- 1Leibniz Centre for Agricultural Landscape Research (ZALF), Müncheberg, Germany
- 2Soil Resources and Land Use, Institute of Soil Science and Site Ecology, TUD Dresden University of Technology, Tharandt, Germany
Phosphorus limitation occurs in many soils as a significant amount of soil P is retained in forms inaccessible to plants, such as bound to iron (Fe) minerals. Prior studies have shown that silicon (Si) can mobilize P from the binding sites of such minerals. Several P extraction methods have been developed to account for different P pools. Nevertheless, each of those methods uses different extractants and mechanisms to extract different P pools in soils. However, there is no study comparing different P extraction methods in the presence of Si fertilization. We tested the effect of Si on P mobility and determined the efficiency of water, Calcium acetate lactate (CAL), Mehlich-III, and Bray and Kurtz extraction methods for extracting P in the presence of amorphous silica (ASi) fertilization using two soils of contrasting characteristics. Significantly higher amounts of P were found at 3% ASi treatments (10 and 21 mg P kg-1) compared to the control (4 and 10 mg P kg-1) in the water extract in the high and low-yield soil, respectively just after 6 hours of extraction and increased with time. This may be explained by Si directly competing with P for sorption to Fe minerals. Using CAL extraction, Si addition showed no effect on P extractability. In contrast, the Mehlich-III and Bray extraction methods showed decreasing P extractability, especially at 3% ASi treatment (95 and 60 mg P kg-1) compared to the control (115 and 80 mg P kg-1) for the high-yield soil. The decreasing P contents in the presence of Si found in the Mehlich-III and Bray extracts may be attributed to the decrease in extraction effectiveness of the extractants to extract P while extracting Si and Fe. Our results showed that the Mehlich-III and Bray extraction methods may not be suitable for the determination of P availability in the presence of ASi fertilization since both extractants also extract Si and this may limit the completeness of P extraction. Therefore, in the presence of Si fertilizer, the water extraction method may be suitable to determine P availability and mobilization due to ASi.
Introduction
Phosphorus (P) is an essential macronutrient for plants, and a lack of P can limit plant growth and yield (Malhotra et al., 2018). To improve crop yield the global demand for P fertilizers is rising by 2.5%–3.0% each year, which is expected to peak by 2030 (Bindraban et al., 2020). Because of the limited amounts of mineable phosphate minerals, the scarcity of P is viewed as a worldwide concern (Bindraban et al., 2020). Additionally, approximately 10%–25% of the P in fertilizers applied to soils are sorbed to soil minerals and are unavailable for plant uptake (López-Arredondo et al., 2014; Bindraban et al., 2020).
Biogeochemical factors, including soil mineral composition, significantly impact soil P availability (Ringeval et al., 2017). The total P content in soils is not necessarily low, but much of it is stored in plant-inaccessible forms like organic P (Bünemann et al., 2010) or P bound to aluminum (Al) and iron (Fe) oxides/hydroxides, or precipitated with calcium (Ca), depending on the physicochemical characteristics of the soil, especially soil pH (Beauchemin Suzanne et al., 2003). At soil pH > 6.5, inorganic P mostly precipitates with Ca forming slightly soluble calcium phosphate. At lower pH, P is adsorbed to Fe-, Mn-, Al-, or their hydrous oxides (Brady and Weil, 1999). At neutral pH, inorganic P adsorbed to e.g., clay minerals (Rajan, 1975a). Therefore, the distribution of inorganic P between Ca, Fe, Al, and silicon (Si) depends on soil pH, mineral composition, parent material, and soil formation stage.
The binding of P to soil minerals is shown to be lowest in the presence of silicate minerals for example, clay minerals with high silicon content (Rajan, 1975a; Brady and Weil, 1999). Dissolved Si species in soils are composed of monomeric silicic acid and polymeric silicic acid, and their release into soil solution is controlled by mineral weathering and dissolution (Dietzel, 2000; Schaller et al., 2021a). Particularly, the dissolved Si species may adsorbed to Fe or Al oxides/hydroxides (Sauer et al., 2006; Cornelis et al., 2010). The solid phase of Si is composed of different forms of amorphous Si (ASi) such as the biogenic amorphous Si (bASi, e. g., phytoliths, testate amoeba shells, diatom shells, silicified sponge spicules, radiolarian shells); and the minerogenic forms (silica nodules, silica included in pedogenic oxides such as iron oxides) (Schaller et al., 2021a). The concentration of silicic acid in soil solution varies from 0.4 to 2000 μmol L-1, with a typical range of 100–500 μmol L-1, and is dependent on the solubility of primary and secondary Si compounds (Sommer et al., 2006).
Studies have shown a positive correlation between Si availability and P release in soils (Schaller et al., 2019; Hömberg et al., 2020; Schaller et al., 2020; Schaller et al., 2022). According to Schaller et al. (2019), increasing Si availability in soils can mobilize P from the surface of Fe minerals, leading to increased P availability and mobility. Additionally, it was shown that Si disrupts the Fe mineralogy of paddy soils, competing with P for sorption, thereby leading to P mobilization and availability (Hömberg et al., 2020; Schaller et al., 2022). Under flooded conditions, Hömberg et al. (2021) showed increased P concentration with increased Si addition to peat in a field experiment.
Soil P is classified into four types: P dissolved in soil water, P sorbed to clay mineral surfaces or Fe and Al oxides/hydroxides, P in primary phosphate minerals, and P in organic compounds and living organisms (Wuenscher et al., 2015). To account for these different P pools, multiple approaches for extracting P from soil exist, and numerous soil P extraction methods have been developed (Fuhrman et al., 2005; Wuenscher et al., 2015). Even in standard soil testing, different extraction processes are utilized nowadays. For example, the Calcium acetate lactate (CAL) extract (Schüller, 1969) is used in regular soil testing for exchangeable P in Austria and Germany, whereas Mehlich-III (Mehlich, 1984) and Bray and Kurtz are utilized in the Czech Republic, major parts of Canada, and the United States (Gartley et al., 2002; Mylavarapu et al., 2014). Each of those methods uses different extractants and mechanisms, extracting different pools of P in soil samples. For example, Mehlich-III and Bray extracts may extract non-target Si (Schaller et al., 2021a) as those extractants contain fluoride subsequently decreasing the extraction effectiveness for P. The CAL extraction method may also extract non-target Si as the extractant contains acetic acid, and the acetic acid extraction method is also used as a routine test for Si (Wu et al., 2020; Schaller et al., 2021a). This may subsequently decrease the extraction effectiveness for P under high Si content. There is, however, no study comparing different methods of P extraction in the presence of Si fertilization, and the relationship between Si and P availability is still poorly understood.
The overall objective of the present study was to investigate and estimate the efficiency of different extraction methods for the extraction of available P in the presence of amorphous silica (ASi) fertilization and to determine if there is any interdependency between Si and P. Specifically, we aimed to 1) determine the effect of Si on P mobilization from soils and to 2) compare different extraction methods for available P. We hypothesized that 1) Si is positively related to P mobilization and 2) results should be independent of the extraction method used. Two different soils: low-yield (low P availability) and high-yield soils (high P availability) were collected from a field used for a landscape experiment in Tempelberg (Germany). This soil was mixed with amorphous silica (ASi) at different concentrations and then extracted for available P using water (Fuhrman et al., 2005; Schüller, 2007; Wuenscher et al., 2015), Mehlich-III extraction (Mehlich, 2008), extraction after Bray and Kurtz (Bray and Kurtz, 1945), CAL extraction (Schüller, 1969). Before mixing the soil with ASi, the initial soils were characterized and extracted for elemental compositions with dithionate and oxalate solution. Both dithionate and oxalate extractions are known to extract different forms of Fe (amorphous and crystalline), and their ratios have been used to determine the crystallinity of the Fe oxides (Mehra and Jackson, 2013). Also, understanding the chemical composition of the soil provides knowledge about the qualitative and quantitative functions of the soil regarding nutrient storage (Rennert, 2018; Oyebiyi, 2024).
Materials and methods
Sampling sites
In this study, we used soils from a landscape experimental field (Tempelberg, Germany, 52°26′37″N 14°09′39″E) (Donat et al., 2022). We selected two different plots to represent soils with different yields (low-yield soil and high-yield soil with different sand contents) and different initial P, Fe, and Al contents (Donat et al., 2022). The pedogenic Fe oxide content of the soils among other factors controls P adsorption/desorption. The agricultural landscape varied in topography with loamy sand to sandy loam as the dominant soil texture derived from glacial deposits. It is also composed of sandy deposits that are partially fine-grained and partly fine-silty (Donat et al., 2022; Koch et al., 2023). This place has an average yearly temperature of 9.6°C and 581 mm of precipitation. We collected three topsoil samples (0–20 cm) each from the two soils (low and high-yield soil) using an auger. The samples were air-dried and sieved through a 2 mm mesh size, and from each sampling site, the samples were mixed into a homogenized (composite) sample per plot. To avoid confounding effects of recent P applications on extractable P contents, samples were collected at least 6 months after the last P applications.
Soil characterization
The initial soil pH was measured based on the (DIN ISO, 1997) in a 0.01 M CaCl2 suspension at a soil-to-solution ratio of 1:2.5 after 1 h of equilibration using a glass electrode (pH/Cond 3320, Xylem Analytics Germany). The elemental concentrations of the soil were determined using dithionate and oxalate extraction at a solid-to-solution ratio of 1:10 (Mehra and Jackson, 2013) and 1:25 (Schwertmann, 1964), respectively just before mixing the soils with ASi. The extracts were filtered using 0.25 µm syringe filters (CHROMAFIL XTRA PET -20/25) and analyzed for Al, Fe, Mn, P, and Si using ICP-OES (Thermo Scientific iCAP 6000, England). Total organic carbon (TOC), total inorganic carbon (TIC), and total carbon (TC) contents of the soil were analyzed using a TOC analyzer (TNM-L Shimadzu, China).
Incubation experiment
In 100 g dried soils were added varying amounts (i.e. 0%, 0.2%, 1%, and 3%) of amorphous silica (Aerosil 300 Evonik, Germany, ASi) in four replicates each. The soil without ASi (0%) served as the control. Aerosil, a pyrogenic ASi was used because of its similar physicochemical properties to biogenic ASi. The samples were homogenized by placing them on an end-over-end shaker for 24 h. For the Mehlich -III, Bray and Kurtz, and CAL extraction, the soil-ASi mixtures were first saturated with ultra-pure water to ensure ASi dissolution and were stored at room temperature for 4 days. After 4 days of soil-ASi incubation, the samples were freeze-dried before extraction. For each soil, the following P extractions were performed: Mehlich-III extraction (Mehlich, 2008), Bray and Kurtz extraction (Bray and Kurtz, 1945), calcium acetate-lactate extraction (CAL) (Schüller, 1969), and water extraction (Fuhrman et al., 2005; Schüller, 2007; Wuenscher et al., 2015).
Water extraction
Water extraction of P was conducted at a soil-to-solution ratio of 1:5 according to Fuhrman et al. (2005) and Wuenscher et al. (2015) but with a longer equilibration time (1 week shaking) by adding 20 mL ultra-pure water to 4 g soil-ASi mixture. Sampling was conducted on a logarithmic timescale at five different times (after 6, 12, 24, 72, and 168 h).
Calcium acetate lactate, CAL extraction
The CAL extraction was performed at a solid-to-solution ratio of 1:20 (Schüller, 1969). Simply, 4 g of the dried soil-ASi mixture was weighed in 100 mL plastic bottles and mixed with 80 mL CAL extraction solution (0.05 M C6H10CaO6, 0.05 M (CH3COO)2Ca, 96% HAc). The samples were shaken for 2 h, and centrifuged at 10,000xg for 5 min.
Mehlich-III extraction
Mehlich-III extraction was conducted at a solid-to-solution ratio of 1:21 by adding 42 mL of Mehlich-III (0.015M NH4F, 0.001 M EDTA, 0.25 M NH4NO3, 0.013 M HNO3, and 0.2 M HAc) extraction solution to a 2 g soil-ASi mixture each in 50 mL falcon tubes (Mehlich, 2008). The samples were shaken on an end-over-end shaker for 5 min at 200 rpm and centrifuged for 5 min at 10,000xg.
Bray and Kurtz extraction
Extraction after Bray and Kurtz was performed at a solid-to-solution ratio of 1:10 by adding 20 mL Bray and Kurtz extraction solution (0.025 M HCl, 0.03 M NH4F) to a 2 g soil-ASi mixture in a 50 mL falcon tube (Bray and Kurtz, 1945). The samples were then shaken on an end-over-end shaker for 5 min at 200 rpm, and centrifuged at 10,000xg for 10 min.
All extracts were filtered through 0.45 µm syringe filters (CHROMAFIL XTRA PET -45/25). Except for the CAL extract, which was only analyzed for P (as it is a standard routine in our central lab measuring only P), all other extract was analyzed for P, Si, and Fe using inductively coupled plasma optical emission spectroscopy ICP-OES (Thermo Scientific iCAP 6000, U.K.).
Statistical analyses
Statistical significance was evaluated using analysis of variance (ANOVA) and visualization was done in the R computational environment (R Core Team, 2013). Figures were plotted using the “geom_barplot” function from the “ggplot2” package (Wickham, 2016).
Results
Characterization of the soils
The pH (CaCl2) differed between the soils, with the low-yield soils showing a pH of 5.1 ± 0.04 and the high-yield soils showing a pH of 6.4 ± 0.02, respectively. The elemental concentrations differed considerably between soils and extraction methods (Table 1). Overall, the elemental content measured was higher using dithionate than the oxalate extraction. For example, the high-yield soil showed a higher content of Fe, P, and Si (2682, 509, and 976 mg kg-1 soil) than the low-yield soil (2434, 232, and 905 mg kg-1 soil), respectively (Table 1) using dithionate extraction method. Overall, the P content was much higher in the high-yield soil compared to the low-yield soil.
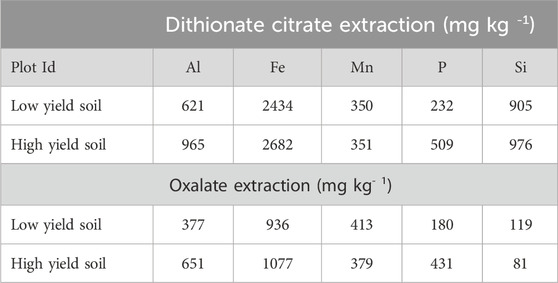
Table 1. Elemental compositions of the initial soil obtained from the two different soils (low and high yield) after oxalate and dithionate citrate extraction (mg kg-1).
We found 1077, 431, 81 mg kg-1 soil Fe, P, and Si for the high-yield soil and 936, 180, and 119 mg kg-1, Fe, P, and Si, respectively for the low-yield soils using the oxalate extraction (Tab. 1). The oxalate -and dithionate Fe (Feo/Fed) ratios are 0.4 and 0.4 for the low and high-yield soils. The carbon contents did not differ between both soils, with about 0.02% TIC, 1.2% TOC, and 1.2% TC for the high-yield soil and 0.03% TIC, 1.03% TOC, and 1.06% TC for the low-yield soil, respectively.
Water extractable P, Fe, and Si
We found a significant increase (p < 0.001, df = 3, F = 69.632; p < 0.001, df = 3, F = 1061.09) in water extractable P for the high and low-yield soils upon ASi addition throughout the incubation time (Figure 1A). Overall, the amount of water extractable P released due to ASi addition, for 3% treatment ranged from 8 to 11 mg P kg-1 soil for the high-yield soil and 20 and 29 mg P kg-1 soil for the low-yield soil, respectively (Figure 1A). In contrast, in the control, the amount of P released ranged from 3 to 4 mg P kg-1 for the high yield soil, and 9 and 11 mg P kg-1 soil for low-yield soil, respectively (Figure 1A). The P released upon ASi addition was higher for the low-yield soil than the high-yield soil and increased with incubation time. The contents of Si in the ASi treatment were higher than in the control treatment (Figure 1B) for both soils. For example, the content of Si in the 3% ASi treatment (89 and 881 mg Si kg-1 soil) was significantly higher (p < 0.001) than in the control treatment (22 and 48 mg Si kg-1 soil) for the high-yield soil over the entire incubation time. The same holds true for the low-yield soil, with 41 and 624 mg Si kg-1 soil in the 3% ASi treatment and 24 and 101 mg Si kg-1 soil in the control (p < 0.001). A decrease (p < 0.001) in the content of water-extractable Fe was found due to ASi addition (especially for the 3% ASi treatment) except for the high-yield soil after 72 h of incubation (Figure 1C). The Fe content in both soils decreased with increased ASi addition (Figure 1C). In the high-yield soil, Fe released during incubation resulted in contents ranging from 12 to 19 mg Fe kg-1 soil in the control treatment, and 3 and 6 mg Fe kg-1 soil in the 3% ASi treatment (Figure 1C). Similar patterns were observed, with decreased Fe content due to ASi addition for the low-yield soil (Figure 1C).
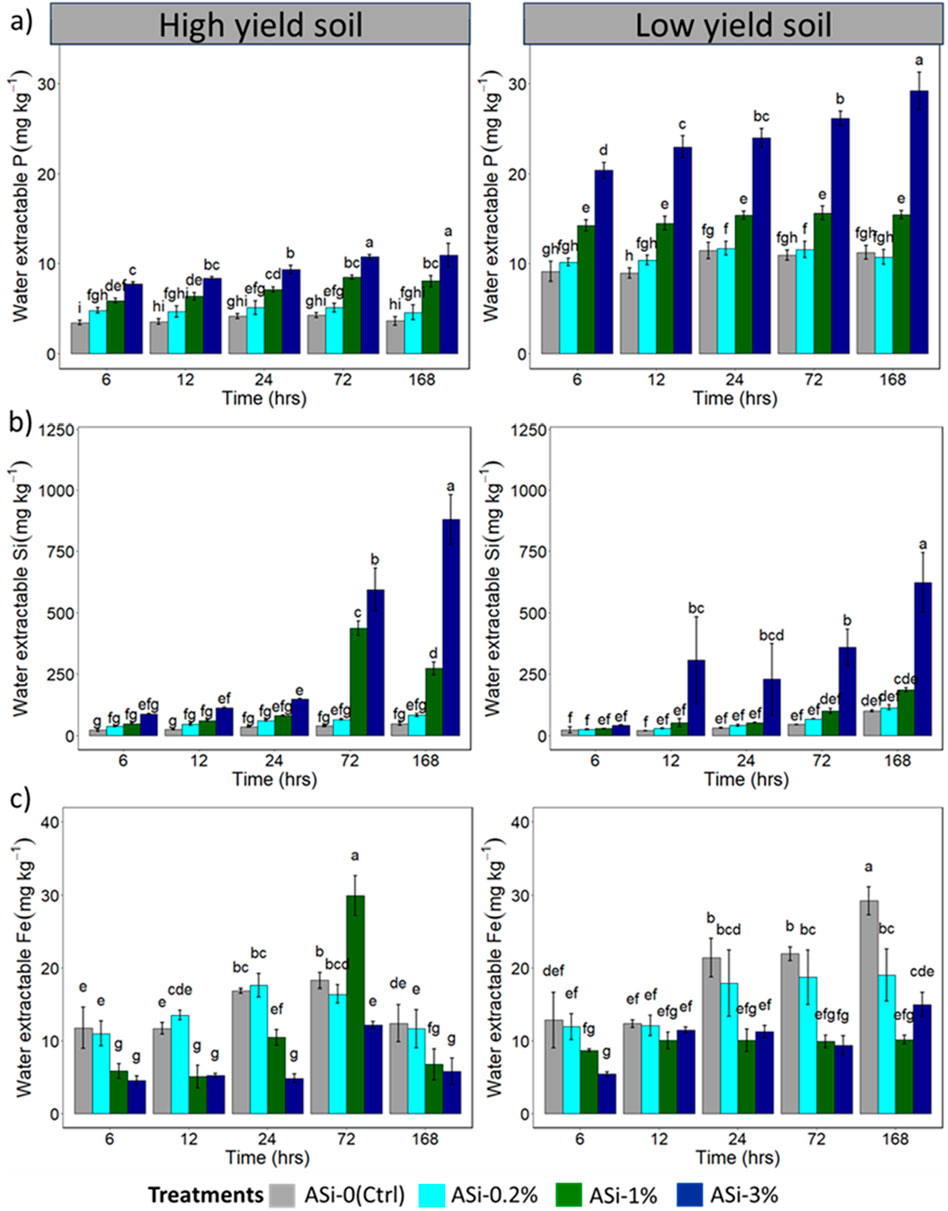
Figure 1. Water-extractable (A) P (B) Si, and (C) Fe (for high and low yield soil) at 6, 12, 24, 72, and 168 h extraction time in mg P kg-1 after incubating soil with and without (control) different Si concentrations. The data showed the relationship between Si concentration and plant-available P along the experimental soil ASi gradient (n = 4). The bar charts represent the mean, and the error bars represent the standard deviation. Treatments with a common letter are not significantly different (p > 0.05).
CAL extractable P
Using the CAL extraction method, we found no significant effects (p = 0.18, df = 3, F = 1.874; p = 0.09, df = 3, F = 2.668) of ASi addition on P extracted from both soils (Figures 2A, B). However, we detected higher P contents in the low-yield soil than in the high-yield soil irrespective of the treatment.
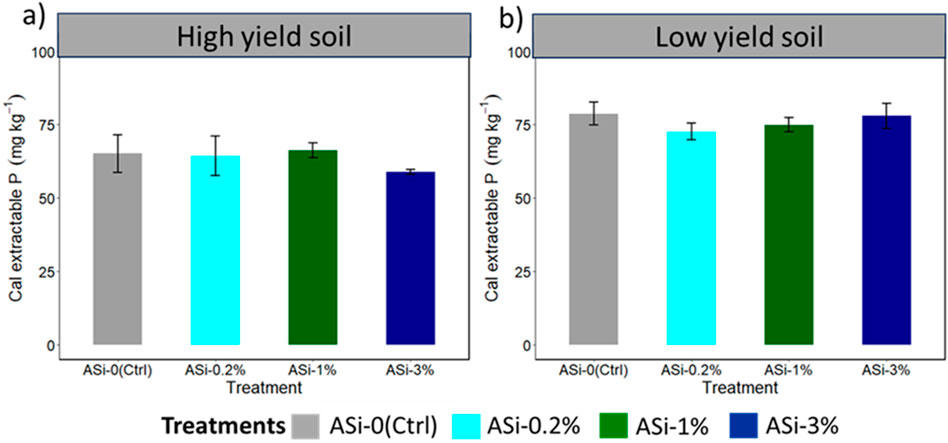
Figure 2. Cal extractable P after incubating soil with different ASi content for (A) high and (B) low-yield soils. The bar charts represent the mean, and the error bars represent the standard deviation (n = 4).
Mehlich-III extractable P, Si, and Fe
The content of P in the Mehlich-III extracts significantly decreased (p < 0.001, df = 3, F = 13.95; p = 0.01, df = 3, F = 4.939) upon ASi addition for both soils (Figure 3A). However, a higher amount of P was released from the low-yield soil than from the high-yield soil irrespective of the treatment. The control treatment showed a P content of 114 mg P kg-1 soil for the high-yield soil which did not differ significantly from the 0.2% ASi treatment (115 mg P kg-1 soil, p = 0.92) but significantly differed from the 1% (95 mg P kg-1 soil, p = 0.01) and 3% ASi treatment (94 mg P kg-1 soil, p = 0.01). In the low-yield soil, P released from the control was 304 mg P kg-1 soil and decreased to 268, 266, and 265 mg P kg-1 soil (p = 0.005, 0.05, 0.01) in the 0.2%, 1% 3% ASi treatments. Extracted Si content significantly increased (p < 0.001, df = 3, F = 480.5; p = 0.001, df = 3, F = 922.4) with ASi addition for both soils (Figure 3B). For instance, the control showed a Si content of 549 mg Si kg-1 soil, which increased upon ASi addition to 993 mg Si kg-1 soil in the 3% ASi treatment for the high yield soil. The low-yield soil followed a similar pattern with increased Si content upon ASi addition. A significant decrease (p < 0.001, df = 3, F = 29.97; p = 0.001, df = 3, F = 44.73) in Fe content was found upon ASi addition for both soils (Figure 3C). About 489 mg Fe kg-1 soil was found in the control, which then decreased to 264 mg Fe kg-1 soil in the 3% ASi treatments for the high yield soil. For the low-yield soil, 540 mg Fe kg-1 soil was found in the control and then decreased to 378 mg Fe kg-1 soil in the 3% treatments.
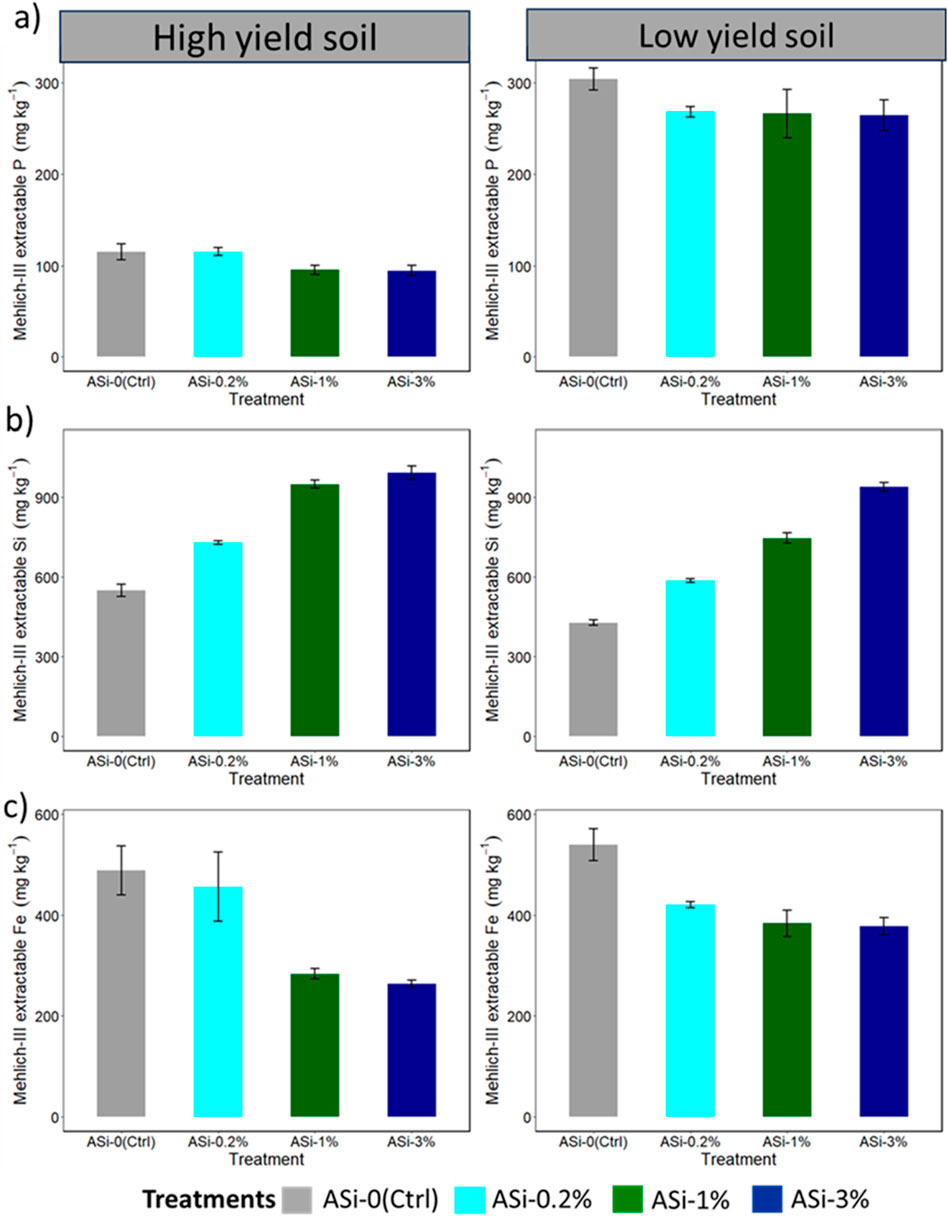
Figure 3. Mehlich-III extractable (A) P, (B) Si, and (C) Fe after incubating soil with different ASi content for high and low-yield soils. The bar charts represent the mean, and the error bars represent the standard deviation (n = 4).
Bray and Kurtz extractable P, Si, and Fe
Similar to Mehlich-III extractable P, the P content in the Bray and Kurtz extract significantly decreased (p < 0.001, df = 3, F = 63.49; p = 0.01, df = 3, F = 14.17) upon ASi addition for both soils (Figure 4A). The P content in the control was found to be 80 mg P kg-1 soil and then decreased upon ASi addition to 60 mg P kg-1 soil in the 3% ASi treatment (Figure 4A) for the high-yield soil. The low-yield soil followed a similar pattern, with a P content of 238 mg P kg-1 soil in the control. Upon ASi addition, the P released decreased to 193 mg P kg-1 soil in the 3% ASi treatments. The extracted Si contents significantly increased (p < 0.001, df = 3, F = 1571; p = 0.001, df = 3, F = 1651) with ASi addition for both soils (Figure 4B). The amount of Si that was mobilized from ASi in the ASi treated soils in the Bray and Kurtz extract are similar and followed the same pattern to that found in the Mehlich–III extract (Figure 4B). A similar decrease and pattern in the amount of Fe released due to ASi treatment found in the Mehlich–III extract was also found in the Bray and Kurtz extract for both soils (Figure 4C).
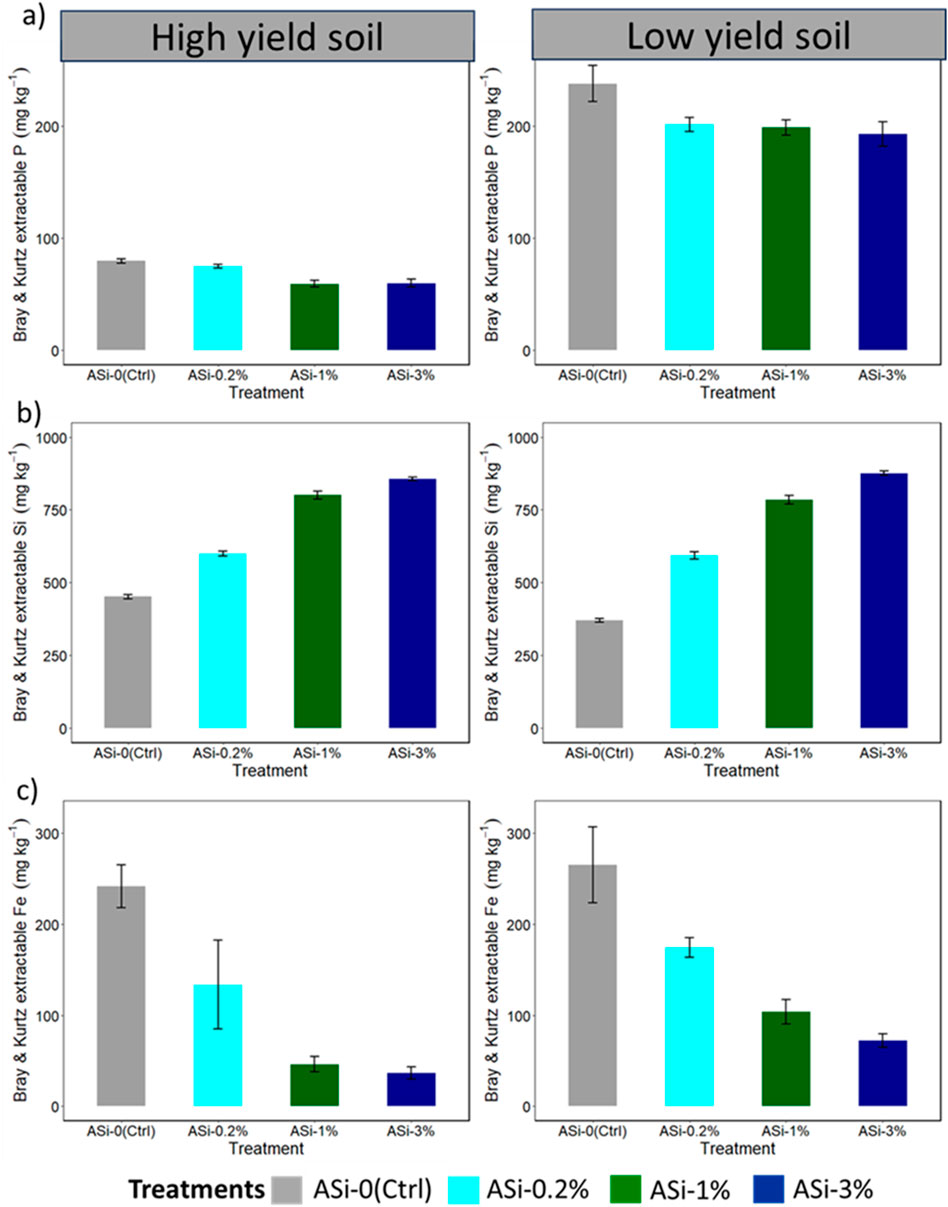
Figure 4. Bray and Kurtz extractable (A) P, (B) Si, and (C) Fe after incubating soil with different ASi content for high and low-yield soils. The bar charts represent the mean, and the error bars represent the standard deviation (n = 4).
Comparing the applied extraction methods in the presence of ASi addition
Irrespective of the different ASi treatments, the extracted P differed strongly with the extraction method used (Figure 5). The amount of P extracted with the different extraction methods increased in the order H2O < Cal < Bray and Kurtz < Mehlich-III for both soils (Figure 5). We found that upon ASi addition (3% ASi addition for example,), the Mehlich-III extraction method showed a higher amount of P (95 mg P kg-1 soil) compared to Bray and Kurtz (60 mg P kg-1), CAL (58 mg P kg-1), and water (10.9 mg P kg-1) extraction methods in the high yield soil. The low-yield soil however showed a much higher P released with all the extraction methods compared to the high-yield soil. Similarly, Mehlich-III showed the highest extraction of P for treatments with and without ASi addition, while the water extraction showed the lowest extraction for P.
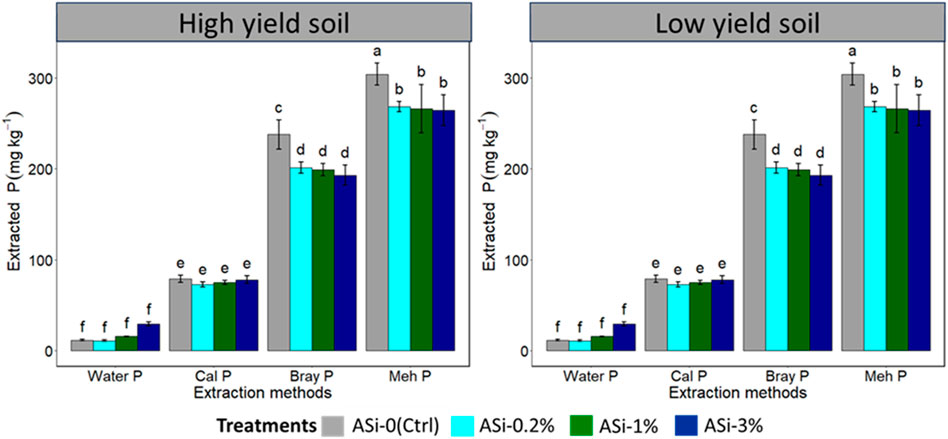
Figure 5. Phosphorus (P) extracted using different extraction methods in increasing order of extraction yield (n = 4). Water, Calcium acetate lactate (CAL), Bray and Kurtz, and Mehlich-III for high and low-yield soils.
Discussion
The studied soils differed in characteristics
We found higher P content in the high-yield soil than in the low-yield soil in both the dithionate and the oxalate extract. Both extraction methods extracted a considerable amount of P (Table 1). However, we found higher P contents in the dithionate extracts compared to the oxalate extracts for both soils. This could be explained by the content of Fe (amorphous and crystalline) of the soils used in our experiment. The dithionate and oxalate extraction methods have been suggested to target crystalline and amorphous forms of Fe oxides rather than those integrated into the structure of silicate minerals (Mehra and Jackson, 2013). Therefore, all the bound P in the respective fraction of the Fe oxides were determined, which also explains the high P amount in both soils as determined by dithionite and oxalate extraction methods. The dithionate extractable Fe was more abundant than the oxalate extractable Fe oxides, and their ratio has been used to estimate their crystallinity (Wuenscher et al., 2015). The results of our investigation showed that the Feo/Fed ratios are <0.5 for both soils, suggesting that our soil samples were mostly composed of well-crystalline iron oxides (Feo/Fed < 0.5) (Lair et al., 2009; Wuenscher et al., 2015). The observed high amount of P in the oxalate and dithionate extract in our study suggests high binding of P to the Fe oxides in both soils, potentially due to the high crystallinity of the soil as measured by the Feo/Fed ratio (Wuenscher et al., 2015).
Phosphorus mobility is increased with ASi addition in the water extract
We found a clear and significant increase in the water-extractable P for the high and low-yield soils upon ASi addition throughout the incubation time (Figure 1A). These findings showing a strong relationship between ASi increasing P mobility in water extracts are consistent with previous studies showing an increase in P mobilization with increasing soil ASi content (Reithmaier et al., 2017; Schaller et al., 2019; Hömberg et al., 2020; Schaller et al., 2020). The observed increase in P mobilization could be attributed to the significant increase in silicic acid mobilized from the ASi for both soils, increasing with incubation time (Figure 1B). This mobilization of silicic acid may explain the observed increase in P extraction. Silicic acid can adsorb to Fe oxides covalently, forming a bidentate complex being in direct competition with P for sorption sites (Swedlund et al., 2010; Schaller et al., 2020). Swedlund et al. (2010) also demonstrated that polymerization of Si-O-Si occurs between silicic acid adsorbed on the Fe oxide surface. Christl et al. (2012) showed that the adsorption of silicic acid to Fe oxides increased with its concentration. At high silicic acid concentrations, polymerization of silicic acid may occur, causing the speciation of silicic acid to shift from monosilicic acid (low binding affinity) to polysilicic acid (high binding affinity) (Christl et al., 2012; Schaller et al., 2021a). It seems possible that the high silicic acid concentration measured in the water extract (Figure 1B) may suggest a high share of polysilicic acid (Hiemstra et al., 2007; Hamid et al., 2011; Christl et al., 2012; Hiemstra, 2018; Schaller et al., 2021a), with polysilicic acid exhibiting higher binding strength (see above) potentially mobilizing more P from the mineral surface compared to monostelic acid. Overall, the mobilization of P by Si in the water extracts confirms our hypothesis that Si is crucial for mobilizing P from phases where it was previously unavailable.
Phosphorus mobility upon ASi addition differed with extraction methods
Comparing the four different extraction methods in the presence of ASi, we found that ASi addition showed a positive effect regarding P extraction (increased P content with increasing ASi content) only for the water extract (Figure 1A), and the potential underlying mechanisms and processes had been explained above. The CAL extraction showed that ASi had no significant effect on P extractability (Figures 2A, B). As CAL extraction is a standard for estimating P availability toward plants, the current data contradicts data from a field study clearly showing better wheat plant P nutrition after ASi addition to the soils (Schaller et al., 2021b). Despite the increase in P concentration in plants due to Si fertilization, the soil P availability in the CAL extract showed no difference between the ASi fertilized soil and the control (Schaller et al., 2021b). It is known that Si can be extracted by the acetic acid extraction method (Wu et al., 2020; Schaller et al., 2021a) thus, it could be that the acetic acid in the CAL solution is extracting non-target Si, thereby decreasing the effectiveness of the extractant to extract P under high ASi content. Hence, CAL extraction may not be a suitable method to determine P availability in soils in the presence of ASi.
The negative effect of ASi decreasing extractable P content with increasing ASi content (especially at 3% ASi treatment compared to the control) was found using the Mehlich-III (Figure 3A) and Bray (Figure 4A) extraction methods for both soils. The data from the Mehlich-III extract are in contrast with the study of Klotz et al. (2023) who showed that Si addition to soil did not increase or decrease P mobility using the Mehlich-III extraction method, testing a soil gradient along the Isthmus of Panama. We found that both Mehlich-III and Bray extraction methods followed a similar pattern in terms of decreasing P concentration from the control to the 3% ASi treatment (Figures 3A, 4A). The close relationship could be due to the underlying similar mechanism for P extraction through ligand exchange with fluoride ion (F−) in both extraction methods. The observed decrease in extractable P (upon ASi addition to soil) in the Mehlich-III and Bray extract could be explained as follows: since F− increases the solubility of Si (Iler, 1979; Wang et al., 2004) thereby increasing the Si content as we had shown (Figure 3B), high ASi content in soils may reduce the extraction strength and efficiency of the extractant to target P as F− is already exhausted by Si.
Comparing the four extraction methods with or without ASi treatment, the highest extractable amounts of P from soil were found for the Mehlich-III extraction method, followed by Bray, CAL, and the lowest amounts were extracted by the water extraction method for both soils (Figure 5). The Mehlich-III and the Bray extractants contain F− and both extraction methods are not only extracting the easily available P but also strongly bound P by mineral dissolution (Sims, 2000). This may explain the very high amount of Mehlich-III and Bray extractable P (with or without Si addition). Overall, the P mobilized with or without ASi addition was found to be higher in the low-yield soil than the high-yield soil. The lower amount of P mobilized from the high-yield soil can be explained by the higher Fe content in the dithionate extract than in the low-yield soil, suggesting a higher reactive surface site in the high-yield soil. The higher Fe content may promote stronger adsorption of P and reduce its solubility and mobility even in the presence of ASi (Von Wandruszka, 2006).
In the absence of ASi addition (control), the water-extractable P was 9 mg P kg-1 soil just after 5 hours of incubation and slightly increased with time (Figure 1A). Upon ASi addition, especially at 3% treatment, a higher amount of P was released (20 mg P kg-1 soil after 5 hours of incubation and 29 mg P kg-1 soil after 1 week of incubation). These amounts of water-extractable P in the ASi treatment were higher than the range of water-extractable p values for common soil as reported by Marschner (2011) (0.8–8.0 mg kg-1) without Si addition to soil. Hence, despite negative ASi effects for Mehlich-III, Bray, and CAL extraction methods, ASi addition might be able to increase P availability for plant uptake (water extracts) as shown in a study for crops in a field plot experiment (Schaller et al., 2021b). However, the very low TIC (mainly referred to as the presence of carbonates, such as calcium carbonate (CaCO3) and other mineral forms of carbon) content (0.03%) measured in our study for both soils, may restrict the applicability of our finding to calcareous soils with high CaCO3 content. In such soils, P may form insoluble calcium phosphate compounds due to the presence of high CaCO3 and pH values (Von Wandruszka, 2006). Future studies should be conducted to include calcareous soils to allow for a better comparison of P behavior and dynamics across different soil pH environments in the presence of ASi fertilization and provide a broader understanding of P interaction.
Conclusion
The water extraction method showed an increased P availability upon ASi addition compared to Mehlich-III, Bray, and CAL extraction methods that showed negative to no effect on P mobility. The increased P mobility in the water extract due to Si treatment supports existing data that Si affects the biogeochemical cycling of P, rendering it more available for plant use. While the Mehlich-III and Bray extraction methods showed a decreasing P content in the presence of ASi, the CAL extraction method showed no effect. As the water-extractable P was found to correlate best with plant P accumulation in other studies, we recommend this method. CAL, Mehlich, and Bray extractions seem to be strongly affected by the soil ASi content (at least for Mehlich, and Bray extractions the extracting agent is dissolving the ASi) making those methods not recommendable for studying the effects of Si on P mobility.
Data availability statement
The raw data supporting the conclusions of this article will be made available by the authors, without undue reservation.
Author contributions
PU: Conceptualization, Data curation, Formal Analysis, Investigation, Methodology, Software, Visualization, Writing–original draft, Writing–review and editing, Project administration. MS: Writing–review and editing. KK: Funding acquisition, Writing–review and editing. JS: Writing–review and editing, Funding acquisition, Supervision, Validation.
Funding
The author(s) declare that financial support was received for the research, authorship, and/or publication of this article. The research leading to these results has received funding from the Deutsche Forschungsgemeinschaft (DFG) (SCHA 1822/14-1 and KA 1737/21-1).
Acknowledgments
We thank the Deutsche Forschungsgemeinschaft for funding (SCHA 1822/14-1 and KA 1737/21-1). We would also like to thank Kristina Holz (head of the ZALF Central Laboratory) and her team, for the analytical support.
Conflict of interest
The authors declare that the research was conducted in the absence of any commercial or financial relationships that could be construed as a potential conflict of interest.
Publisher’s note
All claims expressed in this article are solely those of the authors and do not necessarily represent those of their affiliated organizations, or those of the publisher, the editors and the reviewers. Any product that may be evaluated in this article, or claim that may be made by its manufacturer, is not guaranteed or endorsed by the publisher.
References
Beauchemin Suzanne, D. H., Chou, J., Beauchemin, M., Simard, R. R., and Sayers, D. E. (2003). Speciation of phosphorus in phosphorus-enriched agricultural soils using X-ray. Environ. Qual. 32, 1809–1819. doi:10.2134/jeq2003.1809
Bindraban, P. S., Dimkpa, C. O., and Pandey, R. (2020). Exploring phosphorus fertilizers and fertilization strategies for improved human and environmental health. Biol. Fertil. Soils 56, 299–317. doi:10.1007/s00374-019-01430-2
Brady, N., and Weil, R. (1999). The Nature and properties of soils. New Jersey: 13th EdPrentice-Hall Inc., 7458.
Bray, R. H., and Kurtz, L. T. (1945). Determination of total, organic, and available forms of phosphorus in soils. Soil Sci. 59, 39–46. doi:10.1097/00010694-194501000-00006
Bünemann, E. K., Oberson, A., and Frossard, E. (2010). Phosphorus in action: biological processes in soil phosphorus cycling. Springer Science and Business Media.
Christl, I., Brechbuhl, Y., Graf, M., and Kretzschmar, R. (2012). Polymerization of silicate on hematite surfaces and its influence on arsenic sorption. Environ. Sci. Technol. 46, 13235–13243. doi:10.1021/es303297m
Cornelis, J.-T., Delvaux, B., Cardinal, D., André, L., Ranger, J., and Opfergelt, S. (2010). Tracing mechanisms controlling the release of dissolved silicon in forest soil solutions using Si isotopes and Ge/Si ratios. Geochimica Cosmochimica Acta 74, 3913–3924. doi:10.1016/j.gca.2010.04.056
Dietzel, M. (2000). Dissolution of silicates and the stability of polysilicic acid. Geochimica Cosmochimica Acta 64 (19), 3275–3281. doi:10.1016/s0016-7037(00)00426-9
DIN ISO (1997). Bodenbeschaffenheit: Bestimmung des pH-Wertes, Deutsches Institut für Normung. Beuth, Berlin.
Donat, M., Geistert, J., Grahmann, K., Bloch, R., and Bellingrath-Kimura, S. D. (2022). Patch cropping-a new methodological approach to determine new field arrangements that increase the multifunctionality of agricultural landscapes. Comput. Electron. Agric. 197, 106894. doi:10.1016/j.compag.2022.106894
Fuhrman, J., Zhang, H., Schroder, J., Davis, R., and Payton, M. (2005). Water-soluble phosphorus as affected by soil to extractant ratios, extraction times, and electrolyte. Commun. soil Sci. plant analysis 36, 925–935. doi:10.1081/css-200049482
Gartley, K., Sims, J., Olsen, C., and Chu, P. (2002). Comparison of soil test extractants used in mid-Atlantic United States. Commun. Soil Sci. Plant Analysis 33, 873–895. doi:10.1081/css-120003072
Hamid, R. D., Swedlund, P. J., Song, Y., and Miskelly, G. M. (2011). Ionic strength effects on silicic acid (H4SiO4) sorption and oligomerization on an iron oxide surface: an interesting interplay between electrostatic and chemical forces. Langmuir 27, 12930–12937. doi:10.1021/la201775c
Hiemstra, T. (2018). Ferrihydrite interaction with silicate and competing oxyanions: geometry and Hydrogen bonding of surface species. Geochimica Cosmochimica Acta 238, 453–476. doi:10.1016/j.gca.2018.07.017
Hiemstra, T., Barnett, M. O., and van Riemsdijk, W. H. (2007). Interaction of silicic acid with goethite. J. Colloid Interface Sci. 310, 8–17. doi:10.1016/j.jcis.2007.01.065
Hömberg, A., Broder, T., Knorr, K.-H., and Schaller, J. (2021). Divergent effect of silicon on greenhouse gas production from reduced and oxidized peat organic matter. Geoderma 386, 114916. doi:10.1016/j.geoderma.2020.114916
Hömberg, A., Obst, M., Knorr, K.-H., Kalbitz, K., and Schaller, J. (2020). Increased silicon concentration in fen peat leads to a release of iron and phosphate and changes in the composition of dissolved organic matter. Geoderma 374, 114422. doi:10.1016/j.geoderma.2020.114422
Iler, R. K. (1979). The chemistry of silica, Solubility, Polymerization. Colloid Surf. Prop. Biochem. 866.
Klotz, M., Schaller, J., and Engelbrecht, B. M. (2023). Effects of plant-available soil silicon on seedling growth and foliar nutrient status across tropical tree species. Oikos 2023, e10030. doi:10.1111/oik.10030
Koch, T., Deumlich, D., Chifflard, P., Panten, K., and Grahmann, K. (2023). Using model simulation to evaluate soil loss potential in diversified agricultural landscapes. Eur. J. Soil Sci. 74, e13332. doi:10.1111/ejss.13332
Lair, G. J., Zehetner, F., Hrachowitz, M., Franz, N., Maringer, F.-J., and Gerzabek, M. H. (2009). Dating of soil layers in a young floodplain using iron oxide crystallinity. Quat. Geochronol. 4, 260–266. doi:10.1016/j.quageo.2008.11.003
López-Arredondo, D. L., Leyva-González, M. A., González-Morales, S. I., López-Bucio, J., and Herrera-Estrella, L. (2014). Phosphate nutrition: improving low-phosphate tolerance in crops. Annu. Rev. plant Biol. 65, 95–123. doi:10.1146/annurev-arplant-050213-035949
Malhotra, H., Vandana, , Sharma, S., and Pandey, R. (2018). Phosphorus nutrition: plant growth in response to deficiency and excess. Plant nutrients abiotic stress Toler., 171–190. doi:10.1007/978-981-10-9044-8_7
Mehlich, A. (1984). Mehlich 3 soil test extractant: a modification of Mehlich 2 extractant. Commun. soil Sci. plant analysis 15, 1409–1416. doi:10.1080/00103628409367568
Mehlich, A. (2008). Mehlich 3 soil test extractant: a modification of Mehlich 2 extractant. Commun. Soil Sci. Plant Analysis 15, 1409–1416. doi:10.1080/00103628409367568
Mehra, O., and Jackson, M. (2013). Iron oxide removal from soils and clays by a dithionite–citrate system buffered with sodium bicarbonate. Clays and clay minerals. Elsevier, 317–327.
Mylavarapu, R., Obreza, T., Morgan, K., Hochmuth, G., Nair, V., and Wright, A. (2014). Extraction of soil nutrients using mehlich-3 reagent for acid-mineral soils of Florida: SL407/SS62, 5/2014. EDIS 2014. doi:10.32473/edis-ss620-2014
Oyebiyi, O. O. (2024). Distribution and forms of iron and aluminium oxides in tropical soils of central southwestern Nigeria. Environ. Earth Sci. 83, 95. doi:10.1007/s12665-024-11420-9
Rajan, S. S. S. (1975a). Phosphate adsorption and the displacement of structural silicon in an allophane clay. Soil Sci. 26, 250–256. doi:10.1111/j.1365-2389.1975.tb01949.x
Reithmaier, G. S., Knorr, K. H., Arnhold, S., Planer-Friedrich, B., and Schaller, J. (2017). Enhanced silicon availability leads to increased methane production, nutrient and toxicant mobility in peatlands. Sci. Rep. 7, 8728. doi:10.1038/s41598-017-09130-3
Rennert, T. (2018). Wet-chemical extractions to characterise pedogenic Al and Fe species–a critical review. Soil Res. 57, 1–16. doi:10.1071/sr18299
Ringeval, B., Augusto, L., Monod, H., van Apeldoorn, D., Bouwman, L., Yang, X., et al. (2017). Phosphorus in agricultural soils: drivers of its distribution at the global scale. Glob. Chang. Biol. 23, 3418–3432. doi:10.1111/gcb.13618
Sauer, D., Saccone, L., Conley, D. J., Herrmann, L., and Sommer, M. (2006). Review of methodologies for extracting plant-available and amorphous Si from soils and aquatic sediments. Biogeochemistry 80, 89–108. doi:10.1007/s10533-005-5879-3
Schaller, J., Faucherre, S., Joss, H., Obst, M., Goeckede, M., Planer-Friedrich, B., et al. (2019). Silicon increases the phosphorus availability of Arctic soils. Sci. Rep. 9, 449. doi:10.1038/s41598-018-37104-6
Schaller, J., Frei, S., Rohn, L., and Gilfedder, B. S. (2020). Amorphous silica controls water storage capacity and phosphorus mobility in soils. Front. Environ. Sci. 8, 94. doi:10.3389/fenvs.2020.00094
Schaller, J., Puppe, D., Kaczorek, D., Ellerbrock, R., and Sommer, M. (2021a). Silicon cycling in soils revisited. Plants (Basel) 10, 295. doi:10.3390/plants10020295
Schaller, J., Scherwietes, E., Gerber, L., Vaidya, S., Kaczorek, D., Pausch, J., et al. (2021b). Silica fertilization improved wheat performance and increased phosphorus concentrations during drought at the field scale. Sci. Rep. 11, 20852. doi:10.1038/s41598-021-00464-7
Schaller, J., Wu, B., Amelung, W., Hu, Z., Stein, M., Lehndorff, E., et al. (2022). Silicon as a potential limiting factor for phosphorus availability in paddy soils. Sci. Rep. 12, 16329. doi:10.1038/s41598-022-20805-4
Schüller, H. (1969). Die CAL-Methode, eine neue Methode zur Bestimmung des pflanzenverfügbaren Phosphates in Böden. Z. für Pflanzenernährung Bodenkd. 123, 48–63. doi:10.1002/jpln.19691230106
Schüller, H. (2007). Die CAL-Methode, eine neue Methode zur Bestimmung des pflanzenverfügbaren Phosphates in Böden. Z. für Pflanzenernährung Bodenkd. 123, 48–63. doi:10.1002/jpln.19691230106
Schwertmann, U. (1964). Differenzierung der Eisenoxide des Bodens durch Extraktion mit Ammoniumoxalat-Lösung. J. Plant Nutr. Soil Sci. 105, 194–202. doi:10.1002/jpln.3591050303
Sims, J. T. (2000). Soil test phosphorus: Bray and Kurtz P-1. Methods of phosphorus analysis for soils, sediments, residuals, and waters, 13.
Sommer, M., Kaczorek, D., Kuzyakov, Y., and Breuer, J. (2006). Silicon pools and fluxes in soils and landscapes—a review. J. Plant Nutr. Soil Sci. 169, 310–329. doi:10.1002/jpln.200521981
Swedlund, P. J., Miskelly, G. M., and McQuillan, A. J. (2010). Silicic acid adsorption and oligomerization at the ferrihydrite-water interface: interpretation of ATR-IR spectra based on a model surface structure. Langmuir 26, 3394–3401. doi:10.1021/la903160q
Von Wandruszka, R. (2006). Phosphorus retention in calcareous soils and the effect of organic matter on its mobility. Geochem. Trans. 7, 6–8. doi:10.1186/1467-4866-7-6
Wang, J. J., Dodla, S. K., and Henderson, R. E. (2004). Soil silicon extractability with seven selected extractants in relation to colorimetric and ICP determination. Soil Sci. 169, 861–870. doi:10.1097/00010694-200412000-00005
Wu, W., Limmer, M. A., and Seyfferth, A. L. (2020). Quantitative assessment of plant-available silicon extraction methods in rice paddy soils under different management. Soil Sci. Soc. Am. J. 84, 618–626. doi:10.1002/saj2.20013
Keywords: soil phosphorus, water extraction, CAL extraction, Mehlich-III extraction, amorphous silica, phosphorus availability
Citation: Uhuegbue PO, Stein M, Kalbitz K and Schaller J (2024) Silicon effects on soil phosphorus availability: results obtained depend on the method used. Front. Environ. Sci. 12:1461477. doi: 10.3389/fenvs.2024.1461477
Received: 08 July 2024; Accepted: 21 August 2024;
Published: 30 August 2024.
Edited by:
Krishnaswamy Jayachandran, Florida International University, United StatesReviewed by:
Atif Muhmood, Ayub Agriculture Research Institute, PakistanFotis Bilias, Aristotle University of Thessaloniki, Greece
Copyright © 2024 Uhuegbue, Stein, Kalbitz and Schaller. This is an open-access article distributed under the terms of the Creative Commons Attribution License (CC BY). The use, distribution or reproduction in other forums is permitted, provided the original author(s) and the copyright owner(s) are credited and that the original publication in this journal is cited, in accordance with accepted academic practice. No use, distribution or reproduction is permitted which does not comply with these terms.
*Correspondence: Peter Onyisi Uhuegbue, cGV0ZXIudWh1ZWdidWVAemFsZi5kZQ==