- 1Department of Plant, Food, and Environmental Sciences, Dalhousie University, Truro, NS, Canada
- 2Department of Biology, University of Prince Edward Island Faculty of Science, Charlottetown, PE, Canada
- 3Retired, Charlottetown, PE, Canada
Nitrous oxide (N2O) is highly water soluble and can be readily transported in waters draining from agricultural fields. Relatively few studies have quantified N2O losses through agricultural tile drainage systems and none have compared the effect of different sources of applied nitrogen or their timing of application. While IPCC guidelines provide estimates of emissions from agricultural drainage water, the uncertainty in these estimates is relatively high. This research quantifies N2O loss in tile drainage water, as influenced by nitrogen source and timing. The study site was located at Agriculture Canada research station, Harrington, PE, Canada and consisted of 12 plots with subsurface drainage systems installed at approximately 80 cm, separated by buffer drains. Three swine manure treatments were considered with inorganic fertilizer (ammonium nitrate) as a control, each replicated three times. Manure treatments included fall and spring application of solid swine manure and spring application of liquid swine manure, all applied to supply 120 kg N ha−1. The magnitude of N2O loss, as measured from samples collected at the tile outlets, demonstrated significant episodic emissions. Annual cumulative dissolved N2O emissions ranged from 0.1 to 5.69 kg N ha−1 (mean 0.83 kg N ha−1), while emissions from the soil surface were 0.09–1.16 kg N ha−1 (mean 0.33 kg N ha−1). N2O emissions in tile water were not significantly affected by the form of N applied, however tile drain length significantly impacted tile water N2O concentration. IPCC coefficients for N2O emissions from agricultural drainage water would underestimate actual N2O emissions at this site.
Introduction
Nitrous oxide (N2O) is a long-lived and potent GHG with a global warming potential 298 times that of carbon dioxide and is also the leading anthropogenic cause of stratospheric ozone depletion (IPCC, 2021; WMO, 2014). Agriculture is the largest anthropogenic source of N2O (Janzen et al., 1999; Flessa et al., 2002; IPCC, 2023; Syakila and Kroeze, 2011) producing over 60% of N2O emissions globally from inorganic (fertilizer) and organic (manure) sources (IPCC, 2022), with annual contributions of 2.8 Gt CO2-eq yr−1 (IPCC, 2023). In Prince Edward Island (PEI), Canada, agricultural activities are responsible for approximately 90% of human induced N2O emissions, with direct emissions from denitrification on agricultural soils, and indirect emissions that occur after nitrogen has been transported off agricultural fields, accounting for 80% of N2O released (Environment and Climate Change Canada, 2022). In humid and sub-humid climates such as PEI microbial denitrification is the dominant process responsible for N2O production in agricultural soils due to anoxic conditions that frequently develop as a result of high precipitation rates (Aulakh et al., 1984; Gauder et al., 2012; Granli and Bøckman, 1994; Kavdir et al., 2008).
Our understanding of global N2O dynamics remains incomplete due to the existence of unknown or poorly quantified sources and sinks of N2O and the complexity and interaction of mechanisms controlling the known N2O sources (Baggs, 2011; Chapuis-Lardy et al., 2007). N2O is produced in soils as a result of ammonia oxidation, nitrification and denitrification, and it’s production is affected by climatic factors such as soil temperature, moisture, and freeze-thaw cycles (Shakoor et al., 2021; Choudhary et al., 2002; Maag and Vinther, 1996; Granli and Bøckman, 1994; Gillam et al., 2007), as well as management practices such as nitrogen addition, tillage, and cropping system (Venterea et al., 2011). There is a need to measure N2O emissions from a greater range of agricultural sources and from a greater diversity of areas, both regionally and within the landscape, to allow improved quantification of national N2O emissions inventories (Rochette and McGinn, 2008). Understanding the spatial and temporal nature of emission processes is also important in refining and accurately estimating emissions. The IPCC recognizes indirect N2O emissions from agriculture, including those resulting from nitrogen leaching and runoff (N2OL). There is a greater degree of uncertainty in indirect N2O emissions, as fewer studies have quantified these sources (Nevison, 2000; Quesnel et al., 2019; ECCC, 2022). Furthermore, indirect sources can be difficult to measure, since emissions are removed from their point of origin (Reay et al., 2003; Roper et al., 2013) and, in the case of dissolved N2O emissions, may be confounded by subsurface soil interactions (Quesnel et al., 2019). The steps outlined by the IPCC in calculating indirect N2O emissions involve several assumptions, relying on general nitrogen cycling principles and a limited amount of field data (ECCC, 2022). There is a need for further quantification and study of the factors influencing indirect N2O emissions.
Leaching and run off are estimated to account for more than 75% of indirect N2O emissions from agriculture, with leaching being the largest contributor, and represents one of largest sources of uncertainty in estimates of agricultural N2O emissions (Nevison, 2000; Tian et al., 2019). In Canada’s National Inventory Report to the IPCC the fraction of nitrogen that is lost through leaching and runoff (FRACLEACH) is estimated as a function of the ratio of precipitation to evapotranspiration and ranges from 5% in the more arid regions of the country to 30% in the more humid regions of the country (ECCC, 2022). The percentage of this nitrogen lost as N2O (N2OL) from N leached from agricultural soils is estimated using leaching/runoff emission factor (EF5) of 0.0075 kg N2O-N kg−1 N leached (IPCC, 2019). Nevison (2000) indicated several uncertainties in the proposed IPCC methodology for calculating the N2O emissions through leaching and run off. Since emissions from this source are likely to be significantly influenced by climate there is a need for data from a broader range of agroecosystems. The factor further assumes a relationship between N2O and NO3− concentration in agricultural drainage water. This relationship has not been well documented with some studies (Sawamoto et al., 2003) showing little or no relationship between N2O and NO3− in drainage waters, with high dissolved N2O concentrations in the fall corresponding with low NO3− levels. This disconnect was explained by spatial differences in where these processes were occurring; increased dissolved N2O concentrations were attributed to subsoil denitrification which occurred during or after NO3− leaching had occurred (Sawamoto et al., 2003). As a result, there is a need to develop appropriately documented regional emission coefficients.
Subsurface tile drainage is a management practice used in imperfectly and poorly drained agricultural fields to prevent water logging, lower a perched water table, and drain excess water from fields (Valayamkunnath et al., 2022). Subsurface tile drainage also decreases soil structural damage, erosion, and increases the length of the effective growing season by allowing earlier access to fields (Valayamkunnath et al., 2022). In Atlantic Canada, tile drainage water flows intermittently during year and drainage events can occur during the winter period, when temperatures in the soil profile rise above freezing. Peak drainage events typically occur during spring thaw, which may coincide with peak surface N2O emissions. In northern climates, up to 70% of N2O emissions from agricultural soils are thought to occur during spring thaw, as a result of enhanced microbial activity and the release of N2O trapped under a frozen ice layer (Risk et al., 2013; 2014; Wagner-Riddle et al., 2017). Tile drains intercept the flow of the water draining from the root zone and its composition is often considered to be representative of the nutrients draining from an agricultural field (Valayamkunnath et al., 2022). Several studies have documented that loss of nitrogen through subsurface tile drainage is substantial (Nangia et al., 2010; Quesnel et al., 2019; Milburn et al., 1997; Drury et al., 1993; Randall et al., 1997). In more humid agroecosystems such as PEI, it has been concluded that 15%–50% of the applied nitrogen reaches surface water via subsurface drainage (Jiang et al., 2012). Most studies of nitrogen loss in tile drainage have focused on the losses of NO3 and NH4+ (Drury et al., 1993; Klavidko et al., 1991; Randall et al., 1997), however fewer studies have examined dissolved N2O (Bruun et al., 2017; Hack and Kaupenjohann, 2002; Hama-Aziz et al., 2017; Reay et al., 2004; Reay et al., 2009; Quesnel et al., 2019).
Nitrous oxide produced below the soil surface results in the accumulation of N2O in the soil profile due to impeded gas transport by water-filled pores and/or formation of frozen layer during winter and spring thaw (Burton and Beauchamp, 1994; Heincke and Kaupenjohann, 1999). Nitrous oxide is highly soluble in water and its solubility increases as the water temperature decreases (DelVecchia et al., 2023). During winter and spring, cold water moves through the soil profile when N2O concentrations are elevated (Burton and Beauchamp, 1985). These conditions result in a high potential for N2O transport and loss in tile drainage water (Valayamkunnath et al., 2022). Moreover, agricultural fields with tile drainage expedite the movement of water from the field and therefore decrease the likelihood of further N2O reduction in the subsurface (Hack and Kaupenjohann, 2002; Valayamkunnath et al., 2022). Dowdell et al. (1979) noted significant amounts of dissolved N2O (8.1–277.3 µg N2O-N L−1) in water draining from agricultural fields. Similarly, other studies have variously reported dissolved N2O concentrations in tile drainage water from agricultural systems ranging from 0.3–1,108 ug N L−1 (Bruun et al., 2017; Hama-Aziz et al., 2017; Quesnel et al., 2019; Reay et al., 2003; 2004; 2009; Roper, 2008).
Data is lacking on the impact of N input form (synthetic fertilizers vs. manure) and timing on dissolved N2O losses from agricultural fields. While several studies have examined dissolved N2O losses in agricultural fields under manure inputs (Reay et al., 2009; Quesnel et al., 2019), none have compared different manure/N application treatments. Furthermore, the timing of manure application to agricultural fields is known to impact NO3 leaching, with fall manure applications elevating leaching risk relative to spring (van Es et al., 2006). It is unknown whether this also applies to dissolved N2O losses. Currently, there is limited measured data documenting N2O concentrations and loss from tile drainage systems, particularly during the winter period. The effect of soil management, such as various cropping systems and fertilizer applications, on N2O losses via tile drainage systems also needs more study. The quantification of N2O production during the non-growing period and its transport from the soil profile into the tile drainage water are needed to provide a more complete picture of the impacts of agricultural management on indirect N2O emissions and identify opportunities for mitigation. Furthermore, to date only one study has considered the relative magnitude of dissolved N2O loss compared to surface N2O emissions and NO3 leaching (Quesnel et al., 2019).
This paper investigates N2O losses in agricultural tile drainage water from a 3-year potato cropping rotation in PEI, Canada. Objectives of the study were to 1) quantify and compare the annual temporal variation in N2O and NO3− concentrations in tile drainage systems and surface N2O emissions in PEI 2) determine the impact of swine manure treatment form (solid and liquid) and timing (fall and spring application) on dissolved N2O losses in tile drainage water and 3) evaluate the EF5 for predicting N2O emitted from nitrogen lost through leaching in PEI.
Materials and methods
Study site
The field experiment was initiated in 2000 at Agriculture and Agri-Food Canada’s Harrington Research Farm, situated 14 km north of Charlottetown, PE (46° N lat, 63° W long). The research site covers an area of 2.9-ha with a gentle slope of 1%–3%. Between 1986 and 1989 the site was divided into 13 subsurface drainage plots, each with dimensions ranging from 0.21 to 0.28 ha (Milburn et al., 1997). Tile drainage systems were installed to account for individual plot size and drainage capacity. Each plot contained two tile drains within the plot, and individual plots were separated by buffer drains to limit cross-contamination from adjacent plots due to subsurface or surface flow crossing plot boundaries. Three shorter tile drains were installed in plots 8, 9 and 10 to accommodate the irregular shape of the field. The tile drains were spaced at an interval of 12 m in plots 8, 9 and 10, and 15 m in the remaining plots. The tiles were placed at an average depth of 0.80 m and the drains discharged into tipping buckets housed in a heated discharge building.
The soils at the experimental site are Charlottetown sandy loam (Orthic Humo-Ferric Podzol), with a Malpeque sandy loam (Gleyed Eluviated Drystic Brunisol) occurring in plots 7, 8, 10, and 11 in the lower portions of the field, and a soil pH of 6.6 (Milburn et al., 1997). Charlottetown soils tend to be well drained, while Malpeque soils are imperfectly drained (MacDougall et al., 1988). Daily precipitation and soil temperature was recorded at the Harrington research farm meteorological station, located less than 1 km from the experimental site.
Nitrogen fertility treatments and cropping practices
The agronomic treatments consisted of 4 N fertility treatments applied prior to potato phase of the potato-barley-red clover rotation. The N treatments were: solid and liquid swine manure applied in the spring prior to planting of potatoes; solid manure applied in the fall prior to the potato year and NH4NO3 applied in the spring. Each treatment was replicated three times and the rate of application adjusted to supply 120 kg N ha−1. Swine manure was generated in an adjacent facility that produced both solid and liquid manure (Campbell et al., 2003). During fall 2000 and 2003, solid swine manure was applied, while in spring 2001 and 2004 solid swine manure, liquid swine manure and ammonium nitrate were applied (Table 1). The experimental site was cropped to potato (Solanum tuberosum (L.) var. Shepody) in 2001 and 2004, to barley (Harem vulgare (L.) var. Iona) under-seeded to red clover (Trifolium pretense (L.) var. Marino) in 2002, and to red clover in 2003. In the spring of the barley year all plots received 60 kg N ha−1 as NH4NO3.
Water sampling and analysis
Water sampling for dissolved N2O
The concentration of N2O dissolved in tile drainage water was measured by collecting grab samples at the outlet of the drainage tiles during flow events in 2002, 2003 and 2004 (see supplementary for details on sample collection process). Samples were collected on a weekly basis or when drainage events were occurring. The diurnal pattern of N2O emissions was examined by collecting samples every 4 hours over a 3-day flow event on April 27–29, 2004.
The NO3− and NH4+ content and temperature of tile water discharge was determined from water samples collected as part of a parallel study using ISCO 6712 automatic samplers (Teledyne ISCO Inc., Lincoln, NB) (Jiang et al., 2011) (see supplementary for details on sample collection process).
During the 2002 to 2004 observation period the frequency of sampling depended upon tile flow. During flow events samples were collected between 0800 and 0900 h. The flow rate for each tile drain was calculated by multiplying the total number of tips of the tipping bucket by the amount of water each bucket holds. The tipping buckets were wired to a data logger (Campbell Scientific, Logan, UT). Tile drainage water N2O emissions were calculated by accounting for individual plot flow rate on each sampling date. Cumulative N2O emissions were calculated by linear interpolation of N2O concentrations over the sampling dates for each flow event and these concentrations applied to daily water flow as measured by the tipping bucket flow meters.
There is uncertainty as to the degree of degassing of N2O that occurs as water runs along the tile drainage pipes. The influence of tile length on N2O concentration was evaluated by sampling the three in-field tile drainage systems at plot #8, which was in addition to the 12 experimental plots where the agronomic treatments were replicated. Plot eight received the treatment of 120 kg N ha−1 NH4NO3. The total length of each tile drain in this plot was 81.5 m (see supplementary for details on sample collection process).
Stream and spring water sampling
On 28 May 2004, water samples were collected from streams and springs to compare their dissolved N2O concentration with that of subsurface drainage water. The stream was situated adjacent to the research site, with subsurface drainage water flowing into it. Spring samples were also collected at a nearby location. Stream and spring water samples were collected by 5 mL disposable syringe (Luer-lok tip, latex free, Becton-Dickinson, Franklin Lakes, NJ), injected immediately in an exetainer (12 mL) with sodium azide and sealed with silica sealant.
Water sample analysis
Vials containing water samples for N2O analysis were equilibrated at room temperature (25°C) before being analyzed for headspace N2O concentration. N2O analysis was performed using a Varian Star 3,800 Ga Chromatograph (Varian, Mississauga, ON) fitted with an electron capture detector with a Combi-PAL autosampler (CTC Analytics, Zwingen, Switzerland). The autosampler removes a 2.5 mL volume from the sample tube and injects this into a sample valve that delivers 0.1 mL to the ECD. The ECD was operated at 300°C, 90% Ar, 10% CH4 carrier gas at 20 mL min−1, Haysep N 80/100 pre-column (0.32 cm diameter x 50 cm length) and Haysep D 80/100 mesh analytical columns (0.32 cm diameter x 200 cm length) in a column oven operated at 70°C. Pre-column was used in combination with a four-port valve to remove water from the sample. Operational conditions and data handling was performed with Varian Star software. In each analytical run of 147 samples a single replicate of three concentrations of standard gas mixtures were included for standardization of the instrument. The lab temperature was recorded during the analysis of dissolved N2O concentration. Total N2O dissolved in the water sample is the sum of N2O in the headspace and N2O dissolved in water. The molar mass of N2O in the headspace was calculated from the Ideal gas law (Equation 1).
Where: MN2Og = moles of N2O in vial headspace (mol); PN2O = partial pressure of gas in the headspace (atm); VHS = Volume of headspace (L); R = Gas constant (0.08214 atm/mol k); and T = lab temperature (oK).
Henry’s law (Equation 2) describes the partitioning of a compound between a dilute aqueous and the gas phase:
Where: CN2O = Nitrous oxide concentration in the aqueous solution (moles/L); HN2O = Henry’s law constant for nitrous oxide (2.5 × 10−2 M/atm), and PN2O = partial pressure of gas in the headspace (atm).
MN2Ol (Equation 3) is caclulated from CN2O present in V (5 mL) of the aqueous solution (1 mL of sodium azide and 4 mL of water sample).
Where: MN2Ol = Moles of the nitrous oxide in dissolved in water (moles); V = Volume of the aqueous solution in the vial (L).
Therefore, the total number of moles of nitrous oxide in the vial (Equation 4), less the estimated contribution of nitrogen by sodium azide (5.3 × 10−8 mol) is,
Where: TN2O = Total moles of nitrous oxide in head space and aqueous solution (moles).
The flux of dissolved N2O (g N ha−1 d−1) was calculated by multiplying the dissolved N2O concentration by the volume of tile drainage discharge per unit area (L ha−1) as measured by the tipping bucket gauges and the area of the plot drained by the tile system.
Nitrate and ammonium concentration of the water samples were determined colorimetrically using a TRAACS 800 analyzer (Milburn et al., 1997). Dissolved organic carbon was analyzed by Formacs TOC analyzer (Hasegawa et al., 2000).
Nitrous oxide production from sodium azide
An experiment was carried out to determine if the sodium azide produced any N2O during storage. Sodium azide solution was prepared at the same concentration as used in the exetainers for dissolved N2O sample collection (0.1 M). One mL of NaN3 was injected into a 12 mL exetainers using a 1 mL syringe (Luer-lok tip, latex free, Becton-Dickinson, Franklin Lakes, NJ), followed by flushing with helium and evacuation. After evacuation, 4 mL of nano pure water was transferred to the exetainer by means of a syringe (5 mL, Luer-lok tip, latex free, Becton-Dickinson, Franklin Lakes, NJ), ensuring a positive pressure inside the exetainer. Some vials were analyzed immediately for head space N2O concentration, while others were kept at lab temperature and analyzed after 1 month to determine the extent of N2O production from the sodium azide. Sodium azide was found to contribute background levels of N2O of approximately 5.3 × 10−8 mol of N2O.
Nitrous oxide flux at the soil surface
N2O flux measurements were made using a non-flow-through, non-steady-state (NFT-NSS) chamber with a total volume of 1.6 L covering a soil area of 315 cm2. Deployment times were kept to 30 min with samples being collected at 0, 10, 20 and 30 min. Replicate (5) samples of the atmosphere at chamber height at the time of deployment were used to estimate the ambient (time zero) concentrations. Samples were collected by removing 20 mL of gas from the headspace of the chamber and injecting into previously evacuated (to 500 millitorr) 12 mL Exetainers (Labco, UK), each containing 4 mg of Magnesium Perchlorate-a desiccant to remove water from the gas samples. Five replicates of two standard gases were collected on each sampling occasion and were used to confirm the integrity of sample storage and handling.
N2O flux measurements were made from 9 May to 26 September 2001, 14 May to 28 August 2002 and 16 May to 27 November 2003, and 10 March to 27 May 2004. Flux sampling conducted during the snow cover period (March 10 and 25, 2004), was carried out by inserting chambers into the snow (2–3 cm depth), and with samples collected in a similar manner as in the snow free period described. Two chambers were inserted in each plot. In 2001, when cropped to potato, these two chambers were separated by location: One chamber was installed in the hill and one in the furrow row locations within each plot. The hill and furrow locations were measured separately due to the distinctly different soil, nutrient and crop growth environments within these two locations. The chambers were removed and replaced to accommodate any management practices in the experimental site.
N2O flux (FN2O) was calculated by the following Equation 5 (Hutchinson and Livingston, 1993):
Where, dC/dt is the rate of change of N2O concentration inside the chamber (g N h−1), A is the surface area of the chamber (0.0315 m2), Vc is the total volume of the chamber (1.62 L), Mmol is molar mass of N2O (44 g/mol) and Vmol is the volume of a mole of N2O inside the chamber calculated from the ideal gas law based on temperature and pressure. The relative change in N2O concentration with time (dC/dt), inside the chamber, was calculated by simple linear regression. Cumulative N2O-N losses were calculated by linear interpolation between sampling dates.
N2O analysis was performed using a Varian Star 3,800 Ga Chromatograph using the same method as for headspace N2O concentration of the water samples.
Statistical analysis
An ANOVA on dissolved N2O concentration was performed as a randomized complete block (RCB) design, according to treatment for each sampling date. Correlation analysis was done between dissolved N2O concentration and NO3−, surface N2O flux, dissolved organic carbon, tile flow, water temperature, and plot area. Correlation analysis was also done between cumulative N2O loss and tile length of the experimental plots. Regression analysis was performed between cumulative N2O and NO3− loss in subsurface tile drainage water. All statistical analysis was conducted using Statistical Analysis System (SAS institute, 1990).
Results and discussion
Climatic data
Two of the three non-growing periods examined had lower than normal precipitation (Table 2). Cumulative non-growing season precipitation (November to May) for 2001–2002 and 2003–2004 was 531 and 380 mm respectively significantly lower than the long-term (1971–2000) average of 701 mm (Table 2) (Government of Canada, 2019). In 2002–2003 higher than average rainfall resulted from four high rainfall events including a 90 mm precipitation event (Table 2; Figure 1B). The duration and extent of soil freezing varied considerably between years (Figure 1) despite the average non-growing season air temperature being similar over the three winter periods. In 2001–2002 the lowest soil temperature at 5 cm was - 0.2°C and the soil was only frozen for 24 days (March 6 to March 30; Figure 1A). In contrast, in 2002–2003, the soil was frozen to a depth of 5 cm for 119 days (November 27 to April 29) and was frozen to 10 cm for 93 days (January 16 to April 19; Figure 1B). In 2003–2004, the soil at 5-cm depth was frozen for 41 days (January 5 to February 15) and at 10-cm depth for 22 days (January 13 to February 4; Figure 1C). Differences in soil freezing despite small differences in average air temperatures reflect differences in snow cover with the 2002–2003 period having relatively little precipitation in the early part of the winter and therefore having little or no snow accumulation. The tile flow for the observation period ranged between <1 and 54.5 mm per day (data not shown). The cumulative tile flow for the 2002, 2003 and 2004 calendar years was 156, 99, and 68 mm respectively. The greater cumulative tile flow in 2002 corresponded to the milder temperatures during the 2001–2002 overwinter period. Peak tile flow was observed during spring thaw and following precipitation events.
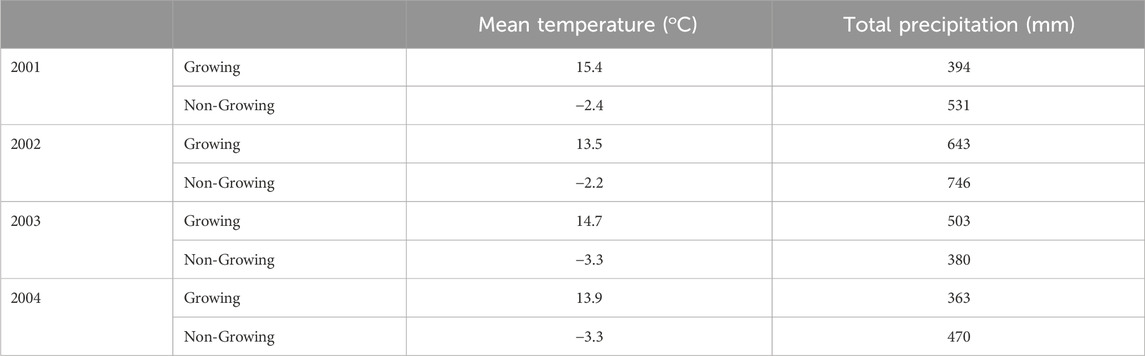
Table 2. Mean temperature and total precipitation at the experimental site during the growing (May–October) and non-growing (November–April) periods.
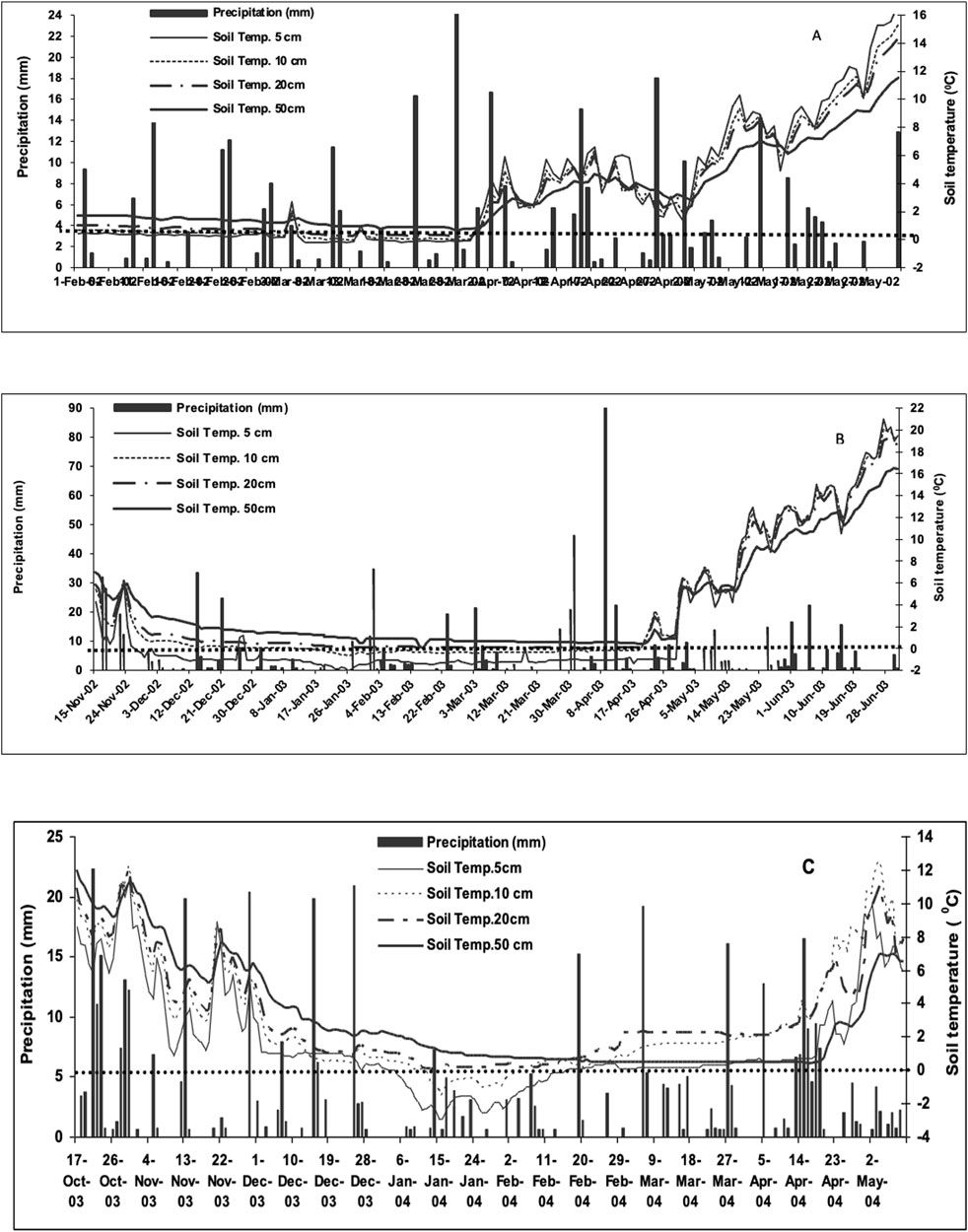
Figure 1. Precipitation and soil temperature during fall to spring periods at the Harrington Research station from (A) February - May 2002, (B) November 2002 to June 2003 and (C) October 2003 to May 2004.
Dissolved N2O emissions
Effect N treatments on dissolved N2O
Dissolved N2O concentrations in tile water discharge exhibited significant differences between sampling dates (p = 0.001) but were not significantly affected by nitrogen source (NH4NO3, solid manure and liquid manure) or timing (spring and fall application) of N application to the potato rotation (Table 3A; Table 3B; Figure 2). There are several possible explanations for this. In their meta-analysis examining the impact of manure applications on surface N2O emissions in agricultural soils, Xia et al. (2020) found that the application of manure and synthetic fertilizers induced similar magnitude N2O emissions when applied at the same N rate. While the overall emission factor following manure application (1.11%) is slightly lower than that of synthetic N fertilizer (1.25%), manure N2O emissions were also impacted by factors such as C:N ratio and C content (Xia et al., 2020), which resulted in similar levels of emissions produced. It is reasonable to assume that this would also apply to dissolved N2O below the soil surface. Additionally, while N fertilizer application has been found to be associated with elevated concentrations of dissolved N2O and NO3− in water draining from agricultural fields (Hasegawa et al., 2000), since the processes responsible for producing dissolved N2O in drainage water are spatially and temporally complex, the relationship between dissolved N2O and N application rate may not be straightforward (Reay et al., 2004; Quesnel et al., 2019). For example, fertilizers and manures are applied and measured at or near the soil surface and can subsequently be transported by leaching and surface runoff. In contrast, N2O can be produced throughout the soil profile; therefore, even after NO3− has been depleted from the soil surface, N2O can still be being produced in the soil, resulting in a temporal disconnect in the presence of these substances due to spatial differences in where they are measured and/or produced. It is possible that these same processes could make it difficult to detect differences in N source treatments. Failure to detect differences in treatments may have also resulted from differences in the length of drainage pipe from the plot to the collection station. We noted an inverse relationship between the lengths of the tile lines and averaged observed dissolved N2O concentrations (Figure 3). To confirm this observation air and water sampling access ports were inserted at three locations along the perforated portion of the tile line and at one location just outside the plot on an adjacent site. Average dissolved N2O concentrations over ten sampling events were observed to decrease from 1.1 mg N2O-N L−1 to 0.3 mg N2O-N L−1 (Figure 4). These observations suggest that degassing of N2O was occurring in the tile line and that concentrations measured at the sampling hut may underestimate total N2O leaving the field. Normalizing all results for length of tile line did not, however, reveal significant N treatment effects. For the remainder of the discussion, we will consider trends observed across all 12 experimental units independent of N treatment.
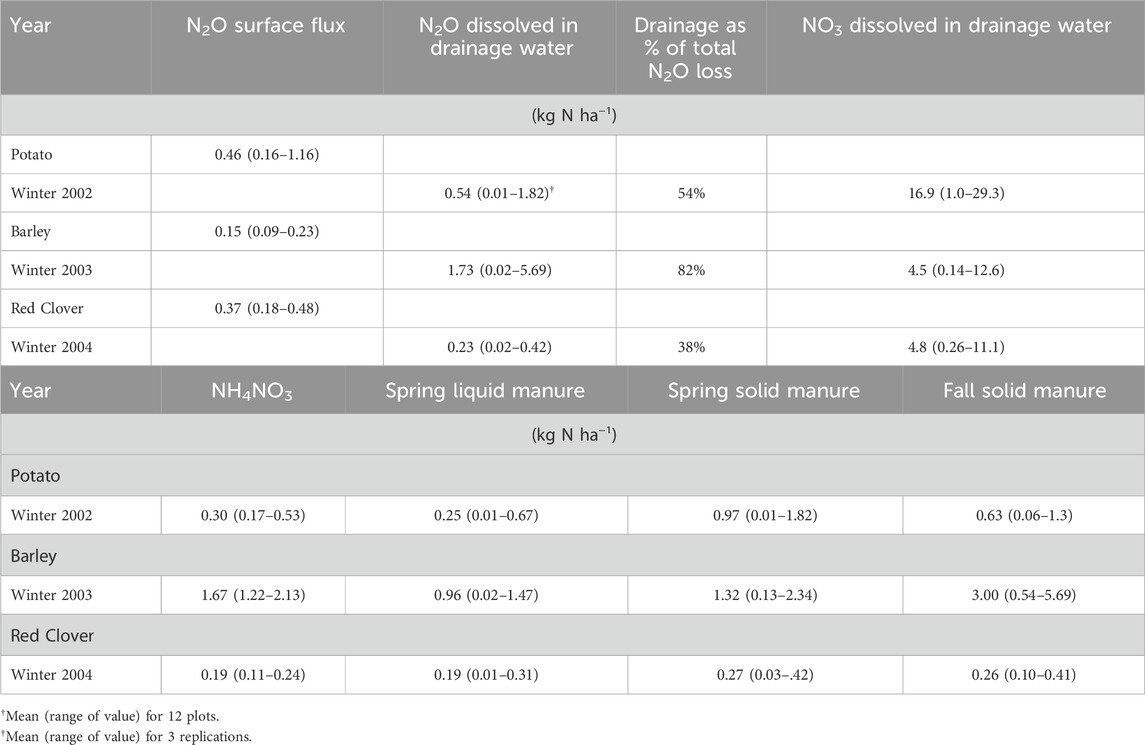
Table 3. (Panel A) Mean and range of values (12 plots) for cumulative annual N2O and NO3 dissolved in drainage water and surface N2O flux from research plots as measured during the 2002, 2003 and 2004 monitoring periods and (Panel B) mean and range of values (3 replicates) for cumulative annual N2O dissolved in drainage water from each N treatment as measured during the 2002, 2003 and 2004 monitoring periods.
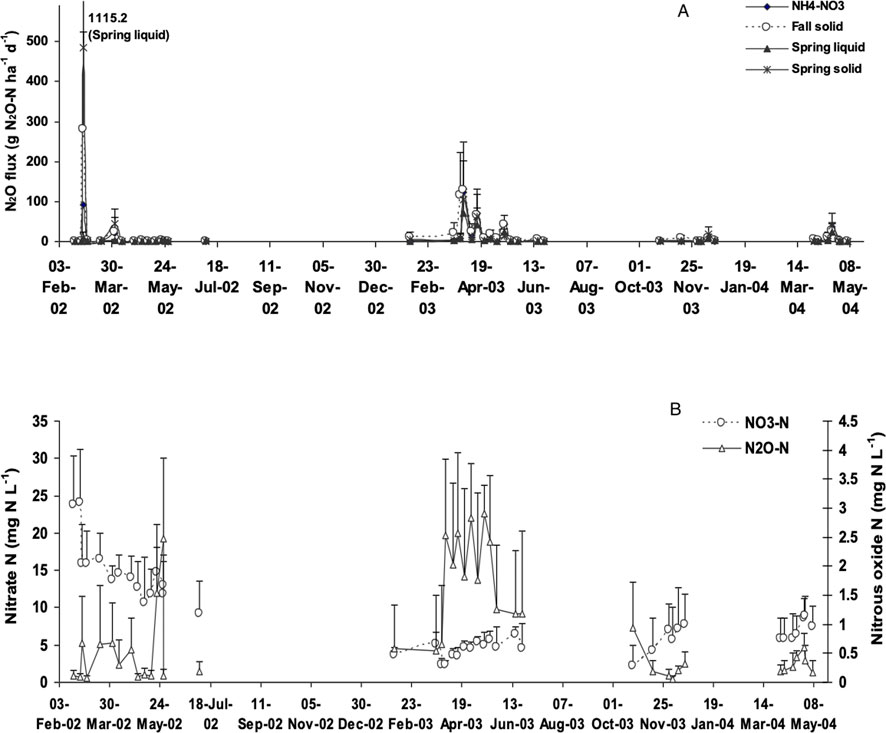
Figure 2. (A) Treatment effect on N2O flux from agricultural drainage water, and (B) N2O-N and NO3-N concentration in weekly samples of agricultural drainage water.
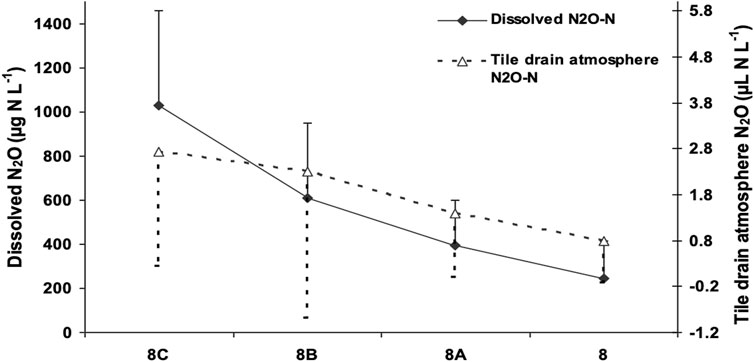
Figure 4. Dissolved N2O and tile drain atmosphere N2O concentration at three locations along a tile drain in plot# 8 (8A, 8B, 8C) and at the tile drain outlet of plot# 8 (8). Error bars represent the standard deviation of the 12 water and air samples taken during different days (between November 14 to 19 Dec 2003 and April 1 to 6 May 2004). Error bars are presented in opposite directions for the clarity of the figure.
Cumulative dissolved and surface N2O losses
Annual cumulative dissolved N2O emissions ranged from 0.01 to 5.69 kg N ha−1 (mean 0.83 kg N ha−1) which were slightly higher than annual cumulative N2O emissions occurring from the soil surface of 0.09–1.16 kg N ha−1 (mean 0.33 kg N ha−1) (Table 3A). The dissolved N2O-N loss through subsurface drainage water was an order of magnitude less than NO3−-N, which ranged from 0.26 to 29.3 kg N ha−1 (mean 8.7 kg N ha−1; Table 3A). The dissolved N2O results in our study are higher than other values of dissolved N2O in tile drains reported in the literature, which ranged from 0.03–0.38 kg N2O-N ha−1yr−1 (Dowdell et al., 1979; Hack and Kaupenjohann, 2002; Reay et al., 2004; 2009; Quesnel et al., 2019). Similarly, Reay et al. (2009), observed annual dissolved losses of N2O in agricultural drainage that were higher than the amount being emitted at the soil surface. In contrast, Bruun et al. (2017) found that the main export pathway was dissolved N2O, with negligible N2O produced at the soil surface, and both Dowdell et al. (1979) and Hack & Kaupenjohann, (2002) concluded that N2O loss in agricultural drainage water was a minor component of the soil N balance. However, Quesnel et al. (2019) found that cumulative annual soil surface N2O emissions (13.8 kg N2O-N ha−1) were much higher than dissolved N2O losses (0.03 kg N2O-N ha−1) in tile drainage systems. Hama-Aziz et al. (2016) observed concentrations of dissolved N2O in agricultural tile drainage systems were comparable to NO3 concentrations. Our results indicate that dissolved N2O emissions from potato cropping systems can be a major source of agricultural N2O emissions in PEI–comparable or higher than surface N2O emissions. This is likely because the majority of precipitation occurs in the non-cropping season in PEI, when conditions at the soil surface may be less favorable for denitrification and may prevent the release of N2O produced in deeper soil layers to the atmosphere. However, large amounts of NO3− are still lost from these tile drainage systems.
Temporal variation in dissolved N2O
N2O loss in tile drainage water was strongly influenced by season with up to 62% of N2O emissions occurred during the non-growing season (Figure 2A). One or two high N2O emission events can contribute a significant amount for that particular year (Figure 2B). Dissolved N2O concentrations exhibited high temporal variability and were independent of the volume of tile flow (data not shown). For instance, in 2003, the highest and lowest dissolved N2O concentrations (3.8 mg N L−1, 0.01 mg N L−1) were observed within a timespan of several days during the same flow event. A study of dissolved N2O emissions over a 36-h monitoring period observed a mean concentration of 0.48 mg N L−1, a coefficient of variation of 46%, with values ranging from 0.13 to 0.81 mg N L−1. Reay et al. (2003) have observed similar variation in dissolved N2O in agricultural drainage waters. Peak dissolved N2O concentrations (4.2 mg N L−1) recorded in this study were higher than those reported by others (Dowdell et al., 1979; Hack and Kaupenjohann, 2002; Hasegawa et al., 2000; Minami and Fukushi, 1984; Minami and Oshawa, 1990; Reay et al., 2003; Sawamoto et al., 2002; Sawamoto et al., 2003).
Over the monitoring period dissolved N2O concentrations of water collected from tile lines ranged from less than 0.01 mg N L−1–4.2 mg N L−1 (Figure 2B). The highest dissolved N2O concentrations occurred during winter 2003 (Figure 2B). A continuous frozen layer was present from 27 November 2002 to 29 April 2003, which may have prevented gas phase transport of N2O to the soil surface and resulted in an accumulation of N2O in the atmosphere of subsurface layers (Burton and Beauchamp, 1994). Elevated N2O concentrations in the soil atmosphere would result in greater N2O dissolution in soil water and a supersaturated soil solution. As N2O is highly soluble in cold water, there is a high probability that the water draining through the soil profile at this time would contain a high concentration of dissolved N2O (Goodroad and Keeney, 1984). In the tile flow of 2001–2002 and 2003–2004, N2O emissions were much lower than 2002–2003. The winter pattern is similar for both years, with the soil at 5 cm depth freezing only for a few days at a time. Furthermore, there was precipitation at regular intervals from November to March in both 2001–2002 and 2003–2004 (Figures 1A, C). The lack of a continuous frozen layer and regular precipitation would favor more rapid NO3− leaching, reducing the opportunity for N2O production and accumulation in the subsurface. The relative contribution of surface and sub-surface pathways appears to site-specific and may vary significantly from year to year.
Relationship between dissolved NO3 and dissolved N2O
During the observation period, dissolved NO3− emissions from tile lines fluctuated between 0.09 and 39 mg N L−1 (Figure 2B). In general, the NO3− concentrations observed in this study are comparable to previous studies conducted at this site (Milburn et al., 1997) and to values reported by others (Dowdell et al., 1979; Hack and Kaupenjohann, 2002; Hasegawa et al., 2000; Minami and Fukushi, 1984; Minami and Oshawa, 1990; Quesnel et al., 2019; Reay et al., 2003; Sawamoto et al., 2002; Sawamoto et al., 2003). The timing of manure and fertilizer applications to the potato crop (fall 2000, spring 2001, fall 2003, spring 2004) influenced the magnitude of NO3− loss in tile water. After the potato harvest in 2001, the research plots were left fallow. The NO3− not utilized by the potato crop and accumulating in the soil increased the loss of NO3− in the tile flow during 2001–2002 non-growing period (Figure 2B). Low rates of nitrogen fertilizer (40 kg N ha−1) were applied to barley crop across all N treatments in 2002, reducing fall NO3− accumulation in the soil and the loss of NO3− via tile drainage flow during the 2002–2003 non-growing period (Figure 2B).
There was a significant (p < 0.01) negative correlation between dissolved N2O-N and NO3−-N concentration for individual observations, but this accounted for less than 10% of the total variation in the data (R2 = 0.05; Figure 5A). These results are similar to Sawamoto et al. (2003) and Reay et al. (2004), who both found little or no relation between N2O-N and NO3−-N concentration in agricultural drainage water. In contrast others have observed a positive relationship between dissolved N2O-N and NO3−-N in agricultural drainage waters (Hack and Kaupenjohann, 2002; Minami and Oshawa, 1990; Reay et al., 2003). Dowdell et al. (1979) and Hack and Kaupenjohann (2002) have noted NO3− concentrations are typically one to three orders of magnitude higher than N2O concentrations. In our study N2O and NO3− concentration were one to two orders of magnitude greater than dissolved N2O concentrations, depending on season (Table 3A).
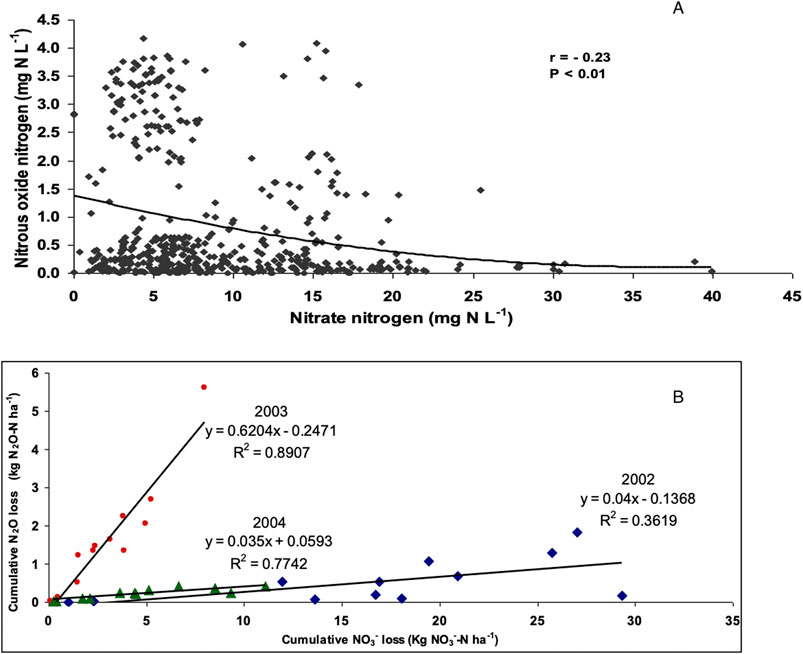
Figure 5. (A) Daily N2O-N loss vs. NO3-N loss in tile drainage water from a potato - barley - red clover rotation for 2002, 2003 and 2004, and (B) Cumulative N2O-N loss vs. cumulative NO3-N loss for 2002, 2003 and 2004.
The strength of the relationship between dissolved N2O-N and NO3−-N was much greater when cumulative losses were examined. A significant (p < 0.001) positive relationship existed between cumulative annual N2O-N and NO3−-N losses (Figure 5B). The slope of this relationship was significantly greater in 2003 than in the other 2 years of study. The relationships within individual years accounted for a much greater percentage of the total variation ranging from 36% to 89% of the total variation (Figure 5B).
The difference in the relationship between N2O and NO3− concentrations in drainage water for individual sampling events (Figure 5A) vs. cumulative annual N losses (Figure 5B) provides an interesting contrast. The rather weak negative relationship in the weekly samples indicates that the processes resulting in these losses are independent at this time scale or that these processes are occurring at different location and are thus exposed to different environmental conditions. However, the positive relationship between N2O and NO3− for each year of observation indicates that over this longer timeframe both processes appear to be responding to similar controls. In essence the leakiness of the system with respect to nitrogen is similarly expressed in terms of both of NO3− and N2O losses. These losses simply are not observed at the same time at the end of the tile drain. These results are similar to Quesnel et al. (2019), who observed a significant positive relationship between mean dissolved N2O-N and NO3−-N concentrations during the growing season, however they found that the relationship became weaker in the non growing season. They attributed this to the greater complexity of N2O production and transport in the non-growing season. Overall, our results indicate that a positive relationship exists between NO3− and N2O, but that these processes are spatially and/or temporally separate.
IPCC coefficient and dissolved N2O emissions
Currently the IPCC coefficients for N2O emissions from agricultural drainage is calculated as a fixed fraction of the NO3−-N estimated to be lost from the soil profile (EF5 = 0.0075 kg N2O-N kg−1 N leached). Here, on an annual basis the ratio of kg N2O-N lost per kg NO3−-N leached ranged from 0.035 to 0.62 kg N2O-N kg−1 N leached. These values are significantly higher than the IPCC coefficient for this site in these years and therefore would underestimate the actual N2O emissions at this site.
Dissolved N2O concentration in different water systems
To determine whether the water being discharged from the agricultural fields was enriched relative to the adjacent streams, water samples were collected on 28 May 2004 from subsurface tile drainage, stream and spring water. These samples displayed significant variation in dissolved N2O concentrations (Table 4). Dissolved N2O concentrations in stream and spring water were 8.4–33.1 μg N L−1 (mean 18.2 μg N L−1) and 9.0–67.1 μg N L−1 (mean 36.1 μg N L−1), respectively, while N2O concentrations of water being discharged from the tile drainage system ranged from 4.0 to 778 μg N L−1(mean 372 μg N L−1). This indicates that the tile drainage water was enriched in dissolved N2O prior to being released into the waterway. Low N2O concentrations in stream water were likely reflect the degassing of dissolved N2O degassing to the atmosphere following discharge, as was observed over the length of the tile line. This suggests that large amounts of indirect N2O loss can occur after the tile drain water enters the stream.
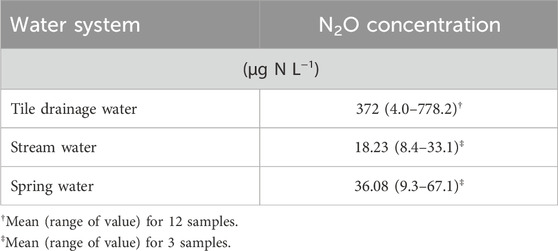
Table 4. Mean and range of values of N2O concentrations in tile drainage water, stream water and spring water.
Conclusions
Annual tile drainage losses of dissolved N2O from this site were substantive, approaching that of surface N2O emissions. The nature of the winter period had important implications for the magnitude and timing of dissolved N2O emissions. The presence of a continuous period of sub-zero soil and air temperatures resulted in greater dissolved N2O emissions than occurred under more moderate winter temperatures. There was significant spatial and temporal complexity in the nature of dissolved N2O emissions that complicated their characterization at a field scale.
Nitrogen application treatments, source and timing had no effect on the magnitude of dissolved N2O loss. On an event basis, dissolved N2O emissions were not correlated with NO3− concentrations. Dissolved N2O and NO3− losses appeared to be occurring at different times, however, examined on a seasonal basis, plots with high NO3− losses also exhibited high dissolved N2O loss.
Subsurface tile length influenced N2O discharge from subsurface tile drainage water, indicating the presence of a sink within the system. Consequently, our results likely underestimate N2O produced at the field level. More study is needed to understand the magnitude and fate of these N2O losses.
Data availability statement
The raw data supporting the conclusions of this article will be made available by the authors, without undue reservation.
Author contributions
DB: Conceptualization, Formal Analysis, Funding acquisition, Investigation, Methodology, Project administration, Resources, Supervision, Writing–original draft, Writing–review and editing. HW: Data curation, Formal Analysis, Visualization, Writing–original draft, Writing–review and editing. JM: Conceptualization, Investigation, Methodology, Supervision, Writing–original draft, Writing–review and editing.
Funding
The author(s) declare that financial support was received for the research, authorship, and/or publication of this article. We would also like to acknowledge Agriculture and Agri-Food Canada’s Biological Greenhouse Gas Sources and Sinks program, Nova Scotia Department of Agriculture, and Natural Sciences and Engineering Research Council for funding this research.
Acknowledgments
We would like to acknowledge the technical assistance of Balakumar Thangaraj, Brian Murray, and Drucie Janes in the collection and analysis of NO3− and N2O concentration of tile water samples.
Conflict of interest
The authors declare that the research was conducted in the absence of any commercial or financial relationships that could be construed as a potential conflict of interest.
Publisher’s note
All claims expressed in this article are solely those of the authors and do not necessarily represent those of their affiliated organizations, or those of the publisher, the editors and the reviewers. Any product that may be evaluated in this article, or claim that may be made by its manufacturer, is not guaranteed or endorsed by the publisher.
Supplementary material
The Supplementary Material for this article can be found online at: https://www.frontiersin.org/articles/10.3389/fenvs.2024.1479754/full#supplementary-material
References
Aulakh, M. S., Rennie, D. A., and Paul, E. A. (1984). Gaseous nitrogen losses from soils under zero-till as compared with conventional-till management systems. J. Environ. Qual. 13 (1), 130–136. doi:10.2134/jeq1984.00472425001300010024x
Baggs, E. M. (2011). Soil microbial sources of nitrous oxide: recent advances in knowledge, emerging challenges and future direction. Curr. Opin. Environ. Sustain. 3 (5), 321–327. doi:10.1016/j.cosust.2011.08.011
Bruun, J., Hoffmann, C. C., and Kjaergaard, C. (2017). Convective transport of dissolved gases determines the fate of the greenhouse gases produced in reactive drainage filters. Ecol. Eng. 98, 1–10. doi:10.1016/j.ecoleng.2016.10.027
Burton, D. L., and Beauchamp, E. G. (1985). Denitrification rate relationships with soil parameters in the field. Commun. Soil Sci. Plant Anal. 16, 539–549. doi:10.1080/00103628509367626
Burton, D. L., and Beauchamp, E. G. (1994). Profile nitrous oxide and carbon dioxide concentrations in a soil subject to freezing. Soil Sci. Soc. Am. J. 58, 115–122. doi:10.2136/sssaj1994.03615995005800010016x
Campbell, A. J., Van Lunen, T. A., MacLeod, J. A., Hurnik, D., and Linkletter, G. (2003). Design and performance of a swine finishing barn for production and manure research. Can. Biosyst. Eng. 45, 5.1–5.6.
Chapuis-Lardy, L. Y. D. I. E., Wrage, N., Metay, A., Chotte, J. L., and Bernoux, M. (2007). Soils, a sink for N2O? A review. Glob. Change Biol. 13 (1), 1–17. doi:10.1111/j.1365-2486.2006.01280.x
Choudhary, M. A., Akramkhanov, A., and Saggar, S. (2002). Nitrous oxide emissions from a New Zealand cropped soil: tillage effects, spatial and seasonal variability. Agric. Ecosyst. Environ. 93, 33–43. doi:10.1016/s0167-8809(02)00005-1
DelVecchia, A. G., Rhea, S., Aho, K. S., Stanley, E. H., Hotchkiss, E. R., Carter, A., et al. (2023). Variability and drivers of CO2, CH4, and N2O concentrations in streams across the United States. Limnol. & Oceanogr. 68 (2), 394–408. doi:10.1002/lno.12281
Dowdell, R. J., Burford, J. R., and Crees, R. (1979). Losses of nitrous oxide dissolved in drainage water from agricultural land. Nature 278, 342–343. doi:10.1038/278342a0
Drury, C. F., McKenney, D. J., Findlay, W. I., and Gaynor, J. D. (1993). Influence of tillage on nitrate loss in surface runoff and tile drainage. Soil Sci. Soc. Am. J. 57, 797–802. doi:10.2136/sssaj1993.03615995005700030028x
ECCC (Environment and Climate Change Canada) (2022). National inventory Report 1990-2020: Greenhouse gas sources and sinks in Canada. Canada: Ottawa.
Flessa, H., Ruser, R., Schilling, R., Loftfield, N., Munch, J. C., Kaiser, E. A., et al. (2002). N2O and CH4 fluxes in potato fields: automated measurement, management effects and temporal variation. Geoder 105, 307–325. doi:10.1016/s0016-7061(01)00110-0
Gauder, M., Butterbach-Bahl, K., Graeff-Hnninger, S., Claupein, W., and Wiegel, R. (2012). Soil-derived trace gas fluxes from different energy crops - results from a field experiment in southwest Germany. Great Britain: Blackwell Publishing Ltd.
Gillam, K. M., Zebarth, B. J., and Burton, D. L. (2007). Nitrous oxide emissions from denitrification and the partitioning of gaseous losses as affected by nitrate and carbon addition and soil aeration. Can. J. Soil Sci. 88, 133–143. (in press). doi:10.4141/cjss06005
Goodroad, L. L., and Keeney, D. R. (1984). Nitrous oxide emissions from soils during thawing. Can. J. Soil Sci. 64, 187–194. doi:10.4141/cjss84-020
Government of Canada (2019). Canadian climate normals 1981-2010 station data. Available at: https://climate.weather.gc.ca/climate_normals/results_1981_2010_e.html?searchType=stnProv&lstProvince=PE&txtCentralLatMin=0&txtCentralLatSec=0&txtCentralLongMin=0&txtCentralLongSec=0&stnID=6526&dispBack=0 (Accessed December 1, 2023).
Granli, T., and Bøckman, O. C. (1994). Nitrous oxide from agriculture. Norw J. Agric. Sci. Suppl. 12, 128.
Hack, J., and Kaupenjohann, M. (2002). “N2O discharge with drain water from agricultural soils of the upper Neckar region in southern Germany,” in Proc. Third int. Symp. Non-CO2 Greenhouse gases: scientific understanding, control options and policy aspects, maastricht, Netherlands. 21-23 january 2002. Editors J. Van Ham, A. P. M. Baede, R. Guicherit, and J. G. F. M. Williams-Jacobse (Millipress, Rotterdam, Netherlands), 185–190.
Hama-Aziz, Z. Q., Hiscock, K. M., and Cooper, R. J. (2016). Indirect nitrous oxide emission factors for agricultural field drains and headwater streams. Environ. Sci. & Technol. 51 (1), 301–307. doi:10.1021/acs.est.6b05094
Hama-Aziz, Z. Q., Hiscock, K. M., and Cooper, R. J. (2017). Dissolved nitrous oxide (N2O) dynamics in agricultural field drains and headwater streams in an intensive arable catchment. Hydrol. Process. 31 (6), 1371–1381. doi:10.1002/hyp.11111
Hasegawa, K., Hanaki, K., Matsuo, T., and Hidaka, S. (2000). Nitrous oxide from the agricultural water system contaminated with high nitrogen. Chemosphere Glob. Change Sci. 2, 335–345. doi:10.1016/s1465-9972(00)00009-x
Heincke, M., and Kaupenjohann, M. (1999). Effects of soil solution on the dynamics of N2O emissions: a review. Nutr. Cycl. Agroecosys. 55, 133–157. doi:10.1023/a:1009842011599
Hutchinson, G., and Livingston, G. (1993). “Use of chamber systems to measure trace gas fluxes. Pages 63–78,” in Agricultural ecosystem effects on trace gases and global climate change. Editors L. A. Harper, A. R. Mosier, J. M. Duxbury, and D. E. Rolston (Madison, WI: ASA, CSSA, SSSA). Special Publication No 55.
IPCC (2019). in 2019 refinement to the 2006 IPCC guidelines for national Greenhouse gas inventories. Volume 4 agriculture, forestry and other land use. Editors E. Calvo Buendia, K. Tanabe, A. Kranjc, J. Baasansuren, M. Fukuda, S. Ngarizeet al. Published: IPCC, Switzerland.
IPCC (2021). in Climate change 2021: the physical science basis. Contribution of working group I to the sixth assessment Report of the intergovernmental panel on climate change. Editors V. Masson-Delmotte, P. Zhai, A. Pirani, S. L. Connors, C. Péan, S. Bergeret al. (Cambridge, United Kingdom and New York, NY, USA: Cambridge University Press). In press. doi:10.1017/9781009157896
IPCC (2022). in Climate change 2022: mitigation of climate change. Contribution of working group III to the sixth assessment Report of the intergovernmental panel on climate change. Editors P. R. Shukla, J. Skea, R. Slade, A. Al Khourdajie, R. van Diemen, D. McCollumet al. (Cambridge, UK and New York, NY, USA: Cambridge University Press). doi:10.1017/9781009157926
IPCC (2023). “Climate change 2023: synthesis Report,” in Contribution of working groups I, II and III to the sixth assessment Report of the intergovernmental panel on climate change core writing team. Editors H. Lee, and J. Romero (Geneva, Switzerland: IPCC), 35–115. doi:10.59327/IPCC/AR6-9789291691647
Janzen, H. H., Desjardins, R. L., Asselin, J. M. R., and Grace, B. (1999). The health of our air: toward a sustainable agriculture in Canada. Ottawa, Canada: Agriculture and Agri Food Canada Publication# 1981/E. Available at: https://publications.gc.ca/site/eng/9.686880/publication.html
Jiang, Y., Zebarth, B., and Love, J. (2011). Long-term simulations of nitrate leaching from potato production systems in Prince Edward Island, Canada. Nutrient Cycl. Agroecosyst. Former. Fertilizer Res. 91 (3), 307–325. doi:10.1007/s10705-011-9463-z
Jiang, Y., Zebarth, B. J., Somers, G. H., MacLeod, J. A., and Savard, M. M. (2012). Nitrate leaching from potato production in eastern Canada. Sustain. Potato Prod. Glob. Case Stud., 233–250. doi:10.1007/978-94-007-4104-1_13
Kavdir, Y., Hellebrand, H. J., and Kern, J. (2008). Seasonal variations of nitrous oxide emission in relation to nitrogen fertilization and energy crop types in sandy soil. Soil & Tillage Res. 98, 175–186. doi:10.1016/j.still.2007.11.002
Klavidko, E. J., Van Scoyoc, G. E., Monke, E. J., Oates, K. M., and Pask, W. (1991). Pesticide and nutrient movement into subsurface tile drains on a silt loam soil in Indiana. J. Environ. Qual. 20, 264–270. doi:10.2134/jeq1991.00472425002000010043x
Maag, M., and Vinther, F. (1996). Nitrous oxide emission by nitrification and denitrification in different soil types and at different soil moisture contents and temperatures. Appl. Soil Ecol. 4, 5–14. doi:10.1016/0929-1393(96)00106-0
MacDougall, J. I., Veer, C., and Wilson, F. (1988). Soils of Prince Edward Island: Prince Edward Island soil sur vey. Research Branch. Agriculture Canada.
Milburn, P., Macleod, J. A., and Sanderson, B. (1997). Control of nitrate leaching from early harvested potatoes on Prince Edward Island. Can. Agri. Eng. 39, 263–271.
Minami, K., and Fukushi, S. (1984). Methods for measurement N2O flux from water surface and N2O dissolved in water from agricultural land. Soil Sci. Plant Nutr. 30, 495–502. doi:10.1080/00380768.1984.10434716
Minami, K., and Oshawa, A. (1990). “Emission of nitrous oxide dissolved in drainage water from agricultural land,” in Soils and the Greenhouse effect. Editor A. F. Bouman (Chichester, UK: John Wiley & Sons), 503–509.
Nangia, V., Gowda, P. H., Mulla, D. J., and Sands, G. R. (2010). Modeling impacts of tile drain spacing and depth on nitrate-nitrogen losses. Artif. Drain. 9 (1), 61–72. doi:10.2136/vzj2008.0158
Nevison, C. (2000). Review of the IPCC methodology for estimating nitrous oxide emissions associated with agricultural leaching and runoff. Chemosphere Glob. Change Sci. 2, 493–500. doi:10.1016/s1465-9972(00)00013-1
Quesnel, J., VanderZaag, A., Crolla, A., Kinsley, C., Gregorich, E., and Wagner-Riddle, C. (2019). Surface and subsurface N2O losses from dairy cropping systems. Nutr. Cycl. Agroecosyst 114, 277–293. doi:10.1007/s10705-019-10004-5
Randall, G. W., Huggins, D. R., Russelle, M. P., Fuchs, D. J., Nelson, W. W., and Anderson, J. L. (1997). Nitrate losses through subsurface tile drainage in conservation reserve program, alfalfa and row crop systems. J. Environ. Qual. 26, 1240–1247. doi:10.2134/jeq1997.00472425002600050007x
Reay, D., Edwards, A., and Smith, K. (2004). Nitrous oxide in agricultural drainage waters following field fertilisation. Water Air Soil Pollut. Focus 4, 437–451. doi:10.1023/b:wafo.0000028370.68472.d2
Reay, D. S., Edwards, A. C., and Smith, K. A. (2009). Importance of indirect nitrous oxide emissions at the field, farm and catchment scale. Agric. Ecosyst. & Environ. 133 (3/4), 163–169. doi:10.1016/j.agee.2009.04.019
Reay, S. D., Smith, K. A., and Edwards, C. A. (2003). Nitrous oxide emission from agricultural drainage waters. Glob. Change Biol. 9, 195–203. doi:10.1046/j.1365-2486.2003.00584.x
Risk, N., Snider, D., and Wagner-Riddle, C. (2013). Mechanisms leading to enhanced soil nitrous oxide fluxes induced by freeze-thaw cycles. Can. J. Soil Sci. 93 (4), 401–414. doi:10.4141/cjss2012-071
Risk, N., Wagner-Riddle, C., Furon, A., Warland, J., and Blodau, C. (2014). Comparison of simultaneous soil profile N2O concentration and surface N2O flux measurements overwinter and at spring thaw in an agricultural soil. Soil Sci. Soc. Am. J. 78 (1), 180–193. doi:10.2136/sssaj2013.06.0221
Rochette, P., and McGinn, S. M. (2008). N2O emissions from agricultural soils in Canada (preface) can. J. Soil Sci. 88 (2), 131–132. doi:10.4141/cjss07301
Roper, J. (2008). Influence of tillage practices on surface and subsurface nitrogen emissions from agricultural soils. Halifax, NS: Dalhousie University. MSc thesis.
Roper, J., Burton, D., Madani, A., and Stratton, G. (2013). A simple method for quantifying dissolved nitrous oxide in tile drainage water. Can. J. Soil Sci. 93 (1), 59–64. doi:10.4141/cjss2012-021
Sawamoto, T., Kusa, K., Hu, R., and Hatano, R. (2002). Dissolved N2O, CH4 and CO2 in pipe drainage, seepage and stream water in a livestock farm in Hokkaido, Japan. Soil Sci. Plant Nutr. 48, 433–439. doi:10.1080/00380768.2002.10409222
Sawamoto, T., Kusa, K., Hu, R., and Hatano, R. (2003). Dissolved N2O, CH4 and CO2 emissions from subsurface drainage in a structured clay soil cultivated with onion in central Hokkaido, Japan. Soil Sci. Plant Nutr. 49, 31–38. doi:10.1080/00380768.2003.10409976
Shakoor, A., Shakoor, S., Rehman, A., Ashraf, F., Abdullah, M., Shahzad, S. M., et al. (2021). Effect of animal manure, crop type, climate zone, and soil attributes on greenhouse gas emissions from agricultural soils-A global meta-analysis. J. Clean. Prod. 278, 1–13. doi:10.1016/j.jclepro.2020.124019
Syakila, A., and Kroeze, C. (2011). The global nitrous oxide budget revisited. Greehn. Gas. Meas. Manage. 1, 17–26. doi:10.3763/ghgmm.2010.0007
Tian, L., Cai, Y., and Akiyama, H. (2019). A review of indirect N2O emission factors from agricultural nitrogen leaching and runoff to update of the default IPCC values. Environ. Pollut. 245, 300–306. doi:10.1016/j.envpol.2018.11.016
Valayamkunnath, P., Gochis, D. J., Chen, F., Barlage, M., and Franz, K. J. (2022). Modeling the hydrologic influence of subsurface tile drainage using the national water model. Water Resour. Res. 58 (4). doi:10.1029/2021WR031242
van Es, H. M., Sogbedji, J. M., and Schindelbeck, R. R. (2006). Effect of manure application timing, crop, and soil type on nitrate leaching. J. Environ. Qual. 35 (2), 670–679. doi:10.2134/jeq2005.0143
Venterea, R., Bijesh, M., and Dolan, M. (2011). Fertilizer source and tillage effects on yield-scaled nitrous oxide emissions in a corn cropping system. J. Environ. Qual. 40 (5), 1521–1531. doi:10.2134/jeq2011.0039
Wagner-Riddle, C., Congreves, K., Abalos, D., Berg, A., Brown, S., Ambadan, J., et al. (2017). Globally important nitrous oxide emissions from croplands induced by freeze-thaw cycles. Nat. Geosci. 10, 279–283. doi:10.1038/ngeo2907
World Meteorological Organization (2014). Scientific assessment of oxone depletion: 2014 global ozone research and monitoring project-rep, 56. Geneva, Switzerland: World Meteorological Organization.
Keywords: nitrous oxide-N2O, drainage water, nitrogen loss by drainage, nitrate, greenhouse gas emmissions, nitrogen source, nitrogen timing
Citation: Burton DL, Wilts HDM and MacLeod JA (2024) Dissolved nitrous oxide emissions associated with agricultural drainage water as influenced by manure application. Front. Environ. Sci. 12:1479754. doi: 10.3389/fenvs.2024.1479754
Received: 12 August 2024; Accepted: 11 November 2024;
Published: 06 December 2024.
Edited by:
Jana Levison, University of Guelph, CanadaReviewed by:
Ziyi Li, University of Illinois at Urbana-Champaign, United StatesHao Wang, University of Minnesota Twin Cities, United States
Copyright © 2024 Burton, Wilts and MacLeod. This is an open-access article distributed under the terms of the Creative Commons Attribution License (CC BY). The use, distribution or reproduction in other forums is permitted, provided the original author(s) and the copyright owner(s) are credited and that the original publication in this journal is cited, in accordance with accepted academic practice. No use, distribution or reproduction is permitted which does not comply with these terms.
*Correspondence: D. L. Burton, ZGJ1cnRvbkBkYWwuY2E=