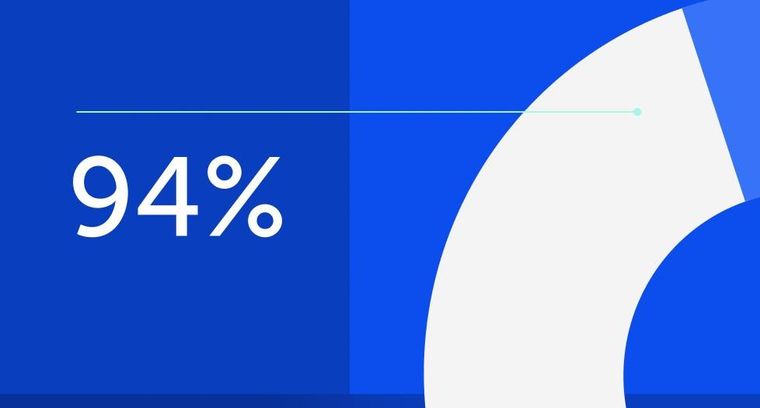
94% of researchers rate our articles as excellent or good
Learn more about the work of our research integrity team to safeguard the quality of each article we publish.
Find out more
ORIGINAL RESEARCH article
Front. Environ. Sci., 08 April 2025
Sec. Toxicology, Pollution and the Environment
Volume 13 - 2025 | https://doi.org/10.3389/fenvs.2025.1531800
Introduction: The primary source of anthropogenic atmospheric mercury (Hg) emissions globally is artisanal and small-scale gold mining (ASGM). Estimates of Hg emissions from ASGM are poorly constrained due to a lack of monitoring data and the informal, generally unregulated nature of this industry. Trees accumulate atmospheric gaseous elemental mercury (GEM) in bolewood following stomatal uptake and thus have the potential to be used as biomonitors to quantify the spatial and temporal footprint of Hg emissions from ASGM.
Methods: We collected tree cores from Ficus insipida at three mining-impacted and two remote, unimpacted sites in the Peruvian Amazon (n = 4 trees per site).
Results: We show that tree ring Hg concentrations were higher near ASGM activity located near mining towns (6.0 ng g−1) compared to remote sites (0.9 ng g−1) and recent tree rings were strongly linearly correlated with atmospheric GEM concentrations across all sites (p < 0.0001, r2 = 0.64), especially in the dry season when there is enhanced ASGM activity (p < 0.0001, r2 = 0.76), highlighting the potential for tree rings to be used as biomonitors for GEM. At the most impacted sites, tree-ring Hg increased over time in response to intensification of ASGM.
Discussion: Thus far, applications of dendrochemistry to quantify Hg pollution have been largely restricted to coniferous species in temperate regions, but this study shows that tropical species also quantify Hg pollution. We conclude that Ficus insipida is a suitable biomonitor and powerful tool for characterizing the spatial, and potentially temporal footprint of GEM emissions from ASGM in the neotropics.
Mercury (Hg) can cause neurological, cardiovascular, reproductive, and behavioral impairment when inhaled as Hg vapor or consumed as methyl Hg (MeHg) in food (Weis, 2009; Evers et al., 2012; Ha et al., 2017). The primary source of anthropogenic Hg emissions to air globally is artisanal and small-scale gold mining (ASGM) (UNEP, 2012). ASGM occurs in over 70 countries throughout the world – primarily in South America, southeast Asia, and sub-Saharan Africa – and is estimated to involve over 10 million people (Telmer and Veiga, 2009; Schmidt, 2012) releasing over 600 tons of Hg to the atmosphere annually (UNEP, 2018; Cheng et al., 2023). Miners add Hg to dredged soil and sediment containing gold, resulting in the formation of an amalgam that can be readily separated. Once the amalgam is recovered, the Hg is burned off, releasing gaseous elemental Hg (GEM or Hg0) into the air, which can lead to contamination of terrestrial and aquatic ecosystems. Due to the severe impacts of high Hg exposure, reducing anthropogenic Hg emissions, including from ASGM, has been a primary target of local, national and international health and environmental agendas, including more recently the UNEP Minamata Convention on Mercury (UNEP, 2018).
ASGM has occurred in Peru for centuries, particularly in the Madre de Dios region, where over 30,000 illegal and informal gold miners (Fraser, 2009) are responsible for most of the country’s gold production. In this Western Amazonia region, there has been an estimated 400% increase in geographic extent of ASGM between 1999 and 2012 (Swenson et al., 2011; Asner et al., 2013), a rate of expansion that is expected to continue as gold prices remain high. This region also has the highest annual fluxes of Hg of any studied site globally (Gerson et al., 2022). Recent increases in Hg pollution in areas of the Peruvian Amazon affected by ASGM are a major concern to both human and wildlife health.
Estimates of Hg emissions from ASGM are poorly constrained due to a lack of monitoring data and the informal, unregulated nature of this industry (Bruno et al., 2023; Keane et al., 2023). Mercury released from ASGM can be converted to toxic MeHg, then bioaccumulated and biomagnified through aquatic food webs, reaching concentrations in fish that may be harmful to the health of consumers (Diringer et al., 2015; Eagles-Smith et al., 2018). To better understand the potential impact of increasing Hg deposition to local wildlife, humans, and the environment, further research is needed on the spatial and temporal dynamics of Hg from ASGM in the Madre de Dios basin.
Once released into the atmosphere, GEM can be taken up into leaves and subsequently oxidized (Liu et al., 2021; Yamakawa et al., 2021; Gustin et al., 2022; McLagan et al., 2022). This oxidized Hg (Hg2+) is then transported via phloem transport to bolewood, where it is archived over time, though the exact mechanism of transport within trees is not fully understood (Zhou et al., 2021; McLagan et al., 2022; Gačnik and Gustin, 2023). Uptake of Hg from soils into bolewood and leaves is minimal (Fleck et al., 1999; Ericksen et al., 2003; Stamenkovic and Gustin, 2009; Arnold et al., 2018). Tree-ring Hg records from northern and temperate regions have been used to reconstruct long-term changes in local atmospheric GEM at both pristine (Clackett et al., 2018; Peckham et al., 2019; Eccles et al., 2020; Ghotra et al., 2020) and polluted sites near industrial point sources (Abreu et al., 2008; Maillard et al., 2016; Navrátil et al., 2017; 2018; Schneider et al., 2019; Clackett et al., 2021; Nováková et al., 2022). Although many tree-ring records appear to preserve temporal Hg trends, some softwoods and hardwoods are likely prone to translocation and diffusion of Hg across ring boundaries, which could distort the apparent timing and amplitude of Hg signals in tree rings (Nováková et al., 2021; Wang et al., 2021). Regardless of temporal uncertainty in tree-ring Hg due to translocation, average bolewood Hg concentration offers a powerful and cost-effective proxy for investigating spatial variability in atmospheric Hg at local to regional scales (Siwik et al., 2010; Odabasi et al., 2016; Wright et al., 2014; Eccles et al., 2020).
This study is the first to examine neotropical tree-ring Hg concentrations as a biomonitoring tool to quantify the spatial and temporal footprint of Hg exposure related to ASGM activity in the Amazon. We analyzed tree-ring total Hg concentrations at sites near and remote from ASGM activity, capturing the pre- (1941–1999) and post-mining expansion (2000–2019) eras. Specifically, we asked: 1) How do tree-ring Hg concentrations differ between mining-impacted and remote sites; 2) How do tree-ring Hg concentrations compare to GEM and leaf Hg concentrations; and 3) What histories of Hg emissions can be traced using tree-ring analysis in the Peruvian Amazon?
We established five study sites along a 200 km reach of the Madre de Dios River (see Gerson et al., 2022; Figure 1) based on proximity to intense ASGM activities. Each site was located approximately 50 km from each other and was accessible by boat along the Madre de Dios River. Three sites were adjacent to ASGM activity (hereafter, “mining sites”): Boca Colorado and Laberinto located in secondary growth forest near (i.e., within 5 km) to mining towns where Hg-gold amalgams are frequently burned, and Los Amigos located in primary growth protected forest. The exact distance of these sampling sites from mining activity is unknown, since these activities are generally illegal and clandestine. Two sites, Boca Manu and Chilive, were located in primary growth forest but were far from ASGM activity (approximately 100 and 50 km from ASGM activity, respectively; hereafter, “remote sites”). In Madre de Dios, mining occurs in rivers and Hg amalgamation occurs on both land and in waterbodies. ASGM activity in Madre de Dios is seasonal, with higher intensity of mining occurring during the dry season. While ASGM has occurred in the Madre de Dios region for several decades, ASGM activity has expanded drastically since 1999 (Asner et al., 2013; Swenson et al., 2011; Espejo et al., 2018).
Figure 1. Sampling sites in Madre de Dios, Peru. Two sites are remote from ASGM activity, and three sites are located near to ASGM activity. Sites shown in green circles have old-growth primary forests, and sites shown in grey circles have young secondary growth forests. Blue triangles represent mining towns where Hg-gold amalgams are burned.
The Madre de Dios region has a relatively equitable thermal regime (∼3°C range in average monthly temperatures from 2001 to 2018) with mean monthly temperatures peaking in October (25.2°C) and reaching a minimum in July (21.8°C). However, the precipitation regime (2001–2018 mean annual precipitation of 2,550 mm) has a distinct seasonal cycle with over 70% of annual precipitation delivered in September-March (Supplementary Figure S1). Note that all climate data are collected as part of the monitoring program at Los Amigos Biological Station (available from Conservación Amazónica upon request).
Tree cores were collected in July-August 2019 at each of the five study sites using a 12 mm diameter, 30 cm long increment borer (Haglöf). All cores were collected according to the “clean hands-dirty hands” protocol (EPA Method 1669), ensuring that only “clean hands” came in contact with samples while “dirty hands” manipulated the cores and opened sample bags and containers. Cores were obtained as close to the study plots as possible from three prominent tree species in the area – Ficus insipida, Bertholletia excelsa, and Cedrelinga catenaformis – as potential targets for tree-ring Hg analysis. Four individual trees were sampled per species per site. Corers were cleaned with milliQ water between each sample collection. In all but one case, tree cores did not reach the tree pith, suggesting both that we sampled mature trees and that the rings do not correspond to juvenile growth. We therefore do not anticipate age effects to be a confounding factor in our analysis.
To use Hg concentrations in tree cores as a proxy for historical ASGM Hg emissions, trees must exhibit annual growth rings. While annual rings are common in temperate forests, they are less common in the tropics due to an equitable year-round climate. Nevertheless, annual rings can occur in tropical trees that experience a robust dry season, which triggers cambial dormancy and the formation of annual tree ring boundaries during the wet season (Worbes, 1989; Worbes, 1995). Three tree species that previous studies have found to produce annual growth rings were targeted for sampling: F. insipida, B. excelsa, and C. catenaformis (Worbes, 2002; Brienen and Zuidema, 2006; Schöngart et al., 2007; Schöngart et al., 2015). However, after a visual inspection of the core samples under magnification, F. insipida was the only species with discernible growth rings and was therefore selected for our tree-ring Hg analysis. Note that F. insipida trees range from Mexico to northern South America. The seasonally dry climate of this area (Supplementary Figure S1) is a likely driver of the F. insipida growth bands, which are defined in transverse section by an alternating pattern of parenchyma and fiber cells, a pattern that is common to other trees in the Moraceae family (Schöngart et al., 2007). All F. insipida trees sampled were from within forested areas and were not located in edge habitats or clearings. Cores were transported on ice and stored at −4°C until processing.
Prior to ring dissection, cores were mounted to wood blocks using a small amount of wood glue, which was later removed with a scalpel prior to Hg analysis. The mounted cores were lightly sanded to allow for macroscopic ring identification and a hand-held vacuum was used to remove dust and prevent contamination. Most samples showed relatively clear growth increments, defined by alternating patterns of parenchyma and fiber cells, that are assumed to represent the annual cycle of cambial dormancy triggered by the dry season (Supplementary Figure S1), as found in other F. insipida studies (Schöngart et al., 2007).
Calendar years were assigned to each identifiable ring counting back in time from the outermost ring, representing the year of sampling (2019). For each tree, a clean scalpel was used to dissect growth rings in 5-year blocks (e.g., 2019–2016, 2015–2011, 2010–2006, 2005–2001, etc) back to 1941, except for one tree that had a pith age of 1946, and to shave the outer surface to remove any potential surficial contamination that accumulated since the time of collection. The scalpel was wiped with ethanol between dissections.
The series of ring widths for the sample trees can be described as complacent, lacking any clear pointer rings; thus, standard tree-ring cross-dating methods (e.g., skeleton plotting) could not be used to confirm calendar year ages. Although each ring is counted as a calendar year, we cannot exclude the possibility that some rings are missing from the total ring sequence due to the well-known issue of ring-wedging in tropical trees related to irregular trunk geometry and understory-canopy growth dynamics (Worbes, 2002). This issue can be largely mitigated if full cross-sections are collected (Worbes, 2002), but was not possible in this study due to the large diameter of the trees (generally >1 m). Therefore, ring dates assigned to the juvenile growth rings (towards the pith) in this study may slightly underestimate the true age of those particular rings. However, dating error is likely negligible in the recent decades of the ring record. Dating uncertainty in complacent ring series can further complicate direct comparison of temporal trends in tree-ring Hg and may explain some minor differences. However, considering the distinct seasonal climate regime of this area (Supplementary Figure S1), it is likely that most growth increments represent a full growth season and that dating uncertainty is small (e.g., less than ±10 years) since the start of the record.
Quantification of total Hg concentrations in tree-ring samples was carried out in the Environmental and Aquatic Biogeochemistry Lab at the University of Toronto–Mississauga following previously described protocols (Ghotra et al., 2020; Clackett et al., 2018; Clackett et al., 2021). Prior to analysis, dissected 5-year blocks of increment tree-ring samples were oven-dried for 48 h at 65°C (Yang et al., 2017) to remove adsorbed and interstitial water. Dried tree-ring samples were weighed to determine dry mass and stored in an airtight jar containing desiccant until Hg analysis, which was carried out within 24 h of the drying process. Total Hg concentrations were quantified using a Milestone tri-cell Direct Mercury Analyzer (DMA-80) by thermal decomposition, reduction, amalgam pre-concentration, and atomic absorption (US EPA Method 7473) (Supplementary Table S1). A certified aqueous Hg standard (High Purity Standards 1,000 μg/mL Hg, 2% HCl) was used to produce a 10-point Hg dilution series to generate the quadratic calibration curve encompassing the range of Hg concentrations measured in the tree-ring samples. Analytical performance was evaluated before and during each sample run using two different Standard Reference Materials (SRMs): NIST 1575a Pine Needle Standard, for which we obtained a mean (± standard deviation) of 36.7 ± 0.5 ng g−1 (n = 37, %RSD = 1.5), corresponding to a 92.0% recovery of the certified concentration (39.9 ± 0.7 ng g−1); and NIST 1547 Peach Leaves Standard, 30.1 ± 0.6 ng g−1 (n = 30, %RSD = 1.9%), corresponding to a 94.8% recovery of the certified concentration (31.7 ± 4.3 ng g−1). Blanks and SRMs (and/or an independent calibration liquid standard) were evaluated every ten samples. All blanks were below the detection limit, and all reported concentrations are for dry mass.
We also collected GEM using passive air samplers (developed by McLagan and colleagues (McLagan et al., 2016); University of Toronto) at each of the five sites for 2 months during the dry season (July–August 2018; n = 1 per site) and 1 month in the wet season (December 2018–January 2019; n = 2 per site). Passive air samplers were transported to and from the field in double resealable plastic bags and sealed with parafilm. They were deployed approximately 1 m above the ground using plastic cable ties. Field blanks and trip blank passive air samplers were also collected. The passive air sampler contains a Radiello© diffusive barrier, which allows GEM to pass through and sorb onto a sulfur-impregnated carbon sorbent (HGR-AC) while preventing the passage of gaseous organic Hg species. More details on the passive air samplers, deployment locations, calculation of GEM concentrations, laboratory quality assurance/quality control (QA/QC), and interpretation are described in Gerson et al., 2022.
We collected canopy leaves from F. insipida using a Notch Big Shot slingshot during the 2018 dry season, 2018 wet season, and 2019 dry season. This method was deployed to ensure that leaves were collected from the tops of the tree canopy, representing the first leaves that intercept air masses. Since total Hg concentrations in leaves and foliar Hg uptake rates vary by height and density of the canopy (Wohlgemuth et al., 2020), this method ensures that leaves collected were comparable by site. Leaves were collected using clean-hands dirty-hands protocol, stored and shipped frozen, rinsed with ultrapure water, and lyophilized prior to analysis in duplicate on a Milestone tri-cell DMA-80 by thermal decomposition, reduction, amalgam pre-concentration, and atomic absorption (US EPA Method 7473). Leaves were collected with a SERFOR collection permit and imported frozen to the United States with a USDA import permit. More details on leaf collection, processing, and analysis QA/QC are described in Gerson et al., 2022.
We compared average total Hg concentrations in 5-year increments of F. insipida tree rings between sites and between years (1941–1999 vs 2000–2019) using an ANOVA followed by Tukey’s post hoc analysis. We also compared average total Hg concentrations in tree rings across the entire time interval (1941–2019) and most recent time interval (2016–2019 for 4 sites; 2011–2019 for Chilive) to GEM concentrations and F. insipida leaf concentrations from the same sites using a linear regression. We evaluated temporal trends in 5-year increments of tree-ring Hg concentrations by site using break point analysis (segmented package in R) and an ANOVA with Tukey’s post hoc analysis comparing pre- and post-2000 Hg concentrations. The year 2000 was chosen due to the general intensification of ASGM activity in the Peruvian Amazon around this time (Swenson et al., 2011; Gerson et al., 2020); ASGM activity increased by ∼400% between 1999 and 2012 and has continued to increase in the past decade (Swenson et al., 2011; Asner et al., 2013; Gerson et al., 2020). Finally, we calculated the variability among individual trees at each site and compared this inter-tree variability between sites. All analyses were performed in R version 4.2.2, and significance was evaluated at an alpha value of 0.05.
Mean total Hg concentrations in tree rings differed across the study sites and were indicative of spatial variability in Hg exposure and proximity to Hg point sources. Across the entire time interval (1941–2019), Hg concentrations were highest at the two mining-impacted sites located near mining towns (i.e., closer to ASGM activity and larger amounts of Hg burned; Laberinto: 5.4 ± 3.2 ng g−1 and Boca Colorado: 6.6 ± 4.3 ng g−1), compared to the mining-impacted site located adjacent to protected forest (Los Amigos: 2.4 ± 1.4 ng g−1) and the two remote sites (Boca Manu: 0.99 ± 1.4 ng g−1 and Chilive: 0.74 ± 0.78 ng g−1) (p < 0.0001; Figure 2). This same trend of elevated Hg concentrations at the two mining-impacted sites near mining towns was apparent when restricting the analysis to the period between 2000 and 2019 (p < 0.05). In contrast, Hg concentrations at Los Amigos – the mining-impacted site located in a protected forest – were only marginally higher than the remote sites of Boca Manu (p = 0.078) and Chilive (p = 0.060). This suggests that bolewood Hg concentrations are primarily driven by proximity to mining towns where Hg-gold amalgams are burned – thus releasing GEM to the air in a constant and concentrated manner – rather than simply the presence of mining activity in the area – where amalgam burning may occur but in a more dispersed manner.
Figure 2. Concentration of total Hg in Ficus insipida tree cores from all five sites (n = 4 trees per site) from before (pre-2000: 1941–1999) and after (post-2000: 2000–2019) ASGM intensification. Sites are shown from most remote to most mining-impacted, with Boca Manu and Chilive as remote sites (to the left of the dotted line) and Los Amigos, Laberinto, and Boca Colorado, as mining-impacted sites (to the right of the dotted line). Additionally, Laberinto and Boca Colorado are located near mining towns where Hg amalgams are burned to recover gold. Each site is located ∼50 km from each other. In the boxplots, the line represents the median value, the box shows Q1 and Q3, and the whiskers denote 1.5 times the interquartile range.
Indeed, across all sites, Hg concentrations in the most recent tree rings (2016–2019) were strongly correlated with atmospheric GEM concentrations (p < 0.0001, r2 = 0.64; Figure 3A). This pattern was particularly apparent for the dry season GEM when most ASGM activity occurs due to the favorable meteorological conditions for mining activity (Gerson et al., 2022), most tropical tree growth is estimated to occur (Zuidema et al., 2022), and thus uptake of GEM by vegetation is likely highest (p < 0.0001, r2 = 0.76; Figure 3B). This relationship ([2016–2019 Tree core total Hg (ng g−1)] = 0.0011* [Dry season GEM ng m−3] – 0.000009) can then be used as a quantitative and predictive model for F. insipida bolewood Hg concentrations. This relationship also held when considering the larger time interval of the full tree ring record for the dry season (p < 0.0001, r2 = 0.69; Figure 3D) and for both seasons (p = 0.0011, r2 = 0.43; Figure 3C).
Figure 3. Comparison of gaseous elemental mercury (GEM) concentrations [reported in Gerson et al. (2022)] to tree core total Hg concentrations for all five sites in Madre de Dios, Peru. (A) GEM concentrations for the 2018 dry season, the season with the majority of ASGM activity, for the most recent (2016–2019) tree-ring Hg record. (B) GEM concentrations averaged across the dry (2018) and wet (2018–2019) seasons for the most recent (2016–2019) tree-ring Hg record. (C) GEM concentrations for the 2018 dry season, the season with the majority of ASGM activity, for the entire tree-ring Hg record. (D) GEM concentrations averaged across the dry (2018) and wet (2018–2019) seasons for the entire tree-ring Hg record.
Using previously reported total Hg concentrations in F. insipida leaves for the five sites (Gerson et al., 2022), we find that tree-ring Hg concentrations were also significantly correlated to F. insipida leaf Hg concentrations. This relationship was stronger using the most recent tree rings (p < 0.0001, r2 = 0.60 for all leaf data; p < 0.0001, r2 = 0.62 for dry season leaf data; Figures 4A, B) compared to average values for all tree-ring data (p = 0.0021, r2 = 0.39 for all leaf data; p = 0.0015, r2 = 0.41 for dry season leaf data; Figures 4C, D). This pattern likely reflects similar uptake pathways of GEM by the leaves and bolewood (Gačnik and Gustin, 2023). It is also possible that variation between the height the passive air samplers were deployed (∼1 m) and the height the leaves were collected (tree canopy) influenced the relationship between leaf Hg concentrations and tree core Hg concentrations; however, we expect impacts of passive air sampler deployment height to be minimal and lead to consistent bias between sites.
Figure 4. Comparison of leaf total Hg concentrations (reported in Gerson et al., 2022) to tree core total Hg concentrations for all five sites in Madre de Dios, Peru. (A) Leaf total Hg concentrations for the 2018 dry season, the season with the majority of ASGM activity, for the most recent (2016–2019) tree-ring Hg record. (B) Leaf total Hg concentrations averaged across the dry (2018) and wet (2018–2019) seasons for the most recent (2016–2019) tree-ring Hg record. (C) Leaf total Hg concentrations for the 2018 dry season, the season with the majority of ASGM activity, for the entire tree-ring Hg record. (D) Leaf total Hg concentrations averaged across the dry (2018) and wet (2018–2019) seasons for the entire tree-ring Hg record.
The presence of temporal trends in tree-ring Hg concentrations at each site was evaluated using two different approaches, a break point analysis and an ANOVA comparing pre- and post-2000 Hg concentrations. Significant increases in Hg concentrations over time were identified at the two sites adjacent to mining towns where amalgams are burned, Laberinto and Boca Colorado. A significant breakpoint occurred in 2005 in the Laberinto time-series (r2 = 0.42, p = 0.0004), after which tree-ring Hg concentrations increased. At Boca Colorado, tree-ring Hg concentrations were higher post-2000 (8.6 ± 6.0 ng g−1) relative to pre-2000 (6.0 ± 3.4 ng g−1; p = 0.030; Figure 2), but no breakpoints or differences in Hg concentrations pre- and post-2000 were observed at the other sites (p > 0.05). While temporal trends were not consistent across all sites, or even detected at all sites, at the mining-impacted sites of Boca Colorado and Laberinto, the maximum Hg concentration (0.0057–0.025 ng g−1) occurred after 2006 in 7 of the 8 sampled trees, likely due to increased Hg emissions from ASGM (Figure 5). By contrast, at the other three sites, the maximum Hg concentration occurred after 2006 in only 58% of sampled trees (7 of 12). These shifts in Hg concentrations in tree rings could be due to various factors, including changes in ASGM activity patterns or intensity as well as socio-political events (e.g., completion of the Interoceanic Highway, policy changes, political office changes) that affect Hg emissions.
Figure 5. Concentration of total Hg in Ficus insipida tree rings over time at all five sites. Sites are shown from most remote to most mining-impacted, with Boca Manu and Chilive as remote sites and Los Amigos, Laberinto and Boca Colorado as mining-impacted sites. Colors represent tree cores taken from different individual trees within the site (n = 4 per site). The dark gray line at the year 2000 indicates the approximate time when ASGM activity intensified.
The absence of an increase in tree-ring Hg concentrations over time in response to increased ASGM activity at Los Amigos (the mining-impacted site located in primary growth protected forest) is consistent with the lower GEM concentrations (2.6 ng m−3) measured there in 2018 relative to the other two mining-impacted sites [∼10 ng m−3, though ∼2.5 times typical South Hemisphere background concentrations (Sprovieri et al., 2016)], suggesting that any potential temporal increase in GEM at Los Amigos may have been more modest. Los Amigos has less intense mining activity compared to the other two mining-impacted sites. It is also likely that ASGM-related Hg emissions at Los Amigos are primarily in the form of particulate Hg and/or gaseous oxidized Hg2+, which is supported by the high Hg concentrations in throughfall measured at this site by Gerson et al. (2022). Any potential increase in particulate or gaseous oxidized Hg is not expected to be reflected in the tree-ring Hg record, due to the specificity of tree-ring Hg as a proxy for GEM. Similarly, the absence of a significant temporal trend at the two remote sites is not unexpected. While the tree-ring Hg record at the remote sites may be expected to follow temporal trends in global or regional background tropospheric GEM concentrations, the length of the tree-ring timeseries and the high degree of inter-tree variability (Figure 5; Supplementary Table S2) suggest that there was insufficient statistical power to detect more subtle temporal trends from changes in background GEM concentrations and that this question could potentially be resolved by sampling more trees per stand in future studies to confirm spatial patterns.
Another factor to consider when analyzing temporal trends in tree-ring Hg is the potential for inward translocation of xylem Hg. While the physical processes driving inward redistribution of xylem Hg via diffusion and advection are not well understood, the degree to which a tree-ring Hg record is affected appears to be highly species dependent (Scanlon et al., 2020; Gustin et al., 2022) and therefore may be related to wood anatomy. For example, a recent study (Nováková et al., 2022) found that some species of Larix with a narrow sapwood zone were relatively unaffected by translocation compared to some Pinus species with a large sapwood zone. This study lays the foundation for the use of F. insipida as a spatial indicator of Hg in trees. Further studies comparing Hg in tree rings at sites with known histories of atmospheric Hg emissions or concentrations are required to determine whether Hg in F. insipida xylem is prone to translocation or suitable for evaluating temporal trends.
Consistent with previously published records of Hg in tree rings, inter-tree variability was relatively high, and individual trees did not always exhibit consistent temporal trends (Figure 5; Supplementary Table S2). Studies from open-canopy Picea glauca stands in northern Canada – where large numbers (up to 20 trees) of tree-ring Hg replicates were examined – have demonstrated systematic differences in mean Hg concentration among trees growing at the same site and exposed to the same atmosphere (Clackett et al., 2018; Clackett et al., 2021; Eccles et al., 2020; Ghotra et al., 2020). As a result, tree-ring Hg series typically have high variability and a low signal-to-noise ratio (Clackett et al., 2018). We found similar evidence of high inter-tree variability in tree-ring Hg concentrations, particularly at the mining-impacted site of Boca Colorado (p < 0.00001; Supplementary Table S1). At Boca Colorado and Laberinto, tree-ring Hg concentrations from some individual trees were elevated by as much as 10 ng g−1 relative to neighboring trees, mirroring patterns of intra-site variance found in leaves from the same trees (Gerson et al., 2022). In contrast, the other sites had 15%–60% lower inter-tree variability. This observation of increased tree-ring Hg variability at sites with high GEM in the Peruvian Amazon is consistent with previous studies from gymnosperms and angiosperms that show higher tree-ring Hg variability during periods of enhanced industrial GEM emissions (Abreu et al., 2008; Navrátil et al., 2018; Scanlon et al., 2020; Clackett et al., 2021; McLagan et al., 2022; Nováková et al., 2022). It is also possible that inter-tree variability was driven by variability in distance to amalgamation source. Given the high inter-tree variability of Hg concentrations in tree rings, it thus important that individual tree replicates are used when employing F. insipida tree rings as biomonitors.
Taken together, the observations of i) higher tree-ring Hg concentrations at sites closer to ASGM emissions sources of GEM, ii) significant positive correlation between atmospheric GEM concentrations and tree-ring Hg concentrations, and iii) significant positive correlation between tree-ring Hg concentrations and leaf Hg concentrations are consistent with stomatal uptake of GEM and subsequent incorporation of Hg into bolewood (Ericksen et al., 2003; Fay and Gustin, 2007; Arnold et al., 2018; Liu et al., 2021; Gustin et al., 2022). These results show that Hg in tree rings can be used a proxy specific to GEM, independent of other forms of atmospheric Hg, such as dry-deposited particulate Hg, though Hg isotopes are important for inferring the source of the Hg. Furthermore, the strong linear relationship between bolewood Hg concentrations and GEM suggests GEM is indeed the major driver of bolewood Hg variation and that F. inspida tree rings could be calibrated to produce quantitative estimates of GEM concentrations to evaluate spatial gradients in ASGM Hg emissions. This is one of only a few studies to report a quantitative and predictive relationship between tree-ring Hg and GEM concentrations (Arnold et al., 2018; Navrátil et al., 2018) and shows that F. inspida is a useful biomonitor for determining spatial patterns of GEM in the neotropics.
Most published tree-ring Hg studies focus on temperate and subarctic regions of the Northern Hemisphere (Gačnik and Gustin, 2023). This study is the first to evaluate the potential of neotropical hardwood as a GEM biomonitor. The use of Hg in ASGM occurs in over 70 countries globally, with the majority of Hg emissions occurring within the Amazon, followed by tropical areas in southeast Asia and sub-Saharan Africa (UNEP, 2018). Currently, GEM from ASGM is monitored through passive air samplers (Gerson et al., 2022; McLagan et al., 2018), which capture GEM concentrations over a one- or two-month deployment period and thus do not aggregate GEM concentrations across longer time scales. Trees therefore provide a much more widely distributed – and cheaper – network of biomonitoring, archiving a record of Hg in bolewood across greater periods of times (decades). We demonstrate here that concentrations of Hg in tree rings correlated strongly with atmospheric GEM concentrations and leaf total Hg concentrations, especially in the dry season when there is more active ASGM activity. Thus, F. insipida is a promising biomonitor to evaluate the spatial, and potentially temporal, footprint of GEM emissions from ASGM in the neotropics.
The findings of this study may have important implications for global Hg regulation efforts, particularly under the UNEP Minamata Convention, which aims to reduce Hg emissions and mitigate health and environmental risks. Tree-ring analysis can provide valuable insights into the historical and ongoing impacts of Hg pollution, making it a useful tool for evaluating the effectiveness of Hg reduction policies, such as the adoption of Hg-free technologies and improved regulatory enforcement. Additionally, integrating tree-ring biomonitoring into broader environmental monitoring programs could improve the establishment of regional baselines of Hg contamination. By leveraging tree-ring data alongside other environmental indicators, policymakers can gain a more comprehensive understanding of the cumulative impacts of Hg emissions and refine intervention strategies accordingly. Including tree-ring analysis in policy evaluations also enables the monitoring of both localized and transboundary Hg pollution, which is crucial for countries like Peru, where ASGM activities can have far-reaching environmental impacts.
The original contributions presented in the study are included in the article’s Supplementary Material.
JG: Conceptualization, Formal Analysis, Methodology, Visualization, Writing–original draft. IL: Formal Analysis, Methodology, Resources, Writing–review and editing. TL: Formal Analysis, Writing–review and editing. BB: Methodology, Resources, Writing - review and editing. NS: Methodology, Formal analysis, Writing - review and editing. LF: Writing–review and editing. CV: Writing–review and editing. TP: Formal Analysis, Methodology, Resources, Writing–review and editing.
The author(s) declare that financial support was received for the research, authorship, and/or publication of this article. Funding was provided to JG by Duke Global Health Institute Dissertation Fieldwork Grant, Duke Global Health Institute Doctoral Scholar Program, Duke University Bass Connections, Duke University Center for Latin American and Caribbean Studies Tinker Research Travel Grant Award, Duke University Center for International and Global Studies Research and Training Grant, Duke University Dissertation Research International Travel Award, Forest History Society, Geological Society of America Grants in Aid of Research, Lewis and Clark Fund for Exploration and Field Research, and National Science Foundation Graduate Research Fellowship. IL acknowledges funding from the Natural Science and Engineering Research Council of Canada (NSERC) Discovery Grant Program and the Canadian Foundation for Innovation (CFI) John R. Evans Leader’s Fund.
We thank Ramiro Cordova Salas, Francisco Phuno Soncco, Bryan Huamantupa Rivera, Cecilio Huamantupa, Arianna Basto, Kelsey Lansdale, Melissa Marchese, Arabella Chen, Annie Lee, Fernanda Machicao, Centro de Innovación Científica Amazónica (CINCIA), Conservación Amazónico (ACCA), and Los Amigos Conservation Concession for field assistance. We thank Emily Bernhardt, and Miles Silman for assistance with study design. We thank the reviewers for their feedback and comments.
The authors declare that the research was conducted in the absence of any commercial or financial relationships that could be construed as a potential conflict of interest.
The author(s) declare that no Generative AI was used in the creation of this manuscript.
All claims expressed in this article are solely those of the authors and do not necessarily represent those of their affiliated organizations, or those of the publisher, the editors and the reviewers. Any product that may be evaluated in this article, or claim that may be made by its manufacturer, is not guaranteed or endorsed by the publisher.
The Supplementary Material for this article can be found online at: https://www.frontiersin.org/articles/10.3389/fenvs.2025.1531800/full#supplementary-material
Abreu, S. N., Soares, A. M. V. M., Nogueira, A. J. A., and Morgado, F. (2008). Tree rings, Populus nigra L., as mercury data logger in aquatic environments: case study of an historically contaminated environment. Bull. Environ. Contam. Toxicol. 80, 294–299. doi:10.1007/s00128-008-9366-0
Arnold, J., Gustin, M. S., and Weisberg, P. J. (2018). Evidence for nonstomatal uptake of Hg by aspen and translocation of Hg from foliage to tree rings in Austrian pine. Environ. Sci. Technol. 52, 1174–1182. doi:10.1021/acs.est.7b04468
Asner, G. P., Llactayo, W., Tupayachi, R., and Luna, E. R. (2013). Elevated rates of gold mining in the Amazon revealed through high-resolution monitoring. Proc. Natl. Acad. Sci. U. S. A. 110, 18454–18459. doi:10.1073/pnas.1318271110
Brienen, R. J. W., and Zuidema, P. A. (2006). The use of tree rings in tropical forest management: projecting timber yields of four Bolivian tree species. For. Ecol. Manag. 226, 256–267. doi:10.1016/j.foreco.2006.01.038
Bruno, D. E., De Simone, F., Cinnirella, S., Amore, F. D., Pirrone, N., and Hedgecock, I. M. (2023). Reducing mercury emission uncertainty from artisanal and small-scale gold mining using bootstrap confidence intervals: an assessment of emission reduction scenarios. Atmosphere 14, 62. doi:10.3390/atmos14010062
Cheng, Y., Watari, T., Seccatore, J., Nakajima, K., Nansai, K., and Takaoka, M. (2023). A review of gold production, mercury consumption, and emission in artisanal and small-scale gold mining (ASGM). Resour. Policy 81, 103370. doi:10.1016/j.resourpol.2023.103370
Clackett, S. P., Porter, T. J., and Lehnherr, I. (2018). 400-year record of atmospheric mercury from tree-rings in northwestern Canada. Environ. Sci. Technol. 52, 9625–9633. doi:10.1021/acs.est.8b01824
Clackett, S. P., Porter, T. J., and Lehnherr, I. (2021). The tree-ring mercury record of Klondike gold mining at Bear Creek, Central Yukon. Environ. Pollut. 268, 115777. doi:10.1016/j.envpol.2020.115777
Diringer, S., Berky, A., Pan, W. K. Y., Hsu-kim, H., Araújo-Flores, J. M., et al. (2015). River transport of mercury from artisanal and small-scale gold mining and risks for dietary mercury exposure in Madre de Dios, Peru. Environ. Sci. Process. and Impacts 17, 478–487. doi:10.1039/c4em00567h
Eagles-Smith, C. A., Silbergeld, E. K., Basu, N., Bustamante, P., Diaz-Barriga, F., Hopkins, W. A., et al. (2018). Modulators of mercury risk to wildlife and humans in the context of rapid global change. Ambio 47, 170–197. doi:10.1007/s13280-017-1011-x
Eccles, K. M., Majeed, H., Porter, T. J., and Lehnherr, I. (2020). A continental and marine-influenced tree-ring mercury record in the old crow flats, yukon, Canada. ACS Earth Space Chem. 4, 1281–1290. doi:10.1021/acsearthspacechem.0c00081
Ericksen, J. A., Gustin, M. S., Schorran, D. E., Johnson, D. W., Lindberg, S. E., and Coleman, J. S. (2003). Accumulation of atmospheric mercury in forest foliage. Atmos. Environ. 37, 1613–1622. doi:10.1016/s1352-2310(03)00008-6
Espejo, J. C., Messinger, M., Rom, F., Ascorra, C., Fernandez, L. E., and Silman, M. (2018). Deforestation and forest degradation due to gold mining in the Peruvian Amazon: a 34-year perspective. Remote Sens. 10, 1–17. doi:10.3390/rs10121903
Evers, D. C., Jackson, A. K., Tear, T. H., and Osborne, C. E. (2012). HIdden risk: Mercury in Terrestrial Ecosystems of the Northeast. Maine: Gorham.
Fay, L., and Gustin, M. (2007). Assessing the influence of different atmospheric and soil mercury concentrations on foliar mercury concentrations in a controlled environment. Water, Air, Soil Pollut. 181, 373–384. doi:10.1007/s11270-006-9308-6
Fleck, J. A., Grigal, D. F., and Nater, E. A. (1999). Mercury uptake by trees: an observational experiment. Water. Air, Soil Pollut. 115, 513–523. doi:10.1023/a:1005194608598
Fraser, B. (2009). Peruvian gold rush threatens health and the environment. Environ. Sci. Technol. Perspective 43, 7162–7164. doi:10.1021/es902347z
Gačnik, J., and Gustin, M. S. (2023). Tree rings as historical archives of atmospheric mercury: a critical review. Sci. Total Environ. 898, 165562. doi:10.1016/j.scitotenv.2023.165562
Gerson, J. R., Szponar, N., Zambrano, A. A., Bergquist, B., Broadbent, E., Driscoll, C. T., et al. (2022). Amazon forests capture high levels of atmospheric mercury pollution from artisanal gold mining. Nat. Commun. 13, 559–610. doi:10.1038/s41467-022-27997-3
Gerson, J. R., Topp, S. N., Vega, C. M., Gardner, J. M., Yang, X., Fernandez, L. E., et al. (2020). Artificial lake expansion amplifies mercury pollution from gold mining. Sci. Adv. 6, eabd4953. doi:10.1126/sciadv.abd4953
Ghotra, A., Lehnherr, I., Porter, T. J., and Pisaric, M. F. J. (2020). Tree-ring inferred atmospheric mercury concentrations in the Mackenzie Delta (NWT, Canada) peaked in the 1970s but are increasing once more. ACS Earth Space Chem. 4, 457–466. doi:10.1021/acsearthspacechem.0c00003
Gustin, M. S., Dunham-Cheatham, S. M., Harper, J. F., Choi, W. G., Blum, J. D., and Johnson, M. W. (2022). Investigation of the biochemical controls on mercury uptake and mobility in trees. Sci. Total Environ. 851, 158101. doi:10.1016/j.scitotenv.2022.158101
Ha, E., Basu, N., Bose-O’Reilly, S., Dorea, J. G., McSorley, E., Sakamoto, M., et al. (2017). Current progress on understanding the impact of mercury on human health. Environ. Res. 152, 419–433. doi:10.1016/j.envres.2016.06.042
Keane, S., Bernaudat, L., Davis, K. J., Stylo, M., Mutemeri, N., Singo, P., et al. (2023). Mercury and artisanal and small-scale gold mining: review of global use estimates and considerations for promoting mercury-free alternatives. Ambio 52, 833–852. doi:10.1007/s13280-023-01843-2
Liu, Y., Lin, C. J., Yuan, W., Lu, Z., and Feng, X. (2021). Translocation and distribution of mercury in biomasses from subtropical forest ecosystems: evidence from stable mercury isotopes. Acta Geochim. 40, 42–50. doi:10.1007/s11631-020-00441-3
Maillard, F., Girardclos, O., Assad, M., Zappelini, C., Pérez Mena, J. M., Yung, L., et al. (2016). Dendrochemical assessment of mercury releases from a pond and dredged-sediment landfill impacted by a chlor-alkali plant. Environ. Res. 148, 122–126. doi:10.1016/j.envres.2016.03.034
McLagan, D. S., Biester, H., Navrátil, T., Kraemer, S. M., and Schwab, L. (2022). Internal tree cycling and atmospheric archiving of mercury: examination with concentration and stable isotope analyses. Biogeosciences 19, 4415–4429. Copernicus Publications. doi:10.5194/bg-19-4415-2022
McLagan, D. S., Mitchell, C. P. J., Huang, H., Lei, Y. D., Cole, A. S., Steffen, A., et al. (2016). A high-precision passive air sampler for gaseous mercury. Environ. Sci. Technol. Lett. 3, 24–29. doi:10.1021/acs.estlett.5b00319
McLagan, D. S., Mitchell, C. P. J., Steffen, A., Hung, H., Shin, C., Stupple, G. W., et al. (2018). Global evaluation and calibration of a passive air sampler for gaseous mercury. Atmos. Chem. Phys. 18, 5905–5919. doi:10.5194/acp-18-5905-2018
Navrátil, T., Nováková, T., Shanley, J. B., Rohovec, J., Matoušková, Š., Vaňková, M., et al. (2018). Larch tree rings as a tool for reconstructing 20th century central European atmospheric mercury trends. Environ. Sci. Technol. 52, 11060–11068. doi:10.1021/acs.est.8b02117
Navrátil, T., Šimeček, M., Shanley, J. B., Rohovec, J., Hojdová, M., and Houška, J. (2017). The history of mercury pollution near the Spolana chlor-alkali plant (Neratovice, Czech Republic) as recorded by Scots pine tree rings and other bioindicators. Sci. Total Environ. 586, 1182–1192. doi:10.1016/j.scitotenv.2017.02.112
Nováková, T., Navrátil, T., Demers, J. D., Roll, M., and Rohovec, J. (2021). Contrasting tree ring Hg records in two conifer species: multi-site evidence of species-specific radial translocation effects in Scots pine versus European larch. Sci. Total Environ. 762, 144022. doi:10.1016/j.scitotenv.2020.144022
Nováková, T., Navrátil, T., Schütze, M., Rohovec, J., Matoušková, Š., Hošek, M., et al. (2022). Reconstructing atmospheric Hg levels near the oldest chemical factory in central Europe using a tree ring archive. Environ. Pollut. 304, 119215. doi:10.1016/j.envpol.2022.119215
Odabasi, M., Tolunay, D., Kara, M., Ozgunerge Falay, E., Tuna, G., Altiok, H., et al. (2016). Investigation of spatial and historical variations of air pollution around an industrial region using trace and macro elements in tree components. Sci. Total Environ. 550, 1010–1021. doi:10.1016/j.scitotenv.2016.01.197
Peckham, M. A., Gustin, M. S., and Weisberg, P. J. (2019). Assessment of the suitability of tree rings as archives of global and regional atmospheric mercury pollution. Environ. Sci. Technol. 53, 3663–3671. doi:10.1021/acs.est.8b06786
Scanlon, T. M., Riscassi, A. L., Demers, J. D., Camper, T. D., Lee, T. R., and Druckenbrod, D. L. (2020). Mercury accumulation in tree rings: observed trends in quantity and isotopic composition in Shenandoah National Park, Virginia. J. Geophys. Res. Biogeosciences 125, 1–18. doi:10.1029/2019jg005445
Schmidt, C. W. (2012). Quicksilver and gold: mercury pollution from artisanal and small-scale gold mining. Environ. Health Perspect. 120, A424–A429. doi:10.1289/ehp.120-a424
Schneider, L., Allen, K., Walker, M., Morgan, C., and Haberle, S. (2019). Using tree rings to track atmospheric mercury pollution in Australia: the legacy of mining in Tasmania. Environ. Sci. Technol. 53, 5697–5706. doi:10.1021/acs.est.8b06712
Schöngart, J., Gribel, R., Ferreira da Fonseca-Junior, S., and Haugaasen, T. (2015). Age and growth patterns of Brazil nut trees (Bertholletia excelsa Bonpl.) in Amazonia, Brazil. Biotropica 47, 550–558. doi:10.1111/btp.12243
Schöngart, J., Wittmann, F., Worbes, M., Piedade, M. T. F., Krambeck, H.-J., and Junk, W. J. (2007). Management criteria for Ficus insipida Willd. (Moraceae) in Amazonian white-water floodplain forests defined by tree-ring analysis. Ann. Sci. 64, 657–664. doi:10.1051/forest:2007044
Siwik, E. I. H., Campbell, L. M., and Mierle, G. (2010). Distribution and trends of mercury in deciduous tree cores. Environ. Pollut. 158, 2067–2073. doi:10.1016/j.envpol.2010.03.002
Sprovieri, F., Pirrone, N., Bencardino, M., D’Amore, F., Carbone, F., Cinnirella, S., et al. (2016). Atmospheric mercury concentrations observed at ground-based monitoring sites globally distributed in the framework of the GMOS network. Atmos. Chem. Phys. 16, 11915–11935. doi:10.5194/acp-16-11915-2016
Stamenkovic, J., and Gustin, M. S. (2009). Nonstomatal versus stomatal uptake of atmospheric mercury. Environ. Sci. Technol. 43, 1367–1372. doi:10.1021/es801583a
Swenson, J. J., Carter, C. E., Domec, J. C., and Delgado, C. I. (2011). Gold mining in the Peruvian Amazon: global prices, deforestation, and mercury imports. PLoS One 6, e18875. doi:10.1371/journal.pone.0018875
Telmer, K. H., and Veiga, M. M. (2009). “World emissions of mercury from artisanal and small scale gold mining,” in Mercury Fate and Transport in the Global Atmosphere. Editors R. Mason, and N. Pirrone (Berlin, Germany: Springer), 131–172.
Wang, X., Yuan, W., Lin, C. J., Wu, F., and Feng, X. (2021). Stable mercury isotopes stored in Masson Pinus tree rings as atmospheric mercury archives. J. Hazard. Mater. 415, 125678. doi:10.1016/j.jhazmat.2021.125678
Weis, J. S. (2009). Reproductive, developmental, and neurobehavioral effects of methylmercury in fishes. J. Environ. Sci. Health C Environ. Carcinog. Ecotoxicol. Rev. 27, 212–225. doi:10.1080/10590500903310088
Wright, G., Woodward, C., Peri, L., Weisberg, P. J., and Gustin, M. S. (2014). Application of tree rings [dendrochemistry] for detecting historical trends in air Hg concentrations across multiple scales. Biogeochemistry 120, 149–162. doi:10.1007/s10533-014-9987-9
Wohlgemuth, L., Osterwalder, S., Joseph, C., Kahmen, A., Hoch, G., Alewell, C., et al. (2020). A bottom-up quantification of foliar mercury uptake fluxes across Europe. Biogeosciences 17, 6441–6456. doi:10.5194/bg-17-6441-2020
Worbes, M. (1989). Growth rings, increment and age of trees in inundation forests, savannas and a mountain forest in the neotropics. IAWA J. 10, 109–122. doi:10.1163/22941932-90000479
Worbes, M. (1995). How to measure growth dynamics in tropical trees a review. IAWA J. 16, 337–351. doi:10.1163/22941932-90001424
Worbes, M. (2002). One hundred years of tree-ring research in the tropics - a brief history and an outlook to future challenges. Dendrochronologia 20, 217–231. doi:10.1078/1125-7865-00018
Yamakawa, A., Amouroux, D., Tessier, E., Bérail, S., Fettig, I., Barre, J. P. G., et al. (2021). Hg isotopic composition of one-year-old spruce shoots: application to long-term Hg atmospheric monitoring in Germany. Chemosphere 279, 130631. doi:10.1016/j.chemosphere.2021.130631
Yang, Y., Yanai, R. D., Montesdeoca, M., and Driscoll, C. T. (2017). Measuring mercury in wood: challenging but important 7319. Inter. J. Environ. Analyt. Chem. 97, 1–12. doi:10.1080/03067319.2017.1324852
Zhou, J., Obrist, D., Dastoor, A., Jiskra, M., and Ryjkov, A. (2021). Vegetation uptake of mercury and impacts on global cycling. Nat. Rev. Earth Environ. 2, 269–284. doi:10.1038/s43017-021-00146-y
Keywords: artisanal and small-scale gold mining, biomonitor, dendrochemistry, mercury, tree-rings
Citation: Gerson J, Lehnherr I, Luu T, Bergquist B, Szponar N, Fernandez LE, Vega C and Porter TJ (2025) Ficus insipida tree rings as biomonitors for gaseous elemental mercury in the artisanal gold mining-impacted Peruvian Amazon. Front. Environ. Sci. 13:1531800. doi: 10.3389/fenvs.2025.1531800
Received: 20 November 2024; Accepted: 11 February 2025;
Published: 08 April 2025.
Edited by:
Željka Fiket, Rudjer Boskovic Institute, CroatiaReviewed by:
Jean Remy Davee Guimaraes, Federal University of Rio de Janeiro, BrazilCopyright © 2025 Gerson, Lehnherr, Luu, Bergquist, Szponar, Fernandez, Vega and Porter. This is an open-access article distributed under the terms of the Creative Commons Attribution License (CC BY). The use, distribution or reproduction in other forums is permitted, provided the original author(s) and the copyright owner(s) are credited and that the original publication in this journal is cited, in accordance with accepted academic practice. No use, distribution or reproduction is permitted which does not comply with these terms.
*Correspondence: Jacqueline Gerson, amFjcXVlbGluZS5nZXJzb25AY29ybmVsbC5lZHU=
Disclaimer: All claims expressed in this article are solely those of the authors and do not necessarily represent those of their affiliated organizations, or those of the publisher, the editors and the reviewers. Any product that may be evaluated in this article or claim that may be made by its manufacturer is not guaranteed or endorsed by the publisher.
Research integrity at Frontiers
Learn more about the work of our research integrity team to safeguard the quality of each article we publish.