- 1Department of Biology, College of Charleston, Charleston, SC, United States
- 2Department of Integrative Biology, University of Colorado Denver, Denver, CO, United States
Several species of stalk-eyed flies exhibit exaggerated sexual dimorphism where females favor males with longer eyespans. Longer eyespan increases a fly’s moment of inertia, and may, therefore, impact flight behavior and fitness, specifically maneuverability and predator evasion. However, these putative costs may be ameliorated by co-selection for compensatory traits, as flies with longer eyespans tend to have larger thoraces and wings, which allows them to perform turns similar to flies with shorter eyespans. Furthermore, the capacity to compensate for a potentially costly ornament may not be fixed across the life-history of the adult stage, as stalk-eyed flies achieve sexual maturity at 3-4 weeks of age, accompanied by significant growth of reproductive tissues and organs. Thus, growth of the abdomen and body mass over time may impose constraints on flight performance that may affect whether an adult reaches the age of reproductive viability. The purpose of this study was to investigate the flight performance of stalk-eyed flies and its relationship to body morphology and development. The flight performance of 1-to-30 day old Teleopsis dalmanni (n=124) and Diasemopsis meigenii (n=83) were assessed by presenting normoxic, variable-density mixtures of heliox (O2, N2 and He) in 10% increments ranging from air to pure heliox; the least-dense gas allowing flight represented maximal performance. Flight kinematics were analyzed using high-speed (5930fps) videography. Immediately following flight assessment, flies were euthanized, photographed, dissected and weighed. In both species, total body mass, thorax and abdominal mass increased across age. Wing kinematics and maximal flight capacity were associated with thorax mass, and increased with age as flies became heavier. Although flies with longer eyespans were indeed heavier, they had larger wings and thoraces; however, maximal flight capacity and kinematics were generally independent of eyespan. Thus, bearing long eye-stalks did not impair flight performance, nor did the increase in mass attributable to reproductive maturation. Instead, variation in flight performance appears associated with the development of the flight motor, and improved ratio of thorax-to-total mass, across age.
1 Introduction
Communication between rivals or potential mates is often accomplished with extremely exaggerated ornaments and armaments in elaborate displays, and the evolution of such signals has received significant attention (Andersson, 1994; Berglund et al., 1996). Nevertheless, despite decades of research aiming to explain how such traits evolve, one of the most elusive questions in animal behavior is how the use of ornaments during mate choice and rival assessment remains a reliable signal over evolutionary time. The predominant hypothesis posits that ornaments are costly to those that bear them (Zahavi, 1975), though this itself remains controversial (Számadó, 2010). Studies designed to test the prediction that ornaments should be costly to their bearer have often failed to measure significant performance costs (Kotiaho, 2001), particularly those using Arnold (1983) Morphology → Performance → Fitness framework. Indeed, despite the intuitive notion that exaggerated ornaments should impact locomotor performance and behaviors by placing an evolutionary constraint on the evolution of ornaments, extreme exaggeration of ornaments has often evolved with little or no measurable decrease in performance (Lailvaux and Irschick, 2006; Husak and Swallow, 2011).
Husak and Swallow (2011) proposed modifications to Arnold (1983) framework to account for female choice of ornaments, or use of ornaments to assess rivals, where direct selection on morphology interacts with the detrimental effects of the ornament to indirectly drive the evolution of performance (Figure 1). This modification recognizes that natural selection may limit elaboration and exaggeration of ornaments (Andersson, 1994; Kotiaho, 2001)and considers there may also be selection on the integrated whole organism, including elements that reduce the negative effects of sexually selected traits such as ornaments. Specifically, there may be correlated selection for compensatory traits (Iwasa et al., 1991; Møller, 1996; Oufiero and Garland, 2007; Irschick et al., 2008; Swallow et al., 2009), which may occur at multiple stages of development and life history (Stearns, 1992; Charnov, 1993; Pitnick et al., 1995; Lailvaux and Husak, 2014).
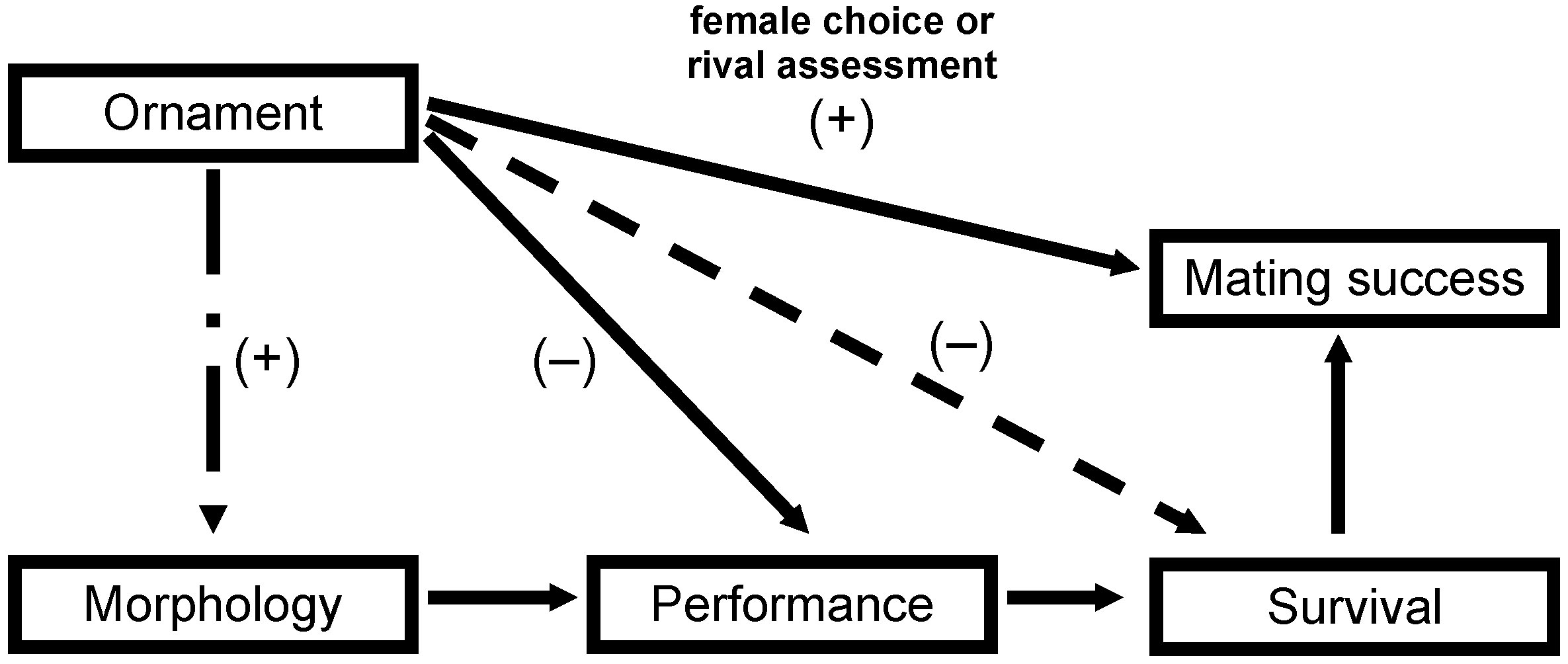
Figure 1 Sexual selection on an ornament that impairs performance may result in selection that enhances the morphological traits underlying performance in order to compensate. Positive (+) and negative (−) symbols indicate an enhancement or impairment of selection pressure between the respective traits. Figure adapted from Husak and Swallow (2011).
Stalk-eyed flies (family Diopsidae) are a tractable model-system to explore the interaction between a detrimental, sexually-selected ornament and morphological traits that offset performance costs. These dipterans possess peculiar head morphology (Figure 2A), with eyes located laterally on long stalks (Burkhardt and de la Motte, 1983). The Diopsidae family consists of monomorphic species, where males and females have similar eyespans, and sexually dimorphic species, where males and females differ significantly in eye stalk length (eyespan), often exhibiting non-overlapping ranges between sexes (Wilkinson and Dodson, 1997; Baker and Wilkinson, 2001). In dimorphic species, the enlarged eyespan in males is influenced by both male-male competition (Burkhardt and de la Motte, 1985; Panhuis and Wilkinson, 1999; Small et al., 2009) and female choice (Burkhardt and de la Motte, 1987; Burkhardt and de la Motte, 1988; Wilkinson et al., 1998). However, in monomorphic species, the eyespan of males does not seem to be sexually selected and may be closer to the size that would result from natural selection (Worthington and Swallow, 2010).
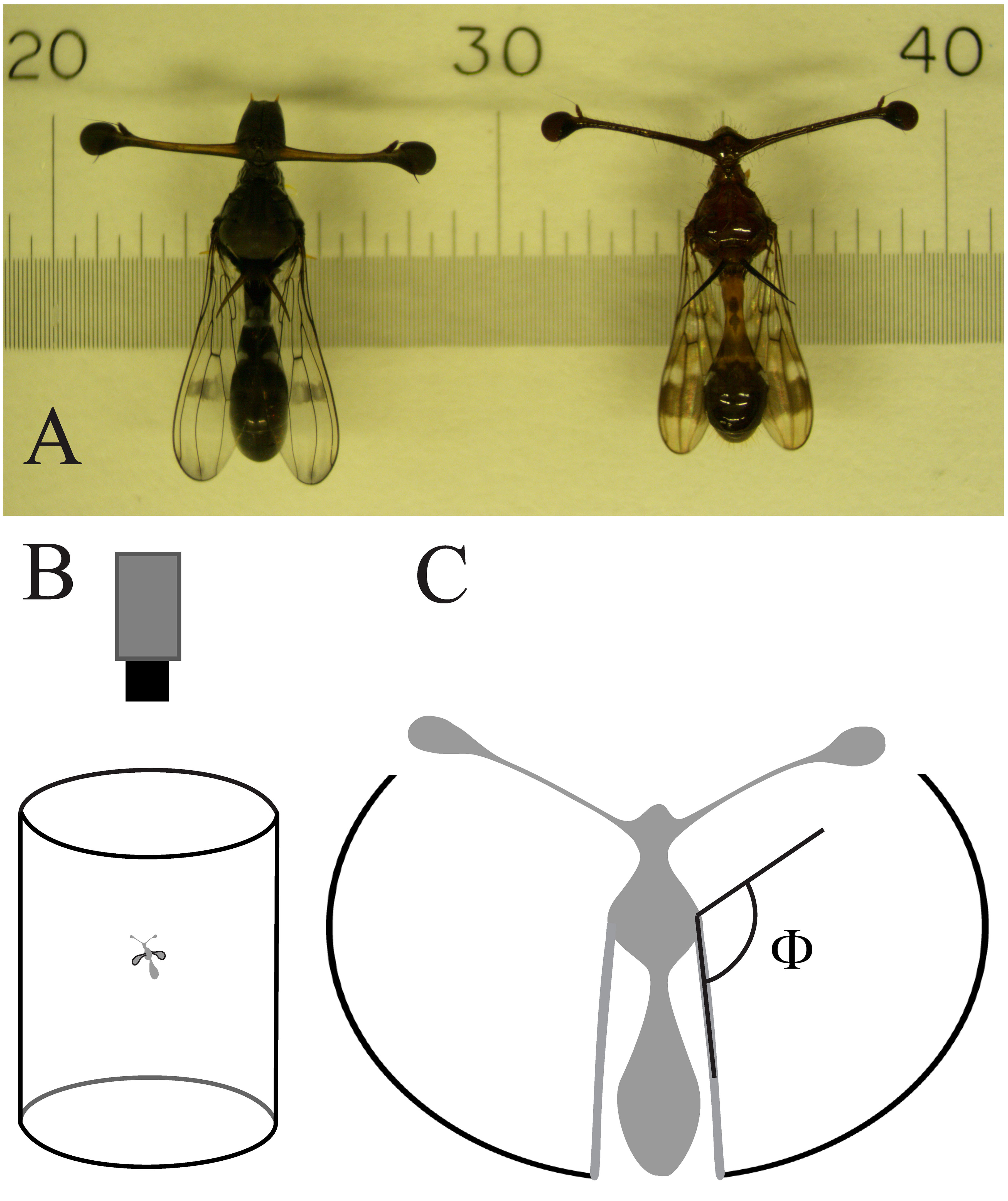
Figure 2 Description of methods. (A) The flight performance of male Teleopsis dalmanni (right; n=123) and Diasemopsis meigenii (left; n=84) were assessed using (B) hypodense, normoxic mixtures of oxygen, nitrogen and helium in an 8-liter cylindrical flight chamber. A high-speed video camera (5930 fps) oriented above the chamber recorded hovering flight. (C) The hovering sequences were digitized, and wing stroke amplitude was calculated from the horizontal angular displacement of the dorsal and ventral wing stroke reversals.
Although larger eyespans increase mating success (Burkhardt and de la Motte, 1985; Burkhardt and de la Motte, 1987; Burkhardt and de la Motte, 1988; Wilkinson et al., 1998; Panhuis and Wilkinson, 1999; Small et al., 2009), the exaggerated eye stalks of males should impose costs on flight behaviors and maneuvering through increased moment of inertia. However, assessing these costs has proven elusive as male stalk-eyed flies perform turns during flight as well as females, despite the significant difference in moment of inertia. The putative costs of bearing these large ornaments appear to be ameliorated by compensatory investment into the flight apparatus; specifically, flies with larger eyespan possess larger thoraces and wings (Swallow et al., 2000; Ribak and Swallow, 2007).
Stalk-eyed flies do not reach sexual maturity until about 3-4 weeks of age, post-eclosion. Many studies investigating competition or mating behaviors of stalk-eyed flies have focused on sexually-mature adults, as these behaviors would likely occur at lesser frequency in sexually-immature individuals (Baker et al., 2001; Pomiankowski et al., 2005; Rogers et al., 2005; Egge et al., 2011; Bellamy et al., 2013; Bath et al., 2015; Bubak et al., 2016). Likewise, studies on stalk-eyed fly flight performance have also focused on sexually-mature adults (Swallow et al., 2000; Ribak and Swallow, 2007; Husak et al., 2011), as Ribak and Swallow (2007) cite that body mass is known to increase over this period of maturation. This anecdotal increase in body mass may be due, in part, to the growth of the testes and accessory glands, which increase in length by 200-300% over this period (Baker et al., 2003). Since the abdomen does not contribute to the production of aerodynamic forces, increased body mass via abdominal mass may pose a further challenge to an insect’s flight ability across age; investigating the flight performance of sexually-mature adults may not accurately reflect the interaction of detrimental ornaments with compensatory morphology across an adult’s life-history.
The purpose of this study is to characterize the allometry of morphology and flight performance of male stalk-eyed flies, Teleopsis dalmanni and Diasemopsis meigenii, across age. Both are dimorphic species where female sexual preference has driven the exaggeration of male eyespan, and where thorax and wing morphology appears to have co-evolved with eyespan, putatively as a compensatory mechanism (Ribak and Swallow, 2007; Husak et al., 2011). Thorax width and wing-size are fixed post-eclosion, and age-related changes in body- and segment-mass that result from sexual maturation may impact flight performance via a negative flight-fecundity tradeoff (Tigeros and Davidowitz, 2019); thus, compensation for the locomotor costs of bearing longer eye-stalks may be limited by life-history. We hypothesize that flight performance will be associated with investments in the flight apparatus, specifically wing size, thorax width and thorax mass (as a proxy for thorax muscle mass), and will vary as the fraction of thorax mass changes with body mass across age.
2 Materials and methods
Populations of stalk-eyed fly species Teleopsis dalmanni and Diasemopsis meigenii were maintained in an environmentally-controlled room at 24C, 80% relative humidity and a 12h/12h light-dark cycle. Adult flies were collected within one day post-eclosion and housed in 3L chambers, separated by age and species. Flies were fed a diet of pureed corn and instant Drosophila food ad libitum, and food cups were replaced every 4 days. Male flies that possessed intact wings and were free of morphological defect (e.g. malformed abdomens, missing legs, etc.) were selected for assessment of flight performance and allometry.
Flight performance was assessed individually (i.e. one fly in the chamber at a time) using variable density, normoxic gas mixtures which consisted of oxygen and nitrogen and/or helium (Dudley, 1995; Vance et al., 2009), and ranged from normodense air (21% O2, 79% N2; 1.21kg m–3) to hypodense heliox (21% O2, 79% He; 0.41kg m–3) in 0.08kg m–3 increments (Table 1). As gas density decreases, the aerodynamic power required to maintain hovering flight increases. Each trial began with air, and successive hypodense gas mixtures were then administered using an interval-halving method, such that successive gas mixtures were not of greater density to a prior atmosphere where successful flight was observed, and not of lesser density to a prior atmosphere where no flight was observed. Maximal flight capacity was determined to be the least-dense gas (LDG, in percent-heliox) where hovering flight was observed. This interval-halving method exposed flies to only 3 or 4 of the 10 hypodense gas mixtures to minimize the influence of fatigue on flight performance.
The gasses were mixed using calibrated, solenoid-actuated valves (Tylan: FC-2910), which were plumbed into an 8L cylindrical acrylic chamber with an inlet port on the side for gas perfusion (Figure 2B). Gas mixtures and flow rates were metered by an electronic flow controller (Sable Systems MFC-4; Las Vegas, NV, USA). When changing the gas mixture, the flight chamber was flushed with the new gas mixture at a flow rate of 20L min-1 for 2 minutes to ensure complete washout. When assessing flight performance and filming hovering flight, total gas flow rate was maintained at 2L min-1. In any given gas mixture, flies were flown until sustained hovering flight was observed and recorded, or hovering flight was attempted but failed. Failure was typically distinguished by flies attempting to initiate flight from the floor of the chamber and being unable to reach at least the middle of the height of the chamber, thus excluding jumping and aerodynamic ‘ground effect’ from contributing to a potentially successful flight. Flies that landed on the floor or sides of the chamber were persuaded to fly by using a small magnetic stir-bar to chase and agitate them. Flies were excluded from the study if 1) they were unable to fly in air, or 2) they initially exhibited flight in air, but could not replicate flight in air after failing to fly in any of the hypodense gas mixtures. In this latter case, it was assumed that some other uncontrolled factor (e.g. motivation, injury, etc.) may have influenced the trial.
Hovering flight kinematics were determined from the wing motions in the horizontal plane, recorded by a high-speed (5930 fps) digital video camera (RedLake IDT MotionPro N3-S4). The camera was oriented above the flight chamber and focused such that the depth of field was in focus in the middle 1/3 of the chamber. Ascending, descending, maneuvers, and lateral flights (e.g. initiated at the side of the chamber, and flown directly across the chamber) were ignored. The digital video sequences were analyzed using DLTdv8 (Hedrick, 2008). Flight kinematics were calculated according to Vance and Roberts (2014): wingbeat frequency and stroke amplitude (the horizontal angular displacement of the wingstroke from the dorsal to ventral stroke reversals; Figure 2C) were determined from the average of 10 successive wingstrokes. Average wing velocity was calculated from these kinematics and individual wing-length data.
Following assessment of flight performance, flies were euthanized, weighed, dissected and photographed. Total body mass was obtained from the intact fly. The legs and wings were then removed, and the fly and wings were placed in the prone position on a slide-micrometer and photographed. The head, thorax, and abdomen were then separated and weighed. Photographs were analyzed using custom software (MatLab, The Mathworks) to determine eyespan (the distance from the lateral edges of the right and left eyes), thorax width, and the following wing morphometrics: wing-length, mean chord length, wing area, aspect ratio, and non-dimensional 2nd and 3rd moments of wing area (Vance and Roberts, 2014).
Multivariate analysis of covariance (MANCOVA, α=0.05) was used to evaluate the effect of species, eyespan and age (independent variables) on morphology and flight performance (dependent variables). Analysis of covariance (ANCOVA) was used to determine the effect of species and thorax mass, and the effect of species and wingtip velocity, on flight performance. Our post hoc analyses consisted of evaluating specific relationships using linear regression.
3 Results
3.1 Body and wing morphology
There was a significant effect of species, eyespan and age on body and wing morphology, and on body and segment masses (MANCOVA, Hotelling trace: P<0.001; Table 2). Although T. dalmanni had slightly longer (3%) eyespan, D. meigenii were generally larger and more massive: D. meigenii had 14% wider thoraces, 10% longer wings, 25% greater mean wing chord length, and 37% greater wing area than T. dalmanni. However, for distribution of wing area, T. dalmanni had 13% greater aspect ratio than D. meigenii; Though statistically significant, the differences in the non-dimensional 2nd and 3rd moments in wing area were small (<3%). D. meigenii had 44% heavier body mass than T. dalmanni, which consisted of 73% heavier heads, 54% heavier thoraces, 52% heavier abdomens, and 8% greater thorax mass-to-body mass ratio.
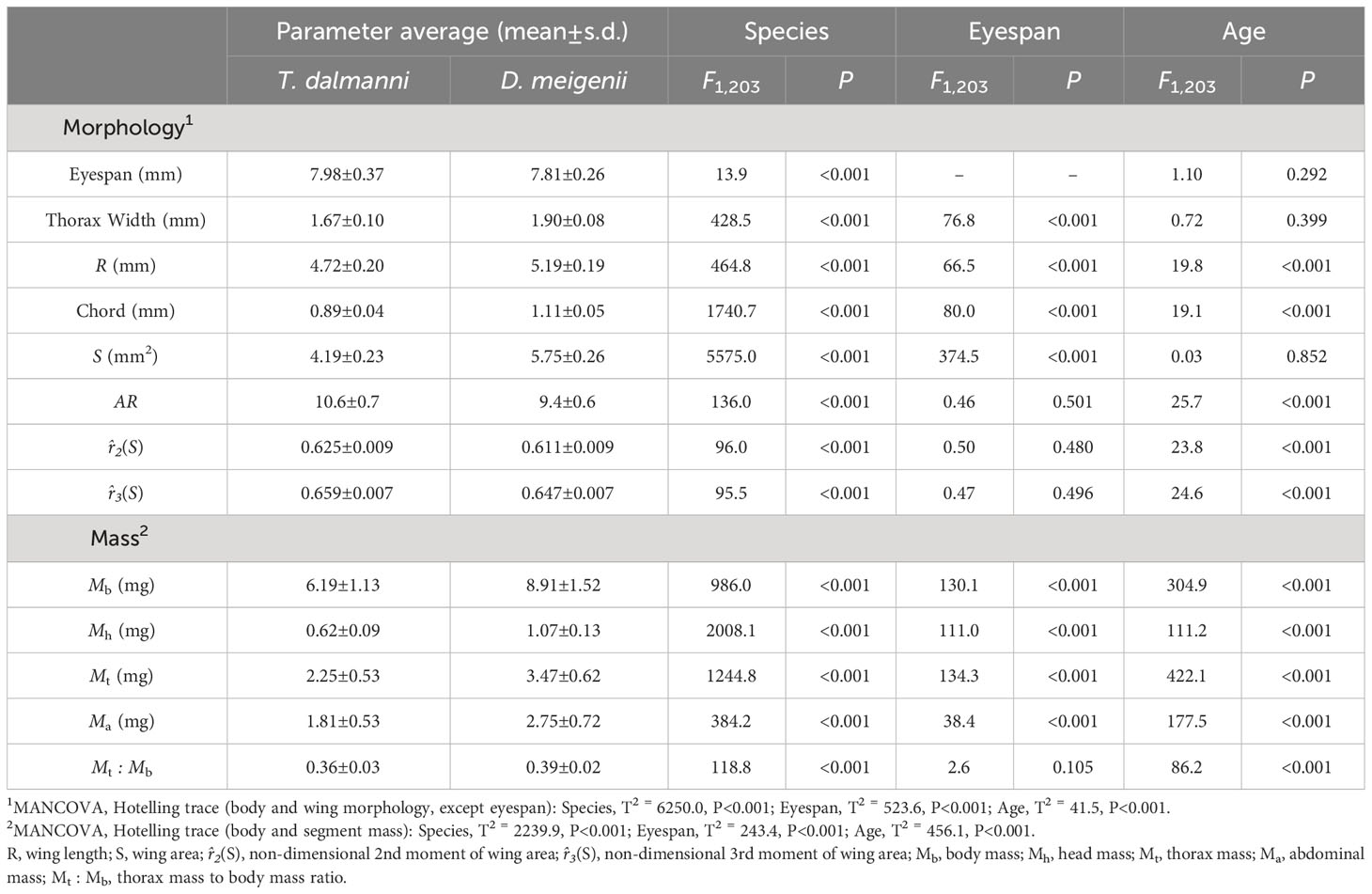
Table 2 Multivariate analysis of covariance (MANCOVA) for the effects of species, eyespan and age on body and wing morphology, and on body and segment mass.
Both species exhibited positive allometric trends in body morphology with respect to eyespan. Flies with longer eyespan had heavier heads (linear regression: T. dalmanni, R2 = 0.28, P<0.001; D. meigenii, R2 = 0.27, P<0.001), and tended to have heavier thoraces (T. dalmanni, R2 = 0.12, P<0.001; D. meigenii, R2 = 0.20, P<0.001) and abdomens (T. dalmanni, R2 = 0.07, P<0.001; D. meigenii, R2 = 0.07, P<0.012). Thus, flies with longer eyespans were generally heavier in overall body mass (linear regression: T. dalmanni, P<0.001, R2 = 0.28; D. meigenii, P<0.001, R2 = 0.19). Flies with longer eyespans had wider thoraces, longer wings and greater wing area (Figure S1). However, the distribution of wing area (e.g. aspect ratio, and non-dimensional 2nd and 3rd moments of wing area) were not associated with variation in eyespan (Table 3).
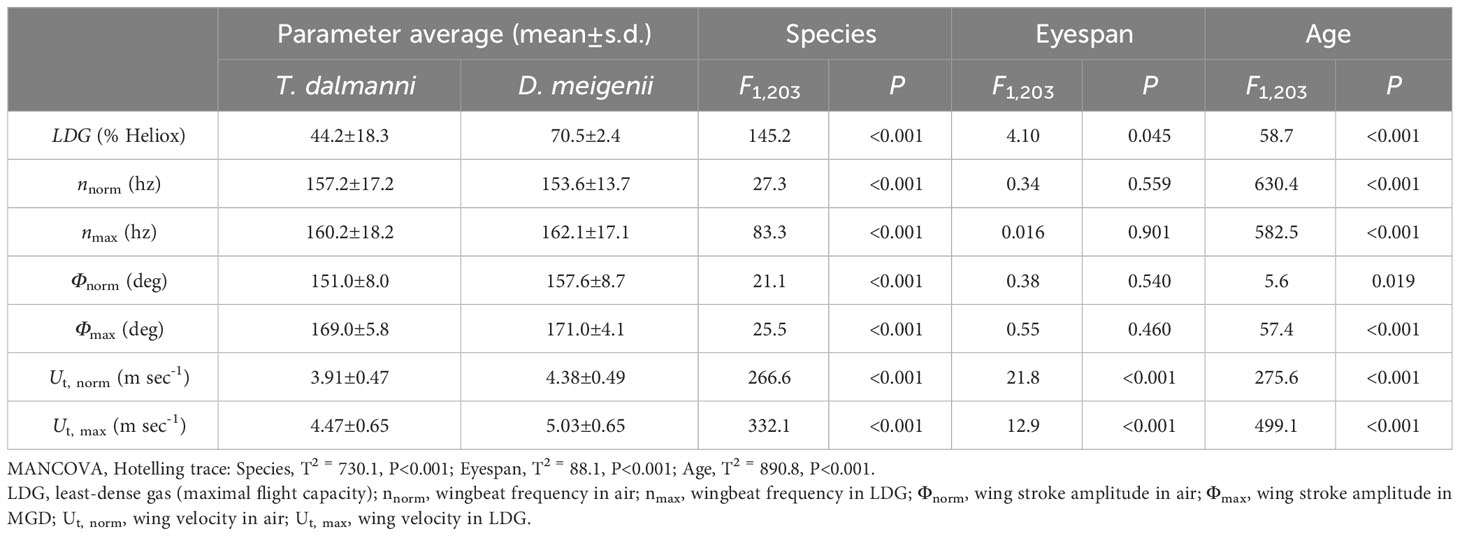
Table 3 Multivariate analysis of covariance (MANCOVA) for the effects of species, eyespan and age on flight performance.
Both species exhibited positive allometry in body morphology with respect to age (Table 3). Body mass, and head, thorax and abdomen mass, increased with age (Figures 3A-D). The ratio of thorax mass-to-body mass increased across age in T. dalmanii and was generally maintained across age in D. meigenii (Figure 3E). Although there was a significant effect for age on wing length, mean chord length, and wing area distribution (Table 2), post hoc analyses revealed weak or non-significant linear regressions for these variables. Likewise, there was no effect of age on wing area (Table 2).
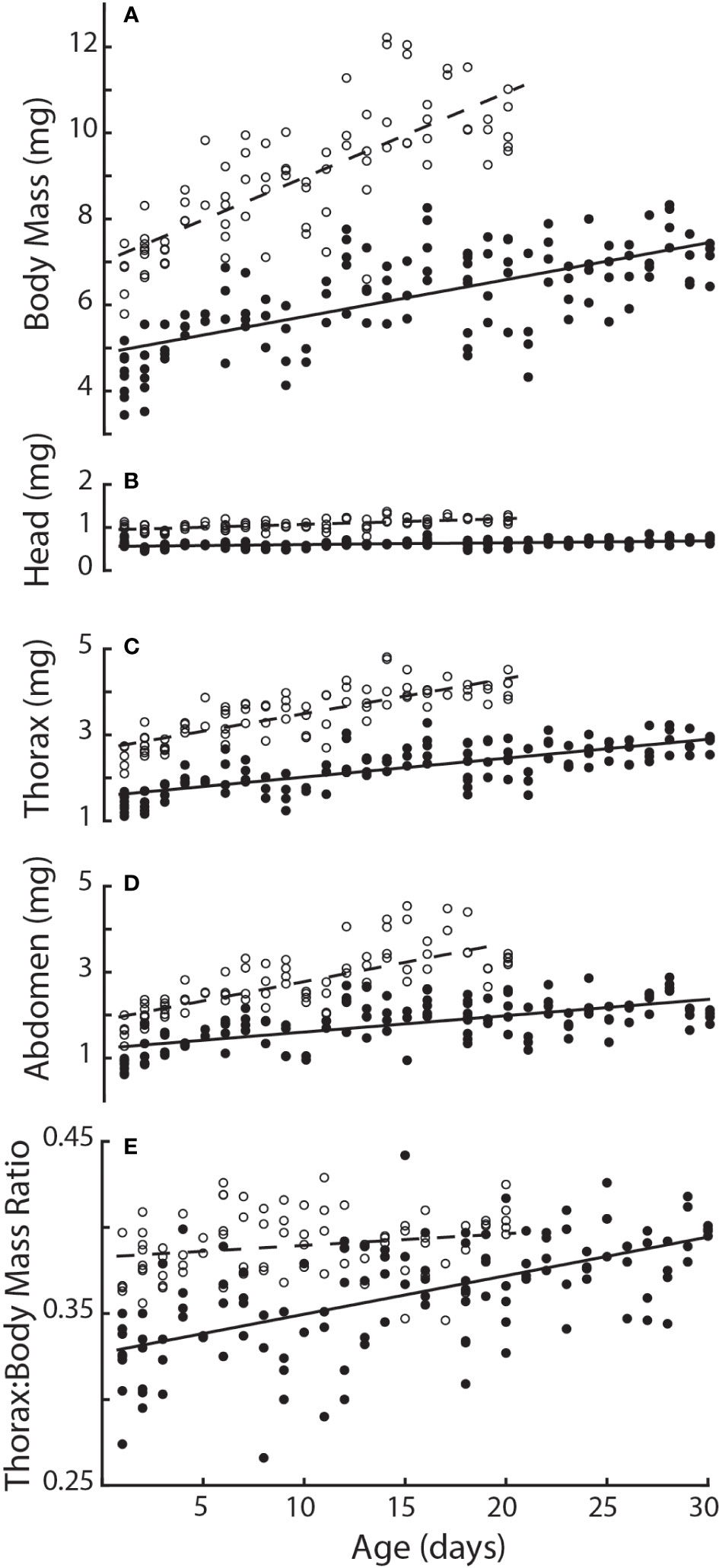
Figure 3 Body and segment masses for T. dalmanni (filled circles) and D. meigenii (open circles) increased with age (linear regression, p<0.001 for all comparisons). (A) Total body mass: T. dalmanni (solid line), Mb = 4.88 + 0.09age, R2 = 0.46; D. meigenii (dashed line), Mb = 7.01 + 0.20age, R2 = 0.59. (B) Head mass T. dalmanni (solid line), Mh = 0.56+.004age, R2 = 0.2; D. meigenii (dashed line), Mh = 0.94 + 0.013age, R2 = 0.40. (C) Thorax mass T. dalmanni (solid line), Mt = 1.58 + 0.04age, R2 = 0.56; D. meigenii (dashed line), Mt = 2.69 + 0.08age, R2 = 0.61. (D) Abdominal mass: T. dalmanni (solid line), Ma = 1.23 + 0.04age, R2 = 0.41; D. meigenii (dashed line), Ma = 1.89 + 0.09age, R2 = 0.55. (E) Thorax mass to body mass ratio: T. dalmanni (solid line), Mt : Mb = 0.33 + 0.002age, R2 = 0.37; D. meigenii (dashed line), Mt : Mb = 0.38 + 0.0007age, R2 = 0.05.
3.2 Flight performance and kinematics
There was a significant effect of species, eyespan and age on flight performance and kinematics (MANCOVA, Hotelling trace: P<0.001; Table 3). Wing kinematics during hovering in air were generally similar: T. dalmanni exhibited 2% greater wingbeat frequency and D. meigenii had 4% larger stroke amplitude. D. meigenii had 12% greater wing velocity than T. dalmanni, largely attributable to their longer wings. At maximal capacity, D. meigenii were able to fly in heliox mixtures that were 25% less-dense than T. dalmanni. This was facilitated by a relatively small increase in wingbeat frequency (<2% for T. dalmanni, 5% for D. meigenii), and a modest 12% and 8% increase in stroke amplitude for T. dalmanni and D. meigenii, respectively. This increase in kinematic output resulted in a 14-15% increase in wing velocity for both species in order to fly in the least dense gas (LDG).
There was no effect of eyespan on wingbeat frequency or stroke amplitude during normal or maximal hovering (Table 3). There was a significant, yet weak relationship between eyespan and wing velocity during normal and maximal hovering (Table 3), however linear regression revealed no significant trends between or across species (R2<0.02 for all comparisons). Likewise, there was a weak relationship between eyespan and maximal flight capacity, however linear regression revealed no significant trends between or across species (R2 ≤ 0.01 for all comparisons).
Maximal flight capacity increased with age in both species (linear regression: T. dalmanni, R2 = 0.20, P<0.001; D. meigenii, R2 = 0.32, P<0.001), and was positively associated with variation in body mass (linear regression: all flies, R2 = 0.42, P<0.001), thorax mass (linear regression: all flies, R2 = 0.49, P<0.001) and thorax mass-to-body mass ratio (T. dalmanni, R2 = 0.35, P<0.001; D. meigenii, R2 = 0.18, P<0.001). As flies aged, wingbeat frequency (T. dalmanni, R2 = 0.80, P<0.001; D. meigenii, R2 = 0.68, P<0.001), and wing velocity (T. dalmanni, R2 = 0.73, P<0.001; D. meigenii, R2 = 0.70, P<0.001) increased during both normal and maximal flight (Table 3). Maximal flight capacity was positively associated with thorax mass and wingtip velocity (Figure 4; ANCOVA: P<0.001 for both comparisons; linear regression: R2 = 0.49 and 0.53, respectively, P<0.001). Thus, as thorax mass increased, wingbeat frequency increased (linear regression: T. dalmanni, R2 = 0.63, P<0.001; D. meigenii, R2 = 0.63, P<0.001) and wing velocity increased (Figure 4), which facilitated the improvement in maximal flight capacity.
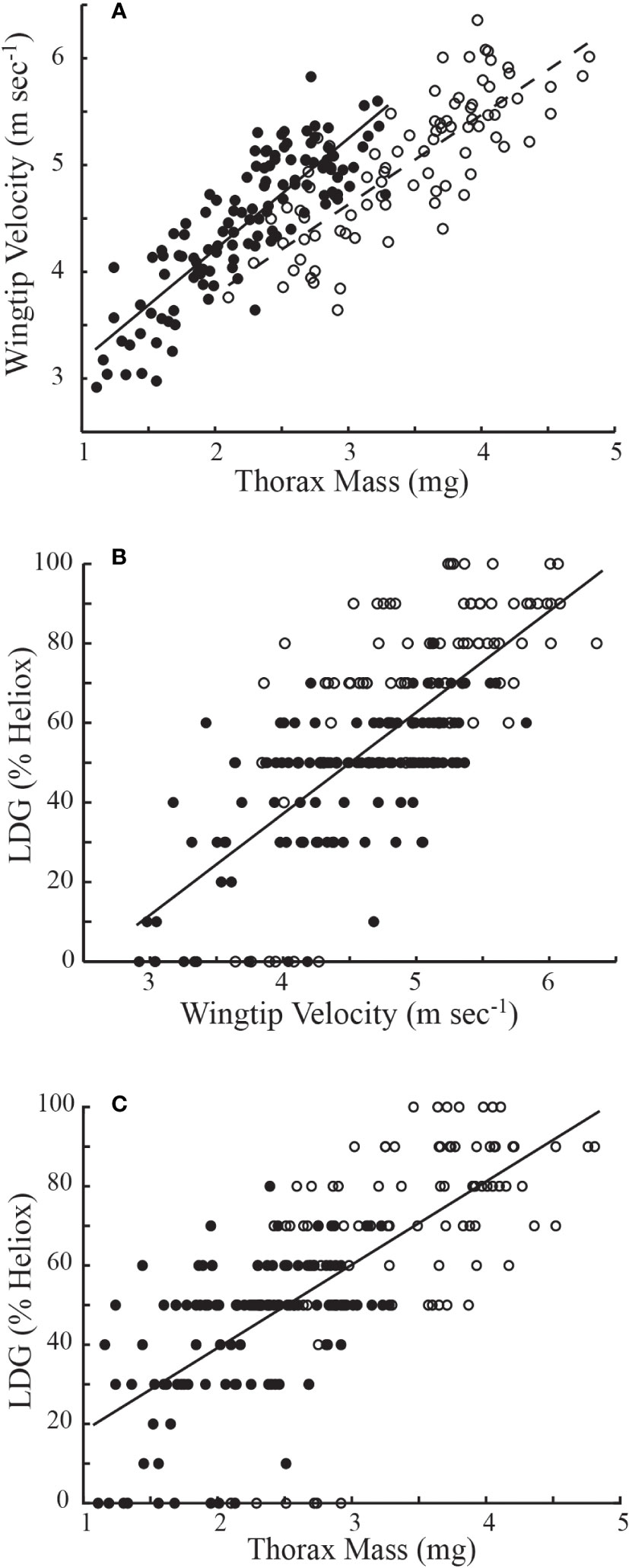
Figure 4 Effects of Morphology on Flight Performance in T. dalmanni (filled circles) and D. meigenii (open circles). Maximal flight capacity, LDG, is defined as the least dense gas allowing for flight, where 0% Heliox is air (21% O2, 79% N2, 0% He; density: 1.21 kg m-3) and 100% is pure Heliox (21% O2, 0% N2, 79% He; density: 0.41 kg m-3). (A) Wingtip velocity increased across thorax mass in both species (P<0.001): linear regression: T. dalmanni (solid line), Ut = 2.13 + 1.04Mt, R2 = 0.72; D. meigenii (dashed line), Ut = 2.11 + 0.84Mt, R2 = 0.65. (B) Maximal flight capacity (LDG, in percent-heliox) increased across wingtip velocity for both species (P<0.001); linear regression: LDG = -6.5 + 2.55Ut, R2 = 0.53. (C) Maximal flight capacity (LDG, in percent-heliox) increased across thorax mass for both species (P<0.001); linear regression: LDG = -0.28 + 2.1Mt, R2 = 0.49.
4 Discussion
Stalk-eyed flies reach sexually maturity 3- to 4-weeks post-eclosion (Baker et al., 2003; Reguera et al., 2004), in part due to a 2- to 3-fold increase in accessory gland and testis size during this period (Baker et al., 2003). This growth of reproductive tissues is reflected by a 166% increase in abdominal mass in 28- to 30-day old T. dalmanni, and a 104% increase in abdominal mass in 18- to 20-day old D. meigenii, compared to 1-day old flies (Figure 3D). Increasing abdominal mass during this developmental period should impact flight-dependent behaviors (Isaacs and Byrne, 1998), as it otherwise does not contribute to the flight motor and requires elevated aerodynamic output, reducing an insect’s available reserve capacity (Vance et al., 2009). However, thorax mass increased 103% across age in T. dalmanni, and increased 67% across age in D. meigenii (Figure 3C). This investment into the growth of the thorax did not appear to lag with respect to abdominal growth, and T. dalmanni exhibited a modest increase in the ratio of thorax mass to body mass across age while D. meigenii maintained this ratio (Figure 3E).
The development of the thorax facilitated an improvement in wingbeat frequency with age. The mass of the thorax cuticle and wings are presumably fixed post-eclosion, and the mechanical properties of resonating and reciprocating passive structures are likewise presumed constant post-sclerotization. Age-dependent improvements in the performance of the flight motor in adults have been observed in several orders of insects. Physiological changes often occur soon after eclosion and/or the onset of flight-dependent behaviors, and may include alternative splicing of troponin variants which affect muscle calcium sensitivity (Marden et al., 1999; Schippers et al., 2006), and increased enzymatic activity, such as pyruvate kinase and citrate synthase which support aerobic metabolism (Harrison 1996); together, these may facilitate an improvement in the ability to maintain and/or increase wingbeat frequency during elevated flight performance (Vance et al., 2009). However, few studies of adult maturation in insects have characterized changes in thorax mass. For example, honeybees do not vary thorax mass across age despite the transition from in-hive (e.g. nursing) to flight-intensive foraging behavior (Harrison 1996). Adult beetles exhibit an ‘income breeder’ strategy leading up to dispersal: thorax mass increased across age, but lipid reserves, not muscle mass, were associated with improvements in flight endurance (David et al., 2015). And dragonflies, though hemimetabolous (as compared to the holometabolous bees, beetles and flies), increased thorax mass 2.5-fold between tenerel and mature stages, which was attributed to muscle growth (Marden, 1989).
Nonetheless, the two-fold increase in thorax mass we observed across age in adult stalk-eyed flies was unexpected. Given the lekking behavior and general localization of lek sites where T. dalmanni and D. meigenii aggregate, we suspect lipid stores to support flight endurance were not the main constituents of this increased thorax mass; rather, we posit this thoracic development results from investment in muscle mass, and subsequent increased muscle stiffness and power to drive the indirect actuation of the wings (Figure 4A). As flies were able to generate greater wing velocity as their thorax mass increased, they were able to produce greater aerodynamic power, resulting in increased maximal flight capacity (Figures 4B, C). Thus, as flies became heavier with age, in part due to the growth of reproductive tissues in the abdomen, the concurrent development of the thorax compensated for this growth and is associated with increased maximal flight capacity across age (Table 3).
Studies of sexually-mature, adult stalk-eyed flies suggest thorax and wing size have co-evolved with eyespan as a mechanism to compensate for the inertial and aerodynamic costs associated with bearing larger ornaments and having a larger body (Ribak and Swallow, 2007; Ribak et al., 2009; Husak et al., 2011; Husak et al., 2013). Indeed, both T. dalmanni and D. meigenii males in our study exhibit positive allometry relative to eyespan (Figure S1). Flies bearing larger ornaments had heavier total body mass and heavier heads, which would increase the moment of inertia and aerodynamic requirements for flight, potentially impairing maneuvering performance. However, significant investment into the ‘flight motor’ accompanied larger ornaments, as flies with longer eyespan also had wider and heavier thoraces, and longer wings with greater wing area (Table 1). This facilitated the maintenance of wingbeat frequency and stroke amplitude during normal and maximal hovering which, when actuating longer wings, contributed to an increase in wing velocity across eyespan. Thus, the larger ‘flight motor’ and kinematic output was sufficient to compensate for larger eyespan and maintain maximal flight capacity, despite these flies being larger overall. This conservation/improvement of maximal flight capacity while bearing longer ornaments provides a mechanistic explanation as to why Ribak and Swallow (2007) found similar turning performance between male and female T. dalmanni.
Our findings generally support prior conclusions, as individual T. dalmanni and D. meigenii with larger eyespan had wider thoraces and larger wings, and were generally heavier. Thus, within this sample, one might predict compensation by comparing relative ornament size to relative trait size (Husak et al., 2011). However, prior work focused on sexually-mature flies, presumably to avoid the sexual development-related variation in body mass in young adults from confounding allometric relationships and behaviors (Ribak and Swallow, 2007; Egge et al., 2011; Husak et al., 2011). The variation in abdomen and thorax mass, kinematic output and flight capacity across age in T. dalmanni and D. meigenii suggests the relationship of compensatory traits to the fixed eyespan is not constant across age. Young, sexually-immature flies, in addition to their reduced fecundity at this age, have reduced kinematic and maximal flight capacity which may impair flight-dependent behaviors, such as maneuvering and predator evasion, and impact survivorship and fitness.
As adult T. dalmanni and D. meigenii males aged, they became stronger fliers. This age-related variation in flight performance critically depends on the development of the thorax, specifically the flight muscle. In this study, the pace of thorax development compensated for the pace of sexual development (i.e. abdominal mass), such that the ratio of thorax mass to body mass was maintained (D. meigenii) and/or increased (T. dalmanni) across age (Figure 3E). If the development of reproductive tissues were prioritized over muscle development, flies may not maintain the ratio of thorax mass to total body mass necessary to improve -or perhaps even conserve- flight capacity as they approach sexual maturity. For example, if 25- to 30-day old T. dalmanni in this study did not exhibit thorax development, but instead maintained thorax mass similar to <5-day old flies, their thorax-to-body mass ratio would be reduced by 31%, as would their ability to generate wing velocity to compensate for their increased body mass across age. Time to sexual maturity and mating frequency critically depends on the growth of the accessory glands (Baker et al., 2003). If the growth of the accessory glands, as well as growth of the testis, are in competition for resources with the thorax muscle, then perhaps the locomotor benefit of maintaining or improving flight capacity during this period confers a fitness advantage—by way of resource acquisition, conspecific competition and predator evasion facilitated by flight and maneuvering performance—even though it may delay the time to sexual maturity. Accelerating the time to sexual maturity at the expense of flight performance may predispose individuals to competition and predation that ultimately limits fecundity.
Finally, consider that the flies in this study were fed ad libitum and were not crowded in their enclosures: our findings may reflect an ideal or optimal scenario for the development of flight muscle. If, instead, food availability was scarce, nutritionally deficient, or subject to strong competition, how might the development of the thorax compare to the development of the abdomen? Deriving fitness from proxies of survival and fecundity gleaned from sexually-mature flies may represent a best-case scenario for the relationships between compensatory traits and ornaments, as such experiments select flies that have made it through a potentially-costly period of development. However, it cannot necessarily extrapolate to prior developmental periods, where age-related fecundity depends on the growth of reproductive tissues (Baker et al., 2003), and where age-related survivorship may be impacted by the dependence of flight behaviors on an individual’s flight capacity. Likewise, our findings here are not limited simply to characterizing the relationships between ornaments and compensatory traits, but may be more broadly applicable to model systems where developmental trajectory—even within the insect adult stage—predisposes individuals to periods of sub-optimal performance while coupled with high resource acquisition, competition and/or predation costs.
Assessing the costs of bearing ornaments has been elusive where morphological traits and behaviors may compensate for impairments (Møller, 1996; Tomkins et al., 2005; Oufiero and Garland, 2007). For example, swordtail fish with the longest ornaments have larger bodies which contribute to faster burst swimming performance, offsetting the drag imposed by their longer tails (Royle et al., 2006); and, wing dimorphism, which has evolved with tail dimorphism in long-tailed birds, is more pronounced in those species where the tail morphologies impose increased aerodynamic drag (Balmford et al., 1993). However, despite ameliorating ornament costs, compensatory morphology and behaviors have their own associated costs: investment into larger body and wing planforms, and subsequent development of tissues, requires increased resources and energy to build up these structures, as well as support the requirements of being larger and heavier while performing metabolically-expensive locomotor behaviors. Under presumably ideal conditions, adult male stalk-eyed flies compensate for increased eyespan with a larger flight ‘motor’ and develop flight performance that should offset costs associated with bearing those ornaments. Future research that investigates the distribution of thoracic and abdominal tissues (particularly, muscle, reproductive tissues, lipids, etc.) may elucidate the investment and allocation costs associated with the age-related growth described here. Furthermore, manipulating factors of nutrition, such as food availability and quality, may potentially skew the developmental trajectory of thorax and/or abdominal tissues, providing insight into the functional and fecundity costs of bearing large ornaments when compensatory mechanisms compete with sexual maturation for resources.
Data availability statement
The raw data supporting the conclusions of this article will be made available by the authors, without undue reservation.
Author contributions
JV and JS contributed to the conception and design of the study. JV and KP performed the experiment and analyzed the data. JV, CA, and JS wrote the manuscript. All authors contributed to the article and approved the submitted version.
Funding
This research was funded by a National Science Foundation grant (NSF IOS 1656478) to JV, and a National Science Foundation grant (NSF IOS 1656465) to JS.
Acknowledgments
The authors acknowledge that neither ChatGPT, nor any LLM, was used in the preparation or editing of this manuscript.
Conflict of interest
The authors declare that the research was conducted in the absence of any commercial or financial relationships that could be constructed as a potential conflict of interest.
The author JS declared that they were an editorial board member of Frontiers, at the time of submission. This had no impact on the peer review process and the final decision.
Publisher’s note
All claims expressed in this article are solely those of the authors and do not necessarily represent those of their affiliated organizations, or those of the publisher, the editors and the reviewers. Any product that may be evaluated in this article, or claim that may be made by its manufacturer, is not guaranteed or endorsed by the publisher.
Supplementary material
The Supplementary Material for this article can be found online at: https://www.frontiersin.org/articles/10.3389/fetho.2023.1242198/full#supplementary-material
Supplementary Figure 1 | Allometry of stalk-eyed flies, T. dalmanni (filled circles) and D. meigenii (open circles). (A) Thorax width in both species increased with eyespan (P<0.001). Linear regression: T. dalmanni (solid line), thorax width = 0.45 + 0.15eye, R2 = 0.30; D. meigenii (dashed line), thorax width = 0.82 + 0.14eye, R2 = 0.20. (B) Wing length in both species increased with eyespan (P<0.001). Linear regression: T. dalmanni (solid line), wing length = 2.53 + 0.27eyespan, R2 = 0.25; D. meigenii (dashed line), wing length = 2.98 + 0.28eye, R2 = 0.16. (C) Wing area in both species increased with eyespan (P<0.001). Linear regression: T. dalmanni (solid line), wing area = 0.04 + 0.52eyespan, R2 = 0.69; D. meigenii (dashed line), S = -0.47 + 0.80eye, R2 = 0.66.
References
Arnold S. J. (1983). Morphology, performance and fitness. Am. Zool. 23, 347–361. doi: 10.1093/icb/23.2.347
Baker R. H., Ashwell R. I. S., Richards T. A., Fowler K., Chapman T., Pomiankowski A. (2001). Effects of multiple mating and male eye span on female reproductive output in the stalk-eyed fly, Cyrtodiopsis dalmanni. Behav. Ecol. 12, 732–739. doi: 10.1093/beheco/12.6.732
Baker R. H., Denniff M., Futerman P., Fowler K., Pokiankowski A., Chapman T. (2003). Acessory gland size influences time to sexual maturity and mating frequency in the stalk-eyed fly, Crytodiopsis dalmanni. Behav. Ecol. 14, 607–611. doi: 10.1093/beheco/arg053
Baker R. H., Wilkinson G. S. (2001). Phylogenetic analysis of sexual dimorphism and eye-span allometry in stalk-eyed flies (Diopsidae). Evolution 55, 1373–1385. doi: 10.1111/j.0014-3820.2001.tb00659.x
Balmford A., Jones I. L., Thomas A. L. R. (1993). How to compensate for costly sexually selected tails: the origin of sexually dimorphic wings in long-tailed birds. Evolution 48, 1062–1070. doi: 10.2307/2410366
Bath E., Wigby S., Vincent C., Tobias J. A., Seddon N. (2015). Condition, not eyespan, predicts contest outcome in female stalk-eyed flies,Teleopsis dalmanni. Ecol. Evol. 5, 1826–1836. doi: 10.1002/ece3.1467
Bellamy L., Chapman N., Fowler K., Pomiankowski A. (2013). Sexual traits are sensitive to genetic stress and predict extinction risk in the stalk-eyed fly, Diasemopsis meigenii. Evolution 67, 2662–2673. doi: 10.1111/evo.12135
Berglund A., Bisazza A., Pilastro A. (1996). Armaments and ornaments: an evolutionary explanation of traits of dual utility. Biol. J. Linn. Soc. 58, 385–399. doi: 10.1111/j.1095-8312.1996.tb01442.x
Bubak A. N., Gerkin A. R., Watt M. J., Costabile J. D., Renner K. J., Swallow J. G. (2016). Assessment strategies and fighting patterns in animal contests: a role for serotonin? Curr. Zool. 62, 257–263. doi: 10.1093/cz/zow040
Burkhardt D., de la Motte I. (1983). How stalk-eyed flies eye stalk-eyed flies: Observations and measurements of the eyes of Cyrtodiopsis whitei (Diopsidae, Diptera). J. Comp. Physiol. 151, 407–421. doi: 10.1007/bf00605457
Burkhardt D., de la Motte I. (1985). Selective pressures, variability and sexual dimorphism in stalk-eyed flies (Diopsidae). Die. Naturwissenschaften. 72, 204–206. doi: 10.1007/BF01195763
Burkhardt D., de la Motte I. (1987). Physiological, behavior, and morphometric data elucidate the evolutive significance of stalked eyes in Diopsidae (Diptera). Entomol. Generalis. 12, 221–233. doi: 10.1127/entom.gen/12/1987/221
Burkhardt D., de la Motte I. (1988). Big ‘antlers’ are favoured: female choice in stalk-eyed flies (Diptera, Insecta), field collected harems and laboratory experiments. J. Comp. Physiol. 162, 649–652. doi: 10.1007/BF01342640
Charnov E. L. (1993). Life history invariants: some explorations of symmetry in evolutionary ecology (Oxford: Oxford University Press).
David G., Giffard B., van Halder Piou I D., Jactel H. (2015). Energy allocation during the maturation of adults in a long-lived insect: implications for dispersal and reproduction. Bull Entomol Res. 105, 629–36. doi: 10.1017/S0007485315000553
Dudley R. (1995). Extraordinary flight performance of orchid bees (Apidae: Euglossini) hovering in heliox (80% He/20% O2). J. Exp. Biol. 198, 1065–1070. doi: 10.1242/jeb.198.4.1065
Egge A. R., Brandt Y., Swallow J. G. (2011). Sequential analysis of aggressive interactions in the stalk-eyed fly Teleopsis dalmanni. Behav. Ecol. Sociobiol. 65, 369–379. doi: 10.1007/s00265-010-1054-5
Harrison J. M. (1996). Caste-specific changes in honeybee flight capacity. Physiol. Zool. 59, 175–187. doi: 10.1086/physzool.59.2.30156031
Hedrick T. L. (2008). Software techniques for two- and three-dimensional kinematic measurements of biological and biomimetic systems. Bioinspiration. Biomimetics. 3, 34001. doi: 10.1088/1748-3182/3/3/034001
Husak J. F., Ribak G., Baker R. H., Rivera G., Wilkinson G. S., Swallow J. G. (2013). Effects of ornamentation and phylogeny on the evolution of wing shape in stalk-eyed flies (Diopsidae). J. Evol. Biol. 26, 1281–1293. doi: 10.1111/jeb.12133
Husak J. F., Ribak G., Wilkinson G. S., Swallow J. G. (2011). Compensation for exaggerated eye stalks in stalk-eyed flies (Diopsidae). Funct. Ecol. 25, 608–616. doi: 10.1111/j.1365-2435.2010.01827.x
Husak J. F., Swallow J. G. (2011). Compensatory traits and the evolution of male ornaments. Behaviour 148, 1–29. doi: 10.1163/000579510x541265
Irschick D. J., Meyers J. J., Husak J. F., Le Galliard J. F. (2008). How does selection operate on whole-organism functional performance capacities? A review and synthesis. Evol. Ecol. Res. 10, 177–196. doi: 10.7275/R58G8HX6
Isaacs B., Byrne D. N. (1998). Aerial distribution, flight behavior and eggload: their inter-relationship during dispersal by the sweetpotato whitefly. J. Anim. Ecol. 76, 741–750. doi: 10.1046/j.1365-2656.1998.00236.x
Iwasa Y., Pomiankowski A., Nee S. (1991). The evolution of costly mate preferences. II. The "handicap" principle. Evolution 45, 1431–1442. doi: 10.1111/j.1558-5646.1991.tb02646.x
Kotiaho J. S. (2001). Costs of sexual traits: A mismatch between theoretical considerations and empirical evidence. Biol. Rev. 76, 365–376. doi: 10.1017/S1464793101005711
Lailvaux S. P., Husak J. F. (2014). The life history of whole-organism performance. Q. Rev. Biol. 89, 285–318. doi: 10.1086/678567
Lailvaux S. P., Irschick D. J. (2006). A functional perspective on sexual selection: insights and future prospects. Anim. Behav. 72, 263–273. doi: 10.1016/j.anbehav.2006.02.003
Marden J. H. (1989). Bodybuilding dragonflies: costs and benefits of maximizing flight muscle. Physiol. Zool. 62, 505–521. doi: 10.1086/physzool.62.2.30156182
Marden J. H., Fitzhugh G. H., Wolf M. R., Rowan B. (1999). Alternative splicing, muscle calcium sensitivity, and the modulation of dragonfly flight performance. Proc. Natl. Acad. Sci. U.S.A. 96, 15304–15309. doi: 10.1073/pnas.96.26.15304
Møller A. P. (1996). The cost of secondary sexual characters and the evolution of cost-reducing traits. Ibis 138, 112–119. doi: 10.1111/j.1474-919X.1996.tb04317.x
Oufiero C. E., Garland (2007). Evaluating performance costs of sexually selected traits. Funct. Ecol. 21, 676–689. doi: 10.1111/j.1365-2435.2007.01259.x
Panhuis T. M., Wilkinson G. S. (1999). Exaggerated male eye span influences contest outcome in stalk-eyed flies (Diopsidae). Behav. Ecol. Sociobiol. 46, 221–227. doi: 10.1007/s002650050613
Pitnick S., Markow T. A., Spicer G. S. (1995). Delayed male maturity is a cost of producing large sperm in Drosophila. Proc. Natl. Acad. Sci. 92, 10614–10618. doi: 10.1073/pnas.92.23.10614
Pomiankowski A., Denniff M., Fowler K., Chapman T. (2005). The costs and benefits of high early mating rates in male stalk-eyed flies, Cyrtodiopsis dalmanni. J. Insect Physiol. 51, 1165–1171. doi: 10.1016/j.jinsphys.2005.06.006
Reguera P., Pomiankowski A., Fowler K., Chapman T. (2004). Low cost of reproduction in female stalk-eyed flies, Cyrtodiopsis dalmanni. J. Insect Physiol. 50, 103–108. doi: 10.1016/j.jinsphys.2003.10.004
Ribak G., Pitts M. L., Wilkinson G. S., Swallow J. G. (2009). Wing shape, wing size, and sexual dimorphism in eye-span in stalk-eyed flies (Diopsidae). Biol. J. Linn. Soc. 98, 860–871. doi: 10.1111/j.1095-8312.2009.01326.x
Ribak G., Swallow J. G. (2007). Free flight maneuvers of stalk-eyed flies: do eye-stalks affect aerial turning behavior? J. Comp. Physiol. A. 193, 1065–1079. doi: 10.1007/s00359-007-0259-1
Rogers D. W., Chapman T., Fowler K., Pomiankowski A. (2005). Mating-induced reduction in accessory reproductive organ size in the stalk-eyed fly Cyrtodiopsis dalmanni. BMC. Evol. Biol. 5, 37. doi: 10.1111/j.1365-2435.2006.01147.x
Royle N. J., Metcalfe N. B., Lindström J. (2006). Sexual Selection, Growth Compensation and Fast-Start Swimming Performance in Green Swordtails, Xiphophorus helleri. Func. Ecol. 20, 662–669. doi: 10.1111/j.1365-2435.2006.01147.x
Schippers M.-P., Dukas R., Smith R. W., Wang J., Smolen K., McClelland G. B. (2006). Lifetime performance in foraging honeybees: behaviour and physiology. J. Exp. Biol. 209, 3828–3836. doi: 10.1242/jeb.02450
Small J., Cotton S., Fowler K., Pomiankowski A. (2009). Male eyespan and resource ownership affect contest outcome in the stalk-eyed fly, Teleopsis dalmanni. Anim. Behav. 78, 1213–1220. doi: 10.1016/j.anbehav.2009.08.009
Swallow J. G., Hayes J. P., Koteja P., Garland J. T. (2009). “Selection experiments and experimental evolution of performance and physiology,” in Experimental evolution: concepts, methods, and applications of selection experiments. Eds. Garland T. Jr, Rose M. R. (Berkeley, CA: University of California Press), 301–351.
Swallow J. G., Wilkinson G. S., Marden J. H. (2000). Aerial performance of stalk-eyed flies that differ in eye span. J. Comp. Physiol. B.: Biochem. Systemic. Environ. Physiol. 170, 481–487. doi: 10.1007/s003600000124
Számadó S. (2010). The cost of honesty and the fallacy of the handicap principle. Anim. Behav. 81, 3–11. doi: 10.1016/j.anbehav.2010.08.022
Tigeros N., Davidowitz G. (2019). Flight-fecundity tradeoffs in wing-morphometric insects. Adv. Insect Physiol. 56, 1–41. doi: 10.1016/bs.aiip.2019.02.001
Tomkins J. L., Kotiaho J. S., LeBas N. R. (2005). Phenotypic plasticity in the developmental integration of morphological trade-offs and secondary sexual trait compensation. Proc.: Biol. Sci. 272, 543–551. doi: 10.1098/rspb.2004.2950
Vance J. T., Roberts S. P. (2014). The effects of artificial wing wear on the flight capacity of the honey bee Apis mellifera. J. Insect Physiol. 65, 27–36. doi: 10.1016/j.jinsphys.2014.04.003
Vance J. T., Williams J. B., Elekonich M. M., Roberts S. P. (2009). The effects of age and behavioral development on honey bee (Apis mellifera) flight performance. J. Exp. Biol. 212, 2604–2611. doi: 10.1242/jeb.028100
Wilkinson G. S., Dodson G. N. (1997). “Function and evolution of antlers and eye stalks in flies,” in The evolution of mating systems in insects and arachnids. Eds. Choe J., Crespi B. (Cambridge: Cambridge University Press), 310–328.
Wilkinson G. S., Kahler H., Baker R. H. (1998). Evolution of female mate preferences in stalk-eyed flies. Behav. Ecol. 9, 525–533. doi: 10.1093/beheco/9.5.525
Worthington A. M., Swallow J. G. (2010). Gender differences in survival and antipredatory behavior in stalk-eyed flies. Behav. Ecol. 21, 759–766. doi: 10.1093/beheco/arq050
Keywords: sexual selection, ornaments, compensation, flight performance, stalk-eyed flies
Citation: Vance JT, Pehl K, Acakpo CJ and Swallow JG (2023) Compensation for a costly ornament depends on the development of flight performance in stalk-eyed flies. Front. Ethol. 2:1242198. doi: 10.3389/fetho.2023.1242198
Received: 18 June 2023; Accepted: 18 September 2023;
Published: 03 October 2023.
Edited by:
Gordon M. Burghardt, The University of Tennessee, Knoxville, United StatesReviewed by:
Lee Drickamer, Northern Arizona University, United StatesRichard Baker, American Museum of Natural History, United States
Copyright © 2023 Vance, Pehl, Acakpo and Swallow. This is an open-access article distributed under the terms of the Creative Commons Attribution License (CC BY). The use, distribution or reproduction in other forums is permitted, provided the original author(s) and the copyright owner(s) are credited and that the original publication in this journal is cited, in accordance with accepted academic practice. No use, distribution or reproduction is permitted which does not comply with these terms.
*Correspondence: Jason T. Vance, dmFuY2VqdEBjb2ZjLmVkdQ==