- 1Department of Biology, Saint Louis University, St. Louis, MO, United States
- 2Department of Biological Sciences, University of Pittsburgh, Pittsburgh, PA, United States
- 3Department of Integrative Biology, University of Colorado Denver, Denver, CO, United States
Producing and maintaining sexually selected ornaments often hinders survival. Because viability-related traits dictate the survival costs conferred by sexual ornaments, the evolution of viability-related traits can limit and/or compensate for ornament evolution. Here, we examine how the ornamental coloration of male dragonflies co-adapts with thermal physiology—a key suite of viability-related traits that influences nearly all reproductive and ecological interactions. Males of many dragonfly species produce dark color patches on their wings to attract potential mates and intimidate reproductive rivals. However, dark coloration also subjects male dragonflies to heat stress in warm climates by absorbing excess solar radiation. Our phylogenetic comparative analyses revealed that dragonfly species with dark sexual coloration have also evolved increased critical thermal maxima, which may allow them to compensate for ornament-induced heating. This pattern of correlated evolution was especially strong for species that inhabit tropical climates, where the heating costs of dark coloration are most severe. Given that darkened sexual coloration is taxonomically widespread and consistently elevates body temperatures, the pattern of co-adaptation between sexual ornaments and thermal physiology found here could represent a key process driving eco-physiological divergence in the past and influencing how populations respond to the changing climates of the future.
Introduction
Being attractive to mates and intimidating to reproductive rivals often comes at a severe cost to survival (Andersson, 1994; Wiens and Tuschhoff, 2020). How animals balance these conflicting demands of survival and reproduction fundamentally influences how they adapt to their environments (Reznick et al., 2000). Because the elaborate sexual ornaments used in mate attraction and competition frequently require extra resources to produce, are more conspicuous to predators, and dramatically impair immune function (Zuk and Kolluru, 1998; Kotiaho, 2001), many animals evolve reduced ornaments to ensure survival under challenging conditions (Wiens, 2001; Cornwallis and Uller, 2010; Maan and Seehausen, 2011; Podos, 2021). Conversely, viability-related traits can also evolve to compensate for the negative fitness effects of sexual traits, allowing ornaments to become enigmatically exaggerated despite their predicted costs (Oufiero and Garland, 2007; Husak et al., 2011). By examining how viability-related traits and sexual ornaments co-adapt across species that inhabit diverse environments, we can gain a more general understanding of how selection jointly operates on all aspects of an organism’s phenotype across multiple ecological and reproductive contexts (Husak and Swallow, 2011).
One important suite of viability-related traits that may limit and/or compensate for the evolution of sexual characters is thermal physiology. Because producing a wide variety of sexually selected traits can alter an organism’s body temperature, the ability to tolerate extreme temperatures should dictate the costs of and limits on sexual trait evolution (reviewed in Leith et al., 2022). For instance, many animals use dark, saturated color patterns to attract mates and intimidate rivals (Cuthill et al., 2017), which can elevate body temperatures by absorbing excess solar radiation (West and Packer, 2002; Moore et al., 2019). Enlarged sexual ornaments and weapons can also predispose organisms to thermal stress by slowing down heating and cooling rates (Shepherd et al., 2008; Darnell and Munguia, 2011). Engaging in courtship and competitive behavior can even require organisms to use thermally stressful microhabitats (Saeki et al., 2005; Darnell et al., 2020; Moore et al., 2024b). When producing a sexual trait pushes body temperatures beyond the limits of physiological performance, the evolution of thermal physiology should limit which species can evolve sexual ornaments, and/or compensate for the heating costs of ornament exaggeration (Leith et al., 2022). Nevertheless, although we are increasingly recognizing that environmental temperatures can limit reproductive performance (Brandt et al., 2018; Macchiano et al., 2019, 2023; Leith et al., 2020) and shape the outcomes sexual selection (Jocson et al., 2019; Moore et al., 2019; Rosenthal and Elias, 2019; García-Roa et al., 2020; Leith et al., 2021), we have only recently come to appreciate how thermal physiology and sexual traits evolve together to enhance mating success (Leith et al., 2022).
Here, we examine how heat-absorbing sexual ornaments co-adapt with physiological heat tolerance in dragonflies. Males of many odonate species (dragonflies and damselflies) produce dark ornamental coloration on their wings to intimidate reproductive rivals, attract potential mates, and signal species identity (Paulson, 1974; Corbet, 1999; Guillermo-Ferreira et al., 2014; Zheng et al., 2017; Suárez-Tovar et al., 2022; Figure 1A). However, producing extensive wing coloration also causes males to absorb more solar radiation, elevating body temperatures (Moore et al., 2019; Schreiner et al., 2020; Svensson et al., 2020; Laakso et al., 2021; Figure 1B). This additional heating can dramatically reduce flight performance in warm environments, forcing males to either abandon their reproductive territories or risk physiological stress (Moore et al., 2019). As a result, hotter historical climates and recent climate warming consistently cause dragonflies to evolve reduced wing coloration, potentially at a severe detriment to mating success (Svensson and Waller, 2013; Moore et al., 2019, 2021). We might therefore expect either that low heat tolerance limits the possession of ornamentation in warm climates, or that ornamented species have evolved higher heat tolerance to compensate for the heating costs of exaggerated ornaments. To test our hypotheses, we used a combination of phylogenetic comparative methods across 19 Nearctic dragonfly species.
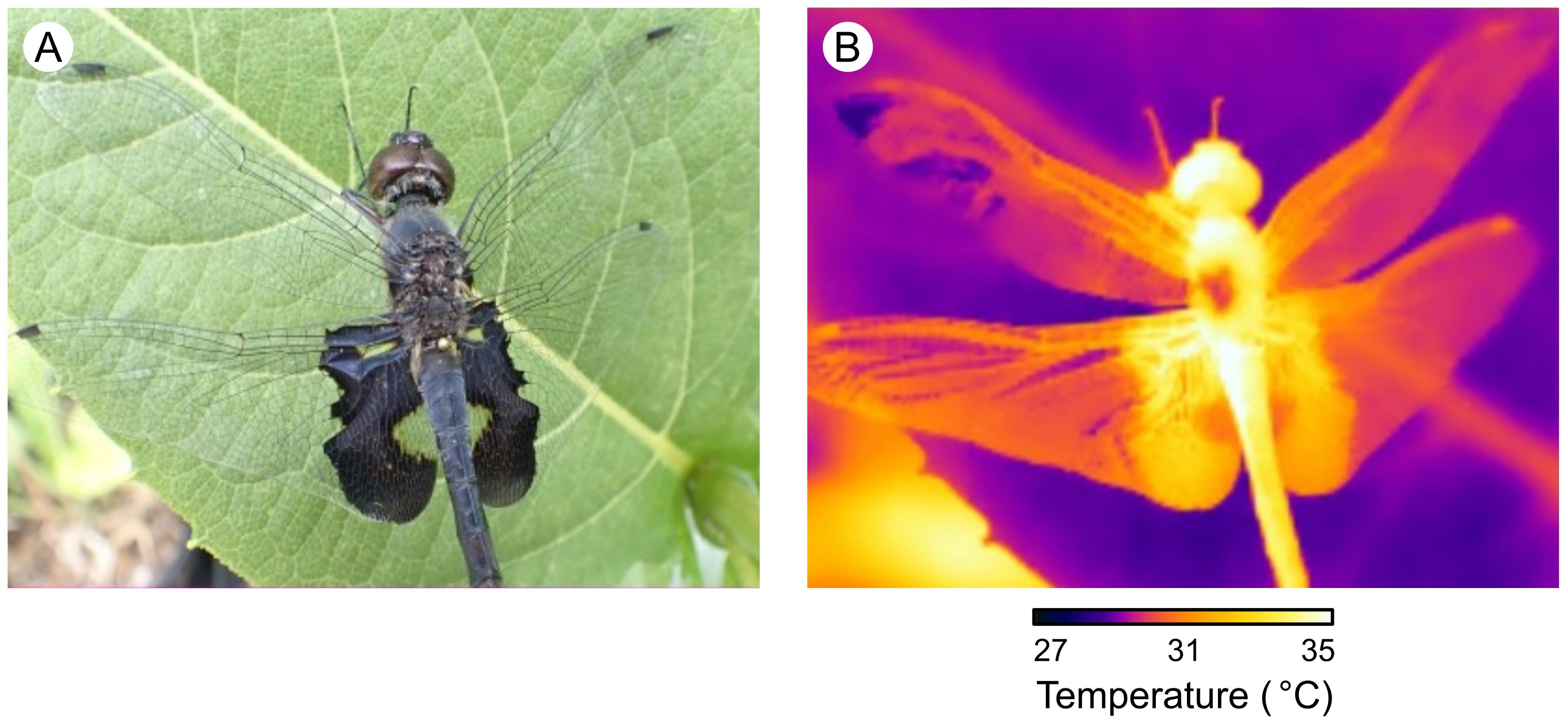
Figure 1. Heating effects of dark wing coloration. (A) A visual-spectrum image showing the dark sexual color patches on the wings of a Tramea sp. dragonfly. (B) An infrared-spectrum image of the dragonfly in (A) showing the heating effect of dark wing coloration, with lighter colors indicating hotter temperatures.
Methods
Study design
We took a phylogenetic comparative approach with published datasets to test how the evolution of critical thermal maxima (CTmax; heat tolerance) limits and/or compensates for the evolution of dark wing coloration. We first gathered data on the presence/absence of male wing coloration and CTmax for 19 species of Nearctic dragonflies from publicly available datasets (Bennett et al., 2018; Moore et al., 2021; Castillo-Pérez et al., 2022; Moore et al., 2024b). Briefly, species were scored as having wing coloration if field guides and images from iNaturalist observations showed that at least some individuals express dark pigmentation on wing cells other than the pterostigma (Paulson, 2009, 2012; Moore et al., 2021). We then pruned the maximum credibility clade of Rocha-Ortega et al. (2020) to include the thirteen species for which CTmax values were measured by May (1976) and compiled by Bennett et al. (2018), along with the seven species for which CTmax values were measured by Castillo-Pérez et al. (2022). Two species in May (1976) do not have a known phylogenetic position and were not included. Because CTmax values for Erythemis plebeja were reported by both sources, we used the mean of the two values for this species in our analyses. Importantly, the procedures used to measure CTmax differ slightly between May (1976) and Castillo-Pérez et al. (2022). However, supplemental analyses that only included the thirteen species measured by May (1976) produced similar results as to including all available species (Supplementary Tables S4, S5; Results). Results were also similar when we only examined the fifteen species in our dataset that are in the family Libellulidae (Supplementary Tables S4, S5; Results). We ultimately used all available species in our analyses to explore patterns of co-adaptation across a broad taxonomic range.
Testing for the correlated evolution of heat tolerance and sexual coloration
To test for the correlated evolution of CTmax and male wing coloration, we constructed phylogenetic generalized least squares models (‘phytools’; Revell, 2012) including CTmax as the response variable, wing coloration (yes/no) as a fixed effect, and the latitude where CTmax was sampled as a covariate. However, the effect of CTmax latitude was not significant and was removed from the model for parity with subsequent analyses. To assess which model of evolutionary history we should use to correct the residuals (Revell, 2010), we used AIC to compare among a Brownian motion model (df = 3; AIC = 91.8; Supplementary Table S1), a model that uses Pagel’s λ branch-length transformation (df = 4; AIC = 81.4; Supplementary Table S1), and a model without phylogeny (df = 3; AIC = 80.5; Supplementary Table S1). The model without phylogeny showed the most support and was used in our final analyses. However, all models produced similar results (see Results; Supplementary Table S2), indicating that any uncertainty in phylogenetic signal likely has little impact on our observed patterns of correlated evolution. To test if species with dark wing coloration have higher heat tolerances, we conducted a likelihood ratio test between a model with an effect of wing coloration (yes/no) and an intercept-only model. We further examined whether the darkness (dark = 2; light = 1; none = 0; Moore et al., 2021) and position of male wing coloration (2 = adjacent to the body; 1 = distal; 0 = none) was correlated with the evolution of CTmax using phylogenetic generalized least squares (Supplementary Material). These analyses show that species with the highest CTmax also have the darkest wing coloration and coloration that is concentrated close to the body—traits that should exacerbate ornament-induced heating (Supplementary Material).
Because the heating costs of dark ornaments should be more severe in hotter environments (Moore et al., 2019), we also tested if the correlation between CTmax and the presence of wing coloration was stronger when we only included species that inhabit tropical climates. We compared the average temperatures found across species’ entire ranges (Vilela and Villalobos, 2015; Fick and Hijmans, 2017; Moore et al., 2021, 2024b) and grouped species in our analysis into five temperate (11.9 – 14.7°C; Supplementary Figure S1; Figure 2B) and fourteen tropical species (18.6 – 25.5°C; Supplementary Figure S1; Figure 2B). See Supplementary Material for methods regarding the climate values for each species. Using only the fourteen tropical species, we constructed another phylogenetic generalized least squares model with CTmax as the response variable and wing coloration (yes/no) as a fixed effect. The model with Pagel’s λ branch-length transformation did not converge and was not considered further. The model without phylogeny (df = 3; AIC = 60.1; Supplementary Table S1) received better support than the model under Brownian motion (df = 3; AIC = 71.7; Supplementary Table S1) and was used in our final analyses; but both models again produced similar results (Supplementary Table S2). We used a likelihood ratio test to confirm that tropical species with wing coloration had significantly higher CTmax than tropical species without wing coloration. To evaluate if CTmax is more strongly correlated with wing coloration in tropical species (sensu Svensson and Waller, 2013), we compared standardized effect estimates of the wing color effect between this model and the model with all species.
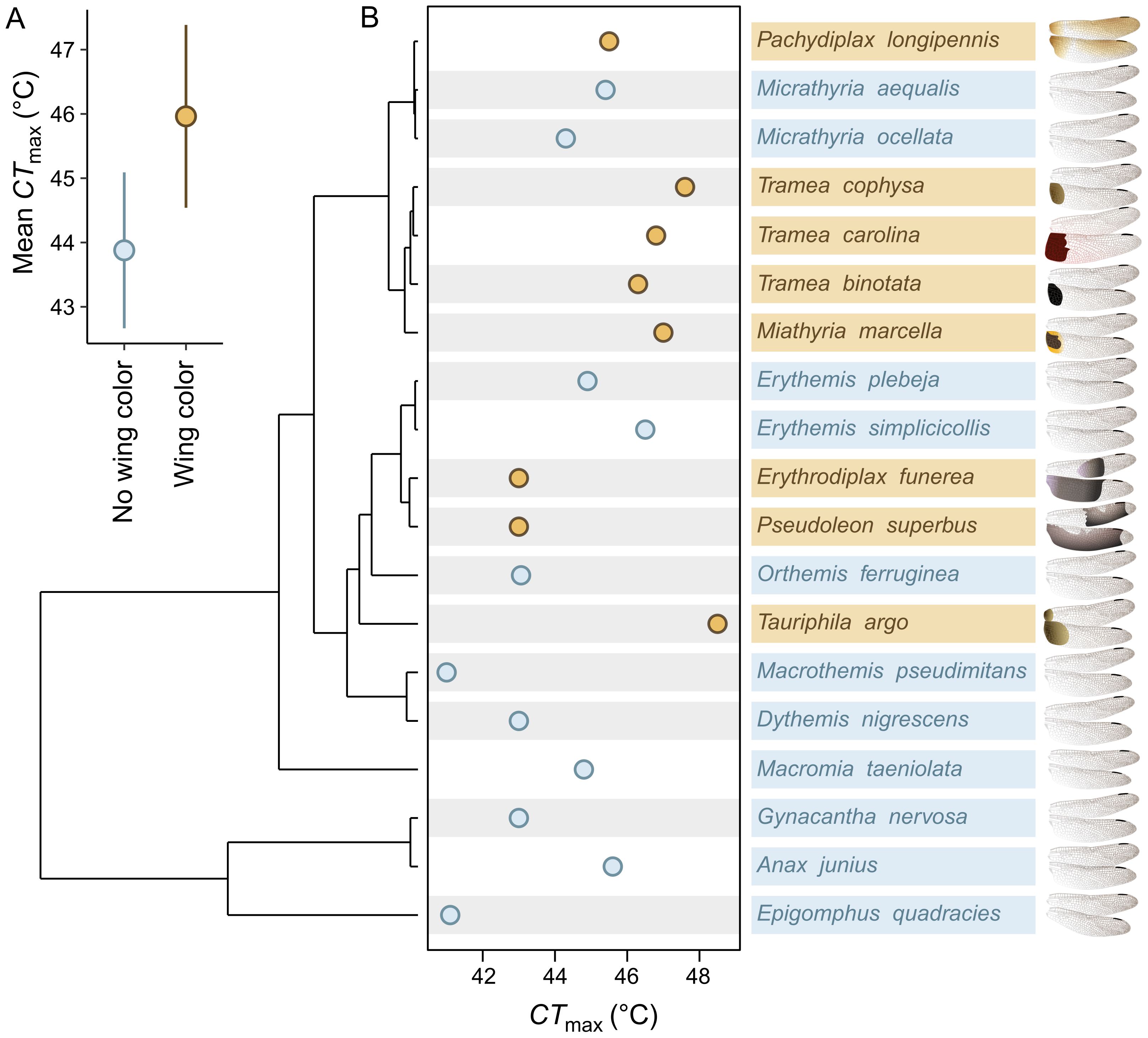
Figure 2. Co-adaptation between heat-absorbing sexual wing coloration and increased heat tolerance. (A) Model-estimated means and 95% confidence intervals for the average heat tolerance (CTmax) of species without wing coloration (blue) and with wing coloration (brown). (B) Phylogeny illustrating the evolutionary relationship between wing coloration and CTmax among 19 Nearctic dragonfly species. Brown points and text indicate species with wing coloration, and blue points and text indicate species without wing coloration. Grey bars indicate species that inhabit tropical climates. The wing illustrations show the color patterns produced by each species (illustrations by NTL).
Evaluating whether heat tolerance limits and/or compensates for sexual coloration
To assess whether the evolutionary history of CTmax and dark wing coloration is more consistent with a scenario of physiological limits or compensation, we performed a phylogenetic path analysis using Pagel’s λ branch-length transformation (‘phylopath’; von Hardenberg and Gonzalez-Voyer, 2013; van der Bijl, 2018). We used Pagel’s λ branch-length transformation because phylopath requires a specified model of evolution (von Hardenberg and Gonzalez-Voyer, 2013; van der Bijl, 2018) and a Pagel’s λ model received better support than a model under Brownian motion in our phylogenetic generalized least squares analysis. Moreover, the Pagel’s λ path model estimated low phylogenetic signal in CTmax (λ < 0.001), similar to the best-supported phylogenetic generalized least squares model, which included no phylogeny (Supplementary Table S1). We compared four path models representing hypothesized causal relationships between CTmax, wing coloration (yes/no), and climate (temperate/tropical). The path models differed along two axes. First, two models hypothesized that the evolution of wing coloration is constrained to species that have already evolved increased CTmax, as these species are able to handle the increased heat absorbed by dark sexual coloration (Figures 3B, D). The other two models hypothesized that species that evolve wing coloration later evolve increased CTmax to compensate for increased heat absorption (Figures 3A, C). Second, the models differed in whether an indirect effect of climate on the correlated evolution of the two traits was removed (Figures 3A, D) or included (Figures 3B, C). We evaluated support for each model by comparing CICc scores and obtained 95% confidence intervals from 500 bootstrapped estimates of each model (von Hardenberg and Gonzalez-Voyer, 2013). Because the two best models showed similar support, we also report model-averaged parameter estimates of the two best-supported models (von Hardenberg and Gonzalez-Voyer, 2013).
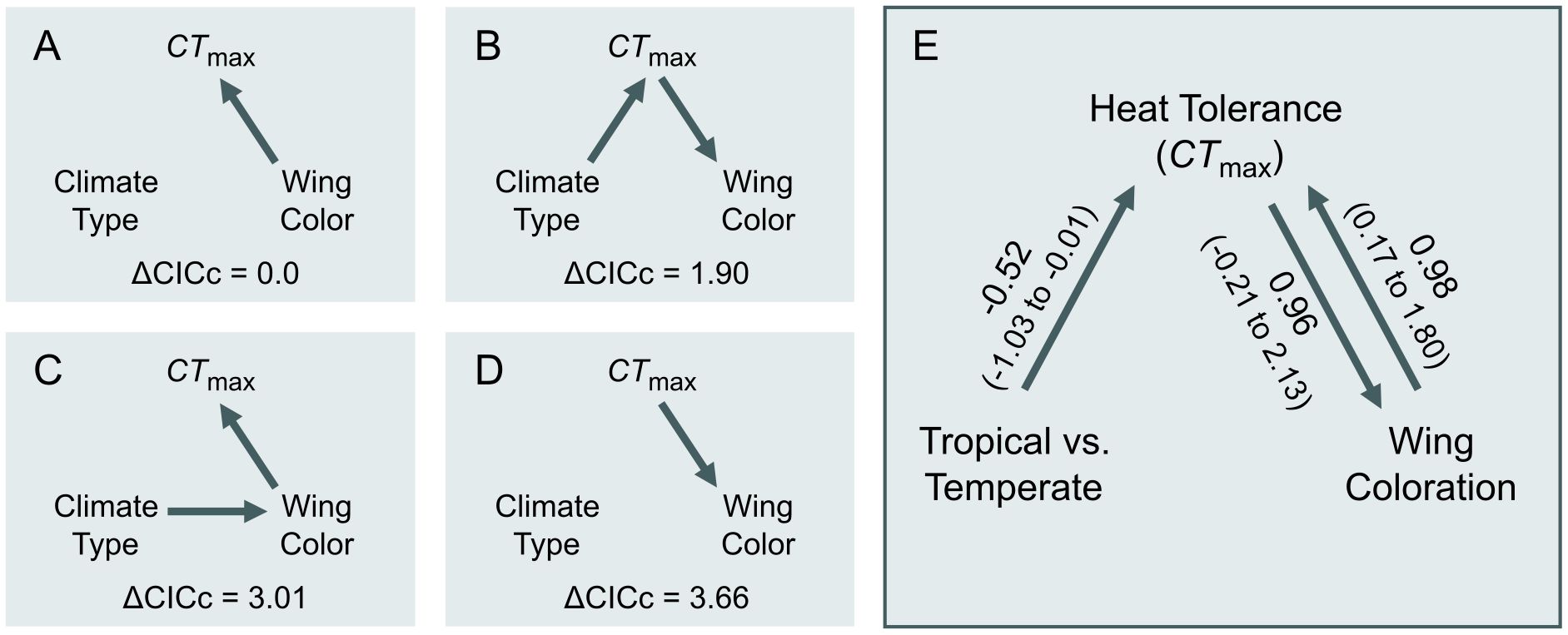
Figure 3. Path diagrams showing models of hypothesized evolutionary relationships among the presence of wing color (“Wing Color”), heat tolerance (“CTmax”), and temperate versus tropical climates (“Climate”). Models differ in whether heat tolerance compensates for (A, C) or limits the evolution of wing coloration (B, D), along with whether an indirect climate affect was removed (A, D) or included (B, D). (E) Model-averaged parameter estimates of the two best supported models (A, B). Confidence intervals that do not overlap zero indicate significant parameter estimates.
Results
Species that possess dark, heat-absorbing wing coloration have also evolved increased CTmax (β ± SE = 2.08 ± 0.88; = 5.40, P = 0.020; Figure 2; Supplementary Table S2). Moreover, this pattern of correlated evolution became stronger when only the fourteen tropical-climate species were included in the model (β ± SE = 2.68 ± 1.06; = 5.99, P = 0.014; Figure 2B; Supplementary Table S2).
The best-supported phylogenetic path model was one in which the evolution of wing coloration drives the compensatory evolution increased CTmax (Figure 3A; Supplementary Table S3). However, a path model where the evolution of wing coloration is limited to species that have already evolved increased CTmax received similar support (ΔCICc = 1.90; Figure 3B; Supplementary Table S3). Averaging these two best-supported models revealed that only the path coefficient for CTmax compensating for wing coloration was significantly greater than zero (β ± SE = 0.98 ± 0.41, 95% CI = 0.17 to 1.79; Figure 3E).
Discussion
Our findings demonstrate how viability-related thermal physiology evolves alongside sexual ornaments to balance the demands of survival and reproduction. We found that dragonfly species that produce dark, heat-absorbing wing coloration can also tolerate greater heat stress. In fact, the elevated CTmax of ornamented species is almost identical to the magnitude of heating caused by ornamentation (1-2°C, Svensson and Waller, 2013; Moore et al., 2019; Figure 2A). The relationship between ornamentation and heat tolerance also became even stronger when we only included species from tropical climates, which should strengthen selection favoring males with both increased heat tolerance and dark ornaments. Given that prior studies have experimentally demonstrated that darker wing coloration subjects male dragonflies to heat stress in warmer conditions (Moore et al., 2019), we can conclude that this correlated evolution is driven by selection jointly acting on male wing color and heat tolerance. Furthermore, phylogenetic path analysis indicated that the most plausible causal relationship underlying this correlated evolution is that higher heat tolerance primarily evolves to compensate for ornament induced heating, but that the evolution of heat tolerance may also (to a lesser degree) limit when wing ornaments can evolve in the first place.
Physiological compensation and limits on thermally costly sexual ornaments could be key factors generating patterns of biodiversity. Sexual ornaments are often the most variable traits among species and are essential to prezygotic reproductive isolation (Mayr, 1963; Servedio and Boughman, 2017). In fact, wing color evolution drives diversification and provides a crucial function for species recognition and coexistence among odonates (Paulson, 1974; Svensson et al., 2018; Hersch and Moore, 2023). Because thermally costly sexual ornaments like wing coloration may be unlikely to evolve at all in lineages with low heat tolerance, we may expect these lineages to show slower rates of diversification (Møller and Cuervo, 1998; Maia et al., 2013; Yuan et al., 2022). However, evolutionary changes in the production or structure of heat-absorbing sexual ornaments could also offset ornament heating, even without increased heat tolerance (Husak and Swallow, 2011; Moore et al., 2021; Leith et al., 2022). Our supplemental analyses revealed that ornamented species show the greatest increase in CTmax when wing color is concentrated close to the body (supplementary material), where heat flow from ornaments into the body could be exacerbated via conduction between the wings and the body, or via convection due the higher density and proximity of wing veins (Liao et al., 2019; Tsai et al., 2020). The evolution of wing color on distal portions of the wings could therefore maintain signal function while avoiding some heating costs. At the same time, when increased heat tolerance does evolve to compensate for ornament-induced heating, the correlated evolution of thermal physiology and sexual ornaments could drive ecological adaptation to novel temperature niches (Leith et al., 2022). For instance, even if thermally costly ornaments are only expressed by males, the compensatory evolution of increased heat tolerance could also benefit juvenile and female ecological performance by granting them access to habitats that contain hotter temperatures (Bonduriansky, 2011). Moreover, a variety of other compensatory adaptations that improve thermoregulation could also evolve to offset the heating costs of ornaments and thereby expand thermal niches. In dragonflies, for example, adaptations involving postural adjustments (May, 1976), enhanced hemolymph circulation (Heinrich and Casey, 1978), and specialized wing tissues (Guillermo-Ferreira et al., 2017; Guillermo-Ferreira and Gorb, 2021) can all dissipate excess heat while defending breeding territories. In general, the eco-physiological demands of mate competition may be a vital, but only recently appreciated source of selection promoting niche expansion and ecological divergence (Brownstein et al., 2024; Moore et al., 2024b).
Beyond affecting how organisms have adapted to climate in the past, co-adaptation between thermal and sexual traits should also influence how organisms respond to the changing climates of the future (Leith et al., 2022). In some cases, the evolution of sexual ornaments can aid population-level fitness in altered environments (Parrett et al., 2019; Moore et al., 2024a; Suárez-Tovar et al., 2024). For instance, sexual ornaments can increase population-level reproductive success by minimizing reproductive interference, injurious combat, and sexual conflict (Parker, 2006; M’Gonigle et al., 2012; Yun et al., 2018; Flintham et al., 2023). If sexual ornaments are limited to species that also possess high heat tolerances, then these species may experience two-fold advantages in warming climates because they incur fewer mating costs and they can already tolerate higher body temperatures than their non-ornamented counterparts. Alternatively, ornamented species may instead be more threatened by rapidly warming climates because the body temperatures they experience during mating activity likely extend closer to their upper thermal limits (Moore et al., 2019), causing them to exhibit narrower “thermal safety margins” for mating success (Sunday et al., 2014). Barring the reduction or loss of ornaments (Moore et al., 2021), reproduction may therefore become exceedingly challenging for ornamented species in warming climates unless they can effectively thermoregulate or evolve even higher heat tolerances (Sunday et al., 2014; Leith et al., 2024).
Overall, our findings emphasize that thermal physiology is another important suite of traits governing the costs and benefits of sexual ornaments. Given the vast breadth of animals that use dark color signals during sexual communication (Cuthill et al., 2017), and the pervasive heating effects of dark pigments (Clusella-Trullas et al., 2007; Rajpurohit et al., 2008), the patterns of co-adaptation between ornamental coloration and thermal physiology observed here may represent a key mechanism driving eco-physiological adaptation across climates.
Data availability statement
The original contributions presented in the study are included in the article/Supplementary material. Further inquiries can be directed to the corresponding author.
Ethics statement
The manuscript presents research on animals that do not require ethical approval for their study.
Author contributions
NL: Conceptualization, Data curation, Formal Analysis, Investigation, Methodology, Visualization, Writing – original draft, Writing – review & editing. MM: Conceptualization, Data curation, Formal analysis, Investigation, Methodology, Visualization, Writing – review & editing.
Funding
The author(s) declare financial support was received for the research, authorship, and/or publication of this article. Support was generously provided by Saint Louis University Presidential and Dissertation fellowships (NTL), a University of Pittsburgh Postdoctoral Fellowship in Ecology and Evolution (NTL), and the University of Colorado Denver (MPM).
Conflict of interest
The authors declare that the research was conducted in the absence of any commercial or financial relationships that could be construed as a potential conflict of interest.
Publisher’s note
All claims expressed in this article are solely those of the authors and do not necessarily represent those of their affiliated organizations, or those of the publisher, the editors and the reviewers. Any product that may be evaluated in this article, or claim that may be made by its manufacturer, is not guaranteed or endorsed by the publisher.
Supplementary material
The Supplementary Material for this article can be found online at: https://www.frontiersin.org/articles/10.3389/fetho.2024.1447637/full#supplementary-material
References
Bennett J. M., Calosi P., Clusella-Trullas S., Martínez B., Sunday J., Algar A. C., et al. (2018). GlobTherm, a global database on thermal tolerances for aquatic and terrestrial organisms. Sci. Data 5, 180022. doi: 10.1038/sdata.2018.22
Bonduriansky R. (2011). Sexual selection and conflict as engines of ecological diversification. Am. Nat. 178, 729–745. doi: 10.1086/662665
Brandt E. E., Kelley J. P., Elias D. O. (2018). Temperature alters multimodal signaling and mating success in an ectotherm. Behav. Ecol. sociobiol 72, 191. doi: 10.1007/s00265-018-2620-5
Brownstein C. D., Zapfe K. L., Lott S., Harrington R. C., Ghezelayagh A., Dornburg A., et al. (2024). Synergistic innovations enabled the radiation of anglerfishes in the deep open ocean. Curr. Biol. 34, 2541–2550.e4. doi: 10.1016/j.cub.2024.04.066
Castillo-Pérez E. U., Suárez-Tovar C. M., González-Tokman D., Schondube J. E., Córdoba-Aguilar A. (2022). Insect thermal limits in warm and perturbed habitats: Dragonflies and damselflies as study cases. J. Thermal Biol. 103, 103164. doi: 10.1016/j.jtherbio.2021.103164
Clusella-Trullas S., van Wyk J. H., Spotila J. R. (2007). Thermal melanism in ectotherms. J. Thermal Biol. 32, 235–245. doi: 10.1016/j.jtherbio.2007.01.013
Cornwallis C. K., Uller T. (2010). Towards an evolutionary ecology of sexual traits. Trends Ecol. Evol. 25, 145–152. doi: 10.1016/j.tree.2009.09.008
Cuthill I. C., Allen W. L., Arbuckle K., Caspers B., Chaplin G., Hauber M. E., et al. (2017). The biology of color. Science 357, eaan0221. doi: 10.1126/science.aan0221
Darnell M. Z., Munguia P. (2011). Thermoregulation as an alternate function of the sexually dimorphic fiddler crab claw. Am. Nat. 178, 419–428. doi: 10.1086/661239
Darnell M. Z., Yeghissian T. G., Lane Z. M. (2020). Balancing risk and reward: mating opportunity influences thermal refuge use in fiddler crabs. Anim. Behav. 169, 51–56. doi: 10.1016/j.anbehav.2020.08.013
Fick S. E., Hijmans R. J. (2017). WorldClim 2: new 1-km spatial resolution climate surfaces for global land areas. Int. J. Climatol 37, 4302–4315. doi: 10.1002/joc.5086
Flintham E. O., Savolainen V., Mullon C. (2023). Male harm offsets the demographic benefits of good genes. Proc. Natl. Acad. Sci. 120, e2211668120. doi: 10.1073/pnas.2211668120
García-Roa R., Garcia-Gonzalez F., Noble D. W. A., Carazo P. (2020). Temperature as a modulator of sexual selection. Biol. Rev. 95, 1607–1629. doi: 10.1111/brv.12632
Guillermo-Ferreira R., Appel E., Urban P., Bispo P. C., Gorb S. N. (2017). The unusual tracheal system within the wing membrane of a dragonfly. Biol. Lett. 13, 20160960. doi: 10.1098/rsbl.2016.0960
Guillermo-Ferreira R., Gorb S. N. (2021). Heat-distribution in the body and wings of the morpho dragonfly Zenithoptera lanei (Anisoptera: Libellulidae) and a possible mechanism of thermoregulation. Biol. J. Linn. Soc. 133, 179–186. doi: 10.1093/biolinnean/blaa216
Guillermo-Ferreira R., Therézio E. M., Gehlen M. H., Bispo P. C., Marletta A. (2014). The role of wing pigmentation, UV and fluorescence as signals in a neotropical damselfly. J. Insect Behav. 27, 67–80. doi: 10.1007/s10905-013-9406-4
Heinrich B., Casey T. M. (1978). Heat transfer in dragonflies: ‘Fliers’ and ‘Perchers.’. J. Exp. Biol. 74, 17–36. doi: 10.1242/jeb.74.1.17
Hersch K., Moore M. P. (2023). Ornamentation diversified faster than eco-morphology across Nearctic dragonflies. Biol. J. Linn. Soc. 139, 70–78. doi: 10.1093/biolinnean/blad008
Husak J. F., Ribak G., Wilkinson G. S., Swallow J. G. (2011). Compensation for exaggerated eye stalks in stalk-eyed flies (Diopsidae). Funct. Ecol. 25, 608–616. doi: 10.1111/fec.2011.25.issue-3
Husak J. F., Swallow J. G. (2011). Compensatory traits and the evolution of male ornaments. Behaviour 148, 1–29. doi: 10.1163/000579510X541265
Jocson D. M. I., Smeester M. E., Leith N. T., Macchiano A., Fowler-Finn K. D. (2019). Temperature coupling of mate attraction signals and female mate preferences in four populations of Enchenopa treehopper (Hemiptera: Membracidae). J. Evolution Biol. 32, 1046–1056. doi: 10.1111/jeb.13506
Kotiaho J. S. (2001). Costs of sexual traits: A mismatch between theoretical considerations and empirical evidence. Biol. Rev. Cambridge Philos. Soc. 76, 365–376. doi: 10.1017/S1464793101005711
Laakso L. K., Ilvonen J. J., Suhonen J. (2021). Phenotypic variation in male Calopteryx splendens damselflies: the role of wing pigmentation and body size in thermoregulation. Biol. J. Linn. Soc. 134, 685–696. doi: 10.1093/biolinnean/blab102
Leith N. T., Fowler-Finn K. D., Moore M. P. (2022). Evolutionary interactions between thermal ecology and sexual selection. Ecol. Lett. 25, 1919–1936. doi: 10.1111/ele.14072
Leith N. T., Jocson D. I., Fowler-Finn K. D. (2020). Temperature-related breakdowns in the coordination of mating in Enchenopa binotata treehoppers (Hemiptera: Membracidae). Ethology 126, 870–882. doi: 10.1111/eth.13033
Leith N. T., Macchiano A., Moore M. P., Fowler-Finn K. D. (2021). Temperature impacts all behavioral interactions during insect and arachnid reproduction. Curr. Opin. Insect Sci. 45, 106–114. doi: 10.1016/j.cois.2021.03.005
Leith N. T., Miller E. A., Fowler-Finn K. D. (2024). Thermoregulation enhances survival but not reproduction in a plant-feeding insect. Funct. Ecol. 38, 1344–1356. doi: 10.1111/1365-2435.14546
Liao H., Du T., Zhang Y., Shi L., Huai X., Zhou C., et al. (2019). Capacity for heat absorption by the wings of the butterfly Tirumala limniace (Cramer). PeerJ 7 (3), e6648. doi: 10.7287/peerj.preprints.27200v2
M’Gonigle L. K., Mazzucco R., Otto S. P., Dieckmann U. (2012). Sexual selection enables long-term coexistence despite ecological equivalence. Nature 484, 506–509. doi: 10.1038/nature10971
Maan M. E., Seehausen O. (2011). Ecology, sexual selection and speciation. Ecol. Lett. 14, 591–602. doi: 10.1111/j.1461-0248.2011.01606.x
Macchiano A., Miller E., Agali U., Ola-Ajose A., Fowler-Finn K. D. (2023). Developmental temperature alters the thermal sensitivity of courtship activity and signal–preference relationships, but not mating rates. Oecologia 202, 97–111. doi: 10.1007/s00442-023-05376-z
Macchiano A., Sasson D. A., Leith N. T., Fowler-Finn K. D. (2019). Patterns of thermal sensitivity and sex-specificity of courtship behavior differs between two sympatric species of enchenopa treehopper. Front. Ecol. Evol. 7. doi: 10.3389/fevo.2019.00361
Maia R., Rubenstein D. R., Shawkey M. D. (2013). Key ornamental innovations facilitate diversification in an avian radiation. Proc. Natl. Acad. Sci. United States America 110, 10687–10692. doi: 10.1073/pnas.1220784110
May M. L. (1976). Thermoregulation and adaptation to temperature in dragonflies (Odonata: anisoptera). Ecol. Monogr. 46, 1–32. doi: 10.2307/1942392
Mayr E. (1963). Animal species and evolution (Cambridge, MA: Harvard University Press). doi: 10.4159/harvard.9780674865327
Møller A. P., Cuervo J. J. (1998). Speciation and feather ornamentation in birds. Evolution 52, 859–869. doi: 10.2307/2411280
Moore M. P., Hersch K., Sricharoen C., Lee S., Reice C., Rice P., et al. (2021). Sex-specific ornament evolution is a consistent feature of climatic adaptation across space and time in dragonflies. Proc. Natl. Acad. Sci. United States America 118, 1–7. doi: 10.1073/pnas.2101458118
Moore M. P., Leith N. T., Fowler-Finn K. D., Medley K. A. (2024a). Human-modified habitats imperil ornamented dragonflies less than their non-ornamented counterparts at local, regional, and continental scales. Ecol. Lett. 27, e14455. doi: 10.1111/ele.14455
Moore M. P., Lis C., Gherghel I., Martin R. A. (2019). Temperature shapes the costs, benefits and geographic diversification of sexual coloration in a dragonfly. Ecol. Lett. 22, 437–446. doi: 10.1111/ele.13200
Moore M. P., Nalley S. E., Hamadah D. (2024b). An evolutionary innovation for mating facilitates ecological niche expansion and buffers species against climate change. Proc. Natl. Acad. Sci. 121, e2313371121. doi: 10.1073/pnas.2313371121
Oufiero C. E., Garland J. T. (2007). Evaluating performance costs of sexually selected traits. Funct. Ecol. 21, 676–689. doi: 10.1111/j.1365-2435.2007.01259.x
Parker G. A. (2006). Sexual conflict over mating and fertilization: an overview. Philos. Trans. R. Soc. B: Biol. Sci. 361, 235–259. doi: 10.1098/rstb.2005.1785
Parrett J. M., Mann D. J., Chung A. Y. C., Slade E. M., Knell R. J. (2019). Sexual selection predicts the persistence of populations within altered environments. Ecol. Lett. 22, 1629–1637. doi: 10.1111/ele.13358
Paulson D. R. (1974). Reproductive isolation in damselflies. Systematic Biol. 23, 40–49. doi: 10.1093/sysbio/23.1.40
Paulson D. (2009). Dragonflies and damselflies of the west (Princeton, NJ: Princeton University Press). doi: 10.1515/9781400832941
Paulson D. (2012). Dragonflies and damselflies of the east (Princeton, NJ: Princeton University Press). doi: 10.1515/9781400839667
Podos J. (2021). Costs, constraints and sexual trait elaboration. Anim. Behav. 184, 209–214. doi: 10.1016/j.anbehav.2021.05.021
Rajpurohit S., Parkash R., Ramniwas S. (2008). Body melanization and its adaptive role in thermoregulation and tolerance against desiccating conditions in drosophilids. Entomol Res. 38, 49–60. doi: 10.1111/j.1748-5967.2008.00129.x
Revell L. J. (2010). Phylogenetic signal and linear regression on species data. Methods Ecol. Evol. 1, 319–329. doi: 10.1111/j.2041-210X.2010.00044.x
Revell L. J. (2012). phytools: An R package for phylogenetic comparative biology (and other things). Methods Ecol. Evol. 3, 217–223. doi: 10.1111/j.2041-210X.2011.00169.x
Reznick D., Nunney L., Tessier A. (2000). Big houses, big cars, superfleas and the costs of reproduction. Trends Ecol. Evol. 15, 421–425. doi: 10.1016/S0169-5347(00)01941-8
Rocha-Ortega M., Rodríguez P., Bried J., Abbott J., Córdoba-Aguilar A. (2020). Why do bugs perish? Range size and local vulnerability traits as surrogates of Odonata extinction risk. Proc. R. Soc. B: Biol. Sci. 287, 20192645. doi: 10.1098/rspb.2019.2645
Rosenthal M. F., Elias D. O. (2019). Nonlinear changes in selection on a mating display across a continuous thermal gradient. Proc. R. Soc. B: Biol. Sci. 286, 20191450. doi: 10.1098/rspb.2019.1450
Saeki Y., Kruse K. C., Switzer P. V. (2005). Physiological costs of mate guarding in the Japanese beetle (Popillia japonica Newman). Ethology 111, 863–877. doi: 10.1111/j.1439-0310.2005.01106.x
Schreiner G. D., Duffy L. A., Brown J. M. (2020). Thermal response of two sexually dimorphic Calopteryx (Odonata) over an ambient temperature range. Ecol. Evol. 10, 12341–12347. doi: 10.1002/ece3.6864
Servedio M. R., Boughman J. W. (2017). The role of sexual selection in local adaptation and speciation. Annu. Rev. Ecol Evol Systemat 48, 85–109. doi: 10.1146/annurev-ecolsys-110316-022905
Shepherd B. L., Prange H. D., Moczek A. P. (2008). Some like it hot: Body and weapon size affect thermoregulation in horned beetles. J. Insect Physiol. 54, 604–611. doi: 10.1016/j.jinsphys.2007.12.007
Suárez-Tovar C. M., Guillermo-Ferreira R., Cooper I. A., Cezário R. R., Córdoba-Aguilar A. (2022). Dragon colors: the nature and function of Odonata (dragonfly and damselfly) coloration. J. Zool 317, 1–9. doi: 10.1111/jzo.12963
Suárez-Tovar C. M., Rocha-Ortega M., Juen L., Córdoba-Aguilar A. (2024). From the forest to the city: the persistence of dragonflies and damselflies in the urban jungle. Biodivers Conserv. 33, 91–113. doi: 10.1007/s10531-023-02733-5
Sunday J. M., Bates A. E., Kearney M. R., Colwell R. K., Dulvy N. K. (2014). Thermal-safety margins and the necessity of thermoregulatory behavior across latitude and elevation. Proc. Natl. Acad. Sci. 111, 5610–5615. doi: 10.1073/pnas.1316145111
Svensson E. I., Gómez-Llano M. A., Torres A. R., Bensch H. M. (2018). Frequency dependence and ecological drift shape coexistence of species with similar niches. Am. Nat. 191, 691–703. doi: 10.1086/697201
Svensson E., Gomez-Llano M., Waller J. (2020). Selection on phenotypic plasticity favors thermal canalization. Proc. Natl. Acad. Sci. 117, 29767–29774. doi: 10.1073/pnas.2012454117
Svensson E. I., Waller J. T. (2013). Ecology and sexual selection: Evolution of wing pigmentation in calopterygid damselflies in relation to latitude, sexual dimorphism, and speciation. Am. Nat. 182, E174–E195. doi: 10.1086/673206
Tsai C. C., Childers R. A., Nan Shi N., Ren C., Pelaez J. N., Bernard G. D., et al. (2020). Physical and behavioral adaptations to prevent overheating of the living wings of butterflies. Nat. Commun. 11, 1–14. doi: 10.1038/s41467-020-14408-8
van der Bijl W. (2018). phylopath: Easy phylogenetic path analysis in R. PeerJ 6, e4718. doi: 10.7717/peerj.4718
Vilela B., Villalobos F. (2015). letsR: a new R package for data handling and analysis in macroecology. Methods Ecol. Evol. 6, 1229–1234. doi: 10.1111/2041-210X.12401
von Hardenberg A., Gonzalez-Voyer A. (2013). Disentangling evolutionary cause-effect relationships with phylogenetic confirmatory path analysis. Evolution 67, 378–387. doi: 10.1111/evo.2013.67.issue-2
West P. M., Packer C. (2002). Sexual selection, temperature, and the lion’s mane. Science 297, 1339–1343. doi: 10.1126/science.1073257
Wiens J. J. (2001). Widespread loss of sexually selected traits: how the peacock lost its spots. Trends Ecol. Evol. 16, 517–523. doi: 10.1016/S0169-5347(01)02217-0
Wiens J. J., Tuschhoff E. (2020). Songs versus colours versus horns: what explains the diversity of sexually selected traits? Biol. Rev. 95, 847–864. doi: 10.1111/brv.12593
Yuan M. L., Westeen E. P., Wogan G. O. U., Wang I. J. (2022). Female dewlap ornaments are evolutionarily labile and associated with increased diversification rates in Anolis lizards. Proc. R. Soc. B: Biol. Sci. 289, 20221871. doi: 10.1098/rspb.2022.1871
Yun L., Chen P. J., Kwok K. E., Angell C. S., Rundle H. D., Agrawal A. F. (2018). Competition for mates and the improvement of nonsexual fitness. Proc. Natl. Acad. Sci. United States America 115, 6762–6767. doi: 10.1073/pnas.1805435115
Zheng D., Nel A., Jarzembowski E. A., Chang S.-C., Zhang H., Xia F., et al. (2017). Extreme adaptations for probable visual courtship behaviour in a Cretaceous dancing damselfly. Sci. Rep. 7, 44932. doi: 10.1038/srep44932
Keywords: sexual selection, thermal melanism, correlational selection, compensatory trait, temperature, ornament coloration, climate change
Citation: Leith NT and Moore MP (2024) Heat-absorbing sexual coloration co-adapts with increased heat tolerance in dragonflies. Front. Ethol. 3:1447637. doi: 10.3389/fetho.2024.1447637
Received: 11 June 2024; Accepted: 16 August 2024;
Published: 10 October 2024.
Edited by:
Adolfo Cordero-Rivera, University of Vigo, SpainReviewed by:
Rhainer Guillermo Ferreira, Universidade Federal do Triângulo Mineiro, BrazilAnuradha Bhat, Indian Institute of Science Education and Research Kolkata, India
Copyright © 2024 Leith and Moore. This is an open-access article distributed under the terms of the Creative Commons Attribution License (CC BY). The use, distribution or reproduction in other forums is permitted, provided the original author(s) and the copyright owner(s) are credited and that the original publication in this journal is cited, in accordance with accepted academic practice. No use, distribution or reproduction is permitted which does not comply with these terms.
*Correspondence: Noah T. Leith, bGVpdGguZWNvLmV2b0BnbWFpbC5jb20=; bnRsMTdAcGl0dC5lZHU=