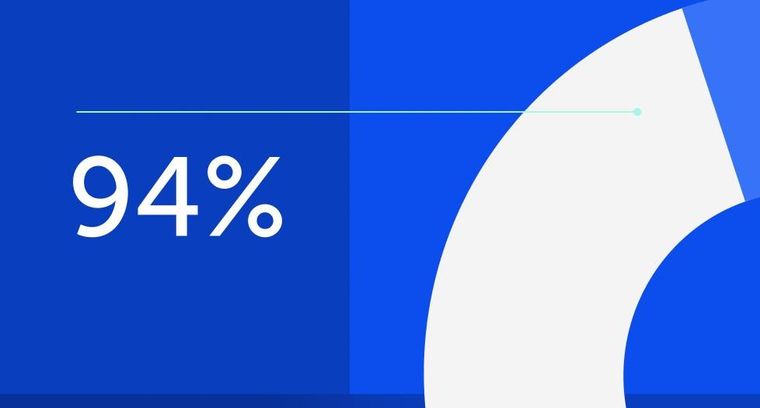
94% of researchers rate our articles as excellent or good
Learn more about the work of our research integrity team to safeguard the quality of each article we publish.
Find out more
ORIGINAL RESEARCH article
Front. Environ. Sci., 06 January 2025
Sec. Toxicology, Pollution and the Environment
Volume 12 - 2024 | https://doi.org/10.3389/fenvs.2024.1511658
This article is part of the Research TopicEmerging Contaminants and Aquatic Ecosystem HealthView all 5 articles
Prometryn is an herbicide widely used in agriculture. Its degradation-resistant properties have raised concerns about its environmental impact on aquatic systems, yet its environmental distribution and bioaccumulation remain to be explored. This research examined the environmental distribution of prometryn within lab-simulated aquatic ecosystems, incorporating water-sediment interactions and bioaccumulation in tilapias (Oreochromis niloticus) and Asian clams (Corbicula fluminea). The research aims to explore the bioaccumulation dynamics of prometryn across both biotic and abiotic components of the ecosystem, providing a comprehensive understanding of its environmental persistence and accumulation in aquatic organisms and sediments. The ecosystems were exposed to a prometryn concentration of 0.50 mg/L for 35 days. During the experiment, significant adsorption and retention of prometryn in the sediment were observed, suggesting that the sediment could be the primary repository. Additionally, tilapias and Asian clams not only served as accumulative pools for prometryn but also influenced its distribution dynamics within the ecosystems. In terms of bioconcentration, the highest bioconcentration factors were observed in the liver of tilapias and the visceral mass of Asian clams, suggesting a strong affinity of prometryn for these tissues. The persistently high levels of prometryn indicate potential risks to aquaculture product safety. The study concludes with a recommendation for ongoing ecological risk assessments, particularly regarding benthic organisms, given the propensity of prometryn to accumulate in sediment within aquaculture systems.
The use of herbicides has become widespread, with global pesticide consumption exceeding 3.5 million tons, of which herbicides accounted for 47.5% (Sharma et al., 2019). Prometryn (2,4-bis(isopropylamino)-6-methylthio-s-triazine, C10H19N5S) was one of the most applied herbicides throughout the world in the second half of the 20th century (Đikić, 2014). It was primarily used to control unwanted vegetation in crops. Notably, only about 10%–30% of applied prometryn is absorbed by plants or soil, with the remainder often entering surface waters through precipitation, irrigation, and runoff (USEPA, 1996b). The substance also finds utility in aquaculture settings to eradicate nuisance algae and weeds, promoting a healthier environment for cultivating aquatic species like fish, shrimp, and sea cucumbers (Zhao and Zhu, 2016). However, research indicates that less than 1% of prometryn reaches the target plant species in these aquatic systems, resulting in a consequential concentration within the ecosystem (Zhang and Wu, 2014). Laboratory studies have confirmed the stability of prometryn in various natural environments, and its high mobility in sandy soils with low organic matter and clay content facilitates its migration into groundwater systems (USEPA, 1996b). Environmental monitoring has recorded high concentrations of prometryn, reaching up to 0.63 μg/L in the Yellow Sea and Bohai Sea of China (Yang et al., 2019), and 0.44 μg/L in seawater samples from the coastal areas of Hainan, China (Dsikowitzky et al., 2020). Additionally, detections have been reported in aquatic systems in Greece (0.078–4.4 μg/L) (Vryzas et al., 2011) and western France (100–330 ng/L) (Caquet et al., 2013). These elevated concentrations underscore the potential environmental impact of prometryn, necessitating careful management and monitoring.
Internationally, regulatory responses to the environmental persistence of prometryn vary. The European Union, citing concerns for water quality and ecological health, has prohibited its use (European Food Safety Authority, 2009). Other nations, including Japan and the United States, categorize prometryn as an herbicide and have established specific residue limits in certain agricultural products; however, these limits exclude aquatic products (USEPA, 1996b; The Japan Food chemical Research Faundation, 2015). In contrast, the approach of China has evolved over time; despite widespread use in agriculture and aquaculture, the Ministry of Agriculture of China enacted Announcement No. 1435 in 2010, which discontinued the use of prometryn in aquaculture owing to uncertainties about its metabolic patterns in fish and associated food safety evaluations (The Ministry of Agriculture of the People’s Republic of China, 2010). However, standards for permissible residue levels in aquatic products remain undefined in China (Yu et al., 2012). Notwithstanding, prometryn is still used irregularly in some Chinese aquaculture to remove filamentous algae, aquatic weeds, and other harmful algae (Liu et al., 2016; Zhao and Zhu, 2016), and leading to high concentrations in aquatic ecosystems (Yang et al., 2019; Dsikowitzky et al., 2020).
Despite its high efficiency against algae and weeds, prometryn has also been reported toxic to non-target fauna. Saka et al. (2018) demonstrated that the 96-h LC50 of prometryn to Silurana tropicalis tadpoles was 2.13 mg/L. After exposure to 1 μg/L or higher concentration of prometryn for 21 days, yolk sac shrinkage, heart malformations, delayed hatching time, and increased heart rate were observed in marine medaka (Oryzias melastigma) embryos (Samreen et al., 2022). While current researches often focus on the bioaccumulation of prometryn within aquatic organisms, such as shellfish, fish, and sea cucumbers. Despite the sustained environmental exposure (Stara et al., 2013; Liu et al., 2016; Yang et al., 2019; Dsikowitzky et al., 2020), there is a noted lack of studies examining the environmental behavior of prometryn within aquatic ecosystems. Laboratory models of aquatic ecosystems present a valuable method for evaluating the indirect effects of variable pollutants, including their interactions with both biotic and abiotic components (e.g., sediment and organisms) and interspecies dynamics (Dai et al., 2016; Felis et al., 2016; Rogers et al., 2016), which make them feasible for evaluating the environmental behaviors of prometryn. In our previous work (Yang et al., 2022), we investigated the potential toxicity and accumulation risks of prometryn using tilapias as a model species in aquaculture. However, this study was limited to a single species, and the distribution and bioaccumulation of prometryn across both biotic and abiotic components in more complex, simulated ecosystems had not been explored. This study aims to construct a simulated model of aquatic ecosystems, enabling a comparative analysis of the adsorption, distribution, and bioaccumulation of prometryn across two different aquatic systems. The concentrations of prometryn were set at 0.50 mg/L, 1/10 of the 96-h LC50 value (Yang et al., 2022), which ensured that prometryn levels in the ecosystems could be detected by the instruments while avoiding mortality of the test organisms within the simulated ecosystems. The construction of the simulation ecosystems was based on previous researches (Jiang et al., 2017; Wang et al., 2019) and adjusted to meet our research objectives. Tilapias were chosen as the fish model in order to be consistent with our previous study, Asian clams were chosen as the invertebrates model because it is a typical invertebrates in Chinese freshwater aquatic ecosystems (Su et al., 2018). Tilapias and Asian clams made up the biotic components of the simulated ecosystem, and water and sediment made up the abiotic components. The primary objective is to provide a comprehensive evaluation of the distribution and bioaccumulation of prometryn on aquatic ecosystems, thereby enhancing our understanding of its environmental and ecological consequences.
Sediment was meticulously sourced from the Juma River Aquatic Wildlife Nature Reserve (N 39.377°, E 115.386°), located in the Fangshan District of Beijing, China. It was confirmed that the sediment did not contain prometryn using the method in Section 2.4. The collected sediment had an organic matter content quantified at 2.32% (Yang et al., 2022). Juvenile tilapias (O. niloticus), with an average weight of 15.00 ± 5.00 g, were procured from the Xiaotangshan facility of the Beijing Fisheries Research Institute (Beijing, China). Additionally, approximately 1,000 Asian clams (C. fluminea), averaging a weight of 2.00 ± 0.50 g, were acquired from the Dagu River, Laixi, Shandong Province, China. After their collection, the specimens underwent an acclimation phase lasting 10 days within 500 L semi-closed aquacultural systems. The water used in the experiment ensured no prometryn using the method in Section 2.4. The water temperature was consistently regulated at 28°C ± 1°C before and throughout the experimental period. During acclimation, the tilapias were provided with feed pellets at a daily rate equivalent to 1% of their body weight, whereas the Asian clams were fed with Chlorella. Food scraps and feces were removed regularly during acclimation period. Throughout the experimental period, tilapias and Asian clams were provided with small quantities of food to prevent the accumulation of uneaten food residues, thereby minimizing potential confounding effects from food scraps. The water did not change until the end of the experiment. Dissolved oxygen levels were preserved at 70%–100% saturation, and a 12-h photoperiod was artificially maintained. Prior to inclusion in the study, all organisms were rigorously examined to ascertain their health and ensure they were devoid of parasitic infections. All protocols involving tilapias and Asian clams were approved by the Fish Ethics Committee of Chinese Academy of Fishery Sciences, Beijing, China.
Aquatic ecosystems were assembled in adherence to protocols established by the United States Environmental Protection Agency (USEPA, 1996a). To assess the environmental dynamics and ecological impacts of prometryn, three model ecosystems were designed:
CK (Control Ecosystem with Biota): This model comprised water, sediment, tilapias, and Asian clams, but without any prometryn application. It served as the baseline to evaluate the natural behavior of the ecosystem without the influence of prometryn.
E1 (Treated Ecosystem with Biota): Similar to CK, this model included water, sediment, tilapias, and Asian clams. However, it differed by the addition of prometryn (99.7% purity, Shanghai Pesticide Research Institute Co. Ltd., Shanghai, China) at a nominal concentration of 0.50 mg/L (approximately 1/10 96 h LC50 of tilapias) (Yang et al., 2022). This model was designed to study the effects of prometryn on a complete aquatic ecosystem, including both abiotic and biotic components.
E2 (Treated Ecosystem without Biota): This model contained only water and sediment, treated with prometryn at the same concentration as E1. The absence of aquatic organisms (tilapias and Asian clams) in this model allowed for the assessment of the impact of prometryn solely on the abiotic components of the ecosystem.
Preparation of CK and E1: Seven aquaria (glass container, 100 × 50 × 50 cm) were lined with a 5 cm layer of sediment and filled with 200 L of uncontaminated groundwater. Each aquarium was stocked with 35 Asian clams and 30 tilapias of comparable size and health. To minimize disturbances to the behavior of Asian clams by tilapias, a metal mesh barrier was positioned approximately 5 cm above the sediment layer (Di et al., 2019). Four aquaria were treated with prometryn at a nominal concentration of 0.50 mg/L, designated as the E1 group, while the remaining three served as the untreated control group (CK).
Preparation of E2: Smaller ecosystems were chosen for E2 to minimize prometryn usage and reduce the environmental risks associated with the experiment. Three additional aquaria (glass container, 40 × 40 × 60 cm) were prepared without biotic components, containing only water and sediment to serve as abiotic control units. These were also treated with prometryn at a concentration of 0.50 mg/L. A uniform 5 cm layer of sediment was laid at the base, followed by the addition of 64 L of uncontaminated groundwater. Aeration stones were installed in each tank to ensure continuous oxygenation and a homogeneous distribution of prometryn.
Throughout the experiment, tilapia and Asian clam mortalities did not exceed 10% (Supplementary Table S1). Dead individuals were removed and disposed of in a harmless manner. Water volume was regularly topped up to compensate for evaporation. Parameters such as pH, dissolved oxygen, ammonium nitrogen (NH4+-N), nitrate nitrogen (NO3--N), and nitrite nitrogen (NO2--N) were systematically monitored across all ecosystems.
Measurement of water quality parameters: Oxygen concentration, temperature, and pH were measured regularly with an HQ 40D portable multi-meter (HACH Company, Loveland, United States) equipped with LDO 101 Dissolved O2 probe (HACH Company, Loveland, United States) and PHC 101 pH electrode (HACH Company, Loveland, United States). Temperature was measured with a built-in sensor of the pH probe. For the analysis of nitrogen, the water sample was first filtered over a 0.45 μm cellulose acetate filter (Whatman, Maidstone, United Kingdom), then analyzed through a water quality rapid test kit (ZKGC Company, Guangzhou, China).
Prometryn dosing was meticulously calculated based on the water volume in each aquarium. Precise quantities of prometryn powder (99.7% purity, produced by Dr. Ehrenstorfer, Augsburg, Germany)—0.10 g for system E1 containing 200 L of water and 0.032 g for system E2 containing 64 L of water—were weighed to achieve a target concentration of 0.50 mg/L. The powder was dissolved in a portion of the water extracted from each respective glass tank, and the resulting solution was then reintroduced. To ensure minimal substance loss, centrifuge tubes used during the process were rinsed multiple times with water from the tanks, and the rinsate was returned to the respective tanks.
Sampling was conducted at predetermined intervals: 1 h, 6 h, 12 h, 1 day, 2 days, 4 days, 7 days, 14 days, 21 days, 28 days, and 35 days post-administration. Two tilapias, two Asian clams, water and sediment samples were collected from each tank of E1 and CK, respectively. In E2, only water and sediment samples were collected. These samples were immediately frozen pending further pretreatment. For tilapias, gills, muscle, and liver tissues were excised and stored separately; similarly, mantle, muscle, and visceral mass were separately stored for Asian clams. All samples were obtained at 1.00 g (wet weight). Water samples, each exceeding 100 mL, and sediment samples exceeding 50 g (wet weight) were also collected from each model ecosystem.
Samples were stored at −20°C in a light-protected environment to prevent any degradation of the prometryn.
Overall, the instrumental analysis method was based on SN/T 1968-2007 (General Administration of Quality SupervisionI. and Q. of the P. R. of C, 2007), and the sample preparation was improved based on methods reported by our previous research (Yang et al., 2022). The specific steps are as follows.
Tissue and sediment samples: Samples weighing 1.00 g (wet weight) of each tilapias and Asian clam tissues, as well as sediment samples, were individually transferred to 50 mL centrifuge tubes. Each sample was treated with 6 mL of acetonitrile and vortexed at 2,500 rpm for 2 min to ensure thorough mixing. Subsequent ultrasonication occurred at 10°C for 20 min. Post-sonication, the samples were centrifuged at 3,040 × g for 5 min at 4°C. The clear supernatant was then carefully decanted into a new tube. This procedure was repeated to maximize extract purity. The combined supernatants were evaporated to dryness under a stream of nitrogen at 40°C. The dry residue was reconstituted in a 2 mL mixture of ethyl acetate and hexane (2:3, V: V). Concurrently, PSA-SPE columns were primed with 6 mL of n-hexane. The extract was then eluted through the column at a controlled flow rate, followed by column rinsing with the ethyl acetate-hexane mixture. The eluate was collected and evaporated using a nitrogen blower at 40°C. The resultant sample was made up to 1 mL with acetonitrile and filtered through a nylon 66 membrane into an amber vial, subsequently vortexed for 2 min before analysis by gas chromatography-mass spectrometry (GC-MS).
Water samples: 100 mL of water was filtered using a 0.45 μm cellulose acetate membrane, and 1 mL of the filtered water sample was aspirated into a 50 mL centrifuge tube. Unlike tissue samples, no centrifugation was necessary. Sodium chloride was added to the combined extracts, mixed thoroughly via vortexing, and left to settle. The clarified supernatant was then transferred to a new tube, anhydrous sodium sulfate was added, and the subsequent steps aligned with the tissue sample preparation.
Analytical detection was performed using an Agilent 7890A gas chromatograph coupled with an Agilent 7,000 mass spectrometer, employing an electron impact ionization source (Agilent Technologies, Santa Clara, United States). The operational parameters were as follows: a DB-5MS quartz capillary column (L × I.D. 30 m × 0.25 mm, df 0.25 μm); temperature programming starting at 70°C for 1 min, rising to 180°C at a rate of 25 °C/min, then to 220°C at 5°C/min, holding for 3 min; carrier gas was helium of ≥99.999% purity; the inlet temperature was set at 260°C; flow rate at 1.0 mL/min; injection was performed in non-split mode with a volume of 1.0 μL; ionization energy at 70 eV; ion source temperature at 230°C; quadrupole at 150°C; GC-MS interface temperature at 280°C. Monitoring ions were set at m/z 241, 184, 226, 199. The detection limit (LOD) was established at 2.00 μg/kg (μg/L) and the quantification limit (LOQ) at 5.00 μg/kg (μg/L) for prometryn. The method exhibited satisfactory recoveries across matrices, ranging from 93% to 115% for gills, 84.1%–105% for muscle, 88%–114% for liver, 87.9%–108% for Asian clam muscles, 87.9%–112% for mantles, 94.6%–115% for viscera mass, 80.1%–96.6% for sediment, and 86.9%–112% for water. The method meets the analytical requirements.
Statistical analyses were conducted utilizing IBM SPSS Statistics software, version 26 (International Business Machines Corporation, New York, United States). The Shapiro-Wilk test was applied to assess the normality of data distribution, and Levene’s test was employed to evaluate the homogeneity of variances. Where data conformed to a normal distribution and variance homogeneity was confirmed, differences in concentration values across the various days were examined using one-way ANOVA followed by Tukey’s post hoc test for pairwise comparisons, with a significance threshold set at 95%. For datasets not meeting these criteria, Dunnett’s T3 test was utilized for pairwise comparisons (Shingala, 2015; Sauder and DeMars, 2019). In the context of this paper, the term “significant” refers to p-values less than 0.05, while “non-significant” corresponds to p-values greater than 0.05. Concentration data are presented as mean values with their corresponding 95% confidence intervals.
SPSS 26.0 nonlinear regression was used to fit the experimental data to a first-order kinetic model (Beulke and Brown, 2001):
where Ct represents the concentration (mg/L) of prometryn in the water at each time point; C0 represents the initial concentration (mg/L) of prometryn in the water; t represents the time point (d).
The adsorption rate constant (ka) and desorption rate constant (ke) of prometryn in the sediment were calculated by fitting the experimental data into a first-order kinetic model, and the equations are shown in formula (Lang et al., 1990):
where Corg represents the concentration of prometryn (mg/kg) in the sediment at each time point (d), and Cw represents the concentration of prometryn (mg/L) in the water at each time point (d).
The half-life period t1/2 was calculated by the formula (Beulke and Brown, 2001):
Bioconcentration factor (BCF) was calculated for this investigation to quantify the uptake of chemicals by organisms relative to their environment.
where C (tissue) represents the concentration (mg/kg) of the chemical within the organism (wet weight), and C (water) denotes the concentration (mg/L) of the chemical in water.
During the entire duration of the experiment, the dissolved oxygen (DO) levels in all test tanks were consistently maintained within the range of 5.22–8.73 mg/L, exceeding the 70% saturation concentration benchmark (refer to Supplementary Table S2 for detailed data). The pH values were stable, fluctuating between 8.18 and 8.91, with an average of 8.56, as detailed in Supplementary Table S3. Temperature control was maintained at 28°C throughout the study, with the actual water temperatures recorded falling within this set parameter, approximately 27°C–28°C, as shown in Supplementary Table S4. Comparative analysis with the blank control group revealed that the introduction of prometryn into the experimental groups exerted no significant influence on the routinely monitored water quality parameters.
Further, as outlined in Figure 1; Supplementary Table S5, the concentrations of ammonium nitrogen in all test tanks were consistently observed within the range of 0–0.5 mg/L throughout the experimental period. Concentrations of nitrate nitrogen were maintained between 0.2 and 5 mg/L, and nitrite nitrogen levels were kept within a range of 0–0.3 mg/L, thereby ensuring the maintenance of a stable and controlled aquatic environment for the duration of the experiment. In addition, the results showed that the presence of prometryn did not significantly impact the inorganic nitrogen levels in the ecosystem (E1 vs. CK). However, the presence of aquatic animals in the ecosystem significantly increased the concentration of inorganic nitrogen (E1 vs. E2).
Figure 1. Concentrations of inorganic nitrogen in ecosystems. (A) NH4+-N; (B) NO3−-N; (C) NO2−-N. Error bars represent the standard deviation of the samples. *represents p < 0.05, **represents p < 0.01.
The dynamics of prometryn concentration in the water component of the ecosystems were illustrated in Figure 2A. In the water-sediment-tilapias-Asian clams ecosystem (E1), the highest concentration of prometryn, 0.208 mg/L, was observed at 24 h. This peak was followed by a gradual decline from day 1 to day 7. The concentration decreased rapidly, reaching 0.0170 mg/L between day 7 and day 14. An increase in prometryn levels was noted from day 14 to day 21, after which the concentration again began to decrease, stabilizing at 0.0164 mg/L and remaining consistent until the end of the experiment; the final concentration at day 35 was 0.0160 mg/L.
Figure 2. Prometryn concentration in the water (A) and sediment (B) of different ecosystems at each time point.
The trend for the water-sediment ecosystem (E2) was characterized by a rapid decrease in prometryn concentration from 0.391 mg/L to 0.165 mg/L within the first 12 h post-exposure. The concentration then stabilized between 12 h and 1 day. A significant reduction was again observed between day 2 and day 7, from 0.132 mg/L to 0.0159 mg/L, after which the prometryn concentration showed minor fluctuations but remained stable within a range of 0.0159 mg/L to 0.0185 mg/L.
Figure 2B illustrates the concentration profile of prometryn in the sediment within the different ecosystems. In E1, prometryn concentrations in the sediment initially exhibited a modest decline over the first 48 h. This was followed by a phase of concentration increase between day 2 and day 7. Subsequently, there was a gradual decrease to 0.254 mg/kg from day 7 to day 14. Beyond this point, the prometryn concentration in the sediment remained relatively stable until the end of the experiment. The final concentration in the sediment at day 35 was 0.212 mg/kg.
Contrastingly, in E2, the sediment showed a rapid accumulation of prometryn within the initial 12 h, the highest concentration reached 1.675 mg/kg. After this period, there was a decreasing trend, culminating in a concentration of 0.245 mg/kg by day 7. From this point on, the concentration of prometryn in the sediment exhibited minimal change, maintaining a steady level until the end of the experimental period. The final concentration at day 35 was 0.235 mg/kg.
The distribution of prometryn in water and sediment was demonstrated in Table 1; Figure 3. It revealed that, after 1 h of dosing in the E1 ecosystem, the prometryn rapidly migrated to all other phases of the system. At this time, the content of prometryn in the sediment hit its peak, comprising 28.76% of the total. Conversely, in the E2 ecosystem, the prometryn mainly distributed in water, with its concentration making up 78.12% of the total at the same time.
Table 1. Distribution proportion of prometryn in water and sediment in the simulated aquaculture ecosystems.
Figure 3. Prometryn content in environmental matrices (water and sediment) of E1 (A) and E2 (B) at each time point.
After 7 days, the concentration of prometryn in the water of E1 ended the plateau and commenced a decline, while the concentration of prometryn in the water of E2 basically reached equilibrium, with prometryn proportions in the water in the E1 and E2 constituting 28.96% and 3.19% of the total, respectively. At the end of the experiment (35 days), the proportions of prometryn content in water (3.21% vs. 3.31%) and sediment (4.25% vs. 5.86%) of E1 and E2 ecosystems were close.
Experimental data were analyzed using Formulas 1–3, resulting in Table 2. The table facilitated a comparison of adsorption-desorption rates and half-life periods of prometryn between the two ecosystems. It was observed that both the dissipation rate of prometryn in water and the adsorption-desorption rate in sediment were higher in E1 than in E2, leading to shorter half-life periods for prometryn in both water and sediment in E1 compared to E2.
In all experimental tanks of E1, the mortality rates of tilapias were less than 10% (Supplementary Table S1). Deceased individuals were promptly removed, and no abnormal behaviors or physical injuries were observed in the remaining individuals. Figure 4 displays the distribution of prometryn across different tilapia tissues over time. The peak concentrations of prometryn varied among tissues but followed a similar pattern of drug elimination. In the gills, the peak was observed at 1 h after exposure, while in the liver, it occurred at 6 h. The muscle tissue displayed the peak concentration 24 h into the experiment. These peak levels were maintained until day 7, after which a marked decline in tissue concentrations was noted between day 7 and day 14, followed by a gradual, continuous decrease. At day 35, the final concentrations in the liver, gills, and muscles were 0.123 mg/kg, 0.00958 mg/kg, and 0.00790 mg/kg, respectively.
Throughout the experimental period, the concentration hierarchy of prometryn within the tilapia tissues was consistently observed as liver > gills > muscle. This pattern indicates differential accumulation and retention rates of prometryn across various organ systems within the tilapias.
In all experimental tanks of E1, the mortality rates of Asian clams were less than 10% (Supplementary Table S1). Deceased individuals were promptly removed, and no abnormal behaviors or physical injuries were observed in the remaining individuals. Figure 5 delineates the concentration trends of prometryn in various tissues of Asian clams. While the concentration fluctuated over time, the overall trend was similar across different tissues. From 1 h to day 14, the patterns of rise and fall in prometryn content within the three tissues were largely consistent across all eight sampling points.
At 1 h post-prometryn introduction, rapid accumulation was observed in all tissues: 0.365 mg/kg in muscle, 0.235 mg/kg in mantle, and 1.350 mg/kg in visceral mass The peak concentrations in these tissues were attained at 6 h, registering at 0.598 mg/kg, 0.470 mg/kg, and 1.500 mg/kg, respectively. By 12 h, these levels had declined to 0.0800 mg/kg in muscle, 0.0892 mg/kg in mantle, and 0.255 mg/kg in visceral mass. On day 4, an upward trend was noted, with concentrations in the tissues increasing to 0.266 mg/kg, 0.410 mg/kg, and 0.990 mg/kg, respectively. Subsequently, a consistent decrease was observed across all tissues, culminating in concentrations of 0.0195 mg/kg, 0.0185 mg/kg, and 0.0228 mg/kg, respectively, by day 14. Post-day 14, the trends diverged across tissues. At day 35, the final concentrations in mantles, muscles, and viscera were 0.0300 mg/kg, 0.0232 mg/kg, and 0.0126 mg/kg, respectively.
Figure 6A presents the bioconcentration factors (BCF) for prometryn in various tilapia tissues. The BCF values were calculated using Formula 4. The muscle tissue exhibited the least ability to bioaccumulate prometryn, with its highest BCF value of 4.52 recorded on day 4, corresponding to a prometryn concentration of 0.713 mg/kg. In contrast, the gills demonstrated the most pronounced initial bioaccumulation, with a peak BCF of 34.38 and a concentration of 2.695 mg/kg.
Figure 6. Bioconcentration factors (BCF) of prometryn in tilapias (A) and Asian clams (B) in the E1 ecosystem.
The liver, however, consistently showed the highest bioconcentration of prometryn among the tissues. At time point 1 h, the BCF in the liver reached an apex of 69.08, with prometryn levels amounting to 4.312 mg/kg. This finding underscores the capacity of the liver as the primary site for prometryn enrichment within the tilapias. The overall bioaccumulation hierarchy in tilapia tissues for prometryn was observed as liver > gill > muscle.
The ecosystem established for this experiment was meticulously designed to mirror the optimal environmental conditions necessary for the thriving of the test organisms: Nile tilapias and Asian clams. CK and E1 were ecosystems with biota, and the tank should be large enough to give enough space for tilapias and Asian clams. E2 was an ecosystem without biota, and to minimize the usage of prometryn and prevent environmental risk, we chose smaller tanks for E2. The pH levels in natural river ecosystems generally exceed 8.0, a range within which Asian clams are known to thrive (pH 5.5–8.3) (Araujo et al., 1993; Vidal et al., 2002). Similarly, studies by El-Sherif and El-Feky suggest that Nile tilapias exhibit optimal growth in water with a pH range of 7-8, which is beneficial for critical growth parameters (El-Sherif et al., 2009). Rebouças et al. (2016) propose a slightly broader optimal pH range for Nile tilapias, extending from 5.5 to 9.0. Throughout our experiment, the pH was consistently maintained at around 8.56, aligning with the optimal pH ranges for both organisms and ensuring an environment conducive to their health and growth (refer to Supplementary Table S3 for pH data).
Concerning nitrogenous waste parameters, the water quality of the experiment was kept within the safety thresholds for aquatic life. Research suggests that NO2−-N concentrations up to 0.6 mg/L and NH4+-N concentrations below 0.5 mg/L are safe for tilapias, while NO3−-N concentrations above 10 mg/L can be harmful (Song, 2011; Pan et al., 2024). Although the nutrient levels in our experiment were close to these thresholds, mainly due to the retention of uneaten feed and fish excreta (Supplementary Table S5), the high dissolved oxygen content in the water (Supplementary Table S2) played a critical role in mitigating the potentially toxic effects of these compounds (Li et al., 2009).
Therefore, the experimental ecosystem not only replicated key aspects of the natural habitats of the test organisms but also maintained water quality parameters within the optimal range. This careful alignment of environmental conditions validates the suitability of our ecosystem model for examining the behavior and effects of prometryn, ensuring that the observed outcomes were a result of the test compound rather than suboptimal living conditions.
A higher concentration in sediment compared to water was observed during the experiment. This trend may be attributable to the hydrophobic nature of prometryn and its high affinity for organic matter (National Center for Biotechnology Information, 2021). Following application, prometryn was rapidly detected in both water and sediment, aligning with findings that prometryn diffuses swiftly from water to sediment particles (Zhang et al., 2024).
Absorption rate constant (Ka), elimination rate constant (Ke), and elimination half-life (t1/2) are pharmacokinetic equations that reflect the rate of absorption and elimination of substances (Shargel and Yu, 2024). These equations were also used to evaluate the absorption and elimination of substances in aquatic systems (Thit and Selck, 2021; Wang et al., 2023). According to Table 2, the presence of organisms in the E1 accelerated the reduction of promerytn in the water and sediments. Adsorption and desorption are two key processes that organic contaminants undergo in water-sediment systems; however, this process is influenced by several factors, including the compositional properties of pesticides in water-sediment systems and environmental conditions (Margoum et al., 2006; Chen et al., 2010). Organisms cause bioturbation of the ecosystem, which in turn accelerates the elimination of drugs from the ecosystem. Some studies have confirmed that the presence of organisms in the environment promotes the abatement of drugs in the water (Wu et al., 2015).
The adsorption of prometryn to sediment is crucial in determining its environmental fate. In our study, prometryn was promptly detected in sediment and persisted for over 35 days, echoing previous research findings (Hand et al., 2020). The persistence of prometryn in sediment is likely due to its rapid adsorption and subsequent difficulty in desorption (Wu et al., 2021). The Koc value of prometryn is between 200 and 1,000 in river sediments (National Center for Biotechnology Information, 2021). This strong adsorption to sediment suggests that prometryn may accumulate in aquacultural environments, where its prolonged persistence in the benthic zone may pose an ecological risk by potentially affecting sediment-dwelling organisms and disrupting local ecosystems (Zhou et al., 2024). Its potential to harm non-target organisms and adversely affect sensitive species underlines the need to manage and monitor such environmental contaminants carefully (Huang et al., 2023).
N-Octanol/water partition coefficient value (Kow) and organic carbon partition coefficient value (Koc) value are not only important indicators of the distribution of pollutants in environmental media, but are also related to the accumulation of pollutants in organisms (Li and Ran, 2012). The log Kow value of prometryn is 3.71 (USEPA, 1996b), and the Koc value of prometryn is 400 (Wauchope et al., 1992), indicating its propensity for bioconcentration in aquatic organisms, as substances with log Kow values between 2 and 6 are known to enrich in biota easily (Kaune et al., 1998). The BCF values, illustrated in Figure 6, confirm this tendency, with prometryn rapidly accumulating in various tissues of both tilapias and Asian clams within 1 h of exposure.
Notably, the liver in tilapias and the visceral mass in Asian clams demonstrated the most significant bioaccumulation. This highlights the sensitivity of these organs to prometryn exposure, with the viscera being particularly susceptible to bioconcentration. From the seventh to the 14th day, a significant decrease in prometryn concentration was noted in tilapia tissues, mirroring the trend observed in previous studies (Yang et al., 2022). The persistent decline in tissue concentrations may be attributable to biota metabolism. As reported by Sun et al. (2023), the major metabolic process of prometryn in oysters was hydroxylation along with N-dealkylation. Although there have not yet been reports on whether the metabolic pathways of prometryn in tilapia and Asian clams are like those in oysters, the distribution trends of prometryn within the system suggest that biological metabolism plays a significant role in the degradation of prometryn.
In tilapias, the peak prometryn concentrations were observed at different times across tissues: day 4 in muscle, day 2 in liver, and immediately (1 h) in gills. The liver consistently exhibited the highest prometryn levels compared to other tissues, aligning with the results from semi-static prometryn solution enrichment experiments (Yang et al., 2022). The reason for the higher accumulation of prometryn in the liver of tilapias has not yet been reported, while other pollutants were reported to be most accumulated in tilapia livers (Parvin et al., 2019), the detailed reason needs to be explored.
Asian clams, as indicative freshwater bivalves, are sensitive to environmental changes and proficient in accumulating chemicals. In this study, the prometryn concentration sequence in Asian clam tissues was visceral mass > muscle > mantle. Distinct from tilapias, the rapid bioaccumulation in the visceral mass is likely due to its composition, which includes lipid-rich gonads known for their ability to concentrate organic pollutants (Jacomini et al., 2006). The peak concentrations in Asian clam tissues were reached within 6 h, faster than in other studied bivalves (Tian et al., 2013; Liu et al., 2016), underscoring species-specific differences in bioaccumulation capacity related to physiological functions and lipid contents. The prolonged presence and slight increase in prometryn levels in some Asian clam tissues by day 35 could be linked to the sediment-bound prometryn, as clams interact closely with the sediment (Liu et al., 2018).
Initially, prometryn levels in the water of the E1 system were markedly lower than those of the E2 system. However, after the first day of exposure, the E1 system exhibited higher water concentrations of prometryn. This could be attributed to the combined bioturbation effects of fish movement and Asian clam activity in the sediment, which potentially remobilized prometryn adsorbed to the sediment back into the water (Peng et al., 2018). Over time, in ecosystems with introduced biota, the concentration of prometryn in the water gradually increased. This could be related to increased organic matter in the water due to biological metabolic waste from fish and Asian clams and various particulates, like uneaten bait, remaining in the water. And the increase in the organic matter could lead to greater adsorption of prometryn.
In ecosystems with introduced biota, the concentration of prometryn in the sediment showed a contrasting trend to that in water. Although the concentration of prometryn in the sediment decreased from the first day, it remained significantly higher than in the water, with levels ranging from 2.44 to 25.44 times greater. The decline in sediment concentration during this period may be related to the bioturbation caused by Asian clams, leading to the resuspension of sediments (Zhang et al., 2009).
At the end of the experiment, the concentrations of prometryn in the water and sediment of the E1 and E2 systems exhibited a slight variance; this may be due to the moderately weak migration ability of prometryn in sediments at relatively low concentrations (Cao et al., 2008). Nonetheless, the underlying mechanisms warrant further comprehensive investigation.
Despite extensive assessments of the environmental and health risks of prometryn in many countries and regions (USEPA, 1996b), research on its accumulation in simulated ecosystems has been limited. Our study reveals that prometryn not only accumulates in sediment but also in tilapias and Asian clams, posing potential risks to food safety. Some countries and regions have set maximum residue limits for prometryn in foods, varying from 0.01–9 mg/kg (Department of Food SafetyMinistry of HealthLabour and Welfare of Japan, 2006; USDA Foreign Agricultural Service, 2023). Our findings indicate that a single application of 0.5 mg/L prometryn in an aquatic system requires 7–14 days for the concentration in tilapias and Asian clams to fall below 0.01 mg/kg, which is the maximum residue limit (MRL) of prometryn in some agricultural products. While typical applications of prometryn may not lead to such high concentrations in water due to permeation, considering extreme scenarios and the illegal use of prometryn by some aquaculturists as a pond-cleaning agent, the environmental accumulation of prometryn deserves attention.
Recent studies have demonstrated that prometryn poses significant toxic effects on various aquatic animals, highlighting its environmental risks and potential harm to aquaculture. For instance, Huang et al. (2023) observed that a 20-day exposure to 0.5 mg/L prometryn and higher concentrations resulted in damage to the hepatopancreas and intestine of Eriocheir sinensis. Similarly, Wang et al. (2023) reported that exposure to 0.5, 5, and 50 μg/L prometryn delayed the first spawning and hatching times, reduced fecundity, and inhibited the population growth rate of Tigriopus japonicus. Additionally, Samreen et al. (2022) found that exposure to 1, 10, 100, and 1,000 μg/L prometryn caused significant malformations and decreased body length in newly hatched larvae of O. melastigma. Considering that environmental concentrations of prometryn as high as 627.5 μg/L have been reported Yang et al. (2019), which exceed the toxicity thresholds for many aquatic organisms mentioned above, the potential risks to aquatic animals and ecosystems are substantial and warrant increased attention. Based on our findings of prometryn bioaccumulation in tilapias and Asian clams, the environmental risks and potential threats to aquaculture posed by prometryn require further assessment. It is imperative that governing regulatory agencies establish standardized guidelines for the use of prometryn to prevent environmental accumulation and mitigate risks to aquatic ecosystems and public health (Zhang et al., 2024).
This study investigated the distribution and bioaccumulation of prometryn in simulated ecosystems with (E1) or without biota (E2). The findings demonstrate that prometryn persistently remains within both the water and sediment of these ecosystems, with the sediment acting as a significant reservoir for the herbicide. Additionally, the presence of aquatic organisms, specifically tilapias and Asian clams, not only served as accumulative pools for prometryn but also influenced its distribution dynamics within the ecosystems. In terms of bioconcentration, pronounced levels of prometryn were detected in the tissues of both tilapias and Asian clams. The highest bioconcentration factors were observed in the liver of tilapias and the visceral mass of Asian clams, suggesting a strong affinity of prometryn for these tissues. This finding highlights the importance of these organs in assessing the environmental and health impacts of prometryn exposure in aquatic species. The long-term ecological effects of prometryn should be concerned in the future research, particularly focusing on its impact on non-target aquatic organisms and the potential pathways through which it may influence human health, to inform more comprehensive environmental management and policy-making.
The original contributions presented in the study are included in the article/Supplementary Material, further inquiries can be directed to the corresponding author.
The animal study was approved by Fish Ethics Committee of Chinese Academy of Fishery Sciences, Beijing, China. The study was conducted in accordance with the local legislation and institutional requirements.
JP: Writing–original draft, Conceptualization, Data curation, Formal Analysis, Investigation, Methodology. RF: Conceptualization, Methodology, Data curation, Formal Analysis, Investigation, Writing–original draft. YR: Data curation, Investigation, Writing–original draft. YY: Conceptualization, Data curation, Formal Analysis, Investigation, Methodology, Writing–original draft. XZ: Data curation, Investigation, Writing–original draft. SW: Data curation, Investigation, Writing–original draft. YM: Conceptualization, Writing–review and editing. BC: Conceptualization, Funding acquisition, Methodology, Project administration, Writing–review and editing.
The author(s) declare that financial support was received for the research, authorship, and/or publication of this article. This research work was supported by Natural Science Foundation of Hainan Province, China (No. 321MS0808) and China Agriculture Research System (CARS-46). This work is supported by the Chinese Academy of Fishery Sciences - quality and standards research center and Beijing Fisheries Research Institute.
The authors declare that the research was conducted in the absence of any commercial or financial relationships that could be construed as a potential conflict of interest.
The author(s) declare that Generative AI was used in the creation of this manuscript. During the preparation of this work, the authors used ChatGPT 4 to fix grammatical and expression errors. After using this tool, the authors reviewed and edited the content as needed and take full responsibility for the content of the publication.
All claims expressed in this article are solely those of the authors and do not necessarily represent those of their affiliated organizations, or those of the publisher, the editors and the reviewers. Any product that may be evaluated in this article, or claim that may be made by its manufacturer, is not guaranteed or endorsed by the publisher.
The Supplementary Material for this article can be found online at: https://www.frontiersin.org/articles/10.3389/fenvs.2024.1511658/full#supplementary-material
Araujo, R., Moreno, D., and Ramos, M. (1993). The asiatic clam corbicula fluminea (müller, 1774) (Bivalvia: corbiculidae) in europe. Am. Malacol. Bull. 10.
Beulke, S., and Brown, C. D. (2001). Evaluation of methods to derive pesticide degradation parameters for regulatory modelling. Biol. Fertil. Soils 33, 558–564. doi:10.1007/s003740100364
Cao, J., Guo, H., Zhu, H. M., Jiang, L., and Yang, H. (2008). Effects of SOM, surfactant and pH on the sorption–desorption and mobility of prometryne in soils. Chemosphere 70, 2127–2134. doi:10.1016/j.chemosphere.2007.08.062
Caquet, T., Roucaute, M., Mazzella, N., Delmas, F., Madigou, C., Farcy, E., et al. (2013). Risk assessment of herbicides and booster biocides along estuarine continuums in the Bay of Vilaine area (Brittany, France). Environ. Sci. Pollut. Res. Int. 20, 651–666. doi:10.1007/s11356-012-1171-y
Chen, G., Lin, C., Chen, L., and Yang, H. (2010). Effect of size-fractionation dissolved organic matter on the mobility of prometryne in soil. Chemosphere 79, 1046–1055. doi:10.1016/j.chemosphere.2010.03.038
Dai, Y.-N., A, D., Yang, Y., Tam, N. F.-Y., Tai, Y.-P., and Tang, X.-Y. (2016). Factors affecting behavior of phenolic endocrine disruptors, estrone and estradiol, in constructed wetlands for domestic sewage treatment. Environ. Sci. Technol. 50, 11844–11852. doi:10.1021/acs.est.6b02026
Department of Food Safety, Ministry of Health, Labour and Welfare of Japan (2006). Positive list system for agricultural chemical residues in food. Available at: https://www.mhlw.go.jp/english/topics/foodsafety/positivelist060228/introduction.html (Accessed October 2, 2024).
Di, S., Diao, J., Wang, X., Qi, P., Wang, Z., Xu, H., et al. (2019). Bioaccumulation of dichlorodiphenyltrichloroethanes (DDTs) in carp in a water/sediment microcosm: important role of sediment particulate matter and bioturbation. Environ. Sci. Pollut. Res. Int. 26, 9500–9507. doi:10.1007/s11356-019-04426-5
Đikić, D. (2014). “Prometryn,” in Encyclopedia of toxicology. Editor P. Wexler Third Edition (Oxford: Academic Press), 1077–1081.
Dsikowitzky, L., Iveta Nguyen, T. M., Konzer, L., Zhao, H., Wang, D. R., Yang, F., et al. (2020). Occurrence and origin of triazine herbicides in a tropical coastal area in China: a potential ecosystem threat. Estuar. Coast. Shelf Sci. 235, 106612. doi:10.1016/j.ecss.2020.106612
El-Sherif, M., Mohamed, A., and El-Feky, I. (2009). Performance of nile Tilapia (Oreochromis niloticus) fingerlings. I. Effect of pH. Int. J. Agric. Biol. 11.
European Food Safety Authority (2009). Regulation - 1107/2009 - EN - EUR-lex. Available at: https://eur-lex.europa.eu/legal-content/EN/TXT/?uri=CELEX%3A32009R1107 (Accessed October 2, 2024).
Felis, E., Sochacki, A., and Magiera, S. (2016). Degradation of benzotriazole and benzothiazole in treatment wetlands and by artificial sunlight. Water Res. 104, 441–448. doi:10.1016/j.watres.2016.08.037
General Administration of Quality Supervision, I. and Q. of the P. R. of C. (2007). Determination of prometryn in food for import and export - GC/MS method.
Hand, L. H., Gougoulias, C., Bramke, I., Thomas, K. A., and Oliver, R. G. (2020). Evaluation of the rhizosphere contribution to the environmental fate of the herbicide prometryn. Environ. Toxicol. Chem. 39, 450–457. doi:10.1002/etc.4604
Huang, P., Cao, L., Du, J., Gao, J., Zhang, Y., Sun, Y., et al. (2023). Effects of prometryn exposure on hepatopancreas oxidative stress and intestinal flora in Eriocheir sinensis (Crustacea: Decapoda). Antioxidants 12, 1548. doi:10.3390/antiox12081548
Jacomini, A. E., Avelar, W. E. P., Martinêz, A. S., and Bonato, P. S. (2006). Bioaccumulation of atrazine in freshwater bivalves Anodontites trapesialis (Lamarck, 1819) and Corbicula fluminea (Müller, 1774). Arch. Environ. Contam. Toxicol. 51, 387–391. doi:10.1007/s00244-005-0238-x
Jiang, H. S., Yin, L., Ren, N. N., Xian, L., Zhao, S., Li, W., et al. (2017). The effect of chronic silver nanoparticles on aquatic system in microcosms. Environ. Pollut. 223, 395–402. doi:10.1016/j.envpol.2017.01.036
Kaune, A., Brüggemann, R., and Kettrup, A. (1998). High-performance liquid chromatographic measurement of the 1-octanol–water partition coefficient of s-triazine herbicides and some of their degradation products. J. Chromatogr. A 805, 119–126. doi:10.1016/S0021-9673(98)00051-X
Lang, P., Zhao, Y., and Ding, Y. (1990). Laboratory simulation for transport and transformation of organic pollutants in river. Environ. Chem., 12–18.
Li, B., Fan, Q., Zhang, L., Fang, W., Yang, Y., Sun, C., et al. (2009). Acute toxic effects of ammonia and nitrite on yellow catfish (Pelteobagrus fulvidraco) at different dissolved oxygen levels. Freshw. Fish. 39, 31–35.
Li, H., and Ran, Y. (2012). Distribution and bioconcentration of polycyclic aromatic hydrocarbons in surface water and fishes. Sci. World J. 2012, 1–14. doi:10.1100/2012/632910
Liu, L., Weiyun, W., Huawei, Z., Xiangyang, J., Lihua, R., Fang, J., et al. (2016). Accumulation and elimination of prometryn in ruditapes philippinarum. FOOD Sci. 37, 252. doi:10.7506/spkx1002-6630-201621043
Liu, W., Zeng, Z., Chen, A., Zeng, G., Xiao, R., Guo, Z., et al. (2018). Toxicity effects of silver nanoparticles on the freshwater bivalve Corbicula fluminea. J. Environ. Chem. Eng. 6, 4236–4244. doi:10.1016/j.jece.2018.06.032
Margoum, C., Malessard, C., and Gouy, V. (2006). Investigation of various physicochemical and environmental parameter influence on pesticide sorption to ditch bed substratum by means of experimental design. Chemosphere 63, 1835–1841. doi:10.1016/j.chemosphere.2005.10.032
National Center for Biotechnology Information (2021). PubChem compound summary for CID 4929, prometryn. Available at: https://pubchem.ncbi.nlm.nih.gov/compound/4929 (Accessed October 2, 2024).
Pan, K., Wang, W., and Zhu, D. (2024). Studies on acute toxicity of ammoniacal nitrogen, nitrite, and nitrate for Pseudorasbora parva. Chin. Agric. Sci. Bull., 96–101.
Parvin, A., Hossain, M., Islam, S., Das, S., Munshi, J., Suchi, P., et al. (2019). Bioaccumulation of heavy metals in different tissues of Nile tilapia (Oreochromis niloticus) in Bangladesh. Malays. J. Nutr. 25, 237–246. doi:10.31246/mjn-2018-0153
Peng, F.-J., Diepens, N. J., Pan, C.-G., Bracewell, S. A., Ying, G.-G., Salvito, D., et al. (2018). Fate and effects of sediment-associated triclosan in subtropical freshwater microcosms. Aquat. Toxicol. 202, 117–125. doi:10.1016/j.aquatox.2018.07.008
Rebouças, V., Lima, F., Cavalcante, D., and Carmo-e-Sá, M. (2016). Reassessment of the suitable range of water pH for culture of Nile tilapia Oreochromis niloticus L. in eutrophic water. Anim. Sci. 38, 361. doi:10.4025/actascianimsci.v38i4.32051
Rogers, H. A., Schmidt, T. S., Dabney, B. L., Hladik, M. L., Mahler, B. J., and Van Metre, P. C. (2016). Bifenthrin causes trophic cascade and altered insect emergence in mesocosms: implications for small streams. Environ. Sci. Technol. 50, 11974–11983. doi:10.1021/acs.est.6b02761
Saka, M., Tada, N., and Kamata, Y. (2018). Chronic toxicity of 1,3,5-triazine herbicides in the postembryonic development of the western clawed frog Silurana tropicalis. Ecotoxicol. Environ. Saf. 147, 373–381. doi:10.1016/j.ecoenv.2017.08.063
Samreen, , Zhang, X., Wang, J., Li, Y., Li, X., Zheng, Y., et al. (2022). Environmental relevant herbicide prometryn induces developmental toxicity in the early life stages of marine medaka (Oryzias melastigma) and its potential mechanism. Aquat. Toxicol. 243, 106079. doi:10.1016/j.aquatox.2022.106079
Sauder, D. C., and DeMars, C. E. (2019). An updated recommendation for multiple comparisons. Adv. Methods Pract. Psychol. Sci. 2, 26–44. doi:10.1177/2515245918808784
Shargel, L., and Yu, A. B. C. (2024). Applied biopharmaceutics and pharmacokinetics. Seventh Edition (7th ed. New York: McGraw-Hill Education. Available at: https://www.ebooks.com/en-us/book/2146871/applied-biopharmaceutics-pharmacokinetics-seventh-edition/leon-shargel/(Accessed October 2, 2024).
Sharma, A., Kumar, V., Shahzad, B., Tanveer, M., Sidhu, G. P. S., Handa, N., et al. (2019). Worldwide pesticide usage and its impacts on ecosystem. SN Appl. Sci. 1, 1446. doi:10.1007/s42452-019-1485-1
Shingala, M. (2015). Comparison of post hoc tests for unequal variance. Available at: https://www.semanticscholar.org/paper/Comparison-of-Post-Hoc-Tests-for-Unequal-Variance-Shingala/0b8a2ee4e38c6c4c41417bc30f4a1ccfb736451f (Accessed October 2, 2024).
Song, R. (2011). Studies on Cyanobacterial Bloom-Producing ammonia and the biological effects on typical aquatic organisms. Nanjing: Nanjing University.
Stara, A., Kristan, J., Zuskova, E., and Velisek, J. (2013). Effect of chronic exposure to prometryne on oxidative stress and antioxidant response in common carp (Cyprinus carpio L.). Pesticide Biochem. Physiology 105, 18–23. doi:10.1016/j.pestbp.2012.11.002
Su, L., Cai, H., Kolandhasamy, P., Wu, C., Rochman, C. M., and Shi, H. (2018). Using the Asian clam as an indicator of microplastic pollution in freshwater ecosystems. Environ. Pollut. 234, 347–355. doi:10.1016/j.envpol.2017.11.075
Sun, X., Xing, L., Xing, J., Zheng, X., Liu, J., Peng, J., et al. (2023). Variation and characterization of prometryn in oysters (Crassostrea gigas) after seawater exposure. Sci. Total Environ. 897, 165375. doi:10.1016/j.scitotenv.2023.165375
The Japan Food chemical Research Faundation (2015). Maximum residue limits (MRLs) list of agricultural chemicals in foods.
The Ministry of Agriculture of the People’s Republic of China (2010). The Ministry of agriculture of the People’s Republic of China announcement. Available at: http://www.moa.gov.cn/gk/tzgg_1/gg/201008/t20100823_1622639.htm (Accessed October 2, 2024).
Thit, A., and Selck, H. (2021). Biodynamics and adverse effects of CuO nanoparticles and CuCl2 in the oligochaete T. tubifex: Cu form influence biodynamics in water, but not sediment. Nanotoxicology 15, 673–689. doi:10.1080/17435390.2021.1913657
Tian, X.-H., Gong, X.-H., Xu, Y.-J., Ren, C.-B., Liu, H.-H., Liu, Y.-H., et al. (2013). Accumulation and elimination of prometryn in apostichopus japonicus. Mod. Food Sci. Technol. 29, 1580–1585. doi:10.13982/j.mfst.1673-9078.2013.07.053
USDA Foreign Agricultural Service (2023). Maximum residue limits (MRL) database. Available at: https://bcglobal.bryantchristie.com/db#login (Accessed October 2, 2024).
Vidal, M.-L., Bassères, A., and Narbonne, J.-F. (2002). Influence of temperature, pH, oxygenation, water-type and substrate on biomarker responses in the freshwater clam Corbicula fluminea (Müller). Comp. Biochem. Physiology Part C Toxicol. and Pharmacol. 132, 93–104. doi:10.1016/S1532-0456(02)00051-0
Vryzas, Z., Alexoudis, C., Vassiliou, G., Galanis, K., and Papadopoulou-Mourkidou, E. (2011). Determination and aquatic risk assessment of pesticide residues in riparian drainage canals in northeastern Greece. Ecotoxicol. Environ. Saf. 74, 174–181. doi:10.1016/j.ecoenv.2010.04.011
Wang, D., Han, B., Li, S., Cao, Y., Du, X., and Lu, T. (2019). Environmental fate of the anti-parasitic ivermectin in an aquatic micro-ecological system after a single oral administration. PeerJ 7, e7805. doi:10.7717/peerj.7805
Wang, D., Yang, G., Ru, S., Zhang, Z., Li, Y., and Wang, J. (2023). Herbicide prometryn adversely affects the development and reproduction of Tigriopus japonicus by disturbing the ecdysone signal pathway and chitin metabolic pathway. Aquat. Toxicol. 254, 106378. doi:10.1016/j.aquatox.2022.106378
Wauchope, R. D., Buttler, T. M., Hornsby, A. G., Augustijn-Beckers, P. W., and Burt, J. P. (1992). The SCS/ARS/CES pesticide properties database for environmental decision-making. Rev. Environ. Contam. Toxicol. 123, 1–155. doi:10.1007/978-1-4612-2862-2_1
Wu, J., Zhang, W., Li, C., and Hu, E. (2021). Effects of Fe(III) and Cu(II) on the sorption of s-triazine herbicides on clay minerals. J. Hazard. Mater. 418, 126232. doi:10.1016/j.jhazmat.2021.126232
Wu, Y., Yang, C., Tang, T., Guo, X., and Dang, Z. (2015). Distribution of erythromycin in aquatic ecosystem: microcosm study. Huanjing Kexue Xuebao/Acta Sci. Circumstantiae 35, 897–902. doi:10.13671/j.hjkxxb.2014.0833
Yang, L., Li, H., Zhang, Y., and Jiao, N. (2019). Environmental risk assessment of triazine herbicides in the Bohai Sea and the Yellow Sea and their toxicity to phytoplankton at environmental concentrations. Environ. Int. 133, 105175. doi:10.1016/j.envint.2019.105175
Yang, Y., Li, S., Wang, Z., Ren, Y., Mu, Y., Zhang, X., et al. (2022). Acute toxicity, bioaccumulation and elimination of prometryn in tilapia (Oreochromis niloticus). Chemosphere 300, 134565. doi:10.1016/j.chemosphere.2022.134565
Yu, C., Wang, R., Zhang, J., Jiang, H., and Lin, R. (2012). Primary aquatic risk assessment of pesticides in European commission: bioconcent-ration factor. Pesticide Sci. Adm. 33, 57–60.
Zhang, C.-Y., Zhou, X.-Z., Chen, J., and Nie, X.-P. (2009). Dynamics and fate of ciprofloxacin in a simulated aquatic system. J. Ecol. Rural Environ. 25, 73–78.
Zhang, K.-W., Zhang, H.-Y., Kong, C., Gu, R.-R., Tian, L.-L., Yang, G.-X., et al. (2024). Exposure level and risk impact assessment of pesticides and veterinary drugs in aquaculture environment. Huan Jing Ke Xue 45, 151–158. doi:10.13227/j.hjkx.202212160
Zhang, Q., and Wu, W. (2014). “Research advance in eco-toxical effects and microbial degradation of prometryne in aquaculture water,” in Biological disaster science. Available at: https://www.semanticscholar.org/paper/Research-Advance-in-Eco-toxical-Effects-and-of-in-Qian-yu/67962832926a8f0ad5425b15d4932338bac87598 (Accessed October 2, 2024).
Zhao, Q., and Zhu, L. (2016). Effect of humic acid on prometryn bioaccumulation and the induction of oxidative stress in zebrafish (Danio rerio). RSC Adv. 6, 16790–16797. doi:10.1039/C5RA21488B
Keywords: prometryn, tilapia (Oreochromis niloticus), Asian clam (Corbicula fluminea), simulated aquatic ecosystems, distribution, bioaccumulation
Citation: Peng J, Fan R, Ren Y, Yang Y, Zhang X, Wang S, Mu Y and Cheng B (2025) Distribution and bioaccumulation of prometryn in simulated aquatic ecosystems. Front. Environ. Sci. 12:1511658. doi: 10.3389/fenvs.2024.1511658
Received: 15 October 2024; Accepted: 13 December 2024;
Published: 06 January 2025.
Edited by:
Fayuan Wang, Qingdao University of Science and Technology, ChinaReviewed by:
Xiaoyan Lin, Chinese Academy of Agricultural Sciences, ChinaCopyright © 2025 Peng, Fan, Ren, Yang, Zhang, Wang, Mu and Cheng. This is an open-access article distributed under the terms of the Creative Commons Attribution License (CC BY). The use, distribution or reproduction in other forums is permitted, provided the original author(s) and the copyright owner(s) are credited and that the original publication in this journal is cited, in accordance with accepted academic practice. No use, distribution or reproduction is permitted which does not comply with these terms.
*Correspondence: Bo Cheng, Y2hlbmdiQGNhZnMuYWMuY24=
†These authors have contributed equally to this work
Disclaimer: All claims expressed in this article are solely those of the authors and do not necessarily represent those of their affiliated organizations, or those of the publisher, the editors and the reviewers. Any product that may be evaluated in this article or claim that may be made by its manufacturer is not guaranteed or endorsed by the publisher.
Research integrity at Frontiers
Learn more about the work of our research integrity team to safeguard the quality of each article we publish.