- Institute of Geological Sciences, University of Wrocław, Wrocław, Poland
This study presents a simplified and optimized bacterial denitrification method using Stenotrophomonas nitritireducens for precise nitrite isotope analysis in low-concentration environmental samples. The improved method reduces the bacterial cultivation period from approximately 3–4 weeks to just 24 h. Additionally, it allows for reliable nitrite analyses at concentrations as low as 150 nmol NO2− L-1, enabling analyses at concentrations of approximately one order of magnitude lower compared to previous methods. Three treatments were tested to evaluate their impact on isotopic precision and accuracy: Treatment 1 used a direct incubation approach, Treatment 2 incorporated an additional growth step of re-inoculation of the bacterial culture into fresh medium, and Treatment 3 included a 24-h stabilization step at 4°C after the initial incubation. The method was validated using internal standards and applied to environmental samples, achieving good precision. Isotope ratio mass spectrometry (IRMS) measurements demonstrated superior accuracy for Treatment 1, with mean accuracies of ±0.7‰ for δ15N and ±0.4‰ for δ18O, while Treatment 2 (±2.0‰ for δ15N and ±1.7‰ for δ18O) and Treatment 3 (±1.8‰ for δ15N and ±4.3‰ for δ18O) showed lower precision. Among the treatments, Treatment 1 delivered the most accurate and reproducible results, showing minimal deviations of the measured N2O values from the true nitrite values. The oxygen isotope exchange between water and NO2− during bacterial conversion ranged from 7% to 16%, which is similar to previous methods. This study integrates advanced analytical tools, such as laser spectroscopy and isotope ratio mass spectrometry, enabling reliable isotopic measurements even at trace nitrite concentrations. IRMS offered higher precision for high concentrations, while laser spectroscopy was better suited for repeated measurements at trace levels in low-concentration samples. The enhancements in the cultivation efficiency, detection sensitivity, and precision make this approach highly valuable for environmental studies, especially in tracing nitrogen transformations in soil and water systems.
1 Introduction
Nitrogen (N) is an essential nutrient for plant growth and plays a critical role in global food production, with synthetic nitrogen fertilizers being vital for maximizing agricultural yields worldwide. However, excessive use of these fertilizers has disrupted the natural nitrogen cycle, leading to severe environmental impacts. The inefficient uptake of nitrogen by crops results in its leaching into water bodies (mostly as nitrate, NO3−) and the emission of gaseous forms of nitrogen oxides (NO, N2O, and NO2_) into the atmosphere. Nitrous oxide (N2O), a greenhouse gas more potent than carbon dioxide, intensifies global warming and depletes the ozone layer. NO3− causes water eutrophication and deterioration of water quality (Rütting et al., 2018). Hence, we deal with a considerable loss of reactive N from crop fields and negative environmental impacts. To combat nitrogen losses, it is crucial to better understand N transformations in the environment (Nikolenko et al., 2018) in order to effectively improve nitrogen management and enhance crop nitrogen-use efficiency (Sainju et al., 2020). Key studies in freshwater and marine ecosystems (Campbell et al., 2002; Sigman et al., 2005), along with subsequent advancements, have emphasized the significance of monitoring nitrogen species and their isotopic signatures. Nitrite is a very important intermediate product in almost all N-transformations. Although it is a short-lived and highly reactive compound, it can provide important insights into ongoing processes and thereby increase our understanding of the N cycle (Müller et al., 2014).
Nitrogen transforms through various processes like nitrification, denitrification, and anammox within ecosystems, with soil microorganisms playing a pivotal role in making it available to plants. Numerous studies have highlighted that stable isotope studies are effective tools for identifying various nitrogen pollution sources and environmental transformations (Sigman et al., 2005; Knöller et al., 2011; Sebilo et al., 2019; Lewicka-Szczebak et al., 2021). Naturally occurring nitrogen and oxygen isotopes (15N/14N and 18O/16O) and their fractionation during microbial transformations have significantly expanded our understanding of the nitrogen cycle (Deb et al., 2024; Denk et al., 2017).
The isotope signatures of NO3− (δ15NNO3 and δ18ONO3) are frequently used to trace the sources and pathways of nitrate contamination and estimate the progress of its reduction during denitrification (Nikolenko et al., 2018). Similarly, isotopic signatures in ammonium—NH4+ (δ15NNH4)—provide information on its natural and anthropogenic sources in the environment (Deb et al., 2024). These insights can be very well supplemented with nitrite (NO2−) isotope signatures (δ15NNO2- and δ18ONO2-), which show a wide range of variations and serve as useful indicators of the active processes involved in its production and consumption (Buchwald and Casciotti, 2013; Dähnke and Thamdrup, 2013). While δ15NNO2- provides a reliable tracer of nitrogen cycling processes, δ18ONO2− is not always informative for identifying sources and sinks. For example, in offshore marine oxygen-deficient zones, slower NO2− turnover times likely facilitate O isotope exchange with water (Hu et al., 2016). Despite such limitations, due to the very fast transformation rates of NO2−, this analysis provides an actual “snapshot” of ongoing transformations and allows for the identification of NO2− sources and sinks (Lewicka-Szczebak et al., 2021).
For measurements of stable isotope ratios of any compound, either with mass spectrometry (MS) or laser spectroscopy, we need to convert dissolved inorganic nitrogen to the N2O gaseous form. Therefore, mineral nitrogen forms dissolved in water, originating either from water samples or from soil extractions, need to be transformed to gas that can be further analyzed. This can be performed with chemical or microbial conversion (Altabet et al., 2019; McIlvin and Altabet, 2005). Although potential problems with reaction completeness and matrix effects have been identified for the azide method (Granger et al., 2020), the study also provides solutions to address these challenges. These refinements enable the azide method to be successfully applied across a wide range of sample types, including those with very low nitrite concentrations (as low as 10 nmol NO2−). However, chemical methods for converting dissolved nitrogen compounds into gaseous forms utilize sodium azide, a reagent associated with serious safety risks. Its high toxicity and the potential to generate hydrazoic acid, a highly explosive and dangerous compound, when in contact with water, acids, or metals present significant hazards (https://www.cdc.gov/niosh/npg/npgd0560.html). The application of bacterial conversion methods allow for alleviating the risks associated with chemical conversion.
First, the bacterial denitrification method for dissolved nitrates analysis was described (Sigman et al., 2001), where Pseudomonas aureofaciens was applied to reduce NO3− and NO2− to N2O and achieve the joint isotopic signature for both NO2− and NO3− from the same sample aliquot. Later, this procedure was further developed by McIlvin and Casciotti (2011) and simplified by Stock et al. (2021). Additionally, Weigand et al. (2016) introduced updates to the method, refining instrumentation and protocols to enhance the precision and reproducibility for δ15N and δ18O measurements while expanding its applicability to diverse environmental samples.
For NO2−, similarly as for NO3−, we can analyze both δ15N and δ18O. Böhlke et al. (2007) described the bacterial denitrification method by S. nitritireducens, which can selectively reduce NO2− to N2O but is unable to reduce NO3− due to the absence of the necessary reductase enzyme. After the bacterial conversion to N2O gas, the sequential N2O isotope analysis for δ15N and δ18O via isotope ratio mass spectrometry provides information on the original nitrite isotope signature in water samples. Water samples were treated with S. nitritireducens in anoxic conditions where the bacteria selectively convert NO2− directly to N2O gas, leaving the nitrate in the sample. The produced N2O was collected, purified through gas chromatography, and subsequently analyzed using isotope ratio mass spectrometry for nitrogen (δ15N) and oxygen isotopes (δ18O) in the N2O, reflecting isotopic compositions of the nitrite in the samples. Following nitrite analysis, the same samples were treated with P. aureofaciens to reduce the remaining NO3− to N2O, enabling sequential analysis of nitrate isotopes in the same sample. The method was calibrated using solutions of known isotopic compositions to ensure accuracy and reproducibility and validated by testing various concentrations of nitrite to differentiate between nitrite and nitrate in mixed samples, assessing method’s sensitivity and selectivity. The method has been widely applied for nitrite analyses in marine studies (Buchwald and Casciotti, 2013; Pajares et al., 2019; Dähnke et al., 2022), freshwater studies (Jacob et al., 2017; Sebilo et al., 2019), soil studies (Lewicka-Szczebak et al., 2021), or pure culture experiments (Knöller et al., 2011).
However, this method (Böhlke et al., 2007) for isotopic analysis of δ15N and δ18O in the NO2− involves multi-step processes for bacterial culture preparation, the bacterial reduction of NO2− to N2O using S. nitritireducens, and subsequent isotopic analysis via mass spectrometry. While comprehensive, the process can be quite time-consuming, particularly during culture preparation and bacteria cultivation. Moreover, for these analyses, quite high NO2− concentrations are required, which are rarely found in natural environments.
This study aims to further improve the denitrification method using S. nitritireducens for reducing NO2− to N2O by optimizing the bacteria cultivation time and lowering the amount of NO2− needed for the analysis. We applied and tested the introduction of modifications to this method, following the improvements to the bacterial denitrification method using P. aureofaciens, as presented by Stock et al. (2021). The final product, N2O, is measured for the isotopic composition using laser spectroscopy (Picarro N2O Isotope Analyzer) combined with a small sample injection module (SSIM) and automatic autosampler (SRI). SSIM allows for the analysis of small-volume gas samples in ambient concentration, making it particularly useful for scientific research where sample sizes may be small and nitrite concentration is usually very low. By integrating automated sample processing systems, employing faster bacterial reduction of nitrite to N2O and reduced sample preparation time, our method could significantly shorten both preparation and reaction times and vastly lower the nitrite amount needed for analysis. This is particularly important for nitrite analysis, which is a very reactive compound and which even after proper conservation should be analyzed as soon as possible, preferably within few days. Additionally, the ability to conduct repeated measurements with high precision ensures the reliability of the results obtained. Overall, this technique provides a robust tool for analyzing both the isotopic composition and concentration of nitrite in various environmental samples.
2 Materials and methods
S. nitritireducens (ATCC No. BAA-12) is an aerobic, Gram-negative mesophilic bacterium producing yellow-pigmented colonies, named after its ability to reduce NO2− (Finkmann et al., 2000). For a quick and precise isotope analysis of NO2−, the traditional denitrifier methods using S. nitritireducens were moderately adjusted, as detailed below (Sections 2.1–2.3).
In the revised method, the bacterial cultivation process was significantly altered, similar to the approach described by Weigand et al. (2016) and further improved by Stock et al. (2021) for the bacterial denitrification method with P. aureofaciens. We omitted recultivating of the previously frozen stock culture on agar plates and instead directly incorporated the stock culture into the liquid medium, as detailed below in Section 2.3.1. This adjustment reduced the process duration from over 2 weeks to just 2 days, minimizing the demanding and time-consuming preliminary preparation steps.
2.1 Materials, solutions, and culture media
All solutions, culture vessels, and other materials were sterilized using an autoclave (V-75, Systec GmbH, Linden, Germany) at 121°C before use and carefully handled afterward to avoid any contamination. The media used for the cultivation of S. nitritireducens were as follows:
(a) Media A—for agar plates (TSA): This medium was prepared by dissolving 30 g L−1 soy agar powder (Merck), 0.25 g L−1 ammonium sulfate ((NH4)2SO4, Merck), 2.45 g L−1 dipotassium phosphate ((K2HPO4), Merck), and 15 g L−1 Caso-bouillon (Merck) in double-distilled H2O up to 1 L in a flask. The medium was solidified using 3% agar and autoclaved at 121°C. Agar plates were stored at 2°C–8°C until use.
(b) Media B—for S. nitritireducens cultivation: This liquid medium consisted of 0.25 g L−1 ammonium sulfate ((NH4)2SO4), 2.45 g L−1 dipotassium phosphate (K2HPO4), and 15 g L−1 Caso-bouillon dissolved in double-distilled H2O up to 1 L. The pH was adjusted to ∼7.3 before autoclaving at 121°C. The prepared medium was stored in tightly closed flasks at 2°C–8°C until use.
(c) Glycerine solution: A glycerine solution (liquid medium) was prepared by mixing water and glycerine in equal proportions (50:50 by weight) to achieve a density of 1.261 g/cm3. The solution was autoclaved at 121°C and stored at 2°C–8°C until use.
(d) Media C—for re-activation of freeze-dried culture (liquid medium and agar plates): A liquid medium was prepared by dissolving 2.5 g L−1 peptone (Merck) and 1.5 g L−1 meat extract in double-distilled H2O up to 1 L. The pH value was adjusted to 7.0 before autoclaving at 121°C. For solid medium, 1.5% agar was added before autoclaving. The prepared flasks and agar plates were stored at 2°C–8°C until use.
2.2 Reactivation of freeze-dried bacterial culture S. nitritireducens and stock culture preparation
The bacterium S. nitritireducens was provided in a freeze-dried state within a glass ampoule, enabling safe storage at temperatures between 2°C and 8°C until it was reactivated. Further reactivation of the dry culture was performed as recommended: under sterile conditions, freeze-dried bacteria from a glass ampoule were rehydrated with Media A, later transferred to Falcon tubes, and inoculated into agar plates with Media A. Unused bacteria were stored at 4°C for up to a month or frozen for longer storage. The inoculated agar plates were incubated at 28°C–30°C, and the Falcon tubes were shaken at 100 rpm for 24–48 h. Single colonies from the plates were selected and transferred to fresh plates every few days, a process that was repeated over 2 weeks to maintain the culture.
For stock culture preparation, single colonies were inoculated into Falcon tubes containing Media B and incubated overnight at room temperature. The following day, 400 μL of media was mixed with 600 μL of the glycerin solution, transferred to 2.5-mL Eppendorf tubes, and stored at −80°C for future use.
2.3 Denitrifier method using S. nitritireducens
2.3.1 Recultivation of the stock culture and inoculation with S. nitritireducens
For S. nitritireducens recultivation, a stock culture was removed from the freezer, thawed at 37°C, and used as the inoculum. The inoculum was transferred to a 500-mL flask containing 50 mL Media B, loosely closed with a screw cap, and incubated at 23°C on the horizontal shaker at 100 rpm for 24 h. On the following day, after the growth phase, the optical density was checked, and to the flask, 10–12 drops of antifoam medium (previously autoclaved at 121°C) were added and mixed. A measure of 4 mL of the overgrown media was pipetted into previously autoclaved headspace vials, which were then closed with crimped seals. A cannula (0.6 × 30 mm) was inserted in each of the septa of the bottle caps for venting. The bottles were placed upside down on the cannulas (0.6 × 30 mm) of the purge gas distributor and flushed with nitrogen gas lines at 0.6 bar for 90 min (the flow rate should be 60–70 mL min-1) to change the conditions in the gas space from oxic to anoxic. After flushing, cannulas were removed, and the vials were placed upside down.
2.3.2 Testing different treatments
In addition to the above-described procedure (Treatment 1), we tested other optional treatments (treatments 2 and 3) to finally select the most efficient procedure that yielded the best results. In alternative treatments, we have modified the bacterial inoculation techniques for the analysis of nitrogen and oxygen isotopes in the denitrification method using S. nitritireducens for reducing NO2− to N2O. Additional methods were also evaluated for their accuracy and reliability.
For Treatment 2, unlike Treatment 1, an additional growth step was introduced, where 1 mL of the overgrown medium/aliquot was transferred to a new medium bottle with 50 mL of Media B and was further incubated at 23°C on the horizontal shaker at 100 rpm for 24 h. This step was implemented to refresh the bacterial culture and ensure that it remains in the exponential growth phase for optimal metabolic activity. It helps prevent the issues of overgrowth, such as reduced activity or nutrient depletion, which may occur if the bacteria are left to grow too long in a single medium. Re-inoculation can help standardize bacterial density and reduce variability caused by the overgrowth or nutrient depletion in the initial medium. On the following day, the optical density was measured to assess bacterial growth, and the subsequent process was conducted as described in Section 2.3.1: vials were autoclaved, filled with 4 mL of the media, sealed, and flushed with nitrogen gas (0.6 bar, 60–70 mL min−1) for 90 min to create anoxic conditions. Samples (20 nmol NO2− or water for blanks) were injected into inverted vials, incubated (1 h, 90 rpm), treated with 6 M NaOH, and gas-transferred to Exetainer vials for isotope analysis.
For Treatment 3, unlike treatments 1 and 2, following the protocol outlined in Section 2.3.1, the overgrown medium (after the initial 24-h incubation at 23°C/100 rpm) was subsequently stored at 4°C for another 24 h to ensure the stability and viability of the bacterial culture. In contrast, Treatment 1 proceeded directly after the initial 24-h incubation, and Treatment 2 involved an additional incubation step in a fresh medium. The following day, the optical density was measured to assess the culture’s growth, and the further process was conducted as described for Treatments 1 and 2 above.
2.3.3 Sample injection and isotope analysis
The required amount of sample containing from 1 to 20 nmol NO2− was injected through a syringe (cannula 0.5 × 16 mm) into the 20-mL crimped vials held upside down. Additionally, for each sample run, bacterial blank N2O production was evaluated by injecting the same volume of double-distilled water. The vials were incubated at room temperature for at least 1 h on the shaker (90 rpm). Afterward, the bacteria must be killed by adding 0.1–0.2 mL of 6 M NaOH to each vial. Then, the gas sample was transferred from each vial into previously evacuated Exetainer vials (Labco Limited, Ceredigion, United Kingdom). This sample transfer step was recommended for our instrumental set-up, but it may possibly be omitted. Isotope analyses were performed with two alternative isotope measurement methods: laser spectroscopy and mass spectrometry. For each measurement, reference standards with known isotopic compositions were analyzed multiple times in the same manner as the samples. Laser spectroscopy measurements were conducted with the cavity ring-down spectroscopy method (CRDS) using the Picarro G5131-i Spectrometer (Picarro, Santa Clara, United States) combined with an SSIM and automatic autosampler (SRI Autosampler, Bad Honnef, Germany). The autosampler automatically flushes the connecting tubes with N2 and evacuates before piercing the sample vial. Then, it transfers the sample to the SSIM module and then to the Picarro instrument with a slight (approximately 30%) dilution with N2 transfer gas. The 12-mL sample vials, with 2 bar pressure and N2O concentrations ranging from 300 ppb to 2,000 ppb, can be successfully measured with this setup. Due to this strict limit in the concentration range, the higher-concentration samples (>1 nmol NO2−) often need dilution before measurements. For such samples, repeated analyses of the same conversion vial are possible.
Mass spectrometry measurements were conducted using an isotope ratio mass spectrometer (MAT 253 Plus, Thermo Fisher Scientific, Bremen, Germany) coupled with a GasBench II and PreCon Gas Pre-concentration Unit (Thermo Fisher Scientific). The PreCon automates the collection, concentration, and purification of trace N2O from air samples, removing non-condensable gases like CO2 and H2O before introducing the sample to the mass spectrometer. The system operates with liquid nitrogen-cooled traps to purify and cryofocus the sample before it is transferred to the mass spectrometer for precise isotopic ratio determination. This setup allows the analysis of N2O at higher concentrations (from ca. 2 ppm) from small sample volumes (12 mL). Samples were loaded using a GC-PAL autosampler. After purifying, pre-concentrating, and cryofocusing, the sample was transferred through Nafion traps for removing water and injected into a gas chromatography column (PoraPLOT). After GC separation, N2O was carried by the helium stream to the mass spectrometer for isotope ratio determination of 18O/16O and 15N/14N values. For this technique, a high amount of N2O gas is preferred. Hence, the total amount of the produced N2O gas in the conversion vials is used for the measurement.
2.4 Isotope ratio normalization
For each run of measurements, five internal standards (Ni1, Ni2, NE3, NE4, and NE5) with known isotope ratios were prepared in the laboratory and implemented to normalize the isotope ratios of the samples, correct for any isotope effects during the conversion, check for the completeness of conversion of NO2− to N2O, and quantify the O-isotope exchange by S. nitritireducens. Two types of commercial nitrite (Ni1 and Ni2) were applied as the internal standards. Additionally, nitrite was equilibrated at low pH with three different δ18O water isotope signatures (NE3, NE4, and NE5) to obtain nitrite standards of a wide range of δ18O, similar to the method applied by Casciotti et al. (2007). After equilibration, water was evaporated, and the residual nitrite was analyzed using an EA (Elemental Analyzer, Thermo Fisher Scientific) for δ15N and a TC-EA (Thermal Combustion Elemental Analyzer, Thermo Fisher Scientific) for δ18O, together with commercially available nitrate standards (USGS 32, USGS 34, and USGS 35) of the known δ15N values (+180‰, −1.8‰, and 2.7‰, respectively) and δ18O values (+25.4‰, −27.9‰, and +57.5‰). This allowed the calibration of the internal nitrite standards in relation to the international standards (atmospheric N2 and Vienna Standard Mean Ocean Water (VSMOW), respectively). Such analysis with total combustion methods allowed us to place the isotope values of our internal standards on the international isotope scale, although there are no commercially available nitrite standards.
For our measurements, we dissolved the standards in double-distilled water to prepare working solutions with an NO2− concentration of 8–80 nmol mL−1. Before the dissolution of the standard, KOH is added to water to increase the pH value to 13 to ensure nitrite stability and prevent any O-isotope exchange. A measure of 0.2 mL of standard working solutions with varying concentrations was added to the conversion vials. This was carried out to test the applicability of this method for lower concentration and check the stability of isotope effects and O-isotope exchange associated with the bacterial conversion by adding different amounts of NO2−.
All isotopic values are expressed as ‰ deviation from the 15N/14N and 18O/16O ratios of the reference materials (i.e., atmospheric N2 and VSMOW, respectively). The measured isotope ratios of the standards were used to normalize measured δ15N and δ18O values of the N2O produced by applying linear regression for the calibration (Casciotti et al., 2007). The true δ values of the standards are presented in Tables 1, 2, and the linear regression lines for calibration with the equation and the coefficient of determination are presented in Figure 1.
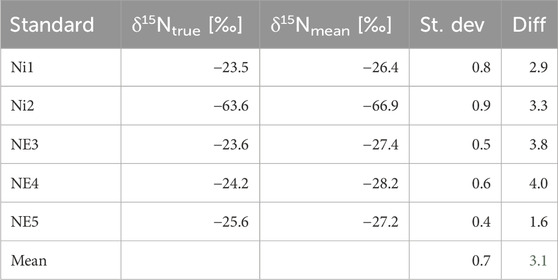
Table 1. True δ15N values of the standards (vs. air-N2) (δ15NNO2-) and mean measured δ15N values (δ15NN2O) in Treatment 1, conversion of 20 nmol NO2−, measured using the CRDS technique. St. dev, standard deviation for n = 6. Diff, difference between true and measured δ15N values. δ15Ntrue [‰] vs. δ15Nmean [‰].
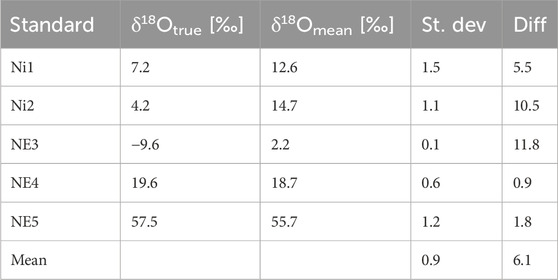
Table 2. True δ18O values of the standards (vs. VSMOW) (δ18ONO2-) and mean measured δ18O values (δ18O N2O) in Treatment 1, conversion of 20 nmol NO2−, measured using the CRDS technique. St. dev, standard deviation for n = 6. Diff, difference between true and measured δ18O values. δ18Otrue [‰] vs. δ18Omean [‰].
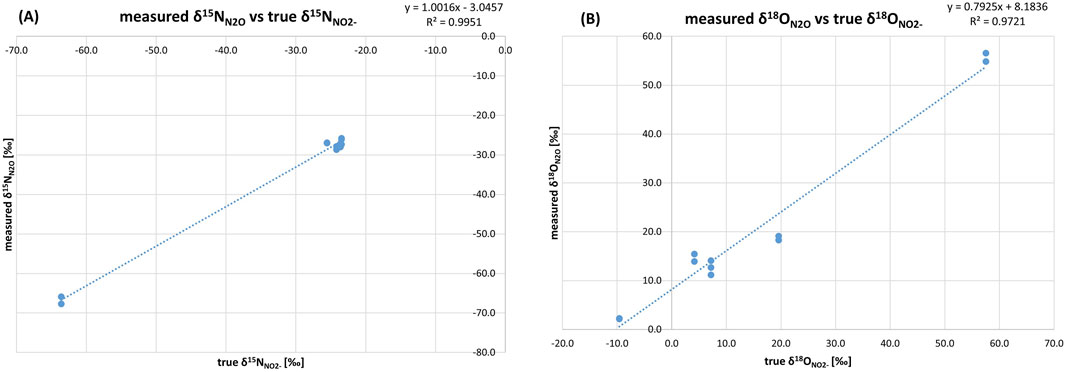
Figure 1. Relationship between N2O isotope data of standards for Treatment 1 and CRDS measurements: (A) true δ15NNO2- [‰] vs. measured δ15NN2O [‰] and (B) true δ18ONO2- [‰] vs. δ18ON2O [‰]. The slopes 1.0016 for δ15N and 0.7925 for δ18O indicate that the method is generally reliable. However, the slope for δ18O suggests oxygen exchange during sample preparation, which can influence the accuracy of the measurements. The intercepts −3.0457 for δ15N and 8.1836 for δ18O show systematic deviations in the measurements. R2 values of 0.9951 and 0.9721 indicate a strong linear relationship between the mean and true values, highlighting the stability of the observed isotopic shifts and reliable isotope normalization procedure.
2.5 Environmental samples from soil extracts
To extract nitrite from the soil samples, a 2 M KCl solution was prepared, and 50 mL of 2 M KOH was added to adjust pH to a range between 10 and 13 when mixed 1:1 with soil (the exact amount of KOH addition depends on the soil type). Then, 50 g of soil was placed in 100-mL extraction bottles, and 50 mL of the KCl + KOH solution was added. After shaking vigorously for 30 s and centrifuging at 2,000 g for 5 min, the supernatant was filtered using Whatman 42 ashless filters, with a pore size of 2.5 µm, and the filtrate was stored at 4°C until further treatment with S. nitritireducens. The pH of the solution was checked, ensuring a pH > 10. The extractions were performed directly in the field to minimize any nitrite transformations, and further bacterial denitrification analyses using S. nitritireducens were performed as soon as possible, but not later than 2 weeks after sample collection. The nitrite stability was positively tested for 1 month of storage. The soil nitrite extracts were added into the conversion vials in the possibly largest volume of 4 mL to prevent too strong dilution of the bacterial medium. The addition of the same sample in two different volumes was tested: 4 mL and 2 mL to check for any dilution effects.
3 Results and discussion
3.1 Isotope standards, Treatment 1
In this experiment, we applied the modified bacterial cultivation method (described in Section 2.3.1—referred to as Treatment 1), and produced N2O was measured using the CRDS technique. The standard amount of 20 nmol NO2− was added, same as in the original method by (Böhlke et al., 2007). Each standard (Ni1, Ni2, NE3, NE4, and NE5) was measured in triplicate repetition from the same conversion vial for two repeated conversions to test the reliability and reproducibility of our results. The measured N2O concentrations for bacterial blanks ranged from 138 to 227 ppb, which is significantly lower than the ambient atmospheric concentration and negligible compared to the N2O concentration of standard conversion, which is approximately 30 ppm (ca. 150 times higher than those of blanks).
Measured δ15NN2O values are very similar to the true δ15NNO2− values, with a mean difference of 3.1 between them, varying in a quite narrow range (Table 1). This difference reflects a consistent but slight systematic deviation across all standards, which highlights the capability of S. nitritireducens to accurately and consistently transfer the δ15N signature of NO2− to N2O, with a minimal and stable isotope shift. The good representation of the true NO2− values by the measured N2O values is further confirmed by the slope of their linear fit, which is 1.0016 (Figure 1A). The mean standard deviation of the measured δ15NN2O values of the six repetitions of ±0.7‰ indicates high reproducibility in a controlled experimental setup, only slightly higher than those previously reported by Böhlke et al. (2007), ranging from ±0.2 to ±0.5.
For δ18O, the mean standard deviation of ±0.9‰ is slightly higher (Table 2) than that of δ15N values (Table 1), but it generally maintains a reasonable accuracy across the dataset, suggesting good precision in our measurements. It is comparable to the values previously reported by Böhlke et al. (2007), ranging from ±0.4 to ±1.0‰. The use of six replicates per standard helped confirm the consistency of the observed data and minimized potential errors, enhancing the overall reliability of our findings.
For oxygen isotopes (Table 2), a mean difference of 6.1‰ for δ18Omean values was observed. The deviations between true δ18ONO2- and measured δ18ON2O ranged from 0.9‰ to a maximum of 11.8‰, with lower difference observed for the higher δ18O values. The δ18O values of the produced N2O are complex outcomes of possible O-isotope exchange between water and the denitrification intermediates and the branching effect by the disintegration of O-atoms by the reduction process (Casciotti et al., 2007; Lewicka-Szczebak et al., 2016). The extent of O-exchange is represented by the slope of the linear fit (a) between the true and measured values and equals (1-a) (Casciotti et al., 2007; Lewicka-Szczebak et al., 2016). For this set of standards, the O-isotope exchange reached 21%.
3.2 Alternative treatments tested
In this experiment, we applied the alternative bacterial cultivation methods (described in Section 2.3.2—referred to as treatments 2 and 3), and produced N2O was measured using the CRDS technique. The standard amount of 20 nmol NO2− was added, similar to the original method described by Böhlke et al. (2007). Each standard (Ni1, Ni2, NE3, NE4, and NE5) was measured in triplicate repetition from the same conversion vial for two repeated conversions. In contrast to Treatment 1 (described in Section 3.1), the alternative approaches (treatments 2 and 3) recorded significantly larger deviations from the true values. For Treatment 2, δ15NN2O deviations from the true δ15NNO2- values spanned from 6.9‰ to 12.6‰, with an average deviation of 10.2‰ ± 0.9‰, and the correlation between true δ15NNO2- [‰] vs. δ15NN2O [‰] values was strong, with an R2 value of the linear fit of 0.9813. Treatment 3 displayed δ15NN2O deviations ranging from 2.8‰ to 10.3‰, but with a smaller average deviation of 5.3‰ ± 1.8‰. The R2 value of the linear fit of 0.9612 indicates a slightly lower yet still significant correlation compared to that of Treatment 2. Blank samples in both treatments 2 and 3 had N2O concentrations significantly below the ambient levels. However, δ15NN2O deviations from the true δ15NNO2- values are substantially higher than any deviations recorded in Treatment 1 (Table 1). This indicated that there are some fractionation effects associated with probably incomplete reduction of NO2− to N2O or different fractionation factors associated with different treatments. Treatments 2 and 3 exhibited significantly higher standard deviations, especially in NE3 and NE5, suggesting reduced accuracy. These findings suggest that Treatment 1 maintained closer approximations to the true values and also ensured greater consistency across different samples, as reflected in lower standard deviations and higher degree of precision across all measurements, making it the preferable method for precise δ15N and δ18O isotope analyses.
Importantly, while these alternative treatments provide results with lower accuracy, the high correlations of the linear fits obtained still indicate a good representation of the true values by the measured values. Hence, by using the equation derived from the linear fits, the values can be well corrected and remain useful. This suggests that S. nitritireducens is a very robust bacterial strain, capable of surviving and quickly recovering under different experimental conditions, still providing adequate isotope results.
3.3 Repeatability and accuracy for isotope standards
To confirm the robustness of the results, the experiment was further repeated focusing exclusively on treatments 1 and 2, and each standard (Ni1, Ni2, NE3, NE4, and NE5) was prepared in five replicates to further ensure accuracy and consistency. In this experiment, we applied MS measurements to eliminate the slightly larger measurement error possibly associated with laser spectroscopy (applied in Sections 3.1, 3.2). However, to compare the repeated samples with mass spectrometry measurements, we needed to prepare more replicates for the standards as the whole gas aliquot needs to be used for single MS measurement. Therefore, five independent repetitions of standards were prepared and measured. Blank samples measured N2O concentrations ranging from 108 ppb to 157 ppb, which were significantly below the ambient levels, eliminating the need for background correction.
The results indicate that for δ15NN2O and δ18ON2O, Treatment 1 demonstrates superior accuracy and precision over Treatment 2. For δ15NN2O (Table 3), the average difference between true δ15NNO2- [‰] and measured δ15NN2O [‰] values was 5.0‰ ± 0.7‰, significantly lower than the 7.8‰ ± 2.0‰ observed for Treatment 2. This indicates that Treatment 1 yields values closer to the true isotopic ratios of the original nitrite. Additionally, it shows greater precision, evidenced by a lower standard deviation of 0.7‰ compared to 2.0‰ for Treatment 2, suggesting more consistent measurements. This consistency is further supported by a higher R2 value of 0.9826 compared to 0.9774 for Treatment 2 (Figure 2), reflecting a stronger linear correlation between the measured and true values and illustrating a closer alignment of Treatment 1 with the expected trend.
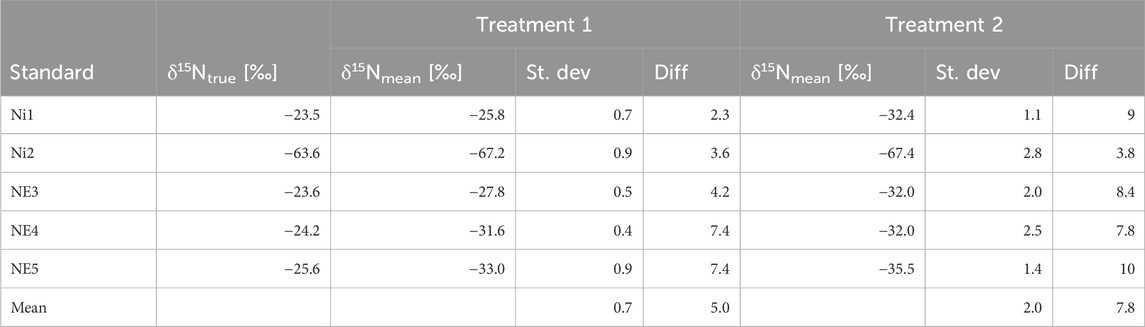
Table 3. True δ15N values of the standards (vs. air-N2) (δ15NNO2-) and mean measured δ15N values (δ15NN2O) in Treatment 1 and Treatment 2, conversion of 20 nmol NO2−, measured using the MS technique. St. dev, standard deviation for n = 5. Diff, difference between true and measured δ15N values. δ15Ntrue [‰] vs. δ15Nmean [‰] for treatments 1 and 2
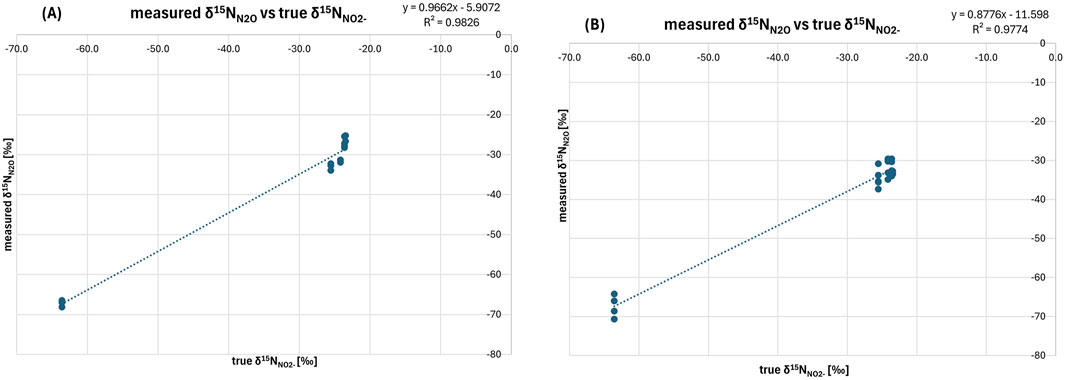
Figure 2. Relation between true δ15NNO2-and measured δ15NN2O [‰] for (A) Treatment 1 and (B) Treatment 2. The slope for Treatment 1 (0.9662) is close to 1, with a minor underestimation of true δ15N values, and a small negative bias shown by the intercept (−5.9072). The high R2 value (0.9826) confirms the consistency of this treatment. In contrast, Treatment 2 shows lower slope (0.8776) further deviating from 1, with a more pronounced negative bias (−11.598). The R2 value (0.9774) indicates a slightly weaker correlation, and the greater deviations in the slope and intercept suggest that Treatment 1 provides more accurate and consistent results overall.
For δ18ON2O (Table 4), the mean difference from true δ18ONO2- values in Treatment 1 was 10.5‰, while Treatment 2 showed a larger mean difference of 16.2‰. Additionally, the R2 value of the linear fit between true and measured δ18O values is higher for Treatment 1 (0.9986) compared to 0.9907 for Treatment 2 (Figure 3), which highlights a better fit, emphasizing the superior reliability of Treatment 1 for a stable isotope analysis.
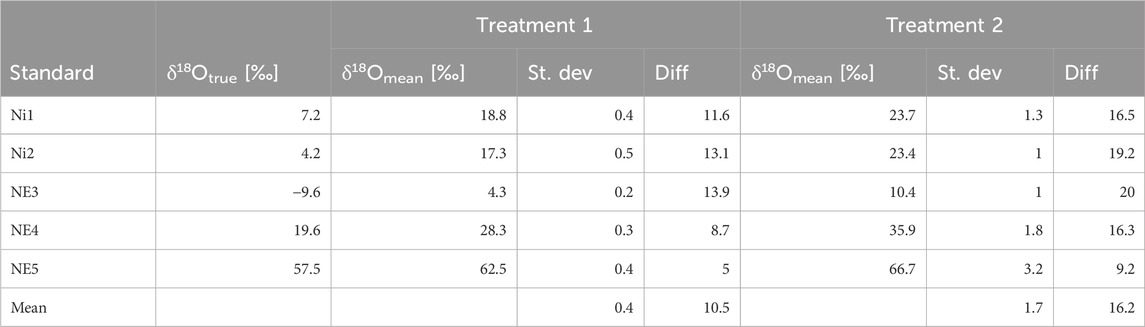
Table 4. True δ18O values of the standards (vs. VSMOW) (δ18ONO2-) and mean measured δ18O values (δ18ON2O) in Treatment 1 and Treatment 2, conversion of 20 nmol NO2−, measured using the MS technique. St. dev, standard deviation for n = 5. Diff, difference between true and measured δ18O values. δ18Otrue [‰] vs. δ18Omean [‰] for treatments 1 and 2
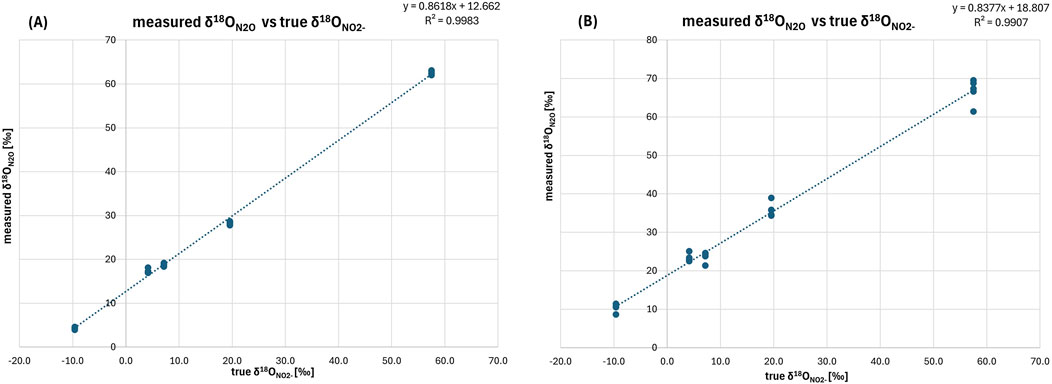
Figure 3. Relation between true δ18ONO2- and measured δ18ON2O [‰] for (A) Treatment 1 and (B) Treatment 2. Treatment 1 shows a slope (0.8618) below 1 indicating the O-isotope exchange of 14%. Despite this, high R2 value (0.9983) indicates the stability of this exchange and strong consistency of the measurements. Treatment 2 shows a slightly lower slope (0.8377), suggesting a slightly higher O-isotope exchange of 16%. The R2 value (0.9907) remains strong, but Treatment 1 demonstrates slightly greater reliability overall.
The slopes observed for δ18O indicate oxygen exchange levels of approximately 13.8% (Treatment 1) and 16.2% (Treatment 2) during sample preparation (Figure 3). These values are consistent with the azide method at pH 4.5 (11%–13%) and up to 88% at pH 1.5 (McIlvin and Altabet, 2005), but they are significantly higher than those obtained from bacterial denitrification methods, which typically exhibit negligible oxygen exchange under optimized conditions. Adjustments with standards of known δ18O values are crucial to address deviations from oxygen exchange, ensuring accurate correction of the results. In conclusion, Treatment 1 outperforms Treatment 2 due to its lower standard deviations, closer alignment with true values, and higher R2 values, indicating better accuracy and precision in both δ15NN2O and δ18ON2O measurements. This makes Treatment 1 more suitable for reliable N2O isotope analyses, particularly in scenarios where high precision is crucial.
Better fit between measured and true values presented in Section 3.3 than in Section 3.1, especially for δ18O (comparing Figures 1, 3), is due to the measurement techniques applied. The MS measurements are more precise since they are made directly from the high-concentration samples. As a result, no additional possible error during dilution occurs, and the final results are not amount-dependent, i.e., within the measured range of variations, no correction for the N2O concentration is needed. However, CRDS measurements allow us to measure lower concentrations. Hence, we can analyze one sample multiple times, thereby increasing precision. Although the results are slightly less accurate, they remain precise enough, showing a similar range of standard deviations for the repeated samples as previous studies with S. nitritireducens (Böhlke et al., 2007). The CRDS measurements are also preferable for environmental samples, with usually very low nitrite concentrations that may fall below the detection limit of MS.
3.4 Tests of low-concentration detection limit and environmental sample analyses
Nitrite, due to its high reactivity, typically occurs in very low concentrations in the environment; hence, it is crucial to test the applicability of this analytical method for the possibly lowest concentrations. We have tested this on our real soil sample extracts, which represent the quite low nitrite contents, typical for unamended field samples (Table 6). Simultaneously, we also measured standards in adequate low concentrations in a similar range to the analyzed samples, where the amounts of 2, 5, and 10 nmol of each standard were added for bacterial conversion. These tests indicated that lowering of the added standard amount (in the tested range) did not significantly change the analytical precision. For each set of concentrations, the standards were measured in at least four repetitions; the summary statistics are presented in Table 5.
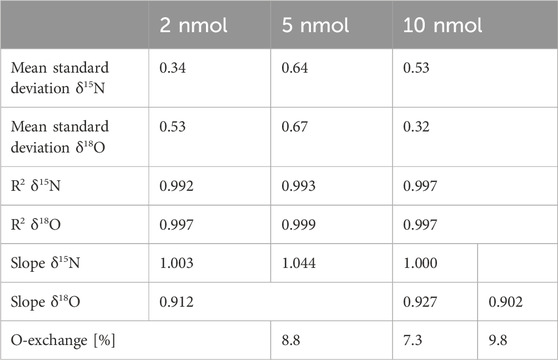
Table 5. Results of nitrite standard measurements of reduced nitrite concentrations with the addition of 1/10, 1/5, and ½ of the original amount of 20 nmol NO2−, as applied in previous studies (Böhlke et al., 2007), yielding NO2− additions of 2, 5, and 10 nmol, respectively. The mean statistics for all the standards (Ni1, Ni2, NE3, NE4, and NE5) are shown. For each standard, n = 4, so the reported values for the five standards represent n = 20.
The results of low-concentration standards present comparable and even slightly better precision compared to the high concentrations of 20 nmol NO2− addition tested initially (Tables 1–4). Importantly, the magnitude of the O-isotope exchange is smaller compared to previous tests, which can be due to the fact that these analyses were performed with freshly prepared standard solutions.
We have also applied the presented enhanced method for successful analyses of environmental samples of soil extracts. Usually, the nitrite concentration is the limiting factor for these analyses. Therefore, for the bacterial medium, prepared according to the presented method, the maximal possible volume of the sample is added: for one sample vial with 4 mL of the bacterial medium, a maximum of 4 mL of sample is added to avoid excessive dilution of the bacterial medium while maximizing the nitrite amount added. To test the reliability of the measurement, we have measured repeated samples of soil extracts. N2O from the conversion of soil extraction samples was measured with MS. The raw data were normalized with the linear fit between true NO2− and measured δN2O values of five internal standards, as described in Section 2.4.
The samples were measured in similar dilutions as the standards; consequently, the blank values were negligible, and the blank corrections were not necessary. We have used the repeated measurements of soil extracts originating from two sampling campaigns with two different study sites and soil types (Table 6). P-samples originate from the Pęgów agricultural study site (Poland) and include sandy and organic soils with mineral fertilizing. F-samples originate from the agricultural Wołczyn study site and include loam-sandy soils with organic fertilizers. The analytical precision is good for all the analyzed nitrite concentrations starting from below 150 nmol NO2− L−1 (Table 6).
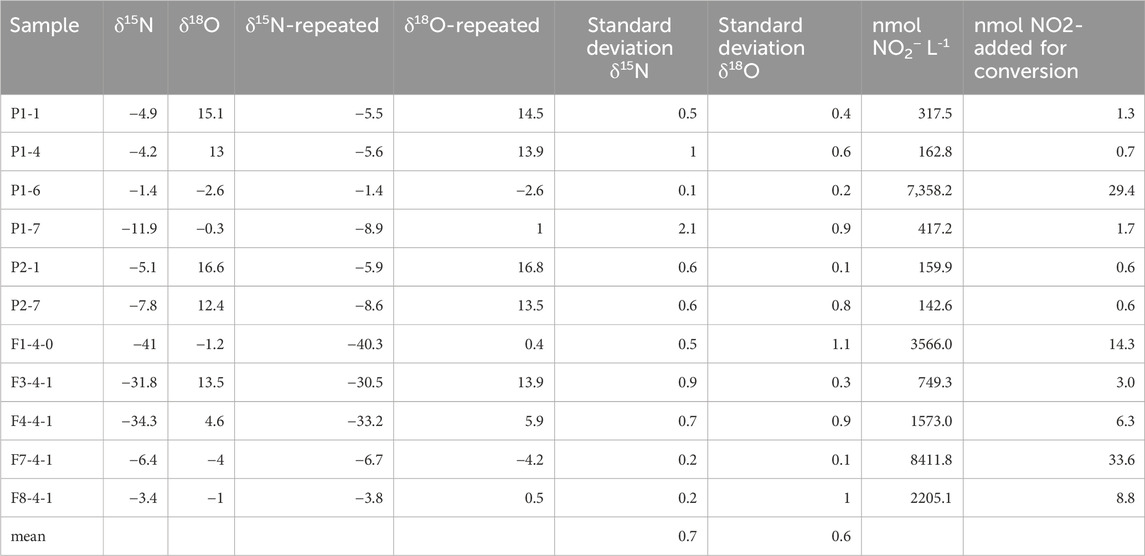
Table 6. Results of repeated soil extracts analyses of δ15N and δ18O. Standard deviations for two repeated samples are provided. The original concentrations of the samples in nmol NO2− L-1 are given and the amount of N-NO2- added for the conversion in the 4-mL sample addition.
4 Conclusion
The presented simplified method for nitrite isotope analyses allows for the preparation of S. nitritireducens bacterial media within 24 h, as opposed to approximately 3 weeks needed by the previously published approach (Böhlke et al., 2007). This simplification does not impact the precision of the measurements, which is comparable to the previous approach, with mean accuracies of 0.7‰ and 0.4‰ for δ15N and δ18O in this study, respectively, versus up to 0.5‰ for δ15N and up to 1‰ for δ18O (Böhlke et al., 2007; Dähnke and Thamdrup, 2013). In this study, we show the application for even lower concentrations, achieving reliable results for samples and standards with NO2− concentration >150 nmol NO2− L−1, whereas the original method was applied to much higher NO2− concentrations in water samples of ca. 2000 nmol NO2− L−1 (Böhlke et al., 2007; Dähnke et al., 2022) and a minimum of 500 nmol NO2− L−1 for soil extract analyses (Lewicka-Szczebak et al., 2021).
Data availability statement
The original contributions presented in the study are included in the article further inquiries can be directed to the corresponding author.
Author contributions
SD: conceptualization, data curation, funding acquisition, investigation, methodology, visualization, writing–original draft, and writing–review and editing. DL-S: conceptualization, formal analysis, funding acquisition, project administration, supervision, validation, writing–original draft, and writing–review and editing.
Funding
The author(s) declare that financial support was received for the research, authorship, and/or publication of this article. This study was financially supported by the “Polish Returns” programme of the Polish National Agency of Academic Exchange and the grants Opus-516204 (PI: Dominika Lewicka-Szczebak) and Preludium-522855 (PI: Sushmita Deb) of the National Science Centre Poland.
Conflict of interest
The authors declare that the research was conducted in the absence of any commercial or financial relationships that could be construed as a potential conflict of interest.
The author(s) declared that they were an editorial board member of Frontiers, at the time of submission. This had no impact on the peer review process and the final decision.
Generative AI statement
The author(s) declare that no Generative AI was used in the creation of this manuscript.
Publisher’s note
All claims expressed in this article are solely those of the authors and do not necessarily represent those of their affiliated organizations, or those of the publisher, the editors and the reviewers. Any product that may be evaluated in this article, or claim that may be made by its manufacturer, is not guaranteed or endorsed by the publisher.
References
Altabet, M. A., Wassenaar, L. I., Douence, C., and Roy, R. (2019). A Ti(III) reduction method for one-step conversion of seawater and freshwater nitrate into N 2 O for stable isotopic analysis of 15 N/14 N, 18 O/16 O and 17 O/16 O. Rapid Commun. Mass Spectrom. 33 (15), 1227–1239. doi:10.1002/rcm.8454
Böhlke, J. K., Smith, R. L., and Hannon, J. E. (2007). Isotopic analysis of N and O in nitrite and nitrate by sequential selective bacterial reduction to N 2 O. Anal. Chem. 79 (15), 5888–5895. doi:10.1021/ac070176k
Buchwald, C., and Casciotti, K. L. (2013). Isotopic ratios of nitrite as tracers of the sources and age of oceanic nitrite. Nat. Geosci. 6 (4), 308–313. doi:10.1038/ngeo1745
Campbell, D. H., Kendall, C., Chang, C. C. Y., Silva, S. R., and Tonnessen, K. A. (2002). Pathways for nitrate release from an alpine watershed: determination using δ 15 N and δ 18 O. Water Resour. Res. 38 (5). doi:10.1029/2001WR000294
Casciotti, K. L., Böhlke, J. K., McIlvin, M. R., Mroczkowski, S. J., and Hannon, J. E. (2007). Oxygen isotopes in nitrite: analysis, calibration, and equilibration. Anal. Chem. 79 (6), 2427–2436. doi:10.1021/ac061598h
Dähnke, K., Sanders, T., Voynova, Y., and Wankel, S. D. (2022). Nitrogen isotopes reveal a particulate-matter-driven biogeochemical reactor in a temperate estuary. Biogeosciences 19 (24), 5879–5891. doi:10.5194/bg-19-5879-2022
Dähnke, K., and Thamdrup, B. (2013). Nitrogen isotope dynamics and fractionation during sedimentary denitrification in Boknis Eck, Baltic Sea. Biogeosciences 10 (5), 3079–3088. doi:10.5194/bg-10-3079-2013
Deb, S., Lewicka-Szczebak, D., and Rohe, L. (2024). Microbial nitrogen transformations tracked by natural abundance isotope studies and microbiological methods: a review. Sci. Total Environ. 926, 172073. doi:10.1016/j.scitotenv.2024.172073
Denk, T. R. A., Mohn, J., Decock, C., Lewicka-Szczebak, D., Harris, E., Butterbach-Bahl, K., et al. (2017). The nitrogen cycle: a review of isotope effects and isotope modeling approaches. Soil Biol. Biochem. 105, 121–137. doi:10.1016/j.soilbio.2016.11.015
Finkmann, W., Altendorf, K., Stackebrandt, E., and Lipski, A. (2000). Characterization of N2O-producing Xanthomonas-like isolates from biofilters as Stenotrophomonas nitritireducens sp. nov., Luteimonas mephitis gen. nov., sp. nov. and Pseudoxanthomonas broegbernensis gen. nov., sp. nov. Int. J. Syst. Evol. Microbiol. 50 (1), 273–282. doi:10.1099/00207713-50-1-273
Granger, J., Boshers, D. S., Böhlke, J. K., Yu, D., Chen, N., and Tobias, C. R. (2020). The influence of sample matrix on the accuracy of nitrite N and O isotope ratio analyses with the azide method. Rapid Commun. Mass Spectrom. 34 (1), e8569. doi:10.1002/rcm.8569
Hu, H., Bourbonnais, A., Larkum, J., Bange, H. W., and Altabet, M. A. (2016). Nitrogen cycling in shallow low-oxygen coastal waters off Peru from nitrite and nitrate nitrogen and oxygen isotopes. Biogeosciences 13 (5), 1453–1468. doi:10.5194/bg-13-1453-2016
Jacob, J., Nowka, B., Merten, V., Sanders, T., Spieck, E., and Dähnke, K. (2017). Oxidation kinetics and inverse isotope effect of marine nitrite-oxidizing isolates. Aquat. Microb. Ecol. 80 (3), 289–300. doi:10.3354/ame01859
Knöller, K., Vogt, C., Haupt, M., Feisthauer, S., and Richnow, H.-H. (2011). Experimental investigation of nitrogen and oxygen isotope fractionation in nitrate and nitrite during denitrification. Biogeochemistry 103 (1–3), 371–384. doi:10.1007/s10533-010-9483-9
Lewicka-Szczebak, D., Dyckmans, J., Kaiser, J., Marca, A., Augustin, J., and Well, R. (2016). Oxygen isotope fractionation during N2O production by soil denitrification. Biogeosciences 13 (4), 1129–1144. doi:10.5194/bg-13-1129-2016
Lewicka-Szczebak, D., Jansen-Willems, A., Müller, C., Dyckmans, J., and Well, R. (2021). Nitrite isotope characteristics and associated soil N transformations. Sci. Rep. 11 (1), 5008. doi:10.1038/s41598-021-83786-w
McIlvin, M. R., and Altabet, M. A. (2005). Chemical conversion of nitrate and nitrite to nitrous oxide for nitrogen and oxygen isotopic analysis in freshwater and seawater. Anal. Chem. 77 (17), 5589–5595. doi:10.1021/ac050528s
McIlvin, M. R., and Casciotti, K. L. (2011). Technical updates to the bacterial method for nitrate isotopic analyses. Anal. Chem. 83 (5), 1850–1856. doi:10.1021/ac1028984
Müller, C., Laughlin, R. J., Spott, O., and Rütting, T. (2014). Quantification of N2O emission pathways via a 15N tracing model. Soil Biol. Biochem. 72, 44–54. doi:10.1016/j.soilbio.2014.01.013
Nikolenko, O., Jurado, A., Borges, A. V., Knӧller, K., and Brouyère, S. (2018). Isotopic composition of nitrogen species in groundwater under agricultural areas: a review. Sci. Total Environ. 621, 1415–1432. doi:10.1016/j.scitotenv.2017.10.086
Pajares, S., Soto-Jiménez, M. F., and Merino-Ibarra, M. (2019). Molecular and isotopic evidence of the distribution of nitrogen-cycling microbial communities in the oxygen minimum zone of the Tropical Mexican Pacific. FEMS Microbiol. Ecol. 95, fiz143. doi:10.1093/femsec/fiz143
Rütting, T., Aronsson, H., and Delin, S. (2018). Efficient use of nitrogen in agriculture. Nutrient Cycl. Agroecosyst. 110 (1), 1–5. doi:10.1007/s10705-017-9900-8
Sainju, M., Ghimire, R., and Pradhan, P. (2020). “Nitrogen fertilization I: impact on crop, soil, and environment,” in Nitrogen fixation. IntechOpen. doi:10.5772/intechopen.86028
Sebilo, M., Aloisi, G., Mayer, B., Perrin, E., Vaury, V., Mothet, A., et al. (2019). Controls on the isotopic composition of nitrite (δ15N and δ18O) during denitrification in freshwater sediments. Sci. Rep. 9 (1), 19206. doi:10.1038/s41598-019-54014-3
Sigman, D. M., Casciotti, K. L., Andreani, M., Barford, C., Galanter, M., and Böhlke, J. K. (2001). A bacterial method for the nitrogen isotopic analysis of nitrate in seawater and freshwater. Anal. Chem. 73 (17), 4145–4153. doi:10.1021/ac010088e
Sigman, D. M., Granger, J., DiFiore, P. J., Lehmann, M. M., Ho, R., Cane, G., et al. (2005). Coupled nitrogen and oxygen isotope measurements of nitrate along the eastern North Pacific margin. Glob. Biogeochem. Cycles 19 (4). doi:10.1029/2005GB002458
Stock, P., Roder, S., and Burghardt, D. (2021). Further optimisation of the denitrifier method for the rapid 15 N and 18 O analysis of nitrate in natural water samples. Rapid Commun. Mass Spectrom. 35 (1), e8931. doi:10.1002/rcm.8931
Keywords: nitrite, isotope, water, soil, bacterial denitrification method
Citation: Deb S and Lewicka-Szczebak D (2025) Simplified bacterial denitrification method using Stenotrophomonas nitritireducens for nitrite dual isotope analysis in low-concentration environmental samples. Front. Environ. Sci. 13:1536882. doi: 10.3389/fenvs.2025.1536882
Received: 29 November 2024; Accepted: 12 February 2025;
Published: 03 March 2025.
Edited by:
Jakob Zopfi, University of Basel, SwitzerlandReviewed by:
Annie Bourbonnais, University of South Carolina, United StatesPaul Magyar, Swiss Federal Laboratories for Materials Science and Technology, Switzerland
Copyright © 2025 Deb and Lewicka-Szczebak. This is an open-access article distributed under the terms of the Creative Commons Attribution License (CC BY). The use, distribution or reproduction in other forums is permitted, provided the original author(s) and the copyright owner(s) are credited and that the original publication in this journal is cited, in accordance with accepted academic practice. No use, distribution or reproduction is permitted which does not comply with these terms.
*Correspondence: Sushmita Deb, c3VzaG1pdGEuZGViQHV3ci5lZHUucGw=