- 1Department of Ecology, Montana State University, Bozeman, MT, United States
- 2Zambian Carnivore Programme, Mfuwe, Eastern Province, Zambia
- 3Institutionen för Vilt, Fisk och Miljö, Sveriges lantbruksuniversitet, Umeå, Sweden
- 4Wildlife Conservation Research Unit, Oxford University, Oxford, United Kingdom
- 5US Agency for International Development, Lusaka, Zambia
- 6Rubenstein School of the Environment, University of Vermont, Burlington, VT, United States
- 7Montana Cooperative Wildlife Research Unit, University of Montana, Missoula, MT, United States
- 8Department of Ecosystem Science and Management, University of Wyoming, Laramie, WY, United States
- 9Marine Mammals Management, US Fish and Wildlife Service, Anchorage, AK, United States
- 10South Luangwa Area Management Unit, Department of National Parks and Wildlife, Mfuwe, Eastern Province, Zambia
- 11Department of National Parks and Wildlife, Kafue National Park, Chunga, Central Province, Zambia
Introduction: Predators can affect prey not only by killing them, but also by causing them to alter their behavior, including patterns of habitat selection. Prey can reduce the risk of predation by moving to habitats where predators are less likely to detect them, less likely to attack, or less likely to succeed. The interaction of such responses to risk with other ecological processes remains relatively unstudied, but in some cases, changes in habitat use to avoid predation may be constrained by competition: larger, dominant competitors should respond freely to predation risk, but the responses of smaller, subordinate competitors may be constrained by the responses of dominant competitors. For large grazing herbivores, an alternative hypothesis proposes that smaller prey species are vulnerable to more predators, and thus should respond more strongly to predation risk.
Methods: Here, we tested these two hypotheses with 775 observations of habitat selection by four species of obligate grazers (zebra, wildebeest, puku and oribi) in the immediate presence or absence of four large carnivores (lion, spotted hyena, African wild dog and cheetah) in three ecosystems (Greater Liuwa, Greater Kafue and Luangwa Valley). Patterns of predation within this set were described by observation of 1,105 kills.
Results: Our results support the hypothesis that responses to predation risk are strongest for larger, dominant competitors. Even though zebras were killed least often, they showed the strongest shift into cover when carnivores were present. Wildebeest, puku and oribi showed weaker habitat shifts, even though they were more frequently killed. These patterns remained consistent in models that controlled for differences in the hunting mode of the predator (stalking, coursing, or intermediate) and for differences among ecosystems. There was no evidence that smaller species were subject to predation by a broader set of predators. Instead, smaller prey were killed often by smaller predators, and larger prey were killed often by larger predators.
Discussion: Broadly, our results show that responses to predation risk interact with interspecific competition. Accounting for such interactions should help to explain the considerable variation in the strength of responses to predation risk that has been observed.
1 Introduction
Considerable research has shown that spatial and temporal variation in predation risk affects patterns of movement and habitat selection by prey. This evidence comes from observational and experimental studies of a broad set of species ranging from aphids and Daphnia to songbirds and elk (Losey and Denno, 1998; Creel et al., 2005; Pangle et al., 2007; Lima, 2009). Much of the early research on trade-offs between foraging and predation risk focused on rodents and aquatic invertebrates, because experiments with semi-natural enclosures are tractable with these species. For example, the introduction of a weasel (Mustela nivalis) into a 150 m2 field enclosure for periods of 2 to 24 hours caused bank voles (Clethrionomys glareolus) to shift to areas that had not been used by the weasel (Jędrzejewski and Jędrzejewska, 1990). For meadow voles (Microtus pennsylvanicus) in field enclosures, experimentally increasing protective cover allowed an increase in vole foraging activity that caused an 85% increase in reproduction (Dehn et al., 2017). In semi-natural experiments, mayflies of several species moved out of areas with the chemical cues of stonefly predators, caged stoneflies, or stoneflies that were rendered non-lethal by gluing their mouths shut (Peckarsky, 1980). This predator avoidance led to reduced foraging success, decreased growth and decreased reproduction (Peckarsky et al., 1993). Snails (Physa and Physella) responded to aquatic predators or their chemical cues by hiding in the substrate or leaving the water, which reduced their foraging efficiency, growth, and reproduction (Crowl and Covich, 1990; Sih and McCarthy, 2002).
Because habitat selection is often sensitive to predation risk, it is clear that predation risk has the potential to alter and be altered by other ecological interactions between prey species (Schmitz et al., 1997; Orrock et al., 2008; Peckarsky et al., 2008). In particular, competition among prey species can affect their exposure to predation, if the antipredator responses of subordinate competitors are constrained by dominant competitors (Orrock et al., 2008). Bouskila (1995) examined this possibility with a field experiment that manipulated the presence of sidewinder (Crotalus cersates) predators for two species of kangaroo rat prey; Dipodomys deserti (the larger and dominant competitor), and Dipodomys merriami (the smaller and subordinate competitor). Bouskila found a strong shift out of dangerous microhabitats by competitively dominant D. deserti when the predator was present, and a weaker shift into dangerous microhabitats by competitively subordinate D. merriami. From this pattern, Bouskila inferred that “the dominant species primarily responds to the distribution of risk from the predator, while the subordinate species appears to respond to both the risk of predation and the risk of interference from the dominant competitor” (Bouskila, 1995).
Bouskila’s inferences might predict general differences among a set of competitors in their responses to temporal variation in predation risk. Dominant competitors should show stronger shifts in habitat selection in response to temporal variation in risk, adopting a strategy that optimizes the trade-off between food and safety (Lima and Dill, 1990; Lima, 1998; Kotler et al., 2004; Lima, 2009) with relatively little constraint from competition. Subordinate competitors should show weaker (or even opposite) responses: if a dominant species shifts into safe habitat in response to short term risk, asymmetry in the costs of competition will disfavor a similar response by subordinate competitors.
In contrast to Bouskila’s hypothesis, Hopcraft et al. (2012), noted that smaller grazers in the Serengeti large herbivore guild are killed by a wider range of predator species, and suggested that predation risk should therefore have a stronger effect on the distribution of small herbivores such as Thomson’s and Grant’s gazelles (Eudorcas thomsoni and Nanger granti) than for large herbivores such as the buffalo (Syncerus caffer) (Hopcraft et al., 2012). However, it is questionablewhether the vulnerability of small herbivores to smaller predators such as cheetahs (Acinonyx jubatus) and wild dogs (Lycaon pictus) causes them to be at greater risk, because large carnivores such as lions (Panthera leo) and spotted hyenas (Crocuta crocuta) typically prefer large prey. These large, competitively dominant carnivores outnumber their smaller competitors in most ecosystems and thus account for the majority of total predation. Because large carnivores usually focus their predation on relatively large prey such as wildebeest, Connochaetes taurinus (Mills and Biggs, 1993; Creel and Creel, 1996; Owen-Smith and Mills, 2008; Durant et al., 2017; Creel et al., 2018), it is not clear that smaller prey will generally experience more exposure to predation.
To summarize, Bouskila’s logic suggests that the larger, dominant competitors within a foraging guild should show stronger habitat shifts when predation risk is high, while Hopcraft’s logic predicts the opposite. Here, we tested these two hypotheses by observing responses of grazing herbivores to the immediate presence or absence of large carnivores in three Zambian ecosystems. These systems are well-suited to this test, for several reasons. First, they hold a guild of obligate grazers whose evolution has been shaped by competition for shared resources, producing considerable variation in size and competitive ability (Sinclair, 1985; Dublin et al., 1990; Hopcraft et al., 2010; Hopcraft et al., 2012; Pansu et al., 2019). Second, the complete guild of large predators that commonly kill these grazers, including both stalking and coursing predators, remains present in these ecosystems. Third, we have good data on patterns of predation on each prey species by each of the predators (Creel et al., 2017; Creel et al., 2018; Creel et al., 2019). Fourth, prior research has shown that species in this foraging guild have strong behavioral responses to spatial and temporal variation in the risk of predation (Valeix et al., 2007; Valeix et al., 2009; Thaker et al., 2011; Barnier et al., 2014; Creel et al., 2014; Creel et al., 2017; Creel et al., 2019).
Finally, prior research has shown that changes in habitat selection are an important component of antipredator responses by this guild. Valeix et al. (2009) examined the responses of eight herbivores, including zebra and wildebeest, to variation in predation risk from lions in Hwange National Park, and found that all species increased their use of open grassland when lions were present (though wildebeest strongly selected open grassland areas at all times), attributing this shift to better detection of stalking predators in open habitats. The generality of this response warrants further investigation in other ecosystems, because predation by lions in Hwange occurs almost entirely at artificial boreholes. Open grassland areas were mainly adjacent to boreholes, so that animals that moved from water to avoid lions were likely to move into grassland by default (Valeix et al., 2009). In Kenya’s Ol Pejeta Conservancy, zebras showed the opposite response, reducing their use of open grassland and shifting into woodland at times of high predation risk from lions (Fischhoff et al., 2007). In South Africa’s Karongwe Game Reserve, wildebeest and zebra avoided areas that were more heavily used by lions over the long term, and zebras (but not wildebeest) avoided the open woodland habitat in which they were both killed most often (Thaker et al., 2011). Data from Tanzania’s Serengeti National Park showed that at coarse spatial (25 km2 cells) and temporal (annual) scales, small grazers were less likely to use areas that were inferred to have high predation risk, when compared to large grazers (Hopcraft et al., 2012).
To summarize these prior studies, African large grazers are known to modify habitat selection in response to both long-term and short-term variation in predation risk, and there appears to be considerable variation among species and ecosystems in these responses. There remains considerable scope to better understand differences between prey species in their responses to predation risk, and whether these differences are typically related to interspecific competition in the manner suggested by Bouskila (stronger responses by larger species) or in the manner suggested by Hopcraft et al. (stronger responses by smaller species).
Observation of short-term changes in habitat selection in response to the immediate presence or absence of predators are well suited to resolving some of this ambiguity. First, this method quantifies the effects of risk by direct comparison of prey behavior when predators are present vs. absent. Methods that assess risk solely from attributes of the landscape are less direct, and create collinearities that make it difficult to disentangle responses to risk from other environmental effects. By testing for changes in habitat selection with predators known to be immediately present or absent, we can test for effects of risk that are independent of other variables that affect habitat selection. Second, this method avoids the inferential problems that arise when risk is simulated using a subset of the cues that are present when prey face real predation risk, which provides a complex and dynamic combination of olfactory, auditory and visual information, including the movements and behavior of the predator. Failure to react to simulated risk is often interpreted as a lack of response to ‘perceived risk’, but it is also plausible to infer that prey can correctly perceive that a simulation poses no real risk. This alternative is particularly plausible for prey such as large herbivores, which have evolved acute senses, well-developed cognition, and behavior that is strongly conditional on sensory information. Third, grazers can affect one another by competition but also by facilitation, where one species alters grazing conditions in a manner that improves the foraging opportunities for another (Murray and Brown, 1993; Arsenault and Owen-Smith, 2002), but the complicating effects of facilitation are not important on the short time scale examined by quantifying changes in habitat selection when a predator is immediately present or absent.
Many factors other than predation risk affect habitat selection (Morris, 2003). Notably, population density alters habitat selection within a species or foraging guild. At low density, all individuals can occupy high quality patches with little competition, but as competitors accumulate in high quality habitat patches, changing cost-benefit ratios favor the use of lower quality patches, particularly by subordinate competitors (Fretwell and Lucas, 1970). Experimental studies have shown that this effect of density on habitat selection is likely to influence the effect of predation risk on habitat selection (Abramsky et al., 1990; Abramsky et al., 2002; Kotler et al., 2004). Here, our intent was to test whether differences among competitors in their response to immediate predation risk were sufficiently consistent to emerge across a broad range of conditions, including differences in density, predator species, herd size and composition. If differences among competitors in their responses to risk are not consistent across these conditions, then they are less likely to affect fitness.
2 Materials and methods
2.1 Species and sites
The prey guild we studied included two large obligate grazers, zebra (Equus quagga) and wildebeest, and two small obligate grazers, puku (Kobus vardonii) and oribi (Ourebia ourebi). We recorded changes in the habitat occupied by these prey when large carnivores were immediately present or absent. These observations included responses to four large carnivores that commonly kill these prey, including two coursers (wild dog and spotted hyena), a stalker (lion), and one with an intermediate hunting strategy (cheetah).
We observed these species using standardized methods (see Field Methods, below) on long term study sites in three Zambian ecosystems that we have previously described in detail: the Greater Liuwa Ecosystem (GLE), the Greater Kafue Ecosystem (GKE), and the Luangwa Valley Ecosystem (LVE) (Creel et al., 2019). We examined responses to all of the large carnivores present in these ecosystems other than leopards, which we observed too infrequently to obtain representative data. Of the 48 combinations of predator (4 levels), prey (4 levels) and ecosystem (3 levels) that would exist in a full factorial design, we obtained data for 33 combinations: cheetahs and oribi are absent from LVE, puku are absent from GLE, and we had insufficient data to examine responses of any prey species to spotted hyenas in GKE, where their density is low (Creel et al., 2018).
2.2 Field methods
We made 775 observations of the four focal grazers between 1 January 2011 and 1 January 2017. We began each observation by locating one of the large carnivores by VHF radio tracking during crespuscular periods of activity. We recorded the carnivore’s identity and group size. When the carnivore group stopped moving, we recorded its location (using GPS), whether or not they were on a kill, and searched for prey. When a prey herd was found within 2 kilometers of the carnivore location, we recorded its location (again using GPS) and the vegetation type in which they were found (together with data on behavior and herd size that have been analyzed previously: (Creel et al., 2019)). For this analysis, vegetation types were classified as ‘grazing’, which were areas dominated by open grassland, ‘cover’, which were areas dominated by trees or shrubs, and ‘grazing-cover’, which were areas with a mixture of grassland and discrete patches of refuge habitat, either woodland edges (obstructive cover) or flooded pans (protective cover into which herbivores can retreat when attacked).
We determined the straight-line distance between the carnivore and prey at the time of observation, and classified an observations as having carnivores present if they were within 450 meters, and absent at greater distances. This threshold was selected because it has previously revealed large effects of risk on the behavior of these species in these ecosystems (Creel et al., 2019), but we confirmed that other thresholds between 250 and 1000 meters yielded similar inferences.
By following radiocollared carnivores, we observed 1,105 cases in which one of the four focal predators killed one of the four focal prey species. We recorded a kill when it was directly observed or when predator was found feeding on a fresh kill with no other predator species present. Fresh kills were identified by fresh blood and stomach contents, including blood on the carnivore’s face.
2.3 Statistical methods
For each of the four grazers, we calculated the proportion of observations in each of the three vegetation (or ‘habitat’) types defined above. Because proportions are bounded between zero and one, we used beta regression models, fit with the betareg package in R, to test the effect of predator presence on habitat use (Ferrari and Cribari-Neto, 2004; Cribari-Neto and Zeileis, 2010; RCoreTeam, 2019). We confirmed goodness of fit with standard diagnostic plots and pseudo-R2 values. For the proportion of locations in each habitat type, our primary analysis tested the effects of prey species identity and carnivore presence/absence. This approach reflects our focus on hypothesis testing, and specifically on testing whether differences among prey species in response to carnivore presence are general enough for a consistent pattern to emerge across ecosystems and in response to multiple predators. If responses to predation risk are not consistent, they are not likely to systematically interact with competition. We then tested whether these broad responses were modified by predator hunting strategy (stalking, mixed, coursing) or differed among ecosystems, by adding these factors to beta regression models that included prey species identity and carnivore presence. We tested the effect of predator hunting strategy and ecosystem identity with two different models, to avoid partitioning the data so finely that power was too limited to be useful (there were 216 possible combinations of prey species identity, carnivore presence, habitat type, carnivore hunting strategy and ecosystem identity).
3 Results
3.1 Habitat type and predation
Figure 1 shows the proportion of 1,105 kills that occurred in each of the three vegetation categories, confirming that members of this guild were less likely to be killed in cover than in grazing areas. Kill sites were much more common in mixed (beta regression, logit scale b = 2.09 ± 0.49 SE, z = 4.30, P < 0.0001) and grazing (logit scale b = 2.02 ± 0.49 SE, z = 4.12, P < 0.0001) areas than in cover. Zebras were killed far less often than the other species (Table 1), so the proportion of zebra kill sites in each vegetation type were estimated less precisely, but showed the same pattern. Pseudo-R2 values confirmed that including prey species identity (pseudo-R2 = 0.63) had only a small effect on the model’s explanatory power (pseudo-R2 = 0.61).
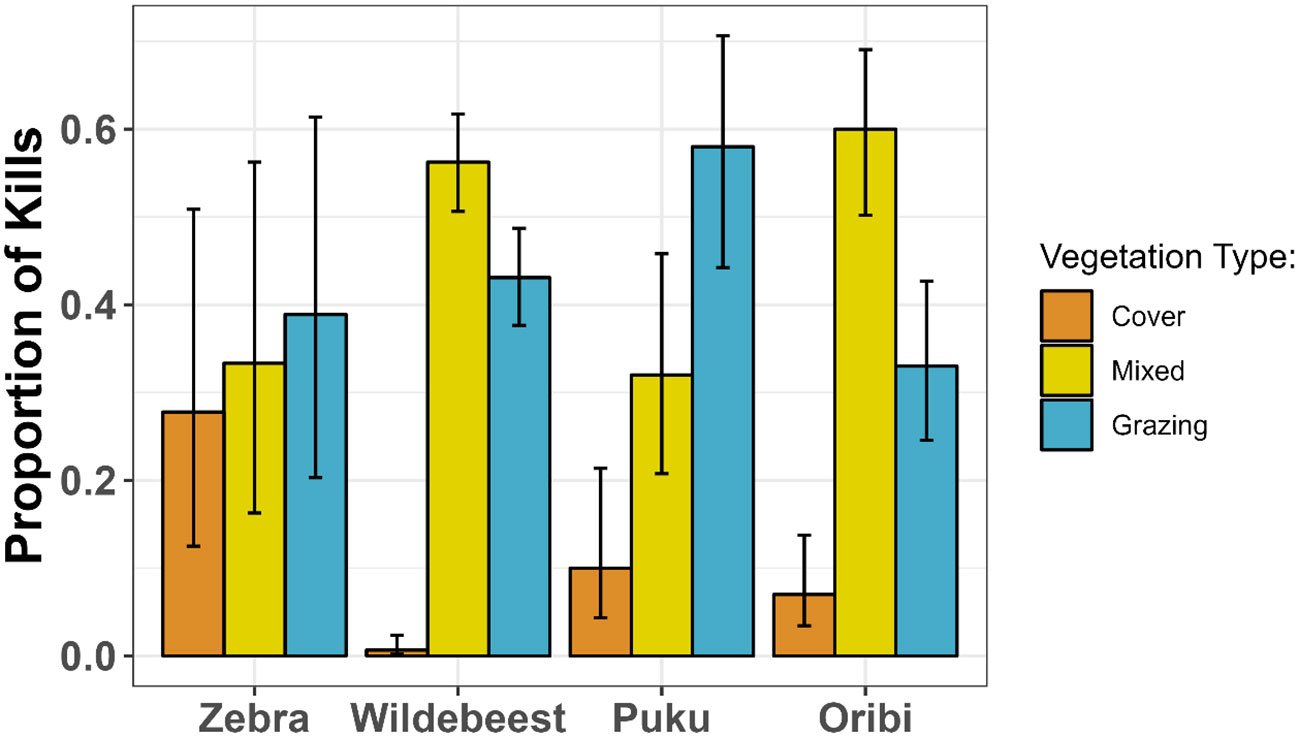
Figure 1 The proportions of 1,105 kills of four grazing prey species that were located in open grazing habitat, cover and mixed habitat. Error bars show 95% binomial confidence intervals using the Wilson method.
3.2 Body size and predation
Table 1 shows the number of kills of each focal prey species by each of the four carnivores. Oribi, the smallest of these grazers, were killed primarily by the two smallest predators, African wild dogs and cheetahs, and were rarely killed by the larger predators, spotted hyenas (twice) and lions (once). Puku, the second smallest prey, were commonly killed by wild dogs and cheetahs, but were rarely killed by spotted hyenas. Puku were commonly killed by lions in Kafue, where depletion of large prey by snaring has shifted lion predation onto small prey species that were rarely killed in the past (Creel et al., 2018). Wildebeest, the second largest prey species, were commonly killed by all of the carnivores, but especially spotted hyenas, which were the second largest predator. Zebras, the largest prey, were killed rarely and primarily by lions, the largest predator. These patterns do not support the hypothesis that smaller grazers are more strongly affected by predation than larger ones (Hopcraft et al., 2012). Instead, they suggest that preferred prey mass is correlated with predator mass, so that intermediate-sized prey are most affected by predation.
3.3 Predator presence and habitat selection
A beta regression model (Figure 2) controlling for differences among prey species (pseudo-R2 = 0.95) showed that the odds of selecting an area with cover increased by 26% when a carnivore was present (logit scale b = 0.24 ± 0.13 SE, z = 1.80, P = 0.07), as would be expected from the patterns of predation shown in Figure 1. This response was strongest for zebras, which selected cover 30% of the time in the absence of a predator (95% binomial CI: 0.20 – 0.43) and 44% of the time in the presence of a predator (95% binomial CI: 0.28 – 0.61) and for puku, which selected cover 38% of the time in the absence of a predator (95% binomial CI: 0.31 – 0.45) and 43% of the time in the presence of a predator (95% binomial CI: 0.35 – 0.50). There was very little change in habitat selection by wildebeest or oribi, which used cover very infrequently regardless of predator presence or absence (Figure 2).
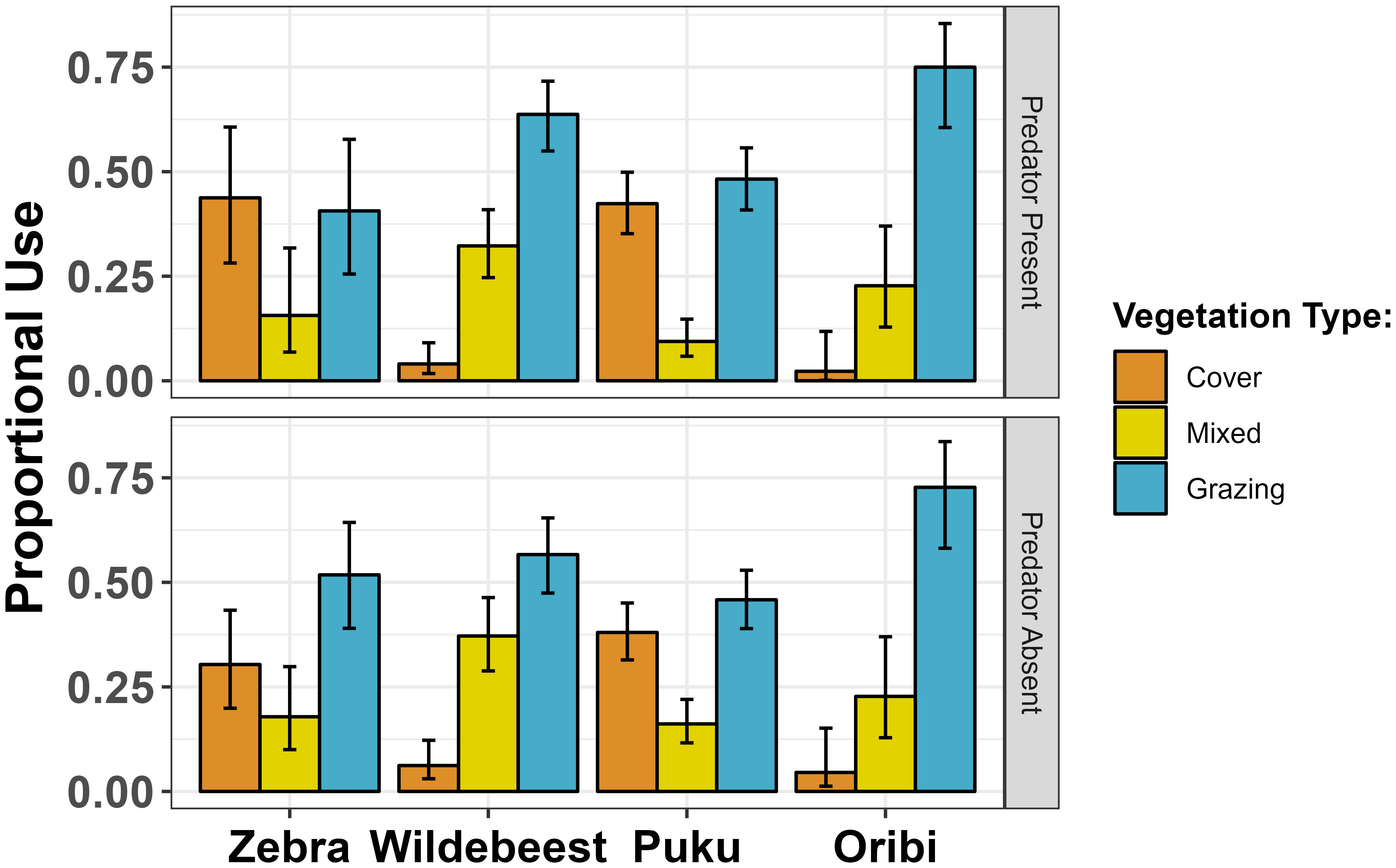
Figure 2 Changes in use open grazing habitat, cover and mixed habitat in the immediate presence (top) and absence (bottom) of a predator. Error bars show 95% binomial confidence limits using the Wilson method.
3.4 Effect of predator hunting mode on prey responses
When the hunting mode of the predator was added to the base model, all of the patterns just described remained similar. As with the prior model, prey were more likely to use cover when a predator was present (logit scale b = 0.46 ± 0.29 SE, z = 1.60, P = 0.11). The differences among prey species followed the same pattern, with zebras and puku using cover more than wildebeest and oribi. While the basic patterns of response remained the same, the strength of responses was affected by the hunting mode of the carnivore that was present. The use of cover was strongest in response to the stalking strategy of lions, weakest in response to the mixed stalking/coursing strategy of cheetahs (difference from lion: logit scale b = -1.24 ± 0.41 SE, z = -3.00, P = 0.003), and intermediate in response to the coursing strategy of spotted hyenas and African wild dogs (difference from lion: logit scale b = -0.82 ± 0.34 SE, z = -2.42 P = 0.015).
3.5 Effect of ecosystem on prey responses
When ecosystem identity was added to the base model, all of the patterns remained similar. Prey were more likely to use cover when a predator was present (logit scale b = 0.95 ± 0.39 SE, z = 2.43, P = 0.015), and the differences among prey species followed the same pattern as in the prior models. While patterns of response to predation risk remained very similar, the use of cover differed appreciably among ecosystems. The Luangwa and Kafue Ecosystems are primarily woodland with patches of grassland, whereas Liuwa is primarily savanna with patches of woodland. As expected from these differences, the use of cover was greater in Kafue (difference from Liuwa: logit scale b = 1.75 ± 0.59 SE, z = 2.90, P = 0.004) and Luangwa (difference from Liuwa: logit scale b = 3.57 ± 0.61 SE, z = 5.88, P = <0.001).
4 Discussion
Our results align well with Bouskila (1995) hypothesis that larger, dominant competitors shift to safe habitats during periods of high risk, causing subordinate competitors to respond weakly or even in the opposite fashion. Across all of the models we examined, grazers tended to shift into cover when predators were present. Zebras, the largest of the prey species, showed the strongest habitat shift even though they were the least frequently killed. Our results did not align with either element of Hopcraft et al. (2012) hypothesis that smaller prey species are more strongly affected by predation and thus should alter habitats in a manner that is more sensitive to predation risk. Although the use of cover differed between ecosystems and in response to predators with different hunting modes, differences between prey species in the strength of their responses remained consistent when these effects were controlled.
Smaller grazers generally require higher quality forage than large ones (Owen-Smith, 1988), and this constraint might make habitat selection by smaller species less sensitive to predation risk. Our data do not allow us to distinguish whether the weak responses of species smaller than zebras were due to foraging constraints or competitive constraints.
Some grazers are well adapted to foraging on early growth stages of grass (Murray and Brown, 1993), and can improve grazing conditions for other species on a time scale of months (McNaughton, 1976; Sinclair, 1985; Arsenault and Owen-Smith, 2002). The opposing effects of facilitation and competition are likely to complicate inferences about interactions with responses to predation risk over long time scales. For example, grazing facilitation might partially explain why large grazers did not alter habitat selection in response to variation in long term risk from lions in Hwange National Park (Valeix et al., 2009). However, the effects of grazing on plant growth cannot directly affect reactive responses to short-term variation in predation risk, such as we observed here. As we build a more complete understanding of interactions between predation risk effects and competition, careful consideration of the distinction between proactive and reactive responses to short-term and long-term variation in risk will be important.
Despite the large number of observations in this study, we had very limited scope to disentangle differences between predator hunting mode and predator species identity. Here, we found stronger habitat shifts in response to stalkers, as has been hypothesized (Preisser et al., 2007; Schmitz, 2008), but other aspects of antipredator response in this guild have shown stronger responses to coursers (Creel et al., 2017). It is plausible that these differences are more directly related predator identity than to the predator’s hunting mode, because lions were the only stalker in our analysis, and zebras, which had the strongest habitat shift in response to predator presence, were killed primarily by lions.
Wildebeest and oribi showed the most consistent use of grazing habitat (>55% overall), and these two species showed no tendency to shift into cover when predators were present. Zebra and puku used mixed habitats and cover more often, and these two species shifted into cover to a greater degree when predators were present. This pattern suggests that factors in addition to competitive dominance affect the strength of habitat shifts in response to predation risk. Species such as impala (Aepyceros melampus) or elk (Cervus elaphus) that can either graze or browse on woody vegetation might be expected to show stronger habitat shifts than the obligate grazers that we examined here (Creel et al., 2005; Valeix et al., 2009). Habitat shifts are just one element in the suite of anti-predator responses by large herbivores, and species that rely more heavily on elements such as vigilance, flight, or dilution of risk might be expected to show weaker habitat shifts.
The low frequency of predation on zebra is probably at least partially be driven by their strong habitat shift in response to risk. Zebras have consistently shown strong responses to predation risk in other ways, including vigilance, foraging and grouping patterns (Thaker et al., 2011; Barnier et al., 2014; Creel et al., 2014; Creel et al., 2017). This pattern reinforces the fundamental point that predation risk and the predation rate are not interchangeable measures: the predation rates that we observe are affected by the responses of prey species on both ecological and evolutionary time scales (Lank and Ydenberg, 2003).
Our results support Bouskila (1995) hypothesis that the competitively subordinate members of a guild may experience higher direct predation rates because their responses to risk are more strongly constrained by competition. Accounting for interactions between predation risk effects and other processes such as competition should help to explain the substantial variation between studies that has been observed. For example, differences between ecosystems in competitor densities and identities might explain why a species responds strongly to risk in one system, but weakly in another.
Data availability statement
The raw data supporting the conclusions of this article will be made available by the authors, without undue reservation.
Ethics statement
The animal study was reviewed and approved by Montana State University Animal Care and Use Committee.
Author contributions
Research design: SC, MB, DC, and PS. Data acquisition: MB, BG, JRdM, ED, JM, ER, TMw, HM, MV, TMu, DS, CS, PS, and TS. Research oversight: TS and CC. Analysis: SC. Writing: SC and MB. All authors contributed to the article and approved the submitted version.
Funding
This research was supported by the National Science Foundation (IOS-1145749, DEB-2032131 and DEB-2221826); National Geographic Society Predator Research Grant & Big Cats Initiative; Dazzle Africa, World Wildlife Fund– Netherlands & Zambia; The Bennink Foundation, Mfuwe Lodge/Bushcamp Company, Tusk Trust, Painted Dog Conservation Inc., Gemfields Inc., Milkywire, Elephant Charge, Robin Pope Safaris, Mulberry Mongoose, Green Safaris, Africa Hope Fund. The authors declare that this study received funding from Mfuwe Lodge/Bushcamp Company Inc., Painted Dog Conservation Inc., Robin Pope Safaris, Mulberry Mongoose and Green Safaris. The funders were not involved in the study design, collection, analysis, interpretation of data, the writing of this article, or the decision to submit it for publication.
Acknowledgments
The findings and conclusions in this article are those of the authors and do not necessarily represent the views of the USFWS.
Conflict of interest
The authors declare that the research was conducted in the absence of any commercial or financial relationships that could be construed as a potential conflict of interest.
The author SC declared that they were an editorial board member of Frontiers, at the time of submission. This had no impact on the peer review process and the final decision.
Publisher’s note
All claims expressed in this article are solely those of the authors and do not necessarily represent those of their affiliated organizations, or those of the publisher, the editors and the reviewers. Any product that may be evaluated in this article, or claim that may be made by its manufacturer, is not guaranteed or endorsed by the publisher.
References
Abramsky Z., Rosenzweig M. L., Pinshow B., Brown J. S., Kotler B., Mitchell W. A. (1990). Habitat selection: an experimental field test with two gerbil species. Ecology 71, 358–2369. doi: 10.2307/1938646
Abramsky Z., Rosenzweig M. L., Subach A. (2002). The costs of apprehensive foraging. Ecology 83, 1330–1340. doi: 10.1890/0012-9658(2002)083[1330:TCOAF]2.0.CO;2
Arsenault R., Owen-Smith N. (2002). Facilitation versus competition in grazing herbivore assemblages. Oikos 97, 313–318. doi: 10.1034/j.1600-0706.2002.970301.x
Barnier F., Valeix M., Duncan P., Chamaille-Jammes S., Barre P., Loveridge A. J., et al. (2014). Diet quality in a wild grazer declines under the threat of an ambush predator. Proc. R. Soc. London B: Biol. Sci. 281, 20140446. doi: 10.1098/rspb.2014.0446
Bouskila A. (1995). Interactions between predation risk and competition: a field study of kangaroo rats and snakes. Ecology 76, 165–178. doi: 10.2307/1940639
Creel S., Becker M., Dröge E., M'soka J., Matandiko W., Rosenblatt E., et al. (2019). What explains variation in the strength of behavioral responses to predation risk? A standardized test with large carnivore and ungulate guilds in three ecosystems. Biol. Conserv. 232, 164–172. doi: 10.1016/j.biocon.2019.02.012
Creel S., Creel N. M. (1996). Limitation of African wild dogs by competition with larger carnivores. Conserv. Biol. 10, 526–538. doi: 10.1046/j.1523-1739.1996.10020526.x
Creel S., Droge E., M'soka J., Smit D., Becker M., Christianson D., et al. (2017). The relationship between direct predation and antipredator responses: a test with multiple predators and multiple prey. Ecology 98, 2081–2092. doi: 10.1002/ecy.1885
Creel S., Matandiko W., Schuette P., Rosenblatt E., Sanguinetti C., Banda K., et al. (2018). Changes in Zambian large carnivore diets over the past half century reveal the loss of large prey. J. Appl. Ecol. 55, 2908–2916. doi: 10.1111/1365-2664.13227
Creel S., Schuette P., Christianson D. (2014). Effects of predation risk on group size, vigilance, and foraging behavior in an African ungulate community. Behav. Ecol. 25, 773–784. doi: 10.1093/beheco/aru050
Creel S., Winnie J., Maxwell B., Hamlin K. L., Creel M. (2005). Elk alter habitat selection as an antipredator response to wolves. Ecology 86, 3387–3397. doi: 10.1890/05-0032
Cribari-Neto F., Zeileis A. (2010). Beta regression in R. J. Stat. Software 34, 1–24. doi: 10.18637/jss.v034.i02
Crowl T. A., Covich A. P. (1990). Predator-induced life-history shifts in a freshwater snail. Science 247, 949–951. doi: 10.1126/science.247.4945.949
Dehn M. M., Ydenberg R. C., Dill L. M. (2017). Experimental addition of cover lowers the perception of danger and increases reproduction in meadow voles (Microtus pennsylvanicus). Can. J. Zool. 95, 463–472. doi: 10.1139/cjz-2016-0169
Dublin H. T., Sinclair A., Boutin S., Anderson E., Jago M., Arcese P. (1990). Does competition regulate ungulate populations? Further evidence from Serengeti, Tanzania. Oecologia 82, 283–288. doi: 10.1007/BF00323546
Durant S. M., Mitchell N., Groom R., Pettorelli N., Ipavec A., Jacobson A. P., et al. (2017). The global decline of cheetah Acinonyx jubatus and what it means for conservation. Proc. Natl. Acad. Sci. 114, 528–533. doi: 10.1073/pnas.1611122114
Ferrari S., Cribari-Neto F. (2004). Beta regression for modelling rates and proportions. J. Appl. Stat. 31, 799–815. doi: 10.1080/0266476042000214501
Fischhoff I. R., Sundaresan S. R., Cordingley J., Rubenstein D. I. (2007). Habitat use and movements of plains zebra (Equus burchelli) in response to predation danger from lions. Behav. Ecol. 18, 725–729. doi: 10.1093/beheco/arm036
Fretwell D. S., Lucas H. L. (1970). On territorial behavior and other factors influencing habitat distribution in birds. Acta Biotheoretica 19, 16–32. doi: 10.1007/BF01601953
Hopcraft J. G. C., Anderson T. M., Pérez-Vila S., Mayemba E., Olff H. (2012). Body size and the division of niche space: food and predation differentially shape the distribution of Serengeti grazers. J. Anim. Ecol. 81, 201–213. doi: 10.1111/j.1365-2656.2011.01885.x
Hopcraft J. G. C., Olff H., Sinclair A. (2010). Herbivores, resources and risks: alternating regulation along primary environmental gradients in savannas. Trends Ecol. Evol. 25, 119–128. doi: 10.1016/j.tree.2009.08.001
Jędrzejewski W., Jędrzejewska B. (1990). Effect of a predator's visit on the spatial distribution of bank voles: experiments with weasels. Can. J. Zool. 68, 660–666. doi: 10.1139/z90-096
Kotler B. P., Brown J. S., Bouskila A. (2004). Apprehension and time allocation in gerbils: The effects of predatory risk and energetic state. Ecology 85, 917–922. doi: 10.1890/03-3002
Lank D. B., Ydenberg R. C. (2003). Death and danger at migratory stopovers: problems with 'predation risk'. J. Avian Biol. 34, 225–228. doi: 10.1034/j.1600-048X.2003.03250.x
Lima S. L. (1998). Nonlethal effects in the ecology of predator-prey interactions. Bioscience 48, 25–34. doi: 10.2307/1313225
Lima S. L. (2009). Predators and the breeding bird: behavioral and reproductive flexibility under the risk of predation. Biol. Rev. 84, 485–513. doi: 10.1111/j.1469-185X.2009.00085.x
Lima S. L., Dill L. M. (1990). Behavioral decisions made under the risk of predation: A review and prospectus. Can. J. Zool. 68, 619–640. doi: 10.1139/z90-092
Losey J. E., Denno R. F. (1998). The escape response of pea aphids to foliar-foraging predators: factors affecting dropping behaviour. Ecol. Entomology 23, 53–61. doi: 10.1046/j.1365-2311.1998.00102.x
McNaughton S. J. (1976). Serengeti migratory wildebeest: facilitation of energy flow by grazing. Science 191, 92–94. doi: 10.1126/science.191.4222.92
Mills M. G. L., Biggs H. C. (1993). “Prey apportionment and related ecological relationships between large carnivores in Kruger National Park,” in Mammals as predators (London: Clarendon Press), 253–268.
Morris D. W. (2003). Toward an ecological synthesis: a case for habitat selection. Oecologia 136, 1–13. doi: 10.1007/s00442-003-1241-4
Murray M. G., Brown D. (1993). Niche separation of grazing ungulates in the Serengeti: an experimental test. J. Anim. Ecol. 62, 380–389. doi: 10.2307/5369
Orrock J. L., Grabowski J. H., Pantel J. H., Peacor S. D., Peckarsky B. L., Sih A., et al. (2008). Consumptive and nonconsumptive effects of predators on metacommunities of competing prey. Ecology 89, 2426–2435. doi: 10.1890/07-1024.1
Owen-Smith R. N. (1988). Megaherbivores: the influence of very large body size on ecology (Cambridge England: Cambridge university press).
Owen-Smith N., Mills M. G. L. (2008). Predator−prey size relationships in an African large−mammal food web. J. Anim. Ecol. 77, 173–183. doi: 10.1111/j.1365-2656.2007.01314.x
Pangle K. L., Peacor S. D., Johannsson O. E. (2007). Large nonlethal effects of an invasive invertebrate predator on zooplankton population growth rate. Ecology 88, 402–412. doi: 10.1890/06-0768
Pansu J., Guyton J. A., Potter A. B., Atkins J. L., Daskin J. H., Wursten B., et al. (2019). Trophic ecology of large herbivores in a reassembling African ecosystem. J. Ecol. 107, 1355–1376. doi: 10.1111/1365-2745.13113
Peckarsky B. L. (1980). Predator-prey interactions between stoneflies and mayflies: behavioral observations. Ecology 61, 932–943. doi: 10.2307/1936762
Peckarsky B. L., Abrams P. A., Bolnick D. I., Dill L. M., Grabowski J. H., Luttbeg B., et al. (2008). Revisiting the classics: considering nonconsumptive effects in textbook examples of predator-prey interactions. Ecology 89, 2416–2425. doi: 10.1890/07-1131.1
Peckarsky B. L., Cowan C. A., Penton M. A., Anderson C. (1993). Sublethal consequences of stream-dwelling predatory stoneflies on mayfly growth and fecundity. Ecology 74, 1836–1846. doi: 10.2307/1939941
Preisser E. L., Orrock J. L., Schmitz O. J. (2007). Predator hunting mode and habitat domain alter nonconsumptive effects in predator-prey interactions. Ecology 88, 2744–2751. doi: 10.1890/07-0260.1
RCoreTeam (2019). R: A language and environment for statistical computing (Vienna, Austria: R Foundation for Statistical Computing).
Schmitz O. J. (2008). Effects of predator hunting mode on grassland ecosystem function. Science 319, 952–954. doi: 10.1126/science.1152355
Schmitz O. J., Beckerman A. P., O'Brien K. M. (1997). Behaviorally mediated trophic cascades: effects of predation risk on food web interactions. Ecology 78, 1388–1399. doi: 10.1890/0012-9658(1997)078[1388:BMTCEO]2.0.CO;2
Sih A., McCarthy T. M. (2002). Prey responses to pulses of risk and safety: testing the risk allocation hypothesis. Anim. Behav. 63, 437–443. doi: 10.1006/anbe.2001.1921
Sinclair A. R. (1985). Does interspecific competition or predation shape the African ungulate community? J. Anim. Ecol. 54, 899–918. doi: 10.2307/4386
Thaker M., Vanak A. T., Owen C. R., Ogden M. B., Niemann S. M., Slotow R. (2011). Minimizing predation risk in a landscape of multiple predators: effects on the spatial distribution of African ungulates. Ecology 92, 398–407. doi: 10.1890/10-0126.1
Valeix M., Fritz H., Dubois S., Kanengoni K., Alleaume S., Said S. (2007). Vegetation structure and ungulate abundance over a period of increasing elephant abundance in Hwange National Park, Zimbabwe. J. Trop. Ecol. 23, 87–93. doi: 10.1017/S0266467406003609
Keywords: predation risk, competition, habitat selection, risk effect, large carnivore, large herbivore
Citation: Creel S, Becker MS, Goodheart B, de Merkle JR, Dröge E, M’soka J, Rosenblatt E, Mweetwa T, Mwape H, Vinks MA, Mukula T, Smit D, Sanguinetti C, Dart C, Christianson D, Schuette P, Simpamba T and Chifunte C (2023) Habitat shifts in response to predation risk are constrained by competition within a grazing guild. Front. Ethol. 2:1231780. doi: 10.3389/fetho.2023.1231780
Received: 30 May 2023; Accepted: 06 July 2023;
Published: 25 July 2023.
Edited by:
Rulon Clark, San Diego State University, United StatesReviewed by:
Zvika Abramsky, Ben-Gurion University of the Negev, IsraelYann Henaut, ECOSUR Conservation and Biodiversity, Mexico
Copyright © 2023 Creel, Becker, Goodheart, de Merkle, Dröge, M’soka, Rosenblatt, Mweetwa, Mwape, Vinks, Mukula, Smit, Sanguinetti, Dart, Christianson, Schuette, Simpamba and Chifunte. This is an open-access article distributed under the terms of the Creative Commons Attribution License (CC BY). The use, distribution or reproduction in other forums is permitted, provided the original author(s) and the copyright owner(s) are credited and that the original publication in this journal is cited, in accordance with accepted academic practice. No use, distribution or reproduction is permitted which does not comply with these terms.
*Correspondence: Scott Creel, c2NyZWVsQG1vbnRhbmEuZWR1