- 1Department of Ecoscience and Arctic Research Centre, Aarhus University, Roskilde, Denmark
- 2Faculty of Environmental and Forest Sciences, Agricultural University of Iceland, Borgarnes, Iceland
- 3Department of Biology, Aarhus University, Aarhus, Denmark
- 4Leverhulme Centre for Nature Recovery, University of Oxford, Oxford, United Kingdom
- 5Department of Environment and Minerals, Greenland Institute of Natural Resources, Nuuk, Greenland
The rapid climatic and environmental changes observed in the Arctic and across the globe in general call for reliable model projections. In recent years our understanding of ongoing and future changes through ecosystem modelling has increased tremendously. Yet, most ecosystem models do not consider many of the feedback loops at play in natural ecosystems. Particularly those influenced by biota, beyond vegetation and to some extent microbes, are often neglected. As a first step towards a better integration of biotic influences into ecosystem models, we provide a broad overview of the various ways biota may influence feedback loops between the high-latitude biosphere and the atmosphere. We focus specifically on three key feedback loops between tundra and atmosphere (carbon dynamics, albedo and permafrost thaw) and the influences of three key ecosystem compartments (vegetation, decomposers and herbivores) on these. The influences of biota on ecosystem feedback loops are multifaceted and may appear patchy in both space and time. However, biota may still play important roles in modulating ecosystem feedback loops, and by including these dynamics into ecosystem models, magnitude, accuracy and credibility of model projections are likely to improve.
1 Introduction
Nowhere else is climate change more apparent than in the Arctic (IPCC, 2021). Because of the long-term and gradual increase in surface air temperatures, loss of snow cover, and permafrost thaw associated with arctic amplification concentrating heat at the poles (Previdi et al., 2021), the arctic biophysical system is now experiencing unprecedented change (Box et al., 2019; Post et al., 2019). In addition to the gradual changes in climate, the Arctic is also experiencing an increase in the intensity, frequency and duration of extreme or erratic events that are directly or indirectly related to weather and climate (Christensen et al., 2021; Descals et al., 2022; Landrum and Holland, 2020; van Beest et al., 2022). The combined impacts of gradual and erratic climate change on the structure and functioning of the biotic components in tundra ecosystems is suspected to have repercussions that extend far beyond the Arctic (Box et al., 2019), not least due to the vast amounts of carbon stored in the circumarctic permafrost region (Palmtag et al., 2022). Over the past few decades, observations and modeling studies have provided compelling evidence that the northernmost regions of the world are experiencing some of the most pronounced and rapid changes in climatic conditions, resulting in pervasive changes to the structure and functioning of the biotic components of the ecosystems. Such changes can in turn influence the magnitude of the feedback loops between the tundra ecosystem and the climate system (Box et al., 2019; Post et al., 2019) that may either amplify or diminish the warming trend. The changes observed in high-latitude ecosystems may therefore have global implications.
Tundra ecosystems are characterized by low productivity, low nutrient availability and low species diversity (Callaghan et al., 2013), yet biotic interactions in the Arctic may still be rather complex (Schmidt et al., 2017). Exposed to the dramatic climatic changes in the northernmost parts of the world, the impacts on tundra ecosystems are numerous, ubiquitous and well-documented, and include changes in phenology (Parmesan, 2007; Roslin et al., 2021; Schmidt et al., 2023), demographic rates (Descamps et al., 2017; Iler et al., 2021; Schmidt et al., 2019) and shifts in distributional ranges (Gilg et al., 2012; Parmesan and Yohe, 2003; van Beest et al., 2023). High-latitude organisms, however, are not only responding to the changing climatic conditions: their physical properties, life histories and behaviors are also influencing processes of importance to the interplay between the tundra ecosystem and the atmosphere. However, compared to other parameters influencing feedback loops, such as the general global warming and changes in precipitation patterns (Bintanja and Andry, 2017; Rantanen et al., 2022), biotic influences are oftentimes patchier in both space and time. Yet, biotic interactions are still key determinants of how ecosystems respond to climate change (Blois et al., 2013; Post et al., 2023).
In this paper, we highlight the importance of biotic processes in amplifying or diminishing feedback loops between tundra ecosystems and the climate system. In doing so, we hope to guide future climate and ecosystem modelling studies in developing more accurate predictions of potential future trajectories of arctic ecosystems, which are currently hampered by our inability to adequately integrate the bidirectional and highly dynamic interplay between biotic and abiotic ecosystem components (Ripple et al., 2023). We synthesize current knowledge on key feedback loops between tundra ecosystems and the climate system operating in high-latitude regions, focusing on how these may be influenced by biotic processes. Specifically, we target three key feedback loops (albedo, carbon dynamics and permafrost thaw), each representing critical components of the high-latitude system and to its overall dynamics of change. In the following sections, we explore how biotic processes may influence each of these key feedback loops, directly as well as indirectly. By providing a broad overview of these processes, we aim to contribute to a better understanding of the key roles biotic interactions play in shaping tundra ecosystem responses to climatic changes, with particular focus on key processes that feed back to the global climate system.
2 Biotic processes relevant to feedback loops
The interplay between biota and albedo, carbon dynamics, and permafrost thaw in arctic ecosystems has profound implications for the global climate system. In the Arctic, surface reflectivity (i.e., albedo) is particularly important for this dynamic relationship, as diminishing ice and snow cover, as well as shifts in vegetation types towards taller, darker vegetation, contribute to increased absorption of solar radiation and increasing soil temperature, which in turn accelerates permafrost thaw. As the permafrost thaws, organic carbon sequestered over thousands of years becomes accessible to soil organisms. Organic matter decomposition in cold environments responds exponentially to increasing temperatures, hence driving a very effective conversion of soil organic matter to carbon dioxide (CO2) or methane (CH4), depending on the oxygen availability to the microbes, which is ultimately released to the atmosphere (García-Palacios et al., 2021; Maes et al., 2024). This creates a positive feedback loop that further amplifies warming. Simultaneously, surface reflectance is influenced by vegetation dynamics such as shifts in vegetation composition and plant phenology, which affects the energy balance directly (albedo, exchange of latent and sensible heat) and indirectly through the exchange of carbon across the soil-plant-atmosphere continuum. Hence, it is evident that biotic influences on ecosystem feedback loops are multifaceted and interlinked and that biotic influences may cascade through the ecosystem. Figure 1 summarizes the main interactions between the three main biotic compartments of the tundra ecosystem (decomposers, vegetation and herbivores) and their influences on the three key feedback loops examined in this paper.
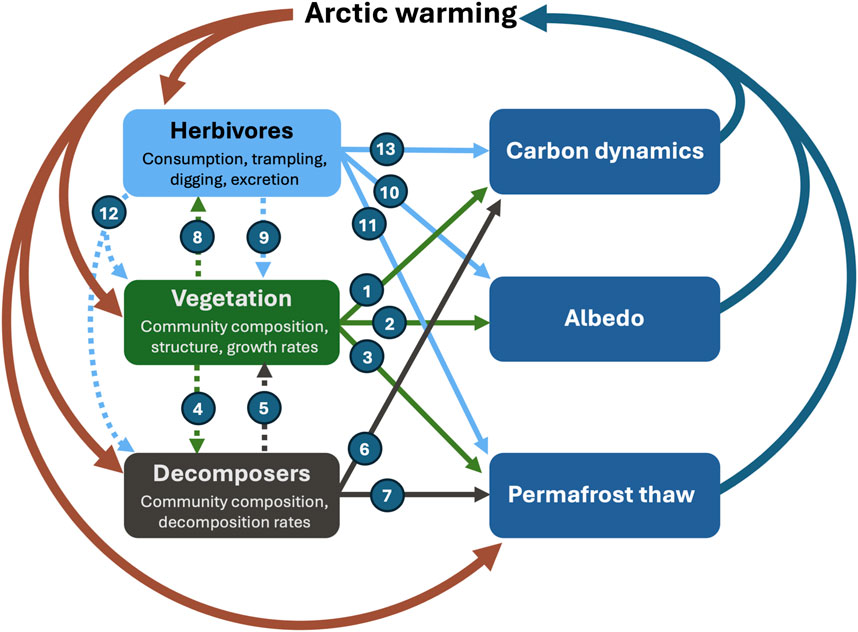
Figure 1. Conceptual figure showing the main processes linking the three key biotic components (decomposers, vegetation, herbivores) and the three key feedback loops (carbon dynamics, albedo, permafrost thaw) from the Arctic terrestrial ecosystem to the atmosphere. Full lines indicate direct influences of biota on feedback loops, while dotted lines indicate indirect influences of biota on feedback loops. Main processes include: 1. Vegetation community composition influencing carbon dynamics via photosynthesis and respiration rates; 2. Physical properties of vegetation community composition and structure influencing surface albedo; 3. Vegetation community composition and structure influencing snow-pack insulation capacity regulating soil temperatures and ultimately permafrost thaw; 4. Vegetation community composition influencing litter composition and in turn decomposer community and turn-over rates; 5. Soil community composition and process rates influencing nutrient availability to plants; 6. Decomposer community composition and decomposition rates influencing respiration rates; 7. Decomposer community decomposition rates influencing permafrost thaw; 8. Vegetation community composition influencing herbivore communities; 9. Herbivores influencing vegetation community composition and biomass; 10. Animals influencing surface albedo via trampling, digging and cratering; 11. Animals influencing permafrost thaw via disruption of snow cover and ultimately soil temperatures; 12. Animals influencing nutrient availability to the decomposer community via excretion and carcasses; 13. Herbivores influencing carbon dynamics via enteric methane emissions and respiration.
3 Effects of vegetation on climate feedback loops
Arctic vegetation is characterized by low diversity, low stature and low productivity, owing to the generally harsh climatic conditions, short growing seasons and low availability of nutrients (Callaghan et al., 2004). Still, arctic vegetation plays a key role in the sequestration of carbon and forms a key interface between the ecosystem and the climate system (Epstein et al., 2012; Pearson et al., 2013), acting both as a carbon sink (photosynthesis) and as a carbon source (respiration).
3.1 Vegetation and carbon dynamics
During the growing season, the vegetation absorbs atmospheric carbon dioxide and converts it into organic carbon through the dominance of photosynthesis over respiratory losses (Figure 1: process 1). Arctic vegetation encompasses a diverse array of taxa, including mosses, lichens, shrubs, and graminoids, each exhibiting distinct carbon sequestration capacities and mechanisms (Huemmrich et al., 2013). Mosses and lichens, often forming a substantial part of arctic vegetation communities, both in terms of species richness and cover, have relatively low growth rates and biomass compared to vascular plants, such as shrubs and graminoids. As a result, they typically sequester less carbon from the atmosphere through photosynthesis during the main growing season. However, their ability to photosynthesize even at low ambient temperatures (Barták, 2014) and ability to supply themselves with nitrogen directly from the atmosphere (Rousk et al., 2017), suggests that they contribute significantly to the overall carbon balance in the Arctic (Street et al., 2012). Conversely, with their higher growth rates and biomass compared to mosses and lichens (Mekonnen et al., 2021), shrubs have a higher capacity to take-up carbon during the growing season (Andreu-Hayles et al., 2020). Because of the changing climatic conditions and the concomitant environmental changes (Box et al., 2019), shrubs are currently expanding across the Arctic (Myers-Smith et al., 2011), particularly in the low arctic regions (Elmendorf et al., 2012a) and at the boundary between the low and high Arctic (Myers-Smith et al., 2015), where permafrost thaw is most extensive (IPCC, 2021). The expansion of shrubs is oftentimes at the expense of lichens and mosses as well as bare ground (Elmendorf et al., 2012a; Elmendorf et al., 2012b). This may affect the tundra carbon balance by enhancing ecosystem carbon uptake and altering ecosystem respiration (Figure 1: process 1), but also through complex feedback mechanisms involving snowpack dynamics and root-microbe interactions accelerating permafrost degradation (Mekonnen et al., 2021; Parker et al., 2021) (Figure 1: process 3). Overall, vegetation carbon stock has increased with global warming, and is predicted to increase further over the coming decades as warming continues. The increase, sometimes referred to as arctic greening, is however by no means homogeneous in space and time. In fact, significant portions of the Arctic have seen a browning trend (i.e., decrease in productivity) over the last 2 decades (Myers-Smith et al., 2020).
Vegetation change can also fundamentally reshape soil food webs (Figure 1: process 4). As the gatekeeper of carbon entering the system, vegetation composition determines the amount and composition of plant-derived carbon inputs, both through litter and root-exudates (Elmendorf et al., 2012b). As dead plant material constitutes the major component of the energy-base of the decomposer community, changes in plant litter availability and quality alter soil microbial community composition and decomposition rates (Adamczyk et al., 2020; Cleveland et al., 2014; McLaren et al., 2017; Saunders et al., 2023) (Figure 1: process 4). For example, shrub-expansion can increase carbon flows through the decomposer food web to higher trophic levels. This is sometimes referred to as a ‘browning’ of the soil food web (Manlick et al., 2024), and is partly a consequence of the chemical composition and amounts of litter entering the soil. The mere amount of litter is important for the decomposer community composition and energy flows (Adamczyk et al., 2020). An often-overlooked pathway of plant-soil carbon transfer is root exudation, although it can constitute up to half of the soil respiration in arctic ecosystems during the growing season (Parker et al., 2021), and has been estimated to amplify the soil carbon respiration by 12% from permafrost-affected ecosystems (Keuper et al., 2020). While similar dramatic losses of soil carbon have been found as a response to fertilizer-addition (Mack et al., 2004), arctic ecosystems also possess compensatory mechanisms, and over long timespans the carbon lost in one pool may be gained in others. For instance, a study from the Alaskan tundra showed warming resulted in a restructuring of carbon from the upper soil horizon towards the mineral horizon underneath, resulting in no net loss in soil carbon (Sistla et al., 2013). Seeing these two studies together (Sistla et al., 2013; Mack et al., 2004), highlights the importance of accounting for carbon dynamics below the topsoil horizon to establish reliable long-term ecosystem carbon balances to validate models. Further, they highlight the caution needed when making general inferences about a process with multiple interacting knock-on effects, such as global warming, to its single components, e.g., increased nutrient availability (Mack et al., 2004). While studying the pathways separately is important for our mechanistic understanding, Sistla et al. (2013) clearly show that by doing so, we risk missing some important compensatory long-term feedbacks.
3.2 Vegetation and surface albedo
Vegetation change affects the surface energy budget of arctic landscapes through changing surface reflectance because of vegetation penetrating the snow surface and altered snow characteristics (Loranty and Goetz, 2012) (Figure 1: process 2). As compared to snow, vegetation has a much lower albedo, and tall shrubs protruding the snowpack increase the absorption of solar radiation (Sturm et al., 2005). During the snow-free season, surface albedo depends on the community composition of the vegetation (Riedel et al., 2005), the relative abundance of different vegetation types (Oehri et al., 2022) and their phenology (Williamson et al., 2016). However, the contribution of vegetation changes to summer albedo, and ultimately to arctic summer warming, is limited compared to changes in duration of the snow-covered period (Chapin et al., 2005).
Other biologically induced changes in albedo have received less attention, yet they may still have profound effects on the energy balance. For instance, the presence of pigmented algae on ice and snow reduces the albedo markedly and contributes to enhanced melting rates (Hotaling et al., 2021).
3.3 Vegetation and permafrost thaw
Changes in vegetation structure and composition can affect permafrost thaw (Figure 1: process 3) through their influence on surface thermal regimes via shading in summer (Schuuring et al., 2024) and through snow accumulation in winter (Heijmans et al., 2022). For example, tall shrubs tend to accumulate more snow (Sturm et al., 2001), which in turn alters the temperature regime in the soil (Zhang, 2005) and snow depletion patterns, thereby altering permafrost thaw but also plant phenology (Wilcox et al., 2019). Thawing permafrost in itself can influence tundra vegetation change, with gradual permafrost thaw promoting plant growth through increased soil nutrient availability (Keuper et al., 2012). The development of thermokarsts creates landscape deformations and alters ground surface conditions, like soil temperature and moisture or depth of the active layer, promoting shrub growth (Mekonnen et al., 2021). However, in some cases abrupt permafrost thaw may lead to local plant mortality due to increased draining (Osterkamp et al., 2009).
4 Effects of decomposers on climate feedback loops
The main impacts of the decomposer community on climate feedback loops are on the carbon flux (Figure 1: process 6) and to some extent the permafrost thaw feedback loop (Figure 1: process 7), whereas direct impacts on albedo are absent.
4.1 Decomposers and carbon dynamics
The Arctic holds vast amounts of carbon (Hugelius et al., 2014), but also nutrients such as nitrogen (Palmtag et al., 2022), locked in permafrost. During the growing season the thaw of the uppermost parts of the soil, the active layer, allows for biological processes to unfold, including the mineralization of organic matter by decomposers with resulting respiratory carbon dioxide release to the atmosphere. These processes will only be amplified as warming progresses (Maes et al., 2024). As described above, shrubification may fuel the decomposition of old carbon accumulated over millennia by increased root growth expanding the root zone and associated rhizosphere processes into soil layers that were previously free of or poor in active roots. This boost of the rhizosphere microbes can both drive a reduction in soil carbon (Keuper et al., 2020) or restructuring of soil carbon with no net loss (Sistla et al., 2013), depending on the context. Microbes are also essential for the overall nitrogen cycle through nitrogen fixation (Rousk et al., 2018), as well as nitrification and denitrification processes (Crowther et al., 2015; Wang et al., 2020). Thawing permafrost can also increase the water saturation of soils over varying spatiotemporal scales, which can inhibit immediate mineralization of organic matter, but drive an increase in methane emissions (Rößger et al., 2022). Thus overall, nutrient and water availability and their spatiotemporal distributions are key parameters for predicting the land-atmosphere exchange of greenhouse gases as ecosystems continue to warm (See et al., 2024). This results in a feedback of accelerated decomposition of organic matter and release of greenhouse gases to the atmosphere (Altshuler et al., 2019).
Most of the organic matter assimilated by decomposers is respired back to the atmosphere as carbon dioxide relatively fast (Figure 1: process 6). In cold systems, typically only a small fraction of the assimilated carbon is turned into microbial biomass (Hicks et al., 2022). Nonetheless, over long periods over time, this seemingly small fraction is an important source of carbon ending up in association with mineral particles. Mineral-associated organic carbon covers carbon that is chemically protected against decomposers due to adsorption to secondary minerals, e.g., iron-oxides and clay, and physically protected in soil aggregates leading to effective long-term carbon preservation. As chemical weathering rates are slow in cold regions (Brantley et al., 2023), concentrations of secondary minerals, such as clay and iron-oxides, are typically low. Further, the lack of soil engineering meso- and macrofauna, such as earthworms, across large parts of the Arctic (Blume-Werry et al., 2020) leads to limited formation of protective aggregates (Angst et al., 2023; Angst et al., 2024). Consequently, arctic soils are dominated by particulate organic matter relatively vulnerable to perturbations and changing abiotic conditions (García-Palacios et al., 2024). Thus, the observed warming-induced increase in arctic ecosystem respiration (Maes et al., 2024) and soil respiration (García-Palacios et al., 2021) is attributed to the combination of improved kinetics under warming and increased availability of highly decomposable carbon in thawing permafrost (Trumbore, 2009). Functional limitations of the decomposer community can occur when old organic compounds ‘exotic’ to the present community thaw from the permafrost, yet, the community usually adjusts relatively fast (Monteux et al., 2020). Hence, thawed permafrost carbon is generally quite bioavailable and hence vulnerable to decomposition (García-Palacios et al., 2024; Kuhry et al., 2020).
4.2 Decomposers and permafrost thaw
Decomposer animals and microbes in soils obtain energy by breaking down dead organic matter from, e.g., plant litter (Figure 1: process 4) or animal excreta and carcasses (Figure 1: process 12). Gradually, organic material is broken down into simple organic compounds (e.g., simple sugars, amino acids, etc.) or all the way to inorganic minerals (mineralization). This releases important plant nutrients, including nitrogen, phosphorus, and potassium, to the soil solution, where it is available for plant uptake via roots and/or their symbiotic microbes (Figure 1: process 5). However, the activity of soil microbes produce heat, which in itself may accelerate permafrost degradation further (Hollesen et al., 2015) (Figure 1: process 7), and may even be sufficient to sustain the decomposition process during periods of low ambient temperatures (Khvorostyanov et al., 2008).
5 Effects of herbivores on climate feedback loops
How interactions between animals, plants and soils shape ecosystem ecology, biogeochemical processes and climate feedback loops have long been a subject of interest and dispute. Generally, animals affect elemental cycling via two direct and one indirect pathway: directly through physical disturbance (e.g., trampling, Tuomi et al., 2021), and deposition of waste products like dung and urine (Van Der Wal et al., 2004), and indirectly by restructuring the vegetation community (e.g., by selective feeding, Post et al., 2023; Post et al., 2022). Herbivores can counteract climate-induced changes in the Arctic, such as the release of nutrients (Petit Bon et al., 2023), changes to plant community composition (Post and Pedersen, 2008), and the expansion of woody species and the advancement of the tree line (Christie et al., 2015; Olofsson et al., 2009; Speed et al., 2010), leading to complex interactions. The indirect effects of herbivores on climate feedback loops through vegetation are well established, for example, the climate-effects of reducing shrub expansion into the tundra (reviewed in Olofsson and Post, 2018), but less attention has been given to the direct pathways.
Herbivores depend on plants as a resource (Figure 1: process 8), and one of the main mechanisms through which herbivores influence climate feedback loops is through the removal or damage of plant material while foraging (Tanentzap and Coomes, 2012). Plant consumption not only impacts the plants themselves but also influences plant traits, such as height, cover, biomass and the composition of plant communities (Figure 1: process 9), which in turn affects the carbon dynamics. By selective foraging and by altering the competitive interactions between plant species, herbivores influence the species composition of plant communities. This is for instance seen as a reduction in the abundance of the most palatable plants (Olofsson et al., 2001), or reduced shrub dominance increasing the plant diversity (Post et al., 2023). Nonetheless, in heavily grazed areas, grazing tends to reduce plant species richness, shifting towards a graminoid-dominated vegetation (Ylänne et al., 2018). Hence, tundra ecosystems appear to follow the general predictions of the intermediate grazing hypothesis predicting the highest plant diversities at intermediate grazing pressure. Yet, a recent meta-analysis suggests that a more climate-specific relationship might better catch differences in the grazing-diversity relationship between dry (decreasing) and wet (hump-shaped) areas (Gao and Carmel, 2020). Further, the effects of herbivores on plant communities are not only determined by the grazing intensity but is highly dependent on the functional traits of the herbivore community (Barbero-Palacios et al., 2024; Lundgren et al., 2024).
Herbivores not only impact the organisms they consume, but they also move around biomass, nutrients, seeds and more (Figure 1: process 12). For instance, when herbivores forage in one area and urinate and defecate in another area, nutrients are redistributed around the landscape. In the nutrient-limited Arctic, such translocation of nutrients can be considerable (Mosbacher et al., 2016) and can, at least at the local scale, impact vegetation composition markedly (Van Der Wal et al., 2004). During the process of decomposing the feces, nutrients become available to both soil microbes and plants (Beard et al., 2023) (Figure 1: process 12). Nutrients in urine on the other hand are directly accessible to both the soil microbes (Barthelemy et al., 2024) and the plants (Barthelemy et al., 2018) (Figure 1: process 12). Depending on the size and ecology of the animal, nutrients may be relocated only locally or across large distances. For example, small animals like lemmings, only move short distances and utilize latrine sites (Klein and Bay, 1991), which creates very localized hotspots of nutrients (Roy et al., 2022). Ultimately, when an herbivore dies, the parts of the carcass that are not consumed by predators or scavengers (Johnson-Bice et al., 2023; Schmidt et al., 2022) are decomposed (Figure 1: process 12). Locally, this results in a substantial release of nutrients (Danell et al., 2002). The impact of larger carcasses may last for several years and may increase the nutrient levels in the soil (Steger, 2023), which in the nutrient-poor arctic tundra leads to increased vegetation surrounding the carcass (Danell et al., 2002) and to changes in the community composition of the vegetation (Steger, 2023). Calving also releases nutrients into the environment and has recently been suggested as an important feedback mechanism for the creation of fertile, revisited calving grounds for reindeer (Ferraro et al., 2024). Nutrient-translocation effects, however, are not limited to herbivores. For instance, the contribution of excreta and prey remains turns Arctic fox den complexes into green islands on the tundra (Johnson-Bice et al., 2023), and marine birds nesting in colonies on land bring in large amounts of “new” nutrients to the terrestrial ecosystem (Otero et al., 2018). The impacts of nutrient additions cascade through the terrestrial food web (González-Bergonzoni et al., 2017), affecting other biotic components (Figure 1: process 12), ultimately influencing climate feedback loops.
Most of our knowledge on the impacts of herbivory in tundra ecosystems refers to vertebrate herbivores, whilst invertebrate herbivory has received far less attention (Barbero-Palacios et al., 2024; Soininen et al., 2021). Invertebrate herbivory at non-outbreak densities, i.e., background herbivory, is widespread across the tundra biome (Barrio et al., 2017; Rheubottom et al., 2019), yet removes only a small proportion of leaf biomass (Kozlov et al., 2015) and its impact on carbon cycling is minor (Kristensen et al., 2020a). However, during population outbreaks invertebrate herbivores can significantly accelerate soil nutrient and carbon cycling resulting in substantial decreases in plant productivity (Kristensen et al., 2020b; Lund et al., 2017). The same is true for, e.g., cyclic populations of small mammals (Roy et al., 2022). Another important aspect to consider regarding the impacts of herbivores on climate feedbacks is that many animals move around and undertake long-distance migrations, carrying along the processes by which they influence the environment (Lundberg and Moberg, 2003; Schmitz et al., 2018). We therefore need a better characterization of the spatial and temporal variability of herbivore impacts to fully understand the changing functional roles of herbivores in arctic ecosystems (Koltz et al., 2022).
5.1 Herbivores and carbon dynamics
The effects of herbivores on vegetation can be substantial (Cahoon et al., 2012; Koltz et al., 2022; Vaisanen et al., 2014) (Figure 1: process 9), ultimately affecting carbon dynamics within the ecosystem through changing carbon stocks and greenhouse gas exchange (Falk et al., 2015; Fischer et al., 2022; Min et al., 2021; Petit Bon et al., 2023) (Figure 1: process 1). However, in addition to the effects mediated by their impacts on other biotic components, herbivores have a direct impact on carbon fluxes through the methane they produce as part of their digestive processes (Clauss et al., 2020). Ruminants, such as reindeer and musk, in particular produce large amounts of methane, thereby releasing carbon directly back to the atmosphere (Figure 1: process 13). In addition, arctic ruminants excrete methane-producing microbes in their feces (Aggerbeck et al., 2022; Andersen-Ranberg et al., 2018; Fritze et al., 2021), which can increase methane production in the soil (Fritze et al., 2021) (Figure 1: process 5).
5.2 Herbivores and surface albedo
Through their impacts on vegetation, herbivores can also contribute to changes in surface albedo (Figure 1: process 10). For example, grazing by reindeer can reduce shrub height and abundance, which increases albedo during the summer (Te Beest et al., 2016). Shorter and sparser vegetation in heavily grazed areas results in delayed snowmelt and increased surface albedo during the snowmelt season (Cohen et al., 2013) (Figure 1: process 2). Higher albedo reduces net energy absorption and the resulting latent and sensible heat fluxes, contributing to a cooling effect. However, herbivore impacts on albedo are mainly detected in areas with high herbivore densities where strong shifts in vegetation from shrub tundra to graminoid tundra have taken place (Te Beest et al., 2016). Heavy grazing by lemmings (Lara et al., 2017) and intense grubbing by geese (Peterson et al., 2013) can lead to the destruction of vegetation cover, exposing bare ground and resulting in lower albedo (Figure 1: process 2) and increased soil temperatures feeding back to enhanced decomposition and permafrost thaw rates.
5.3 Herbivores and permafrost thaw
Herbivores can indirectly influence climate feedback loops in tundra ecosystems through permafrost thaw (Figure 1: process 11). In the snow-covered period, animals may crater through the snowpack to access the vegetation underneath (Beumer et al., 2017; Schaefer and Messier, 1995), thereby reducing snow cover and depth whilst increasing its density (Beer et al., 2020). This reduces the insulative capacity of snow, thereby lowering soil temperatures during the arctic winter (Rixen et al., 2022). Indeed, evidence from Pleistocene Park in Siberia suggests that high densities of herbivores reduce permafrost thaw, by reducing snow depth and keeping soil temperatures low (Beer et al., 2020; Zimov, 2005). During the snow-free season, herbivore-induced changes in plant structure and community composition may also alter the temperature regime in the soil, with direct effects on permafrost thaw (Figure 1: process 3). Most obviously due to the shading-effects of woody vegetation during summers (Kropp et al., 2021), but herbivores can also significantly reduce the depth of the moss layer through trampling (Mosbacher et al., 2019). Both have implications for the temperatures in the soil and microbial activity (Gornall et al., 2007). Trampling can also compact soils, thereby altering the moisture regime in the soil (Tuomi et al., 2021). This in turn may reduce soil respiration and the release of carbon from the soil (Figure 1: process 6). Trampling and digging by animals may in some cases be (locally) pronounced, resulting in the destruction of the vegetation and exposure of bare ground, which in turn may influence several feedback loops. Hence, while animal trampling and digging alone may not directly cause permafrost thaw, they can exacerbate existing environmental factors that lead to thawing soils (Hall and Lamont, 2003).
6 Discussion and concluding remarks
Given the importance of biotic interactions in shaping ecosystem processes and responses (Barbero-Palacios et al., 2024; Blois et al., 2013; Koltz et al., 2022) and the multifaceted ways by which biota influence ecosystem feedback loops with the climate (this study), accurate predictions of the future trajectories of arctic ecosystems are hampered by our ability to adequately integrate the two-way interplay between biotic and abiotic components into climate and ecosystem models. Over the past decades, significant progress has indeed been made in climate and ecosystem modelling (see, e.g., Eyring et al., 2016; Fisher et al., 2018). Vegetation and microbial communities have long been recognized as an important bridge between the ecosystem and the climate and thus important components in climate and ecosystem models, but other biotic influences on key feedback loops are rarely incorporated into the models (but see Rizzuto et al., 2024). Many of the biotic influences examined in this study may indeed appear patchy in both space and time, yet their role in modulating feedback loops between the tundra ecosystems and the atmosphere can be important, particularly at long timescales. Indeed, the importance of zoogeochemical processes is increasingly documented and recognized (Schmitz et al., 2018), and natural biogeochemical processes are estimated to remove as much as half of anthropogenic carbon emissions across terrestrial and marine ecosystems globally (Schmitz et al., 2023). Inclusion of biotic influences in ecosystem models may therefore improve the accuracy of ecosystem-climate models.
Many of the biotic drivers and processes influencing feedback loops highlighted here can operate on time scales ranging from short (e.g., months or years) to very long (e.g., millennia) and on spatial scales ranging from fine (e.g., km2) to very large (e.g., continental) (Ripple et al., 2024). As an analogue, arctic climate change generates disturbances that can manifest themselves as ‘press driver’ (i.e., disturbances or stressors that operate over large spatial scales and remain in place for a long time, or slowly increase in pressure) and those that act as ‘pulse driver’ (i.e., sudden and short events that often operate on local spatial scales, though their effects may be long lasting). A press driver can be described as extensive, pervasive, or subtle (e.g., rising mean temperature) and a pulse driver as infrequent, sudden event (rain-on-snow or insect outbreaks) (Ratajczak et al., 2017). The impacts and consequences of large-scale press drivers have historically received most scientific focus, yet the accelerating stress levels associated with increasing frequencies of extreme events in the Arctic may suggest that conventional modelling approaches based on incremental changes in a single long-term stress provide poor estimates of the impact of climate on ecosystems. In developing and evaluating climate and ecosystem models of real-world systems, it is imperative to capture complex feedback networks and feedback loops, as well as the effects of multiple drivers of change operating on different spatiotemporal scales. In fact, a multi-scale integration of press and pulse drivers in feedback network analyses can reveal novel insights into antagonistic or synergistic relationships but also provide more realistic trajectories on potential ecosystem tipping points and/or collapse (Willcock et al., 2023).
As a first step towards a better integration into ecosystem models, we have provided here a broad overview of the many ways in which biota may influence key feedback loops between the ecosystem and the climate system. As a necessary next step, we propose to conduct a thorough meta-analysis on biotic influences on feedback loops in high-latitude ecosystems, focusing specifically on the magnitude and direction of the impact. Understanding the intricate interplay between biota and feedback loops between the tundra and the atmosphere is crucial for understanding and predicting the consequences of climate change in the Arctic and beyond.
Author contributions
NS: Conceptualization, Methodology, Visualization, Writing–original draft, Writing–review and editing. IB: Conceptualization, Methodology, Writing–original draft, Writing–review and editing. JK: Conceptualization, Methodology, Writing–original draft, Writing–review and editing. EL-B: Writing–review and editing. FB: Writing–review and editing.
Funding
The author(s) declare that financial support was received for the research, authorship, and/or publication of this article. This work was financially supported by the Danish Energy Agency for the joint AMAP/CAFF initiative (grant no. TAS 4005-520975) and by the Danish Environmental Protection Agency for supporting CBMP (grant no. MST 2022–86635). This work is a contribution to IB’s NordForsk project NordBorN (grant no. 164079), which also includes contributions from NS and EL-B. JAK was supported by the Carlsberg Foundation (CARBONZOO, grant no. CF23_0641). EL-B considers this study a contribution to GreenFeedBack (Greenhouse gas fluxes and earth system feedbacks) funded by the European Union’s HORIZON research and innovation program under grant agreement No 101056921.
Acknowledgments
We thank the Arctic Council Working Groups AMAP (Arctic Monitoring and Assessment Program) and CAFF (Conservation of Arctic Flora and Fauna) as well as Greenland Ecosystem Monitoring for their continued efforts to unravel high latitude ecosystem dynamics.
Conflict of interest
The authors declare that the research was conducted in the absence of any commercial or financial relationships that could be construed as a potential conflict of interest.
Publisher’s note
All claims expressed in this article are solely those of the authors and do not necessarily represent those of their affiliated organizations, or those of the publisher, the editors and the reviewers. Any product that may be evaluated in this article, or claim that may be made by its manufacturer, is not guaranteed or endorsed by the publisher.
References
Adamczyk, M., Perez-Mon, C., Gunz, S., and Frey, B. (2020). Strong shifts in microbial community structure are associated with increased litter input rather than temperature in High Arctic soils. Soil Biol. biochem. 151, 108054. doi:10.1016/j.soilbio.2020.108054
Aggerbeck, M. R., Nielsen, T. K., Mosbacher, J. B., Schmidt, N. M., and Hansen, L. H. (2022). Muskoxen homogenise soil microbial communities and affect the abundance of methanogens and methanotrophs. Sci. Total Environ. 827, 153877. doi:10.1016/j.scitotenv.2022.153877
Altshuler, I., Hamel, J., Turney, S., Magnuson, E., Lévesque, R., Greer, C. W., et al. (2019). Species interactions and distinct microbial communities in high Arctic permafrost affected cryosols are associated with the CH4 and CO2 gas fluxes. Environ. Microbiol. 21, 3711–3727. doi:10.1111/1462-2920.14715
Andersen-Ranberg, E. U., Barnes, C. J., Rasmussen, L., Salgado-Flores, A., Grøndahl, C., Mosbacher, J. B., et al. (2018). A comparative study on the faecal bacterial community and potential zoonotic bacteria of muskoxen (Ovibos moschatus) in northeast Greenland, northwest Greenland and Norway. Microorganisms 6, 76. doi:10.3390/microorganisms6030076
Andreu-Hayles, L., Gaglioti, B. V., Berner, L. T., Levesque, M., Anchukaitis, K. J., Goetz, S. J., et al. (2020). A narrow window of summer temperatures associated with shrub growth in Arctic Alaska. Environ. Res. Lett. 15, 105012. doi:10.1088/1748-9326/ab897f
Angst, G., Mueller, K. E., Castellano, M. J., Vogel, C., Wiesmeier, M., and Mueller, C. W. (2023). Unlocking complex soil systems as carbon sinks: multi-pool management as the key. Nat. Commun. 14, 2967. doi:10.1038/s41467-023-38700-5
Angst, G., Potapov, A., Joly, F.-X., Angst, Š., Frouz, J., Ganault, P., et al. (2024). Conceptualizing soil fauna effects on labile and stabilized soil organic matter. Nat. Commun. 15, 5005. doi:10.1038/s41467-024-49240-x
Barbero-Palacios, L., Barrio, I. C., García Criado, M., Kater, I., Petit Bon, M., Kolari, T. H. M., et al. (2024). Herbivore diversity effects on Arctic tundra ecosystems: a systematic review. Environ. Evid. 13, 6. doi:10.1186/s13750-024-00330-9
Barrio, I. C., Lindén, E., Te Beest, M., Olofsson, J., Rocha, A., Soininen, E. M., et al. (2017). Background invertebrate herbivory on dwarf birch (Betula glandulosa-nana complex) increases with temperature and precipitation across the tundra biome. Polar Biol. 40, 2265–2278. doi:10.1007/s00300-017-2139-7
Barták, M. (2014). Lichen photosynthesis. Scaling from the cellular to the organism level. Netherlands: Springer, 379–400.
Barthelemy, H., Nobel, L. A., Stark, S., Väisänen, M., Olofsson, J., and Michelsen, A. (2024). Short- and long-term plant and microbial uptake of 15N-labelled urea in a mesic tundra heath, West Greenland. Polar Biol. 47, 1–15. doi:10.1007/s00300-023-03209-6
Barthelemy, H., Stark, S., Michelsen, A., and Olofsson, J. (2018). Urine is an important nitrogen source for plants irrespective of vegetation composition in an Arctic tundra: insights from a 15N-enriched urea tracer experiment. J. Ecol. 106, 367–378. doi:10.1111/1365-2745.12820
Beard, K. H., Kelsey, K. C., Choi, R. T., Welker, J. M., and Leffler, A. J. (2023). Goose feces effects on subarctic soil nitrogen availability and greenhouse gas fluxes. Ecosystems 26, 187–200. doi:10.1007/s10021-022-00752-x
Beer, C., Zimov, N., Olofsson, J., Porada, P., and Zimov, S. (2020). Protection of permafrost soils from thawing by increasing herbivore density. Sci. Rep. 10, 4170. doi:10.1038/s41598-020-60938-y
Beumer, L. T., Varpe, Ø., and Hansen, B. B. (2017). Cratering behaviour and faecal C:N ratio in relation to seasonal snowpack characteristics in a High-Arctic ungulate. Polar Res. 36, 1286121. doi:10.1080/17518369.2017.1286121
Bintanja, R., and Andry, O. (2017). Towards a rain-dominated Arctic. Nat. Clim. Change 7, 263–267. doi:10.1038/nclimate3240
Blois, J. L., Zarnetske, P. L., Fitzpatrick, M. C., and Finnegan, S. (2013). Climate change and the past, present, and future of biotic interactions. Science 341, 499–504. doi:10.1126/science.1237184
Blume-Werry, G., Krab, E. J., Olofsson, J., Sundqvist, M. K., Väisänen, M., and Klaminder, J. (2020). Invasive earthworms unlock arctic plant nitrogen limitation. Nat. Commun. 11, 1766. doi:10.1038/s41467-020-15568-3
Box, J. E., Colgan, W. T., Christensen, T. R., Schmidt, N. M., Lund, M., Parmentier, F. J., et al. (2019). Key indicators of Arctic climate change: 1971-2017. Environ. Res. Lett. 14, 045010. doi:10.1088/1748-9326/aafc1b
Brantley, S. L., Shaughnessy, A., Lebedeva, M. I., and Balashov, V. N. (2023). How temperature-dependent silicate weathering acts as Earth’s geological thermostat. Science 379, 382–389. doi:10.1126/science.add2922
Cahoon, S. M. P., Sullivan, P. F., Post, E., and Welker, J. M. (2012). Large herbivores limit CO2 uptake and suppress carbon cycle responses to warming in West Greenland. Glob. Change Biol. 18, 469–479. doi:10.1111/j.1365-2486.2011.02528.x
Callaghan, T. V., Björn, L. O., Chernov, Y., Chapin, T., Christensen, T. R., Huntley, B., et al. (2004). Effects on the structure of arctic ecosystems in the short-and long-term perspectives. Ambio 33, 436–447. doi:10.1579/0044-7447-33.7.436
Callaghan, T. V., Matveyeva, N., Chernov, Y., Schmidt, N. M., Brooker, R., and Johansson, M. (2013) “Arctic terrestrial ecosystems,” in Encyclopedia of biodiversity. Volume 1, Second ed. Waltham, MA: Academic Press, 227–244.
Chapin, F. S., Sturm, M., Serreze, M. C., McFadden, J. P., Key, J. R., Lloyd, A. H., et al. (2005). Role of land-surface changes in arctic summer warming. Science 310, 657–660. doi:10.1126/science.1117368
Christensen, T. R., Lund, M., Skov, K., Abermann, J., López-Blanco, E., Scheller, J., et al. (2021). Multiple ecosystem effects of extreme weather events in the arctic. Ecosystems 24, 122–136. doi:10.1007/s10021-020-00507-6
Christie, K. S., Bryant, J. P., Gough, L., Ravolainen, V. T., Ruess, R. W., and Tape, K. D. (2015). The role of vertebrate herbivores in regulating shrub expansion in the arctic: a synthesis. Bioscience 65, biv137–1133. doi:10.1093/biosci/biv137
Clauss, M., Dittmann, M.-T., Vendl, C., Hagen, K. B., Frei, S., Ortmann, S., et al. (2020). Review: comparative methane production in mammalian herbivores. Animal 14, s113–s123. doi:10.1017/S1751731119003161
Cleveland, C. C., Reed, S. C., Keller, A. B., Nemergut, D. R., O’Neill, S. P., Ostertag, R., et al. (2014). Litter quality versus soil microbial community controls over decomposition: a quantitative analysis. Oecologia 174, 283–294. doi:10.1007/s00442-013-2758-9
Cohen, J., Pulliainen, J., Ménard, C. c.B., Johansen, B., Oksanen, L., Luojus, K., et al. (2013). Effect of reindeer grazing on snowmelt, albedo and energy balance based on satellite data analyses. Remote Sens. Environ. 135, 107–117. doi:10.1016/j.rse.2013.03.029
Crowther, T. W., Thomas, S. M., Maynard, D. S., Baldrian, P., Covey, K., Frey, S. D., et al. (2015). Biotic interactions mediate soil microbial feedbacks to climate change. Proc. Natl. Acad. Sci. 112, 7033–7038. doi:10.1073/pnas.1502956112
Danell, K., Berteaux, D., and Brathen, K. A. (2002). Effect of musk carcasses on nitrogen concentration in tundra vegetation. Arctic 55, 389–392. doi:10.14430/arctic723
Descals, A., Gaveau, D. L. A., Verger, A., Sheil, D., Naito, D., and Peñuelas, J. (2022). Unprecedented fire activity above the Arctic Circle linked to rising temperatures. Science 378, 532–537. doi:10.1126/science.abn9768
Descamps, S., Aars, J., Fuglei, E., Kovacs, K. M., Lydersen, C., Pavlova, O., et al. (2017). Climate change impacts on wildlife in a High Arctic archipelago − Svalbard, Norway. Glob. Change Biol. 23, 490–502. doi:10.1111/gcb.13381
Elmendorf, S. C., Henry, G. H. R., Hollister, R. D., Björk, R. G., Bjorkman, A. D., Callaghan, T. V., et al. (2012a). Global assessment of experimental climate warming on tundra vegetation: heterogeneity over space and time. Ecol. Lett. 15, 164–175. doi:10.1111/j.1461-0248.2011.01716.x
Elmendorf, S. C., Henry, G. H. R., Hollister, R. D., Bjork, R. G., Boulanger-Lapointe, N., Cooper, E. J., et al. (2012b). Plot-scale evidence of tundra vegetation change and links to recent summer warming. Nat. Clim. Change 2, 453–457. doi:10.1038/nclimate1465
Epstein, H. E., Raynolds, M. K., Walker, D. A., Bhatt, U. S., Tucker, C. J., and Pinzon, J. E. (2012). Dynamics of aboveground phytomass of the circumpolar Arctic tundra during the past three decades. Environ. Res. Lett. 7, 015506. doi:10.1088/1748-9326/7/1/015506
Eyring, V., Bony, S., Meehl, G. A., Senior, C. A., Stevens, B., Stouffer, R. J., et al. (2016). Overview of the coupled model intercomparison project phase 6 (CMIP6) experimental design and organization. Geosci. Model Dev. 9, 1937–1958. doi:10.5194/gmd-9-1937-2016
Falk, J. M., Schmidt, N. M., Christensen, T. R., and Ström, L. (2015). Large herbivore grazing affects the vegetation structure and greenhouse gas balance in a high arctic mire. Environ. Res. Lett. 10, 045001. doi:10.1088/1748-9326/10/4/045001
Ferraro, K. M., Albrecht, D., Hendrix, J. G., Wal, E. V., Schmitz, O. J., Webber, Q. M. R., et al. (2024). The biogeochemical boomerang: site fidelity creates nutritional hotspots that may promote recurrent calving site reuse. Ecol. Lett. 27, e14491. doi:10.1111/ele.14491
Fischer, W., Thomas, C., Zimov, N., and Göckede, M. (2022). Grazing enhances carbon cycling but reduces methane emission during peak growing season in the Siberian Pleistocene Park tundra site. Biogeosci. Disc. 19, 1611–1633. doi:10.5194/bg-19-1611-2022
Fisher, J. B., Hayes, D. J., Schwalm, C. R., Huntzinger, D. N., Stofferahn, E., Schaefer, K., et al. (2018). Missing pieces to modeling the Arctic-Boreal puzzle. Environ. Res. Lett. 13, 020202. doi:10.1088/1748-9326/aa9d9a
Fritze, H., Penttilä, T., Mäkiranta, P., Laiho, R., Tuomivirta, T., Forsman, J., et al. (2021). Exploring the mechanisms by which reindeer droppings induce fen peat methane production. Soil Biol. biochem. 160, 108318. doi:10.1016/j.soilbio.2021.108318
Gao, J., and Carmel, Y. (2020). Can the intermediate disturbance hypothesis explain grazing–diversity relations at a global scale? Oikos 129, 493–502. doi:10.1111/oik.06338
García-Palacios, P., Bradford, M. A., Benavente-Ferraces, I., De Celis, M., Delgado-Baquerizo, M., García-Gil, J. C., et al. (2024). Dominance of particulate organic carbon in top mineral soils in cold regions. Nat. Geosci. 17, 145–150. doi:10.1038/s41561-023-01354-5
García-Palacios, P., Crowther, T. W., Dacal, M., Hartley, I. P., Reinsch, S., Rinnan, R., et al. (2021). Evidence for large microbial-mediated losses of soil carbon under anthropogenic warming. Nat. Rev. Earth and Environ. 2, 507–517. doi:10.1038/s43017-021-00178-4
Gilg, O., Kovacs, K. M., Aars, J., Fort, J., Gauthier, G., Gremillet, D., et al. (2012). Climate change and the ecology and evolution of Arctic vertebrates. Ann. N. Y. Acad. Sci. 1249, 166–190. doi:10.1111/j.1749-6632.2011.06412.x
González-Bergonzoni, I., Johansen, K. L., Mosbech, A., Landkildehus, F., Jeppesen, E., and Davidson, T. A. (2017). Small birds, big effects: the little auk (Alle alle) transforms high Arctic ecosystems. Proc. R. Soc. B Biol. Sci. 284, 20162572. doi:10.1098/rspb.2016.2572
Gornall, J. L., Jónsdóttir, I. S., Woodin, S. J., and Van Der Wal, R. (2007). Arctic mosses govern below-ground environment and ecosystem processes. Oecologia 153, 931–941. doi:10.1007/s00442-007-0785-0
Hall, K., and Lamont, N. (2003). Zoogeomorphology in the Alpine: some observations on abiotic–biotic interactions. Geomorphology 55, 219–234. doi:10.1016/s0169-555x(03)00141-7
Heijmans, M. M. P. D., Magnússon, R. Í., Lara, M. J., Frost, G. V., Myers-Smith, I. H., Van Huissteden, J., et al. (2022). Tundra vegetation change and impacts on permafrost. Nat. Rev. Earth and Environ. 3, 68–84. doi:10.1038/s43017-021-00233-0
Hicks, L. C., Yuan, M., Brangarí, A., Rousk, K., and Rousk, J. (2022). Increased above- and belowground plant input can both trigger microbial nitrogen mining in subarctic tundra soils. Ecosystems 25, 105–121. doi:10.1007/s10021-021-00642-8
Hollesen, J., Matthiesen, H., Møller, A. B., and Elberling, B. (2015). Permafrost thawing in organic Arctic soils accelerated by ground heat production. Nat. Clim. Change 5, 574–578. doi:10.1038/nclimate2590
Hotaling, S., Lutz, S., Dial, R. J., Anesio, A. M., Benning, L. G., Fountain, A. G., et al. (2021). Biological albedo reduction on ice sheets, glaciers, and snowfields. Earth-Sci. Rev. 220, 103728. doi:10.1016/j.earscirev.2021.103728
Huemmrich, K. F., Gamon, J. A., Tweedie, C. E., Campbell, P. K. E., Landis, D. R., and Middleton, E. M. (2013). Arctic tundra vegetation functional types based on photosynthetic physiology and optical properties. IEEE J. Sel. Top. Appl. Earth Observations Remote Sens. 6, 265–275. doi:10.1109/jstars.2013.2253446
Hugelius, G., Strauss, J., Zubrzycki, S., Harden, J. W., Schuur, E. A. G., Ping, C. L., et al. (2014). Estimated stocks of circumpolar permafrost carbon with quantified uncertainty ranges and identified data gaps. Biogeosciences 11, 6573–6593. doi:10.5194/bg-11-6573-2014
Iler, A. M., Caradonna, P. J., Forrest, J. R. K., and Post, E. (2021). Demographic consequences of phenological shifts in response to climate change. Annu. Rev. Ecol. Evol. Syst. 52, 221–245. doi:10.1146/annurev-ecolsys-011921-032939
IPCC (2021). Climate change 2021: the physical science basis. Contribution of working group I to the sixth assessment report of the intergovernmental panel on climate change. Cambridge University Press.
Johnson-Bice, S. M., Gable, T. D., Roth, J. D., and Bump, J. K. (2023). Patchy indirect effects of predation: predators contribute to landscape heterogeneity and ecosystem function via localized pathways. Oikos 2023. doi:10.1111/oik.10065
Keuper, F., Van Bodegom, P. M., Dorrepaal, E., Weedon, J. T., Van Hal, J., Van Logtestijn, R. S. P., et al. (2012). A frozen feast: thawing permafrost increases plant-available nitrogen in subarctic peatlands. Glob. Change Biol. 18, 1998–2007. doi:10.1111/j.1365-2486.2012.02663.x
Keuper, F., Wild, B., Kummu, M., Beer, C., Blume-Werry, G., Fontaine, S., et al. (2020). Carbon loss from northern circumpolar permafrost soils amplified by rhizosphere priming. Nat. Geosci. 13, 560–565. doi:10.1038/s41561-020-0607-0
Khvorostyanov, D. V., Krinner, G., Ciais, P., Heimann, M., and Zimov, S. A. (2008). Vulnerability of permafrost carbon to global warming. Part I: model description and role of heat generated by organic matter decomposition. Tellus B Chem. Phys. Meteorology 60, 250. doi:10.1111/j.1600-0889.2007.00333.x
Klein, D. R., and Bay, C. (1991). Diet selection by vertebrate herbivores in the high arctic of Greenland. Holarct. Ecol. 14, 152–155. doi:10.1111/j.1600-0587.1991.tb00646.x
Koltz, A. M., Gough, L., and McLaren, J. R. (2022). Herbivores in Arctic ecosystems: effects of climate change and implications for carbon and nutrient cycling. Ann. N. Y. Acad. Sci. 1516, 28–47. doi:10.1111/nyas.14863
Kozlov, M. V., Filippov, B. Y., Zubrij, N. A., and Zverev, V. (2015). Abrupt changes in invertebrate herbivory on woody plants at the forest–tundra ecotone. Polar Biol. 38, 967–974. doi:10.1007/s00300-015-1655-6
Kristensen, J. A., Michelsen, A., and Metcalfe, D. B. (2020a). Background insect herbivory increases with local elevation but makes minor contribution to element cycling along natural gradients in the Subarctic. Ecol. Evol. 10, 11684–11698. doi:10.1002/ece3.6803
Kristensen, J. A., Rousk, J., and Metcalfe, D. B. (2020b). Below-ground responses to insect herbivory in ecosystems with woody plant canopies: a meta-analysis. J. Ecol. 108, 917–930. doi:10.1111/1365-2745.13319
Kropp, H., Loranty, M. M., Natali, S. M., Kholodov, A. L., Rocha, A. V., Myers-Smith, I., et al. (2021). Shallow soils are warmer under trees and tall shrubs across Arctic and Boreal ecosystems. Environ. Res. Lett. 16, 015001. doi:10.1088/1748-9326/abc994
Kuhry, P., Bárta, J., Blok, D., Elberling, B., Faucherre, S., Hugelius, G., et al. (2020). Lability classification of soil organic matter in the northern permafrost region. Biogeosciences 17, 361–379. doi:10.5194/bg-17-361-2020
Landrum, L., and Holland, M. M. (2020). Extremes become routine in an emerging new Arctic. Nat. Clim. Change 10, 1108–1115. doi:10.1038/s41558-020-0892-z
Lara, M. J., Johnson, D. R., Andresen, C., Hollister, R. D., and Tweedie, C. E. (2017). Peak season carbon exchange shifts from a sink to a source following 50+ years of herbivore exclusion in an Arctic tundra ecosystem. J. Ecol. 105, 122–131. doi:10.1111/1365-2745.12654
Loranty, M. M., and Goetz, S. J. (2012). Shrub expansion and climate feedbacks in Arctic tundra. Environ. Res. Lett. 7, 011005. doi:10.1088/1748-9326/7/1/011005
Lund, M., Raundrup, K., Westergaard-Nielsen, A., López-Blanco, E., Nymand, J., and Aastrup, P. (2017). Larval outbreaks in West Greenland: instant and subsequent effects on tundra ecosystem productivity and CO2 exchange. Ambio 46, 26–38. doi:10.1007/s13280-016-0863-9
Lundberg, J., and Moberg, F. (2003). Mobile link organisms and ecosystem functioning: implications for ecosystem resilience and management. Ecosystems 6, 0087–0098. doi:10.1007/s10021-002-0150-4
Lundgren, E. J., Bergman, J., Trepel, J., Le Roux, E., Monsarrat, S., Kristensen, J. A., et al. (2024). Functional traits—not nativeness—shape the effects of large mammalian herbivores on plant communities. Science 383, 531–537. doi:10.1126/science.adh2616
Mack, M. C., Schuur, E. A. G., Bret-Harte, M. S., Shaver, G. R., and Chapin, F. S. (2004). Ecosystem carbon storage in arctic tundra reduced by long-term nutrient fertilization. Nature 431, 440–443. doi:10.1038/nature02887
Maes, S. L., Dietrich, J., Midolo, G., Schwieger, S., Kummu, M., Vandvik, V., et al. (2024). Environmental drivers of increased ecosystem respiration in a warming tundra. Nature 629, 105–113. doi:10.1038/s41586-024-07274-7
Manlick, P. J., Perryman, N. L., Koltz, A. M., Cook, J. A., and Newsome, S. D. (2024). Climate warming restructures food webs and carbon flow in high-latitude ecosystems. Nat. Clim. Change 14, 184–189. doi:10.1038/s41558-023-01893-0
McLaren, J. R., Buckeridge, K. M., Van De Weg, M. J., Shaver, G. R., Schimel, J. P., and Gough, L. (2017). Shrub encroachment in Arctic tundra: Betula nana effects on above- and belowground litter decomposition. Ecology 98, 1361–1376. doi:10.1002/ecy.1790
Mekonnen, Z. A., Riley, W. J., Berner, L. T., Bouskill, N. J., Torn, M. S., Iwahana, G., et al. (2021). Arctic tundra shrubification: a review of mechanisms and impacts on ecosystem carbon balance. Environ. Res. Lett. 16, 053001. doi:10.1088/1748-9326/abf28b
Min, E., Wilcots, M. E., Naeem, S., Gough, L., McLaren, J. R., Rowe, R. J., et al. (2021). Herbivore absence can shift dry heath tundra from carbon source to sink during peak growing season. Environ. Res. Lett. 16, 024027. doi:10.1088/1748-9326/abd3d0
Monteux, S., Keuper, F., Fontaine, S., Gavazov, K., Hallin, S., Juhanson, J., et al. (2020). Carbon and nitrogen cycling in Yedoma permafrost controlled by microbial functional limitations. Nat. Geosci. 13, 794–798. doi:10.1038/s41561-020-00662-4
Mosbacher, J. B., Kristensen, D. K., Michelsen, A., Stelvig, M., and Schmidt, N. M. (2016). Quantifying musk plant biomass removal and spatial relocation of nitrogen in a High Arctic tundra ecosystem. Arct. Antarct. Alp. Res. 48, 229–240. doi:10.1657/AAAR0015-034
Mosbacher, J. B., Michelsen, A., Stelvig, M., Hjermstad-Sollerud, H., and Schmidt, N. M. (2019). Muskoxen modify plant abundance, phenology, and nitrogen dynamics in a High Arctic fen. Ecosystems 22, 1095–1107. doi:10.1007/s10021-018-0323-4
Myers-Smith, I. H., Elmendorf, S. C., Beck, P. S. A., Wilmking, M., Hallinger, M., Blok, D., et al. (2015). Climate sensitivity of shrub growth across the tundra biome. Nat. Clim. Change 5, 887–891. doi:10.1038/nclimate2697
Myers-Smith, I. H., Forbes, B. C., Wilmking, M., Hallinger, M., Lantz, T., Blok, D., et al. (2011). Shrub expansion in tundra ecosystems: dynamics, impacts and research priorities. Environ. Res. Lett. 6, 045509. doi:10.1088/1748-9326/6/4/045509
Myers-Smith, I. H., Kerby, J. T., Phoenix, G. K., Bjerke, J. W., Epstein, H. E., Assmann, J. J., et al. (2020). Complexity revealed in the greening of the Arctic. Nat. Clim. Change 10, 106–117. doi:10.1038/s41558-019-0688-1
Oehri, J., Schaepman-Strub, G., Kim, J.-S., Grysko, R., Kropp, H., Grünberg, I., et al. (2022). Vegetation type is an important predictor of the arctic summer land surface energy budget. Nat. Commun. 13, 6379. doi:10.1038/s41467-022-34049-3
Olofsson, J., Kitti, H., Rautiainen, P., Stark, S., and Oksanen, L. (2001). Effects of summer grazing by reindeer on composition of vegetation, productivity and nitrogen cycling. Ecography 24, 13–24. doi:10.1034/j.1600-0587.2001.240103.x
Olofsson, J., Oksanen, L., Callaghan, T., Hulme, P. E., Oksanen, T., and Suominen, O. (2009). Herbivores inhibit climate-driven shrub expansion on the tundra. Glob. Change Biol. 15, 2681–2693. doi:10.1111/j.1365-2486.2009.01935.x
Olofsson, J., and Post, E. (2018). Effects of large herbivores on tundra vegetation in a changing climate, and implications for rewilding. Philosophical Trans. R. Soc. B Biol. Sci. 373, 20170437. doi:10.1098/rstb.2017.0437
Osterkamp, T. E., Jorgenson, M. T., Schuur, E. A. G., Shur, Y. L., Kanevskiy, M. Z., Vogel, J. G., et al. (2009). Physical and ecological changes associated with warming permafrost and thermokarst in Interior Alaska. Permafr. Periglac. Process. 20, 235–256. doi:10.1002/ppp.656
Otero, X. L., De La Peña-Lastra, S., Pérez-Alberti, A., Ferreira, T. O., and Huerta-Diaz, M. A. (2018). Seabird colonies as important global drivers in the nitrogen and phosphorus cycles. Nat. Commun. 9, 246. doi:10.1038/s41467-017-02446-8
Palmtag, J., Obu, J., Kuhry, P., Richter, A., Siewert, M. B., Weiss, N., et al. (2022). A high-spatial resolution soil carbon and nitrogen dataset for the northern permafrost region, based on circumpolar land cover upscaling. Earth Syst. Sci. Data 14, 4095–4110. doi:10.5194/essd-2022-8
Parker, T. C., Thurston, A. M., Raundrup, K., Subke, J.-A., Wookey, P. A., and Hartley, I. P. (2021). Shrub expansion in the Arctic may induce large-scale carbon losses due to changes in plant-soil interactions. Plant Soil 463, 643–651. doi:10.1007/s11104-021-04919-8
Parmesan, C. (2007). Influences of species, latitudes and methodologies on estimates of phenological response to global warming. Glob. Change Biol. 13, 1860–1872. doi:10.1111/j.1365-2486.2007.01404.x
Parmesan, C., and Yohe, G. (2003). A globally coherent fingerprint of climate change impacts across natural systems. Nature 421, 37–42. doi:10.1038/nature01286
Pearson, R. G., Phillips, S. J., Loranty, M. M., Beck, P. S. A., Damoulas, T., Knight, S. J., et al. (2013). Shifts in Arctic vegetation and associated feedbacks under climate change. Nat. Clim. Change 3, 673–677. doi:10.1038/nclimate1858
Peterson, S. L., Rockwell, R. F., Witte, C. R., and Koons, D. N. (2013). The legacy of destructive snow goose foraging on supratidal marsh habitat in the hudson Bay lowlands. Arct. Antarct. Alp. Res. 45, 575–583. doi:10.1657/1938-4246.45.4.575
Petit Bon, M., Hansen, B. B., Loonen, M. J. J. E., Petraglia, A., Bråthen, K. A., Böhner, H., et al. (2023). Long-term herbivore removal experiments reveal how geese and reindeer shape vegetation and ecosystem CO2-fluxes in high-Arctic tundra. J. Ecol. 111, 2627–2642. doi:10.1111/1365-2745.14200
Post, E., Alley, R. B., Christensen, T. R., Macias-Fauria, M., Forbes, B. C., Gooseff, M. N., et al. (2019). The polar regions in a 2°C warmer world. Sci. Adv. 5, eaaw9883. doi:10.1126/sciadv.aaw9883
Post, E., Kaarlejärvi, E., Macias-Fauria, M., Watts, D. A., Bøving, P. S., Cahoon, S. M. P., et al. (2023). Large herbivore diversity slows sea ice-associated decline in arctic tundra diversity. Science 380, 1282–1287. doi:10.1126/science.add2679
Post, E., and Pedersen, C. (2008). Opposing plant community responses to warming with and without herbivores. Proc. Natl. Acad. Sci. 105, 12353–12358. doi:10.1073/pnas.0802421105
Post, E., Pedersen, C., and Watts, D. A. (2022). Large herbivores facilitate the persistence of rare taxa under tundra warming. Sci. Rep. 12, 1292. doi:10.1038/s41598-022-05388-4
Previdi, M., Smith, K. L., and Polvani, L. M. (2021). Arctic amplification of climate change: a review of underlying mechanisms. Environ. Res. Lett. 16, 093003. doi:10.1088/1748-9326/ac1c29
Rantanen, M., Karpechko, A. Y., Lipponen, A., Nordling, K., Hyvärinen, O., Ruosteenoja, K., et al. (2022). The Arctic has warmed nearly four times faster than the globe since 1979. Commun. Earth and Environ. 3, 168. doi:10.1038/s43247-022-00498-3
Ratajczak, Z., D'Odorico, P., Collins, S. L., Bestelmeyer, B. T., Isbell, F. I., and Nippert, J. B. (2017). The interactive effects of press/pulse intensity and duration on regime shifts at multiple scales. Ecol. Monogr. 87, 198–218. doi:10.1002/ecm.1249
Rheubottom, S. I., Barrio, I. C., Kozlov, M. V., Alatalo, J. M., Andersson, T., Asmus, A. L., et al. (2019). Hiding in the background: community-level patterns in invertebrate herbivory across the tundra biome. Polar Biol. 42, 1881–1897. doi:10.1007/s00300-019-02568-3
Riedel, S. M., Epstein, H. E., and Walker, D. A. (2005). Biotic controls over spectral reflectance of arctic tundra vegetation. Int. J. Remote Sens. 26, 2391–2405. doi:10.1080/01431160512331337754
Ripple, W. J., Wolf, C., Lenton, T. M., Gregg, J. W., Natali, S. M., Duffy, P. B., et al. (2023). Many risky feedback loops amplify the need for climate action. One Earth 6, 86–91. doi:10.1016/j.oneear.2023.01.004
Ripple, W. J., Wolf, C., van Vuuren, D. P., Gregg, J. W., and Lenzen, M. (2024). An environmental and socially just climate mitigation pathway for a planet in peril. Environ. Res. Lett. 19, 021001. doi:10.1088/1748-9326/ad059e
Rixen, C., Høye, T. T., Macek, P., Aerts, R., Alatalo, J. M., Anderson, J. T., et al. (2022). Winters are changing: snow effects on Arctic and alpine tundra ecosystems. Arct. Sci. 8, 572–608. doi:10.1139/as-2020-0058
Rizzuto, M., Leroux, S. J., and Schmitz, O. J. (2024). Rewiring the carbon cycle: a theoretical framework for animal-driven ecosystem carbon sequestration. J. Geophys. Res. Biogeosciences 129. doi:10.1029/2024jg008026
Roslin, T., Antão, L., Hällfors, M., Meyke, E., Lo, C., Tikhonov, G., et al. (2021). Phenological shifts of abiotic events, producers and consumers across a continent. Nat. Clim. Change 11, 241–248. doi:10.1038/s41558-020-00967-7
Rousk, K., Sorensen, P. L., and Michelsen, A. (2017). Nitrogen fixation in the High Arctic: a source of ‘new’ nitrogen? Biogeochemistry 136, 213–222. doi:10.1007/s10533-017-0393-y
Rousk, K., Sorensen, P. L., and Michelsen, A. (2018). What drives biological nitrogen fixation in high arctic tundra: moisture or temperature? Ecosphere 9, e02117. doi:10.1002/ecs2.2117
Roy, A., Gough, L., Boelman, N. T., Rowe, R. J., Griffin, K. L., and McLaren, J. R. (2022). Small but mighty: impacts of rodent-herbivore structures on carbon and nutrient cycling in arctic tundra. Funct. Ecol. 36, 2331–2343. doi:10.1111/1365-2435.14127
Rößger, N., Sachs, T., Wille, C., Boike, J., and Kutzbach, L. (2022). Seasonal increase of methane emissions linked to warming in Siberian tundra. Nat. Clim. Change 12, 1031–1036. doi:10.1038/s41558-022-01512-4
Saunders, T., Adkins, J., Beard, K. H., Atwood, T. B., and Waring, B. G. (2023). Herbivores influence biogeochemical processes by altering litter quality and quantity in a subarctic wetland. Biogeochemistry 166, 67–85. doi:10.1007/s10533-023-01098-9
Schaefer, J. A., and Messier, F. (1995). Winter foraging by muskoxen: a hierarchical approach to patch residence time and cratering behaviour. Oecologia 104, 39–44. doi:10.1007/BF00365560
Schmidt, N. M., Hardwick, B., Gilg, O., Høye, T. T., Krogh, P. H., Meltofte, H., et al. (2017). Interaction webs in arctic ecosystems: determinants of arctic change? Ambio 46, S12–S25. doi:10.1007/s13280-016-0862-x
Schmidt, N. M., Kankaanpää, T., Tiusanen, M., Reneerkens, J., Versluijs, T. S. L., Hansen, L. H., et al. (2023). Little directional change in the timing of Arctic spring phenology over the past 25 years. Curr. Biol. 33, 3244–3249.e3. doi:10.1016/j.cub.2023.06.038
Schmidt, N. M., Reneerkens, J., Christensen, J. H., Olesen, M., and Roslin, T. (2019). An ecosystem-wide reproductive failure with more snow in the Arctic. PLoS Biol. 17, e3000392. doi:10.1371/journal.pbio.3000392
Schmidt, N. M., Roslin, T., Hansen, L. H., Gilg, O., Lang, J., Sittler, B., et al. (2022). Spatio-temporal patterns in arctic fox (Vulpes alopex) diets revealed by molecular analysis of scats from Northeast Greenland. Polar Sci. 32, 100838. doi:10.1016/j.polar.2022.100838
Schmitz, O. J., Sylvén, M., Atwood, T. B., Bakker, E. S., Berzaghi, F., Brodie, J. F., et al. (2023). Trophic rewilding can expand natural climate solutions. Nat. Clim. Change 13, 324–333. doi:10.1038/s41558-023-01631-6
Schmitz, O. J., Wilmers, C. C., Leroux, S. J., Doughty, C. E., Atwood, T. B., Galetti, M., et al. (2018). Animals and the zoogeochemistry of the carbon cycle. Science 362, eaar3213. doi:10.1126/science.aar3213
Schuuring, S., Halvorsen, R., Bronken Eidesen, P., Niittynen, P., Kemppinen, J., and Lang, S. I. (2024). High arctic vegetation communities with a thick moss layer slow active layer thaw. J. Geophys. Res. Biogeosciences 129. doi:10.1029/2023jg007880
See, C. R., Virkkala, A.-M., Natali, S. M., Rogers, B. M., Mauritz, M., Biasi, C., et al. (2024). Decadal increases in carbon uptake offset by respiratory losses across northern permafrost ecosystems. Nat. Clim. Change 14, 853–862. doi:10.1038/s41558-024-02057-4
Sistla, S. A., Moore, J. C., Simpson, R. T., Gough, L., Shaver, G. R., and Schimel, J. P. (2013). Long-term warming restructures Arctic tundra without changing net soil carbon storage. Nature 497, 615–618. doi:10.1038/nature12129
Soininen, E. M., Barrio, I. C., Bjørkås, R., Björnsdóttir, K., Ehrich, D., Hopping, K. A., et al. (2021). Location of studies and evidence of effects of herbivory on Arctic vegetation: a systematic map. Environ. Evid. 10, 25. doi:10.1186/s13750-021-00240-0
Speed, J. D. M., Austrheim, G., Hester, A. J., and Mysterud, A. (2010). Experimental evidence for herbivore limitation of the treeline. Ecology 91, 3414–3420. doi:10.1890/09-2300.1
Steger, B. (2023). Influence of musk carcasses on soil nutrients and vegetation in a High Arctic ecosystem in northeast Greenland. Borgarnes: Agricultural University of Iceland, 28.
Street, L. E., Stoy, P. C., Sommerkorn, M., Fletcher, B. J., Sloan, V. L., Hill, T. C., et al. (2012). Seasonal bryophyte productivity in the sub-Arctic: a comparison with vascular plants. Funct. Ecol. 26, 365–378. doi:10.1111/j.1365-2435.2011.01954.x
Sturm, M., Douglas, T., Racine, C., and Liston, G. E. (2005). Changing snow and shrub conditions affect albedo with global implications. J. Geophys. Res. Biogeosciences 110, G01004. doi:10.1029/2005JG000013
Sturm, M., Holmgren, J., McFadden, J. P., Liston, G. E., Chapin, F. S., and Racine, C. H. (2001). Snow–shrub interactions in Arctic tundra: a hypothesis with climatic implications. J. Clim. 14, 336–344. doi:10.1175/1520-0442(2001)014<0336:ssiiat>2.0.co;2
Tanentzap, A. J., and Coomes, D. A. (2012). Carbon storage in terrestrial ecosystems: do browsing and grazing herbivores matter? Biol. Rev. 87, 72–94. doi:10.1111/j.1469-185x.2011.00185.x
Te Beest, M., Sitters, J., Ménard, C. B., and Olofsson, J. (2016). Reindeer grazing increases summer albedo by reducing shrub abundance in Arctic tundra. Environ. Res. Lett. 11, 125013. doi:10.1088/1748-9326/aa5128
Trumbore, S. (2009). Radiocarbon and soil carbon dynamics. Annu. Rev. Earth Planet. Sci. 37, 47–66. doi:10.1146/annurev.earth.36.031207.124300
Tuomi, M., Väisänen, M., Ylänne, H., Brearley, F. Q., Barrio, I. C., Bråthen, K. A., et al. (2021). Stomping in silence: conceptualizing trampling effects on soils in polar tundra. Funct. Ecol. 35, 306–317. doi:10.1111/1365-2435.13719
Vaisanen, M., Ylanne, H., Kaarlejarvi, E., Sjogersten, S., Olofsson, J., Crout, N., et al. (2014). Consequences of warming on tundra carbon balance determined by reindeer grazing history. Glob. Change Biol. 21, 384–388. doi:10.1038/NCLIMATE2147
van Beest, F. M., Barry, T., Christensen, T., Heiðmarsson, S., McLennan, D., and Schmidt, N. M. (2022). Extreme event impacts on terrestrial and freshwater biota in the arctic: a synthesis of knowledge and opportunities. Front. Environ. Sci. 10. doi:10.3389/fenvs.2022.983637
van Beest, F. M., López-Blanco, E., Hansen, L. H., and Schmidt, N. M. (2023). Extreme shifts in habitat suitability under contemporary climate change for a high-Arctic herbivore. Clim. Change 176, 31. doi:10.1007/s10584-023-03510-7
Van Der Wal, R., Bardgett, R. D., Harrison, K. A., and Stien, A. (2004). Vertebrate herbivores and ecosystem control: cascading effects of faeces on tundra ecosystems. Ecography 27, 242–252. doi:10.1111/j.0906-7590.2004.03688.x
Wang, B., Wu, L., Chen, D., Wu, Y., Hu, S., Li, L., et al. (2020). Grazing simplifies soil micro-food webs and decouples their relationships with ecosystem functions in grasslands. Glob. Change Biol. 26, 960–970. doi:10.1111/gcb.14841
Wilcox, E. J., Keim, D., Jong, T. d., Walker, B., Sonnentag, O., Sniderhan, A. E., et al. (2019). Tundra shrub expansion may amplify permafrost thaw by advancing snowmelt timing. Arct. Sci. 5, 202–217. doi:10.1139/as-2018-0028
Willcock, S., Cooper, G. S., Addy, J., and Dearing, J. A. (2023). Earlier collapse of Anthropocene ecosystems driven by multiple faster and noisier drivers. Nat. Sustain. 6, 1331–1342. doi:10.1038/s41893-023-01157-x
Williamson, S. N., Barrio, I. C., Hik, D. S., and Gamon, J. A. (2016). Phenology and species determine growing-season albedo increase at the altitudinal limit of shrub growth in the sub-Arctic. Glob. Change Biol. 22, 3621–3631. doi:10.1111/gcb.13297
Ylänne, H., Olofsson, J., Oksanen, L., and Stark, S. (2018). Consequences of grazer-induced vegetation transitions on ecosystem carbon storage in the tundra. Funct. Ecol. 32, 1091–1102. doi:10.1111/1365-2435.13029
Zhang, T. (2005). Influence of the seasonal snow cover on the ground thermal regime: an overview. Rev. Geophys. 43. doi:10.1029/2004rg000157
Keywords: albedo, arctic, biotic interactions, carbon dynamics, decomposers, herbivores, permafrost, vegetation
Citation: Schmidt NM, Barrio IC, Kristensen JA, López-Blanco E and van Beest FM (2024) Highlighting the role of biota in feedback loops from tundra ecosystems to the atmosphere. Front. Environ. Sci. 12:1491604. doi: 10.3389/fenvs.2024.1491604
Received: 05 September 2024; Accepted: 06 December 2024;
Published: 20 December 2024.
Edited by:
Per Fauchald, Norwegian Institute for Nature Research (NINA), NorwayReviewed by:
Michael M. Loranty, Colgate University, United StatesJeffrey Welker, University of Oulu, Finland
Copyright © 2024 Schmidt, Barrio, Kristensen, López-Blanco and van Beest. This is an open-access article distributed under the terms of the Creative Commons Attribution License (CC BY). The use, distribution or reproduction in other forums is permitted, provided the original author(s) and the copyright owner(s) are credited and that the original publication in this journal is cited, in accordance with accepted academic practice. No use, distribution or reproduction is permitted which does not comply with these terms.
*Correspondence: Niels M. Schmidt, bm1zQGVjb3MuYXUuZGs=