- 1Norwegian College of Fishery Science, UiT, The Arctic University of Norway, Tromsø, Norway
- 2GeoHydrodynamics and Environment Research (GHER), University of Liège, Liège, Belgium
- 3National Centre for Climate Research, Danish Meteorological Institute, Copenhagen, Denmark
- 4Geophysical Institute, University of Bergen, Bjerknes Centre for Climate Research, Bergen, Norway
- 5Institute of Marine Research, Flødevigen Research Station, His, Norway
- 6School of Biological Sciences and UWA Oceans Institute, University of Western Australia, Perth, WA, Australia
- 7U.S. Fish and Wildlife Service, Anchorage, AK, United States
- 8Jet Propulsion Laboratory, NASA/California Institute of Technology, Pasadena, CA, United States
- 9Geosciences Research Division, Scripps Institution of Oceanography, University of California - San Diego, La Jolla, CA, United States
- 10Department of Ecoscience, Aarhus University, Aarhus, Denmark
- 11Alaska Fisheries Science Center, NOAA, National Marine Fisheries Service, Juneau, AK, United States
The Arctic and Subarctic seas are predicted to become hotspots for marine heatwaves (MHWs). High-latitude marine ecosystems face unique consequences from accelerated warming and sea ice loss, challenging species adapted to cold conditions. We review the literature on MHW characteristics and ecological impacts in the Arctic and Subarctic seas, and contrast MHW characteristics between the Bering Sea and Barents Sea. We uncover the pervasive impacts of MHWs across widely different organism groups, including benthic foundation species, phytoplankton, zooplankton, fish, seabirds, and marine mammals. MHWs in the Arctic marginal seas are especially prevalent in areas experiencing sea ice retreat, such as seasonal sea ice zones, highlighting the complex interplay between MHWs and sea ice dynamics. Overall, few studies have documented the ecological impacts of MHWs on high-latitude ecosystems, with the notable exception of the impacts from the Bering Sea and Chukchi Sea MHWs in 2017–2019. Many Arctic species, with their cold and narrow thermal preferences, appear vulnerable to MHWs, as they might not have access to cold climate refugia, while boreal species appear to benefit from Arctic and Subarctic MHWs. Sessile foundation species, such as kelp and seagrasses, are especially at risk during MHWs, although in the Arctic evidence of MHWs impacts remains limited. Reproductive failure and mass mortality events have been documented for several species in the Pacific Arctic (e.g., seabirds, fish, crabs). MHWs have been observed to have ecosystem-wide repercussions in the northern Bering Sea and Chukchi Sea with shifts in plankton communities affecting the entire food web. The ecological responses to MHWs in the Arctic and Subarctic ecosystems are still not fully understood, highlighting a need for further research to assess the direct and indirect impacts on various taxa and to improve predictive models for better management and conservation strategies. MHWs can also have large consequences for ecosystem services and socio-ecological systems, for example, closures of economically valuable and culturally important fisheries, as seen in Alaska, degradation of traditional ice-hunting practices, and compromised wellbeing of coastal communities. Large and abrupt ecosystem changes following MHWs underscore the urgent need for adaptive management strategies in the face of ongoing climate change.
1 Introduction
The Arctic marine ecosystem is characterised by extremely cold temperatures, extreme seasonality, and the presence of permanent and seasonal sea ice. Consequently, the Arctic supports specialised species adapted to these extreme conditions (Gradinger, 2001; Blix, 2005; Castellani et al., 2022). Arctic marine life has evolved to thrive in environments with uniquely prolonged periods of darkness (and light), cold temperatures, salinity variation, and seasonal sea ice cover. However, the Arctic marine ecosystem is changing fast due to anthropogenic climate change. Arctic sea ice is currently at the lowest level since at least 1850, and late summer sea ice loss is unprecedented for at least 1,000 years (IPCC, 2023). Since the late 1970s, Arctic sea ice has decreased in both area and thickness, with a larger area of first-year ice (Stroeve et al., 2012). The Arctic Ocean is expected to become sea ice free in late summer by the end of the 21st century regardless of CO2 emissions scenarios (Notz and Community, 2020; Jahn et al., 2024). Sea ice is a critical habitat for many Arctic species and its disappearance will transform the Arctic ecosystem. Sea surface temperatures have increased in almost all regions of the Arctic and Subarctic (Figure 1A). Gradual warming of the Arctic Ocean has significantly transformed Arctic marine ecosystems (Mueter and Litzow, 2008; Huntington et al., 2020; Mueter et al., 2021; Husson et al., 2024).
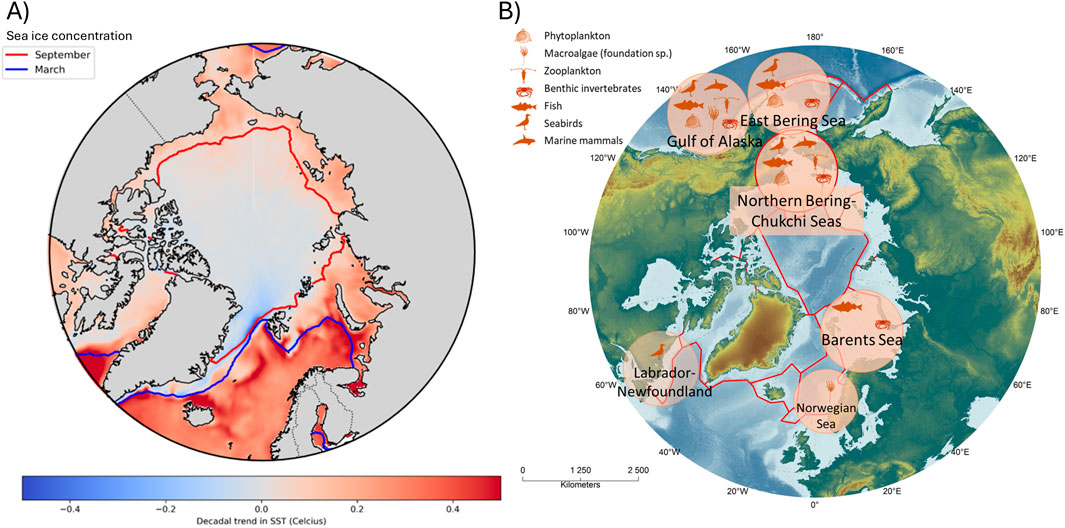
Figure 1. (A) Decadal trends in sea surface temperature (SST) calculated using Sen’s slope (Sen, 1968) for the Optimally Interpolated SST (OISST) dataset between 1st September 1981 to 31st December 2023. The blue and red line represent the minimum (September) and maximum (March) sea ice extent, respectively, using the climatological mean of the period 1981–2023 and a 15% sea ice concentration cut-off. Multiyear ice, is the area between the North Pole (90°N) and the red line; first-year ice, the area between the blue and red lines; and open water, the area below the blue line. (B) Circumpolar map depicting current knowledge on ecosystem responses to MHWs in the Arctic and Subarctic large marine ecosystems. Symbols represent different taxonomic groups and illustrate where their response to MHWs have been studied in peer-reviewed scientific literature (see references in Supplementary Table S1). The underlying Arctic large marine ecosystem (LME) map was obtained from PAME at https://pame.is/projects/ecosystem-approach/arctic-large-marine-ecosystems-lme-s.
Climate-driven changes are observed throughout the entire food web, from lower trophic levels to top predators. For example, sea ice loss causes an extension of the phytoplankton growing season and alters the timing of the blooms (Kahru et al., 2011; Ardyna et al., 2014; Oziel et al., 2017), while zooplankton populations shift from high to low-lipid content species (Møller and Nielsen, 2020; Kimmel et al., 2023), which can have ramifications for the entire marine food web. Upper trophic levels are also shifting their migration and distribution patterns (Kuletz et al., 2024a), as boreal species become increasingly dominant and compete with Arctic species, a process known as borealisation (Fossheim et al., 2015; Mueter et al., 2021; Stafford et al., 2022; von Biela et al., 2023; Husson et al., 2024).
In addition to being a hotspot of decadal warming, the Arctic Ocean is also predicted to be a future marine heatwave (MHW) hotspot (IPCC, 2023). MHWs are periods where ocean temperatures substantially exceed historical norms in a given region, with durations ranging from several days to in some cases, years (Hobday et al., 2016). These events have varying spatial scales, spanning from just a few to many thousands of km (Sen Gupta et al., 2020; Oliver et al., 2021). Whilst some studies define MHWs by biological impacts, such as the threshold for coral bleaching (Pearce and Feng, 2013), they are most commonly defined when anomalies in sea surface temperature (SST) surpass their local 90th percentile threshold for at least five consecutive days or longer (Hobday et al., 2016). The category of a MHW is then assigned based on how much temperatures exceed the local climatology, ranging from moderate to extreme in intensity (Hobday et al., 2018).
MHWs are complex and highly multifaceted events, making it challenging to identify the underlying drivers of their onset, duration, and dissipation (Frölicher and Laufkötter, 2018; Holbrook et al., 2020). Localised factors such as temperature fluxes between the atmosphere and the ocean, or horizontal heat transport such as from Ekman advection, contribute to the onset and duration of MHWs (Holbrook et al., 2020). Over larger scales, MHWs are attributed to a combination of hydrodynamic and atmospheric forcings, as well as global-scale teleconnections (Holbrook et al., 2020). El Niño Southern Oscillation (ENSO), and the Atlantic Multidecadal Oscillation (AMO), have been shown to contribute towards some of the largest MHWs (Pearce and Feng, 2013; Holbrook et al., 2020; Ren and Liu, 2021). Some of the most notable MHWs, such as the northeastern Pacific “Blob” (the 2014–2016 MHW) have been linked to ENSO (Bond et al., 2015; Di Lorenzo and Mantua, 2016), while shifts in atmospheric jet streams contributed to MHWs in the Atlantic (Chen et al., 2014; Gawarkiewicz et al., 2019). ENSO has been one of the most prominent causes of MHWs globally, associated with warming events in the Pacific, the Atlantic, and the Southern Ocean. In addition to these physical drivers, human-induced global warming has contributed directly to many of the most intense MHWs (Laufkötter et al., 2020).
On a global scale, the frequency and duration of MHWs has increased, with their occurrence nearly doubling over the last century (Frölicher and Laufkötter, 2018; Oliver et al., 2018). Earth system model simulations have indicated that nearly 90% of MHWs have been attributed to anthropogenic warming, which is likely to increase with greater greenhouse gas emissions (Frölicher et al., 2018; Laufkötter et al., 2020). By the end of the century MHWs are expected to become more severe in their frequency, length, and intensity (Frölicher et al., 2018; Plecha and Soares, 2020). The number of MHWs are projected to increase significantly, with a global rise at the end of the century of 2–9 times under the low CO2 emissions scenarios, up to 3–15 times under the very high CO2 emissions scenario, with the largest changes projected for the Arctic and tropical oceans (IPCC, 2023). These prolonged periods of anomalously high SST will likely have large and lasting consequences for marine ecosystems (Arimitsu et al., 2021; Suryan et al., 2021).
While long-term ocean warming gradually reshapes the distribution of marine life and ecosystem structures, functions, and associated services (Vergés et al., 2014; Pecl et al., 2017), a single MHW can have large and long-lasting consequences on the whole ecosystem (Smale et al., 2019; Wernberg, 2021; Smith K. E. et al., 2023). MHWs can lead to the displacement of marine life at a much larger geographic scale compared with gradual, long-term, warming (Jacox et al., 2020). MHWs in tropical and temperate regions have been linked with depletion of kelp forests (Wernberg et al., 2019; Smith K. E. et al., 2023), extensive loss of seagrass (Strydom et al., 2020), bleaching of coral reefs (Hughes et al., 2017), harmful algal blooms, and mass mortality of fish, seabirds, and marine mammals (Roberts et al., 2019; Gabriele et al., 2022; Jones et al., 2023).
The ecological impacts of MHWs on high-latitude ecosystems are just beginning to emerge. Here, we review the current knowledge of MHW characteristics in the Arctic and Subarctic seas, by discussing the unique features of MHWs in these regions, notably their interactions with sea ice. We then calculate and compare the temporal trends of MHWs metrics in the Bering Sea and Barents Sea, two major Arctic gateways and transition regions between Subarctic and Arctic conditions. Next, we reviewed documented impacts of MHWs on Arctic and Subarctic marine ecosystems across trophic levels and functional groups (Figure 1B, a summary table of which and how taxonomic groups have been documented to respond to MHWs can be found in Supplementary Table S1). The ecological impacts of MHWs in Arctic and Subarctic marine ecosystems are being increasingly documented. Throughout the review, we especially draw on studies linked to the Bering Sea and Chukchi Sea MHWs in 2017–2019 (Box 1), and to a lesser extent to the 2014–2016 MHW event (the “Blob”) in the northeast Pacific as well as some global model studies. We also discuss the social and economic implications of MHWs, notably their impacts on commercial and subsistence harvest opportunities and disruption of traditional livelihoods in the Arctic. Finally, we discuss how more adaptive management options could help the social-ecological system become more resilient to MHWs, as well as highlights current knowledge gaps and research needs.
Box 1 Case study: ecosystem-wide impacts of the 2017–2019 MHW in the northern Bering and Chukchi seas
The northern Bering Sea and Chukchi Sea experienced a series of MHW events during 2017–2019 (Baker et al., 2020; Kuletz et al., 2024b (Supplementary Appendix 1A) (Figure 3F). The winters of 2017/2018 and 2018/2019 had extremely low sea ice extent, and the Bering Sea cold pool, the area where near bottom water is less than 2°C on the Bering Sea shelf, disappeared in the summers of 2018 and 2019. This extreme event triggered complex responses throughout the marine food web in the northern Bering Sea and Chukchi Sea, impacting the entire ecosystem from primary production, zooplankton, fish populations, seabirds, and marine mammals (Figure 4). Reduced ice cover and warmer seas altered thermal, light, and stratification conditions, influencing the timing and extent of phytoplankton blooms. In 2018, while the phytoplankton bloom was delayed in the southern Bering Sea, and significantly lower than usual in the northern Bering Sea, the ice-associated bloom occurred early and was extensive (Duffy-Anderson et al., 2019). Cases of harmful algal blooms arose which could impact UTLs (Walsh et al., 2018), such as occurred in the northern Bering and Chukchi seas, where seabird die-offs were associated with toxic algae blooms (Van Hemert et al., 2020). In the Chukchi Sea, changes in zooplankton and benthic populations were observed, with copepods (Calanus glacialis/marshallae) and epibenthos having much lower abundance compared to previous years (Huntington et al., 2020).
In the Bering Sea, during the MHW a shift in the zooplankton community was observed, with an increase in small, low-lipid copepods and a decrease in large, high-lipid copepods (Duffy-Anderson et al., 2019). Young fish also seem to have responded to the MHW, with a large increase in the abundances in 2017 of juvenile pink salmon, juvenile walleye pollock, and age-0 polar cod, although with overall low energy content for the latter (Huntington et al., 2020; Copeman et al., 2022; Levine et al., 2023). The effects of the warm period extended to higher trophic levels. With the lack of the cold pool in the Bering Sea in 2018, adult walleye pollock, Pacific cod, and northern rock sole biomass increased in the northeastern Bering Sea, likely due to northward expansion (Stevenson and Lauth, 2019), whereas the snow crab population collapsed as a result of reduced habitat and increased competition, which led to mass mortality events, likely due to starvation (Szuwalski et al., 2023). Seabird populations also experienced mass mortality events, such as puffins in 2017 (Jones et al., 2019), and murres, fulmars (Fulmarus glacialis), and shearwaters in the Bering Sea in 2018 (Duffy-Anderson et al., 2019; Jones et al., 2023). Changes in seabird distribution were observed with notably an increase in short-tailed shearwater densities in the Chukchi Sea (Kuletz et al., 2024b), and breeding success was low for seabirds in the Bering Sea (Romano et al., 2020; Will et al., 2020a). The ecosystem changes also affected marine mammals. Bowhead whales were sighted earlier than usual in northern Alaska, and their wintering patterns shifted to the Chukchi Sea (Huntington et al., 2020). Spotted seal pups were found in poorer condition, and a significant increase in seal carcasses, particularly in the northern Bering and southern Chukchi seas, was observed (Huntington et al., 2020). The MHW in the northern Bering and Chukchi seas has had consequences on all trophic levels, which ultimately can impact ecosystem structure and functioning. The MHWs can have direct and indirect effects on the populations, yet which effect dominates remains unknown and will vary across trophic levels, with food web mediated effects likely affecting UTLs. Huntington et al. (2020) suggests that observed changes in the composition and abundance of zooplankton towards lower-lipid species, along with an increased competition due to a higher biomass of predator fish, might have caused the observed mass mortality of seabirds and seals. While the northern Bering and Chukchi seas have shown signs of transition towards a Subarctic ecosystem in response to ocean warming, the ecosystem response to the 2017–2019 MHW events may be preludes to future changes.
2 Specificity of marine heatwaves in the Arctic and Subarctic
The intensity, duration, and frequency of MHWs have increased in the Arctic Ocean and its marginal seas (Carvalho et al., 2021; Golubeva et al., 2021; Huang et al., 2021). Between 2007 and 2021, 11 MHWs occurred with peak anomalies up to 4°C (Barkhordarian et al., 2024). These MHWs coincide with significant declines in Arctic sea ice (relative Arctic sea ice extent anomalies), notably in 2007, 2012, and 2020, with the 2020 event being the most severe in terms of intensity and duration (Barkhordarian et al., 2024). Between 1982 and 2020, the frequency and duration of MHWs in the Barents Sea significantly increased, with more than half of the MHW days having occurred between 2011 and 2020, and the most intense event in 2016 (Mohamed et al., 2022b). Similarly, in the Bering Sea, Carvalho et al. (2021) showed that in the period 1990–2019 the number of MHWs days increased, with the last decade (2010–2019) having the largest number of MHWs and MHW days. The increased trends of MHWs in the Arctic are closely associated with the long-term decrease in sea ice concentration and increase in surface air temperature (Huang et al., 2021; Barkhordarian et al., 2024).
2.1 Drivers of MHWs in the Arctic
In the Arctic Ocean, MHW dynamics are unique due to the complex links between the ocean, sea ice, and atmosphere (Hu et al., 2020; Barkhordarian et al., 2024). To date, relatively few studies have analysed the drivers of MHW onset and decay in the Arctic. The Arctic Ocean is relatively isolated from the rest of the global ocean, except for inflow shelfs such as the Barents and Bering Seas (Carmack et al., 2015). Consequently, the primary driver of MHWs in the Arctic is heat exchange between the atmosphere and the ocean, mainly through radiative fluxes (Hu et al., 2020; Richaud et al., 2024). The advection of oceanic heat plays a more important role in the inflow regions (Richaud et al., 2024). In general, Arctic MHWs dissipate through bottom heat fluxes, in spring and summer, and through surface fluxes in autumn, but there is spatial heterogeneity in the driving heat fluxes (Richaud et al., 2024). As such, common drivers of Arctic MHWs are similar to more temperate regions.
A unique feature of the Arctic is the presence of sea ice, which influences the occurrence and intensity of surface MHWs in complex ways. By reflecting large parts of incoming solar radiation, and through heat uptake and release during melting and freezing, the presence of sea ice keeps surface ocean temperatures close to the freezing point and temperature variability low (Carton et al., 2015). MHWs therefore mainly occur in open water or partially ice-covered waters (Hu et al., 2020; Huang et al., 2021), and the frequency and intensity of MHWs are inversely correlated to the sea ice cover (Carvalho et al., 2021; Golubeva et al., 2021; Mohamed et al., 2022b; Barkhordarian et al., 2024). Sea ice is thought to influence MHWs in two ways. First, sea ice melt in spring exposes the surface ocean to more solar heating, which can create or enhance MHWs (Barkhordarian et al., 2024; Richaud et al., 2024). Second, released freshwater from sea ice melt shoals the surface mixed layer, which can accelerate the heating by surface fluxes. Through this process, the presence of sea ice in seasonally ice-covered waters can potentially amplify the intensity and duration of MHWs (Hu et al., 2020; Richaud et al., 2024).
Recently, Zhang et al. (2024) investigated the main factors influencing Arctic MHWs from 1982 to 2020 in different ice cover regions, including multiyear ice, seasonal or first-year ice, and open water (Figure 1A). They found that the MHWs in the MYI region are mainly influenced by freshwater dilution processes such as sea ice concentration, precipitation and salinity of the mixed layer. In the FYI region, MHWs were influenced by surface air temperature and total heat flux mainly through thermodynamic processes, and the 500-hPa geopotential height also influences MHWs mainly through large-scale atmospheric circulation. MHWs in the OPW region were associated with sea ice, 850-hPa geopotential height, and meridional wind components, suggesting that MHWs in this region are correlated with atmospheric processes and wind fields. Generally, MHWs in shallow water regions (e.g., Barents Sea and North Sea) are coupled with the atmosphere (Mohamed et al., 2023; 2022b). However, MHWs occurred more frequently in the Barents Sea than in the North Sea. This could be due to Arctic amplification (Screen and Simmonds, 2010).
Because of the strong control of sea ice on surface temperatures, the recent decline in Arctic sea ice cover strongly contributed to the increased occurrence and intensity of Arctic MHWs (Mohamed et al., 2022b; Barkhordarian et al., 2024). First, sea ice loss plays a key role in Arctic amplification (Screen and Simmonds, 2010), leading to more extreme warming of the Arctic Ocean compared to the global oceans (Shu et al., 2022). Second, sea ice loss exposes regions with a low temperature variability to strong temperature increase, which can facilitate the occurrence of MHWs (Frölicher et al., 2018; Huang et al., 2021). Changes in the timing of Arctic MHWs are also influenced by changes in sea ice phenology. Huang et al. (2021) found a strong extension of MHW conditions during autumn, which is caused by the stronger trend in later sea ice advances compared to the trend in earlier sea ice retreats. In addition, the more severe Arctic MHWs occurr predominantly in regions with first-year ice, driven by ocean stratification (Zhang et al., 2024). As the extent of first-year ice increases in the Arctic, MHWs are expected to become more frequent and intense (Hu et al., 2020).
Defining MHWs from satellite observations of SST poses challenges in polar environments due to interannual variability in sea ice cover, limiting the number of open-water days from year to year. In a circumpolar study of MHWs in the Arctic, Huang et al. (2021) used the under-ice SSTs in areas of seasonal ice cover, allowing for long-term assessments of MHWs in historically ice-covered regions. Sea ice acts as a physical barrier to the atmosphere, thereby limiting atmospheric forcings on the ocean, and as such, both statistical (Huang et al., 2021) and mechanistic (Banzon et al., 2020) approaches can be used to estimate under-ice SST. Furthermore, because of the low temperature variability, defining MHWs in sea ice covered regions can be challenging, because the MHW threshold is very close to the climatological average. For example, regions permanently ice-covered in winter experience MHW conditions due to long-term upper-ocean freshening, which raises the freezing point of water (Richaud et al., 2024).
2.2 Characteristics of MHWs in the Subarctic marginal seas: example of the Bering and Barents seas
In this section, we compare the trends of SST anomalies (SSTA) and MHW characteristics in the two main pathways to the Arctic Ocean (i.e., the Barents Sea and the Bering Sea) by reviewing and extending the results of Carvalho et al. (2021) and Mohamed et al. (2022b). Following Hobday et al. (2016), daily SST data from the NOAA Optimum Interpolation SST (OISST) V2.1 product (Reynolds et al., 2007) were used to detect MHW events in these two regions over the period 1982–2022 (41 years). MHWs are “prolonged periods of anomalously warm water in which the SST exceeds the 90th percentile of the 30-year local mean for five consecutive days or longer” (Hobday et al., 2016). Here, we consider all the criteria of Hobday et al. (2016), including the SST baseline climatology, which should be based on at least 30 years, and if two consecutive MHW events occur with gaps of 2 days or less, they are considered as a single event. The climatological mean and the 90th percentile threshold are thus calculated based on the entire period (1982–2022). We compare trends in SSTA and MHWs metrics frequency (MHW events/decade) and total duration (number of days). The SSTA is calculated by subtracting the corresponding monthly climatology value from the observed SST value for each calendar month in the dataset.
In the Barents Sea, there was high spatial variability in SSTA with the highest SSTA trends in the regions affected by the accumulation of warm Atlantic water (Skagseth et al., 2020). Specifically, in the southeastern part of the Barents Sea and the Storfjorden Trough, SSTA trends were greatest, while the lowest SSTA trend was observed in the northern part of the Barents Sea and the Storbanken region (Figure 2A). Non-significant SSTA trends were found over the north and east of Svalbard, south of Franz Joseph Land, and on Spitsbergen Bank (Figure 2A), which were mainly influenced by the sea ice in these regions. For the Bering Sea, significant (p < 0.05) SSTA trends were observed over the entire region (Figure 2B), with a few exceptions that showed non-significant trends.
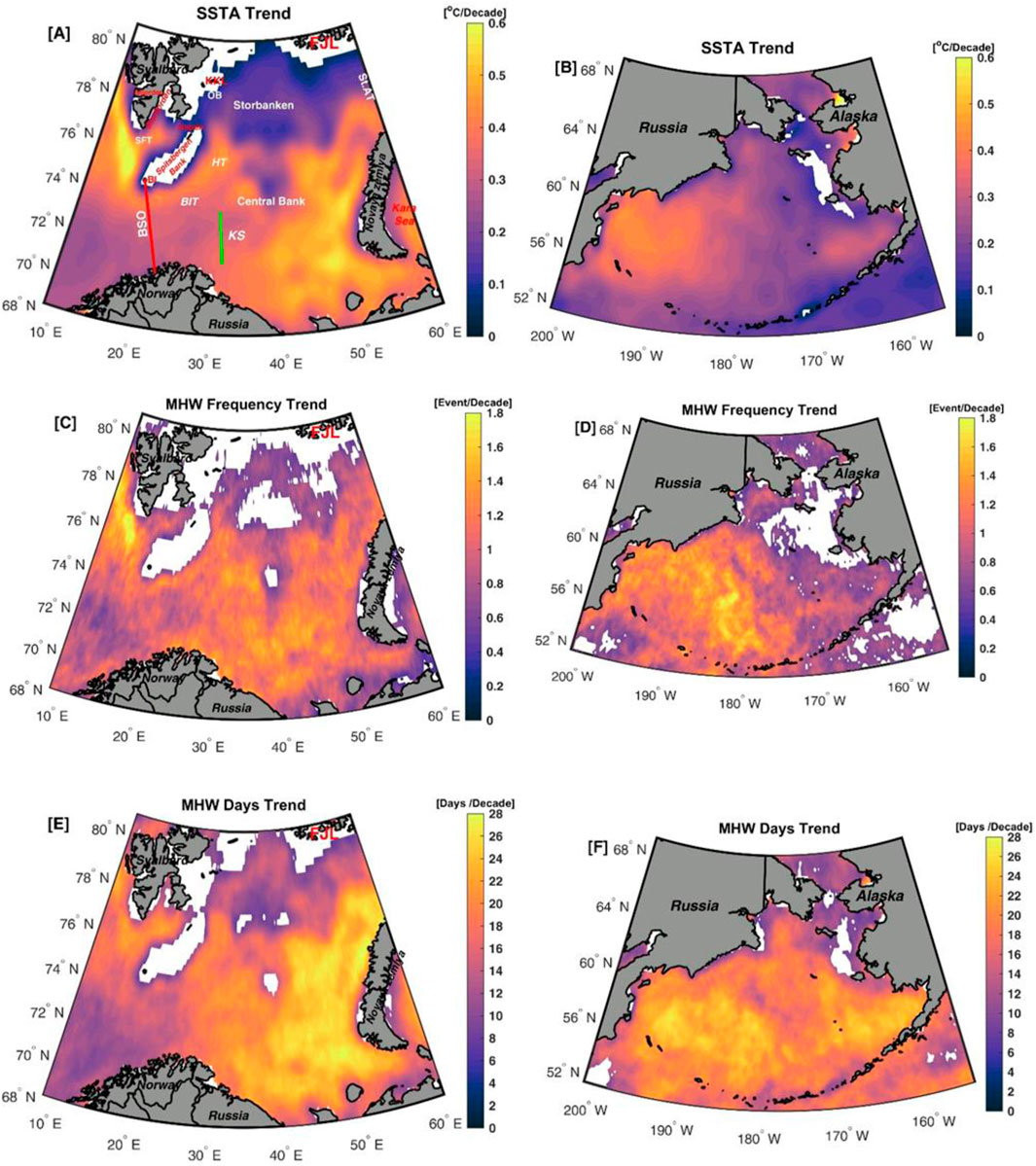
Figure 2. Trend maps for (A, B) sea surface temperature anomalies (°C/decade), (C, D) MHW frequency (events/decade), (E, F) MHW days (days/decade) between 1982 and 2022 for the two pathways to the Arctic Ocean - the Barents Sea (left column) and the Bering Sea (right column). The white regions indicate that the trend is non-significant (p > 0.05) at a 95% confidence interval. The abbreviations in panel (A) refer to the Barents Sea Opening (BSO), Hopen Trench (HT), Bear Island Trough (BIT), Bear Island (BI), Storfjorden Trough (SFT), Franz Joseph Land (FJL), Kong Karls Land (KKL), Olga Basin (OB), and the St. Anna Trough (St.AT). The Kola Section (KS) is marked with a straight green line.
The highest SSTA trend was observed in the western Bering Sea, while the lowest trends were found in the eastern Bering Sea (Figure 3B). The highest SSTA values in the Barents Sea were observed in 2013 and 2016, which were associated with a strong positive phase of the East Atlantic Pattern (EAP), while the lowest values were recorded in 1982 and 1998 (Figure 3A). In the Bering Sea, the highest SSTA values were observed in 2003 and between 2014 and 2020, while the lowest anomalies were recorded in 1999 and 2012 (Figure 3B). It is noteworthy that the SSTA in the two basins showed opposing fluctuations (i.e., anti-correlated positive and negative anomalies) in 1997, 2003, 2012, and 2019. The overall temporal trends of SSTA were 0.31 ± 0.11 and 0.24°C ± 0.09°C/decade for the Barents Sea and Bering Sea, respectively (Figures 3A, B; Table 1).
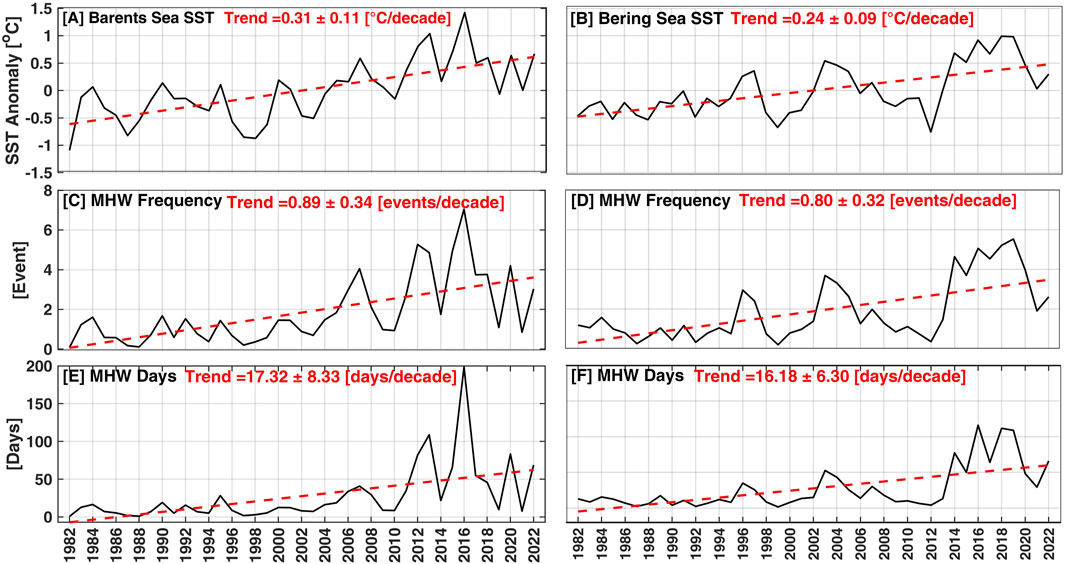
Figure 3. Temporal annual variability and trends for (A, B) sea surface temperature anomalies (°C/decade), (C, D) MHW frequency (events/decade), (E, F) MHW days (days/decade) between 1982 and 2022 for the two pathways to the Arctic Ocean: the Barents Sea (left column) and the Bering Sea (right column). The red dashed lines represent the best-fit linear curves of the SST and MHW characteristics. Note that the trend values are also displayed at the top of each subgraph.
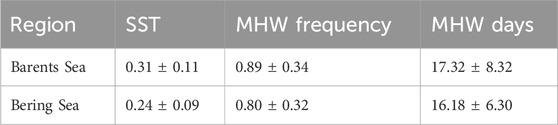
Table 1. Summary of the long-term trend in SST (°C/decade), MHW frequency (events/decade), and MHW days (days/decade) for the Barents Sea and Bering Sea between 1982 and 2022.
Statistically significant trends in MHW frequency and total number of days were observed in most areas of the Barents and Bering seas (Figures 2C–F). In the Barents Sea, the strongest trend in both MHW frequency and total number of MHW days was observed in southwestern Svalbard and the southern part of the Barents Sea (Figures 2C, E), i.e., in the regions affected by the inflow of warm Atlantic water. These patterns were broadly consistent with SST trends (Figures 1B, 2A), confirming the role of long-term warming in the formation of MHWs. The lowest trends in MHW frequency and total number of days are found in the south-western part and northern part of the Barents Sea. The low trends in these regions could be due to lower SST variability (Mohamed et al., 2022a), as the southwestern region is influenced by warm Atlantic water, while the northern regions are mainly influenced by cold Arctic water. An increase in the trend of MHW frequency was observed for most of the Bering Sea, excluding the Alaskan coast (Figure 2D). The largest MHW frequency trends (up to 1.8 events/decade) were observed in the western Bering Sea and along the Russian coasts, while the lowest MHW frequency trends were observed in the eastern Bering Sea and the Bering Strait (Figure 2D). The MHW days showed significant trends over the Bering Sea (Figure 2F). The highest MHW days trends (up to 28 days/decade) were observed in the central Bering Sea, while the lowest MHW days trends were found in the northern Bering Sea and the Bering Strait (Figure 2D).
A temperature shift was observed in the Barents Sea after 2004, which was associated with an increase in MHW events from 1 event per year to >3 events per year compared to the period before 2004 (Mohamed et al., 2022a; 2022b). The highest annual MHW frequency (>5 events/year) and total days (>100 days/year) were observed in 2012, 2013, and 2016 (Figures 3C–E), which were also the warmest years in the Barents Sea (Figure 3A). Sea ice duration in the Barents Sea in these warm years was <80 days/year (Mohamed et al., 2022a), which is considered a favourable condition for the MHWs and increases the probability of their occurrence. In the Bering Sea, the highest annual MHW frequency (>3 events/year) and total days (>40 days/year) were observed in 2003, and between 2014 and 2020 (Figures 3D, F). The occurrence of a MHW in the Bering Sea is highly correlated with the reduction of the sea ice concentration in the Chukchi Sea and the increase in the Alaskan air temperature (Carvalho et al., 2021). The average trends of MHW frequency and total days in the Barents Sea (Bering Sea) were 0.89 ± 0.34 (0.80 ± 0.32) events/decade and 17.32 ± 8.32 (16.18 ± 6.30) days/decade, respectively. The year 2016 stands alone with a record of 7 (5) events and 200 (110) MHW days in the Barents Sea (Bering Sea) (Figures 3C–F). In general, an increase in MHW frequency and total days were observed in both the Barents and Bering seas during the study period. The distribution of frequencies and total days followed similar temporal distributions of SST, indicating the role of Arctic amplification in the generation of MHWs. This suggests that the MHW metrics might be very different in these Subarctic regions if the MHWs were detected by removing the SSTs trend instead of using a fixed baseline.
One of the longest-lasting and most impactful MHW events in the northeast Pacific is known as the warm “Blob” that occurred in 2014–2016, while in the Bering Sea, multiple MHWs occurred in 2017–2019, as shown by SSTA (Figure 2B). The “Blob” was mainly caused by a persistent high-pressure system that led to a reduction in winds and heat exchange between the ocean and the atmosphere. This led to an accumulation of warm water in this region, causing ocean temperatures to reach record-breaking levels. The SST associated with the “Blob” was exceptionally high; in some regions, the SSTA was about 3°C–6°C above average (Gentemann et al., 2017).
3 Ecological responses to MHWs
The impacts of MHWs are superimposed on substantial climate-driven changes occurring across Arctic marine ecosystems. The Arctic is warming at a rate that is four times faster than the global average (Rantanen et al., 2022), which has significantly altered the Arctic and Subarctic marine ecosystems through the reduction in sea ice thickness and extent, warming sea temperatures, increased freshening and stratification, and higher turbidity due to melting land ice and run-off (Meredith et al., 2019). These climate-driven changes impact the entire food web, from the lower trophic level to top predators (Wassmann et al., 2011; Husson et al., 2024). For pelagic species in the western Arctic Ocean, increased stratification reduces nutrient supply (Polyakov et al., 2020; Zhuang et al., 2021), which appears to favour smaller phytoplankton types over larger diatoms, such as in the Beaufort Sea (Li et al., 2009) and Chukchi Sea (Neeley et al., 2018), and can cause harmful algal blooms (Anderson et al., 2022). Modelling studies also indicate that future climate change in the Arctic might favour phytoplankton species adapted to mid-latitude environments, such as coccolithophores, leading to a potential shift in planktonic ecosystems (Manizza et al., 2010; Neukermans et al., 2018). Additionally, sea ice loss affects the timing of phytoplankton blooms, extending the growing season (Manizza et al., 2023), while the delayed freeze-up of Arctic sea ice has also triggered a new and unprecedented novel fall bloom (Ardyna et al., 2014). Changes in sea ice cover and ocean warming also impact zooplankton populations, for example, large, high-lipid copepods tend to decline while smaller copepods with lower lipid content tend to increase in number and range, such as occurred in the Bering Sea (Kimmel et al., 2023) and Western Greenland (Møller and Nielsen, 2020). The population changes from high to low-lipid content has large ramifications for the marine food web. Reduced Arctic sea ice also increases the light reaching the sea floor in some areas, which can increase benthic primary production. This is driving increases in biomass and depth extent of macroalgal forests, seagrass meadows, and microalgal mats along some Arctic coastlines (Krause-Jensen et al., 2019; Attard et al., 2024). Yet, long-term data on these changes remains rare, and the impacts of sea ice loss are likely offset by increased turbidity from melting ice in other areas (Bonsell and Dunton, 2018; Filbee-Dexter et al., 2019). Upper trophic levels in the Arctic are also responding to long-term warming and sea ice retreat by altering their distributions, migration patterns, and phenology (Stafford et al., 2022; Husson et al., 2024; Kuletz et al., 2024a), and there is evidence of changes in their feeding, reproductive success, and survival (Mueter and Litzow, 2008; Fossheim et al., 2015; Stevenson and Lauth, 2019; Mueter et al., 2021; Renner et al., 2024).
The ecological response to MHWs can vary significantly based on the duration and intensity of the event (Joyce et al., 2024). Short and high-intensity MHWs are expected to cause acute stress, potentially increasing mortality, especially for sessile species (Smith K. E. et al., 2023; Garrabou et al., 2022). Longer, but not especially more intense, MHWs might in contrast alter the fitness of the organisms, such as altering their feeding, growth and reproduction, and ultimately lead to changes in mortality rates (Piatt et al., 2020). Long-lasting MHWs can also cause a distributional shift of species, impacting ecological communities and food webs. A global model study showed that while short MHWs might result in moderate biomass reduction, especially in the polar biomes, longer MHWs might cause larger ecosystem impacts, which would take longer to recover in polar systems than in temperate or tropical systems (Guibourd de Luzinais et al., 2024). We review below observed and modelled MHW’ ecological impact studies. We acknowledge that MHWs can have differentiated impacts on the ecosystems based on their characteristics, such as their duration, but in this review, we do not explicitly separate the ecological impacts based on the MHW characteristics, nor disentangle the effects of a MHW event from long-term warming.
3.1 Phytoplankton
Changes in upper-ocean stratification in the Arctic are directly attributed to MHW events, through increased warming and buoyancy to the top layers of the water column (Hu et al., 2020; Richaud et al., 2024). This change in stratification may consequently impact the availability of nutrients and light available to phytoplankton, thus altering the species composition of the planktonic ecosystem. During the recent MHWs in the subpolar North Pacific Ocean, several studies have shown shifts in phytoplankton functional groups (Suryan et al., 2021; Wyatt et al., 2022; Arteaga and Rousseaux, 2023; Strom, 2023). The 2014–2016 MHWs in the Gulf of Alaska (GoA) caused a shift in the phytoplankton communities through a reduction in surface biomass and shift from large to smaller cells (Suryan et al., 2021; Strom, 2023). Modelling studies in the GoA agree with observations and showed that the warm “Blob” in 2014 caused the occurrence of a phytoplankton shift, with dinoflagellates being favoured at the expense of diatoms due to the reduction in silicate availability caused by increased stratification (Wyatt et al., 2022; Arteaga and Rousseaux, 2023). Similarly, MHWs in the polar waters of the southern hemisphere caused a shift from cryptophyceae to nano-phytoflagellates and other heterotrophic groups (Latorre et al., 2023).
Studies on both long-term effects and on MHW events are aligned with previous literature on phytoplankton succession being driven by nutrients and turbulence (Margalef, 1978). Anthropogenic climate change in the Arctic and Subarctic regions has been transforming the physical and chemical habitat of planktonic organisms and thus driving ecological shifts. As these regions warm and become more stratified, smaller phytoplankton tend to dominate in calm, low nutrient environments, whereas larger phytoplankton succeed in areas of higher turbulence and greater nutrient concentrations (Ardyna and Arrigo, 2020). The modelling results focusing on MHW events, and those with projections into a future warmer climate, tend to converge on the response of the phytoplankton assemblages responding to large thermal perturbations, from the lower atmosphere to the upper ocean (Ardyna and Arrigo, 2020; Henson et al., 2021).
MHW events, and ocean warming more generally, can affect the bloom phenology. For example, in the Bering Sea, during years of intense warming (2017–2019) and low sea ice coverage, the phytoplankton bloom phenology markedly changed, with an increased importance of winds at controlling their seasonal timing and onset (Nielsen et al., 2024). Furthermore, MHWs can be associated with other extreme events such as acidification and de-oxygenation throughout the water column, as modelled in the GoA (Hauri et al., 2024). These compound extreme events could impact planktonic organisms in multiple ways, such as shifting from diatoms to nanophytoplankton (Hare et al., 2007), a decrease in taxonomic diversity (Hoppe et al., 2018), and competition changes within and between phytoplankton functional groups (Dutkiewicz et al., 2015). Shifts in phytoplankton composition can be furthered by consecutive MHW events. For example, coastal Arctic phytoplankton exposed to repeated heatwave conditions in incubation experiments revealed that both cool and warm phases of consecutive MHWs can have long-term impacts on phytoplankton physiology and composition (Wolf et al., 2024). Ultimately, additional studies are needed to determine the consequences of the co-occurrence of MHWs and ocean acidification on phytoplankton community composition.
The response of the phytoplankton to MHWs in the global ocean varies with latitude (Noh et al., 2022). Chlorophyll responses to MHW switch from negative to positive in both hemispheres around the 40°–50° latitude bands (subpolar regions), the areas where the strongest meridional gradient in nitrate concentration exists (Noh et al., 2022). In these response-changing regions, the latitudinal contrast of the chlorophyll response is starker in the warm season rather than in the cold season due to the shallower climatological mixed layer. The Noh et al. (2022) study highlights that the phytoplankton responses to MHWs highly depend on the upper-ocean interactions between phytoplankton and the mixed-layer that ultimately impacts the light and nutrient availability. Similarly, the magnitude of phytoplankton blooms during a MHW occurrence depends on background nutrient concentration (Hayashida et al., 2020). Considering that model projections suggest a poleward expansion of the nutrient-poor waters in the future, we would expect the development of weaker phytoplankton blooms during MHWs in the coming decades. However, their future model projections do not show a great impact on the Arctic and Subarctic oceans, where phytoplankton blooms during MHWs are expected to remain strong.
3.2 Zooplankton
Changes in the composition of phytoplankton could affect zooplankton assemblages, both directly (impacting their metabolism) and indirectly (changing their food source and trophic interactions). The impact of MHWs on Arctic zooplankton communities is still sparsely studied, but some observations from the “Blob” allow us to draw some general understanding of zooplankton response. In the northern California Current, there was an observed increase in the species richness of copepods but an overall decrease in the biomass of both copepods and euphausiids (krill) (Peterson et al., 2017), while other studies observed a decrease in euphausiid biomass concomitant with the “Blob” in the California Current (Cavole et al., 2016; Lavaniegos et al., 2019). In the GoA, a decline of euphausiids in the walleye pollock (Gadus chalcogrammus) diet during 2015 points to a potential negative impact of the MHW on euphausiid populations (Rogers et al., 2021), but contrasting observations reflect that the euphausiid response was not clear (Batten et al., 2022). Generally, warm-water associated zooplankton appeared to have increased during the MHW event in the GoA (Suryan et al., 2021; Batten et al., 2022), while the cool-water associated zooplankton community did not show any evident response (Suryan et al., 2021). Consistent with the observed impacts of long-term warming, MHWs are expected to impact the zooplankton communities by switching the dominance of high-lipid, cold-associated copepods towards low-lipid, warm-associated copepods, as observed in the Bering Sea (Duffy-Anderson et al., 2019). Being at the base of the food web, lower zooplankton food quality (i.e., low-lipid organisms), can have repercussions on the rest of the food web, such as resulting in a decrease in fish larvae and juvenile survival, and ultimately increased mortality of upper trophic levels (Figure 4, Box 1).
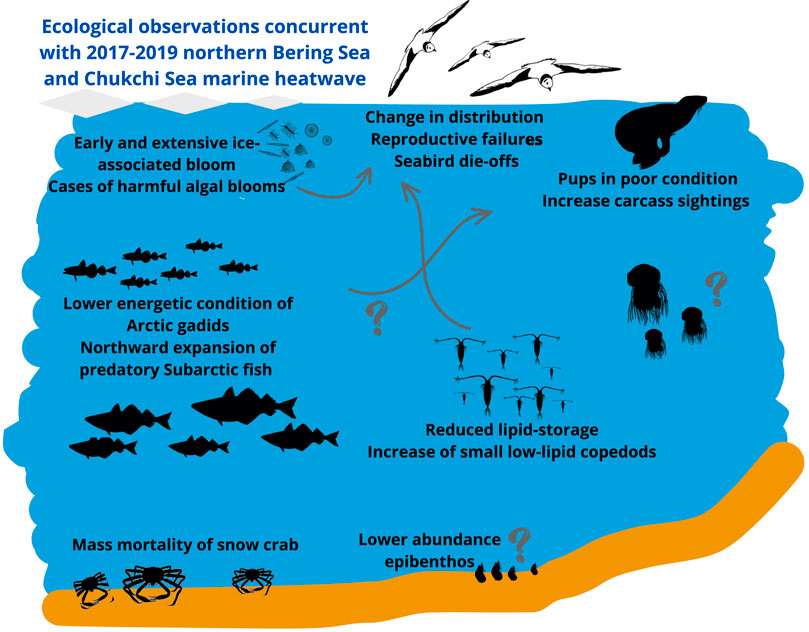
Figure 4. Schematic representation of ecological observations attributed to the 2017–2019 MHWs in the northern Bering Sea and the Chukchi Sea. The question marks indicate that there remain large knowledge gaps on the ecological response to MHWs in this Arctic LME (e.g., the response of the pelagic and benthic invertebrates and the indirect, food web mediated effects).
Jellyfish distributional response to warming temperatures has been examined in the Bering Sea (Brodeur et al., 2008; Decker et al., 2023), but linkages to MHWs in the Arctic have not been studied. Summer trawl surveys in the eastern Bering Sea of jellyfish biomass from 1975 to 2005 showed an increase in jellyfish biomass throughout the 1990s. Starting in the 2000s, warmer temperatures co-occurred with a peak in jellyfish biomass, which then stabilized throughout the rest of the study period. On the other hand, a hindcast model for the Eastern Bering Sea from 1985 to 2017 observed no significant increases in jellyfish biomass in warmer years, which may be evidence of jellyfish adaptation to ecosystem shifts (Decker et al., 2023). These long-term observations and models indicate the sensitivity of jellyfish biomass to thermal regime changes, underscoring the need to understand MHWs impacts on jellyfish populations, as changes in jellyfish biomass can significantly impact the Subarctic ecosystems, particularly affecting zooplankton and fish communities through direct predation and competition (Brodeur et al., 2002).
3.3 Benthic species
Sessile foundation species provide ecosystem structure and function, through the establishment of a physical framework that supports other species (Wernberg et al., 2024). For example, Arctic kelp species, which are large brown seaweeds occurring throughout Arctic coastal waters, provide habitat and food for many ecologically and commercially important species, due to their high standing biomass, productivity, and physical habitat structure (Filbee-Dexter et al., 2019; Lavoie et al., 2024). Changes in the composition and abundance of sessile foundation species may severely degrade the health and resilience of marine systems to other stressors (Thomson et al., 2015). These sessile species are particularly vulnerable to MHWs due to their inability to escape, making them more sensitive compared to mobile species such as fish (Smale et al., 2019). Although sessile organisms can acclimate to tolerate slightly warmer conditions (Staehr and Wernberg, 2009; Staehr and Borum, 2011), temperatures above a certain optimum will affect performance negatively, for example, through reduced growth and reproduction, while exposure to temperatures near the upper level of tolerance lead to enhanced mortality. Severe heat stress occurring under a MHW may thus lead to reduced fitness, local extinctions, and eventually, to large-scale changes in range distribution.
Only a few studies have documented the ecological effects of MHWs on Arctic sessile foundation species, however studies from other areas indicate negative effects at all levels (species, communities, ecosystems) with some effects persisting long after the MHW event. MHWs are most damaging at the warm range edges of species, because they push the local temperatures across thermal thresholds for mortality (Smale et al., 2019; Filbee-Dexter et al., 2020). In the Arctic, most foundation species are at their cool range edges and therefore may not yet be vulnerable to MHWs. This is particularly true for Arctic macrophytes, which have only recently recolonized the Arctic after it reopened following the last ice age. These plants are therefore near their cool range edges and experience water temperatures well below their thermal maxima (Bringloe et al., 2022). Climate-driven increased water temperature and decreased sea ice cover can therefore benefit foundation species in the Arctic. For example, persistent losses in sea ice cover along Arctic coastlines are expected to expand habitats for macroalgae and seagrass (Attard et al., 2024), although there is yet little evidence of these impacts (Krause-Jensen et al., 2020).
An analysis of the vulnerability of seagrass to MHWs across their global distribution, using empirically- or experimentally determined seagrass upper thermal limits, found that seagrass growing in boreal and Subarctic regions will not cross their thermal limits within the next 200 years, making direct mortality from a MHW unlikely (Marbà et al., 2022). Similarly, kelps are often living below their thermal optima in the Arctic. This lack of vulnerability is supported by a laboratory study of MHW impacts on two kelp species (Agarum clathratum and Saccharina latissima) at their cold range limit in Greenland. The study found no impact of higher temperature during a simulated MHW on the species, and showed that warmer temperatures mitigated (to some extent) the impact of low light stress (Niedzwiedz et al., 2024). Yet, in some regions of the Subarctic, kelps are near their thermal maxima and can be susceptible to summer MHW events. An example of a dominance shift following a MHW is the observed decline in macroalgal foundation species in the northern GoA following the “Blob,” which caused a region-wide shift in rocky intertidal habitats from a state dominated by autotroph-macroalgal (Fucus distichus) to a heterotroph-filter-feeder dominated state (Weitzman et al., 2021). Other examples of vulnerable kelps could include the Arctic endemic kelp Laminaria solidungula, which is range restricted to 6°C summer temperatures, although it can tolerate up to 16°C for up to 2 weeks (Roleda, 2016).
Seagrasses generally display a wide temperature tolerance range with the ability to acclimate making them capable of dominating the sublittoral soft bottom zone of climatic zones extending from tropical to arctic conditions (Staehr and Borum, 2011 and references therein). While seagrasses generally display a capacity for range shifts, some slow growing species are less tolerant and capable of acclimating to sudden temperature elevations, potentially causing negative local impacts of MHWs for seagrasses living near their upper temperature tolerance limits. Evidence of such negative local impacts on seagrasses has so far not been found in Arctic waters. Overall, the impact of MHWs on the sessile foundation species adds to other important human related pressures, such as overgrazing from sea-urchins (stimulated by overfishing of top-predators), coastal eutrophication, and physical disturbance from human activities like sediment extraction and bottom trawling. Reducing these pressures is therefore crucial for enhancing coastal ecosystem resilience to MHW impacts (Wernberg et al., 2024).
High-latitude marine ecosystems are characterized by relatively high benthic biomass, with the Bering Sea and the Chukchi Sea being notable “hotspots” of benthic biomass (Wei et al., 2010; Grebmeier et al., 2015). Despite this, the impact of MHWs on high-latitude benthic invertebrates has generally been little studied. One significant exception is the documented mass die-offs of snow crab (Chionoecetes opilio) in the Bering Sea between 2018 and 2021 (Figure 4, Box 1), making it one of the largest observations of the negative ecological effects of MHWs (Szuwalski et al., 2023). A combination of increased crab densities due to reduced habitats, through the disappearance of water masses lower than 2°C on the sea floor (i.e., the “cold pool”), and increased caloric demands due to the heatwave, likely resulted in the starvation of up to 10 billion snow crabs (Szuwalski et al., 2023). Prior to this MHW event, the snow crab population was assessed to be at historically high abundance, with a large recruitment of immature crabs. Other negative impacts of MWHs on benthic invertebrate communities were observed in the southeastern Chukchi Sea, where the epibenthic biomass declined in 2017 following multiple warm years (Huntington et al., 2020). In the GoA, contrasting trends in benthic invertebrate populations were observed after the onset of the “Blob,” with sea star abundance generally declining while mussels, a prey of sea stars, generally increased (Suryan et al., 2021). In the Barents Sea, benthic monitoring in a Svalbard fjord showed that benthic invertebrate’s abundance and richness declined sharply after a MHW event in 2006, but the benthic community appeared to recover after several colder years, pointing towards some potential resilience of benthic communities (Jordà-Molina et al., 2023).
3.4 Fish
Fish responses to MHWs in the Arctic and Subarctic ecosystems are also relatively unknown, whereas many studies from non-Arctic regions have documented MHW impacts on fish. Notable exceptions are the 2014–2016 northeastern Pacific “Blob” and the Bering Sea and Chukchi Sea MHWs in 2017–2019, which had profound and cascading impacts on fish populations in the North Pacific. In the GoA, forage fish species such as Pacific capelin (Mallotus catervarius), Pacific sand lance (Ammodytes personatus), and Pacific herring (Clupea pallasii) experienced historically low abundance and decreased body conditions due to the MHW (i.e., lower quality prey for top predators) (Biela et al., 2019; Arimitsu et al., 2021; Robinson et al., 2023). The polar cod (Boreogadus saida), another crucial forage fish, also experienced large declines in nutritional value due to the MHW in the Chukchi Sea (Copeman et al., 2022). These changes in the body conditions of fish can have ramifications for energy transfer to top predators. Additionally, the “Blob” had large impacts on fish early life stages, such as the walleye pollock in the GoA. Record-low levels of pollock larvae were observed in 2015, and their summer survival rates were significantly reduced, resulting in very low abundances of juvenile pollock (Rogers et al., 2021). Not only the extreme temperature, but multiple factors such as low-saline conditions, low zooplankton density, and poor body condition of age-0 pollock, contributed to the juvenile pollock declines (Rogers et al., 2021), illustrating the complex direct and indirect interactions influencing fish responses to climate warming. In the northern Bering and Chukchi seas, a large influx of juvenile walleye pollock and increase in the abundances of juvenile pink salmon (Oncorhyncus gorbuscha) and age-0 polar cod were concomitant to the 2017–2019 MHW (Huntington et al., 2020; Levine et al., 2023) (Box 1).
MHWs can cause demographic responses in fish populations, influencing recruitment success, growth patterns, and natural mortality (Box 1). For example, in the GoA, MHWs induced changes in the phenology and growth of Pacific cod (Gadus macrocephalus) juveniles, causing earlier hatching and faster growth (Almeida et al., 2024). The biomass of adult Pacific cod in the GoA, which sustains an important commercial fishery, had severely declined in 2017 following the MHW (Barbeaux et al., 2020). The decline was most likely due to increased metabolic demand combined with lower prey availability, but also reduced survival of recruits and loss of suitable spawning habitat (Barbeaux et al., 2020; Laurel and Rogers, 2020). Fish thermal tolerance range is often the smallest during early-life stages, e.g., eggs and larvae, which are highly sensitive to temperature variations (Dahlke et al., 2020). If a MHW coincides with the distribution of critical life stages (in space and time), it can result in high mortality; for instance, walleye pollock in the GoA experienced significant early-life stage mortality (i.e., recruitment failure) during the “Blob” (Rogers et al., 2021). Analyses of species’ thermal niches can inform on species vulnerability to MHWs, for example, in the Pacific Arctic, juvenile polar cod are more likely to suffer from increased temperatures during MHWs compared to other gadids such as saffron cod (Eleginus gracilis), walleye pollock, and Pacific cod, due to negative impacts of warm temperature on their activity, growth, and survival at a lower temperature threshold (16°C; Laurel et al., 2016).
A behavioural response of fish to abnormal sea temperatures is to move to more suitable areas, for example, by moving to climatic refugia and/or following their thermal niche (Jacox et al., 2020; Alabia et al., 2021). These displacements can cause large changes in the composition of the fish assemblages (abundance, biomass, and diversity), but in many instances these changes appear to be short-term. For example, the “Blob” in the northern California Current led to large but short-term changes in fish species abundances in eelgrass meadows, with the fish assemblage quickly returning to baseline levels (Robinson et al., 2022). In the northern Bering Sea, spatial distributions of several commercially important fish species shifted during the warm summer of 2017, with increased biomass of walleye pollock, Pacific cod, and northern rock sole (Lepidopsetta polyxystra) in the northeastern Bering Sea (Stevenson and Lauth, 2019). In the Barents Sea, community-level redistributions were apparent during MHWs, but they appeared to affect different fish species and groups, and long-term effects were not evident (Husson et al., 2022). In accordance, a large-scale analysis of demersal fish communities across Europe and North America, including the Subarctic Barents Sea and Bering Sea did not find significant impacts of MHWs on the fish biomass, abundance, and biodiversity (Fredston et al., 2023). Still, MHWs could facilitate the northward range expansion of Subarctic fish species into the Arctic. This is exemplified by walleye pollock, which extended its northern range into the southern and central parts of the Chukchi Sea during the 2017–2019 MHW events (Levine et al., 2023).
Global simulation models indicate that MHWs in exclusive economic zones could lead to a 6% drop in maximum commercial fish catch potential, affecting 77% of exploited fishes and invertebrates (Cheung et al., 2021). However, negative impacts from MHWs in Subarctic regions are expected to be on average smaller than for the temperate and tropical regions (Cheung et al., 2021). These global models also highlight that MHWs can cause a substantial and rapid biomass decrease and shifts in fish distribution, with a projected doubling of MHW-related effects on major commercial fish species by 2050 (Cheung and Frölicher, 2020).
3.5 Upper trophic levels: seabirds and marine mammals
Upper trophic level (UTL), homeothermic vertebrates like seabirds (here, defined as all birds that use the marine environment), and marine mammals generally show a delayed response to MHW events, due to their trophic distance from primary productivity and lower trophic levels, longer life spans, and high mobility (Burger and Piatt, 1990; Johns et al., 2022; Orgeret et al., 2022). In addition, at least for marine mammals, their large bodies and capacity for energy storage via fat reserves buffer interruptions to the food web. However, the thick blubber of some Arctic cetaceans, like narwhals (Monodon monoceros) and bowhead whales (Balaena mysticetus), may reduce their ability to dissipate heat. Narwhals, in particular, have limited physiological flexibility to adjust their swimming or diving behaviour to thermoregulate if water temperatures become extreme (Chambault et al., 2020). Given evidence that the distributional range of Arctic marine mammals is temperature driven, MHW events could have potentially direct negative impacts on these species. There have been multiple examples of the delayed response of seabirds and marine mammals to MHWs, ranging from weeks to years (Gulland et al., 2022; Jones et al., 2023; Welch et al., 2023; Renner et al., 2024). Most of these examples are from non-Arctic regions, but have included Subarctic marine species that use the Arctic for some portions of their life cycles.
Although UTL animals may show longer-term impacts or delayed responses to MHWs, there are more immediate direct and indirect effects. Physiological responses to intense heat, i.e., heat stress, can occur in the water and on land. For instance, the high atmospheric temperatures that typically accompany MHWs can cause heat stress for seabirds incubating eggs or attending chicks on open cliff nests (Cook et al., 2020). MHWs can result in toxic algae blooms and diseases that directly affect UTLs. In the northern Bering and Chukchi seas, seabird die-offs were associated with toxic algae blooms (Van Hemert et al., 2020), and in the northern Bering Sea, avian influenza was detected in murres (Uria spp) during the 2018 heatwave (Will et al., 2020b). In the Canadian Arctic, avian cholera outbreaks increased with climate change, threatening populations of northern common eiders (Somateria mollissima borealis) (Iverson, 2015). The first recorded mortality of a polar bear (Ursus maritimus) from avian influenza occurred in the winter of 2024; the bear was likely infected by scavenging on infected marine birds, which has broad conservation and health issues for mammalian species, including humans (Ward, 2024).
High marine mammal mortality has been documented during extended warm periods, including humpback whales (Megaptera novaeangliae) and fin whales (Balaenoptera physalus) following the 2014–2016 GoA MHW (Gabriele et al., 2022). However, the spatio-temporal dispersal of observations makes it difficult to link their deaths to specific MHWs (Albouy et al., 2020; Gabriele et al., 2022). For marine mammals, reduced survival can be a long-term impact from MHWs, including reduced abundance and low calf production (Suryan et al., 2021; Gabriele et al., 2022).
Variation in impacts can vary even within a broad ecological region, such as documented for endangered Steller’s sea lions (Eumetopias jubatus) during and after the GoA MHW (Hastings et al., 2023). Indirect impacts of MHWs on UTLs include changes in the prey field and/or foraging habitat. Following the GoA MHW, changes in distribution by humpback whales and their prey resulted in higher entanglement of whales in fishing gear farther south, due to greater temporal and spatial overlap of whales and fisheries (Santora et al., 2020). A specifically Arctic example is that, as sea ice retreats during exceptionally warm years, forage fish like polar cod move farther offshore or into waters too deep for diving seabirds [e.g., black guillemots (Cepphus grylle); Divoky et al., 2021].
In the northern GoA, the 2014–2016 MHW reduced the abundance of three important forage fish species, which impacted UTL consumers during the MHW (Arimitsu et al., 2021), and for years afterward (Suryan et al., 2021), with long-term demographic consequences (Schoen et al., 2022). Demographic impacts were also documented for common murres (Uria aalge) in the North Atlantic, with evidence that the behavioural flexibility of murres in response to higher SSTs was not sufficient to ameliorate immediate and long-term negative impacts on reproductive success (Wanless et al., 2023). Some seabirds, such as black-legged kittiwakes (Rissa tridactyla) in the GoA, altered foraging behaviour and searched a wider area for prey to feed chicks. However, this species experienced widespread breeding failure in the GoA, which continued for 5 years after the MHW (Osborne et al., 2020). In contrast, benthic-feeding sea ducks did not show evidence of negative impacts from MHW conditions in the northern GoA, illustrating the importance of considering trophic interactions when predicting resilience to anomalous conditions (Robinson et al., 2023).
MHW effects on prey species, such as reduced size and nutrient content of zooplankton (Kimmel et al., 2023), were detrimental for planktivorous seabirds nesting in the northern Bering Sea (Will et al., 2020a). Indeed, an ecosystem model suggested that loss of high-lipid Arctic copepod species may have the greatest impact on UTLs, including forage fish, planktivorous seabirds and a variety of marine mammals (Gillie et al., 2024). Forage fish also had reduced size-at-age and lower fat content following the extremely warm period (with multiple MHW events) in the Bering Sea during 2017–2019 (Copeman et al., 2022). These changes in prey were associated with unusual seabird die-offs and breeding failures (Romano et al., 2020; Will et al., 2020a; Kaler and Kuletz, 2022; Jones et al., 2023). The 2017–2019 MHW events in the northern Bering and Chukchi seas also facilitated a massive influx of juvenile walleye pollock into the Chukchi Sea (Levine et al., 2023), which, along with changes in other prey species and foraging conditions, influenced the distribution of planktivorous and piscivorous seabirds there (Kuletz et al., 2020; 2024b) (Box 1).
The timing of prey availability in the Arctic is critical to UTLs, particularly those that attempt to raise young (i.e., many seabirds, walrus, and seals) or must obtain adequate food for long migrations, e.g., shearwaters Ardenna sp., and large cetaceans (Kuletz et al., 2024a). In one cross-taxa modelling exercise focused on the eastern North Pacific, Welch et al. (2023) found that top predators could vary widely in their response to MHWs, with some species benefiting from newly available suitable habitat, while others faced reduced habitat, and in some cases, species-specific responses varied between MHW events. Coastal UTL species, and more southerly species, showed the greatest spatial displacement during MHW events (Welch et al., 2023).
Most UTLs can “buffer” against short-term ecosystem perturbations via moving from impacted areas, behavioural flexibility, or switching to alternative prey (Sinclair et al., 2008; Jones et al., 2023 and references therein; Woehler and Hobday, 2023). However, mass mortality events of seabirds and marine mammals are often associated with MHWs (or a series of brief MHW events) in Arctic and Subarctic regions, indicating significant and long-term impacts to the ecosystem that overrode the behavioural flexibility of UTLs (Jones et al., 2023). Examples include tufted puffins (Fratercula cirrhata) and crested auklets (Aethia cristatella) in the Bering Sea in 2017 (Jones et al., 2019), and murres and shearwaters in the same region in 2018 (Duffy-Anderson et al., 2019; Romano et al., 2020). In the North Atlantic, northern gannets (Morus bassanus) starved during the 2012 MHW off Newfoundland (Montevecchi et al., 2021). Seabird mortality during the GoA MHW, originally estimated at 3 million seabirds, primarily common murres (Piatt et al., 2020; Jones et al., 2023) is now estimated to have claimed 4 million common murres, half of the Alaskan population, and the largest seabird mortality event on record (Renner et al., 2024). Notably, 5 of the 13 colonies monitored for this study are located in the southeastern Bering Sea, reflecting impacts to populations that overwinter in the GoA but breed elsewhere.
Animals often redistribute in response to MHWs, e.g., chick-rearing kittiwakes foraged over a wider area in the northern GoA (Osborne et al., 2020), and short-tailed shearwaters (Ardenna tenuirostris) spread across the Chukchi Shelf in 2017 and 2019; the latter was concurrent with the invection of warm Bering Sea water there, and the lack of concentrations of euphausiids (Kuletz et al., 2024b). For long-distance migrants like the shearwaters, MHW conditions may result in longer, more wide-spread foraging trips (Yamamoto et al., 2015) and be reflected in later arrival at southern hemisphere breeding areas and low breeding success (Glencross et al., 2021). While some Subarctic breeding animals may adjust to warm conditions by foraging farther north (e.g., thick-billed murres Uria lomvia, and short-tailed shearwaters (Kuletz et al., 2020), those that reside in the Arctic year-round do not have that option. For example, following the warm conditions in the northern Bering and Chukchi seas, ice-seals like spotted seal (Phoca largha) had high mortality and their pups were in poor condition (Huntington et al., 2020).
4 Discussion
4.1 Social, economic, and cultural impacts on Arctic and Subarctic communities
The multifaceted MHW impacts outlined above have had substantial social and economic impacts across fishing and coastal communities, subsistence-based communities, and diverse marine users. MHWs reverberate across the spectrum of human wellbeing, which is defined as the state when basic needs are met and individuals and communities can pursue their goals and enjoy a satisfactory quality of life (Breslow et al., 2016). Social scientists have identified the numerous dimensions of human wellbeing tied to marine ecosystems, including mental and physical health, social connections to place and people, intergenerational knowledge transfer, cultural and spiritual practices (Breslow et al., 2016). The following two paragraphs describe overarching effects of MHWs on human dimensions, while the final three paragraphs detail the impacts specifically on the social, economic, and cultural systems of Arctic and Subarctic communities.
MHW impacts on commercial and subsistence fisheries such as fish stock downturns, harvest restrictions, closures, and shifts in fishery timing have been particularly detrimental for communities. Direct economic impacts of these changes have been estimated at hundreds of millions of US dollars for a single fishery (Smith et al., 2021; Szuwalski et al., 2023). Not all impacts have been adverse, with some species responding positively with range extensions and increasing abundance from favorable conditions (Smith et al., 2021; Free et al., 2023). Yet, even these seemingly positive impacts have been associated with intensified conflicts across multiple political boundaries (Pershing et al., 2018; Szymkowiak and Steinkruger, 2023).
MHWs have also disrupted traditional ways of life and subsistence lifestyles across the world as ecological changes have altered the marine food web upon which indigenous coastal communities rely. Fisheries downturns associated with MHWs have included critical subsistence and cultural keystone species, undermining multi-generational connections to these resources and food security (Falardeau et al., 2022; Free et al., 2023). Mass mortality events of seabirds and marine mammals associated with MHWs have also undermined subsistence harvests with profound food security implications for indigenous communities (Young et al., 2014; Naves, 2018; Siddon et al., 2020). Large-scale harmful algal bloom events associated with MHWs have increased safety hazards associated with paralytic shellfish poisoning in shellfish, hindering traditional shellfish gathering practices and eliminating this critical food source for indigenous peoples (Kourantidou et al., 2022). Kelp mortality from MHWs has undermined traditional knowledge and spiritual values for indigenous communities that have utilised kelp for thousands of years in numerous cultural practices (Smith K. E. et al., 2023). Subsistence harvest practices have also been affected by altered migratory patterns and timing of seabirds and marine mammals, and their accessibility to hunters due to sea ice loss (Kuletz et al., 2024a and references therein).
In the Arctic and Subarctic, MHWs have been increasing in frequency and extent, disrupting coastal socio-ecological systems grounded in marine resource access and use. The “Blob” drove unprecedented fisheries downturns in the GoA across both groundfish and salmon fisheries, which were exacerbated by the return of MHW conditions in 2019 (Barbeaux et al., 2020; Free et al., 2023). From 2015 to 2017, the Pacific cod fishery in the region experienced a 70% decline, and after multiple years of increases in harvest restrictions, the fishery closed in 2020 due to concerns over the sustainability of the resource (Barbeaux et al., 2020; Laurel and Rogers, 2020; Peterson Williams et al., 2022). Starting in 2016, the region also experienced disasters across several different salmon species and runs, attributed to poor oceanographic conditions (Bellquist et al., 2021). Rapid and repeated declines in multiple fisheries over a 5-year timespan precipitated an exodus of participants from these fisheries and created uncertainty and stress in fishing communities (Suryan et al., 2021; Abelman et al., 2023). Although sablefish had an unprecedented recruitment class following the heatwave, the tremendous influx of small fish into the commercial fishery disrupted the market and led to substantial declines in prices (Szymkowiak and Rhodes-Reese, 2020). At the same time, fishermen’s attempts at exploiting the increased abundance of warm water species in the region to buffer declining revenues were largely unsuccessful due to conservation concerns over traditional target species (Szymkowiak et al., 2024). The MHW also precipitated increased polarization of viewpoints about fisheries allocations and enhancements amongst fisheries stakeholders in the face of declining abundance, signifying the impact of these abrupt changes on social conflict in the region (Szymkowiak and Steinkruger, 2023).
In addition to disruptions in fisheries, the “Blob” created tremendous upheaval across diverse marine user groups. Large-scale die offs of seabirds in the GoA disrupted traditional seabird harvest (Piatt et al., 2020). The warm waters precipitated the largest harmful algal bloom on record, with paralytic shellfish poisoning events and oyster farm closures in Kachemak Bay near Homer, Alaska (Walsh et al., 2018). Increased harmful algal blooms and paralytic shellfish poisoning generated food safety concerns throughout Alaska, disrupting shellfish gathering for commercial and subsistence harvesters (Walsh et al., 2018). The MHW also resulted in declines in cetaceans (see Section 3.5), which had adverse impacts on the marine tourism industry in the region (Suryan et al., 2021).
The socio-ecological systems that depend on the Bering and Chukchi seas have also experienced unprecedented disruptions in the years following the 2017–2019 MHWs in the region. Warm water temperature and declines in the extent and duration of sea ice cover prevented traditional ice-based harvesting of marine resources, i.e., fish, crabs, seals, and whales (Walsh et al., 2018). Access issues were compounded by seabird and marine mammal die offs in the region and downturns in availability of these resources due to breeding failures and changes in migratory patterns (Walsh et al., 2018; Siddon et al., 2020; Kuletz et al., 2024a). HAB events and associated food safety concerns also extended to the Bering Strait region at this time (Walsh et al., 2018; Siddon et al., 2020).
Reductions in lipid-rich prey, due to warmer waters in the region, also precipitated steep declines in chum salmon (Oncorhynchus keta) run sizes, leading to closures of the commercial and subsistence fisheries in western Alaska (Farley et al., 2024). This exacerbated ongoing Chinook salmon (Oncorhynchus tshawytscha) run failures in the region, which are critical for the cultural fabric and spirituality of the indigenous people in the region (Carothers et al., 2021), and food security issues (Farley et al., 2024). In addition to impacts on salmon, the MHWs in the Bering and Chukchi seas led to large scale shifts in fisheries resources in the region. There were substantial northward migrations of highly valuable commercial fish populations – Pacific cod and walleye pollock – associated with the MHW (Duffy-Anderson et al., 2019; Siddon et al., 2020; Levine et al., 2023). In response, fishing fleets experienced longer travel times between fishing grounds and ports and the shift triggered long-term considerations about the potential need to shift stock assessment survey grounds, fisheries management boundaries, and processing capacity to meet the shifting spatial distribution of the resources (Siddon et al., 2020). The mass mortality of the Bering Sea snow crab led to the closure of the fishery for the first time in history for the 2022–2023 season, causing the largely indigenous, island community of St. Paul, which relies heavily on revenue from processing crab and servicing the crab fishing fleet, to declare a cultural, economic, and social emergency (Szuwalski et al., 2023).
4.2 Adaptive management and mitigation of impacts from MHWs
Arctic and Subarctic fisheries are feeling the impact of MHWs due to the ecological disturbances affecting harvested marine populations (Barbeaux et al., 2020; Szuwalski et al., 2023; Farley et al., 2024). Considering the expected increase in the frequency of MHWs, there is a need to transition towards adaptive management of marine resources (Free et al., 2023). Adaptive resource management, a more flexible decision-making process, involves managing natural resources while simultaneously learning from outcomes and adapting strategies based on new information and changing conditions (Allen et al., 2011; Williams and Brown, 2014). By enhancing flexible harvest strategies and quick responses it can better mitigate and adapt to the impact of MHWs on socio-ecological systems (Caputi et al., 2016; 2019). The socio-economic consequences and management decisions following the two extreme MHWs in Australia in 2010–2011 and the northeast Pacific in 2014–2016, provided useful lessons about the management of fisheries following MHWs (Caputi et al., 2019; Free et al., 2023). Caputi et al. (2019) highlighted the importance of adaptive fisheries management to protect ecological populations that suffered recruitment failures due to MHWs, suggesting that early identification of temperature hot spots and abundance changes as well as flexible harvest strategies, can allow for a quick response, to minimize the effect of fishing on reduced commercial populations. Fisheries catch data from Alaskan fishing communities revealed that diversifying resource portfolios and adapting to changing fishing opportunities can help mitigate economic instability during major ecological and/or economic disruption (Cline et al., 2017). This emphasizes the importance of maintaining diverse economic options to strengthen the resilience of the socio-ecological systems to extreme events (Cline et al., 2017).
As species’ distributions shift with warming or in temporary response to MHWs (Kuletz et al., 2024a), changes in monitoring design and fisheries management boundaries might be needed (Siddon et al., 2020; Free et al., 2023). Early detection of ecological impacts through monitoring of fish and invertebrates’ early-life stages and abundance would require increasing monitoring efforts, which although desirable might not be possible due to the economic costs of scientific surveys. Yet, monitoring programs could be modified to increase knowledge of the system response and identify early-warning signals by increasing the use of alternative monitoring sources, such as eDNA and fishery-dependent data (Borgman et al., 2022; Free et al., 2023). For example, eDNA sampling could be sampled by ships-of-opportunity and thereby help expand spatial and temporal coverage and reduce costs (Valsecchi et al., 2021). This approach could be especially useful in the Arctic, due to its remoteness and limited seasonal accessibility. In addition, early identification of SST anomalies through satellite monitoring can provide an early warning of MHWs occurrence (Hobday et al., 2016). This can inform of potential socio-ecological disturbances and thus trigger adaptive management strategies. Improved SST forecasts can also be used for short-term predictive models to inform dynamic management measures, such as adjusting harvest guidelines and reducing fisheries bycatch (Holsman et al., 2019). In Arctic coastal regions, which harbor critical habitats like kelp forests, monitoring of sea temperatures should be supplemented with continuous in situ observations (Borgman et al., 2022). There is thus a need for increased funding and support for the development and implementation of advanced detection and monitoring technologies, such as satellite monitoring for early detection of SST anomalies, eDNA sampling for ecological monitoring and in-situ observations.
Additionally, expanding Marine Protected Areas (MPAs) and effectively enforcing them, particularly by covering climate refugia, could significantly enhance the resilience of Arctic marine ecosystems to climate-induced stressors (Ainsworth et al., 2020; Queirós et al., 2021). MPAs, restricting some to all human activities within a defined area, are often seen as a key tool to protect marine biodiversity and build ecosystem resilience. While MPAs have had successes in terms of restoring biodiversity due to the relaxation of human pressures (e.g., of no-take MPAs; Sala and Giakoumi, 2018), the performance of MPAs to limit the ecosystem effects of uncontrollable external stressors and extreme events (e.g., storms or temperature rise) is less obvious. In the Arctic, the multi-level impacts of sea ice loss could prove particularly challenging in terms of applying MPAs to mitigate detrimental effects. Evidence suggests that MPAs, compared to unprotected sites, may provide refuge and contribute to the resilience of fish communities during MHWs by, e.g., dampening the loss of trophic diversity and accelerating the recovery of taxonomic diversity (Ziegler et al., 2023; Benedetti-Cecchi et al., 2024). Yet, the potential of MPAs as a tool to mitigate the impacts of MHWs on ecological communities is still unclear (Freedman et al., 2020; Smith J. G. et al., 2023), indicating the need for further research to better understand the role of area-based protection in mitigating climate impacts. In addition, the efficacy of MPAs can be compromised by inadequate enforcement or design, for example, by not spatially covering climate refugia. Climate refugia are areas that remain relatively detached from climate change impacts, which could provide important sanctuaries for vulnerable Arctic species during extreme events like MHWs. The Arctic currently has several MPAs; as of 2020, over 5% of the Arctic’s marine areas had some level of protection (CAFF/PAME, 2022). This coverage fell short of the 2020 Aichi Biodiversity Target 11 of 10% marine protection and is far from the 2030 target 3 of the Kunming-Montreal Global Biodiversity Framework of 30% of ecologically representative and effective protection and management of global marine areas. In addition to MPAs, other regulations and spatial restrictions can play important roles in protecting marine biodiversity, such as the Central Arctic Ocean (CAO) fisheries agreement, which is a 16-year ban of commercial fishing in the CAO. Finally, international collaborations should be strengthened to designate Other Effective Conservation Measures (OECMs) and identify vulnerable marine ecosystems, supporting global efforts towards achieving the biodiversity targets set for 2030 (CAFF/PAME, 2022).
4.3 Conclusion and future research needs
The intensity, duration, and frequency of MHWs have increased in the Arctic Ocean and its marginal seas. Significant progress has been made in understanding the onset and dynamics of MHWs, as well as in improving the forecasting of MHW events globally. In the Arctic and Subarctic marine ecosystems, MHW dynamics are unique due to the interplay between sea ice, ocean, and atmospheric dynamics, where declining sea ice amplifies MHW occurrence, duration, and intensity by exposing open water to solar heating and reducing temperature variability. Subarctic marginal seas like the Barents and Bering seas experience high spatial variability in MHW frequency and duration due to regional influences such as warm Atlantic water inflow, Arctic amplification, and sea ice retreat.
While Arctic MHWs appear to be primarily driven by atmospheric heat fluxes and oceanic heat advection in inflow regions, there remain substantial knowledge gaps regarding the subsurface mechanism of MHWs, compound events, the potential impacts on marine ecosystems and fisheries, and the predictability and forecasting of MHWs in the Arctic regions. Also, identifying MHWs in the Arctic based on satellite observations of SST include challenges due to the sea ice cover limiting the number of open-water days, and, definning relevant. Defining relevant MHW threshold is difficult in the Arctic regions due to the low sea temperature variability which causes the MHW threshold to be very close to the climatology. This highlights the need for further research to understand better the detection and mechanisms of MHWs in the polar regions, such as increased knowledge about subsurface mechanisms, the effect on the MHW identifications of removing the SSTs trend instead of using a fixed baseline, and more generally the limitations of applying MHWs detection methods developed in non-polar regions to the Arctic.
Many Arctic species have narrow thermal preferences, making them especially vulnerable to MHWs, as many are already at, or near their upper thermal limits. The limited behavioural flexibility caused by extreme photoperiods further exacerbates the risks for these species. MHWs can thus be disastrous for Arctic species that are losing large parts of their suitable habitats and might not be able to find and move to suitable colder areas to avoid extreme heat. Sessile species, such as benthic foundation species like kelp and seagrass, are likely more vulnerable to MHWs in contrast to mobile species (Smale et al., 2019), but in the Arctic, many of these sessile species are distributed at the cold end of their thermal range and evidence of MHWs impacts there remains limited. In addition, by affecting the stratification of the upper ocean, MHWs can influence nutrient and light availability for phytoplankton, thereby altering their community composition and size. MHWs can also cause shifts in the lipid content of zooplankton communities (from high- to low-lipid species). Changes in the planktonic system can have repercussions throughout Arctic and Subarctic food webs, and ultimately impact UTLs (Gillie et al., 2024). Fish, seabirds, and marine mammals in the Arctic and Subarctic regions exhibit varied responses to MHWs. Demographic responses through decline in body condition, increased mortality rates and recruitment failures were documented. Distributional shifts of UTL animals during and after MHWs were also observed. UTL animals may exhibit longer-term impacts (Renner et al., 2024) or delayed responses to MHWs (e.g., black-legged kittiwakes following the GoA MHW; Schoen et al., 2022), but current assessments focus mainly on short-term impacts, leaving a knowledge gap regarding the long-term or population-level effects of MHW events on UTL species.
Future research is needed to assess the comprehensive ecological impacts of MHWs, particularly how variations in their duration and frequency affect Arctic ecosystems. In addition, current observational studies often focus on short-term impacts. However, there is a critical need for long-term or population-level studies to understand the enduring effects of MHWs on the resilience of Arctic species. To investigate how MHWs duration influence the ecological responses of Arctic species, both field-based observations and laboratory-controlled experiments are needed (Joyce et al., 2024). Experimental studies are valuable for isolating the impacts of specific MHW characteristics (e.g., intensity, duration, frequency), and their combined effects, on marine species adaptation and resilience. Experimental settings could also be useful in distinguishing the ecological effects of MHWs from the impacts of long-term warming. Additionally, shifts in the planktonic Arctic ecosystem due to climate change are expected to significantly impact the functioning of both the carbon export and the biological carbon pump, affecting CO2 uptake in these waters (Manizza et al., 2010). Predictive models incorporating all these processes are needed to correctly represent the response of the ocean carbon cycle of Arctic regions in relationship with the potential carbon-climate feedback. More generally, as anthropogenic activities and their associated pressures continue to rise in the Arctic, it is essential to evaluate how MHWs interact with other climatic variables and human-induced stressors, and to understand their cumulative impact on marine biodiversity.
Currently, 15% of the world’s marine fish are caught in the Arctic and Subarctic, mostly in the southern Arctic inflow shelves, while the interior shelves and Central Arctic Ocean mainly support subsistence catches (Zeller et al., 2011; 2016; Mueter, 2022). Arctic fisheries, both commercial and subsistence, are undergoing significant changes in response to a northward shift in the distribution of commercial fish and shellfish species such as mackerel, cod, haddock, and capelin (Haug et al., 2017). Diminishing sea ice and warmer conditions gave opportunities for increasing fishing activities, especially trawling, in previously ice-covered Arctic shelf areas (Fauchald et al., 2021). The northward expansion of trawling is posing environmental concerns for these relatively commercially unexploited marine ecosystems. Arctic coastal states need to take stronger measures to regulate and manage the growing fishing pressure, to protect vulnerable Arctic shelf ecosystems from potential negative impacts (Christiansen et al., 2014; Fauchald et al., 2021; Mueter, 2022). In addition to fishing, other extractive human activities such as oil and gas exploration and extraction, and deep-sea mining, as well as shipping activities, might take advantage of the retreating sea ice to expand poleward in the future, increasing the risk of the vulnerable Arctic marine ecosystems to these cumulative pressures.
The summary for policymakers of the IPCC sixth report concludes “human activities, principally through emissions of greenhouse gases, have unequivocally caused global warming, with global surface temperature reaching 1.1°C above 1850–1900 in 2011–2020. Global greenhouse gas emissions have continued to increase, with unequal historical and ongoing contributions arising from unsustainable energy use, land use and land-use change, lifestyles and patterns of consumption and production across regions, between and within countries, and among individuals (high confidence)” (IPCC, 2023). As long as the greenhouse-gas emissions (GHG) from human industrial activities continue to rise, the ocean will keep getting warmer by absorbing the excess heat, and thus extreme heat events such as MHWs will occur. Attribution analysis indicates that GHG forcing is a necessary condition for the observed extreme MHWs in the Arctic, and there is a high probability that such intense MHWs would not have occurred if it were not for the GHG forcing (Barkhordarian et al., 2024). IPPC sixth report also states that “adaptation options that are feasible and effective today will become constrained and less effective with increasing global warming” (IPCC, 2023). While adapting and transforming our societies, governance and management systems are necessary to face the already existential threat of MHWs to Arctic ecosystems and livelihood, reducing global GHG emissions remains our best chance to safeguard a liveable planet for all and reduce human suffering and ecological collapse.
Author contributions
LP: Conceptualization, Project administration, Visualization, Writing–original draft, Writing–review and editing. BM: Conceptualization, Formal Analysis, Visualization, Writing–original draft, Writing–review and editing. AH: Conceptualization, Formal Analysis, Visualization, Writing–original draft, Writing–review and editing. AA-A: Writing–original draft, Writing–review and editing. JD: Writing–original draft, Writing–review and editing. KF-D: Writing–original draft, Writing–review and editing. KK: Writing–original draft, Writing–review and editing. KL: Writing–original draft, Writing–review and editing. MM: Writing–original draft, Writing–review and editing. CM: Writing–original draft, Writing–review and editing. PS: Writing–original draft, Writing–review and editing. MS: Writing–original draft, Writing–review and editing. TW: Writing–review and editing, Writing–original draft.
Funding
The author(s) declare that financial support was received for the research, authorship, and/or publication of this article. LP acknowledges financial support from the European Union’s Horizon Europe research and innovation program project “B-USEFUL” (ID:101059823) and “ACTNOW” (ID:101060072). BM and AA-A (University of Liège) acknowledge financial support from the STEREO-IV (Support To Exploitation and Research in Earth Observation) program administered by BELSPO (Belgian Science Policy Office) through the North-Heat project (STEREO-IV BELSPO # project SR/00/404). MM acknowledges financial support from the National Science Foundation (OPP-1922922) and from NASA (IDS19-0113).
Conflict of interest
The authors declare that the research was conducted in the absence of any commercial or financial relationships that could be construed as a potential conflict of interest.
Publisher’s note
All claims expressed in this article are solely those of the authors and do not necessarily represent those of their affiliated organizations, or those of the publisher, the editors and the reviewers. Any product that may be evaluated in this article, or claim that may be made by its manufacturer, is not guaranteed or endorsed by the publisher.
Supplementary material
The Supplementary Material for this article can be found online at: https://www.frontiersin.org/articles/10.3389/fenvs.2025.1473890/full#supplementary-material
References
Abelman, A., Dalton, M., Fissel, B., Garber-Yonts, B., Kasperski, S., Lee, J., et al. (2023). Stock assessment and fishery evaluation report for the groundfish fisheries of the Gulf of Alaska and Bering Sea/Aleutian Islands area.
Ainsworth, T. D., Hurd, C. L., Gates, R. D., and Boyd, P. W. (2020). How do we overcome abrupt degradation of marine ecosystems and meet the challenge of heat waves and climate extremes? Glob. Change Biol. 26, 343–354. doi:10.1111/gcb.14901
Alabia, I. D., García Molinos, J., Hirata, T., Mueter, F. J., Hirawake, T., and Saitoh, S.-I. (2021). Marine biodiversity refugia in a climate-sensitive subarctic shelf. Glob. Change Biol. 27, 3299–3311. doi:10.1111/gcb.15632
Albouy, C., Delattre, V., Donati, G., Frölicher, T. L., Albouy-Boyer, S., Rufino, M., et al. (2020). Global vulnerability of marine mammals to global warming. Sci. Rep. 10, 548. doi:10.1038/s41598-019-57280-3
Allen, C. R., Fontaine, J. J., Pope, K. L., and Garmestani, A. S. (2011). Adaptive management for a turbulent future. J. Environ. Manag. 92, 1339–1345. doi:10.1016/j.jenvman.2010.11.019
Almeida, L. Z., Laurel, B. J., Thalmann, H. L., and Miller, J. A. (2024). Warmer, earlier, faster: cumulative effects of Gulf of Alaska heatwaves on the early life history of Pacific cod. Elem. Sci. Anthropocene 12, 00050. doi:10.1525/elementa.2023.00050
Anderson, D. M., Fachon, E., Hubbard, K., Lefebvre, K. A., Lin, P., Pickart, R., et al. (2022). Harmful algal blooms in the Alaskan Arctic: an emerging threat as the ocean warms. Oceanography 35, 130–139. doi:10.5670/oceanog.2022.121
Ardyna, M., and Arrigo, K. R. (2020). Phytoplankton dynamics in a changing Arctic Ocean. Nat. Clim. Change 10 (10), 892–903. doi:10.1038/s41558-020-0905-y
Ardyna, M., Babin, M., Gosselin, M., Devred, E., Rainville, L., and Tremblay, J.-É. (2014). Recent Arctic Ocean sea ice loss triggers novel fall phytoplankton blooms. Geophys. Res. Lett. 41, 6207–6212. doi:10.1002/2014GL061047
Arimitsu, M. L., Piatt, J. F., Hatch, S., Suryan, R. M., Batten, S., Bishop, M. A., et al. (2021). Heatwave-induced synchrony within forage fish portfolio disrupts energy flow to top pelagic predators. Glob. Change Biol. 27, 1859–1878. doi:10.1111/gcb.15556
Arteaga, L. A., and Rousseaux, C. S. (2023). Impact of Pacific Ocean heatwaves on phytoplankton community composition. Commun. Biol. 6. doi:10.1038/s42003-023-04645-0
Attard, K., Singh, R. K., Gattuso, J.-P., Filbee-Dexter, K., Krause-Jensen, D., Kühl, M., et al. (2024). Seafloor primary production in a changing Arctic Ocean. Proc. Natl. Acad. Sci. 121, e2303366121. doi:10.1073/pnas.2303366121
Baker, M. R., Kivva, K. K., Pisareva, M. N., Watson, J. T., and Selivanova, J. (2020). Shifts in the physical environment in the Pacific Arctic and implications for ecological timing and conditions. Deep Sea Res. Part II Top. Stud. Oceanogr. 177, 104802. doi:10.1016/j.dsr2.2020.104802
Banzon, V., Smith, T. M., Steele, M., Huang, B., and Zhang, H.-M. (2020). Improved estimation of proxy sea surface temperature in the Arctic. J. Atmos. Ocean. Technol. 37, 341–349. doi:10.1175/JTECH-D-19-0177.1
Barbeaux, S. J., Holsman, K., and Zador, S. (2020). Marine heatwave stress test of ecosystem-based fisheries management in the Gulf of Alaska pacific cod fishery. Front. Mar. Sci. 7. doi:10.3389/fmars.2020.00703
Barkhordarian, A., Nielsen, D. M., Olonscheck, D., and Baehr, J. (2024). Arctic marine heatwaves forced by greenhouse gases and triggered by abrupt sea ice melt. Commun. Earth Environ. 5, 57–11. doi:10.1038/s43247-024-01215-y
Batten, S. D., Ostle, C., Hélaouët, P., and Walne, A. W. (2022). Responses of Gulf of Alaska plankton communities to a marine heat wave. Deep Sea Res. Part II Top. Stud. Oceanogr. 195, 105002. doi:10.1016/j.dsr2.2021.105002
Bellquist, L., Saccomanno, V., Semmens, B. X., Gleason, M., and Wilson, J. (2021). The rise in climate change-induced federal fishery disasters in the United States. PeerJ 9, e11186. doi:10.7717/peerj.11186
Benedetti-Cecchi, L., Bates, A. E., Strona, G., Bulleri, F., Horta e Costa, B., Edgar, G. J., et al. (2024). Marine protected areas promote stability of reef fish communities under climate warming. Nat. Commun. 15, 1822. doi:10.1038/s41467-024-44976-y
Biela, V. R. von, Arimitsu, M. L., Piatt, J. F., Heflin, B., Schoen, S. K., Trowbridge, J. L., et al. (2019). Extreme reduction in nutritional value of a key forage fish during the Pacific marine heatwave of 2014-2016. Mar. Ecol. Prog. Ser. 613, 171–182. doi:10.3354/meps12891
Bond, N. A., Cronin, M. F., Freeland, H., and Mantua, N. (2015). Causes and impacts of the 2014 warm anomaly in the NE Pacific. Geophys. Res. Lett. 42, 3414–3420. doi:10.1002/2015GL063306
Bonsell, C., and Dunton, K. H. (2018). Long-term patterns of benthic irradiance and kelp production in the central Beaufort sea reveal implications of warming for Arctic inner shelves. Prog. Oceanogr. 162, 160–170. doi:10.1016/j.pocean.2018.02.016
Borgman, E., Pedersen, M. F., Upadhyay, S., Anton, P., and Fischer-Bogason, R. (2022). Marine heatwaves in Northen Sea areas: occurrence, effects, and expected frequencies. PlanMiljø. Available at: https://www.miljodirektoratet.no/publikasjoner/2022/mars/marine-heatwaves-in-northern-sea-areas-occurrence-effects-and-expected-frequencies/ (Accessed July 23, 2024).
Breslow, S. J., Sojka, B., Barnea, R., Basurto, X., Carothers, C., Charnley, S., et al. (2016). Conceptualizing and operationalizing human wellbeing for ecosystem assessment and management. Environ. Sci. and Policy 66, 250–259. doi:10.1016/j.envsci.2016.06.023
Bringloe, T. T., Wilkinson, D. P., Goldsmit, J., Savoie, A. M., Filbee-Dexter, K., Macgregor, K. A., et al. (2022). Arctic marine forest distribution models showcase potentially severe habitat losses for cryophilic species under climate change. Glob. Change Biol. 28, 3711–3727. doi:10.1111/gcb.16142
Brodeur, R. D., Decker, M. B., Ciannelli, L., Purcell, J. E., Bond, N. A., Stabeno, P. J., et al. (2008). Rise and fall of jellyfish in the eastern Bering Sea in relation to climate regime shifts. Prog. Oceanogr. 77, 103–111. doi:10.1016/j.pocean.2008.03.017
Brodeur, R. D., Sugisaki, H., and Hunt Jr, G. L. (2002). Increases in jellyfish biomass in the Bering Sea: implications for the ecosystem. Mar. Ecol. Prog. Ser. 233, 89–103. doi:10.3354/meps233089
Burger, A. E., and Piatt, J. F. (1990). Flexible time budgets in breeding Common Murres: buffers against variable prey availability. Stud. Avian Biol. 14, 71–83.
CAFF/PAME (2022) “Status and trends for arctic conservation measures,” in Conservation of arctic flora and fauna and protection of the arctic marine environment. Akureyri, Iceland. Available at: https://pame.is/images/03_Projects/MPA/ST_ACM_2022.pdf.
Caputi, N., Kangas, M., Chandrapavan, A., Hart, A., Feng, M., Marin, M., et al. (2019). Factors affecting the recovery of invertebrate stocks from the 2011 Western Australian extreme marine heatwave. Front. Mar. Sci. 6. doi:10.3389/fmars.2019.00484
Caputi, N., Kangas, M., Denham, A., Feng, M., Pearce, A., Hetzel, Y., et al. (2016). Management adaptation of invertebrate fisheries to an extreme marine heat wave event at a global warming hot spot. Ecol. Evol. 6, 3583–3593. doi:10.1002/ece3.2137
Carmack, E., Polyakov, I., Padman, L., Fer, I., Hunke, E., Hutchings, J., et al. (2015). Toward quantifying the increasing role of oceanic heat in sea ice loss in the new Arctic. Bull. Am. Meteorological Soc. 96 (12), 2079–2105. doi:10.1175/bams-d-13-00177.1
Carothers, C., Black, J., Langdon, S. J., Donkersloot, R., Ringer, D., Coleman, J., et al. (2021). Indigenous peoples and salmon stewardship: a critical relationship. Ecol. Soc. 26, art16. doi:10.5751/es-11972-260116
Carton, J. A., Ding, Y., and Arrigo, K. R. (2015). The seasonal cycle of the Arctic Ocean under climate change. Geophys. Res. Lett. 42, 7681–7686. doi:10.1002/2015GL064514
Carvalho, K. S., Smith, T. E., and Wang, S. (2021). Bering Sea marine heatwaves: patterns, trends and connections with the Arctic. J. Hydrology 600, 126462. doi:10.1016/j.jhydrol.2021.126462
Castellani, G., Veyssière, G., Karcher, M., Stroeve, J., Banas, S. N., Bouman, A. H., et al. (2022). Shine a light: under-ice light and its ecological implications in a changing Arctic Ocean. Ambio 51, 307–317. doi:10.1007/s13280-021-01662-3
Cavole, L. M., Demko, A. M., Diner, R. E., Giddings, A., Koester, I., Pagniello, C. M. L. S., et al. (2016). Biological impacts of the 2013–2015 warm-water anomaly in the northeast Pacific: winners, losers, and the future. Oceanography 29, 273–285. doi:10.5670/oceanog.2016.32
Chambault, P., Tervo, O. M., Garde, E., Hansen, R. G., Blackwell, S. B., Williams, T. M., et al. (2020). The impact of rising sea temperatures on an Arctic top predator, the narwhal. Sci. Rep. 10, 18678. doi:10.1038/s41598-020-75658-6
Chen, K., Gawarkiewicz, G. G., Lentz, S. J., and Bane, J. M. (2014). Diagnosing the warming of the Northeastern U.S. Coastal Ocean in 2012: a linkage between the atmospheric jet stream variability and ocean response. J. Geophys. Res. Oceans 119, 218–227. doi:10.1002/2013JC009393
Cheung, W. W. L., and Frölicher, T. L. (2020). Marine heatwaves exacerbate climate change impacts for fisheries in the northeast Pacific. Sci. Rep. 10, 6678. doi:10.1038/s41598-020-63650-z
Cheung, W. W. L., Frölicher, T. L., Lam, V. W. Y., Oyinlola, M. A., Reygondeau, G., Sumaila, U. R., et al. (2021). Marine high temperature extremes amplify the impacts of climate change on fish and fisheries. Sci. Adv. 7, eabh0895. doi:10.1126/sciadv.abh0895
Christiansen, J. S., Mecklenburg, C. W., and Karamushko, O. V. (2014). Arctic marine fishes and their fisheries in light of global change. Glob. Change Biol. 20, 352–359. doi:10.1111/gcb.12395
Cline, T. J., Schindler, D. E., and Hilborn, R. (2017). Fisheries portfolio diversification and turnover buffer Alaskan fishing communities from abrupt resource and market changes. Nat. Commun. 8, 14042. doi:10.1038/ncomms14042
Cook, T. R., Martin, R., Roberts, J., Häkkinen, H., Botha, P., Meyer, C., et al. (2020). Parenting in a warming world: thermoregulatory responses to heat stress in an endangered seabird. Conserv. Physiol. 8, coz109. doi:10.1093/conphys/coz109
Copeman, L. A., Salant, C. D., Stowell, M. A., Spencer, M. L., Kimmel, D. G., Pinchuk, A. I., et al. (2022). Annual and spatial variation in the condition and lipid storage of juvenile Chukchi Sea gadids during a recent period of environmental warming (2012 to 2019). Deep Sea Res. Part II Top. Stud. Oceanogr. 205, 105180. doi:10.1016/j.dsr2.2022.105180
Dahlke, F. T., Wohlrab, S., Butzin, M., and Pörtner, H.-O. (2020). Thermal bottlenecks in the life cycle define climate vulnerability of fish. Science 369, 65–70. doi:10.1126/science.aaz3658
Decker, M. B., Brodeur, R. D., Ciannelli, L., Britt, L. L., Bond, N. A., DiFiore, B. P., et al. (2023). Cyclic variability of eastern Bering Sea jellyfish relates to regional physical conditions. Prog. Oceanogr. 210, 102923. doi:10.1016/j.pocean.2022.102923
Di Lorenzo, E., and Mantua, N. (2016). Multi-year persistence of the 2014/15 North Pacific marine heatwave. Nat. Clim. Change 6, 1042–1047. doi:10.1038/nclimate3082
Divoky, G. J., Brown, E., and Elliott, K. H. (2021). Reduced seasonal sea ice and increased sea surface temperature change prey and foraging behaviour in an ice-obligate Arctic seabird, Mandt’s black guillemot (Cepphus grylle mandtii). Polar Biol. 44, 701–715. doi:10.1007/s00300-021-02826-3
Duffy-Anderson, J. T., Stabeno, P., Andrews III, A. G., Cieciel, K., Deary, A., Farley, E., et al. (2019). Responses of the northern Bering Sea and southeastern Bering Sea pelagic ecosystems following record-breaking low winter Sea Ice. Geophys. Res. Lett. 46, 9833–9842. doi:10.1029/2019GL083396
Dutkiewicz, S., Morris, J. J., Follows, M. J., Scott, J., Levitan, O., Dyhrman, S. T., et al. (2015). Impact of ocean acidification on the structure of future phytoplankton communities. Nat. Clim. Change 5, 1002–1006. doi:10.1038/nclimate2722
Falardeau, M., Bennett, E. M., Else, B., Fisk, A., Mundy, C. J., Choy, E. S., et al. (2022). Biophysical indicators and Indigenous and Local Knowledge reveal climatic and ecological shifts with implications for Arctic Char fisheries. Glob. Environ. Change. 74, 102469. doi:10.1016/j.gloenvcha.2022.102469
Farley, E. V., Yasumiishi, E. M., Murphy, J. M., Strasburger, W., Sewall, F., Howard, K., et al. (2024). Critical periods in the marine life history of juvenile western Alaska chum salmon in a changing climate. Mar. Ecol. Prog. Ser. 726, 149–160. doi:10.3354/meps14491
Fauchald, P., Arneberg, P., Debernard, J. B., Lind, S., Olsen, E., and Hausner, V. H. (2021). Poleward shifts in marine fisheries under Arctic warming. Environ. Res. Lett. 16, 074057. doi:10.1088/1748-9326/ac1010
Filbee-Dexter, K., Wernberg, T., Fredriksen, S., Norderhaug, K. M., and Pedersen, M. F. (2019). Arctic kelp forests: diversity, resilience and future. Glob. Planet. Change 172, 1–14. doi:10.1016/j.gloplacha.2018.09.005
Filbee-Dexter, K., Wernberg, T., Grace, S. P., Thormar, J., Fredriksen, S., Narvaez, C. N., et al. (2020). Marine heatwaves and the collapse of marginal North Atlantic kelp forests. Sci. Rep. 10, 13388. doi:10.1038/s41598-020-70273-x
Fossheim, M., Primicerio, R., Johannesen, E., Ingvaldsen, R. B., Aschan, M. M., and Dolgov, A. V. (2015). Recent warming leads to a rapid borealization of fish communities in the Arctic. Nat. Clim. Change 5, 673–677. doi:10.1038/nclimate2647
Fredston, A. L., Cheung, W. W. L., Frölicher, T. L., Kitchel, Z. J., Maureaud, A. A., Thorson, J. T., et al. (2023). Marine heatwaves are not a dominant driver of change in demersal fishes. Nature 621, 324–329. doi:10.1038/s41586-023-06449-y
Free, C. M., Anderson, S. C., Hellmers, E. A., Muhling, B. A., Navarro, M. O., Richerson, K., et al. (2023). Impact of the 2014–2016 marine heatwave on US and Canada West Coast fisheries: surprises and lessons from key case studies. Fish Fish. 24, 652–674. doi:10.1111/faf.12753
Freedman, R. M., Brown, J. A., Caldow, C., and Caselle, J. E. (2020). Marine protected areas do not prevent marine heatwave-induced fish community structure changes in a temperate transition zone. Sci. Rep. 10, 21081. doi:10.1038/s41598-020-77885-3
Frölicher, T. L., Fischer, E. M., and Gruber, N. (2018). Marine heatwaves under global warming. Nature 560, 360–364. doi:10.1038/s41586-018-0383-9
Frölicher, T. L., and Laufkötter, C. (2018). Emerging risks from marine heat waves. Nat. Commun. 9, 650. doi:10.1038/s41467-018-03163-6
Gabriele, C. M., Amundson, C. L., Neilson, J. L., Straley, J. M., Baker, C. S., and Danielson, S. L. (2022). Sharp decline in humpback whale (Megaptera novaeangliae) survival and reproductive success in southeastern Alaska during and after the 2014–2016 Northeast Pacific marine heatwave. Mamm. Biol. 102, 1113–1131. doi:10.1007/s42991-021-00187-2
Garrabou, J., Gómez-Gras, D., Medrano, A., Cerrano, C., Ponti, M., Schlegel, R., et al. (2022). Marine heatwaves drive recurrent mass mortalities in the Mediterranean Sea. Glob. Change Biol. 28 (19), 5708–5725. doi:10.1111/gcb.16301
Gawarkiewicz, G., Chen, K., Forsyth, J., Bahr, F., Mercer, A. M., Ellertson, A., et al. (2019). Characteristics of an advective marine heatwave in the middle atlantic bight in early 2017. Front. Mar. Sci. 6. doi:10.3389/fmars.2019.00712
Gentemann, C. L., Fewings, M. R., and García-Reyes, M. (2017). Satellite sea surface temperatures along the West Coast of the United States during the 2014–2016 northeast Pacific marine heat wave. Geophys. Res. Lett. 44, 312–319. doi:10.1002/2016GL071039
Gillie, E. R., Bryndum-Buchholz, A., Willis, S. G., and Eddy, T. D. (2024). Exploring novel North Water Polynya ecosystems under climate change. PLOS Clim. 3 (10), e0000490. doi:10.1371/journal.pclm.0000490
Glencross, J. S., Lavers, J. L., and Woehler, E. J. (2021). Breeding success of short-tailed shearwaters following extreme environmental conditions. Mar. Ecol. Prog. Ser. 672, 193–203. doi:10.3354/meps13791
Golubeva, E., Kraineva, M., Platov, G., Iakshina, D., and Tarkhanova, M. (2021). Marine heatwaves in Siberian Arctic seas and adjacent region. Remote Sens. 13, 4436. doi:10.3390/rs13214436
Gradinger, R. R. (2001). Adaptation of Arctic and Antarctic ice metazoa to their habitat. Zoology 104, 339–345. doi:10.1078/0944-2006-00039
Grebmeier, J. M., Bluhm, B. A., Cooper, L. W., Danielson, S. L., Arrigo, K. R., Blanchard, A. L., et al. (2015). Ecosystem characteristics and processes facilitating persistent macrobenthic biomass hotspots and associated benthivory in the Pacific Arctic. Prog. Oceanogr. 136, 92–114. doi:10.1016/j.pocean.2015.05.006
Guibourd de Luzinais, V., Gascuel, D., Reygondeau, G., and Cheung, W. W. (2024). Large potential impacts of marine heatwaves on ecosystem functioning. Glob. Change Biol. 30 (7), e17437. doi:10.1111/gcb.17437
Gulland, F. M. D., Baker, J. D., Howe, M., LaBrecque, E., Leach, L., Moore, S. E., et al. (2022). A review of climate change effects on marine mammals in United States waters: past predictions, observed impacts, current research and conservation imperatives. Clim. Change Ecol. 3, 100054. doi:10.1016/j.ecochg.2022.100054
Hare, C. E., Leblanc, K., DiTullio, G. R., Kudela, R. M., Zhang, Y., Lee, P. A., et al. (2007). Consequences of increased temperature and CO2 for phytoplankton community structure in the Bering Sea. Mar. Ecol. Prog. Ser. 352, 9–16. doi:10.3354/meps07182
Hastings, K. K., Gelatt, T. S., Maniscalco, J. M., Jemison, L. A., Towell, R., Pendleton, G. W., et al. (2023). Reduced survival of Steller sea lions in the Gulf of Alaska following marine heatwave. Front. Mar. Sci. 10. doi:10.3389/fmars.2023.1127013
Haug, T., Bogstad, B., Chierici, M., Gjøsæter, H., Hallfredsson, E. H., Høines, Å. S., et al. (2017). Future harvest of living resources in the Arctic Ocean north of the Nordic and Barents Seas: a review of possibilities and constraints. Fish. Res. 188, 38–57. doi:10.1016/j.fishres.2016.12.002
Hauri, C., Pagès, R., Hedstrom, K., Doney, S. C., Dupont, S., Ferriss, B., et al. (2024). More than marine heatwaves: a new regime of heat, acidity, and low oxygen compound extreme events in the Gulf of Alaska. AGU Adv. 5, e2023AV001039. doi:10.1029/2023AV001039
Hayashida, H., Matear, R. J., and Strutton, P. G. (2020). Background nutrient concentration determines phytoplankton bloom response to marine heatwaves. Glob. Change Biol. 26, 4800–4811. doi:10.1111/gcb.15255
Henson, S. A., Cael, B. B., Allen, S. R., and Dutkiewicz, S. (2021). Future phytoplankton diversity in a changing climate. Nat. Commun. 12 (1), 5372. doi:10.1038/s41467-021-25699-w
Hobday, A. J., Alexander, L. V., Perkins, S. E., Smale, D. A., Straub, S. C., Oliver, E. C. J., et al. (2016). A hierarchical approach to defining marine heatwaves. Prog. Oceanogr. 141, 227–238. doi:10.1016/j.pocean.2015.12.014
Hobday, A. J., Oliver, E. C. J., Gupta, A. S., Benthuysen, J. A., Burrows, M. T., Donat, M., et al. (2018). Categorizing and naming marine heatwaves. Oceanography 31, 162–173. doi:10.5670/oceanog.2018.205
Holbrook, N. J., Sen Gupta, A., Oliver, E. C. J., Hobday, A. J., Benthuysen, J. A., Scannell, H. A., et al. (2020). Keeping pace with marine heatwaves. Nat. Rev. Earth Environ. 1, 482–493. doi:10.1038/s43017-020-0068-4
Holsman, K. K., Hazen, E. L., Haynie, A., Gourguet, S., Hollowed, A., Bograd, S. J., et al. (2019). Towards climate resiliency in fisheries management. ICES J. Mar. Sci. 76, 1368–1378. doi:10.1093/icesjms/fsz031
Hoppe, C. J. M., Wolf, K. K. E., Schuback, N., Tortell, P. D., and Rost, B. (2018). Compensation of ocean acidification effects in Arctic phytoplankton assemblages. Nat. Clim. Change 8, 529–533. doi:10.1038/s41558-018-0142-9
Hu, S., Zhang, L., and Qian, S. (2020). Marine heatwaves in the arctic region: variation in different ice covers. Geophys. Res. Lett. 47, e2020GL089329. doi:10.1029/2020GL089329
Huang, B., Wang, Z., Yin, X., Arguez, A., Graham, G., Liu, C., et al. (2021). Prolonged marine heatwaves in the arctic: 1982−2020. Geophys. Res. Lett. 48, e2021GL095590. doi:10.1029/2021GL095590
Hughes, T. P., Kerry, J. T., Álvarez-Noriega, M., Álvarez-Romero, J. G., Anderson, K. D., Baird, A. H., et al. (2017). Global warming and recurrent mass bleaching of corals. Nature 543, 373–377. doi:10.1038/nature21707
Huntington, H. P., Danielson, S. L., Wiese, F. K., Baker, M., Boveng, P., Citta, J. J., et al. (2020). Evidence suggests potential transformation of the Pacific Arctic ecosystem is underway. Nat. Clim. Chang. 10, 342–348. doi:10.1038/s41558-020-0695-2
Husson, B., Bluhm, B. A., Cyr, F., Danielson, S. L., Eriksen, E., Fossheim, M., et al. (2024). Borealization impacts shelf ecosystems across the Arctic. Front. Environ. Sci. 12, 1481420. doi:10.3389/fenvs.2024.1481420
Husson, B., Lind, S., Fossheim, M., Kato-Solvang, H., Skern-Mauritzen, M., Pécuchet, L., et al. (2022). Successive extreme climatic events lead to immediate, large-scale, and diverse responses from fish in the Arctic. Glob. Change Biol. 28, 3728–3744. doi:10.1111/gcb.16153
IPCC (2023). “Summary for policymakers,” in Climate change 2023: synthesis report. Contribution of working groups I, II and III to the sixth assessment report of the intergovernmental panel on climate change. (Core writing team). Editors H. Lee, and J. Romero (Geneva, Switzerland: IPCC), 1–34.
Iverson, S. (2015). Quantifying the demographic and population impact of avian cholera on northern common eiders in the face of ancillary threats and changing environmental circumstances.
Jacox, M. G., Alexander, M. A., Bograd, S. J., and Scott, J. D. (2020). Thermal displacement by marine heatwaves. Nature 584, 82–86. doi:10.1038/s41586-020-2534-z
Jahn, A., Holland, M. M., and Kay, J. E. (2024). Projections of an ice-free Arctic Ocean. Nat. Rev. Earth Environ. 5, 164–176. doi:10.1038/s43017-023-00515-9
Johns, M. E., Warzybok, P., Jahncke, J., Doak, P., Lindberg, M., and Breed, G. A. (2022). Episodes of high recruitment buffer against climate-driven mass mortality events in a North Pacific seabird population. J.Anim. Ecol. 91, 345–355. doi:10.1111/1365-2656.13630
Jones, T., Divine, L. M., Renner, H., Knowles, S., Lefebvre, K. A., Burgess, H. K., et al. (2019). Unusual mortality of tufted puffins (Fratercula cirrhata) in the eastern Bering Sea. PLoS ONE 14, e0216532. doi:10.1371/journal.pone.0216532
Jones, T., Parrish, J. K., Lindsey, J., Wright, C., Burgess, H. K., Dolliver, J., et al. (2023). Marine bird mass mortality events as an indicator of the impacts of ocean warming. Mar. Ecol. Progr. Ser. 737, 161–181. doi:10.3354/meps14330
Jordà-Molina, È., Renaud, P. E., Silberberger, M. J., Sen, A., Bluhm, B. A., Carroll, M. L., et al. (2023). Seafloor warm water temperature anomalies impact benthic macrofauna communities of a high-Arctic cold-water fjord. Mar. Environ. Res. 189, 106046. doi:10.1016/j.marenvres.2023.106046
Joyce, P. W., Tong, C. B., Yip, Y. L., and Falkenberg, L. J. (2024). Marine heatwaves as drivers of biological and ecological change: implications of current research patterns and future opportunities. Mar. Biol. 171 (1), 20. doi:10.1007/s00227-023-04340-y
Kahru, M., Brotas, V., Manzano-Sarabia, M., and Mitchell, B. G. (2011). Are phytoplankton blooms occurring earlier in the Arctic? Glob. Change Biol. 17, 1733–1739. doi:10.1111/j.1365-2486.2010.02312.x
Kaler, R. S. A., and Kuletz, K. (2022). Alaskan seabird die-offs. Oceanography. doi:10.5670/oceanog.2022.118
Kimmel, D. G., Eisner, L. B., and Pinchuk, A. I. (2023). The northern Bering Sea zooplankton community response to variability in sea ice: evidence from a series of warm and cold periods. Mar. Ecol. Prog. Ser. 705, 21–42. doi:10.3354/meps14237
Kourantidou, M., Jin, D., and Schumacker, E. J. (2022). Socioeconomic disruptions of harmful algal blooms in indigenous communities: the case of Quinault Indian nation. Harmful Algae 118, 102316. doi:10.1016/j.hal.2022.102316
Krause-Jensen, D., Archambault, P., Assis, J., Bartsch, I., Bischof, K., Filbee-Dexter, K., et al. (2020). Imprint of climate change on pan-arctic marine vegetation. Front. Mar. Sci. 7. doi:10.3389/fmars.2020.617324
Krause-Jensen, D., Sejr, M. K., Bruhn, A., Rasmussen, M. B., Christensen, P. B., Hansen, J. L. S., et al. (2019). Deep penetration of kelps offshore along the west coast of Greenland. Front. Mar. Sci. 6. doi:10.3389/fmars.2019.00375
Kuletz, K. J., Cushing, D., and Labunski, E. (2020). Distributional shifts among seabird communities of the Northern Bering and Chukchi seas in response to ocean warming during 2017–2019. Deep Sea Res. II 181–182, 104913. doi:10.1016/j.dsr2.2020.104913
Kuletz, K. J., Ferguson, S. H., Frederiksen, M., Gallagher, C. P., Hauser, D. D. W., Hop, H., et al. (2024a). A review of climate change impacts on migration patterns of marine vertebrates in Arctic and Subarctic ecosystems. Front. Environ. Sci. 2, 1434549. doi:10.3389/fenvs.2024.1434549
Kuletz, K. J., Gall, A. E., Morgan, T. C., Prichard, A. K., Eisner, L. B., Kimmel, D. G., et al. (2024b). Seabird responses to ecosystem changes driven by marine heatwaves in a warming Arctic. Mar. Ecol. Progr. Ser. 737, 59–88. doi:10.3354/meps14493
Latorre, M. P., Iachetti, C. M., Schloss, I. R., Antoni, J., Malits, A., de la Rosa, F., et al. (2023). Summer heatwaves affect coastal Antarctic plankton metabolism and community structure. J. Exp. Mar. Biol. Ecol. 567, 151926. doi:10.1016/j.jembe.2023.151926
Laufkötter, C., Zscheischler, J., and Frölicher, T. L. (2020). High-impact marine heatwaves attributable to human-induced global warming. Science 369, 1621–1625. doi:10.1126/science.aba0690
Laurel, B. J., and Rogers, L. A. (2020). Loss of spawning habitat and prerecruits of Pacific cod during a Gulf of Alaska heatwave. Can. J. Fish. Aquat. Sci. 77, 644–650. doi:10.1139/cjfas-2019-0238
Laurel, B. J., Spencer, M., Iseri, P., and Copeman, L. A. (2016). Temperature-dependent growth and behavior of juvenile Arctic cod (Boreogadus saida) and co-occurring North Pacific gadids. Polar Biol. 39, 1127–1135. doi:10.1007/s00300-015-1761-5
Lavaniegos, B. E., Jiménez-Herrera, M., and Ambriz-Arreola, I. (2019). Unusually low euphausiid biomass during the warm years of 2014–2016 in the transition zone of the California Current. Deep Sea Res. Part II Top. Stud. Oceanogr. 169–170, 104638. doi:10.1016/j.dsr2.2019.104638
Lavoie, C., Howland, K. L., Filbee-Dexter, K., Massicotte, P., Goldsmit, J., McKindsey, C. W., et al. (2024). Living under Arctic kelp forests: linking soft-bottom communities to kelp cover in the Canadian Arctic. Mar. Ecol. Prog. Ser. 740, 1–22. doi:10.3354/meps14628
Levine, R. M., De Robertis, A., Grünbaum, D., Wildes, S., Farley, E. V., Stabeno, P. J., et al. (2023). Climate-driven shifts in pelagic fish distributions in a rapidly changing Pacific Arctic. Deep Sea Res. Part II Top. Stud. Oceanogr. 208, 105244. doi:10.1016/j.dsr2.2022.105244
Li, W. K. W., McLaughlin, F. A., Lovejoy, C., and Carmack, E. C. (2009). Smallest algae thrive as the Arctic Ocean freshens. Science 326, 539. doi:10.1126/science.1179798
Manizza, M., Buitenhuis, E. T., and Le Quéré, C. (2010). Sensitivity of global ocean biogeochemical dynamics to ecosystem structure in a future climate. Geophys. Res. Lett. 37. doi:10.1029/2010GL043360
Manizza, M., Carroll, D., Menemenlis, D., Zhang, H., and Miller, C. E. (2023). Modeling the recent changes of phytoplankton blooms dynamics in the Arctic Ocean. J. Geophys. Res. Oceans 128, e2022JC019152. doi:10.1029/2022JC019152
Marbà, N., Jordà, G., Bennett, S., and Duarte, C. M. (2022). Seagrass thermal limits and vulnerability to future warming. Front. Mar. Sci. 9. doi:10.3389/fmars.2022.860826
Margalef, R. (1978). Life-forms of phytoplankton as survival alternatives in an unstable environment. Oceanol. acta 1, 493–509.
Meredith, M., Sommerkorn, M., Cassota, S., Derksen, C., Ekaykin, A., Hollowed, A., et al. (2019). Polar regions. University of Tasmania. Available at: https://figshare.utas.edu.au/articles/chapter/Polar_Regions/23122904/1 (Accessed July 23, 2024).
Mohamed, B., Barth, A., and Alvera-Azcárate, A. (2023). Extreme marine heatwaves and cold-spells events in the Southern North Sea: classifications, patterns, and trends. Front. Mar. Sci. 10, 1258117. doi:10.3389/FMARS.2023.1258117
Mohamed, B., Nilsen, F., and Skogseth, R. (2022a). Interannual and decadal variability of sea surface temperature and Sea Ice concentration in the Barents Sea. Remote Sens. 14, 4413. doi:10.3390/rs14174413
Mohamed, B., Nilsen, F., and Skogseth, R. (2022b). Marine heatwaves characteristics in the Barents Sea based on high resolution satellite data (1982–2020). Front. Mar. Sci. 9. doi:10.3389/fmars.2022.821646
Møller, E. F., and Nielsen, T. G. (2020). Borealization of Arctic zooplankton—smaller and less fat zooplankton species in Disko Bay, Western Greenland. Limnol. Oceanogr. 65, 1175–1188. doi:10.1002/lno.11380
Montevecchi, W. A., Regular, P. M., Rail, J.-F., Power, K., Mooney, C., D’entremont, K. J. N., et al. (2021). Ocean heat wave induces breeding failure at the southern breeding limit of the Northern Gannet Morus bassanus. Mar. Ornithol. 49, 71–78.
Mueter, F. J. (2022). “Arctic fisheries in a changing climate,” in Global arctic: an introduction to the multifaceted dynamics of the arctic. Editors, M. Finger,, and G. Rekvig (Cham: Springer International Publishing), 279–295. doi:10.1007/978-3-030-81253-9_14
Mueter, F. J., Iken, K., Cooper, L. W., Grebmeier, J. M., Kuletz, K. J., Hopcroft, R. R., et al. (2021). Changes in diversity and species composition across multiple assemblages in the eastern Chukchi Sea during two contrasting years are consistent with borealization. Oceanography 34, 38–51. doi:10.5670/oceanog.2021.213
Mueter, F. J., and Litzow, M. A. (2008). Sea ice retreat alters the biogeography of the Bering Sea continental shelf. Ecol. Appl. 18, 309–320. doi:10.1890/07-0564.1
Naves, L. C. (2018). Geographic and seasonal patterns of seabird subsistence harvest in Alaska. Polar Biol. 41, 1217–1236. doi:10.1007/s00300-018-2279-4
Neeley, A. R., Harris, L. A., and Frey, K. E. (2018). Unraveling phytoplankton community dynamics in the northern Chukchi Sea under sea ice-covered and sea ice-free conditions. Geophys. Res. Lett. 45, 7663–7671. doi:10.1029/2018GL077684
Neukermans, G., Oziel, L., and Babin, M. (2018). Increased intrusion of warming Atlantic water leads to rapid expansion of temperate phytoplankton in the Arctic. Glob. Change Biol. 24, 2545–2553. doi:10.1111/gcb.14075
Niedzwiedz, S., Vonnahme, T. R., Juul-Pedersen, T., Bischof, K., and Diehl, N. (2024). Light-mediated temperature susceptibility of kelp species (Agarum clathratum, Saccharina latissima) in an Arctic summer heatwave scenario. Camb. Prisms Coast. Futur. 2, e6. doi:10.1017/cft.2024.5
Nielsen, J. M., Sigler, M. F., Eisner, L. B., Watson, J. T., Rogers, L. A., Bell, S. W., et al. (2024). Spring phytoplankton bloom phenology during recent climate warming on the Bering Sea shelf. Prog. Oceanogr. 220, 103176. doi:10.1016/j.pocean.2023.103176
Noh, K. M., Lim, H.-G., and Kug, J.-S. (2022). Global chlorophyll responses to marine heatwaves in satellite ocean color. Environ. Res. Lett. 17, 064034. doi:10.1088/1748-9326/ac70ec
Notz, D., and Community, S. (2020). Arctic Sea ice in CMIP6. Geophys. Res. Lett. 47, e2019GL086749. doi:10.1029/2019GL086749
Oliver, E. C. J., Benthuysen, J. A., Darmaraki, S., Donat, M. G., Hobday, A. J., Holbrook, N. J., et al. (2021). Marine heatwaves. Annu. Rev. Mar. Sci. 13, 313–342. doi:10.1146/annurev-marine-032720-095144
Oliver, E. C. J., Donat, M. G., Burrows, M. T., Moore, P. J., Smale, D. A., Alexander, L. V., et al. (2018). Longer and more frequent marine heatwaves over the past century. Nat. Commun. 9, 1324. doi:10.1038/s41467-018-03732-9
Orgeret, F., Thiebault, A., Kovacs, K. M., Lydersen, C., Hindell, M. A., Thompson, S. A., et al. (2022). Climate change impacts on seabirds and marine mammals: the importance of study duration, thermal tolerance and generation time. Ecol. Lett. 25, 218–239. doi:10.1111/ele.13920
Osborne, O. E., Ohara, P. D., Whelan, S., Zandbergen, P., Hatch, S. A., and Elliott, K. H. (2020). Breeding seabirds increase foraging range in response to an extreme marine heatwave. Mar. Ecol. Prog. Ser. 646, 161–173. doi:10.3354/meps13392
Oziel, L., Neukermans, G., Ardyna, M., Lancelot, C., Tison, J.-L., Wassmann, P., et al. (2017). Role for Atlantic inflows and sea ice loss on shifting phytoplankton blooms in the Barents Sea. J. Geophys. Res. Oceans 122, 5121–5139. doi:10.1002/2016JC012582
Pearce, A. F., and Feng, M. (2013). The rise and fall of the “marine heat wave” off Western Australia during the summer of 2010/2011. J. Mar. Syst. 111 (112), 139–156. doi:10.1016/j.jmarsys.2012.10.009
Pecl, G. T., Araújo, M. B., Bell, J. D., Blanchard, J., Bonebrake, T. C., Chen, I.-C., et al. (2017). Biodiversity redistribution under climate change: impacts on ecosystems and human well-being. Science 355, eaai9214. doi:10.1126/science.aai9214
Pershing, A. J., Mills, K. E., Dayton, A. M., Franklin, B. S., and Kennedy, B. T. (2018). Evidence for adaptation from the 2016 marine heatwave in the northwest atlantic ocean. Oceanography 31, 152–161. doi:10.5670/oceanog.2018.213
Peterson, W. T., Fisher, J. L., Strub, P. T., Du, X., Risien, C., Peterson, J., et al. (2017). The pelagic ecosystem in the Northern California Current off Oregon during the 2014–2016 warm anomalies within the context of the past 20 years. J. Geophys. Res. Oceans 122, 7267–7290. doi:10.1002/2017JC012952
Peterson Williams, M. J., Robbins Gisclair, B., Cerny-Chipman, E., LeVine, M., and Peterson, T. (2022). The heat is on: Gulf of Alaska Pacific cod and climate-ready fisheries. ICES J. Mar. Sci. 79, 573–583. doi:10.1093/icesjms/fsab032
Piatt, J. F., Parrish, J. K., Renner, H. M., Schoen, S. K., Jones, T. T., Arimitsu, M. L., et al. (2020). Extreme mortality and reproductive failure of common murres resulting from the northeast Pacific marine heatwave of 2014-2016. Plos One 15, e0226087. doi:10.1371/journal.pone.0226087
Plecha, S. M., and Soares, P. M. M. (2020). Global marine heatwave events using the new CMIP6 multi-model ensemble: from shortcomings in present climate to future projections. Environ. Res. Lett. 15, 124058. doi:10.1088/1748-9326/abc847
Polyakov, I. V., Alkire, M. B., Bluhm, B. A., Brown, K. A., Carmack, E. C., Chierici, M., et al. (2020). Borealization of the Arctic Ocean in response to anomalous advection from sub-arctic seas. Front. Mar. Sci. 7. doi:10.3389/fmars.2020.00491
Queirós, A. M., Talbot, E., Beaumont, N. J., Somerfield, P. J., Kay, S., Pascoe, C., et al. (2021). Bright spots as climate-smart marine spatial planning tools for conservation and blue growth. Glob. Change Biol. 27, 5514–5531. doi:10.1111/gcb.15827
Rantanen, M., Karpechko, A. Y., Lipponen, A., Nordling, K., Hyvärinen, O., Ruosteenoja, K., et al. (2022). The Arctic has warmed nearly four times faster than the globe since 1979. Commun. Earth Environ. 3, 168. doi:10.1038/s43247-022-00498-3
Ren, X., and Liu, W. (2021). The role of a weakened atlantic meridional overturning circulation in modulating marine heatwaves in a warming climate. Geophys. Res. Lett. 48, e2021GL095941. doi:10.1029/2021GL095941
Renner, H. M., Piatt, J. F., Renner, M., Drummond, B. A., Laufenberg, J. S., and Parrish, J. K. (2024). Catastrophic and persistent loss of common murres after a marine heatwave. Science 386 (6727), 1272–1276. doi:10.1126/science.adq4330
Reynolds, R. W., Smith, T. M., Liu, C., Chelton, D. B., Casey, K. S., and Schlax, M. G. (2007). Daily high-resolution-blended analyses for sea surface temperature. J. Clim. 20, 5473–5496. doi:10.1175/2007JCLI1824.1
Richaud, B., Hu, X., Darmaraki, S., Fennel, K., Lu, Y., and Oliver, E. C. J. (2024). Drivers of marine heatwaves in the Arctic Ocean. J. Geophys. Res. Oceans 129, e2023JC020324. doi:10.1029/2023JC020324
Roberts, S. D., Van Ruth, P. D., Wilkinson, C., Bastianello, S. S., and Bansemer, M. S. (2019). Marine heatwave, harmful algae blooms and an extensive fish kill event during 2013 in south Australia. Front. Mar. Sci. 6. doi:10.3389/fmars.2019.00610
Robinson, C. L. K., Bertram, D. F., Shannon, H., Biela, V. R. von, Greentree, W., Duguid, W., et al. (2023). Reduction in overwinter body condition and size of Pacific sand lance has implications for piscivorous predators during marine heatwaves. Mar. Ecol. Prog. Ser. Heat 737, 89–99. doi:10.3354/meps14257
Robinson, C. L. K., Yakimishyn, J., and Evans, R. (2022). Minimal effects of the 2014-16 marine heatwave on fish assemblages found in eelgrass meadows on the southwestern coast of Vancouver Island, British Columbia, Canada. Front. Mar. Sci. 9. doi:10.3389/fmars.2022.980703
Rogers, L. A., Wilson, M. T., Duffy-Anderson, J. T., Kimmel, D. G., and Lamb, J. F. (2021). Pollock and “the Blob”: impacts of a marine heatwave on walleye pollock early life stages. Fish. Oceanogr. 30, 142–158. doi:10.1111/fog.12508
Roleda, M. Y. (2016). Stress physiology and reproductive phenology of Arctic endemic kelp Laminaria solidungula J. Agardh. Polar Biol. 39, 1967–1977. doi:10.1007/s00300-015-1813-x
Romano, M. D., Renner, H. M., Kuletz, K. J., Parrish, J. K., Jones, T., Burgess, H. K., et al. (2020). Die–offs, reproductive failure, and changing at–sea abundance of murres in the Bering and Chukchi Seas in 2018. Deep Sea Res. Part II Top. Stud. Oceanogr. 181–182, 104877. doi:10.1016/j.dsr2.2020.104877
Sala, E., and Giakoumi, S. (2018). No-take marine reserves are the most effective protected areas in the ocean. ICES J. Mar. Sci. 75, 1166–1168. doi:10.1093/icesjms/fsx059
Santora, J. A., Mantua, N. J., Schroeder, I. D., Field, J. C., Hazen, E. L., Bograd, S. J., et al. (2020). Habitat compression and ecosystem shifts as potential links between marine heatwave and record whale entanglements. Nat. Commun. 11 (1), 536. doi:10.1038/s41467-019-14215-w
Schoen, S. K., Arimitsu, M. L., Marsteller, C. E., and Piatt, J. F. (2022). Lingering impacts of the 2014-2016 northeast Pacific marine heatwave on seabird demography in Cook Inlet, Alaska (USA). Mar. Ecol. Prog. Ser. Heat 737, 121–136. doi:10.3354/meps14177
Screen, J. A., and Simmonds, I. (2010). The central role of diminishing sea ice in recent Arctic temperature amplification. Nature 464, 1334–1337. doi:10.1038/nature09051
Sen, P. K. (1968). Estimates of the regression coefficient based on Kendall’s tau. J. Am. Stat. Assoc. 63 (324), 1379–1389.
Sen Gupta, A., Thomsen, M., Benthuysen, J. A., Hobday, A. J., Oliver, E., Alexander, L. V., et al. (2020). Drivers and impacts of the most extreme marine heatwave events. Sci. Rep. 10, 19359. doi:10.1038/s41598-020-75445-3
Shu, Q., Wang, Q., Årthun, M., Wang, S., Song, Z., Zhang, M., et al. (2022). Arctic Ocean Amplification in a warming climate in CMIP6 models. Sci. Adv. 8, eabn9755. doi:10.1126/sciadv.abn9755
Siddon, E. C., Zador, S. G., and Hunt, G. L. (2020). Ecological responses to climate perturbations and minimal sea ice in the northern Bering Sea. Deep Sea Res. Part II Top. Stud. Oceanogr. 181–182, 104914. doi:10.1016/j.dsr2.2020.104914
Sinclair, E. H., Vlietstra, L. S., Johnson, D. S., Zeppelin, T. K., Byrd, G. V., Springer, A. M., et al. (2008). Patterns in prey use among fur seals and seabirds in the Pribilof Islands. Deep Sea Res. Part II Top. Stud. Oceanogr. 55, 1897–1918. doi:10.1016/j.dsr2.2008.04.031
Skagseth, Ø., Eldevik, T., Årthun, M., Asbjørnsen, H., Lien, V. S., and Smedsrud, L. H. (2020). Reduced efficiency of the Barents Sea cooling machine. Nat. Clim. Chang. 10, 661–666. doi:10.1038/s41558-020-0772-6
Smale, D. A., Wernberg, T., Oliver, E. C. J., Thomsen, M., Harvey, B. P., Straub, S. C., et al. (2019). Marine heatwaves threaten global biodiversity and the provision of ecosystem services. Nat. Clim. Chang. 9, 306–312. doi:10.1038/s41558-019-0412-1
Smith, J. G., Free, C. M., Lopazanski, C., Brun, J., Anderson, C. R., Carr, M. H., et al. (2023). A marine protected area network does not confer community structure resilience to a marine heatwave across coastal ecosystems. Glob. Change Biol. 29, 5634–5651. doi:10.1111/gcb.16862
Smith, K. E., Burrows, M. T., Hobday, A. J., King, N. G., Moore, P. J., Sen Gupta, A., et al. (2023). Biological impacts of marine heatwaves. Annu. Rev. Mar. Sci. 15, 119–145. doi:10.1146/annurev-marine-032122-121437
Smith, K. E., Burrows, M. T., Hobday, A. J., Sen Gupta, A., Moore, P. J., Thomsen, M., et al. (2021). Socioeconomic impacts of marine heatwaves: global issues and opportunities. Science 374, eabj3593. doi:10.1126/science.abj3593
Staehr, P. A., and Borum, J. (2011). Seasonal acclimation in metabolism reduces light requirements of eelgrass (Zostera marina). J. Exp. Mar. Biol. Ecol. 407, 139–146. doi:10.1016/j.jembe.2011.05.031
Staehr, P. A., and Wernberg, T. (2009). Physiological responses of ecklonia radiata (laminariales) to a latitudinal gradient in ocean temperature. J. Phycol. 45, 91–99. doi:10.1111/j.1529-8817.2008.00635.x
Stafford, K. M., Farley, E. V., Ferguson, M., Kuletz, K. J., and Levine, R. (2022). Northward range expansion of subarctic upper trophic level animals into the Pacific Arctic region. Oceanography 35, 158–166. doi:10.5670/oceanog.2022.101
Stevenson, D. E., and Lauth, R. R. (2019). Bottom trawl surveys in the northern Bering Sea indicate recent shifts in the distribution of marine species. Polar Biol. 42, 407–421. doi:10.1007/s00300-018-2431-1
Stroeve, J. C., Serreze, M. C., Holland, M. M., Kay, J. E., Malanik, J., and Barrett, A. P. (2012). The Arctic’s rapidly shrinking sea ice cover: a research synthesis. Clim. Change 110, 1005–1027. doi:10.1007/s10584-011-0101-1
Strom, S. (2023). Recent marine heatwaves affect marine ecosystems from plankton to seabirds in the northern Gulf of Alaska. Oceanography 36, 31–33. doi:10.5670/oceanog.2023.s1.9
Strydom, S., Murray, K., Wilson, S., Huntley, B., Rule, M., Heithaus, M., et al. (2020). Too hot to handle: unprecedented seagrass death driven by marine heatwave in a World Heritage Area. Glob. Change Biol. 26, 3525–3538. doi:10.1111/gcb.15065
Suryan, R. M., Arimitsu, M. L., Coletti, H. A., Hopcroft, R. R., Lindeberg, M. R., Barbeaux, S. J., et al. (2021). Ecosystem response persists after a prolonged marine heatwave. Sci. Rep. 11, 6235. doi:10.1038/s41598-021-83818-5
Szuwalski, C. S., Aydin, K., Fedewa, E. J., Garber-Yonts, B., and Litzow, M. A. (2023). The collapse of eastern Bering Sea snow crab. Science 382, 306–310. doi:10.1126/science.adf6035
Szymkowiak, M., and Rhodes-Reese, M. (2020). Adaptive behaviors to marine ecosystem shifts: examining fishermen’s strategies in response to abundant juvenile sablefish (Anoplopoma fimbria) in Alaska. Front. Mar. Sci. 7. doi:10.3389/fmars.2020.602281
Szymkowiak, M., and Steinkruger, A. (2023). Alaska Fishers attest to climate change impacts in discourse on resource management under marine heatwaves. Environ. Sci. and Policy 140, 261–270. doi:10.1016/j.envsci.2022.12.019
Szymkowiak, M., Steinkruger, A., and Hayes, A. L. (2024). Alaska’s emergent fisheries processes. Ocean and Coast. Manag. 249, 107004. doi:10.1016/j.ocecoaman.2023.107004
Thomson, J. A., Burkholder, D. A., Heithaus, M. R., Fourqurean, J. W., Fraser, M. W., Statton, J., et al. (2015). Extreme temperatures, foundation species, and abrupt ecosystem change: an example from an iconic seagrass ecosystem. Glob. Change Biol. 21, 1463–1474. doi:10.1111/gcb.12694
Valsecchi, E., Arcangeli, A., Lombardi, R., Boyse, E., Carr, I. M., Galli, P., et al. (2021). Ferries and environmental DNA: underway sampling from commercial vessels provides new opportunities for systematic genetic surveys of marine biodiversity. Front. Mar. Sci. 8. doi:10.3389/fmars.2021.704786
Van Hemert, C., Dusek, R. J., Smith, M. M., Kaler, R., Sheffield, G., Divine, L. M., et al. (2020). Investigation of algal toxins in a multispecies seabird die-off in the bering and Chukchi seas. J. Wildl. Dis. 57, 399–407. doi:10.7589/JWD-D-20-00057
Vergés, A., Steinberg, P. D., Hay, M. E., Poore, A. G. B., Campbell, A. H., Ballesteros, E., et al. (2014). The tropicalization of temperate marine ecosystems: climate-mediated changes in herbivory and community phase shifts. Proc. R. Soc. B Biol. Sci. 281, 20140846. doi:10.1098/rspb.2014.0846
von Biela, V. R., Laske, S. M., Stanek, A. E., Brown, R. J., and Dunton, K. H. (2023). Borealization of nearshore fishes on an interior Arctic shelf over multiple decades. Glob. Change Biol. 29, 1822–1838. doi:10.1111/gcb.16576
Walsh, J. E., Thoman, R. L., Bhatt, U. S., Bieniek, P. A., Brettschneider, B., Brubaker, M., et al. (2018). The high latitude marine heat wave of 2016 and its impacts on Alaska. Bull. Am. Meteorol. Soc. 99, S39–S43. doi:10.1175/bams-d-17-0105.1
Wanless, S., Albon, S. D., Daunt, F., Sarzo, B., Newell, M. A., Gunn, C., et al. (2023). Increased parental effort fails to buffer the cascading effects of warmer seas on common guillemot demographic rates. J. Animal Ecol. 92, 1622–1638. doi:10.1111/1365-2656.13944
Ward, A. (2024). First polar bear to die of bird flu; what are the implications? Conversat. (UK Ed.).
Wassmann, P., Duarte, C. M., Agustí, S., and Sejr, M. K. (2011). Footprints of climate change in the Arctic marine ecosystem. Glob. Change Biol. 17, 1235–1249. doi:10.1111/j.1365-2486.2010.02311.x
Wei, C.-L., Rowe, G. T., Escobar-Briones, E., Boetius, A., Soltwedel, T., Caley, M. J., et al. (2010). Global patterns and predictions of seafloor biomass using random forests. PLOS ONE 5, e15323. doi:10.1371/journal.pone.0015323
Weitzman, B., Konar, B., Iken, K., Coletti, H., Monson, D., Suryan, R., et al. (2021). Changes in rocky intertidal community structure during a marine heatwave in the northern Gulf of Alaska. Front. Mar. Sci. 8. doi:10.3389/fmars.2021.556820
Welch, H., Savoca, M. S., Brodie, S., Jacox, M. G., Muhling, B. A., Clay, T. A., et al. (2023). Impacts of marine heatwaves on top predator distributions are variable but predictable. Nat. Commun. 14, 5188. doi:10.1038/s41467-023-40849-y
Wernberg, T. (2021). “Marine heatwave drives collapse of kelp forests in western Australia,” in Ecosystem collapse and climate change. Editors J. G. Canadell,, and R. B. Jackson (Cham: Springer International Publishing), 325–343. doi:10.1007/978-3-030-71330-0_12
Wernberg, T., Krumhansl, K., Filbee-Dexter, K., and Pedersen, M. F. (2019). “Chapter 3 - status and trends for the world’s kelp forests,” in World seas: an environmental evaluation. Editor C. Sheppard Second Edition (Academic Press), 57–78. doi:10.1016/B978-0-12-805052-1.00003-6
Wernberg, T., S. Thomsen, M., K. Baum, J. J., Bishop, M. F., Bruno, J., A. Coleman, M., et al. (2024). Impacts of climate change on marine foundation species. Annu. Rev. Mar. Sci. 16, 247–282. doi:10.1146/annurev-marine-042023-093037
Will, A., Takahashi, A., Thiebot, J., Martinez, A., Kitaiskaia, E., Britt, L., et al. (2020a). The breeding seabird community reveals that recent sea ice loss in the Pacific Arctic does not benefit piscivores and is detrimental to planktivores. Deep Sea Res. II 181, 104902. doi:10.1016/j.dsr2.2020.104902
Will, A., Thiebot, J.-B., Ip, H. S., Shoogukwruk, P., Annogiyuk, M., Takahashi, A., et al. (2020b). Investigation of the 2018 thick-billed murre (Uria lomvia) die-off on St. Lawrence Island rules out food shortage as the cause. Deep Sea Res. Part II Top. Stud. Oceanogr. 181–182. doi:10.1016/j.dsr2.2020.104879
Williams, B. K., and Brown, E. D. (2014). Adaptive management: from more talk to real action. Environ. Manag. 53, 465–479. doi:10.1007/s00267-013-0205-7
Woehler, E. J., and Hobday, A. J. (2023). Impacts of marine heatwaves may be mediated by seabird life history strategies. Mar. Ecol. Prog. Ser. HEAT 737, 9–23. doi:10.3354/meps14333
Wolf, K. K. E., Hoppe, C. J. M., Rehder, L., Schaum, E., John, U., and Rost, B. (2024). Heatwave responses of Arctic phytoplankton communities are driven by combined impacts of warming and cooling. Sci. Adv. 10, eadl5904. doi:10.1126/sciadv.adl5904
Wyatt, A. M., Resplandy, L., and Marchetti, A. (2022). Ecosystem impacts of marine heat waves in the northeast Pacific. Biogeosciences 19, 5689–5705. doi:10.5194/bg-19-5689-2022
Yamamoto, T., Hoshina, K., Nishizawa, B., Meathrel, C. E., Phillips, R. A., and Watanuki, Y. (2015). Annual and seasonal movements of migrating short-tailed shearwaters reflect environmental variation in sub-Arctic and Arctic waters. Mar. Biol. 162, 413–424. doi:10.1007/s00227-014-2589-1
Young, R. C., Kitaysky, A. S., Carothers, C., and Dorresteijn, I. (2014). Seabirds as a subsistence and cultural resource in two remote Alaskan communities. Ecol. Soc. 19, art40. doi:10.5751/es-07158-190440
Zeller, D., Booth, S., Pakhomov, E., Swartz, W., and Pauly, D. (2011). Arctic fisheries catches in Russia, USA, and Canada: baselines for neglected ecosystems. Polar Biol. 34, 955–973. doi:10.1007/s00300-010-0952-3
Zeller, D., Palomares, M. L. D., Tavakolie, A., Ang, M., Belhabib, D., Cheung, W. W. L., et al. (2016). Still catching attention: sea Around Us reconstructed global catch data, their spatial expression and public accessibility. Mar. Policy 70, 145–152. doi:10.1016/j.marpol.2016.04.046
Zhang, X., Zheng, F., and Gong, Z. (2024). Regulatory factors and climatic impacts of marine heatwaves over the Arctic Ocean from 1982 to 2020. Int. J. Climatol. 44, 5297–5319. doi:10.1002/JOC.8630
Zhuang, Y., Jin, H., Cai, W.-J., Li, H., Jin, M., Qi, D., et al. (2021). Freshening leads to a three-decade trend of declining nutrients in the western Arctic Ocean. Environ. Res. Lett. 16, 054047. doi:10.1088/1748-9326/abf58b
Keywords: marine heatwave, sea ice, Arctic, Subarctic, marine ecosystem, climate change, extreme events, ecological change
Citation: Pecuchet L, Mohamed B, Hayward A, Alvera-Azcárate A, Dörr J, Filbee-Dexter K, Kuletz KJ, Luis K, Manizza M, Miller CE, Staehr PAU, Szymkowiak M and Wernberg T (2025) Arctic and Subarctic marine heatwaves and their ecological impacts. Front. Environ. Sci. 13:1473890. doi: 10.3389/fenvs.2025.1473890
Received: 31 July 2024; Accepted: 08 January 2025;
Published: 19 February 2025.
Edited by:
Per Fauchald, Norwegian Institute for Nature Research (NINA), NorwayReviewed by:
Jing Ma, Nanjing University of Information Science and Technology, ChinaJaclyn Clement Kinney, Naval Postgraduate School, United States
Copyright © 2025 Pecuchet, Mohamed, Hayward, Alvera-Azcárate, Dörr, Filbee-Dexter, Kuletz, Luis, Manizza, Miller, Staehr, Szymkowiak and Wernberg. This is an open-access article distributed under the terms of the Creative Commons Attribution License (CC BY). The use, distribution or reproduction in other forums is permitted, provided the original author(s) and the copyright owner(s) are credited and that the original publication in this journal is cited, in accordance with accepted academic practice. No use, distribution or reproduction is permitted which does not comply with these terms.
*Correspondence: Laurene Pecuchet, bGF1cmVuZS5wZWN1Y2hldEB1aXQubm8=
†Present address: Manfredi Manizza, National Institute of Oceanography and Applied Geophysics, Trieste, Italy