- 1Urban Water Systems Engineering, TUM School of Engineering and Design, Technical University of Munich, Munich, Germany
- 2University of Applied Science and Art of Western Switzerland HEPIA HES-SO, Geneva, Switzerland
- 3Urban Productive Ecosystems, TUM School of Life Sciences, Technical University of Munich, Munich, Germany
- 4Restoration Ecology, TUM School of Life Sciences, Technical University of Munich, Munich, Germany
- 5Research Unit Comparative Microbiome Analysis, Helmholtz Zentrum München, Neuherberg, Germany
- 6Strategic Landscape Planning and Management, TUM School of Life Sciences, Technical University of Munich, Munich, Germany
- 7Terrestrial Ecology, TUM School of Life Sciences, Technical University of Munich, Munich, Germany
- 8Environmental Microbiology, TUM School of Life Sciences, Technical University of Munich, Munich, Germany
Sustainable Urban Drainage Systems (SUDS) are ecosystems that are based on engineered soil and designed plant communities to manage stormwater on-site and to enhance infiltration, evapotranspiration, and cooling, thus reducing flooding and urban heat islands. In addition, SUDS may act as hotspots for biodiversity and could be more socially accepted if they work well and are multifunctional. However, we still lack a critical understanding of the techno-ecological basis to construct SUDS sustainably. Due to climate change and pollutants such as de-icing salts, SUDS are confronted with harmful environmental triggers that interfere with their sustainable development. Thus, the challenge is to combine stormwater treatment and urban drainage with principles of restoration ecology, while implementing expertise from soil science, microbiome research, and plant ecology. In this perspective paper, we will discuss the SUDS development and maintenance principle and the role of interdisciplinary research in reaching these goals.
1 Introduction
With two billion more people living in urban areas by 2050 (Bai et al., 2018), urbanization poses unprecedented challenges. Economic, social, and cultural promises associated with urbanization are counteracted by the depletion or degradation of natural resources, biodiversity, and ecosystem services (WBGU, 2016). These degradation trends harm human health and wellbeing (Sarkar and Webster, 2017). Moreover, cities are significant drivers of climate change (Revi et al., 2014) and are particularly vulnerable to its effects, including heat waves, droughts, hurricanes, and flooding (Baccini et al., 2007). This resulted in more than 20,000 additional heat-related death cases in German cities in the period 2018–2020 (Winklmayr et al., 2022). Current challenges are to increase the capacity of urban areas to deal with a changing environment, mitigate ecological impacts, enhance resource efficiency, human health, social inclusiveness, and equality, and harness the productivity of local economies and value-added activities (United Nations, 2017).
Many studies have suggested that turning urban environments into biodiversity hotspots might improve the quality of urban life and its resilience against extreme weather events and other stressors. This would enhance the wellbeing and health of people living in urban areas (Dearborn and Kark, 2010; Faeth et al., 2011; Kazemi et al., 2011; Guilland et al., 2018; Rega-Brodsky et al., 2022). In the past decades, several concepts were developed for an improved green infrastructure (Pauleit et al., 2020) that could enhance urban biodiversity, e.g., Biodiversity Sensitive Urban Design (Kirk et al., 2021), and Animal Aided Design (Weisser and Hauck, 2017). This includes implementing novel types of buildings and infrastructure with more natural elements, as done in Singapore (Rowe and Hee, 2019). In addition to adapting entire cities to environmental challenges, solutions at the local level of urban districts, streets, or building blocks have been discussed and implemented. These concepts can provide synergies for people and the environment. However, often they may need more socioeconomic acceptance due to the high costs of their implementation. A potential solution is combining different urban functions as green-blue-grey infrastructure also considered nature-based solutions (Hoang and Fenner, 2016). For example, Sustainable Urban Drainage Systems (SUDS) are infiltration sites that combine the function of dewatering with other functions such as decontamination, microclimate regulation, and habitat for biodiversity (Fletcher et al., 2015) (Figure 1).
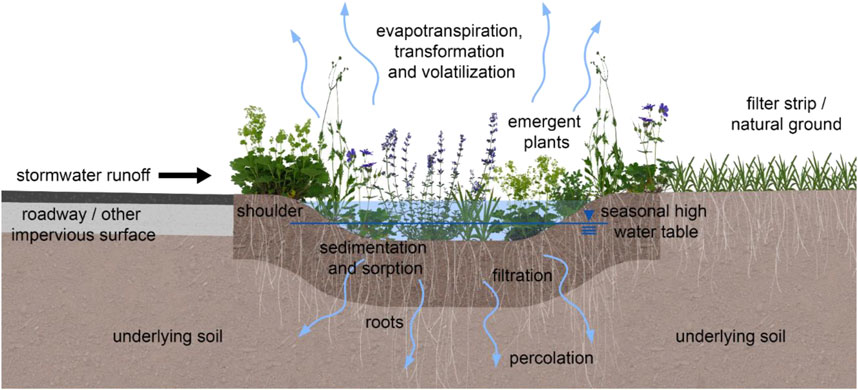
Figure 1. Sustainable Urban Drainage Systems (SUDS) combine the function of an infiltration swale with other ecological functions, such as decontamination of runoff, cooling, habitat for biodiversity, and recreation.
Contemporary SUDS have largely been designed from a technical perspective as infiltration systems without fully considering the potential of such systems to benefit biodiversity or human recreation (Zhou, 2014; Zhang and Chui, 2019), or by developing functional plant communities with more efficient ecological functions including water cycle regulation (Rojas-Botero et al., 2021; Rojas-Botero et al., 2023a). This would generate positive feedback loops for the urban environment, including mitigating climate-related issues (Monberg et al., 2019; Monberg et al., 2018). Thus, the implementation of SUDS can provide multiple ESS, that range from supporting biodiversity (Filazzola et al., 2019), increasing human wellbeing (Coutts and Hahn, 2015), and reducing the urban heat island effect (Norton et al., 2015). The conversion of SUDS into those of multifunctional green infrastructure could reduce land-use conflicts between built infrastructure and biodiversity hotspots, as infiltration systems are already present in some urban areas, albeit often poorly designed and managed. In this perspective paper, the term “multifunctionality” generally refers to the ecological and environmental functions that are provided by SUDS, while the potential social functions (educational value, recreation, etc.) are not addressed in detail.
Despite the obvious advantages of SUDS, we still need a critical understanding of their techno-ecological basis that hinders further engineering of such elements. Thus, more research is necessary to identify optimal design parameters, especially under flooding and drought. Improved SUDS should take up large amounts of stormwater, remove pollutants, prevent erosion, be drought- and flood-tolerant, cope with de-icing salts, and integrate attractive vegetation with low maintenance costs. Thus, the challenge is to combine stormwater treatment and urban drainage with principles of ecological restoration while implementing the latest knowledge in soil science, microbiome research, and vegetation ecology. Principles of restoration include self-supporting populations of native species, normal ecological functions, ecosystem resilience, and landscape connectivity (Holl, 2020). These aims require an in-depth understanding of which plants tolerate the site conditions at SUDS, including stress caused by extreme redox conditions, biocides, and heavy metals, and how these plants can be supported by the specific soil physical and chemical properties and the soil microbiome. Well-designed plant communities will induce positive feedback to the soil by improving carbon stocks as well as the biodiversity of soil biota. The following sections present the key characteristics of SUDS, and discuss how they can be advanced by performing integrative and interdisciplinary research.
2 Soil multifunctionality of SUDS
From the engineering perspective, SUDS have two main functions: de-watering and purification (Dierkes et al., 2015). Appropriate de-watering is important for the infiltration of stormwater runoff so that SUDS do not have too large of storage volumes. According to German regulations (DWA-A 138-1, 2024), for example, the hydraulic conductivity of the vegetated soil zone of infiltration swales must be in the range of 5·10−5 m/s to 1·10−5 m/s. This can be achieved if the mass fraction of organic carbon is max. 4%, and the clay and silt content is <20%. To maintain the infiltration capacity, the soil zone must be covered with vegetation. The purification performance such as pollutant retention and decomposition (Horstmeyer et al., 2016; Deeb et al., 2020; Rommel et al., 2019; Ekka et al., 2021; Galster and Helmreich, 2022; Adhikari et al., 2023) varies depending on the nature of the contaminants and the intensity of the upload charge on the stormwater runoff captured by the SUDS. Contaminants such as nutrients, pathogens, metals, biocides (pesticides, herbicides, etc.), and hydrocarbons can have a significant negative impact on SUDS functions, and the effect of their interaction with other contaminants is still not well understood (Peng et al., 2024). In addition, the effect of emerging contaminants like per- and polyfluoroalkyl substances and others on SUDS performance is not fully identified (Sultan et al., 2024).
Although soil is inherently capable of buffering against pollution, overloading the system in the urban environment threatens some soil functions (Li et al., 2018) and spreading de-icing salt can stress plants and negatively influence the performance of SUDS (Denich et al., 2013). Implementing large-scale SUDS programs requires filling the gap in knowledge and research questions regarding how the engineering soil system and associated biotic interactions (microorganisms and plants) can be optimized for maximum contaminant buffering while ensuring the required functions of stormwater storage and plant community growth.
The functionality of soils as a growth medium and habitat, i.e., supplying water and nutrients, and dealing with contaminants (Lehmann et al., 2020), are intimately linked to the formation and structure of soil aggregates (Totsche et al., 2018). Soil structural stability is a prerequisite for SUDS functions since it guarantees the regulation of water retention and improves infiltration capacity. Soil structure is also essential for other SUDS functions, including gaseous exchange, nutrient dynamics, root growth, and erosion susceptibility. It also provides a habitat for diverse soil organisms and regulates their activity. Improved soil structure can enhance soil remediation of contaminants for improved plant quality and water-holding capacity to support diverse plant communities. The formation and stability of soil aggregate structure strongly depend on the continuous supply of organic matter from plant residues and rhizodeposits, and their turnover by the soil microbial communities. Previous experiments showed a high potential for artificial and immature soils to sequester carbon (Pronk et al., 2017). Therefore, the type of vegetation used and its root system, as well as plant litter, control residue input into the soil and thus decomposition and the formation of soil organic matter. Mowing and removal of the mown materials negatively affect soil development.
The soil organic-mineral composition and the thickness of the topsoil layers play a central role as they largely determine the sorption kinetics and capacities for pollutants (Rommel et al., 2019). Charcoal is a potential approach to improve the capacities of soils to handle different kinds of contaminants (Spahr et al., 2022). In urban environments, high-carbon amendments, such as lawn clippings and compost, are common and cheap, potentially boosting soil carbon and structure (Egerer et al., 2018). Yet, it needs clarification on how varying amounts and types of organic amendments can improve carbon sequestration and binding of water and soil contaminants, and how these benefits support multifunctional SUDS implementation.
Finally, nutrient availability in soils strongly impacts plant development as well as the structure and function of the soil microbiome. Fertilizer addition improves the activity of soil microbiota and triggers plant biomass development but reduces above- and below-ground biodiversity. On the other hand, low nutrient concentrations reduce microbial activity and plant growth. Thus, nutrient management must fit the actual demands of plants and microbiota.
3 SUDS microbiome as a driver of environmental and human health
Soils are a hotspot for microbial diversity (Mguni et al., 2016). This enormous diversity forms the basis for many ESS that soils can provide, including carbon sequestration, degradation or fixation of pollutants, safeguarding drinking water resources, and supporting plant growth (Berg et al., 2020). The diversity of bacteria, archaea, fungi, and other eukaryotes determines the resilience of soils. However, like many other biota, a substantial decline in functional microbial richness has been observed in the past decades due to soil degradation followed by a loss of soil-based ecosystem functions (Felipe-Lucia et al., 2020). Recent studies indicate that reduced microbial diversity in the environment drives several common diseases, including infections and allergies (Foesel et al., 2019). At the same time, an increase of microbes that carry antibiotic resistance genes increased due to co-selection of these traits in the presence of other pollutants, which is a risk factor for human health (Seiler and Berendonk, 2012).
Thus, strategies are needed to stabilize the soil microbiome and to maintain functional potentials that improve environmental and human health. In urban environments, such systems are strongly needed, as sealing, compaction, salt, animal feces, microplastic, and pollution of soils induce a substantial decline in functional microbial activities. At the same time, invasive pathogens might increase or the number of antibiotic-resistant microbiota rises (Bodus et al., 2024). In addition, poor soil qualities regarding nutrient availability and soil structuring often interfere with strategies to promote microbial diversity in urban soils.
Approaches to improve biodiversity in soils are well known from restoration ecology and agroecology. In restoration ecology, legumes are often used as pioneer plants, which will enhance nutrient contents of soils (nitrogen, phosphorous) and foster with their rooting network soil structure development (Pihlap et al., 2019). This can induce positive feedback loops for the diversity of the soil microbiome and its functionality (Vuko et al., 2020). In agriculture, soil biodiversity can be enhanced by intercropping, in which two plants with contrasting properties are grown simultaneously (Mwakilili et al., 2021). These approaches are often supported by selected bio inocula, which provide certain functionalities essential for the soil microbiome to benefit the growth of the crop plants (Ptaszek et al., 2023). Translating this knowledge to develop SUDS could mean transplanting a highly diverse microflora from natural grassland to urban soils. This would support beneficial microbiota and their colonization of the soil by designed plant mixtures, which provide the needed niches for the inoculated bacteria, fungi archaea, and protists.
4 Designed plant communities for SUDS
A current challenge in SUDS is to develop plant communities that provide better soil functions, while tolerating chemical (contamination, pharmaceuticals, metals, etc.) and physical stressors (temperature, high water saturation, etc.). Plant communities at SUDS have to be selected to provide at least some of the ESS mentioned by Monberg et al. (2018); Monberg et al. (2019) and Papuga et al. (2022), and innovative plantings are based on the theory of community assembly and trait-based restoration (Laughlin and Laughlin, 2013; Eben et al., 2024b; Eben et al., 2024a). Plant communities should be designed using ecological criteria that combine water management, urban soil development, pollutant immobilization, and biodiversity maintenance, thus boosting synergies of ESS within SUDS. The design must be based on the regional species pool (Bauer et al., 2023). In an urban environment, it can also include non-native species that cope with the extreme site conditions of SUDS. However, the latter must be considered with great caution to avoid biological invasions that do not support principles of ecological restoration (Holl, 2020). Future mixtures should balance life forms, plant traits, and phylogenetic diversity (Yannelli et al., 2017). In some cases, the interaction between various urban stressors could negatively affect plant communities and soil biodiversity (Rojas-Botero et al., 2023b). Thus, the selected plant communities must be tested under heat waves, drought, waterlogging, and pollutants to develop the best practical recommendations.
Moreover, there are functional linkages between above- and belowground processes, and vegetation structure and composition that may modify belowground functioning, including microbial communities, nutrient cycling, and soil composition (Bardgett et al., 2014; Wardle et al., 2004). One of the main insights of biodiversity research is that increasing species richness in a plant community increases overall functioning with respect to many processes (Weisser et al., 2017). Thus, by combining different plant species the added properties of the community may increase the overall provisioning of ecosystem service. For example, plant species richness and composition influence the water balance of herbaceous plant communities through several mechanisms (Leimer et al., 2014). Plant community composition, for example, affects earthworm communities in experimental plant communities, which in turn influence plant species traits and soil water infiltration (Fischer et al., 2014; Fischer et al., 2015). These interactions can together have a strong effect on how plant communities respond to extreme events, whereby plant species richness can stabilize ecosystem functions (e.g., Isbell et al., 2015; Huang et al., 2024). In addition, further plant species may colonize SUDS as so-called spontaneous vegetation. Whether such species that can live under the conditions of SUDS further increase functionality needs further investigation. Thus, when developing novel plant communities for SUDS, the species-specific root systems affecting belowground carbon storage, water retention, and interactions with microorganisms that degrade some pollutants must be considered (Rojas-Botero et al., 2023a), along with the emerging properties of the resulting plant communities. Extreme scenarios, such as flooding or drought, as well as the stability of the plant community over time should also be examined.
However, there is no guarantee that other components of the belowground soil communities will follow suit because faunal diversity is poorly understood in SUDS soils. Thus, besides plant selection, soil components could directly affect soil diversity; for instance, adding topsoil and encouraging connectivity between SUDS will increase faunal richness (Deeb et al., 2020). Indeed, the way SUDS are built affects the functional microbiome (Deeb et al., 2020). The combination of landscape design, selected plants, location, and the desired ratio of the mineral-organic mixture in the soil (Deeb et al., 2020; Deeb et al., 2017) is expected to influence microorganism activity in SUDS. Finally, the landscape connectivity of urban green and blue infrastructure must be considered to realize the full biodiversity potential of SUDS, as this could influence species colonization as well as the ability of SUDS to act as a stepping stone habitat in the landscape.
5 Conclusion, recommendation and outlook
An integrated approach to understand and to create evidence-based multifunctional SUDS should be the aim of interdisciplinary studies. The soil, microbiome, and plants must be considered as functional components of SUDS. Thus, approaches derived from systems biology are needed to promote positive feedback loops, encouraging functionalities of SUDS, including increased biodiversity. To understand the factors that drive SUDS multifunctionality, we must investigate and test how different combinations of soil and plants can influence functions including infiltration, water storage, and carbon sequestration. This knowledge informs how SUDS can be engineered to optimize such tasks in the urban environment while promoting biodiversity and aesthetics (Backhaus and Fryd, 2013). Specifically, the engineering of SUDS needs to integrate various functions that are tailored to the location of where the installation is feasible and recommended to achieve the best balance, e.g., of organic adsorption to minerals, and how to apply them sequentially as soil layers. This can then predict the development of soil structure, including soil aggregation processes and soil porosity, which can facilitate habitat installation while increasing carbon storage. In addition, plant communities with various root systems should be selected to increase water retention capacity, soil stability, and faunal activity.
Furthermore, it requires working with urban planners and policymakers to adapt and integrate SUDS within the respective city landscape, as green infrastructure may require working with laws, regulations, and city ordinances that enable or hinder their implementation (Dhakal and Chevalier, 2017). Cross-sectoral and well-coordinated governance, availability of resources (e.g., human, financial, data), stakeholder awareness, socially inclusive and just planning approaches, and reliable evidence of the long-term costs and benefits, are key factors for the establishment of multifunctional SUDS.
Future research should test how soil composition (e.g., different ratios of organic mineral components), along with specific plant communities (combinations of native and non-native plants of various functional traits), influence the soil microbiome and the ability of the SUDS to filter pollutants. In addition to regulating and purifying water, future experiments should evaluate other soil functions, such as carbon and water storage. One could, for example, use locally excavated mineral materials from urban construction work and organic materials such as biochar and compost, taking a circular economy approach in the urban environment as they are locally available. The materials are then mixed in different ratios and organized into specific horizons to maximize promising ecosystem services and increase the sustainability of SUDS. These can provide nutrients that promote certain combinations of plant and microbial communities, while soil structural elements support pollutant removal.
Data availability statement
The original contributions presented in the study are included in the article/supplementary material, further inquiries can be directed to the corresponding author.
Author contributions
BH: Conceptualization, Writing–original draft. MD: Writing–original draft. PE: Visualization, Writing–review and editing. ME: Writing–original draft. JK: Writing–original draft. SS: Writing–original draft. SP: Funding acquisition, Project administration, Writing–review and editing. WW: Writing–review and editing. MS: Conceptualization, Writing–original draft.
Funding
The author(s) declare that financial support was received for the research, authorship, and/or publication of this article. The German Research Foundation (DFG) funds the Research Training Group Urban Green Infrastructure – Training Next- Generation of Professionals for Integrated Urban Planning Research (GRK 2679/1).
Conflict of interest
The authors declare that the research was conducted without any commercial or financial relationships that could be construed as a potential conflict of interest.
Generative AI statement
The author(s) declare that no Generative AI was used in the creation of this manuscript.
Publisher’s note
All claims expressed in this article are solely those of the authors and do not necessarily represent those of their affiliated organizations, or those of the publisher, the editors and the reviewers. Any product that may be evaluated in this article, or claim that may be made by its manufacturer, is not guaranteed or endorsed by the publisher.
References
Adhikari, B., Perlman, R., Rigden, A., Walter, M. T., Clark, S., and McPhillips, L. (2023). Field assessment of metal and base cation accumulation in green stormwater infrastructure soils. Sci. Total Environ. 875 (6), 162500. doi:10.1016/j.scitotenv.2023.162500
Baccini, P., Baumgartner, F., Lichtensteiger, T., Michaeli, M., and Thalmann, E. (2007). “Urbane schweiz,” in Klimaänderung und die Schweiz 2050. Erwartete Auswirkungen auf Umwelt, Gesellschaft und Wirtschaft, OcCC ProClim (Bern, Switzerland), 123–136.
Backhaus, A., and Fryd, O. (2013). The aesthetic performance of urban landscape-based stormwater management systems: a review of twenty projects in Northern Europe. J. Landsc. Archit. 8 (2), 52–63. doi:10.1080/18626033.2013.864130
Bai, X., Dawson, R. J., Ürge-Vorsatz, D., Delgado, G. C., Salisu Barau, A., Dhakal, S., et al. (2018). Six research priorities for cities and climate change. Nature 555 (7694), 23–25. doi:10.1038/d41586-018-02409-z
Bardgett, R. D., Mommer, L., and De Vries, F. T. (2014). Going underground: root traits as drivers of ecosystem processes. Trends Ecol. and Evol. 29 (12), 692–699. doi:10.1016/j.tree.2014.10.006
Bauer, M., Huber, J., and Kollmann, J. (2023). Fit by design: developing seed–substrate combinations to adapt dike grasslands to microclimatic variation. J. Appl. Ecol. 60 (11), 2413–2424. doi:10.1111/1365-2664.14497
Berg, G., Rybakova, D., Fischer, D., Cernava, T., Vergès, M.-C. C., Charles, T., et al. (2020). Microbiome definition re-visited: old concepts and new challenges. Microbiome 8 (1), 103–122. doi:10.1186/s40168-020-00875-0
Bodus, B., O'Malley, K., Dieter, G., Gunawardana, C., and McDonald, W. (2024). Review of emerging contaminants in green stormwater infrastructure: antibiotic resistance genes, microplastics, tire wear particles, PFAS, and temperature. Sci. Total Environ. 906 (1), 167195. doi:10.1016/j.scitotenv.2023.167195
Coutts, C., and Hahn, M. (2015). Green infrastructure, ecosystem services, and human health. Int. J. Environ. Res. Public Health 12 (8), 9768–9798. doi:10.3390/ijerph120809768
Dearborn, D. C., and Kark, S. (2010). Motivations for conserving urban biodiversity. Conserv. Biol. 24 (2), 432–440. doi:10.1111/j.1523-1739.2009.01328.x
Deeb, M., Groffman, P. M., Blouin, M., Egendorf, S. P., Vergnes, A., Vasenev, V., et al. (2020). Using constructed soils for green infrastructure–challenges and limitations. Soil 6 (2), 413–434. doi:10.5194/soil-6-413-2020
Deeb, M., Desjardins, T., Podwojewski, P., Pando, A., Blouin, M., and Lerch, T. Z. (2017). Interactive effects of compost, plants and earthworms on the aggregations of constructed Technosols. Geoderma 305, 305–313. doi:10.1016/j.geoderma.2017.06.014
Denich, C., Bradford, A., and Drake, J. (2013). Bioretention: assessing effects of winter salt and aggregate application on plant health, media clogging and effluent quality. Water Qual. Res. J. Can. 48 (4), 387–399. doi:10.2166/wqrjc.2013.065
Dhakal, K. P., and Chevalier, L. R. (2017). Managing urban stormwater for urban sustainability: barriers and policy solutions for green infrastructure application. J. Environ. Manag. 203 (1), 171–181. doi:10.1016/j.jenvman.2017.07.065
Dierkes, C., Lucke, T., and Helmreich, B. (2015). General technical approvals for decentralised sustainable urban drainage systems (SUDS) – the current situation in Germany. Sustainability 7 (3), 3031–3051. doi:10.3390/su7033031
DWA-A 138-1 (2024). “Anlagen zur Versickerung von Niederschlagswasser -Teil 1: Planung, Bau und Betrieb,” in Deutsche Vereinigung für Wasserwirtschaft, Abwasser und Abfall e.V., Hennef.
Eben, P., Duthweiler, S., Helmreich, B., Knoll, S., Stinshoff, P., Pauleit, S., et al. (2024a). Thriving under multiple stressors: performance of drought-tolerant perennials and their suitability for infiltration swales. Urban For. and Urban Green. 101, 128535. doi:10.1016/j.ufug.2024.128535
Eben, P., Mohri, M., Pauleit, S., Duthweiler, S., and Helmreich, B. (2024b). Phytoextraction potential of herbaceous plant species and the influence of environmental factors – a meta-analytical approach. Ecol. Eng. 199 (2), 107169. doi:10.1016/j.ecoleng.2023.107169
Egerer, M. H., Lin, B. B., and Philpott, S. M. (2018). Water use behavior, learning, and adaptation to future change in urban gardens. Front. Sustain. Food Syst. 2 (10), 71. doi:10.3389/fsufs.2018.00071
Ekka, S. A., Rujner, H., Leonhardt, G., Blecken, G. T., Viklander, M., and Hunt, W. F. (2021). Next generation swale design for stormwater runoff treatment: a comprehensive approach. J. Environ. Manag. 279 (6), 111756. doi:10.1016/j.jenvman.2020.111756
Faeth, S. H., Bang, C., and Saari, S. (2011). Urban biodiversity: patterns and mechanisms. Ann. N. Y. Acad. Sci. 1223 (1), 69–81. doi:10.1111/j.1749-6632.2010.05925.x
Felipe-Lucia, M. R., Soliveres, S., Penone, C., Fischer, M., Ammer, C., Boch, S., et al. (2020). Land-use intensity alters networks between biodiversity, ecosystem functions, and services. Proc. Natl. Acad. Sci. 117 (45), 28140–28149. doi:10.1073/pnas.2016210117
Filazzola, A., Shrestha, N., and MacIvor, J. S. (2019). The contribution of constructed green infrastructure to urban biodiversity: a synthesis and meta-analysis. J. Appl. Ecol. 56 (9), 2131–2143. doi:10.1111/1365-2664.13475
Fischer, C., Roscher, C., Jensen, B., Eisenhauer, N., and Baade, J. (2014). How do Earthworms, soil texture and plant composition affect infiltration along an experimental plant diversity gradient in Grassland?. PLoS. One 9 (6), e98987. doi:10.1371/journal.pone.0098987
Fischer, C., Tischer, J., Roscher, C., Eisenhauer, N., Ravenek, J., Gleixner, G., et al. (2015). Plant species diversity affects infiltration capacity in an experimental grassland through changes in soil properties. Plant Soil 397 (12), 1–16. doi:10.1007/s11104-014-2373-5
Fletcher, T. D., Shuster, W., Hunt, W. F., Ashley, R., Butler, D., Arthur, S., et al. (2015). SUDS, LID, BMPs, WSUD and more – the evolution and application of terminology surrounding urban drainage. Urban Water J. 12 (7), 525–542. doi:10.1080/1573062X.2014.916314
Foesel, B. U., Pfeiffer, S., Raj, A. C. D., Etschmann, S. K., and Schloter, M. (2019). Applying ecological theories in research: lessons learned from microbial ecology and evolution? The Lung Microbiome. Sheffield: ERS Monograph, 50–66. doi:10.1183/2312508X.10015718
Galster, S., and Helmreich, B. (2022). Copper and zinc as roofing materials – a review on the occurrence and mitigation measures of runoff pollution. Water 14 (3), 291. doi:10.3390/w14030291
Guilland, C., Maron, P. A., Damas, O., and Ranjard, L. (2018). Biodiversity of urban soils for sustainable cities. Environ. Chem. Lett. 16 (4), 1267–1282. doi:10.1007/s10311-018-0751-6
Hoang, L., and Fenner, R. A. (2016). System interactions of stormwater management using sustainable urban drainage systems and green infrastructure. Urban Water J. 13 (7), 739–758. doi:10.1080/1573062X.2015.1036083
Horstmeyer, N., Huber, M., Drewes, J. E., and Helmreich, B. (2016). Evaluation of site-specific factors influencing heavy metal contents in the topsoil of vegetated infiltration swales. Sci. Total Environ. 560–561 (8), 19–28. doi:10.1016/j.scitotenv.2016.04.051
Huang, Y., Stein, G., Kolle, O., Kübler, K., Schulze, E. D., Dong, H., et al. (2024). Enhanced stability of grassland soil temperature by plant diversity. Nat. Geosci. 17, 44–50. doi:10.1038/s41561-023-01338-5
Isbell, F., Craven, D., Connolly, J., Loreau, M., Schmid, B., Beierkuhnlein, C., et al. (2015). Biodiversity increases the resistance of ecosystem productivity to climate extremes. Nature 526 (7574), 574–577. doi:10.1038/nature15374
Kazemi, F., Beecham, S., and Gibbs, J. (2011). Streetscape biodiversity and the role of bioretention swales in an Australian urban environment. Landsc. Urban Plan. 101 (2), 139–148. doi:10.1016/j.landurbplan.2011.02.006
Kirk, H., Garrard, G. E., Croeser, T., Backstrom, A., Berthon, K., Furlong, C., et al. (2021). Building biodiversity into the urban fabric: a case study in applying Biodiversity Sensitive Urban Design (BSUD). Urban For. and Urban Green. 62 (7), 127176. doi:10.1016/j.ufug.2021.127176
Laughlin, D. C., and Laughlin, D. E. (2013). Advances in modeling trait-based plant community assembly. Trends Plant Sci. 18 (10), 584–593. doi:10.1016/j.tplants.2013.04.012
Lehmann, J., Bossio, D. A., Kögel-Knabner, I., and Rillig, M. C. (2020). The concept and future prospects of soil health. Nat. Rev. Earth and Environ. 1 (10), 544–553. doi:10.1038/s43017-020-0080-8
Leimer, S., Kreutziger, Y., Rosenkranz, S., Beßler, H., Engels, C., Hildebrandt, A., et al. (2014). Plant diversity effects on the water balance of an experimental grassland. Ecohydrology 7 (5), 1378–1391. doi:10.1002/eco.1464
Li, G., Sun, G.-X., Ren, Y., Luo, X.-S., and Zhu, Y.-G. (2018). Urban soil and human health: a review. Eur. J. Soil Sci. 69 (1), 196–215. doi:10.1111/ejss.12518
Mguni, P., Herslund, L., and Jensen, M. B. (2016). Sustainable urban drainage systems: examining the potential for green infrastructure-based stormwater management for Sub-Saharan cities. Nat. Hazards 82 (2), 241–257. doi:10.1007/s11069-016-2309-x
Monberg, R. J., Howe, A. G., Kepfer-Rojas, S., Ravn, H. P., and Jensen, M. B. (2019). Vegetation development in a stormwater management system designed to enhance ecological qualities. Urban For. and Urban Green. 46 (12), 126463. doi:10.1016/j.ufug.2019.126463
Monberg, R. J., Howe, A. G., Ravn, H. P., and Jensen, M. B. (2018). Exploring structural habitat heterogeneity in sustainable urban drainage systems (SUDS) for urban biodiversity support. Urban Ecosyst. 21 (6), 1159–1170. doi:10.1007/s11252-018-0790-6
Mwakilili, A. D., Mwaikono, K. S., Herrera, S. L., Midega, C. A. O., Magingo, F., Alsanius, B., et al. (2021). Long-term maize-Desmodium intercropping shifts structure and composition of soil microbiome with stronger impact on fungal communities. Plant Soil 467 (8), 437–450. doi:10.1007/s11104-021-05082-w
Norton, B. A., Coutts, A. M., Livesley, S. J., Harris, R. J., Hunter, A. M., and Williams, N. S. G. (2015). Planning for cooler cities: a framework to prioritise green infrastructure to mitigate high temperatures in urban landscapes. Landsc. Urban Plan. 134 (2), 127–138. doi:10.1016/j.landurbplan.2014.10.018
Papuga, S. A., Seifert, E., Kopeck, S., and Hwang, K. (2022). Ecohydrology of green stormwater infrastructure in shrinking cities: a two-year case study of a retrofitted bioswale in Detroit, MI. Water 14 (19), 3064. doi:10.3390/w14193064
Pauleit, S., Hansen, R., Rall, E. L., and Rolf, W. (2020). “Urban green infrastructure: strategic planning of urban green and blue for multiple benefits,” in The routledge handbook of urban ecology. Editors I. Douglas, P. M. L. Anderson, D. Goode, M. C. Houck, D. Maddox, H. Nagendraet al. 2nd ed. (London: Routledge), 931–941.
Peng, X., Zhang, Z., Chen, H., Zhang, X., Zhang, X., Tan, C., et al. (2024). The investigation of the binding ability between sodium dodecyl sulfate and Cu (II) in urban stormwater runoff. J. Environ. Manag. 350 (1), 119671. doi:10.1016/j.jenvman.2023.119671
Pihlap, E., Vuko, M., Lucas, M., Steffens, M., Schloter, M., Vetterlein, D., et al. (2019). Initial soil formation in an agriculturally reclaimed open-cast mining area - the role of management and loess parent material. Soil Tillage Res. 191 (8), 224–237. doi:10.1016/j.still.2019.03.023
Pronk, G. J., Heister, K., Vogel, C., Babin, D., Bachmann, J., Ding, G.-C., et al. (2017). Interaction of minerals, organic matter, and microorganisms during biogeochemical interface formation as shown by a series of artificial soil experiments. Biol. Fertil. Soils 53 (1), 9–22. doi:10.1007/s00374-016-1161-1
Ptaszek, M., Canfora, L., Pugliese, M., Pinzari, F., Gilardi, G., Trzciński, P., et al. (2023). Microbial-based products to control soil-borne pathogens: methods to improve efficacy and to assess impacts on microbiome. Microorganisms 11 (1), 224. doi:10.3390/microorganisms11010224
Rega-Brodsky, C. C., Aronson, M. F., Piana, M. R., Carpenter, E.-S., Hahs, A. K., Herrera-Montes, A., et al. (2022). Urban biodiversity: state of the science and future directions. Urban Ecosyst. 25 (4), 1083–1096. doi:10.1007/s11252-022-01207-w
Revi, A., Satterthwaite, D. E., Aragón-Durand, F., Corfee-Morlot, J., Kiunsi, R. B. R., Pelling, M., et al. (2014). “Urban areas,” in Climate change 2014: impacts, adaptation, and vulnerability. Part A: global and sectoral aspects. Contribution of working Group II to the fifth assessment report of the intergovernmental panel on climate change. Editors C. B. Field, V. R. Barros, D. J. Dokken, K. J. Mach, M. D. Mastrandrea, T. E. Biliret al. (Cambridge, United Kingdom: Cambridge University Press), 535–612.
Rojas-Botero, S., Kollmann, J., and Teixeira, L. H. (2021). Competitive trait hierarchies of native communities and invasive propagule pressure consistently predict invasion success during grassland establishment. Biol. Invasions 24 (1), 107–122. doi:10.1007/s10530-021-02630-4
Rojas-Botero, S., Teixeira, L. H., and Kollmann, J. (2023a). Low precipitation due to climate change consistently reduces multifunctionality of urban grasslands in mesocosms. PLOSone 18 (2), e0275044. doi:10.1371/journal.pone.0275044
Rojas-Botero, S., Teixeira, L. H., Prucker, P., Kloska, V., Kollmann, J., and Le Stradic, S. (2023b). Root traits of grasslands rapidly respond to climate change, while community biomass mainly depends on functional composition. Funct. Ecol. 37 (7), 1841–1855. doi:10.1111/1365-2435.14345
Rommel, S. H., Ebert, V., Huber, M., Drewes, J. E., and Helmreich, B. (2019). Spatial distribution of zinc in the topsoil of four vegetated infiltration swales treating zinc roof runoff. Sci. Total Environ. 672 (7), 806–814. doi:10.1016/j.scitotenv.2019.04.016
Rowe, P. G., and Hee, L. (2019). “A city in blue and green,” in The Singapore Story. Springer Open, Springer Nature Singapore Pte Ltd. doi:10.1007/978-981-13-9597-0
Sarkar, C., and Webster, C. (2017). Urban environments and human health: current trends and future directions. Curr. Opin. Environ. Sustain. 25 (4), 33–44. doi:10.1016/j.cosust.2017.06.001
Seiler, C., and Berendonk, T. U. (2012). Heavy metal driven co-selection of antibiotic resistance in soil and water bodies impacted by agriculture and aquaculture. Front. Microbiol. 3 (12), 399. doi:10.3389/fmicb.2012.00399
Spahr, S., Teixidó, M., Gall, S. S., Pritchard, J. C., Hagemann, N., Helmreich, B., et al. (2022). Performance of biochars for the elimination of trace organic contaminants and metals from urban stormwater. Environ. Sci. Water Resour. Technol. 8 (5), 1287–1299. doi:10.1039/d1ew00857a
Sultan, M. B., Anik, A. H., and Rahman, Md. M. (2024). Emerging contaminants and their potential impacts on estuarine ecosystems: are we aware of it? Mar. Pollut. Bull. 199 (2), 115982. doi:10.1016/j.marpolbul.2023.115982
Totsche, K. U., Amelung, W., Gerzabek, M. H., Guggenberger, G., Klumpp, E., Knief, C., et al. (2018). Microaggregates in soils. J. Plant Nutr. Soil Sci. 181 (1), 104–136. doi:10.1002/jpln.201600451
United Nations (2017). “New urban agenda,” in Resolution adopted by the general assembly on 23 december 2016. A/RES/256.
Vuko, M., Cania, B., Vogel, C., Kublik, S., Schloter, M., and Schulz, S. (2020). Shifts in reclamation management strategies shape the role of exopolysaccharide and lipopolysaccharide-producing bacteria during soil formation. Microb. Biotechnol. 13 (2), 584–598. doi:10.1111/1751-7915.13532
Wardle, D. A., Bardgett, R. D., Klironomos, J. N., Setälä, H., Van Der Putten, W. H., and Wall, D. H. (2004). Ecological linkages between aboveground and belowground biota. Science 304 (5677), 1629–1633. doi:10.1126/science.1094875
WBGU (2016). Humanity on the move: unlocking the transformative power of cities. Ger. Advis. Counc. Glob. Change (WBGU) Berlin.
Weisser, W., and Hauck, T. R. (2017). AnimAl-Aided design – using a species’ life-cycle to improve open space planning and conservation in cities and elsewhere. BioRxiv 150359. doi:10.1101/150359
Weisser, W. W., Roscher, C., Meyer, S. T., Ebeling, A., Luo, G., Allan, E., et al. (2017). Biodiversity effects on ecosystem functioning in a 15-year grassland experiment: patterns, mechanisms, and open questions. Basic Appl. Ecol. 23 (9), 1–73. doi:10.1016/j.baae.2017.06.002
Winklmayr, C., Muthers, S., Niemann, H., Mücke, H.-G., and van der Heiden, M. (2022). Heat-related mortality in Germany from 1992 to 2021. Dtsch. Ärzteblatt Int. 119 (26), 451–457. doi:10.3238/arztebl.m2022.0202
Yannelli, F. A., Koch, C., Jeschke, J. M., and Kollmann, J. (2017). Limiting similarity and Darwin’s naturalization hypothesis: understanding the drivers of biotic resistance against invasive plant species. Oecologia 183 (1), 775–784. doi:10.1007/s00442-016-3798-8
Zhang, K., and Chui, T. F. M. (2019). Linking hydrological and bioecological benefits of green infrastructures across spatial scales – a literature review. Sci. Total Environ. 646 (1), 1219–1231. doi:10.1016/j.scitotenv.2018.07.355
Keywords: ecosystem service, engineered substrate, microbiome, multifunctionality, plant communities, stormwater, urban environment
Citation: Helmreich B, Deeb M, Eben P, Egerer M, Kollmann J, Schulz S, Pauleit S, Weisser WW and Schloter M (2025) Converting infiltration swales to sustainable urban drainage systems can improve water management and biodiversity. Front. Environ. Sci. 12:1524239. doi: 10.3389/fenvs.2024.1524239
Received: 08 November 2024; Accepted: 30 December 2024;
Published: 20 January 2025.
Edited by:
Cristina Matos, University of Trás-os-Montes and Alto Douro, PortugalReviewed by:
Marc Gimenez Maranges, University of Salzburg, AustriaCopyright © 2025 Helmreich, Deeb, Eben, Egerer, Kollmann, Schulz, Pauleit, Weisser and Schloter. This is an open-access article distributed under the terms of the Creative Commons Attribution License (CC BY). The use, distribution or reproduction in other forums is permitted, provided the original author(s) and the copyright owner(s) are credited and that the original publication in this journal is cited, in accordance with accepted academic practice. No use, distribution or reproduction is permitted which does not comply with these terms.
*Correspondence: Brigitte Helmreich, Yi5oZWxtcmVpY2hAdHVtLmRl