. doi: 10.1086/663681
Field E. C., Stein P. S. G. (1997). Spinal cord coordination of hindlimb movements in the turtle: Intralimb temporal relationships during scratching and swimming. J. Neurophysiol. 78, 1394–1403. doi: 10.1152/jn.1997.78.3.1394
FitzJohn R. G., Maddison W. P., Otto S. P. (2009). Estimating trait-dependent speciation and extinction rates from incompletely resolved phylogenies. Syst. Biol. 58, 595–611. doi: 10.1093/sysbio/syp067
Foster S. A., Baker J. A. (2019). Loss and re-emergence of plastic ancestral behavioural traits: Influences on phenotypic and evolutionary pattern. Anim. Behav. 155, 271–277. doi: 10.1016/j.anbehav.2019.06.013
Fukson O. I., Berkinblit M. B., Feldman A. G. (1980). The spinal frog takes into account the scheme of its body during the wiping reflex. Science 209, 1261–1263. doi: 10.1126/science.7403886
Garcia I. G. A., Dueñas-Jiménez J. M., Castillo L., Oscuna-Carrasco L. P., de la Torre Valdovinos B., Castañeda-Arrellano R., et al. (2020). Fictive scratching patterns in brain cortex-ablated midcollicular decerebrate and spinal cats. Front. Neural Circuits 14. doi: 10.3389/fncir.2020.00001
Garland T. Jr., Ives A. R. (2000). Using the past to predict the present: confidence intervals for regression equations in phylogenetic comparative methods. Am. Nat. 155, 346–364. doi: 10.1086/303327
Gearty W., Allen B. J., Godoy P. L., Chiarenza A. A. (2024). The impact of tip age distribution on reconstructing trait evolution using phylogenetic comparative methods. ecoevorxiv.org. doi: 10.32942/X27K79
Giszter S. F., McIntyre J., Bizzi E. (1989). Kinematic strategies and sensorimotor transformations in the wiping movements of frogs. J. Neurophysiol. 62, 750–767. doi: 10.1152/jn.1989.62.3.750
Gutiérrez-Ibáñez C., Amaral-Peçanha C., Iwaniuk A. N., Wylie D. R., Baron J. (2023). Online repositories of photographs and videos provide insights into the evolution of skilled hindlimb movements in birds. Commun. Biol. 6, 781. doi: 10.1038/s42003-023-05151-z
Hackett S. J., Kimball R. T., Reddy S., Bowie R. C. K., Braun E. L., Braun M. J., et al. (2008). A phylogenetic study of birds reveals their evolutionary history. Science 320, 1763–1767. doi: 10.1126/science.1157704
Hailman J. P. (1960). Direct and indirect scratching by fledged mockingbird Mimus polyglottis. Ibis 102, 129–131. doi: 10.1111/j.1474-919X.1960.tb05102.x
Hamilton T. H. (1961). The adaptive significances of intraspecific trends of variation in wing length and body size among bird species. Evol. 15, 180–195. doi: 10.1111/j.1558-5646.1961.tb03142.x
Hertel F., Campbell K. E. (2007). The antitrochanter of birds: form and function in balance. Auk 124, 789–805. doi: 10.1093/auk/124.3.789
Hinde R. A. (1956). Ethological models and the concept of ‘drive’. Brit. J. Philos. Sci. 6, 321–331. doi: 10.1093/bjps/vi.24.321
Huang C. C., Lau C. M., Peng M. T. (1970). Elicitation of scratching movements by mechanical stimulation to spinal cord in decerebrate cats. Jpn. J. Physiol. 20, 365–369. doi: 10.2170/jjphysiol.20.365
Ives A. R., Garland T. Jr. (2010). Phylogenetic logistic regression for binary dependent variables. Syst. Biol. 59, 9–26. doi: 10.1093/sysbio/syp074
Iwaniuk A. N. (2004). Brood parasitism and brain size in cuckoos: A cautionary tale of the use of modern comparative methods. Int. J. Comp. Psych. 17, 17–33. doi: 10.46867/IJCP.2004.17.01.05
Iwaniuk A. N., Hurd P. L. (2005). The evolution of cerebrotypes in birds. Brain Behav. Evol. 65, 215–230. doi: 10.1159/000084313
Jetz W., Thomas G. H., Joy J. B., Hartmann K., Mooers A. O. (2012). The global diversity of birds in space and time. Nature 491, 444–448. doi: 10.1038/nature11631
Jønsson K. A., Borregaard M. K., Carstensen D. W., Hansen L. A., Kennedy J. D., Machac A., et al. (2017). Biogeography and biotic assembly of Indo-Pacific corvoid passerine birds. Annu. Rev. Ecol. Evol. Syst. 48, 231–253. doi: 10.1146/annurev-ecolsys-110316-022813
Jønsson K. A., Fabre P.-H., Ricklefs R. E., Fjeldså J. (2011). Major global radiation of corvoid birds originated in the proto-Papuan archipelago. Proc. Natl. Acad. Sci. U. S. A. 108, 2328–2333. doi: 10.1073/pnas.1018956108
Katz P. S. (2011). Neural mechanisms underlying the evolvability of behavior. Philos. Trans. R. Soc B Biol. Sci. 366, 2086–2099. doi: 10.1098/rstb.2010.0336
Kavanau J. L. (1990). Conservative behavioural evolution the neural substrate. Anim. Behav. 39, 758–767. doi: 10.1016/S0003-3472(05)80387-2
Keating J. N. (2023). What is the best method for estimating ancestral states from discrete characters? bioRxiv. doi: 10.1101/2023.08.31.555762
Kimball R. T., Oliveros C. H., Wang N., White N. D., Barker F. K., Field D. J., et al. (2019). A phylogenomic supertree of birds. Diversity 11, 109. doi: 10.3390/d11070109
Kolb B., Whishaw I. Q. (2015). Fundamentals of human neuropsychology. 7th Ed (New York: Worth Publishing).
Komorowska J., Pellis S. M. (2004). Regulatory mechanisms underlying novelty-induced grooming in the laboratory rat. Behav. Process. 67, 287–293. doi: 10.1016/j.beproc.2004.05.001
Kramer P. (1964). Kratz- und andere Putzbewegungen bei Fregattvögeln. J. Ornithol. 105, 340–343. doi: 10.1007/BF01672247
Kuhta P. C., Smith J. L. (1990). Scratching in normal cats: Hindlimb kinematics and muscle synergies. J. Neurophysiol. 64, 1653–1667. doi: 10.1152/jn.1990.64.6.1653
Lehrman D. S. (1953). A critique of Konrad Lorenz’s theory of instinctive behavior. Quart. Rev. Biol. 28, 337–363. doi: 10.1086/399858
Lewis P. O. (2001). A likelihood approach to estimating phylogeny from discrete morphological character data. Syst. Biol. 50, 913–925. doi: 10.1080/106351501753462876
Li G., Steel M., Zhang L. (2008). More taxa are not necessarily better for the reconstruction of ancestral character states. Syst. Biol. 57, 647–653. doi: 10.1080/10635150802203898
Lockwood R., Swaddle J. P., Rayner J. M. V. (1998). Avian wingtip shape reconsidered: wingtip shape indices and morphological adaptations to migration. J. Avian Biol. 29, 273–292. doi: 10.2307/3677110
Lorenz K. (1950). The comparative method in studying innate behavior patterns. Symp. Soc Exp. Biol. 4, 221–268.
Lorenz K. (1958). The evolution of behavior. Sci. Am. 199, 67–78. doi: 10.1038/scientificamerican1258-67
Lorenz K. Z. (1973). The fashionable fallacy of dispensing with description. Naturwiss. 60, 1–19. doi: 10.1007/BF00591758
Lüdecke D. (2024). sjPlot: Data visualization for statistics in social science. Available online at: https://strengejacke.github.io/sjPlot/ (Accessed October 07, 2024).
Macaulay S., Hoehfurtner T., Cross S. R. R., Marek R. D., Hutchinson J. R., Schachner E. R., et al. (2023). Decoupling body shape and mass distribution in birds and their dinosaurian ancestors. Nat. Commun. 14, 1575. doi: 10.1038/s41467-023-37317-y
McKinney F. (1965). The comfort movements of Anatidae. Behaviour 25, 120–220. doi: 10.1163/156853965X00138
McTavish E. M., Gerbracht J. A., Holder M. T., Iliff M. J., Lepage D., Rasmussen P., et al. (2024). A complete and dynamic tree of birds. bioRxiv. doi: 10.1101/2024.05.20.595017
Medicus G. (1992). The inapplicability of the biogenetic rule to behavioral development. Hum. Dev. 35, 1–8. doi: 10.1159/000277108
Miller E., McTavish E. J. (2024). clootl: Fetches and manipulates the clootl avian phylogeny. Available online at: https://github.com/eliotmiller/clootl (Accessed October 07, 2024).
Mortin L. I., Stein P. S. G. (1989). Spinal cord segments containing key elements of the central pattern generators for three forms of scratch reflex in the turtle. J. Neurosci. 9, 2285–2296. doi: 10.1523/JNEUROSCI.09-07-02285.1989
Nice M. M., Schantz W. E. (1959a). Head-scratching movements in birds. Auk 76, 339–342. doi: 10.2307/4081811
Pagel M. (1999). Inferring the historical patterns of biological evolution. Nature 401, 877–884. doi: 10.1038/44766
Paradis E., Claude J., Strimmer K. (2004). APE: analyses of phylogenetics and evolution in R language. Bioinform. 20, 289–290. doi: 10.1093/bioinformatics/btg412
Paul D. H. (2007). “Evolutionary neuroethology: a case study. Origins and evolution of novel forms of locomotion in hippid sand crabs. (Malacostraca, Decapoda, Anomala),” in Evolution of nervous systems. Ed. Kaas J. H. (Academic Press, Oxford, UK), 99–119. doi: 10.1016/b0-12-370878-8/00109-9
Payevsky V. A. (2014). Phylogeny and classification of passerine birds, Passerformes. Biol. Bull. Rev. 4, 143–156. doi: 10.1134/S2079086414020054
Peiper A. (1963). Cerebral function in infancy and childhood (New York: Consultants Bureau). doi: 10.1542/peds.34.2.270
Pellis S. M. (1983). Development of head and foot coordination in the Australian magpie Gymnorhina tibicen, and the function of play. Bird Behav. 4, 57–62. doi: 10.46867/IJCP.2011.24.03.04
Pellis S. M. (1996). ““Righting and the modular organization of motor programs,” in Measuring movement and locomotion: From invertebrates to humans. Eds. Ossenkopp K.-P., Kavaliers M., Sanberg P. R. (Landes Company, Austin, TX), 115–133.
Pellis S. M. (2010). Conservative motor systems, behavioral modulation and neural plasticity. Behav. Brain Res. 214, 25–29. doi: 10.1016/j.bbr.2010.04.048
Pellis S. M. (2011). Head and foot coordination in head scratching and food manipulation by purple swamp hens (Porphyrio porphyrio): rules for minimizing the computational costs of combining movements from multiple parts of the body. Int. J. Comp. Psychol. 24, 255–271. doi: 10.46867/IJCP.2011.24.03.04
Pellis S. M., Pellis V. C. (1982). Do post-hatching factors limit clutch size in the Cape Barren goose (Cereopsis novaehollandiae Latham)? Aust. Wildlife Res. 9, 145–149. doi: 10.1071/WR9820145
Pellis S. M., Pellis V. C., Iwaniuk A. N. (2014). Pattern in behavior: the characterization, origins and evolution of behavior patterns. Adv. Stud. Behav. 46, 127–189. doi: 10.1016/B978-0-12-800286-5.00004-3
Phillimore A. B., Price T. D. (2008). Density-dependent cladogenesis in birds. PloS Biol. 6, e71. doi: 10.1371/journal.pbio.0060071
Pinheiro J., Bates D., DebRoy S., Sarkar D., Heisterkamp S., Van Willigen B., et al. (2017). Package ‘nlme.’ Linear and nonlinear mixed effects models, version 3. R package version 3.57 (2007), 1–89.
Prum R., Berv J., Dornburg A., Field D. J., Townsend J. P., Lemmon E. M., et al. (2015). Nature 526, 569–573. doi: 10.1038/nature15697
Raikow R. J., Bledsoe A. H. (2000). Phylogeny and evolution of passerine birds. BioSci. 50, 487–499. doi: 10.1641/0006-3568(2000)050[0487:PAEOTP]2.0.CO;2
Rayner J. M. V. (1988). Form and function in avian flight. Curr. Ornithol. 5, 1–66. doi: 10.1007/978-1-4615-6787-5_1
R Core Team (2022). R: A language and environment for statistical computing. Available online at: https://www.R-project.org/ (Accessed October 07, 2024).
Reese A. M. (1923). Some reactions of Alligator mississipiensis. J. Comp. Psychol. 3, 51. doi: 10.1037/h0075964
Revell L. J. (2012). Phytools: an R package for phylogenetic comparative biology (and other things). MEE. 3, 217–223. doi: 10.1111/j.2041-210X.2011.00169.x
Revell L. J. (2013). Two new graphical methods for mapping trait evolution on phylogenies. MEE. 4, 754–759. doi: 10.1111/2041-210X.12066
Revell L. J. (2014). Ancestral character estimation under the threshold model from quantitative genetics. Evol. 68, 743–759. doi: 10.1111/evo.12300
Revell L. J., Harmon L. J. (2022). Phylogenetic comparative methods in R (Princeton, NJ: Princeton University Press).
Salisbury B. A., Kim J. (2001). Ancestral state estimation and taxon sampling density. Syst. Biol. 50, 557–564. doi: 10.1080/10635150119819
Schall J. S., Sarni G. A. (1987). Malarial parasitism and behavior of the lizard, Sceloporus occidentalis. Copeia 1987, 84–93. doi: 10.2307/1446041
Schluter D., Price T., Mooers A. Ø., Ludwig D. (1997). Likelihood of ancestor states in adaptive radiation. Evol. 51, 1699–1711. doi: 10.1111/j.1558-5646.1997.tb05095.x
Selvatti A. P., Gonzaga L. P., de Moraes Russo C. A. (2015). A paleogene origin for crown passerines and the diversification of oscines in the New World. Mol. Phylogenet. Evol. 88, 1–15. doi: 10.1016/j.ympev.2015.03.018
Simmons K. E. L. (1957). The taxonomic significance of the head-scratching methods of birds. Ibis 99, 178–181. doi: 10.1111/j.1474-919X.1957.tb01944.x
Simmons K. E. L. (1961). Problems of head-scratching in birds. Ibis 103, 37–49. doi: 10.1111/j.1474-919X.1961.tb02419.x
Stein P. S. G., Victor J. C., Field E. C., Currie S. N. (1995). Bilateral control of hindlimb scratching in the spinal turtle: Contralateral spinal circuitry contributes to the normal ipsilateral motor pattern of fictive rostral scratching. J. Neurosci. 15, 4343–4355. doi: 10.1523/JNEUROSCI.15-06-04343.1995
Stewart I. F. (1963). Variation of wing length with age. Bird Study 10, 1–9. doi: 10.1080/00063656309476036
Stiller J., Feng S., Chowdhury A.-A., Rivas-González I., Duchêne D. A., Fang Q., et al. (2024). Complexity of avian evolution revealed by family-level genomes. Nature 629, 851–860. doi: 10.1038/s41586-024-07323-1
Stoessel A., Kilbourne B. M., Fischer M. S. (2013). Morphological integration versus ecological plasticity in the avian pelvic limb skeleton. J. Morphol. 274, 483–495. doi: 10.1002/jmor.20109
Teitelbaum P., Pellis S. M. (1992). Towards a synthetic physiological psychology. Psychol. Sci. 3, 4–20. doi: 10.1111/j.1467-9280.1992.tb00249.x
Ten Cate C. (1985). Functional aspects of head-scratching methods and other preening movements in birds. Ardea 73, 99–104.
Tinbergen N. (1959). Comparative studies of the behaviour of gulls (Laridae): A progress report. Behaviour 15, 1–70. doi: 10.1163/156853960X00098
Tinbergen N. (1963). On aims and methods of ethology. Z. Tierpsychol. 20, 410–433. doi: 10.1111/j.1439-0310.1963.tb01161.x
Tobias J. A., Sheard C., Pigot A. L., Devenish A. J. M., Yang J., Sayol F., et al. (2022). AVONET: Morphological, ecological and geographical data for all birds. Ecol. Lett. 25, 581–597. doi: 10.1111/ele.13898
Tudge C. (2008). The bird. A natural history of who birds are, where they come from and how they live (Crown Publishers, NY).
Van Tests G. F. (1965). A comparative study of some social communication patterns in the Pelecaniformes. Ornithol. Monogr. 2, 1–88. doi: 10.2307/40166662
Wang S., Ma Y., Wu Q., Wang M., Hu D., Sullivan C., et al. (2022). Digital restoration of the pectoral girdles of two Early Cretaceous birds and implications for early-flight evolution. eLife 0, e76086. doi: 10.7554/eLife.76086
Widrig K., Field D. J. (2022). The evolution and fossil record of palaeognathous birds (Neornithes: Palaeognathae). Diversity 14, 105. doi: 10.3390/d1402025
Keywords: bird phylogeny, behavior patterns, evolution, inhibition, disinhibition, atavism
Citation: Gutiérrez-Ibáñez C, Pellis VC, Iwaniuk AN and Pellis SM (2025) Over or under: new phylogenetic insights in the evolution of head scratching in birds. Front. Ethol. 4:1520935. doi: 10.3389/fetho.2025.1520935
Received: 31 October 2024; Accepted: 26 February 2025;
Published: 20 March 2025.
Edited by:
Michel Baguette, Muséum National d’Histoire Naturelle, FranceReviewed by:
Virginia Pallante, Netherlands Institute for the Study of Crime and Law Enforcement (NSCR), NetherlandsCopyright © 2025 Gutiérrez-Ibáñez, Pellis, Iwaniuk and Pellis. This is an open-access article distributed under the terms of the Creative Commons Attribution License (CC BY). The use, distribution or reproduction in other forums is permitted, provided the original author(s) and the copyright owner(s) are credited and that the original publication in this journal is cited, in accordance with accepted academic practice. No use, distribution or reproduction is permitted which does not comply with these terms.
*Correspondence: Cristián Gutiérrez-Ibáñez, Y2d1dGllcnJAdWFsYmVydGEuY2E=; Vivien C. Pellis, cGVsbHZjQHVsZXRoLmNh; Andrew N. Iwaniuk, YW5kcmV3Lml3YW5pdWtAdWxldGguY2E=; Sergio M. Pellis, cGVsbGlzQHVsZXRoLmNh
Disclaimer: All claims expressed in this article are solely those of the authors and do not necessarily represent those of their affiliated organizations, or those of the publisher, the editors and the reviewers. Any product that may be evaluated in this article or claim that may be made by its manufacturer is not guaranteed or endorsed by the publisher.
Research integrity at Frontiers
Learn more about the work of our research integrity team to safeguard the quality of each article we publish.