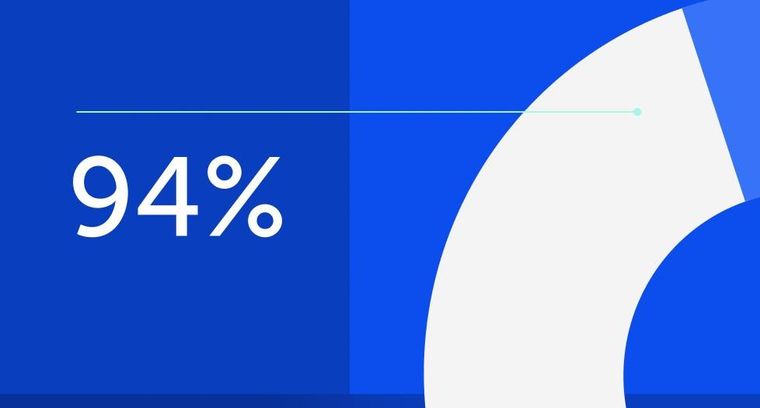
94% of researchers rate our articles as excellent or good
Learn more about the work of our research integrity team to safeguard the quality of each article we publish.
Find out more
MINI REVIEW article
Front. Environ. Sci., 07 April 2025
Sec. Interdisciplinary Climate Studies
Volume 13 - 2025 | https://doi.org/10.3389/fenvs.2025.1544586
This article is part of the Research TopicClimate Change Impacts on Arctic Ecosystems and Associated Climate FeedbacksView all 9 articles
The Net Ecosystem Carbon Balance (NECB) is a crucial metric for understanding integrated carbon dynamics in Arctic and boreal regions, which are vital to the global carbon cycle. These areas are associated with significant uncertainties and rapid climate change, potentially leading to unpredictable alterations in carbon dynamics. This mini-review examines key components of NECB, including carbon sequestration, methane emissions, lateral carbon transport, herbivore interactions, and disturbances, while integrating insights from recent permafrost region greenhouse gas budget syntheses. We emphasize the need for a holistic approach to quantify the NECB, incorporating all components and their uncertainties. The review highlights recent methodological advances in flux measurements, including improvements in eddy covariance and automatic chamber techniques, as well as progress in modeling approaches and data assimilation. Key research priorities are identified, such as improving the representation of inland waters in process-based models, expanding monitoring networks, and enhancing integration of long-term field observations with modeling approaches. These efforts are essential for accurately quantifying current and future greenhouse gas budgets in rapidly changing northern landscapes, ultimately informing more effective climate change mitigation strategies and ecosystem management practices. The review aligns with the goals of the Arctic Monitoring and Assessment Program (AMAP) and Conservation of Arctic Flora and Fauna (CAFF), providing important insights for policymakers, researchers, and stakeholders working to understand and protect these sensitive ecosystems.
The delicate balance of carbon (C) exchange within ecosystems, particularly in Arctic and northern boreal catchments, holds significant implications for understanding and mitigating the impacts of climate change. The Net Ecosystem Carbon Balance (NECB) considers all carbon fluxes, including both vertical net exchange of C between ecosystems and the atmosphere and lateral C transfer downstream. The NECB integrates processes such as photosynthesis and autotrophic and heterotrophic respiration (López-Blanco et al., 2019; See et al., 2024), methane emissions (McNicol et al., 2023; Parmentier et al., 2024; Yuan et al., 2024), and lateral C transport (Rocher-Ros et al., 2019; Casas-Ruiz et al., 2023). Only few studies so far have included all components (Roulet et al., 2007; Nilsson et al., 2008; Juutinen et al., 2013; Pumpanen et al., 2014). Most often land-atmosphere exchanges, lateral dissolved organic C (DOC) fluxes, and their links to hydrological pathways or the impacts of grazing and environmental disturbances are studied in isolation. Addressing all flux components, i.e., compiling NECB, provides a comprehensive measure of an ecosystem’s capacity at the landscape level to act as either a C sink or a source. This is essential for evaluating ecosystem health—referring to its resilience and functional stability—and its role in climate regulation (Schuur et al., 2015).
Understanding the regulation of all flux components is pivotal now when the Arctic and boreal ecosystems are undergoing some of the fastest warming on the planet, with temperatures increasing three to four times faster than the global average (AMAP, 2022; Rantanen et al., 2022). Recent climate models project Arctic temperature increases of 3°C–4°C by mid-century, far outpacing global averages due to Arctic amplification (Box et al., 2019). This phenomenon, driven by feedback mechanisms such as albedo changes from sea ice and snow loss, shifts in atmospheric and oceanic circulation, and variations in cloud cover and water vapor, has profound implications for C cycling in these regions (Serreze and Barry, 2011).
Arctic amplification alters precipitation patterns and form (Bintanja and Andry, 2017; Bintanja et al., 2020), disrupts permafrost stability (Koven et al., 2011; Turetsky et al., 2020), and accelerates greenhouse gas (GHG) emissions (Natali et al., 2019; Hugelius et al., 2024; Ramage et al., 2024). While extended growing seasons may enhance plant productivity, increased soil respiration can counteract these gains by releasing stored C (Natali et al., 2019). The overall intensification of biogeochemical activities in soil and water bodies further amplifies this dynamic. Moreover, permafrost destabilization risks releasing vast amounts of stored C, altering hydrological regimes and affecting CO2 and CH4 emissions and lateral C transport (Schuur et al., 2009; Vonk et al., 2023). Additionally, warming increases the likelihood of extreme events (Walsh et al., 2020), such as droughts or heavy precipitation, which can exacerbate ecosystem instability and further disrupt C and water cycles (Frank et al., 2015).
The NECB in Arctic-boreal systems plays a central role in global climate change projections due to the enormous C stocks stored in these regions and their sensitivity to warming (McGuire et al., 2009; Schuur et al., 2015). Permafrost regions store approximately 1,000 ± 200 Pg of organic C within the upper 3 m (Hugelius et al., 2014; Mishra et al., 2021; Palmtag et al., 2022)—almost double the C present in the atmosphere. Understanding the NECB responses to warming is crucial for predicting potential carbon-climate feedbacks, which can significantly impact global climate trajectories (Vonk and Gustafsson, 2013; Turetsky et al., 2020). These feedback effects represent a significant challenge for maintaining the stability of the Earth’s climate system (Vonk and Gustafsson, 2013; Schuur et al., 2015; Turetsky et al., 2020).
Recent studies (Hugelius et al., 2024; Ramage et al., 2024) have underscored the critical role of permafrost regions in the global C cycle, providing pan-Arctic insights into GHG dynamics from 2000 to 2020. For example, GHG flux upscaling estimates indicate the permafrost region is a net CO2 sink but a significant source of CH4 and N2O emissions (Ramage et al., 2024). Modeling approaches reveal a weak CO2 sink and substantial CH4 and N2O emissions, with a net warming effect over short timescales (20 years) but a neutral effect over 100 years (Hugelius et al., 2024). These findings highlight substantial uncertainties and mismatches between field observations and models, emphasizing the need for localized investigations to better understand site-specific dynamics and interactions affecting NECB across diverse Arctic ecosystems. Watts et al. (2023) estimated the terrestrial domain of Arctic-Boreal zone be a C sink as a whole, but if accounting the aquatic ecosystems, the sink decreased notably and there were substantial differences in the C sink strength across the boreal-arctic domain.
Understanding the components of the NECB is key to comprehending how Arctic and boreal ecosystems operate and adapt to environmental changes. These components encompass net ecosystem CO2 exchange, methane emissions, lateral C transport, interactions with herbivores, and ecosystem disturbances. Below, we delve into each component in detail to explore their individual and collective roles in shaping NECB.
NEE is the balance between gross primary production (GPP) and ecosystem respiration (ER), and both are fundamental components of the NECB. GPP represents the total CO2 assimilated by plants through photosynthesis, while ER encompasses the release of CO2 from organisms and decaying matter, including both autotrophic (plant) and heterotrophic (microbial) respiration (López-Blanco et al., 2019). The balance between these two processes determines whether an ecosystem acts as an atmospheric CO2 sink or source (McGuire et al., 2009; See et al., 2024).
In Arctic regions, GPP is typically constrained by short growing seasons, low temperatures, snow cover, and limited nutrient availability (Chapin III et al., 2000). However, climate change alters these limitations, potentially enhancing GPP by extending the growing season, increasing temperatures in air, soil and water bodies, and augmenting nutrient availability through permafrost thaw (Natali et al., 2015; López-Blanco et al., 2020). Studies have shown that GPP in tundra ecosystems is highly sensitive to temperature fluctuations and can significantly influence NECB (Euskirchen et al., 2006). Longer-term changes in plant community composition, such as shrub expansion, can alter photosynthetic rates and GPP across different landscapes (Myers-Smith et al., 2011; Bjorkman et al., 2018). The NEE is highly sensitive to annual variations in water table levels and temperature. For instance, dry summers or periods with atmospheric drought can shift peatlands, typically net CO2 sinks, into net CO2 sources, primarily due to reductions in GPP (Alm et al., 1999; Aurela et al., 2007; Rinne et al., 2020). Historically, peatlands have functioned as C sinks in most years, facilitating peat accumulation over time, which has cooled the climate due to the stored C (Frolking and Roulet, 2007).
In high latitudes, ER, on the other hand, is expected to increase with warming temperatures, potentially offsetting gains in GPP (Schuur et al., 2009; Commane et al., 2017). Soil respiration, a major component of ER, is particularly sensitive to temperature changes in permafrost regions (Bond-Lamberty and Thomson, 2010). Recent research has highlighted the importance of winter respiration, which can significantly contribute to annual C budgets (Natali et al., 2019). Helbig et al. (2022) analyzed multiyear eddy covariance data from boreal-Arctic peatland sites and found that warm anomalies increased CO2 uptake relative to average conditions when warming occurred in early summer, whereas late-summer warming resulted in increased CO2 release. These anomalies were linked to earlier vegetation development during early summer and typically lower water levels in late summer, possibly suppressing GPP and increasing ER.
The Arctic-boreal zone exhibits substantial variability in CO2 fluxes, with observed annual NEE ranging from −27.9 g C m−2 yr−1 (net CO2 uptake) to net release of CO2 in certain years. Seasonal dynamics are pronounced, with monthly GPP varying from −2 to −516 g C m−2 and ER from 0 to 550 g C m−2 (Virkkala et al., 2022; See et al., 2024). Notably, more than 30% of the region functions as a net CO2 source, and when fire emissions are included, the permafrost region approaches a net zero CO2 balance, highlighting the critical role of fire in shaping regional C dynamics (Virkkala et al., 2025). The complex interplay between GPP and ER under changing climate conditions underscores the need for continued monitoring and improved modeling of these processes to accurately predict future C dynamics in Arctic-boreal ecosystems (Virkkala et al., 2022). Respiratory outputs (particularly heterotrophic respiration), and C turnover and decomposition processes remain highly uncertain and poorly constrained in models (Carvalhais et al., 2014; López-Blanco et al., 2019). Precipitation and soil moisture have been highlighted as the key drivers of heterotrophic respiration interannual variability (Yao et al., 2021; Guenet et al., 2024). Such uncertainties ultimately propagate over the rate and magnitude of C accumulation.
CH4 emissions play a crucial role in the NECB of Arctic-boreal ecosystems, particularly in wetlands and peatlands, and areas affected by permafrost thaw. Methane is produced by microbes under anaerobic conditions and has a much higher global warming potential than CO2 over short time scales (Turetsky et al., 2014; Kuhn et al., 2021). Even without considering the global warming potential, methane emissions in wet ecosystems also form a significant component of the mass transfer of C and may account for 20% of the total C turnover (Christensen et al., 2007). In many, if not all, wetlands CH4-C losses decrease substantially the C gain of NEE (Juutinen et al., 2013; Rinne et al., 2020). Recent studies have shown that methane emissions in the Arctic are higher than previously estimated, especially during the cold season (Zona et al., 2016). Treat et al. (2018a) estimated that methane emissions from freshwater bodies (observationally measured) contribute 4%–17% of the total annual methane emissions for the circumpolar Arctic region, corresponding to 6.1 ± 1.5 Tg CH4/year north of 40° latitude. This is also the case in results obtained from ecosystems models for which the calibration is mainly driven by growing season datasets and for which cold season processes may be missing or may not be well accounted for (Ito et al., 2023). CH4 emissions from lakes and streams are crucial components to consider in the boreal-Arctic region, especially as part of catchment-scale assessments. Some studies even identify freshwater bodies as the largest CH4 source in the boreal-Arctic area (Wik et al., 2016).
Arctic-boreal wetlands, including peatlands, are significant sources of methane, with emissions modulated by warming and vegetation activity. Wetlands emit in the order of 48.7 (13.3–86.9) Tg CH4 yr-1, while freshwater systems contribute approximately 12.5 Tg CH4 yr−1 (Parmentier et al., 2024). These emissions are influenced by temperature, vegetation activity, and permafrost thaw. For instance, a recent study found that temperature explains 52.3% of the increasing CH4 emission trend, followed by GPP (40.7%) (Yuan et al., 2024). Thawing permafrost can lead to the formation of thermokarst lakes and extend wetland areas, potentially releasing large amounts of previously frozen organic matter and increasing methane production (Treat et al., 2018b; Turetsky et al., 2020; Parmentier et al., 2024).
The spatial and temporal variability of methane emissions across Arctic-boreal landscapes presents significant challenges for accurate quantification and prediction. Recent efforts, such as the BAWLD-CH4 dataset, have advanced our understanding of methane flux patterns across diverse boreal and Arctic ecosystems (Kuhn et al., 2021). However, uncertainties remain, particularly regarding the fate of methane in the water column and its transport through soil, surface runoff and snow (Saunois et al., 2020). For instance, non-growing seasons, particularly in autumn and spring, remain difficult periods for maintaining continuous methane flux measurements (Jentzsch et al., 2024). Vegetation composition and the presence or absence of specific vascular plant species also significantly influence methane emissions, making the impacts of climate change on future vegetation composition a critical area for further study (AMAP, 2015). Uncertainties surrounding lateral methane transport from wet tundra and peatlands are compounded by limited understanding of dissolved methane dynamics—how much is oxidized versus emitted from streams and ponds (Oh et al., 2020). These processes, currently underrepresented in ecosystem models, demand further investigation across diverse Arctic landscapes. Challenges persist in both experimental and observational approaches to accurately measure methane emissions on an annual scale in the context of NECB. Addressing these gaps is essential for improving the parameterizations used in process models. Ultimately, enhancing our understanding of methane dynamics is key to accurately assessing the greenhouse gas budget of Arctic-boreal regions and predicting their feedback to global climate change (Hugelius et al., 2024).
Lateral C transport, the movement of DOC, particulate organic C (POC), and dissolved inorganic C (DIC), plays a crucial role in the Arctic-boreal C balance, yet it remains underrepresented in NECB assessments (Dean et al., 2020). Carbon moves from terrestrial ecosystems to aquatic systems via groundwater leaching, runoff, streams, and rivers, contributing to substantial C losses from terrestrial ecosystems, influencing both local and regional C budgets (Tank et al., 2012; Tank et al., 2018; Vonk et al., 2023). A significant portion of this C is either emitted as CO2 and CH4 from freshwater systems or transported to the ocean, with global fluxes estimated at 5.1 Pg C yr−1—of which 0.9–1.3 Pg C yr−1 reaches the ocean, 2.1–2.9 Pg C yr−1 is released as CO2, and 0.6–1.5 Pg C yr−1 is buried in sediments (Tank et al., 2018). Globally, lateral C fluxes have been estimated to be comparable to the terrestrial CO2 sink, approximately 3.1 Pg C yr−1 (Le Quéré et al., 2016), ranging from 1.1 Pg C yr−1 to 5.1 Pg of C yr−1 (Drake et al., 2018). In Arctic-boreal ecosystems, studies suggest that lateral C fluxes represent 0.2%–1.4% of the terrestrial C stock, depending on the region and landscape characteristics (Martens et al., 2022), and nearly 20% of the net terrestrial C uptake (Kling et al., 1991).
Recent high-resolution studies have revealed complex seasonal and interannual variations in DOC transport processes in subarctic headwater catchments (Croghan et al., 2024) and indicated complex variations between C sources at the landscape level. Climate change is altering these transport mechanisms, with spring snowmelt floods and summer/autumn storm events becoming increasingly important for DOC export (Rawlins and Karmalkar, 2024), and also winter runoff in southern Arctic sites. The thawing of permafrost is expected to enhance the mobilization and transport of previously frozen organic matter, potentially leading to increased DOC and DIC fluxes to aquatic systems (Vonk and Gustafsson, 2013).
The fate of this laterally transported C is crucial for understanding its impact on the global C cycle. While some of the C may be deposited in sediments or transported to the ocean, a significant portion can be evaded directly to the atmosphere or processed within inland waters, leading to CO2 and CH4 emissions (Casas-Ruiz et al., 2023; Mustonen et al., 2024). Recent research has highlighted that small watersheds and water bodies including streams, ponds and lakes may play a disproportionate role in Arctic land-ocean fluxes, emphasizing the need for better representation of these systems in C budget assessments (Vonk et al., 2023). However, the lack of watershed-scale studies across Arctic limit our ability to identify the main controlling factors and key locations at the landscape level.
Integrating aquatic C fluxes and the ecosystem-atmosphere exchange of C remain a challenge due to the high spatial and temporal variability of these processes. Improved monitoring networks including high-resolution sensors, coupled with advanced modeling approaches, are necessary to better constrain estimates of lateral C transport and its contribution to NECB in Arctic-boreal ecosystems (Rocher-Ros et al., 2019; Olefeldt et al., 2021).
The role of herbivores in C cycling within high-latitude ecosystems has gained increasing recognition in recent years (Schmitz et al., 2014). Both large herbivores, such as reindeer/caribou (Ylänne et al., 2018), muskox (Falk et al., 2015) and small mammals (Tuomi et al., 2019), significantly influence C cycling in Arctic and boreal ecosystems through grazing, trampling, and nutrient deposition (Koltz et al., 2022), also during the snowy seasons. Their trampling and cratering in search of food under the snow can damage the vegetation, potentially hindering treeline expansion (Heggenes et al., 2017). Additionally, tree girdling caused by reindeer rubbing against young trees (“buck rub”) can further inhibit forest regeneration (Roturier and Bergsten, 2006). Their interactions can alter vegetation dynamics, soil properties, hydrology, energy balances, and C and nutrient cycling, ultimately affecting the NECB (Ylänne et al., 2015; Schmitz et al., 2023; Schmidt et al., 2024). In the Arctic, grazing and trampling activities compact snow reducing insulation, which consequently prevents permafrost thawing, thereby reducing CH4 emissions. For example, increasing herbivore densities in Arctic regions could protect up to 80% of the Yedoma permafrost domain, which stores around 500 Gt of organic C (Schmitz et al., 2023). However, herbivory can also have mixed effects on C dynamics, with some studies showing reductions in C uptake by 15%–70% due to changes in plant community composition and ecosystem respiration rates (Schmitz et al., 2018).
Large herbivores are known to influence plant community structure, often reducing shrub and moss abundance and promoting graminoid-dominated vegetation (Olofsson et al., 2009), or reducing overall plant biomass (Olofsson et al., 2014). This shift can have cascading effects on ecosystem processes, including C sequestration, soil respiration (Väisänen et al., 2014), and methane emissions (Falk et al., 2015), as well as on ecosystem energy balance via changes in, e.g., evapotranspiration (Zimov et al., 1995), albedo (te Beest et al., 2016), soil thermal regimes, and thaw depth (Windirsch et al., 2022). Recent studies have highlighted the substantial impacts of herbivores on C fluxes and stocks; for example, muskox and lemming herbivory can reduce net CO2 uptake in the short term, though vegetation often recovers quickly (Falk et al., 2015; Plein et al., 2022), while different types of large animals can increase the C storage in permafrost soils (Zimov, 2005; Windirsch et al., 2022). Further research is needed however to disentangle whether increased C content results from reduced decomposition or higher C input. Herbivory-induced changes in plant communities not only impact NECB directly but also indirectly through various belowground alterations, such as changes in the quantity and quality of litter (Francini et al., 2014) and soil organic matter (Väisänen et al., 2015). Additionally, herbivores can induce shifts in soil faunal (Sørensen et al., 2009) and microbial communities (Ahonen et al., 2021). Herbivores also influence their environment through the redistribution of nutrients, contributing to local fertilization (Van Der Wal et al., 2004) and physical disturbances, such as trampling (Mosbacher et al., 2019).
The overall strength and direction of herbivore effects on NECB can vary spatially and temporally, influenced by factors such as herbivore traits and density, plant community composition, and climate conditions (Schmitz and Leroux, 2020). Understanding these complex herbivore-ecosystem interactions is essential for accurately assessing and predicting C dynamics in rapidly changing Arctic and boreal regions (Koltz et al., 2022).
Disturbances can significantly impact the NECB of tundra and boreal ecosystems (Foster et al., 2022) by altering C storage and fluxes (Phoenix and Bjerke, 2016). Climate change is intensifying various disturbance regimes in the Arctic, including extreme weather events (Christensen et al., 2020; van Beest et al., 2022), thermokarst formation (Lewkowicz and Way, 2019; Turetsky et al., 2020), wildfires (Mack et al., 2011; Byrne et al., 2024), and insect outbreaks (Heliasz et al., 2011; Lund et al., 2017).
These natural disturbances, combined with anthropogenic activities like resource extraction (mining, land use and settlements) and infrastructure development (Raynolds et al., 2014), are reshaping tundra landscapes and C dynamics. Extreme weather events disrupt vegetation growth and soil processes (Phoenix and Bjerke, 2016), while thermokarst formation mobilizes frozen soil C (Turetsky et al., 2020). Increasingly frequent wildfires consume surface vegetation and alter post-fire succession (Mack et al., 2011), and expanding insect outbreaks can reduce productivity and increase shrub and tree mortality (Heliasz et al., 2011; López-Blanco et al., 2017; Lund et al., 2017). The northward expansion of beavers into Arctic tundra ecosystems is emerging as a significant disturbance regime, profoundly altering hydrological patterns, accelerating permafrost thawing and enhancing methane emissions (Tape et al., 2022; Clark et al., 2023). The complex interplay between these disturbances creates feedbacks that amplify climate change impacts in tundra and boreal ecosystems (Raynolds et al., 2014; Phoenix and Bjerke, 2016), highlighting the need for comprehensive monitoring and modeling of Arctic C balance.
Understanding and quantifying these components together provides a comprehensive view of the key ecosystem processes influencing NECB in Arctic and boreal ecosystems. Future research should focus on integrating these components to better predict ecosystem responses to ongoing climate change.
Recent years have seen significant advancements in our understanding of high-latitude C dynamics, driven by improvements in both observational techniques and modeling approaches. These advances have enhanced our ability to understand, quantify, and predict NECB in Arctic and boreal ecosystems.
Eddy covariance (EC) has become the gold standard for measuring ecosystem-scale greenhouse gas fluxes (Baldocchi, 2003), and recent years have seen significant advancements in this technique. High-frequency open-path and closed-path gas analyzers have improved, allowing for more precise measurements of CO2, CH4, and H2O fluxes (Burba, 2013). The development of low-power, low-maintenance EC systems has enabled year-round measurements in remote Arctic and boreal locations, addressing critical data gaps during the non-growing season (Oechel et al., 2014). Additionally, novel approaches such as the use of unmanned aerial vehicles (UAVs) equipped with miniaturized EC systems (Bolek et al., 2024) or connected with high-resolution portable GHG analysers (Scheller et al., 2022) have emerged, allowing for spatial mapping of fluxes and concentration hot spots over heterogeneous landscapes.
Recent studies have also focused on improving flux gap-filling and partitioning methods. Vekuri et al. (2023) have recently shown that the commonly used marginal distribution sampling (MDS) method produces significant systematic error for data sets collected from northern (>60°N) sites, and should be replaced by machine learning methods which avoid this error. The partitioning methods, which are typically used after gap-filling the NEE time series, separate NEE into its component fluxes of GPP and ER. There are novel machine learning approaches that have been developed to improve the accuracy of flux partitioning, particularly in Arctic ecosystems where traditional methods may fall short (Tramontana et al., 2020). Furthermore, advances in CH4 isotope measurement techniques have provided new insights into the sources and sinks of C in these ecosystems, revealing significant spatial variations in δ13C-CH4 values and highlighting the importance of substrate availability for methanogenesis in driving CH4 emissions patterns (Rinne et al., 2022).
Automatic chamber systems have become increasingly important for measuring GHG fluxes in Arctic-boreal ecosystems, particularly during the challenging non-growing season (Koskinen et al., 2014) and in aquatic systems (Thanh Duc et al., 2020). These systems allow for continuous, high-frequency measurements of CO2 and CH4 fluxes, providing crucial data on temporal and spatial variability (Pirk et al., 2017; Natali et al., 2019), also documenting surprising seasonal dynamics and episodic events (Mastepanov et al., 2008). Recent advancements in automatic chamber design have improved their reliability in harsh Arctic conditions, with better insulation and heating systems to prevent snow and ice accumulation (Mastepanov et al., 2013; Korkiakoski et al., 2017).
Multi-chamber systems have been developed to capture spatial heterogeneity in flux patterns, especially important in ecosystems with high microtopographic variability (Mastepanov et al., 2013; Pirk et al., 2017). Additionally, the integration of soil temperature and moisture sensors within chamber systems has enhanced our understanding of the environmental drivers of flux variability (Göckede et al., 2019). These systems can now be coupled with real-time gas analyzers, allowing for immediate data processing and quality control (Korkiakoski et al., 2020). This advancement enables researchers to obtain and analyze high-quality greenhouse gas flux data in near real-time, improving the efficiency and accuracy of field measurements in Arctic and boreal ecosystems.
Lateral transport of C, with particular focus on understanding the speciation of the C pool, has gained attention as a crucial component of the NECB in Arctic-boreal systems (Tank et al., 2018). Recent methodological advances have improved our ability to quantify these fluxes. High-frequency in situ sensors for DOC and POC using optical sensors have been deployed in river systems, allowing for continuous monitoring of C export from terrestrial to aquatic ecosystems (Shogren et al., 2021; Rawlins and Karmalkar, 2024). Coupling high-frequency dissolved C concentrations with discharge (e.g., concentration-discharge relationships) provides a tool to identify processes that control C export (Gómez-Gener et al., 2021; Speir et al., 2024). To understand the fate of this C export, there is a need to couple these measurements with in-situ aquatic flux measurements, especially given the spatial variability of these fluxes (Bretz et al., 2021).
Tracer techniques, using both stable and radioactive isotopes, have been refined to better understand the sources and ages of water and laterally transported C. These methods have revealed the importance of old C mobilisation from thawing permafrost in lateral fluxes (Serikova et al., 2018), novel approaches combining hydrological measurements with C concentration data have improved estimates of annual C export, particularly during the critical spring freshet period (Beel et al., 2021). Further, recent studies using 222Rn have provided estimations of methane transport in groundwater (Olid et al., 2022).
There has been a recent call for more spatially resolute sampling to identify landscape control points that influence lateral C transport and, consequently, emissions from land-water systems (Bernhardt et al., 2017). To accurately scale these fluxes to the catchment level, it is essential to account for both the landscape features that supply C to freshwater systems (e.g., wetlands, thaw slumps) and in-situ controls such as gas transfer velocity (Kokelj et al., 2013; Rocher-Ros et al., 2019; Shogren et al., 2019).
Fences that either exclude or enclose herbivores are an essential tool for quantifying the impact of herbivory on NECB in Arctic-boreal ecosystems. Long-term exclosure studies, spanning several decades to over a century, have provided valuable insights into the cumulative effects of herbivores on vegetation structure, soil C stocks, and greenhouse gas fluxes (Ylänne et al., 2018). Moreover, exclosure designs that selectively exclude different herbivore guilds (e.g., large, small mammals, and geese) help the disentanglement of their specific impacts (Köster et al., 2017; Petit Bon et al., 2023), which is particularly relevant in the context of shifting tundra herbivory communities (Barbero-Palacios et al., 2024).
The integration of flux measurement techniques with exclosure and enclosure experiments has significantly improved our understanding of herbivory effects on C cycling. For instance, portable flux chambers have been used to compare CO2 and CH4 fluxes inside and outside exclosures, revealing how herbivores influence both primary productivity and soil respiration (Cahoon et al., 2012; Lara et al., 2017; Silfver et al., 2020), and how plant abundance, phenology, and nitrogen dynamics change (Mosbacher et al., 2019). Some studies have combined exclosures with manipulative experiments (e.g., warming, fertilization) to investigate how herbivory interacts with other environmental changes to affect NECB (Sjögersten et al., 2012; Väisänen et al., 2014), and to provide crucial data on potential feedbacks between climate change and herbivore impacts on C cycling (Post et al., 2021).
Long-term monitoring networks have become increasingly crucial for understanding the complex dynamics of Arctic-boreal C cycling. The FLUXNET network, for example, and its regional counterparts (e.g., ICOS in Europe, AmeriFlux and NEON in North America), has been instrumental to provide continuous, multi-year datasets of C, water, and energy fluxes from numerous sites in high-latitude regions (Pastorello et al., 2020). Specifically, the FLUXNET-CH4 community network has greatly enhanced our understanding of methane dynamics in wetland ecosystems across the Arctic-boreal zone (Knox et al., 2019). The Greenland Ecosystem Monitoring (GEM) program (Christensen et al., 2017), established in 1995, provides a unique integrated and interdisciplinary approach to understanding Arctic ecosystems and climate change effects, by measuring a wide range of cross-cutting variables across a catchment scale, from glaciers to marine systems within a 20 km range. GEM serves as example of coordinated observational data gathering across meteorological, hydrological, terrestrial and limnic ecosystem domains in a confined catchment area providing the opportunity for true data-based NECB budgeting (Figure 1). This may in turn serve as pivotal data for NECB model calibration and validation.
Figure 1. Integrated assessment of Net Ecosystem Carbon Balance (NECB) components (Sections 2.1–4) in the Rylekærene fen, Zackenberg, East Greenland. The 3D terrain representation, viewed from the southwest corner, illustrates the spatial distribution and measurement approaches for key C flux processes: net ecosystem CO2 exchange (orange box, measured via eddy covariance towers), methane emissions (purple box, quantified through automatic chambers), herbivore-vegetation interactions (green box, studied using exclosure experiments), and lateral C transport (blue box, monitored via river discharge and DOC measurements). This landscape-scale integration enables comprehensive NECB modeling and assessment of ecosystem responses to environmental changes. The map has been edited for illustrative purposes, combining hand-drawn features with enhancements using GIS and photo-editing software. It is based on original image mosaics from Greenland Ecosystem Monitoring (2014 and 2020). The topography is vertically exaggerated by a factor of 5, and the locations of buildings and instruments have been modified for presentation purposes. The stream network is simplified for clarity. Picture acknowledgements, from top to bottom: Efrén López-Blanco, Lars Holst Hansen, Falk et al. (2015) from Fig. 3, Efrén López-Blanco.
These networks not only provide essential data for understanding current C dynamics but also serve as early warning systems for detecting ecosystem changes. For instance, the International Tundra Experiment (ITEX) network (Henry et al., 2022), established in the early 1990s, continues to provide valuable long-term data on the impacts of experimental warming on tundra vegetation and associated C fluxes (Bjorkman et al., 2018). Likewise, the Back to the Future project reveals multi-decadal changes in vegetation and soil C stocks (Callaghan et al., 2011).
In-situ GHG budget synthesis efforts have been instrumental in integrating diverse datasets to derive comprehensive insights across the pan-Arctic region. For example, the recent second phase of the Regional Carbon Cycle Assessment and Processes project (RECCAP2) (Ciais et al., 2022) has specifically focused on the permafrost region (Hugelius et al., 2024; Ramage et al., 2024), synthesizing multiple lines of evidence to deliver thorough assessments of current C dynamics in these critical areas. This budgeting initiative includes three extensive compilations of GHG flux datasets for CO2 (Virkkala et al., 2022), CH4 (Kuhn et al., 2021), and nitrous oxide, N2O (Voigt et al., 2020), all derived from valuable in-situ observations. These collective efforts have not only advanced our understanding of existing C cycling patterns but have also underscored the numerous unresolved uncertainties that persist in this field.
Since the 1970s, Earth System Models (ESM) have been used to study the NECB by accounting for biophysical processes. However, it is only in the 2000s that these models began to incorportate the full complexity of the C cycle and its interactions with the other biophysical components (Fisher and Koven, 2020). Since then, significant progress has been made in modeling approaches and data assimilation techniques to improve Arctic-boreal C cycling representation. The development of more sophisticated ESM that incorporate permafrost dynamics, coupled carbon-nitrogen cycles, and improved representations of Arctic vegetation has enhanced our ability to project future changes in the NECB. Specifically, ESM have been refined to explicitly represent key processes specific to high-latitude ecosystems, such as permafrost dynamics (Guimberteau et al., 2018; Chaudhary et al., 2020), snow insulation effects (Wang et al., 2013; Pongracz et al., 2021; Charbit et al., 2024), Sphagnum dominated peatland ecosystems (Qiu et al., 2022), vegetation shifts (van den Hurk et al., 2016) and lateral transfer of C from land to rivers (Bowring et al., 2019; Bowring et al., 2020). The incorporation of microbial dynamics and soil organic matter decomposition models has improved simulations of soil C responses to warming (Huang et al., 2021).
Data assimilation techniques have evolved to better integrate diverse observational datasets with model simulations and ultimately allowing for better constraints on regional and pan-Arctic C budgets. For example, the Carbon data model Framework (CARDAMOM) (López-Blanco et al., 2019; Hugelius et al., 2024) or the Carbon Cycle Data Assimilation System (CCDAS) (Kemp et al., 2014; Scholze et al., 2019) now incorporate a wide range of observations, including atmospheric CO2 concentrations, satellite-derived vegetation indices, soil organic C, plant biomass, burned area, and forest loss to provide more accurate estimates of C fluxes, stocks, and transit times and quantify their uncertainties.
Moreover, machine learning approaches, such as neural networks and random forests, have been increasingly used to upscale site-level flux measurements to regional and pan-Arctic scales, providing new insights into spatial patterns and drivers of C fluxes (Väisänen et al., 2014; Peltola et al., 2019; Virkkala et al., 2021; Yao et al., 2021; McNicol et al., 2023; Nelson et al., 2024). Additionally, the development of benchmarking systems that use multiple observational constraints has enhanced our ability to evaluate and improve Earth System Models for high-latitude regions (Collier et al., 2018; Hou et al., 2023).
In situ data are also crucial for the calibration and validation of process-based models (Le Noë et al., 2023). Specifics of Arctic regions promoted sensitivity model analysis studies assessing models’ capacity in simulating Arctic ecosystems (Dantec-Nédélec et al., 2017; Pongracz et al., 2021). Today, more and more models are employing harmonized monitoring databases with statistical optimisation approaches such Bayesian methods and history matching (Salmon et al., 2022; Bacour et al., 2023; McNeall et al., 2024), among others, to calibrate model parameters at regional or global scales and to pinpoint model weaknesses. Additionally, in situ data synthesis and meta-analysis are guiding modellers to assess model development priority that aims to reduce model uncertainty or to enhance physical process representation.
Despite the recent advancements in our understanding of C cycling dynamics, significant uncertainties persist, particularly in permafrost regions. The release of CO2, CH4, and N2O, and also lateral DOC transport from thawing permafrost represents a critical yet poorly constrained component of the global C budget. Climate change hydrologically activates different layers of Arctic soils, potentially triggering new C processes. The recently published permafrost RECCAP2 update, following an initial budgeting effort (McGuire et al., 2012), aimed to address these uncertainties by synthesizing two decades of observations and modeling efforts (Hugelius et al., 2024; Ramage et al., 2024). Insights from RECCAP2 highlight the complexity of permafrost region GHG dynamics and underscore the need for improved monitoring and modeling approaches to accurately quantify their contribution to atmospheric GHG concentrations. Two important take-home messages have been found: First, there are large discrepancies between bottom-up and top-down estimates (Hugelius et al., 2024) - Bottom-up approaches (data-driven upscaling and process-based models) generally estimate higher land-to-atmosphere fluxes for all GHGs compared to top-down approaches (atmospheric inversions). This points to fundamental differences in methodologies that need to be reconciled. From a modeling perspective, priorities for future research include improved representation of inland water ecosystems including rivers, lakes, reservoirs and materials lateral transfer, and disturbances like fire dynamics and abrupt permafrost thaw in process-based models, and the compilation of process-based model ensembles for CH4 and N2O (Hugelius et al., 2024). The increased complexity of models, driven by the explicit representation of processes, is both challenging and essential to accurately capture the spatial heterogeneity and temporal dynamics of NECB. Second, there is a need for more and better well-distributed in-situ data coverage - there are significant gaps in spatial and temporal coverage of in situ GHG measurements, especially for winter and shoulder seasons (Ramage et al., 2024). On a related note, the ongoing geopolitical conflict and war between Russia and Ukraine have severely deteriorated our ability to study and understand not only current but also future pan-Arctic changes (López-Blanco et al., 2024).
The northward advancement of the tree- (Harsch et al., 2009) and shrub- (Myers-Smith and Hik, 2018) lines in the boreal-Arctic transition zone represents two significant ecological processes driven by climate warming, with implications for vegetation composition, surface albedo, and C dynamics. These shifts can enhance aboveground C storage but may also reduce surface albedo, as darker canopies replace tundra vegetation, thereby amplifying regional warming through feedback mechanisms (Sturm et al., 2005; Bjorkman et al., 2018; Schmidt et al., 2024). However, the advancement of tree and shrub lines is neither uniform nor as rapid as anticipated (Myers-Smith et al., 2011; Rees et al., 2020), due to local factors such as nutrient availability, soil conditions, and herbivory, which further modulate these processes. For example, nitrogen limitation in Arctic soils has been shown to constrain tree growth despite warming (Körner and Paulsen, 2004). Moreover, shifts in vegetation composition can alter soil organic C dynamics and decomposition rates, influencing net C balance (Natali et al., 2019). Herbivory also plays a significant role by altering vegetation structure and soil properties; for example, reindeer grazing can reduce shrub density and limit C uptake, further effecting ecosystem C storage (Koltz et al., 2022).
At a local-to-regional scale, addressing these uncertainties will require expanded and comparable long-term monitoring measurements, continued data synthesis efforts for CO2, CH4 and N2O (e.g., , improved resolution in upscaling techniques (Ramage et al., 2024), and ultimately advancing integration between field observations, remote sensing data, and numerical models to more effectively constrain previously unconstrained ecosystem processes (Hugelius et al., 2024). Reducing these uncertainties is critical for accurately quantifying the contemporary and future GHG budgets of the permafrost region.
This paper highlights the critical importance of Arctic and boreal ecosystems in the global C cycle and their vulnerability to rapid climate change. Our review of the key NECB components, methodological advances, and remaining uncertainties, emphasizes the critical need to address and quantify uncertainties in GHG budgeting for permafrost regions and provides several key insights for future research and policy directions:
1. Integrated monitoring approaches: There is a pressing need for more comprehensive, year-round monitoring networks that integrate multiple NECB components, including CO2 and CH4 fluxes, lateral C transport, and disturbance impacts. Long-term, catchment-scale studies like the one flagged in Zackenberg Valley offer valuable models for future research efforts.
2. Focus on understudied components: Greater attention should be given to quantifying and understanding the roles of winter fluxes, lateral transport of C, disturbance regimes, and herbivore interactions in the NECB of Arctic-boreal ecosystems. This will likely help reduce discrepancies between bottom-up and top-down GHG estimates.
3. Advancing methodologies: Reducing uncertainties in NECB assessments requires the adoption of state-of-the-art technologies and methods. For example, this includes deploying high-resolution GHG analyzers that can operate in extreme Arctic winters and remote areas with limited power supply, using high-resolution remote sensing tools to improve upscaling capabilities, and integrating isotopic/radioactive tracing techniques to better understand the sources and ages of transported C. Additionally, heavily data-constrained modeling approaches and innovative field experiments are crucial to quantifying and disentangling the individual processes shaping the Arctic-boreal C budget.
4. Interdisciplinary collaboration: Addressing the complex challenges of Arctic C cycling requires increased collaboration across disciplines, including ecology, biogeochemistry, hydrology, and climate science and across approaches, including measurements, remote sensing and modeling. This interdisciplinary approach is essential for developing a holistic understanding of NECB dynamics.
5. Policy and collaboration frameworks: Strengthening NECB research within the context of the Arctic Council, integrating the AMAP and CAFF agendas, is vital. This includes fostering collaboration between local data-model initiatives and pan-Arctic networks, encouraging the development of holistic, site-specific programs with broader regional relevance, and aligning these efforts with international climate goals.
By addressing these key areas, researchers can significantly enhance our ability to predict and mitigate the impacts of climate change on Arctic and boreal C cycles. This improved understanding will be crucial for informing effective climate policy, ecosystem management strategies, and global climate change mitigation efforts in these rapidly changing northern landscapes.
EL-B: Conceptualization, Methodology, Visualization, Writing – original draft, Writing – review and editing. MV: Writing – review and editing, Investigation, Resources. ES: Writing – review and editing, Investigation, Resources. CJ: Writing – review and editing, Investigation, Resources. NS: Writing – review and editing, Investigation, Resources. HM: Writing – review and editing, Investigation, Resources. AL: Writing – review and editing, Investigation, Resources. SJ: Writing – review and editing, Investigation, Resources. JS: Visualization, Writing – review and editing, Investigation, Resources. TC: Conceptualization, Writing – review and editing, Investigation, Resources.
The author(s) declare that financial support was received for the research and/or publication of this article. This work was financially supported by the Danish Energy Agency for the joint AMAP/CAFF initiative (grant no. TAS 4005–520975). This work is a contribution to NordForsk project NordBorN (grant no. 164079), which also includes contributions from ELB and NMS. ELB, ES, and TRC considers this study a contribution to GreenFeedBack (Greenhouse gas fluxes and earth system feedbacks) funded by the European Union’s HORIZON research and innovation program under grant agreement No 101056921. More here AL, JS and ES acknowledge the projects “Upgrading knowledge and solutions to fast-track wetland restoration across Europe” (WetHorizon) project (no. 101056848) from the European Union’s Horizon Europe Framework Programme for Research and Innovation; “Peatland restoration for greenhouse gas emission reduction and carbon sequestration in the Baltic Sea region” (EU LIFE + LIFE21-CCM-LV-LIFE PeatCarbon, no. 101074396) funded by the European Union; and “Sustainable growth for Northern Ostrobothnia–the monitoring system for green transition (VISIO)” partly funded by the European Commission Just Transition Fund, through Council of Oulu Region (2021/900320/09).
We thank the Arctic Council Working Groups AMAP (Arctic Monitoring and Assessment Program) and CAFF (Conservation of Arctic Flora and Fauna) as well as Greenland Ecosystem Monitoring for their continued efforts to unravel high latitude ecosystem dynamics.
The authors declare that the research was conducted in the absence of any commercial or financial relationships that could be construed as a potential conflict of interest.
The author(s) declare that no Generative AI was used in the creation of this manuscript.
All claims expressed in this article are solely those of the authors and do not necessarily represent those of their affiliated organizations, or those of the publisher, the editors and the reviewers. Any product that may be evaluated in this article, or claim that may be made by its manufacturer, is not guaranteed or endorsed by the publisher.
Ahonen, S. H. K., Ylänne, H., Väisänen, M., Ruotsalainen, A. L., Männistö, M. k., Markkola, A., et al. (2021). Reindeer grazing history determines the responses of subarctic soil fungal communities to warming and fertilization. New Phytol. 232, 788–801. doi:10.1111/nph.17623
Alm, J., Schulman, L., Walden, J., Nykänen, H., Martikainen, P. J., and Silvola, J. (1999). Carbon balance of a boreal bog during a year with an exceptionally dry summer. Ecology 80, 161–174. doi:10.2307/176987
Amap (2015). AMAP assessment 2015: methane as an arctic climate forcer. Arct. Monit. Assess. Programme (AMAP).
Amap (2022). Arctic climate change update 2021: key trends and impacts. Arctic monitoring and assessment Programme (AMAP). Tromsø, Nor. viii+148pp.
Aurela, M., Riutta, T., Laurila, T., Tuovinen, J.-P., Vesala, T., Tuittila, E.-S., et al. (2007). CO2 exchange of a sedge fen in southern Finland—the impact of a drought period. Tellus B 59, 826–837. doi:10.3402/tellusb.v59i5.17062
Bacour, C., Macbean, N., Chevallier, F., Léonard, S., Koffi, E. N., and Peylin, P. (2023). Assimilation of multiple datasets results in large differences in regional-to global-scale NEE and GPP budgets simulated by a terrestrial biosphere model. Biogeosciences 20, 1089–1111. doi:10.5194/bg-20-1089-2023
Baldocchi, D. D. (2003). Assessing the eddy covariance technique for evaluating carbon dioxide exchange rates of ecosystems: past, present and future. Glob. Change Biol. 9, 479–492. doi:10.1046/j.1365-2486.2003.00629.x
Barbero-Palacios, L., Barrio, I. C., García Criado, M., Kater, I., Petit Bon, M., Kolari, T. H. M., et al. (2024). Herbivore diversity effects on Arctic tundra ecosystems: a systematic review. Environ. Evid. 13, 6. doi:10.1186/s13750-024-00330-9
Beel, C. R., Heslop, J. K., Orwin, J. F., Pope, M. A., Schevers, A. J., Hung, J. K. Y., et al. (2021). Emerging dominance of summer rainfall driving High Arctic terrestrial-aquatic connectivity. Nat. Commun. 12, 1448. doi:10.1038/s41467-021-21759-3
Bernhardt, E. S., Blaszczak, J. R., Ficken, C. D., Fork, M. L., Kaiser, K. E., and Seybold, E. C. (2017). Control points in ecosystems: moving beyond the hot spot hot moment concept. Ecosystems 20, 665–682. doi:10.1007/s10021-016-0103-y
Bintanja, R., and Andry, O. (2017). Towards a rain-dominated Arctic. Nat. Clim. Change 7, 263–267. doi:10.1038/nclimate3240
Bintanja, R., Van Der Wiel, K., Van Der Linden, E. C., Reusen, J., Bogerd, L., Krikken, F., et al. (2020). Strong future increases in Arctic precipitation variability linked to poleward moisture transport. Sci. Adv. 6, eaax6869. doi:10.1126/sciadv.aax6869
Bjorkman, A. D., Myers-Smith, I. H., Elmendorf, S. C., Normand, S., Rüger, N., Beck, P. S. A., et al. (2018). Plant functional trait change across a warming tundra biome. Nature 562, 57–62. doi:10.1038/s41586-018-0563-7
Bolek, A., Heimann, M., and Göckede, M. (2024). UAV-based in situ measurements of CO2 and CH4 fluxes over complex natural ecosystems. Atmos. Meas. Tech. 17, 5619–5636. doi:10.5194/amt-17-5619-2024
Bond-Lamberty, B., and Thomson, A. (2010). Temperature-associated increases in the global soil respiration record. Nature 464, 579–582. doi:10.1038/nature08930
Bowring, S. P. K., Lauerwald, R., Guenet, B., Zhu, D., Guimberteau, M., Regnier, P., et al. (2020). ORCHIDEE MICT-LEAK (r5459), a global model for the production, transport, and transformation of dissolved organic carbon from Arctic permafrost regions – Part 2: model evaluation over the Lena River basin. Geosci. Model Dev. 13, 507–520. doi:10.5194/gmd-13-507-2020
Bowring, S. P. K., Lauerwald, R., Guenet, B., Zhu, D., Guimberteau, M., Tootchi, A., et al. (2019). ORCHIDEE MICT-LEAK (r5459), a global model for the production, transport, and transformation of dissolved organic carbon from Arctic permafrost regions – Part 1: rationale, model description, and simulation protocol. Geosci. Model Dev. 12, 3503–3521. doi:10.5194/gmd-12-3503-2019
Box, J. E., Colgan, W. T., Christensen, T. R., Schmidt, N. M., Lund, M., Parmentier, F.-J. W., et al. (2019). Key indicators of Arctic climate change: 1971–2017. Environ. Res. Lett. 14, 045010. doi:10.1088/1748-9326/aafc1b
Bretz, K. A., Jackson, A. R., Rahman, S., Monroe, J. M., and Hotchkiss, E. R. (2021). Integrating ecosystem patch contributions to stream corridor carbon dioxide and methane fluxes. J. Geophys. Res. Biogeosciences 126, e2021JG006313. doi:10.1029/2021jg006313
Burba, G. (2013). Eddy covariance Method for scientific, industrial, Agricultural and regulatory applications: a field Book on measuring ecosystem gas Exchange and areal emission rates. Lincoln, Nebraska: LI-COR biosciences.
Byrne, B., Liu, J., Bowman, K. W., Pascolini-Campbell, M., Chatterjee, A., Pandey, S., et al. (2024). Carbon emissions from the 2023 Canadian wildfires. Nature 633, 835–839. doi:10.1038/s41586-024-07878-z
Cahoon, S. M. P., Sullivan, P. F., Post, E., and Welker, J. M. (2012). Large herbivores limit CO2 uptake and suppress carbon cycle responses to warming in West Greenland. Glob. Change Biol. 18, 469–479. doi:10.1111/j.1365-2486.2011.02528.x
Callaghan, T. V., Tweedie, C. E., Åkerman, J., Andrews, C., Bergstedt, J., Butler, M. G., et al. (2011). Multi-decadal changes in tundra environments and ecosystems: synthesis of the international polar year-back to the future project (IPY-btf). AMBIO 40, 705–716. doi:10.1007/s13280-011-0179-8
Carvalhais, N., Forkel, M., Khomik, M., Bellarby, J., Jung, M., Migliavacca, M., et al. (2014). Global covariation of carbon turnover times with climate in terrestrial ecosystems. Nature 514, 213–217. doi:10.1038/nature13731
Casas-Ruiz, J. P., Bodmer, P., Bona, K. A., Butman, D., Couturier, M., Emilson, E. J. S., et al. (2023). Integrating terrestrial and aquatic ecosystems to constrain estimates of land-atmosphere carbon exchange. Nat. Commun. 14, 1571. doi:10.1038/s41467-023-37232-2
Chapin Iii, F. S., Mcguire, A. D., Randerson, J., Pielke, R., Baldocchi, D., Hobbie, S. E., et al. (2000). Arctic and boreal ecosystems of western North America as components of the climate system. Glob. Change Biol. 6, 211–223. doi:10.1046/j.1365-2486.2000.06022.x
Charbit, S., Dumas, C., Maignan, F., Ottlé, C., Raoult, N., Fettweis, X., et al. (2024). Modelling snowpack on ice surfaces with the ORCHIDEE land surface model: application to the Greenland ice sheet. Cryosphere 18, 5067–5099. doi:10.5194/tc-18-5067-2024
Chaudhary, N., Westermann, S., Lamba, S., Shurpali, N., Sannel, A. B. K., Schurgers, G., et al. (2020). Modelling past and future peatland carbon dynamics across the pan-Arctic. Glob. Change Biol. 26, 4119–4133. doi:10.1111/gcb.15099
Christensen, T. R., Johansson, T., Olsrud, M., Ström, L., Lindroth, A., Mastepanov, M., et al. (2007). A catchment-scale carbon and greenhouse gas budget of a subarctic landscape. Philosophical Trans. R. Soc. A Math. Phys. Eng. Sci. 365, 1643–1656. doi:10.1098/rsta.2007.2035
Christensen, T. R., Lund, M., Skov, K., Abermann, J., López-Blanco, E., Scheller, J., et al. (2020). Multiple ecosystem effects of extreme weather events in the arctic. Ecosystems 24, 122–136. doi:10.1007/s10021-020-00507-6
Christensen, T. R., Topp-Jørgensen, E., Sejr, M. K., and Schmidt, N. M. (2017). Foreword: synthesis of the Greenland ecosystem monitoring program. Ambio 46, 1–2. doi:10.1007/s13280-016-0860-z
Ciais, P., Bastos, A., Chevallier, F., Lauerwald, R., Poulter, B., Canadell, J. G., et al. (2022). Definitions and methods to estimate regional land carbon fluxes for the second phase of the REgional Carbon Cycle Assessment and Processes Project (RECCAP-2). Geosci. Model Dev. 15, 1289–1316. doi:10.5194/gmd-15-1289-2022
Clark, J. A., Tape, K. D., Baskaran, L., Elder, C., Miller, C., Miner, K., et al. (2023). Do beaver ponds increase methane emissions along Arctic tundra streams? Environ. Res. Lett. 18, 075004. doi:10.1088/1748-9326/acde8e
Collier, N., Hoffman, F. M., Lawrence, D. M., Keppel-Aleks, G., Koven, C. D., Riley, W. J., et al. (2018). The international land model benchmarking (ILAMB) system: design, theory, and implementation. J. Adv. Model. Earth Syst. 10, 2731–2754. doi:10.1029/2018ms001354
Commane, R., Lindaas, J., Benmergui, J., Luus, K. A., Chang, R.Y.-W., Daube, B. C., et al. (2017). Carbon dioxide sources from Alaska driven by increasing early winter respiration from Arctic tundra. Proc. Natl. Acad. Sci. U. S. A. 114, 5361–5366. doi:10.1073/pnas.1618567114
Croghan, D., Ala-Aho, P., Welker, J., Mustonen, K. R., Khamis, K., Hannah, D. M., et al. (2024). Seasonal and interannual dissolved organic carbon transport process dynamics in a subarctic headwater catchment revealed by high-resolution measurements. Hydrol. Earth Syst. Sci. 28, 1055–1070. doi:10.5194/hess-28-1055-2024
Dantec-Nédélec, S., Ottlé, C., Wang, T., Guglielmo, F., Maignan, F., Delbart, N., et al. (2017). Testing the capability of ORCHIDEE land surface model to simulate Arctic ecosystems: sensitivity analysis and site-level model calibration. J. Adv. Model. Earth Syst. 9, 1212–1230. doi:10.1002/2016ms000860
Dean, J. F., Meisel, O. H., Martyn Rosco, M., Marchesini, L. B., Garnett, M. H., Lenderink, H., et al. (2020). East Siberian Arctic inland waters emit mostly contemporary carbon. Nat. Commun. 11, 1627. doi:10.1038/s41467-020-15511-6
Drake, T. W., Raymond, P. A., and Spencer, R. G. M. (2018). Terrestrial carbon inputs to inland waters: a current synthesis of estimates and uncertainty. Limnol. Oceanogr. Lett. 3, 132–142. doi:10.1002/lol2.10055
Euskirchen, E. S., Mcguire, A. D., Kicklighter, D. W., Zhuang, Q., Clein, J. S., Dargaville, R. J., et al. (2006). Importance of recent shifts in soil thermal dynamics on growing season length, productivity, and carbon sequestration in terrestrial high-latitude ecosystems. Glob. Change Biol. 12, 731–750. doi:10.1111/j.1365-2486.2006.01113.x
Falk, J. M., Schmidt, N. M., Christensen, T. R., and Ström, L. (2015). Large herbivore grazing affects the vegetation structure and greenhouse gas balance in a high arctic mire. Environ. Res. Lett. 10, 045001. doi:10.1088/1748-9326/10/4/045001
Fisher, R. A., and Koven, C. D. (2020). Perspectives on the future of land surface models and the challenges of representing complex terrestrial systems. J. Adv. Model. Earth Syst. 12, e2018MS001453. doi:10.1029/2018ms001453
Foster, A. C., Wang, J. A., Frost, G. V., Davidson, S. J., Hoy, E., Turner, K. W., et al. (2022). Disturbances in North American boreal forest and Arctic tundra: impacts, interactions, and responses. Environ. Res. Lett. 17, 113001. doi:10.1088/1748-9326/ac98d7
Francini, G., Liiri, M., Männistö, M., Stark, S., and Kytöviita, M.-M. (2014). Response to reindeer grazing removal depends on soil characteristics in low Arctic meadows. Appl. Soil Ecol. 76, 14–25. doi:10.1016/j.apsoil.2013.12.003
Frank, D., Reichstein, M., Bahn, M., Thonicke, K., Frank, D., Mahecha, M. D., et al. (2015). Effects of climate extremes on the terrestrial carbon cycle: concepts, processes and potential future impacts. Glob. Change Biol. 21, 2861–2880. doi:10.1111/gcb.12916
Frolking, S., and Roulet, N. T. (2007). Holocene radiative forcing impact of northern peatland carbon accumulation and methane emissions. Glob. Change Biol. 13, 1079–1088. doi:10.1111/j.1365-2486.2007.01339.x
Göckede, M., Kwon, M. J., Kittler, F., Heimann, M., Zimov, N., and Zimov, S. (2019). Negative feedback processes following drainage slow down permafrost degradation. Glob. Change Biol. 25, 3254–3266. doi:10.1111/gcb.14744
Gómez-Gener, L., Hotchkiss, E. R., Laudon, H., and Sponseller, R. A. (2021). Integrating discharge-concentration dynamics across carbon forms in a boreal landscape. Water Resour. Res. 57, e2020WR028806. doi:10.1029/2020wr028806
Guenet, B., Orliac, J., Cécillon, L., Torres, O., Sereni, L., Martin, P. A., et al. (2024). Spatial biases reduce the ability of Earth system models to simulate soil heterotrophic respiration fluxes. Biogeosciences 21, 657–669. doi:10.5194/bg-21-657-2024
Guimberteau, M., Zhu, D., Maignan, F., Huang, Y., Yue, C., Dantec-Nédélec, S., et al. (2018). ORCHIDEE-MICT (v8.4.1), a land surface model for the high latitudes: model description and validation. Geosci. Model Dev. 11, 121–163. doi:10.5194/gmd-11-121-2018
Harsch, M. A., Hulme, P. E., Mcglone, M. S., and Duncan, R. P. (2009). Are treelines advancing? A global meta-analysis of treeline response to climate warming. Ecol. Lett. 12, 1040–1049. doi:10.1111/j.1461-0248.2009.01355.x
Heggenes, J., Odland, A., Chevalier, T., Ahlberg, J., Berg, A., Larsson, H., et al. (2017). Herbivore grazing—or trampling? Trampling effects by a large ungulate in cold high-latitude ecosystems. Ecol. Evol. 7, 6423–6431. doi:10.1002/ece3.3130
Helbig, M., Živković, T., Alekseychik, P., Aurela, M., El-Madany, T. S., Euskirchen, E. S., et al. (2022). Warming response of peatland CO2 sink is sensitive to seasonality in warming trends. Nat. Clim. Change 12, 743–749. doi:10.1038/s41558-022-01428-z
Heliasz, M., Johansson, T., Lindroth, A., Mölder, M., Mastepanov, M., Friborg, T., et al. (2011). Quantification of C uptake in subarctic birch forest after setback by an extreme insect outbreak. Geophys. Res. Lett. 38, n/a. doi:10.1029/2010gl044733
Henry, G. H. R., Hollister, R. D., Klanderud, K., Björk, R. G., Bjorkman, A. D., Elphinstone, C., et al. (2022). The International Tundra Experiment (ITEX): 30 years of research on tundra ecosystems. Arct. Sci. 8, 550–571. doi:10.1139/as-2022-0041
Hou, E., Ma, S., Huang, Y., Zhou, Y., Kim, H.-S., López-Blanco, , et al. (2022). Across-model spread and shrinking in predicting peatland carbon dynamics under global change. Global Change Biology 29, 2759–2775.
Huang, Y., Guenet, B., Wang, Y. L., and Ciais, P. (2021). Global simulation and evaluation of soil organic matter and microbial carbon and nitrogen stocks using the microbial decomposition model ORCHIMIC v2.0. Glob. Biogeochem. Cycles 35, e2020GB006836. doi:10.1029/2020gb006836
Hugelius, G., Ramage, J., Burke, E., Chatterjee, A., Smallman, T. L., Aalto, T., et al. (2024). Permafrost region greenhouse gas budgets suggest a weak CO2 sink and CH4 and N2O sources, but magnitudes differ between top-down and bottom-up methods. Glob. Biogeochem. Cycles 38, e2023GB007969. doi:10.1029/2023gb007969
Hugelius, G., Strauss, J., Zubrzycki, S., Harden, J. W., Schuur, E. a.G., Ping, C. L., et al. (2014). Estimated stocks of circumpolar permafrost carbon with quantified uncertainty ranges and identified data gaps. Biogeosciences 11, 6573–6593. doi:10.5194/bg-11-6573-2014
Ito, A., Li, T., Qin, Z., Melton, J. R., Tian, H., Kleinen, T., et al. (2023). Cold-season methane fluxes simulated by GCP-CH4 models. Geophys. Res. Lett. 50, e2023GL103037. doi:10.1029/2023gl103037
Jentzsch, K., Männistö, E., Marushchak, M. E., Korrensalo, A., Van Delden, L., Tuittila, E. S., et al. (2024). Shoulder season controls on methane emissions from a boreal peatland. Biogeosciences 21, 3761–3788. doi:10.5194/bg-21-3761-2024
Juutinen, S., Väliranta, M., Kuutti, V., Laine, A. M., Virtanen, T., Seppä, H., et al. (2013). Short-term and long-term carbon dynamics in a northern peatland-stream-lake continuum: a catchment approach. J. Geophys. Res. Biogeosciences 118, 171–183. doi:10.1002/jgrg.20028
Kemp, S., Scholze, M., Ziehn, T., and Kaminski, T. (2014). Limiting the parameter space in the carbon cycle data assimilation system (CCDAS). Geosci. Model Dev. 7, 1609–1619. doi:10.5194/gmd-7-1609-2014
Kling, G. W., Kipphut, G. W., and Miller, M. C. (1991). Arctic lakes and streams as gas conduits to the atmosphere: implications for tundra carbon budgets. Science 251, 298–301. doi:10.1126/science.251.4991.298
Knox, S. H., Jackson, R. B., Poulter, B., Mcnicol, G., Fluet-Chouinard, E., Zhang, Z., et al. (2019). FLUXNET-CH4 synthesis activity: objectives, observations, and future directions. Bull. Am. Meteorological Soc. 100, 2607–2632. doi:10.1175/bams-d-18-0268.1
Kokelj, S. V., Lacelle, D., Lantz, T. C., Tunnicliffe, J., Malone, L., Clark, I. D., et al. (2013). Thawing of massive ground ice in mega slumps drives increases in stream sediment and solute flux across a range of watershed scales. J. Geophys. Res. Earth Surf. 118, 681–692. doi:10.1002/jgrf.20063
Koltz, A. M., Gough, L., and Mclaren, J. R. (2022). Herbivores in Arctic ecosystems: effects of climate change and implications for carbon and nutrient cycling. Ann. N. Y. Acad. Sci. 1516, 28–47. doi:10.1111/nyas.14863
Korkiakoski, M., Ojanen, P., Penttilä, T., Minkkinen, K., Sarkkola, S., Rainne, J., et al. (2020). Impact of partial harvest on CH4 and N2O balances of a drained boreal peatland forest. Agric. For. Meteorology 295, 108168. doi:10.1016/j.agrformet.2020.108168
Korkiakoski, M., Tuovinen, J. P., Aurela, M., Koskinen, M., Minkkinen, K., Ojanen, P., et al. (2017). Methane exchange at the peatland forest floor – automatic chamber system exposes the dynamics of small fluxes. Biogeosciences 14, 1947–1967. doi:10.5194/bg-14-1947-2017
Körner, C., and Paulsen, J. (2004). A world-wide study of high altitude treeline temperatures. J. Biogeogr. 31, 713–732. doi:10.1111/j.1365-2699.2003.01043.x
Koskinen, M., Minkkinen, K., Ojanen, P., Kämäräinen, M., Laurila, T., and Lohila, A. (2014). Measurements of CO2 exchange with an automated chamber system throughout the year: challenges in measuring night-time respiration on porous peat soil. Biogeosciences 11, 347–363. doi:10.5194/bg-11-347-2014
Köster, K., Köster, E., Kulmala, L., Berninger, F., and Pumpanen, J. (2017). Are the climatic factors combined with reindeer grazing affecting the soil CO2 emissions in subarctic boreal pine forest? CATENA 149, 616–622. doi:10.1016/j.catena.2016.06.011
Koven, C. D., Ringeval, B., Friedlingstein, P., Ciais, P., Cadule, P., Khvorostyanov, D., et al. (2011). Permafrost carbon-climate feedbacks accelerate global warming. Proc. Natl. Acad. Sci. 108, 14769–14774. doi:10.1073/pnas.1103910108
Kuhn, M. A., Varner, R. K., Bastviken, D., Crill, P., Macintyre, S., Turetsky, M., et al. (2021). BAWLD-CH4: a comprehensive dataset of methane fluxes from boreal and arctic ecosystems. Earth Syst. Sci. Data 13, 5151–5189. doi:10.5194/essd-13-5151-2021
Lara, M. J., Johnson, D. R., Andresen, C., Hollister, R. D., and Tweedie, C. E. (2017). Peak season carbon exchange shifts from a sink to a source following 50+ years of herbivore exclusion in an Arctic tundra ecosystem. J. Ecol. 105, 122–131. doi:10.1111/1365-2745.12654
Le Noë, J., Manzoni, S., Abramoff, R., Bölscher, T., Bruni, E., Cardinael, R., et al. (2023). Soil organic carbon models need independent time-series validation for reliable prediction. Commun. Earth and Environ. 4, 158. doi:10.1038/s43247-023-00830-5
Le Quéré, C., Andrew, R. M., Canadell, J. G., Sitch, S., Korsbakken, J. I., Peters, G. P., et al. (2016). Global carbon budget 2016. Earth Syst. Sci. Data 8, 605–649. doi:10.5194/essd-8-605-2016
Lewkowicz, A. G., and Way, R. G. (2019). Extremes of summer climate trigger thousands of thermokarst landslides in a High Arctic environment. Nat. Commun. 10, 1329. doi:10.1038/s41467-019-09314-7
López-Blanco, E., Exbrayat, J. F., Lund, M., Christensen, T. R., Tamstorf, M. P., Slevin, D., et al. (2019). Evaluation of terrestrial pan-Arctic carbon cycling using a data-assimilation system. Earth Syst. Dynam. 10, 233–255. doi:10.5194/esd-10-233-2019
López-Blanco, E., Jackowicz-Korczynski, M. A., Mastepanov, M., Skov, K., Westergaard-Nielsen, A., Williams, M., et al. (2020). Multi-year data-model evaluation reveals the importance of nutrient availability over climate in arctic ecosystem C dynamics. Environ. Res. Lett. doi:10.1088/1748-9326/ab865b
López-Blanco, E., Lund, M., Williams, M., Tamstorf, M. P., Westergaard-Nielsen, A., Exbrayat, J. F., et al. (2017). Exchange of CO2 in Arctic tundra: impacts of meteorological variations and biological disturbance. Biogeosciences 14, 4467–4483. doi:10.5194/bg-14-4467-2017
López-Blanco, E., Topp-Jørgensen, E., Christensen, T. R., Rasch, M., Skov, H., Arndal, M. F., et al. (2024). Towards an increasingly biased view on Arctic change. Nat. Clim. Change 14, 152–155. doi:10.1038/s41558-023-01903-1
Lund, M., Raundrup, K., Westergaard-Nielsen, A., López-Blanco, E., Nymand, J., and Aastrup, P. (2017). Larval outbreaks in West Greenland: instant and subsequent effects on tundra ecosystem productivity and CO(2) exchange. Ambio 46, 26–38. doi:10.1007/s13280-016-0863-9
Mack, M. C., Bret-Harte, M. S., Hollingsworth, T. N., Jandt, R. R., Schuur, E. a.G., Shaver, G. R., et al. (2011). Carbon loss from an unprecedented Arctic tundra wildfire. Nature 475, 489–492. doi:10.1038/nature10283
Martens, J., Wild, B., Semiletov, I., Dudarev, O. V., and Gustafsson, Ö. (2022). Circum-Arctic release of terrestrial carbon varies between regions and sources. Nat. Commun. 13, 5858. doi:10.1038/s41467-022-33541-0
Mastepanov, M., Sigsgaard, C., Dlugokencky, E. J., Houweling, S., Strom, L., Tamstorf, M. P., et al. (2008). Large tundra methane burst during onset of freezing. Nature 456, 628–630. doi:10.1038/nature07464
Mastepanov, M., Sigsgaard, C., Tagesson, T., Ström, L., Tamstorf, M. P., Lund, M., et al. (2013). Revisiting factors controlling methane emissions from high-Arctic tundra, Biogeosciences, 10, 5139–5158. doi:10.5194/bg-10-5139-2013
Mcguire, A. D., Anderson, L. G., Christensen, T. R., Dallimore, S., Guo, L., Hayes, D. J., et al. (2009). Sensitivity of the carbon cycle in the Arctic to climate change. Ecol. Monogr. 79, 523–555. doi:10.1890/08-2025.1
Mcguire, A. D., Christensen, T. R., Hayes, D., Heroult, A., Euskirchen, E., Kimball, J. S., et al. (2012). An assessment of the carbon balance of Arctic tundra: comparisons among observations, process models, and atmospheric inversions. Biogeosciences 9, 3185–3204. doi:10.5194/bg-9-3185-2012
Mcneall, D., Robertson, E., and Wiltshire, A. (2024). Constraining the carbon cycle in JULES-ES-1.0. Geosci. Model Dev. 17, 1059–1089. doi:10.5194/gmd-17-1059-2024
Mcnicol, G., Fluet-Chouinard, E., Ouyang, Z., Knox, S., Zhang, Z., Aalto, T., et al. (2023). Upscaling wetland methane emissions from the FLUXNET-CH4 eddy covariance network (UpCH4 v1.0): model development, network assessment, and budget comparison. AGU Adv. 4, e2023AV000956. doi:10.1029/2023av000956
Mishra, U., Hugelius, G., Shelef, E., Yang, Y., Strauss, J., Lupachev, A., et al. (2021). Spatial heterogeneity and environmental predictors of permafrost region soil organic carbon stocks. Sci. Adv. 7, eaaz5236. doi:10.1126/sciadv.aaz5236
Mosbacher, J. B., Michelsen, A., Stelvig, M., Hjermstad-Sollerud, H., and Schmidt, N. M. (2019). Muskoxen modify plant abundance, phenology, and nitrogen dynamics in a high arctic fen. Ecosystems 22, 1095–1107. doi:10.1007/s10021-018-0323-4
Mustonen, K.-R., Marttila, H., Lehosmaa, K., Chapman, J., Juutinen, S., Koivunen, I., et al. (2024). From thaw till fall: interacting hydrology, carbon cycle, and greenhouse gas dynamics in a subarctic stream-lake continuum. Sci. Total Environ. 957, 177434. doi:10.1016/j.scitotenv.2024.177434
Myers-Smith, I. H., Forbes, B. C., Wilmking, M., Hallinger, M., Lantz, T., Blok, D., et al. (2011). Shrub expansion in tundra ecosystems: dynamics, impacts and research priorities. Environ. Res. Lett. 6, 045509. doi:10.1088/1748-9326/6/4/045509
Myers-Smith, I. H., and Hik, D. S. (2018). Climate warming as a driver of tundra shrubline advance. J. Ecol. 106, 547–560. doi:10.1111/1365-2745.12817
Natali, S. M., Schuur, E. a.G., Mauritz, M., Schade, J. D., Celis, G., Crummer, K. G., et al. (2015). Permafrost thaw and soil moisture driving CO2 and CH4 release from upland tundra. J. Geophys. Res. Biogeosciences 120, 525–537. doi:10.1002/2014jg002872
Natali, S. M., Watts, J. D., Rogers, B. M., Potter, S., Ludwig, S. M., Selbmann, A.-K., et al. (2019). Large loss of CO2 in winter observed across the northern permafrost region. Nat. Clim. Change 9, 852–857. doi:10.1038/s41558-019-0592-8
Nelson, J. A., Walther, S., Gans, F., Kraft, B., Weber, U., Novick, K., et al. (2024). X-BASE: the first terrestrial carbon and water flux products from an extended data-driven scaling framework, FLUXCOM-X. Biogeosciences 21, 5079–5115. doi:10.5194/bg-21-5079-2024
Nilsson, M., Sagerfors, J., Buffam, I., Laudon, H., Eriksson, T., Grelle, A., et al. (2008). Contemporary carbon accumulation in a boreal oligotrophic minerogenic mire – a significant sink after accounting for all C-fluxes. Glob. Change Biol. 14, 2317–2332. doi:10.1111/j.1365-2486.2008.01654.x
Oechel, W. C., Laskowski, C. A., Burba, G., Gioli, B., and Kalhori, A. a.M. (2014). Annual patterns and budget of CO2 flux in an Arctic tussock tundra ecosystem. J. Geophys. Res. Biogeosciences 119, 323–339. doi:10.1002/2013jg002431
Oh, Y., Zhuang, Q., Liu, L., Welp, L. R., Lau, M. C. Y., Onstott, T. C., et al. (2020). Reduced net methane emissions due to microbial methane oxidation in a warmer Arctic. Nat. Clim. Change 10, 317–321. doi:10.1038/s41558-020-0734-z
Olefeldt, D., Hovemyr, M., Kuhn, M. A., Bastviken, D., Bohn, T. J., Connolly, J., et al. (2021). The boreal–arctic wetland and lake dataset (BAWLD). Earth Syst. Sci. Data 13, 5127–5149. doi:10.5194/essd-13-5127-2021
Olid, C., Rodellas, V., Rocher-Ros, G., Garcia-Orellana, J., Diego-Feliu, M., Alorda-Kleinglass, A., et al. (2022). Groundwater discharge as a driver of methane emissions from Arctic lakes. Nat. Commun. 13, 3667. doi:10.1038/s41467-022-31219-1
Olofsson, J., Oksanen, L., Callaghan, T., Hulme, P. E., Oksanen, T., and Suominen, O. (2009). Herbivores inhibit climate-driven shrub expansion on the tundra. Glob. Change Biol. 15, 2681–2693. doi:10.1111/j.1365-2486.2009.01935.x
Olofsson, J., Oksanen, L., Oksanen, T., Tuomi, M., Hoset, K. S., Virtanen, R., et al. (2014). Long-term experiments reveal strong interactions between lemmings and plants in the fennoscandian highland tundra. Ecosystems 17, 606–615. doi:10.1007/s10021-013-9740-6
Palmtag, J., Obu, J., Kuhry, P., Richter, A., Siewert, M. B., Weiss, N., et al. (2022). A high spatial resolution soil carbon and nitrogen dataset for the northern permafrost region based on circumpolar land cover upscaling. Earth Syst. Sci. Data 14, 4095–4110. doi:10.5194/essd-14-4095-2022
Parmentier, F.-J. W., Thornton, B. F., Silyakova, A., and Christensen, T. R. (2024). Vulnerability of Arctic-Boreal methane emissions to climate change. Front. Environ. Sci. 12. doi:10.3389/fenvs.2024.1460155
Pastorello, G., Trotta, C., Canfora, E., Chu, H., Christianson, D., Cheah, Y.-W., et al. (2020). The FLUXNET2015 dataset and the ONEFlux processing pipeline for eddy covariance data. Sci. Data 7, 225. doi:10.1038/s41597-020-0534-3
Peltola, O., Vesala, T., Gao, Y., Räty, O., Alekseychik, P., Aurela, M., et al. (2019). Monthly gridded data product of northern wetland methane emissions based on upscaling eddy covariance observations. Earth Syst. Sci. Data 11, 1263–1289. doi:10.5194/essd-11-1263-2019
Petit bon, M., Hansen, B. B., Loonen, M. J. J. E., Petraglia, A., Bråthen, K. A., Böhner, H., et al. (2023). Long-term herbivore removal experiments reveal how geese and reindeer shape vegetation and ecosystem CO2-fluxes in high-Arctic tundra. J. Ecol. 111, 2627–2642. doi:10.1111/1365-2745.14200
Phoenix, G. K., and Bjerke, J. W. (2016). Arctic browning: extreme events and trends reversing arctic greening. Glob. Change Biol. 22, 2960–2962. doi:10.1111/gcb.13261
Pirk, N., Mastepanov, M., López-Blanco, E., Christensen, L. H., Christiansen, H. H., Hansen, B. U., et al. (2017). Toward a statistical description of methane emissions from arctic wetlands. Ambio 46, 70–80. doi:10.1007/s13280-016-0893-3
Plein, J., Clark, R. W., Arndt, K. A., Oechel, W. C., Stow, D., and Zona, D. (2022). Response of vegetation and carbon fluxes to brown lemming herbivory in northern Alaska. Biogeosciences 19, 2779–2794. doi:10.5194/bg-19-2779-2022
Pongracz, A., Wårlind, D., Miller, P. A., and Parmentier, F. J. W. (2021). Model simulations of arctic biogeochemistry and permafrost extent are highly sensitive to the implemented snow scheme in LPJ-GUESS. Biogeosciences 18, 5767–5787. doi:10.5194/bg-18-5767-2021
Post, E., Cahoon, S. M. P., Kerby, J. T., Pedersen, C., and Sullivan, P. F. (2021). Herbivory and warming interact in opposing patterns of covariation between arctic shrub species at large and local scales. Proc. Natl. Acad. Sci. U. S. A. 118, e2015158118. doi:10.1073/pnas.2015158118
Pumpanen, J., Lindén, A., Miettinen, H., Kolari, P., Ilvesniemi, H., Mammarella, I., et al. (2014). Precipitation and net ecosystem exchange are the most important drivers of DOC flux in upland boreal catchments. J. Geophys. Res. Biogeosciences 119, 1861–1878. doi:10.1002/2014jg002705
Qiu, C., Ciais, P., Zhu, D., Guenet, B., Chang, J., Chaudhary, N., et al. (2022). A strong mitigation scenario maintains climate neutrality of northern peatlands. One Earth 5, 86–97. doi:10.1016/j.oneear.2021.12.008
Ramage, J., Kuhn, M., Virkkala, A.-M., Voigt, C., Marushchak, M. E., Bastos, A., et al. (2024). The net GHG balance and budget of the permafrost region (2000–2020) from ecosystem flux upscaling. Glob. Biogeochem. Cycles 38, e2023GB007953. doi:10.1029/2023gb007953
Rantanen, M., Karpechko, A. Y., Lipponen, A., Nordling, K., Hyvärinen, O., Ruosteenoja, K., et al. (2022). The Arctic has warmed nearly four times faster than the globe since 1979. Commun. Earth and Environ. 3, 168. doi:10.1038/s43247-022-00498-3
Rawlins, M. A., and Karmalkar, A. V. (2024). Regime shifts in Arctic terrestrial hydrology manifested from impacts of climate warming. Cryosphere 18, 1033–1052. doi:10.5194/tc-18-1033-2024
Raynolds, M. K., Walker, D. A., Ambrosius, K. J., Brown, J., Everett, K. R., Kanevskiy, M., et al. (2014). Cumulative geoecological effects of 62 years of infrastructure and climate change in ice-rich permafrost landscapes, Prudhoe Bay Oilfield, Alaska. Glob. Change Biol. 20, 1211–1224. doi:10.1111/gcb.12500
Rees, W. G., Hofgaard, A., Boudreau, S., Cairns, D. M., Harper, K., Mamet, S., et al. (2020). Is subarctic forest advance able to keep pace with climate change? Glob. Change Biol. 26, 3965–3977. doi:10.1111/gcb.15113
Rinne, J., Łakomiec, P., Vestin, P., White, J. D., Weslien, P., Kelly, J., et al. (2022). Spatial and temporal variation in δ13C values of methane emitted from a hemiboreal mire: methanogenesis, methanotrophy, and hysteresis. Biogeosciences 19, 4331–4349. doi:10.5194/bg-19-4331-2022
Rinne, J., Tuovinen, J.-P., Klemedtsson, L., Aurela, M., Holst, J., Lohila, A., et al. (2020). Effect of the 2018 European drought on methane and carbon dioxide exchange of northern mire ecosystems. Philosophical Trans. R. Soc. B Biol. Sci. 375, 20190517. doi:10.1098/rstb.2019.0517
Rocher-Ros, G., Sponseller, R. A., Lidberg, W., Mörth, C.-M., and Giesler, R. (2019). Landscape process domains drive patterns of CO2 evasion from river networks. Limnol. Oceanogr. Lett. 4, 87–95. doi:10.1002/lol2.10108
Roturier, S., and Bergsten, U. (2006). Influence of soil scarification on reindeer foraging and damage to planted Pinus sylvestris seedlings. Scand. J. For. Res. 21, 209–220. doi:10.1080/02827580600759441
Roulet, N. T., Lafleur, P. M., Richard, P. J. H., Moore, T. R., Humphreys, E. R., and Bubier, J. (2007). Contemporary carbon balance and late Holocene carbon accumulation in a northern peatland. Glob. Change Biol. 13, 397–411. doi:10.1111/j.1365-2486.2006.01292.x
Salmon, E., Jégou, F., Guenet, B., Jourdain, L., Qiu, C., Bastrikov, V., et al. (2022). Assessing methane emissions for northern peatlands in ORCHIDEE-PEAT revision 7020. Geosci. Model Dev. 15, 2813–2838. doi:10.5194/gmd-15-2813-2022
Saunois, M., Stavert, A. R., Poulter, B., Bousquet, P., Canadell, J. G., Jackson, R. B., et al. (2020). The global methane budget 2000–2017. Earth Syst. Sci. Data 12, 1561–1623. doi:10.5194/essd-12-1561-2020
Scheller, J. H., Mastepanov, M., and Christensen, T. R. (2022). Toward UAV-based methane emission mapping of Arctic terrestrial ecosystems. Sci. Total Environ. 819, 153161. doi:10.1016/j.scitotenv.2022.153161
Schmidt, N. M., Barrio, I. C., Kristensen, J. A., López-Blanco, E., and Van Beest, F. M. (2024). Highlighting the role of biota in feedback loops from tundra ecosystems to the atmosphere. Front. Environ. Sci. 12. doi:10.3389/fenvs.2024.1491604
Schmitz, O. J., and Leroux, S. J. (2020). Food webs and ecosystems: linking species interactions to the carbon cycle. Annu. Rev. Ecol. Evol. Syst. 51, 271–295. doi:10.1146/annurev-ecolsys-011720-104730
Schmitz, O. J., Raymond, P. A., Estes, J. A., Kurz, W. A., Holtgrieve, G. W., Ritchie, M. E., et al. (2014). Animating the carbon cycle. Ecosystems 17, 344–359. doi:10.1007/s10021-013-9715-7
Schmitz, O. J., Sylvén, M., Atwood, T. B., Bakker, E. S., Berzaghi, F., Brodie, J. F., et al. (2023). Trophic rewilding can expand natural climate solutions. Nat. Clim. Change 13, 324–333. doi:10.1038/s41558-023-01631-6
Schmitz, O. J., Wilmers, C. C., Leroux, S. J., Doughty, C. E., Atwood, T. B., Galetti, M., et al. (2018). Animals and the zoogeochemistry of the carbon cycle. Science 362, eaar3213. doi:10.1126/science.aar3213
Scholze, M., Kaminski, T., Knorr, W., Voßbeck, M., Wu, M., Ferrazzoli, P., et al. (2019). Mean European carbon sink over 2010–2015 estimated by simultaneous assimilation of atmospheric CO2, soil moisture, and vegetation optical depth. Geophys. Res. Lett. 46, 13796–13803. doi:10.1029/2019gl085725
Schuur, E. a.G., Mcguire, A. D., Schadel, C., Grosse, G., Harden, J. W., Hayes, D. J., et al. (2015). Climate change and the permafrost carbon feedback. Nature 520, 171–179. doi:10.1038/nature14338
Schuur, E. a.G., Vogel, J. G., Crummer, K. G., Lee, H., Sickman, J. O., and Osterkamp, T. E. (2009). The effect of permafrost thaw on old carbon release and net carbon exchange from tundra. Nature 459, 556–559. doi:10.1038/nature08031
See, C. R., Virkkala, A.-M., Natali, S. M., Rogers, B. M., Mauritz, M., Biasi, C., et al. (2024). Decadal increases in carbon uptake offset by respiratory losses across northern permafrost ecosystems. Nat. Clim. Change 14, 853–862. doi:10.1038/s41558-024-02057-4
Serikova, S., Pokrovsky, O. S., Ala-Aho, P., Kazantsev, V., Kirpotin, S. N., Kopysov, S. G., et al. (2018). High riverine CO2 emissions at the permafrost boundary of Western Siberia. Nat. Geosci. 11, 825–829. doi:10.1038/s41561-018-0218-1
Serreze, M. C., and Barry, R. G. (2011). Processes and impacts of Arctic amplification: a research synthesis. Glob. Planet. Change 77, 85–96. doi:10.1016/j.gloplacha.2011.03.004
Shogren, A. J., Zarnetske, J. P., Abbott, B. W., Iannucci, F., Frei, R. J., Griffin, N. A., et al. (2019). Revealing biogeochemical signatures of Arctic landscapes with river chemistry. Sci. Rep. 9, 12894. doi:10.1038/s41598-019-49296-6
Shogren, A. J., Zarnetske, J. P., Abbott, B. W., Iannucci, F., Medvedeff, A., Cairns, S., et al. (2021). Arctic concentration–discharge relationships for dissolved organic carbon and nitrate vary with landscape and season. Limnol. Oceanogr. 66, S197–S215. doi:10.1002/lno.11682
Silfver, T., Heiskanen, L., Aurela, M., Myller, K., Karhu, K., Meyer, N., et al. (2020). Insect herbivory dampens Subarctic birch forest C sink response to warming. Nat. Commun. 11, 2529. doi:10.1038/s41467-020-16404-4
Sjögersten, S., Van Der Wal, R., and Woodin, S. J. (2012). Impacts of grazing and climate warming on C pools and decomposition rates in arctic environments. Ecosystems 15, 349–362. doi:10.1007/s10021-011-9514-y
Sørensen, L. I., Mikola, J., Kytöviita, M.-M., and Olofsson, J. (2009). Trampling and spatial heterogeneity explain decomposer abundances in a sub-arctic grassland subjected to simulated reindeer grazing. Ecosystems 12, 830–842. doi:10.1007/s10021-009-9260-6
Speir, S. L., Rose, L. A., Blaszczak, J. R., Kincaid, D. W., Fazekas, H. M., Webster, A. J., et al. (2024). Catchment concentration–discharge relationships across temporal scales: a review. WIREs Water 11, e1702. doi:10.1002/wat2.1702
Sturm, M., Schimel, J., Michaelson, G., Welker, J. M., Oberbauer, S. F., Liston, G. E., et al. (2005). Winter biological processes could help convert arctic tundra to shrubland. BioScience 55, 17–26. doi:10.1641/0006-3568(2005)055[0017:wbpchc]2.0.co;2
Tank, S. E., Fellman, J. B., Hood, E., and Kritzberg, E. S. (2018). Beyond respiration: controls on lateral carbon fluxes across the terrestrial-aquatic interface. Limnol. Oceanogr. Lett. 3, 76–88. doi:10.1002/lol2.10065
Tank, S. E., Frey, K. E., Striegl, R. G., Raymond, P. A., Holmes, R. M., Mcclelland, J. W., et al. (2012). Landscape-level controls on dissolved carbon flux from diverse catchments of the circumboreal. Glob. Biogeochem. Cycles 26. doi:10.1029/2012gb004299
Tape, K. D., Clark, J. A., Jones, B. M., Kantner, S., Gaglioti, B. V., Grosse, G., et al. (2022). Expanding beaver pond distribution in Arctic Alaska, 1949 to 2019. Sci. Rep. 12, 7123. doi:10.1038/s41598-022-09330-6
Te Beest, M., Sitters, J., Ménard, C. B., and Olofsson, J. (2016). Reindeer grazing increases summer albedo by reducing shrub abundance in Arctic tundra. Environ. Res. Lett. 11, 125013. doi:10.1088/1748-9326/aa5128
Thanh Duc, N., Silverstein, S., Wik, M., Crill, P., Bastviken, D., and Varner, R. K. (2020). Technical note: greenhouse gas flux studies: an automated online system for gas emission measurements in aquatic environments. Hydrol. Earth Syst. Sci. 24, 3417–3430. doi:10.5194/hess-24-3417-2020
Tramontana, G., Migliavacca, M., Jung, M., Reichstein, M., Keenan, T. F., Camps-Valls, G., et al. (2020). Partitioning net carbon dioxide fluxes into photosynthesis and respiration using neural networks. Glob. Change Biol. 26, 5235–5253. doi:10.1111/gcb.15203
Treat, C. C., Bloom, A. A., and Marushchak, M. E. (2018a). Nongrowing season methane emissions–a significant component of annual emissions across northern ecosystems. Glob. Change Biol. 24, 3331–3343. doi:10.1111/gcb.14137
Treat, C. C., Marushchak, M. E., Voigt, C., Zhang, Y., Tan, Z., Zhuang, Q., et al. (2018b). Tundra landscape heterogeneity, not interannual variability, controls the decadal regional carbon balance in the Western Russian Arctic. Glob. Change Biol. 24, 5188–5204. doi:10.1111/gcb.14421
Tuomi, M., Stark, S., Hoset, K. S., Väisänen, M., Oksanen, L., Murguzur, F. J. A., et al. (2019). Herbivore effects on ecosystem process rates in a low-productive system. Ecosystems 22, 827–843. doi:10.1007/s10021-018-0307-4
Turetsky, M. R., Abbott, B. W., Jones, M. C., Anthony, K. W., Olefeldt, D., Schuur, E. a.G., et al. (2020). Carbon release through abrupt permafrost thaw. Nat. Geosci. 13, 138–143. doi:10.1038/s41561-019-0526-0
Turetsky, M. R., Kotowska, A., Bubier, J., Dise, N. B., Crill, P., Hornibrook, E. R. C., et al. (2014). A synthesis of methane emissions from 71 northern, temperate, and subtropical wetlands. Glob. Change Biol. 20, 2183–2197. doi:10.1111/gcb.12580
Väisänen, M., Sjögersten, S., Large, D., Drage, T., and Stark, S. (2015). Long-term reindeer grazing limits warming-induced increases in CO2 released by tundra heath soil: potential role of soil C quality. Environ. Res. Lett. 10, 094020. doi:10.1088/1748-9326/10/9/094020
Väisänen, M., Ylänne, H., Kaarlejärvi, E., Sjögersten, S., Olofsson, J., Crout, N., et al. (2014). Consequences of warming on tundra carbon balance determined by reindeer grazing history. Nat. Clim. Change 4, 384–388. doi:10.1038/nclimate2147
Van Beest, F. M., Barry, T., Christensen, T., Heiðmarsson, S., Mclennan, D., and Schmidt, N. M. (2022). Extreme event impacts on terrestrial and freshwater biota in the arctic: a synthesis of knowledge and opportunities. Front. Environ. Sci. 10. doi:10.3389/fenvs.2022.983637
Van Den Hurk, B., Kim, H., Krinner, G., Seneviratne, S. I., Derksen, C., Oki, T., et al. (2016). LS3MIP (v1.0) contribution to CMIP6: the Land Surface, Snow and Soil moisture Model Intercomparison Project – aims, setup and expected outcome. Geosci. Model Dev. 9, 2809–2832. doi:10.5194/gmd-9-2809-2016
Van Der Wal, R., Bardgett, R. D., Harrison, K. A., and Stien, A. (2004). Vertebrate herbivores and ecosystem control: cascading effects of faeces on tundra ecosystems. Ecography 27, 242–252. doi:10.1111/j.0906-7590.2004.03688.x
Vekuri, H., Tuovinen, J.-P., Kulmala, L., Papale, D., Kolari, P., Aurela, M., et al. (2023). A widely-used eddy covariance gap-filling method creates systematic bias in carbon balance estimates. Sci. Rep. 13, 1720. doi:10.1038/s41598-023-28827-2
Virkkala, A.-M., Aalto, J., Rogers, B. M., Tagesson, T., Treat, C. C., Natali, S. M., et al. (2021). Statistical upscaling of ecosystem CO2 fluxes across the terrestrial tundra and boreal domain: regional patterns and uncertainties. Glob. Change Biol. 27, 4040–4059. doi:10.1111/gcb.15659
Virkkala, A. M., Natali, S. M., Rogers, B. M., Watts, J. D., Savage, K., Connon, S. J., et al. (2022). The ABCflux database: arctic–boreal CO2 flux observations and ancillary information aggregated to monthly time steps across terrestrial ecosystems. Earth Syst. Sci. Data 14, 179–208. doi:10.5194/essd-14-179-2022
Virkkala, A.-M., Rogers, B. M., Watts, J. D., Arndt, K. A., Potter, S., Wargowsky, I., et al. (2025). Wildfires offset the increasing but spatially heterogeneous Arctic–boreal CO2 uptake. Nat. Clim. Change 15, 188–195. doi:10.1038/s41558-024-02234-5
Voigt, C., Van Delden, L., Marushchak, M. E., Biasi, C., Abbott, B. W., Elberling, B., et al. (2020). Nitrous oxide fluxes from permafrost regions. PANGAEA. doi:10.1594/PANGAEA.919217
Vonk, J. E., and Gustafsson, Ö. (2013). Permafrost-carbon complexities. Nat. Geosci. 6, 675–676. doi:10.1038/ngeo1937
Vonk, J. E., Speetjens, N. J., and Poste, A. E. (2023). Small watersheds may play a disproportionate role in arctic land-ocean fluxes. Nat. Commun. 14, 3442. doi:10.1038/s41467-023-39209-7
Walsh, J. E., Ballinger, T. J., Euskirchen, E. S., Hanna, E., Mård, J., Overland, J. E., et al. (2020). Extreme weather and climate events in northern areas: a review. Earth-Science Rev. 209, 103324. doi:10.1016/j.earscirev.2020.103324
Wang, T., Ottlé, C., Boone, A., Ciais, P., Brun, E., Morin, S., et al. (2013). Evaluation of an improved intermediate complexity snow scheme in the ORCHIDEE land surface model. J. Geophys. Res. Atmos. 118, 6064–6079. doi:10.1002/jgrd.50395
Watts, J. D., Farina, M., Kimball, J. S., Schiferl, L. D., Liu, Z., Arndt, K. A., et al. (2023). Carbon uptake in Eurasian boreal forests dominates the high-latitude net ecosystem carbon budget. Glob. Change Biol. 29, 1870–1889. doi:10.1111/gcb.16553
Wik, M., Varner, R. K., Anthony, K. W., Macintyre, S., and Bastviken, D. (2016). Climate-sensitive northern lakes and ponds are critical components of methane release. Nat. Geosci. 9, 99–105. doi:10.1038/ngeo2578
Windirsch, T., Grosse, G., Ulrich, M., Forbes, B. C., Göckede, M., Wolter, J., et al. (2022). Large herbivores on permafrost— a pilot study of grazing impacts on permafrost soil carbon storage in northeastern Siberia. Front. Environ. Sci. 10. doi:10.3389/fenvs.2022.893478
Yao, Y., Ciais, P., Viovy, N., Li, W., Cresto-Aleina, F., Yang, H., et al. (2021). A data-driven global soil heterotrophic respiration dataset and the drivers of its inter-annual variability. Glob. Biogeochem. Cycles 35, e2020GB006918. doi:10.1029/2020gb006918
Ylänne, H., Olofsson, J., Oksanen, L., and Stark, S. (2018). Consequences of grazer-induced vegetation transitions on ecosystem carbon storage in the tundra. Funct. Ecol. 32, 1091–1102. doi:10.1111/1365-2435.13029
Ylänne, H., Stark, S., and Tolvanen, A. (2015). Vegetation shift from deciduous to evergreen dwarf shrubs in response to selective herbivory offsets carbon losses: evidence from 19 years of warming and simulated herbivory in the subarctic tundra. Glob. Change Biol. 21, 3696–3711. doi:10.1111/gcb.12964
Yuan, K., Li, F., Mcnicol, G., Chen, M., Hoyt, A., Knox, S., et al. (2024). Boreal–Arctic wetland methane emissions modulated by warming and vegetation activity. Nat. Clim. Change 14, 282–288. doi:10.1038/s41558-024-01933-3
Zimov, S. A. (2005). Pleistocene park: return of the mammoth's ecosystem. Science 308, 796–798. doi:10.1126/science.1113442
Zimov, S. A., Chuprynin, V. I., Oreshko, A. P., Chapin, F. S., Reynolds, J. F., and Chapin, M. C. (1995). Steppe-tundra transition: a herbivore-driven biome shift at the end of the pleistocene. Am. Nat. 146, 765–794. doi:10.1086/285824
Keywords: net ecosystem carbon balance, high Arctic, boreal zone, permafrost region, carbon sequestration, methane emissions, lateral transport, herbivore interactions
Citation: López-Blanco E, Väisänen M, Salmon E, Jones CP, Schmidt NM, Marttila H, Lohila A, Juutinen S, Scheller J and Christensen TR (2025) The net ecosystem carbon balance (NECB) at catchment scales in the Arctic. Front. Environ. Sci. 13:1544586. doi: 10.3389/fenvs.2025.1544586
Received: 13 December 2024; Accepted: 11 March 2025;
Published: 07 April 2025.
Edited by:
Christina Biasi, University of Eastern Finland, FinlandReviewed by:
Sigrid Dengel, Berkeley Lab (DOE), United StatesCopyright © 2025 López-Blanco, Väisänen, Salmon, Jones, Schmidt, Marttila, Lohila, Juutinen, Scheller and Christensen. This is an open-access article distributed under the terms of the Creative Commons Attribution License (CC BY). The use, distribution or reproduction in other forums is permitted, provided the original author(s) and the copyright owner(s) are credited and that the original publication in this journal is cited, in accordance with accepted academic practice. No use, distribution or reproduction is permitted which does not comply with these terms.
*Correspondence: Efrén López-Blanco, ZWxiQGVjb3MuYXUuZGs=
Disclaimer: All claims expressed in this article are solely those of the authors and do not necessarily represent those of their affiliated organizations, or those of the publisher, the editors and the reviewers. Any product that may be evaluated in this article or claim that may be made by its manufacturer is not guaranteed or endorsed by the publisher.
Research integrity at Frontiers
Learn more about the work of our research integrity team to safeguard the quality of each article we publish.