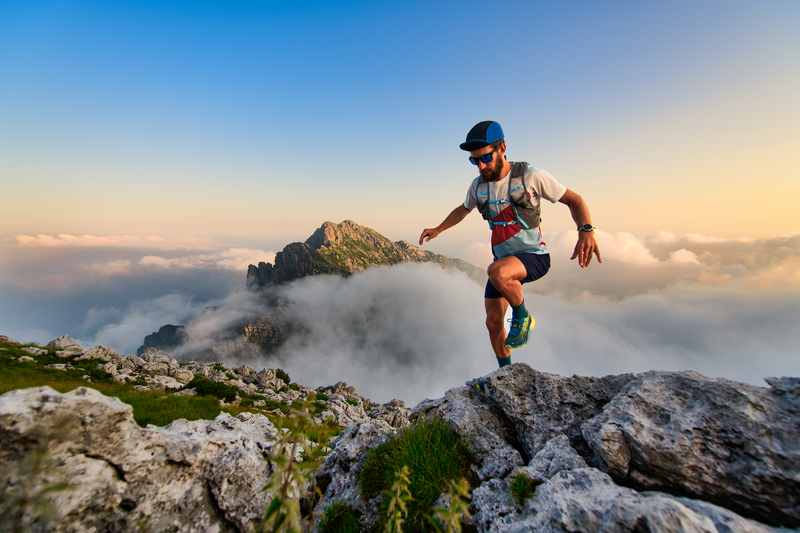
95% of researchers rate our articles as excellent or good
Learn more about the work of our research integrity team to safeguard the quality of each article we publish.
Find out more
ORIGINAL RESEARCH article
Front. Epidemiol. , 13 July 2022
Sec. Occupational and Environmental Epidemiology
Volume 2 - 2022 | https://doi.org/10.3389/fepid.2022.934715
This article is part of the Research Topic Prenatal Chemical Exposures and Child Development View all 6 articles
Introduction: Perfluoroalkyl and polyfluoroalkyl substances (PFAS) are persistent synthetic chemicals found in household products that can cross the placenta during pregnancy. We investigated whether PFAS exposure during pregnancy was associated with infant birth outcomes in a predominantly urban Hispanic population.
Methods: Serum concentrations of perfluorooctanoic acid (PFOA), perfluorooctanesulfonic acid (PFOS), perfluorohexanesulfonic acid (PFHxS), perfluorononanoic acid (PFNA), and perfluorodecanoic acid (PFDA) were measured in 342 prenatal biospecimens (mean gestational age: 21 ± 9 weeks) from participants in the ongoing Maternal And Developmental Risks from Environmental and Social Stressors (MADRES) cohort. PFAS compounds were modeled continuously or categorically, depending on the percentage of samples detected. The birth outcomes assessed were birthweight, gestational age at birth, and birthweight for gestational age (BW-for-GA) z-scores that accounted for parity or infant sex. Single pollutant and multipollutant linear regression models were performed to evaluate associations between PFAS exposures and birth outcomes, adjusting for sociodemographic, perinatal, and study design covariates.
Results: Maternal participants (n = 342) were on average 29 ± 6 years old at study entry and were predominantly Hispanic (76%). Infants were born at a mean of 39 ± 2 weeks of gestation and weighed on average 3,278 ± 522 g. PFOS and PFHxS were detected in 100% of the samples while PFNA, PFOA, and PFDA were detected in 70%, 65%, and 57% of the samples, respectively. PFAS levels were generally lower in this cohort than in comparable cohorts. Women with detected levels of PFOA during pregnancy had infants weighing on average 119.7 g less (95% CI −216.7, −22.7) than women with undetected levels of PFOA in adjusted single pollutant models. PFOA results were also statistically significant in BW-for-GA z-score models that were specific for sex or parity. In models that were mutually adjusted for five detected PFAS compounds, PFOA results remained comparable; however, the association was only significant in BW-for-GA z-scores that were specific for parity (β = −0.3; 95% CI −0.6, −0.01). We found no significant adjusted associations with the remaining PFAS concentrations and the birth outcomes assessed.
Conclusion: Prenatal exposure to PFOA was associated with lower birthweight in infants, suggesting that exposure to these chemicals during critical periods of development might have important implications for children's health.
Perfluoroalkyl and polyfluoroalkyl substances (PFAS) are a class of ubiquitous synthetic chemicals that have bioaccumulation properties with a long half-life and are commonly found in household products due to their water- and stain-resistant qualities. Across the world, these persistent chemicals have been found in humans, marine and land animals, and soil and water within the environment (1). Within this class of chemicals, most research has focused on perfluorooctanoic acid (PFOA) and perfluorooctanesulfonic acid (PFOS); however, there are more than 4,700 compounds that are considered PFAS (2). Animal and in vitro studies have found that PFOA and PFOS have a toxic effect on the liver, kidney, reproductive system, cardiovascular system, and endocrine system (3–6). Prenatal exposure to PFOA and PFOS has also consistently led to lower birthweight and offspring mortality in mice and rats (7, 8). Although PFOA, PFOS, and PFAS with similar chemical structures have been phased out of manufacturing in the USA (9), products containing them can still be imported. In addition, due to their long half-lives [PFOA 4 years and PFOS 5 years (10)], the substances are still commonly found in the blood serum of the US population, even in children born after the phase-out in 2002 (11, 12). A wide range of items contain PFAS, such as nonstick cookware, shampoos, waterproof clothing, fire-fighting foam, electronics, and fast-food packaging (2, 13, 14). The most common exposure routes of PFAS to humans include ingestion through drinking water or contaminated food (i.e., fish or food stored in PFAS-containing packaging transferred into food), inhalation of indoor air and dust, and occupational exposure through inhalation or dermal contact (14–19).
Exposure to these substances may be particularly critical during fetal development due to their ability to cross the placenta during pregnancy (20). The placenta has been shown to be vulnerable to environmental insults, to be a common target of PFAS, and to accumulate across gestation via the maternal circulation (20–22). No consensus has been reached on the exact biological mechanism by which PFAS influence fetal growth; however, proposed hypotheses include impacts on bone development, maternal hormone disruption—particularly sex and thyroid hormones—and adverse effects on placental development and function (23–30). Several recent meta-analyses and systematic literature reviews suggest that prenatal exposure to PFOA and PFOS is associated with lower infant birthweight (8, 31–34). However, many individual studies have failed to detect significant associations. Additionally, several biases have been discussed, especially for studies that measure concentrations late in pregnancy or at birth, due to biological and physiological changes that occur in later pregnancy, such as increased glomerular filtration (33).
Epidemiological studies have also found statistically significant associations between some PFAS compounds, such as perfluorohexanesulfonic acid (PFHxS), perfluorononanoic acid (PFNA), and perfluorodecanoic acid (PFDA) with infant birthweight (35–40); however, these relationships have been assessed in far fewer studies than in studies with PFOA and PFOS. Many recently published studies analyzing these associations used specimens collected in the early 2000s, which likely do not represent current exposure levels due to the phase outs and use of replacement compounds that have occurred in recent decades (37, 38, 40, 41).
A better understanding of the association between prenatal PFAS exposure, particularly compounds other than PFOA and PFOS, and infant birthweight is important as low birthweight, defined as <2,500 g, is a strong predictor of childhood obesity and subsequent metabolic health morbidities, such as type 2 diabetes later in life (42–44). In the USA, pregnant persons who identify as Black or Hispanic have a higher rate of infants born with low birthweight compared to their non-Hispanic white counterparts (45). PFAS concentration levels also differ by race and ethnicity. The National Health and Nutrition Examination Survey (NHANES) found that non-Hispanic whites have higher concentration levels with reference to Blacks or Hispanics (11). However, few epidemiologic studies have investigated the influence of PFAS exposure on birth outcomes among racially and ethnically diverse populations, specifically within Hispanic populations.
The objective of this study was to determine if maternal serum PFAS concentrations measured in pregnancy were associated with infant birthweight and birthweight for gestational age (BW-for-GA) z-scores in a prospective pregnancy cohort representing a structurally marginalized population. Additionally, we examined the relationship between prenatal PFAS serum concentrations and gestational age at birth.
Participants included in this study were drawn from the ongoing Maternal And Developmental Risks from Environmental and Social Stressors (MADRES) pregnancy cohort, which is predominately comprised of Hispanic participants and their children residing in urban Los Angeles, CA, USA. Methods of the MADRES study and protocol have been described previously (46). Briefly, participants were enrolled during pregnancy at four prenatal clinic sites. These included two community health clinics, one county hospital prenatal clinic, and one private obstetrics and gynecology practice. Eligibility for participants at the time of recruitment included: (1) <30 weeks pregnant (2) at least 18 years of age; and (3) being fluent in English or Spanish. Exclusion criteria for the study included: (1) multiple gestation; (2) having a physical, mental, or cognitive disability that would prevent participation or ability to provide consent; (3) being currently incarcerated; or (4) HIV positive status. At study entry, informed consent and The Health Insurance Portability and Accountability Act (HIPAA) authorization were obtained for medical record abstraction from all participants. The University of Southern California's Institutional Review Board approved all study procedures.
Blood serum samples were collected from 359 MADRES participants between December 2015 and February 2019, and PFAS concentrations were measured in Spring 2019. Of the 359 participants with PFAS measurements, 347 participants had available data on infant birthweight. We removed five participants who withdrew from the study prior to birth (two study dropouts and three miscarriages) as well as seven participants whose infants lacked birthweight. Additionally, five participants were removed due to missing race/ethnicity. A total of 342 mother–infant dyads with PFAS analyte concentrations, birth outcomes data, and key covariate information were included in the current study.
Blood samples were collected during pregnancy using red top 10-ml serum tubes at an in-person study visit (median gestational age: 19.1 weeks; range 5.7–38.3 weeks). Samples were processed, and serum was aliquoted into appropriately labeled 0.5-ml cryovials before storage in a −80°C freezer prior to shipment to the analytical laboratory.
Samples were sent to the Wadsworth Center's Human Health Exposure Assessment Resource (WC-HHEAR) laboratory at the NYU Langone Medical Center (Dr. Kannan's laboratory) for analysis. A total of 14 PFAS, namely, PFHxS, PFOS, PFOA, PFNA, PFDA, perfluorobutanesulfonic acid (PFBS), perfluoroheptanoic acid (PFHPA), perfluroundecanoic acid (PFUNDA), perfluorododecanoic (PFDODA), perfluorooctanesulfonamide (PFOSA), n-ethyl perfluorooctane sulfonamido acetic acid (NETFOSAA), n-methyl perfluorooctane sulfonamido acetic acid (NMFOSAA), perfluoro-n-pentanoic acid (PFPEA), and perfluorohexanoic acid (PFHxA) were analyzed. The method for the analysis of 14 PFAS in serum has been described elsewhere (47). In brief, serum samples (0.25 ml) were aliquoted into 15-ml polypropylene (PP) tubes and spiked with 5 ng of 13C-labeled internal standard (IS) mixture and 0.7 ml of 1% ammonium formate (w/v) in methanol (MeOH). The mixture was centrifuged for 5 min at 5,000 rpm, and the supernatant was collected and passed through the Hybrid-SPE cartridge (Phospholipid, 30 mg, 1 cc, Sigma-Aldrich, St. Louis, MO, USA). Cartridges were conditioned with 1 ml of MeOH containing 1% ammonium formate (w/v). Samples were eluted through the cartridge and collected in a PP tube for the LC-MS/MS analysis.
Target analytes were quantified by the isotopic dilution method, and a 12-point calibration (at concentrations ranging from 0.02 to 100 ng/ml) with the regression coefficient of ≥ 0.999 was used. A pure solvent (MeOH) and a mid-point calibration standard (5 ng/ml) were injected after every 10 samples to check for the carryover of target chemicals and instrumental drift in sensitivity. Several procedural blanks were analyzed to monitor for contamination that potentially arise from reagents and materials used in sample preparation steps. For each batch of 100 samples, five duplicates of procedural blanks and QC spiked samples (water spiked with native standards at 5 ng for all analytes and IS) were processed. Duplicates of Standard Reference Material (SRM1958, NIST, Gaithersburg, MD, USA; IS spiked) containing certified values for PFHxS, PFOS, PFOA, and PFNA were analyzed. Trace levels of NETFOSAA (0.001–0.047 ng/ml) and NMFOSAA (0.002–0.012 ng/ml) were found in procedural blanks, and the concentrations of these chemicals in samples were subtracted from blank values. Spiked sample and SRM1958 recoveries were in the range of 81.5–112% [Relative standard deviation (RSD): ±4.3–8.7%] and 86.7–111% (RSD: ±2.1–6.8%), respectively. The limit of detection (LOD) of target analytes ranged from 0.02 to 0.05 ng/ml. Five of the 14 PFAS were detected in at least 50% of MADRES participant samples and were included in subsequent data analysis. These included PFOS (100% detected), PFHxS (100% detected), PFNA (70% detected), PFOA (65% detected), and PFDA (57% detected).
Infant birthweight was directly abstracted from electronic medical records (EMR) for all but one participant (99.7%). The remaining participant did not have a physician measurement of birthweight recorded on the EMR, and birthweight was obtained from the participant via an interviewer-administered questionnaire with a MADRES staff member 7–14 days post-birth. Gestational age at birth was calculated and standardized using a hierarchy of methods (48). A first trimester (<14 weeks of gestation) ultrasound measurement of crown-rump length was deemed ideal and was used if available (59.7%). If unavailable, a second trimester (<28 weeks of gestation) ultrasound measurement of fetal biparietal diameter was used (26.9%). If measurements from an early ultrasound were unavailable, gestational age at birth was calculated based on a physician's best clinical estimate from EMR (13.2%). If none of the above mentioned measurements were available, gestational age was calculated from the estimated last menstrual period (<1%). We evaluated the birthweight by modeling it continuously as well as by calculating sex-specific or parity specific BW-for-GW z-scores based on Aris et al. (49). These z-scores reflect the current sociodemographic composition of the USA using 2017 US natality files from obstetric estimated gestational age on singleton births, which replaced previous BW-for-GA z-scores estimated using self-reported last menstrual period (49).
Potential covariates were identified a priori from the literature and included participant demographics, aspects related to study design, and pregnancy- and birth-related variables. Study design variables were the study recruitment site and gestational age at the time of blood serum collection.
Participant demographic covariates included race/ethnicity, country of birth, the highest attained education, annual household income, participant's age at the time of study enrollment, any reported personal smoking during pregnancy, and pre-pregnancy body mass index (BMI: kg/m2). These variables were self-reported via interviewer-administered questionnaires in English or Spanish. Pre-pregnancy BMI was calculated using self-reported pre-pregnancy weight and standing height at the first study visit (<30 weeks of gestation) measured by a study staff member via a stadiometer (perspectives enterprises model PE-AIM-101).
Pregnancy-related covariates included parity, which was self-reported via an interviewer-administered questionnaire at the first study visit, infant sex abstracted from EMR (96.8%) or self-reported from the participant (3.2%), and the best available gestational age at the time of birth for continuous birthweight models. In addition, information on maternal seafood consumption during the pregnancy was obtained via an interviewer-administered questionnaire in English or Spanish during the third trimester. Participants were asked if they had ever consumed any of the following types of seafood during the pregnancy: fish sticks, fresh oily fish, other fresh fish, canned tuna, shellfish, or fried shellfish. A combined variable was then created with four categories: never (62.6%), monthly (16.1%), at least weekly (12.3%), or unknown fish consumption status (9.0%).
DAGitty was used to create a directed acyclic graph (DAG) using the above mentioned covariates to assess relationships (Supplementary Figure S1) (50). Minimal sufficient adjustment sets for estimating the total effect of prenatal PFAS exposure on birthweight were child's sex, country of birth, fish consumption, gestational age at the time of blood sampling, household income, maternal age, maternal education, parity, race/ethnicity, and pre-pregnancy BMI. Continuous birthweight models were adjusted for child's sex (male, female), country of birth (USA, other, missing indicator), fish consumption (never, monthly, at least weekly, missing indicator), gestational age at birth (weeks), gestational age at the time of blood sampling (weeks), household income (<30 k, 30–99 k, >100 k, or reported “Do Not Know”), maternal education (high school or less, some/completed college, or some graduate training), parity (first born, second or more born, missing indicator), race/ethnicity (Hispanic, non-Hispanic white, non-Hispanic Black, non-Hispanic other), pre-pregnancy BMI (kg/m2), maternal age at study recruitment (years), and recruitment site. BW-for-GA z-score models included the same covariates except for gestational age at birth. Sex specific BW-for-GA z-score models removed infant sex while parity-specific BW-for-GA z-score models removed parity.
Bivariate analyses were conducted with covariates to assess their relationships with the PFAS compounds ran continuously as well as with birthweight and gestational age at birth. Due to the right skewed distributions of the PFAS compounds, Kruskal–Wallis one way analysis of variance (ANOVA) tests were used to assess relationships with categorical variables including education, household income, country of birth, race/ethnicity, parity, fish consumption, and recruitment site. Spearman correlations were performed for associations between PFAS compounds and continuous variables including participant's age, pre-pregnancy BMI, and gestational age at the time of blood serum collection. Spearman correlations were also used to assess the correlation between the five compounds. For PFAS samples that were below the LOD, values were imputed using the equation of LOD/2 (51).
We used multiple linear regression models to assess the relationships between PFAS concentrations and birthweight and BW-for-GA z-scores that were parity or sex specific. Depending on the percentage of the samples detected for each PFAS compound, we either modeled the exposure as a continuous exposure or categorical exposure (e.g., detected/non-detected or quartiles of exposure). Because PFHxS and PFOS were detected in 100% of samples, we log transformed these exposures and modeled them on the continuous scale. We also modeled these two compounds categorically by quartiles. PFOA, PFNA, and PFDA were detected in 57–70% of samples; therefore, we modeled these exposures categorically (detected vs. non-detected). Additionally, multipollutant models were carried out to mutually for the five PFAS. Single pollutant linear regression models and multipollutant models had no evidence of multicollinearity based on the variance inflation factor (VIF) (all individual variables VIF < 10, mean VIF < 2), and no influential points were identified (Cook's D < 1). Modeling assumptions for linear regression were checked and met for all models.
A statistical interaction between each PFAS compound and infant sex was tested within single pollutant models, and models were stratified by infant sex to assess for effect modification. A sensitivity analysis was carried out by excluding participants who had gestational diabetes mellitus (GDM) (n = 33), gestational hypertension (n = 26), or preeclampsia/eclampsia (n = 25) recorded on their medical record, although some women overlapped with GDM and a gestational hypertension disorder (n = 3). Lastly, an additional sensitivity analysis was carried out where models were restricted to only participants who had prenatal PFAS concentrations measured in blood samples prior to 30 weeks of gestation (n = 239).
Adjusted linear regression models were also performed to assess the relationship between prenatal PFAS blood serum and gestational age at birth to investigate whether gestational age was a biological intermediate between prenatal PFAS and infant birthweight (31, 52).
The significance level for all analyses was set at an alpha of 0.05, and all data were analyzed using SAS version 9.4 (SAS Institute, Inc., Cary, NC, USA).
Participant and infant characteristics are reported in Table 1. Participants were on average 29 ± 6 years old at study recruitment, 76% were Hispanic, the majority (53%) had a high school diploma or less and had a mean pre-pregnancy BMI of 28 ± 6 kg/m2. Their infants were born at a mean of 39 ± 2 weeks of gestation and weighed on average 3,278 ± 522 g at birth. The majority of infants were at least the second born child (62%). Maternal smoking during any point in pregnancy was low in this population (n = 6).
Five PFAS analytes had > 50% of samples above the LOD (PFOS 100%, PFHxS 100%, PFNA 70%, PFOA 65%, and PFDA 57%). The remaining analytes had 17% or less of samples that were above the LOD and were excluded from this analysis. The median concentrations were the highest for PFOS (1.34 ng/ml) followed by PFHxS (1.09 ng/ml). Distributions for all measured analytes are shown in Table 2. The natural log-transformed PFAS concentrations were also significantly positively correlated with each other and are shown in Figure 1 (Spearman's R = 0.21 to 0.78, p < 0.0001 for all correlations). PFAS concentration levels seen within the MADRES cohort, with the exception of PFHxS, were lower than the levels reported in comparable cohorts (53–55).
Table 2. Distribution of perfluoroalkyl and polyfluoroalkyl substances (PFAS) (ng/ml) concentrations in maternal blood serum (n = 342).
Figure 1. Spearman correlation coefficients for natural log transformed PFAS (ng/mL) in maternal blood serum (N = 342). p < 0.0001 for all correlations.
Median levels of PFAS by demographic characteristics are shown in Supplementary Table S1. Significant differences were observed in median PFOA, PFNA, PFHxS, and PFOS by education levels, and participants with at least some graduate training had the highest levels. These trends were consistent with household income with median PFOA, PFNA, PFHxS, and PFOS differing across income bracket and those making at least $100,000 annually having the highest levels. Significant differences in median PFOA, PFNA, PFHxS, and PFOS were observed by maternal race with non-Hispanic white participants having the highest levels. Additionally, there were significant differences in median PFOA, PFDA, PFNA, PFHxS, and PFOS by the parity status with participants pregnant with their first child having the highest levels. Median PFNA, PFHxS, and PFOS significantly differed in fish consumption habits during pregnancy with those who consumed fish at least weekly having the highest levels. Significant differences in median PFOS and PFHxS were observed by country of birth, with participants born outside of the USA having lower levels. All PFAS concentrations were significantly inversely associated with pre-pregnancy BMI (Spearman's R = −0.29 to −0.13, p < 0.02 for all correlations) while no relationships were observed with PFAS and maternal age (p > 0.14 for all correlations).
No significant associations were observed with any PFAS compound and gestational age at birth in univariate or adjusted models (Supplementary Table S2). Therefore, we concluded that gestational age was not acting as a mediator of the association between prenatal PFAS exposure and birthweight within the MADRES cohort. We included gestational age at birth as a covariate in continuous birthweight models. The results for single prenatal PFAS exposures with continuous infant birthweight and BW-for-GA z-scores that were parity or sex specific are shown in Table 3. Women with detected levels of PFOA (β = −119.7 g; 95% CI −216.7, −22.7) and PFNA (β = −105.8 g; 95% CI −206.3, −5.3) had infants with significantly lower birthweight on average compared to women without detected levels. No significant associations were seen with the remaining PFAS compounds and infant birthweight, although non-significant inverse relationships were seen with PFOS and PFDA and a non-significant positive relationship was seen with PFHxS. The results for all PFAS were similar in BW-for-GA z-score models compared to continuous birthweight models, although only PFOA remained significant in models with sex-specific BW-for-GA z-scores (β = −0.3; 95% CI −0.5, −0.1) and parity-specific BW-for-GA z-scores (β = −0.3; 95% CI −0.5, −0.1). No evidence was found for a statistical interaction between any PFAS compound and infant sex (all interaction p > 0.29), and there were no significant differences in stratified models by sex (data not shown).
Table 3. Adjusted single PFAS exposure models with continuous birthweight and sex- or parity-specific birthweight for gestational age (BW-for-GA) z-scores (n = 342).
In models that were mutually adjusted for all five PFAS compounds, the effect estimate was slightly attenuated from independent models for the detected/non-detected PFOA and continuous birthweight and the detected/non-detected PFOA was no longer statistically significant (β = −108.8 g; 95% CI −229.9, 12.3). In contrast, the effect estimates for the detected/non-detected PFOA and parity-specific BW-for-GA z-scores and sex-specific BW-for-GA z-scores remained unchanged from single pollutant to multipollutant models, although statistical significance was only reached in the parity-specific model (β= −0.3; 95% CI −0.6, −0.01). PFNA, PFDA, PFOS, and PFHxS were not associated with any birthweight measure in multipollutant models. The results are shown in Table 4.
Table 4. Linear regression model results for five PFAS compounds simultaneously on continuous infant birthweight and sex– or parity–specific BW–for–GA z–scores (n, 342).
In models that were restricted to participants without GDM, gestational hypertension, or preeclampsia/eclampsia recorded on their medical records (n = 261), estimates were comparable to both single pollutant and multipollutant models with the full sample (Supplementary Table S3). We also found that restricting to women with PFAS concentrations measured prior to 30 weeks of gestation (n = 239) did not materially change our results (Supplementary Table S4).
We found that women with detectable levels of PFOA during pregnancy had infants who weighed on average 120 g less than women without detectable levels of PFOA. Within models that were mutually adjusted for five detected PFAS compounds, the effect size for the associations of birthweight with PFOA remained comparable. We did not find significant adjusted associations with the remaining PFAS concentrations and the birth outcomes assessed. Additionally, many PFAS compounds analyzed within the MADRES cohort had limited to no detection.
In the MADRES cohort, median PFNA, PFOA, PFOS, and PFDA concentrations were lower in relation to several other pregnancy cohorts within Asian, European, and North American countries, although this trend was not consistent with PFHxS, which was often comparable or had higher concentration levels (38, 40, 53–55). Although we do not know why PFHxS concentrations were higher in this cohort, PFHxS did have one of the longest half-lives of PFAS compounds (5–9 years) (10, 56). The highest levels of PFAS concentrations were found in participants who were pregnant with their first-born child. This is consistent with the prior literature (57–62), as breastfeeding has been shown to be a probable excretion route for PFAS (59, 61). Prior studies have also reported higher levels of PFAS within non-Hispanic white women, although research is scarce for comparisons to Hispanic women outside NHANES. PFOA and PFHxS have been seen to be higher in non-Hispanic white women compared to Black/African-American women (63) as well as to Chinese-American and Japanese-American women (64). These results are surprising given that communities of color are disproportionately burdened by environmental chemical exposures including hazardous waste sites, air pollution, and water pollution (65). PFAS exposure sources related to race and ethnicity merit further examination.
The observation that higher education levels and annual household income were associated with higher PFAS levels within MADRES is also consistent with previous studies (58, 66, 67). Foreign-born participants having lower levels of PFOS and PFHxS as seen in this study is consistent with a previous study of middle-aged women, which found that within Chinese and Japanese women, those born outside of the USA had lower PFAS concentrations compared to those born within the USA (64).
Our findings that PFAS levels were inversely related with pre-pregnancy BMI are more complex. A previous study in Norway consisting of white and primarily of normal weight women showed prenatal PFHxS levels to be significantly positively associated with pre-pregnancy BMI (66), which is contrary to our findings. We found that PFAS levels were higher among women who reported higher intakes of seafood. This is consistent with a study among Norwegian women in which unadjusted PFAS serum levels were associated with increased dietary intakes of shellfish and oily fish (66).
The majority of studies on prenatal PFAS exposure and infant birthweight have investigated individual PFAS exposures. Steenland et al. found prenatal PFOA measured in maternal or cord blood to be associated with a decrease of 10.5 g (95% CI −16.7, −4.4) in infant birthweight/ng/ml increase in PFOA in the primary random effect meta-analysis (33). Starling et al. found that significant results are similar to those reported in this current study in 628 mother–infant pairs within the Healthy Start Study. They found that women in the highest tertile of mid-pregnancy measured PFOA exposure had infants who weighed significantly less compared to those in the lowest tertile of PFOA exposure (β = −92.4 g; 95% CI −166.2, −18.5) (55). A recent study found that prenatal PFOA measured in the first trimester serum were significantly associated with decreased infant birthweight in 1,533 participants from the Swedish Environmental, Longitudinal, Mother and child, Asthma and allergy (SELMA) study. Mothers in the highest quartile of PFOA exposure had infants who weighed 90 g less (95% CI −159, −91) compared to those in the lowest quartile (37).
In this present study, we observed no consistent significant findings between PFDA, PFNA, PFOS, and PFHxS and infant birthweight. However, we found that non-significant inverse associations was observed for prenatal PFNA, PFDA, and PFOS with birthweight and a non-significant positive association was observed with PFHxS and birthweight. Previous studies have shown significant inverse relationships between PFOS exposure and birthweight in a subset of several studies (68–72), and three other studies have found non-significant positive relationships with PFHxS and birthweight (35, 38, 70). Few studies have examined relationships between PFNA and PFDA with birthweight, although significant inverse relationships have been observed (35, 37–40). Our study did not find any significant results with PFAS and gestational age at birth and, therefore, gestational age was not considered to be a likely mediator of the association of PFAS on infant birthweight (31, 52). Several other studies have also not found significant associations between prenatal PFAS exposure and gestational age at birth (41, 53, 73).
Many important strengths are found within the current study. First, multiple PFAS exposures were analyzed with the same panel and, in particular, we had measurements of PFDA, PFNA, and PFHxS, which have not been as comprehensively studied as PFOA and PFOS. Second, PFAS concentrations were measured in maternal blood serum, which is a superior biospecimen to whole blood or urine, with the serum to whole blood ratio for PFAS being 2:1 (74, 75). Third, our study population is comprised of predominately low-income Hispanic participants, which is an understudied group. Additionally, given that pregnant persons are exposed to more than one compound at once, we also assessed associations within a multipollutant framework and found that PFOA was consistently associated with birthweight. Lastly, the prospective cohort design and high quality covariate data are additional strengths.
The limitations of this study include only one measurement of PFAS concentrations, and PFAS were measured in the serum collected across a wide range of gestational age. For these reasons, this analysis was unable to determine critical windows of exposure of PFAS on infant birthweight. As such, while we did control for gestational age at the time of collection, we assume that PFAS levels are relatively stable across pregnancy due to their long half-lives. A previous study with PFOA and PFOS blood serum levels taken from the first and second trimester found a high degree of correlation between the two time points, but the averages were lower in the second trimester (69). An additional study with blood samples taken at all three trimesters of pregnancy found PFOA, PFOS, PFNA, and PFDA to slightly decrease over pregnancy while PFHxS levels remained consistent (35). We were unable to confirm the PFAS levels at birth measured in cord blood within this study. We also did not have available information on the glomerular filtration rate (GFR), which can influence both PFAS levels later in pregnancy and infant birthweight (76) as GFR peaks during 30–35 weeks of gestation (77). In our study, we conducted a sensitivity analysis limiting our analysis to samples collected prior to 30 weeks of gestation and we found no measurable impact on our effect estimates. Additionally, although many key covariates were adjusted for final models, residual confounding is still possible, as in all observational studies.
Prenatal exposure to PFOA was associated with lower birthweight in infants in the MADRES cohort in Los Angeles, CA. These findings suggest that exposure to these chemicals during in utero development may have important implications for children's health. While we found the highest exposures to PFAS among non-Hispanic white women as well as women with higher household incomes and higher attained education levels in our cohort, future studies should focus on how mixtures of newer PFAS, especially those that have become substitutes for legacy PFAS including PFOA and PFOS, impact populations facing disparities in birth and other health outcomes.
The original contributions presented in the study are included in the article/Supplementary Materials, further inquiries can be directed to the corresponding author.
The studies involving human participants were reviewed and approved by University of Southern California Institutional Review Board (IRB). The patients/participants provided their written informed consent to participate in this study.
Conceptualization: CB, TB, and AP. Data curation: AP, DF, KK, MR, and TY. Formal analysis, validation, visualization, and writing—original draft: AP. Funding acquisition, supervision, investigation, resources, and project administration: CB and TB. Methodology: BG, CB, DW, EG, LA-M, RH, SF, SE, and TB. Software: AP and TY. Writing—review and editing: AP, SE, RH, TY, DF, SF, BG, KK, MR, DL, LA-M, DW, EG, TB, and CB. All authors contributed to the article and approved the submitted version.
This work was supported by the MADRES Center (Grant Nos. P50ES026086, 83615801, and P50MD015705) funded by the National Institute of Environmental Health Sciences, the National Institute for Minority Health and Health Disparities and the Environmental Protection Agency; the Southern California Environmental Health Sciences Center (Grant No. 5P30ES007048) funded by the National Institute of Environmental Health Sciences, and the Life course Approach to Developmental Repercussions of Environmental Agents on Metabolic and Respiratory health (LA DREAMERs) (Grant No. UH3OD023287) funded by the National Institutes of Health Office of the Director ECHO Program. The funding agencies had no role in the design of the study, the collection, analysis, or interpretation of data or in the writing of the manuscript.
The authors declare that the research was conducted in the absence of any commercial or financial relationships that could be construed as a potential conflict of interest.
All claims expressed in this article are solely those of the authors and do not necessarily represent those of their affiliated organizations, or those of the publisher, the editors and the reviewers. Any product that may be evaluated in this article, or claim that may be made by its manufacturer, is not guaranteed or endorsed by the publisher.
We would like to thank the MADRES participants, our community clinic partners, and our MADRES study team.
The Supplementary Material for this article can be found online at: https://www.frontiersin.org/articles/10.3389/fepid.2022.934715/full#supplementary-material
1. Houde M, De Silva AO, Muir DC, Letcher RJ. Monitoring of perfluorinated compounds in aquatic biota: an updated review. Environ Sci Technol. (2011) 45:7962–73. doi: 10.1021/es104326w
2. Sinclair GM, Long SM, Jones OAH. What are the effects of PFAS exposure at environmentally relevant concentrations? Chemosphere. (2020) 258:127340. doi: 10.1016/j.chemosphere.2020.127340
3. Bassler J, Ducatman A, Elliott M, Wen S, Wahlang B, Barnett J, et al. Environmental perfluoroalkyl acid exposures are associated with liver disease characterized by apoptosis and altered serum adipocytokines. Environ Pollut. (2019) 247:1055–63. doi: 10.1016/j.envpol.2019.01.064
4. Chen J, Zheng L, Tian L, Wang N, Lei L, Wang Y, et al. Chronic PFOS exposure disrupts thyroid structure and function in zebrafish. Bull Environ Contam Toxicol. (2018) 101:75–9. doi: 10.1007/s00128-018-2359-8
5. Chou H-C, Wen L-L, Chang C-C, Lin C-Y, Jin L, Juan S-H. From the cover: l-carnitine via PPARγ-and Sirt1-dependent mechanisms attenuates epithelial-mesenchymal transition and renal fibrosis caused by perfluorooctanesulfonate. Toxicolog Sci. (2017) 160:217–29. doi: 10.1093/toxsci/kfx183
6. Zeng Z, Song B, Xiao R, Zeng G, Gong J, Chen M, et al. Assessing the human health risks of perfluorooctane sulfonate by in vivo and in vitro studies. Environ Int. (2019) 126:598–610. doi: 10.1016/j.envint.2019.03.002
7. Koustas E, Lam J, Sutton P, Johnson PI, Atchley DS, Sen S, et al. The Navigation Guide - evidence-based medicine meets environmental health: systematic review of nonhuman evidence for PFOA effects on fetal growth. Environ Health Perspect. (2014) 122:1015–27. doi: 10.1289/ehp.1307177
8. Negri E, Metruccio F, Guercio V, Tosti L, Benfenati E, Bonzi R, et al. Exposure to PFOA and PFOS and fetal growth: a critical merging of toxicological and epidemiological data. Crit Rev Toxicol. (2017) 47:489–515. doi: 10.1080/10408444.2016.1271972
9. EPA U. 2010/2015 PFOA Stewardship Program. Washington, DC: United States Environmental Protection Agency (2006).
10. Olsen GW, Burris JM, Ehresman DJ, Froehlich JW, Seacat AM, Butenhoff JL, et al. Half-life of serum elimination of perfluorooctanesulfonate,perfluorohexanesulfonate, and perfluorooctanoate in retired fluorochemical production workers. Environ Health Perspect. (2007) 115:1298–305. doi: 10.1289/ehp.10009
11. Calafat AM, Wong LY, Kuklenyik Z, Reidy JA, Needham LL. Polyfluoroalkyl chemicals in the U.S. population: data from the National Health and Nutrition Examination Survey (NHANES) 2003-2004 and comparisons with NHANES 1999-2000. Environ Health Perspect. (2007) 115:1596–602. doi: 10.1289/ehp.10598
12. Ye X, Kato K, Wong L-Y, Jia T, Kalathil A, Latremouille J, et al. Per-and polyfluoroalkyl substances in sera from children 3 to 11 years of age participating in the National Health and Nutrition Examination Survey 2013–2014. Int J Hyg Environ Health. (2018) 221:9–16. doi: 10.1016/j.ijheh.2017.09.011
13. Dauchy X, Boiteux V, Bach C, Rosin C, Munoz J-F. Per-and polyfluoroalkyl substances in firefighting foam concentrates and water samples collected near sites impacted by the use of these foams. Chemosphere. (2017) 183:53–61. doi: 10.1016/j.chemosphere.2017.05.056
14. Schaider LA, Balan SA, Blum A, Andrews DQ, Strynar MJ, Dickinson ME, et al. Fluorinated Compounds in U.S fast food packaging. Environ Sci Technol Lett. (2017) 4:105–11. doi: 10.1021/acs.estlett.6b00435
15. Fair PA, Wolf B, White ND, Arnott SA, Kannan K, Karthikraj R, et al. Perfluoroalkyl substances (PFASs) in edible fish species from charleston harbor and tributaries, South Carolina, United States: exposure and risk assessment. Environ Res. (2019) 171:266–77. doi: 10.1016/j.envres.2019.01.021
16. Hoffman K, Webster TF, Bartell SM, Weisskopf MG, Fletcher T, Vieira VM. Private drinking water wells as a source of exposure to perfluorooctanoic acid (PFOA) in communities surrounding a fluoropolymer production facility. Environ Health Perspect. (2011) 119:92–7. doi: 10.1289/ehp.1002503
17. Sunderland EM, Hu XC, Dassuncao C, Tokranov AK, Wagner CC, Allen JG, et al. review of the pathways of human exposure to poly-and perfluoroalkyl substances (PFASs) and present understanding of health effects. J Expo Sci Environ Epidemiol. (2019) 29:131–47. doi: 10.1038/s41370-018-0094-1
18. Tanner EM, Bloom MS, Wu Q, Kannan K, Yucel RM, Shrestha S, et al. Occupational exposure to perfluoroalkyl substances and serum levels of perfluorooctanesulfonic acid (PFOS) and perfluorooctanoic acid (PFOA) in an aging population from upstate New York: a retrospective cohort study. Int Arch Occup Environ Health. (2018) 91:145–54. doi: 10.1007/s00420-017-1267-2
19. Tittlemier SA, Pepper K, Seymour C, Moisey J, Bronson R, Cao XL, et al. Dietary exposure of Canadians to perfluorinated carboxylates and perfluorooctane sulfonate via consumption of meat, fish, fast foods, and food items prepared in their packaging. J Agric Food Chem. (2007) 55:3203–10. doi: 10.1021/jf0634045
20. Mamsen LS, Jönsson BAG, Lindh CH, Olesen RH, Larsen A, Ernst E, et al. Concentration of perfluorinated compounds and cotinine in human foetal organs, placenta, and maternal plasma. Sci Total Environ. (2017) 596–97:97–105. doi: 10.1016/j.scitotenv.2017.04.058
21. Blake BE, Fenton SE. Early life exposure to per- and polyfluoroalkyl substances (PFAS) and latent health outcomes: a review including the placenta as a target tissue and possible driver of peri- and postnatal effects. Toxicology. (2020) 443:152565. doi: 10.1016/j.tox.2020.152565
22. Szilagyi JT, Avula V, Fry RC. Perfluoroalkyl Substances (PFAS) and their effects on the placenta, pregnancy, and child development: a potential mechanistic role for placental Peroxisome Proliferator-Activated Receptors (PPARs). Curr Environ Health Rep. (2020) 7:222–30. doi: 10.1007/s40572-020-00279-0
23. Du G, Hu J, Huang H, Qin Y, Han X, Wu D, et al. Perfluorooctane sulfonate (PFOS) affects hormone receptor activity, steroidogenesis, and expression of endocrine-related genes in vitro and in vivo. Environ Toxicol Chem. (2013) 32:353–60. doi: 10.1002/etc.2034
24. Kaijser M, Granath F, Jacobsen G, Cnattingius S, Ekbom A. Maternal pregnancy estriol levels in relation to anamnestic and fetal anthropometric data. Epidemiology (Cambridge, Mass). (2000) 11:315–9. doi: 10.1097/00001648-200005000-00015
25. Benninghoff AD, Bisson WH, Koch DC, Ehresman DJ, Kolluri SK, Williams DE. Estrogen-like activity of perfluoroalkyl acids in vivo and interaction with human and rainbow trout estrogen receptors in vitro. Toxicol Sci. (2011) 120:42–58. doi: 10.1093/toxsci/kfq379
26. Henry ND, Fair PA. Comparison of in vitro cytotoxicity, estrogenicity and anti-estrogenicity of triclosan, perfluorooctane sulfonate and perfluorooctanoic acid. J Appl Toxicol. (2013) 33:265–72. doi: 10.1002/jat.1736
27. Kjeldsen LS, Bonefeld-Jørgensen EC. Perfluorinated compounds affect the function of sex hormone receptors. Environ Sci Pollut Res Int. (2013) 20:8031–44. doi: 10.1007/s11356-013-1753-3
28. Suh CH, Cho NK, Lee CK, Lee CH, Kim DH, Kim JH, et al. Perfluorooctanoic acid-induced inhibition of placental prolactin-family hormone and fetal growth retardation in mice. Mol Cell Endocrinol. (2011) 337:7–15. doi: 10.1016/j.mce.2011.01.009
29. Glazier JD, Cetin I, Perugino G, Ronzoni S, Grey AM, Mahendran D, et al. Association between the activity of the system A amino acid transporter in the microvillous plasma membrane of the human placenta and severity of fetal compromise in intrauterine growth restriction. Pediatr Res. (1997) 42:514–9. doi: 10.1203/00006450-199710000-00016
30. Koskela A, Koponen J, Lehenkari P, Viluksela M, Korkalainen M, Tuukkanen J. Perfluoroalkyl substances in human bone: concentrations in bones and effects on bone cell differentiation. Sci Rep. (2017) 7:6841. doi: 10.1038/s41598-017-07359-6
31. Bach CC, Bech BH, Brix N, Nohr EA, Bonde JP, Henriksen TB. Perfluoroalkyl and polyfluoroalkyl substances and human fetal growth: a systematic review. Crit Rev Toxicol. (2015) 45:53–67. doi: 10.3109/10408444.2014.952400
32. Johnson PI, Sutton P, Atchley DS, Koustas E, Lam J, Sen S, et al. The navigation guide—evidence-based medicine meets environmental health: systematic review of human evidence for PFOA effects on fetal growth. Environ Health Perspect. (2014) 122:1028–39. doi: 10.1289/ehp.1307893
33. Steenland K, Barry V, Savitz D. Serum perfluorooctanoic acid and birthweight: an updated meta-analysis with bias analysis. Epidemiology (Cambridge, Mass). (2018) 29:765–76. doi: 10.1097/EDE.0000000000000903
34. Dzierlenga MW, Crawford L, Longnecker MP. Birth weight and perfluorooctane sulfonic acid: a random-effects meta-regression analysis. LWW. (2020). doi: 10.1097/EE9.0000000000000095
35. Chen L, Tong C, Huo X, Zhang J, Tian Y, Cohort SB. Prenatal exposure to perfluoroalkyl and polyfluoroalkyl substances and birth outcomes: A longitudinal cohort with repeated measurements. Chemosphere. (2021) 267:128899. doi: 10.1016/j.chemosphere.2020.128899
36. Maisonet M, Terrell ML, McGeehin MA, Christensen KY, Holmes A, Calafat AM, et al. Maternal concentrations of polyfluoroalkyl compounds during pregnancy and fetal and postnatal growth in British girls. Environ Health Perspect. (2012) 120:1432–7. doi: 10.1289/ehp.1003096
37. Wikström S, Lin PI, Lindh CH, Shu H, Bornehag CG. Maternal serum levels of perfluoroalkyl substances in early pregnancy and offspring birth weight. Pediatr Res. (2020) 87:1093–9. doi: 10.1038/s41390-019-0720-1
38. Meng Q, Inoue K, Ritz B, Olsen J, Liew Z. Prenatal exposure to perfluoroalkyl substances and birth outcomes; an updated analysis from the Danish National Birth Cohort. Int J Environ Res Public Health. (2018) 15:1832. doi: 10.3390/ijerph15091832
39. Marks KJ, Cutler AJ, Jeddy Z, Northstone K, Kato K, Hartman TJ. Maternal serum concentrations of perfluoroalkyl substances and birth size in British boys. Int J Hyg Environ Health. (2019) 222:889–95. doi: 10.1016/j.ijheh.2019.03.008
40. Kashino I, Sasaki S, Okada E, Matsuura H, Goudarzi H, Miyashita C, et al. Prenatal exposure to 11 perfluoroalkyl substances and fetal growth: a large-scale, prospective birth cohort study. Environ Int. (2020) 136:105355. doi: 10.1016/j.envint.2019.105355
41. Manzano-Salgado CB, Casas M, Lopez-Espinosa MJ, Ballester F, Iñiguez C, Martinez D, et al. Prenatal exposure to perfluoroalkyl substances and birth outcomes in a Spanish birth cohort. Environ Int. (2017) 108:278–84. doi: 10.1016/j.envint.2017.09.006
42. Barker DJ, Hales CN, Fall CH, Osmond C, Phipps K, Clark PM. Type 2 (non-insulin-dependent) diabetes mellitus, hypertension and hyperlipidaemia (syndrome X): relation to reduced fetal growth. Diabetologia. (1993) 36:62–7. doi: 10.1007/BF00399095
43. Evensen E, Emaus N, Kokkvoll A, Wilsgaard T, Furberg A-S, Skeie G. The relation between birthweight, childhood body mass index, and overweight and obesity in late adolescence: a longitudinal cohort study from Norway, The Tromsø Study, Fit Futures. BMJ Open. (2017) 7:15576. doi: 10.1136/bmjopen-2016-015576
44. Jornayvaz FR, Vollenweider P, Bochud M, Mooser V, Waeber G, Marques-Vidal P. Low birth weight leads to obesity, diabetes and increased leptin levels in adults: the CoLaus study. Cardiovasc Diabetol. (2016) 15:1–10. doi: 10.1186/s12933-016-0389-2
45. Martin JA, Hamilton BE, Osterman MJK, Driscoll AK. Births: Final data for 2019. Natl Vital Stat Rep. (2021) 70:1–51.
46. Bastain TM, Chavez T, Habre R, Girguis MS, Grubbs B, Toledo-Corral C, et al. Study design, protocol and profile of the Maternal And Developmental Risks from Environmental and Social Stressors (MADRES) Pregnancy Cohort: a prospective cohort study in predominantly low-income hispanic women in Urban Los Angeles. BMC Pregnancy Childbirth. (2019) 19:189. doi: 10.1186/s12884-019-2330-7
47. Honda M, Robinson M, Kannan K, A. rapid method for the analysis of perfluorinated alkyl substances in serum by hybrid solid-phase extraction. Environ Chemistry. (2018) 15:92–9. doi: 10.1071/EN17192
48. Practice C. Medicine SfM-F. Committee opinion no 700: methods for estimating the due date Obstet Gynecol. (2017) 129:e150–e4. doi: 10.1097/AOG.0000000000002046
49. Aris IM, Kleinman KP, Belfort MB, Kaimal A, Oken E. A 2017 US reference for singleton birth weight percentiles using obstetric estimates of gestation. Pediatrics. (2019) 144:e20190076. doi: 10.1542/peds.2019-0076
50. Textor J, van der Zander B, Gilthorpe MS, Liskiewicz M, Ellison GT. Robust causal inference using directed acyclic graphs: the R package 'dagitty'. Int J Epidemiol. (2016) 45:1887–94. doi: 10.1093/ije/dyw341
51. Hornung RW, Reed LD. Estimation of average concentration in the presence of nondetectable values. Appl Occup Environ Hyg. (1990) 5:46–51. doi: 10.1080/1047322X.1990.10389587
52. Wilcox AJ, Weinberg CR, Basso O. On the pitfalls of adjusting for gestational age at birth. Am J Epidemiol. (2011) 174:1062–8. doi: 10.1093/aje/kwr230
53. Eick SM, Hom Thepaksorn EK, Izano MA, Cushing LJ, Wang Y, Smith SC, et al. Associations between prenatal maternal exposure to per- and polyfluoroalkyl substances (PFAS) and polybrominated diphenyl ethers (PBDEs) and birth outcomes among pregnant women in San Francisco. Environ Health. (2020) 19:100. doi: 10.1186/s12940-020-00654-2
54. Sagiv SK, Rifas-Shiman SL, Webster TF, Mora AM, Harris MH, Calafat AM, et al. Sociodemographic and Perinatal Predictors of Early Pregnancy Per- and Polyfluoroalkyl Substance (PFAS) concentrations. Environ Sci Technol. (2015) 49:11849–58. doi: 10.1021/acs.est.5b02489
55. Starling AP, Adgate JL, Hamman RF, Kechris K, Calafat AM, Ye X, et al. Perfluoroalkyl substances during pregnancy and offspring weight and adiposity at birth: examining mediation by maternal fasting glucose in the healthy start study. Environ Health Perspect. (2017) 125:067016. doi: 10.1289/EHP641
56. Fenton SE, Ducatman A, Boobis A, DeWitt JC, Lau C, Ng C, et al. Per- and polyfluoroalkyl substance toxicity and human health review: current state of knowledge and strategies for informing future research. Environ Toxicol Chem. (2021) 40:606–30. doi: 10.1002/etc.4890
57. Barbarossa A, Masetti R, Gazzotti T, Zama D, Astolfi A, Veyrand B, et al. Perfluoroalkyl substances in human milk: a first survey in Italy. Environ Int. (2013) 51:27–30. doi: 10.1016/j.envint.2012.10.001
58. Kato K, Wong LY, Chen A, Dunbar C, Webster GM, Lanphear BP, et al. Changes in serum concentrations of maternal poly- and perfluoroalkyl substances over the course of pregnancy and predictors of exposure in a multiethnic cohort of Cincinnati, Ohio pregnant women during 2003-2006. Environ Sci Technol. (2014) 48:9600–8. doi: 10.1021/es501811k
59. Mondal D, Weldon RH, Armstrong BG, Gibson LJ, Lopez-Espinosa MJ, Shin HM, et al. Breastfeeding: a potential excretion route for mothers and implications for infant exposure to perfluoroalkyl acids. Environ Health Perspect. (2014) 122:187–92. doi: 10.1289/ehp.1306613
60. Tao L, Kannan K, Wong CM, Arcaro KF, Butenhoff JL. Perfluorinated compounds in human milk from Massachusetts, U.S.A. Environ Sci Technol. (2008) 42:3096–101. doi: 10.1021/es702789k
61. Thomsen C, Haug LS, Stigum H, Frøshaug M, Broadwell SL, Becher G. Changes in concentrations of perfluorinated compounds, polybrominated diphenyl ethers, and polychlorinated biphenyls in Norwegian breast-milk during twelve months of lactation. Environ Sci Technol. (2010) 44:9550–6. doi: 10.1021/es1021922
62. Winkens K, Vestergren R, Berger U, Cousins IT. Early life exposure to per-and polyfluoroalkyl substances (PFASs): a critical review. Emerging Contaminants. (2017) 3:55–68. doi: 10.1016/j.emcon.2017.05.001
63. Boronow KE, Brody JG, Schaider LA, Peaslee GF, Havas L, Cohn BA. Serum concentrations of PFASs and exposure-related behaviors in African American and non-Hispanic white women. J Expo Sci Environ Epidemiol. (2019) 29:206–17. doi: 10.1038/s41370-018-0109-y
64. Park SK, Peng Q, Ding N, Mukherjee B, Harlow SD. Determinants of per- and polyfluoroalkyl substances (PFAS) in midlife women: evidence of racial/ethnic and geographic differences in PFAS exposure. Environ Res. (2019) 175:186–99. doi: 10.1016/j.envres.2019.05.028
65. Cushing L, Faust J, August LM, Cendak R, Wieland W, Alexeeff G. Racial/Ethnic disparities in cumulative environmental health impacts in california: evidence from a statewide environmental justice screening tool (CalEnviroScreen 1. 1). Am J Public Health. (2015) 105:2341–8. doi: 10.2105/AJPH.2015.302643
66. Brantsæter AL, Whitworth KW, Ydersbond TA, Haug LS, Haugen M, Knutsen HK, et al. Determinants of plasma concentrations of perfluoroalkyl substances in pregnant Norwegian women. Environ Int. (2013) 54:74–84. doi: 10.1016/j.envint.2012.12.014
67. Tsai MS, Miyashita C, Araki A, Itoh S, Bamai YA, Goudarzi H, et al. Determinants and temporal trends of perfluoroalkyl substances in pregnant women: the hokkaido study on environment and children's health. Int J Environ Res Public Health. (2018) 15:989. doi: 10.3390/ijerph15050989
68. Darrow LA, Stein CR, Steenland K. Serum perfluorooctanoic acid and perfluorooctane sulfonate concentrations in relation to birth outcomes in the Mid-Ohio Valley, 2005-2010. Environ Health Perspect. (2013) 121:1207–13. doi: 10.1289/ehp.1206372
69. Fei C, McLaughlin JK, Tarone RE, Olsen J. Perfluorinated chemicals and fetal growth: a study within the Danish National Birth Cohort. Environ Health Perspect. (2007) 115:1677–82. doi: 10.1289/ehp.10506
70. Hamm MP, Cherry NM, Chan E, Martin JW, Burstyn I. Maternal exposure to perfluorinated acids and fetal growth. J Expo Sci Environ Epidemiol. (2010) 20:589–97. doi: 10.1038/jes.2009.57
71. Monroy R, Morrison K, Teo K, Atkinson S, Kubwabo C, Stewart B, et al. Serum levels of perfluoroalkyl compounds in human maternal and umbilical cord blood samples. Environ Res. (2008) 108:56–62. doi: 10.1016/j.envres.2008.06.001
72. Washino N, Saijo Y, Sasaki S, Kato S, Ban S, Konishi K, et al. Correlations between prenatal exposure to perfluorinated chemicals and reduced fetal growth. Environ Health Perspect. (2009) 117:660–7. doi: 10.1289/ehp.11681
73. Chen MH, Ha EH, Wen TW, Su YN, Lien GW, Chen CY, et al. Perfluorinated compounds in umbilical cord blood and adverse birth outcomes. PLoS ONE. (2012) 7:e42474. doi: 10.1371/journal.pone.0042474
74. Calafat A, Kato K, Ye X editors. Urine and Serum Biomarkers of Per-and Polyfluoroalkyl Substances (PFAS) and Fluorinated Alternatives for Human Exposure Assessment. ISEE Conference Abstracts. (2018).
75. Ehresman DJ, Froehlich JW, Olsen GW, Chang SC, Butenhoff JL. Comparison of human whole blood, plasma, and serum matrices for the determination of perfluorooctanesulfonate (PFOS), perfluorooctanoate (PFOA), and other fluorochemicals. Environ Res. (2007) 103:176–84. doi: 10.1016/j.envres.2006.06.008
76. Verner MA, Loccisano AE, Morken NH, Yoon M, Wu H, McDougall R, et al. Associations of Perfluoroalkyl Substances (PFAS) with Lower Birth Weight: An Evaluation of Potential Confounding by Glomerular Filtration Rate Using a Physiologically Based Pharmacokinetic Model (PBPK). Environ Health Perspect. (2015) 123:1317–24. doi: 10.1289/ehp.1408837
Keywords: PFAS, perfluorooctanoic acid (PFOA), birthweight, health disparities, pregnancy
Citation: Peterson AK, Eckel SP, Habre R, Yang T, Faham D, Farzan SF, Grubbs BH, Kannan K, Robinson M, Lerner D, Al-Marayati LA, Walker DK, Grant EG, Bastain TM and Breton CV (2022) Prenatal Perfluorooctanoic Acid (PFOA) Exposure Is Associated With Lower Infant Birthweight Within the MADRES Pregnancy Cohort. Front. Epidemiol. 2:934715. doi: 10.3389/fepid.2022.934715
Received: 03 May 2022; Accepted: 13 June 2022;
Published: 13 July 2022.
Edited by:
Ruby H. N. Nguyen, University of Minnesota Twin Cities, United StatesReviewed by:
Marcela Tamayo-Ortiz, Instituto Mexicano del Seguro Social, MexicoCopyright © 2022 Peterson, Eckel, Habre, Yang, Faham, Farzan, Grubbs, Kannan, Robinson, Lerner, Al-Marayati, Walker, Grant, Bastain and Breton. This is an open-access article distributed under the terms of the Creative Commons Attribution License (CC BY). The use, distribution or reproduction in other forums is permitted, provided the original author(s) and the copyright owner(s) are credited and that the original publication in this journal is cited, in accordance with accepted academic practice. No use, distribution or reproduction is permitted which does not comply with these terms.
*Correspondence: Carrie V. Breton, YnJldG9uQHVzYy5lZHU=
Disclaimer: All claims expressed in this article are solely those of the authors and do not necessarily represent those of their affiliated organizations, or those of the publisher, the editors and the reviewers. Any product that may be evaluated in this article or claim that may be made by its manufacturer is not guaranteed or endorsed by the publisher.
Research integrity at Frontiers
Learn more about the work of our research integrity team to safeguard the quality of each article we publish.