- 1Leibniz Centre for Agricultural Landscape Research (ZALF), Müncheberg, Germany
- 2Department of Environmental Systems Science, ETH Zurich, Universitätstrasse 2, Zurich, Switzerland
- 3Department of Seed, Crop and Horticultural Sciences, School of Agriculture and Biotechnology, University of Eldoret, Eldoret, Kenya
Soil acidification and low nutrient availability are two major challenges facing agriculture in most regions of East Africa, resulting in aluminum toxicity and poor crop yields. The amendment of local sediments to cropland can potentially alleviate these challenges, but responses are variable. In this study, we investigated the potential of two different local sediments influenced by volcanic deposits to increase soil pH, Si and P availability and reduce Al toxicity, thereby improve barley yield. Hence, a field experiment was established in Eldoret, Western Kenya, using 1% and 3% addition by weight of two sediments in barley cultivated plots. The Baringo 3% amendment significantly increased soil pH (from 4.7 to 7.0), the available P content (from 0.01 mg g−1 to 0.02 mg g−1) and decreased the Al availability (from 3.03 mg g−1–2.17 mg g−1). This resulted in a barley yield of 4.7 t/ha (+1061%). The Nakuru 3% and Baringo 1% amendments increased yield to 2–3 t/ha, while the Nakuru 1% did not significantly increase yield. These results highlight that, from a biophysical perspective, there are natural and local opportunities to reduce soil acidification and to partly replace mineral fertilizer, but its magnitude depends on the sediment and the amendment rate.
1 Introduction
Food security is a big challenge in many areas of tropical Africa. Soils in these regions are characterized by a low pH and nutrient availability, resulting in low crop yields (Du et al., 2020). About 29% of the tropical land area in tropical Africa is defined as acidic. In Kenya, acidic soils account for 13% of the total agricultural land, especially in the western part of the country (Pandey et al., 1994; Kanyanjua et al., 2002). This is due to the humid to sub-humid tropical climate with moderate to heavy rainfall and high temperatures, which promotes intense weathering and forms soils such as Ferralsols and Acrisols (Soil Survey Staff, 1999). As soils acidify, cations such as potassium (K+), calcium (Ca2+), magnesium (Mg2+), and sodium (Na+) are leached and replaced by hydrogen (H+) and aluminum (Al3+) ions (von Uexküll and Mutert, 1995; Agegnehu et al., 2021). In addition to the loss of nutrients through leaching, the increase in mobile and available Al3+ ions is a major problem of increasing soil acidification (von Uexküll and Mutert, 1995). Especially at pH < 5.5, Al toxicity increases because solubilized Al as Al3+ is plant available and phytotoxic (Sade et al., 2016; Vega et al., 2019). The main toxic effect of Al on higher plants is inhibition of root elongation and alteration of root system structure and functions, resulting in inadequate water and nutrient supply to affected plants (Foy et al., 2003; Kochian et al., 2004). In addition, high Al availability increases direct uptake and accumulation of Al in the plant, resulting in strongly decreasing crop yields (Cocker et al., 1998; Lal and Singh, 1998). Besides the very low pH value (pH 4–5), Ferralsols and Acrisols are also characterized by high contents of iron (Fe) and Al (Balland-Bolou-Bi et al., 2018). Minerals of these elements have a very high phosphorous (P)-sorption capacity, resulting in a P deficiency (Russel et al., 1974; Sanchez and Uehara, 1980; Beauchemin et al., 2003). The issue of P fixation in tropical soils has been under discussion for many decades and many studies on this topic have already been published (Kellogg, 1956; Russel et al., 1974; Sanchez and Uehara, 1980; Ayodele and Agboola, 1981). Due to the strong binding of P to Fe- and Al-oxides and hydroxides, relatively high total P contents are found, but very little of this total P is available to plants (Russel et al., 1974; Hengl et al., 2017; Du et al., 2020). Soil acidity, which occurs in large parts of tropical Africa, can additionally promote the P fixation in the soil (Agegnehu et al., 2021). A very low pH (<5) can result in precipitation of Al- and Fe-phosphates, but already at pH < 5.5, phosphate can easily become unavailable to plants (Russel et al., 1974; Sanchez and Logan, 1992; Agegnehu and Sommer, 2000). As a consequence, farmers in tropical Africa need to apply large quantities of P fertilizer (up to 150 kg ha−1) to obtain high enough yields because the P deficiency caused by P fixation needs to be compensated for (Russel et al., 1974; Nziguheba et al., 2002). However, most smallholders do not have access, due to poor availability and high prices, to these amounts of P fertilizer, resulting in a lack of P for crop production and low plus further declining yields (Sanchez et al., 1997; Nziguheba et al., 2002).
One option, to reduce the dependency on P fertilizer and to reduce Al toxicity might be the amendment of local sediments influenced by volcanic deposits. Kenya experienced high volcanic activities in the past, due to the East African Rift System (EARS) (Blegen et al., 2016). Large volcanoes, such as Menengai in the Pleistocene, have released large amounts of alkaline volcanic tephra during their eruptions, which have been deposited in large parts of the EARS (Tryon and McBrearty, 2006; Blegen et al., 2016). Volcanic tephra is characterized by a high content of silicon (Si), which can be released in large quantities by weathering (Dahlgren et al., 1999). The deposition of alkaline tephra over large areas of the EARS may have affected many sediments in the region, enriching the initial sediments in Si and increasing their pH (Blegen et al., 2016).
Silicon is the second most abundant element in earth’s crust and is a component of many minerals (Wedepohl, 1995). It occurs in the soil in solid phases (primary, secondary minerals, and amorphous phases) and liquid phases (monomeric silicic acid, polymeric silicic acid) (Schaller et al., 2021a). The weathering of the solid Si phases releases silicic acid in solution, which is an important nutrient for many growth-promoting processes in plants (Schaller et al., 2021a). Depending on, e.g., Si concentration in soil solution and solution pH, the forms of silicic acid can convert from monomeric to polymeric silicic acid or the other way around (Schaller et al., 2021a). Both forms may adsorb to mineral surfaces and compete with nutrients like P for binding sites, with a higher affinity of polymeric silicic acid than monomeric silicic acid (Dietzel, 2000; 2002; Reithmaier et al., 2017). Taylor (2004) even found that silicic acid can exchange phosphate from iron oxides, although having a slightly lower binding energy. Schaller et al. (2019; 2022) revealed that amorphous Si (ASi) fertilization increases soil P mobility and plant availability. They suggest that the addition of ASi to soil may have promoted reductive dissolution of the Fe phases/minerals releasing P initially bound to or occluded in these minerals into solution. Additionally, the weathering of the added ASi releases silicic acid (mono- and polymeric) into solution which may exchange P adsorbed to Fe mineral surfaces. Thus, higher soil Si concentration may lead to higher P mobilization in soil, which in turn may result in better plant nutrition, biomass production and crop yields (Neu et al., 2017). Additionally, it was shown that Si fertilization may reduce the bioavailability of Al due to the precipitation of Al and Si as aluminosilicates (Pačes, 1978; Exley et al., 2019). Next to this, numerous studies have shown that Si mediates various physiological processes in plants to mitigate metal toxicity of Al, As, Cd, Mn and Pb (Horiguchi, 1988; Li et al., 2009; Singh et al., 2011; Li et al., 2012; Emamverdian et al., 2018). Silicon modifies cell walls by depositing hydrous SiO2, which provides physical and mechanical protection against biotic stresses. It has also been suggested that Si causes changes in plant cell metabolism, reducing root uptake of potentially toxic elements and inducing the excretion of certain compounds such as organic acids (OAs) and phenolics (Cocker et al., 1998; Gu et al., 2011; Luyckx et al., 2017; Wang et al., 2017). In numerous crops like barley, maize and wheat, the amelioration of Al toxicity by Si has been shown to result from a competition for Si versus Al uptake by roots (Cocker et al., 1998; Pontigo et al., 2015; Vega et al., 2019). Therefore, a Si fertilization effect by addition of local sediments with volcanic deposits could increase the P availability as well as decrease the aluminum toxicity in plants. However, it is important to note that, in addition to Si, other essential nutrients may also be enriched in sediment materials. As the sediments weather over time after incorporation into the soil, nutrients such as P, Na, Ca and K may be released into the soil solution and become available to plants (Manning, 2022).
While volcanic ash is known to improve soil fertility (Fiantis et al., 2019), much less is known about the effects of Si derived from local sediments of areas influenced by volcanic deposits on P availability and Al toxicity in acidic soils. Therefore, we investigated the effects on Si and P availability and Al toxicity of adding local sediments influenced by volcanic deposition to Kenyan arable soils where barley was grown and whether barley yields may be improved by the addition of sediments. We hypothesized that 1) the addition of Si rich sediments will increase P availability and decrease availability of potential toxic Al and 2) the increase in soil pH by the addition of local sediments will also lead to an increased P availability and decreased availability of potential toxic Al. We further hypothesized that 3) the increase in P availability and decrease in Al availability will strongly improve the plant growth and yield of barley.
2 Materials and methods
2.1 Chemical characterization of the sediments
To examine the effects of local sediment amendments to soil properties and plant growth in Kenya, two potentially suitable materials from different locations in the EARS were identified. One of the sediments was from a site in the Nakuru district, south of Lake Nakuru (0.49726S, 36.091794E), which is defined as tuffs, diatomaceous silts and superficial deposits (McCall, 1966). The other sediment was taken from an area west of Lake Baringo (0.574643N, 35.984597E) and is defined as a Ca-rich lacustrine sediment (Hackman, 1987). Both sediments are alkaline, with a pH of 8.6 for the Baringo sediment and 9.4 for the Nakuru sediment. Because of their location inside the rift valley of the EARS, they might be influenced by volcanic tephra deposition (Tryon and McBrearty, 2006; Blegen et al., 2016).
To characterize the sediments, X-Ray fluorescence analysis (XRF), Energy dispersive X-ray analyses at a scanning electron microscope (SEM-EDX), calcium chloride extraction (CaCl2) and sodium carbonate extraction (Na2CO3) were performed. The elemental composition of the sediments was determined by XRF analysis (SPECTRO XEPOS HE, SPECTRO Analytical Instruments GmbH, Kleve, Germany). Pellets for analysis were prepared from 4 g ground sample material and 0.9 g Licowax (Cereox, Fluxana GmbH, Bedburg-Hau, Germany) using a hand-operated hydraulic press (Specac Atlas 15t, Portman Instruments AG, Biel-Benken, Switzerland). The SEM-EDX analysis was examined using a SEM (EVO MA10, ZEISS, Jena, Germany) equipped with an element detector (QUANTAX EDS, Bruker, Billerica, Massachusetts, United States). The plant-available Si was extracted by CaCl2 according to Haysom and Chapman (1975). Briefly, 1 g of sample material was weighed into 50 mL centrifuge tubes and added with 10 mL of a 0.01 M CaCl2 solution, followed by shaking on a roller shaker for 16 h. Subsequently, the tubes were centrifuged (12,800 g for 5 min) and filtered with 0.2 µm membrane filters. The ASi was determined by Na2CO3 extraction (DeMaster, 1981). For this, 0.03 g of sample material was weighed into 50 mL centrifuge tubes and added with 40 mL of 0.1 M Na2CO3 solution. After shaking, the samples were placed in a heating block for 5 h at 85°C. Subsamples were taken after 1 h, 3 h and 5 h by taking 10–15 mL of the supernatant and filtered through 0.2 µm membrane filters. For both CaCl2 and Na2CO3 extracts, Si was determined by inductively coupled plasma optical emission spectroscopy (ICP-OES; iCAP 6300 DUO, ThermoFisher Scientific Inc., Walham, Massachusetts, United States). The cation exchange capacity (CEC) was determined with 0.1 M BaCl2 solution and measured by ICP-OES. Total organic carbon (TOC) was measured by dry combustion using a multiphase detector (RC612, LECO Corporation, St. Joseph, Michigan, United States).
2.2 Study site and experimental design
The study site is located near Eldoret in the Uasin Gishu County in western Kenya (0.4448° N, 35.4685° E) on the cropland of a local farmer, and at an elevation of 2410 m. The climate in Eldoret is cool and temperate with diurnal mean temperature range from 8.4°C to 27°C. It has two rainy seasons with an annual mean of 900–1200 mm (Tsuma et al., 2015). The soils around Eldoret are predominantly characterized as Plinthic Ferralsols with a low pH and high Fe content (Nyachiro and Briggs, 1987). The soil of this study has a pH of 4.7, the cation exchange capacity (CEC) is 9.7 cmol+/kg and the total organic carbon (TOC) is 2.6%. The total Al content is 104 g kg−1, the plant-available Al content (CaCl2) is 0.04 mg g−1. An oxalate/dithionite Fe (Feo/Fed) ratio of 0.04 indicates that it is a strongly weathered soil (Moody and Graham, 1995).
The area of our experimental site was 24 × 19 m with single plot size of 3 × 4 m and was located within a farmland of a local farmer. The experimental design included for an addition of 1% and 3% by weight of each sediment to a depth of 20 cm. For Baringo, this resulted in 24 t ha−1 (1%) and 72 t ha−1 (3%) and for Nakuru, it was in 18 t ha−1 (1%) and 54 t ha−1 (3%). Additionally, control plots without any amendments were established and each treatment was replicated four times. We used a non-randomised block design (stripe design) for practical reason and set up buffering zones of 1 m between each treatment and plot, to avoid interferences between the treatments (Supplementary Figure S1). The sediments were incorporated by hand followed by mixing to a depth of 20 cm. The mixing was performed in the same way for the control plots to ensure the same physical disturbance for all treatments.
Barley (Hordeum vulgare L., ev. Hessekwa) was sown by hand in all plots on 30th of May 2022. Nitrogen (N) fertilizer was applied on every plot after germination (50 kg N ha−1) and at stem extension (90 kg N ha−1). Low P fertilization (2.5 L ha−1, YaraVita Crop Boost, Yara UK Ltd., York, United Kingdom) was performed on all treatments, including the controls, in form of foliar application during the growing season. Harvest was conducted on the 13th of October 2022.
2.3 Sampling and analyses
Plant samples were taken at harvest in form of whole plants. Plants were randomly collected in areas of 40 × 40 cm, weighed, washed, and dried to determine differences in crop yield and biomass. Additionally, soil samples (0–15 cm) of each plot were taken with a small shovel and air dried. The pH of each plot was determined in water at 1:2.5 solid to solution ratio (WTW pH/Cond 3320 with Sentix41 electrode, Xylem Water Solutions, Washington, DC, United States). Soil samples were analyzed for available P, Si and Al using Mehlich III extraction according to the procedure used by Sparks and Bartels (2009). For extraction, 2 g of soil were weighed into 50 mL centrifuge tubes, mixed with 42 mL of Mehlich III extraction solution, and shaken on the overhead shaker for 5 min. The suspension was centrifuged (3,200 g for 5 min), filtered through 0.2 µm filters and analyzed by ICP-OES (iCAP 6300 DUO, ThermoFisher Scientific Inc., Walham, Massachusetts, United States).
To examine the nutrient translocation and accumulation in plant tissues during the growing season, the whole plants were separated into leaves, leaf sheaths, roots and grains and were subsequently analyzed using different extraction methods. Microwave digestion was performed to estimate Fe, Al, and P contents in plant tissues. For this, 0.1 g of plant sample was weighed into closed vessels and mixed with 2 mL of H2O2 and 3 mL of HNO3 and placed in a closed vessel microwave digestion system (CEM-Mars6, CEM Corporation, Matthews, NC, United States). Subsequently, the extracts were made up to 20 mL with deionized water and filtered through 0.2 µm membrane filters. The ASi in plant tissues was determined by a Tiron extraction (Kodama and Ross, 1991). For this extraction, 0.03 g of plant samples were weighed into 50 mL centrifuge tubes, mixed with 30 mL of 0.1 M Tiron solution and heated in a water bath at 85°C for 1 h. Immediately before heating and after 30 min, the samples were gently shaken by hand. Subsequently, the extracted solutions were centrifuged (5,000 g for 5 min) and filtered with 0.2 µm pore size. Both, the microwave and Tiron extracts were analyzed for elemental contents by ICP-OES (iCAP 6300 DUO, ThermoFisher Scientific Inc., Walham, Massachusetts, United States). The C/N ratio of the dried and ground plant material was determined with CNS-Analyzer to provide information about the nitrogen supply of the plants (CNS928-MLC, Leco Instruments Inc., St. Joseph, Michigan, United States).
2.4 Statistics
The data of our study were analyzed using R Studio (R Core Team, 2021). A mixed effect model with subsequent ANOVA and Tukey post hoc test was carried out to determine statistical differences between the treatments. Blocks were treated as a random effect.
3 Results
3.1 Characterization of the sediments
SEM-EDX analyses revealed that the Baringo sediment is characterized by a Ca-rich and aggregated sediment matrix (5–25 µm) with attached particles (<1–5 µm) containing Al and Si (Supplementary Figure S2). The XRF analyses confirmed the high Ca content (10.2%), but showed that Si is the main component of the sediment at 21% by weight (Table 1). In addition, the sediment was enriched in P (0.13%) and Mg (3.24%). The pH value of the Baringo sediment was 8.6 ± 0.1. The Nakuru sediment was characterized with SEM-EDX revealing the presence of a mixture of splinter-like/molten Si-Al-containing particles of varying sizes. The small fraction, measuring between 20 and 50 μm, and the coarse fraction, between 80 and 220 μm, were observed to contain rounded particles (171.4 µm) with soil adhesions in the nanometer range. XRF analyses showed, that Si is the main component (30.2%) followed by Al (8.3%) and showed that it is enriched in Na (4.46%) and K (4.27%). The pH value of the Nakuru sediment was 9.4 ± 0.2. There was no amorphous Si detectable in Nakuru sediment, but the Baringo sediment had an ASi content of 2.62 ± 0.37 mg g−1. Calcium chloride extraction showed that the Baringo sediment had a much higher soluble Si (99.7 ± 1.1 mg kg−1) content than the Nakuru sediment (22.3 ± 0.7 mg kg−1) (Supplementary Figure S3). Both, the Baringo and Nakuru sediment did not show any enrichments and contaminations with potentially toxic elements like As, Cr, Pb or Cd. The Baringo sediment had a high CECeff of 45.49 cmol+ kg−1, with Ca2+ (28.86 cmol+ kg−1), Mg2+ (11.48 cmol+ kg−1) and Na+ (6.78 cmol+ kg−1) being the dominant cations in this sediment (Table 2). The CECeff of the Nakuru sediment was low (4.46 cmol+ kg−1), with Na+ (3.78 cmol+ kg−1) and K+ (1.15 cmol+ kg−1) being the dominant cations in this sediment.
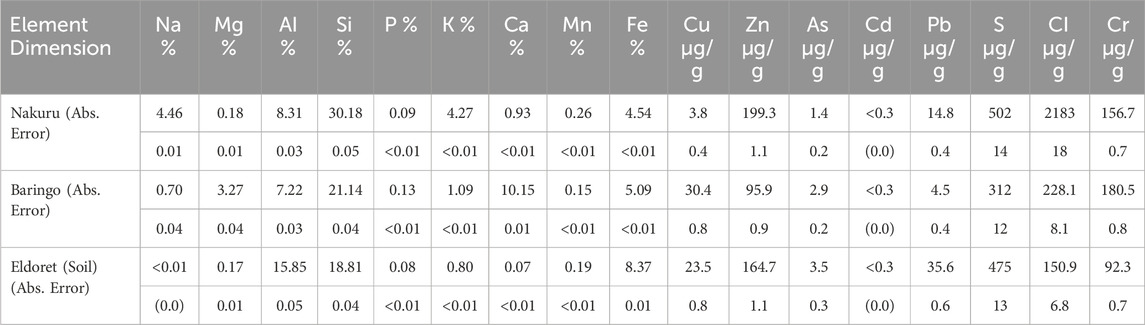
Table 1. Element composition in weight percent or µg/g of the sediment and soil samples measured by XRF analysis. The absolute error of each measurement listed below each measured element. Detection limits are shown in Supplementary Table 1. Voltage=60kV under vacuum.
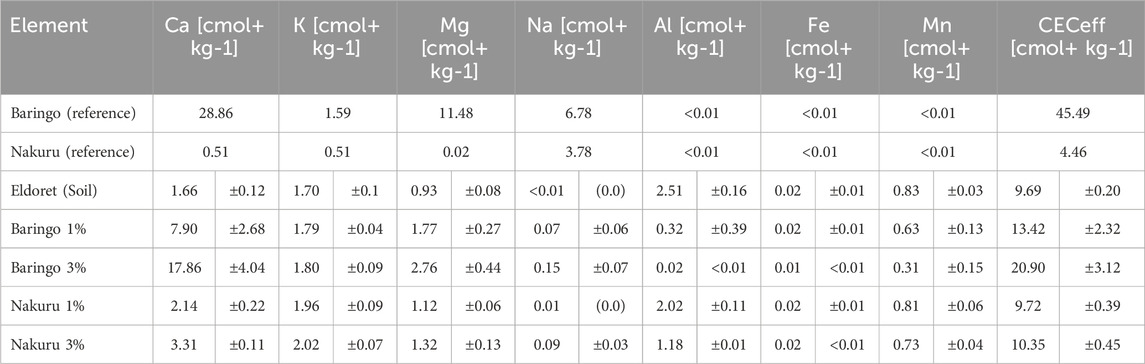
Table 2. CEC data of the sediment materials, the control soil (Eldoret) and the soil after treatment with different sediment amendments in cmol+ kg-1.Standard deviation is given (n=4, sediment referencees were homogenized, thus only one value is shown).
3.2 Effect of local sediment amendments on biomass and yield
Amending soils with local sediments significantly increased both the dry yield (p < 0.001, F = 33.83, df = 4, ANOVA) and dry biomass (p < 0.001, F = 38.48, df = 4, ANOVA) of barley (Figure 1). Both 3% Baringo and 1% Baringo treatment had significantly higher dry yield compared with the control, with 3% Baringo having the highest yield of 4.7 ± 0.3 t ha−1 (p < 0.001 for both Baringo, Tukey). For Nakuru, only the 3% addition had a significantly higher dry yield (2.2 ± 0.6 t ha−1) compared with the control (0.41 ± 0.37 t ha−1; p = 0.003, Tukey). The same pattern was observed for dry biomass, with significantly highest biomass for 3% Baringo, and significantly higher biomass for 1% Baringo and 3% Nakuru than the control (p < 0.001 for both Baringo, p = 0.002 for Nakuru 3%, Tukey). For both biomass and yield, 1% Nakuru had higher values than the control, but not significantly.
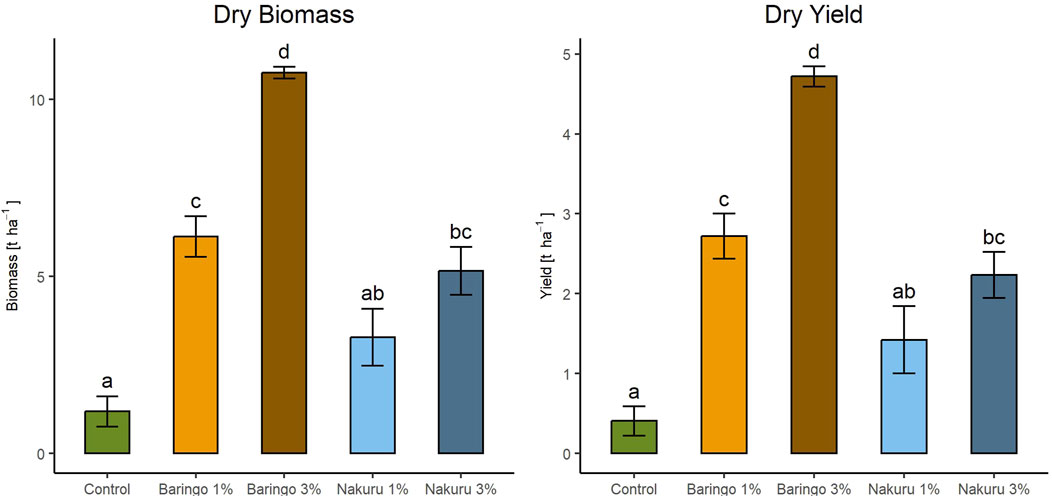
Figure 1. Increased biomass and yield of barley after amendment of different local sediments with 1% and 3% addition. Error bars indicate standard errors. Significant differences between all treatments are shown as different letters, common letters indicate no significant difference (p < 0.05).
3.3 Plant nutrition and toxicants due to sediment amendments
The P content in grains was significantly higher (3.4 ± 0.1 mg g−1) with the 3% amendment of Baringo sediment, compared with the control (2.3 ± 0.3 mg g−1; p < 0.001 Tukey; Figure 2C). Regarding to Al accumulation in grains, only the 3% Baringo treatment had a lower Al content (0.011 ± 0.002 mg g−1) compared with the control (0.021 ± 0.005 mg g−1; p = 0.017, Tukey), but not significantly (p = 0.307, Tukey). In contrast, the grains of both Nakuru treatments tended to have higher Al content and similar P content compared with the control, with lacking any significant differences. Baringo 3% treatment had significantly higher Si contents in the grains (1.9 ± 0.1 mg g−1) than the control (1.0 ± 0.4 mg g−1; p < 0.001 for 3%, Tukey). However, both Nakuru treatments and the Baringo 1% treatment did not significantly increase the Si content in grains (p = 0.138 for Baringo 1%, p = 1 for Nakuru 1% and p = 0.291 for Nakuru 3%, Tukey).
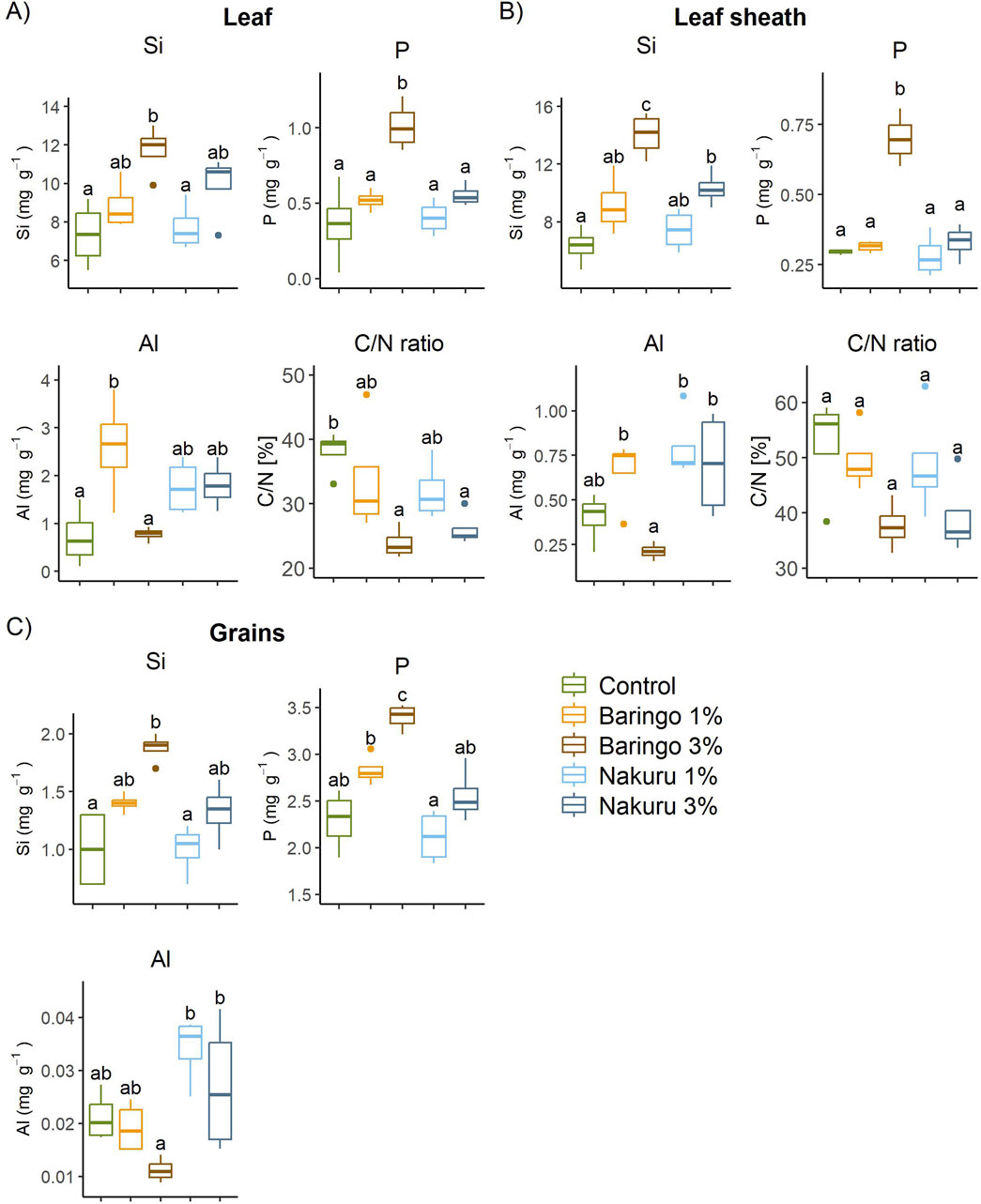
Figure 2. Element contents and C/N ratio at harvest in (A) leaves (B) leaf sheaths and (C) grains of barley with different sediment amendments. Significant differences between all treatments are shown as different letters, common letters indicate no significant difference (p < 0.05).
Silicon contents showed significant differences between the sediment treatments and the control for both, leaves (p = 0.007, F = 5.581, df = 4, ANOVA) and leaf sheaths (p < 0.001, F = 14.75, df = 4, ANOVA) at the stage of harvest (Figures 2A, B). However, only 3% Baringo treatment (11.73 ± 1.31 mg g−1) significantly increased Si contents in the leaves (7.4 ± 1.7 mg g−1 for control, p = 0.005, Tukey). Regarding the leaf sheaths, both sediments with a 3% amendment significantly increased the Si content (6.3 ± 1.3 mg g−1 for control; 14.0 ± 1.5 mg g−1, p < 0.001 for Baringo 3%; 10.3 ± 1.2 mg g−1, p = 0.014 for Nakuru 3%, Tukey), but not the 1% treatments. There are significant differences in P contents of leaves and leaf sheaths (p < 0.001, F = 11.07, df = 4 for leaves; p < 0.001, F = 5.915, df = 4 for leaf sheath, ANOVA), but again the significant effect was only shown with 3% Baringo treatment (1.0 ± 0.2 mg g−1, p < 0.001 for leaves, 0.7 ± 0.1 mg g−1, p < 0.001 for leaf sheath respectively, Tukey). Adding Nakuru sediment had no significant effect on P contents in leaves and leaf sheaths. The Al contents in leaf sheaths decreased significantly with Baringo 3% compared with all other treatments, except of the control, which had also very low Al contents in leaf sheath tissues (p = 0.032 for Baringo 1%, p = 0.005 for Nakuru 1%, p = 0.018 for Nakuru 3%, p = 0.634 for control, Tukey). In leaf tissue, the Al content of control and Baringo 3% were significantly lower than in the Baringo 1% treatment (p = 0.007 for control, p = 0.009 for Baringo 3%, Tukey), but not than in all other treatments. Regarding the C/N ratio at harvest, there are significant differences in leaves (p = 0.01, F = 5.084, df = 4, ANOVA), but not in leaf sheaths (p = 0.066, F = 2.814, df = 4, ANOVA). However, differences were only significant between the 3% treatments and the control (Leaves: 23.8% ± 2.4%, p = 0.009 for Baringo 3%; 26% ± 2.7%, p = 0.03 for Nakuru 3%; Tukey).
3.4 Soil nutrient availability after local sediment amendment
The Baringo sediment amendment significantly increased the pH in an amount-dependent manner (p < 0.001, F = 38.62, df = 4, ANOVA), with the 1% amendment increasing the pH from 4.7 ± 0.1 to 5.7 ± 0.3 (p = 0.004, Tukey) and the 3% amendment increasing it to 7.0 ± 0.6 (p < 0.001, Tukey; Figure 3). In contrast, the Nakuru sediment amendment caused a slight but not signficant increase in pH, rising to around 5.1 at both the 1% (5.1 ± 0.1, p = 0.673, Tukey) and 3% amendments (5.1 ± 0.2, p = 0.602, Tukey).
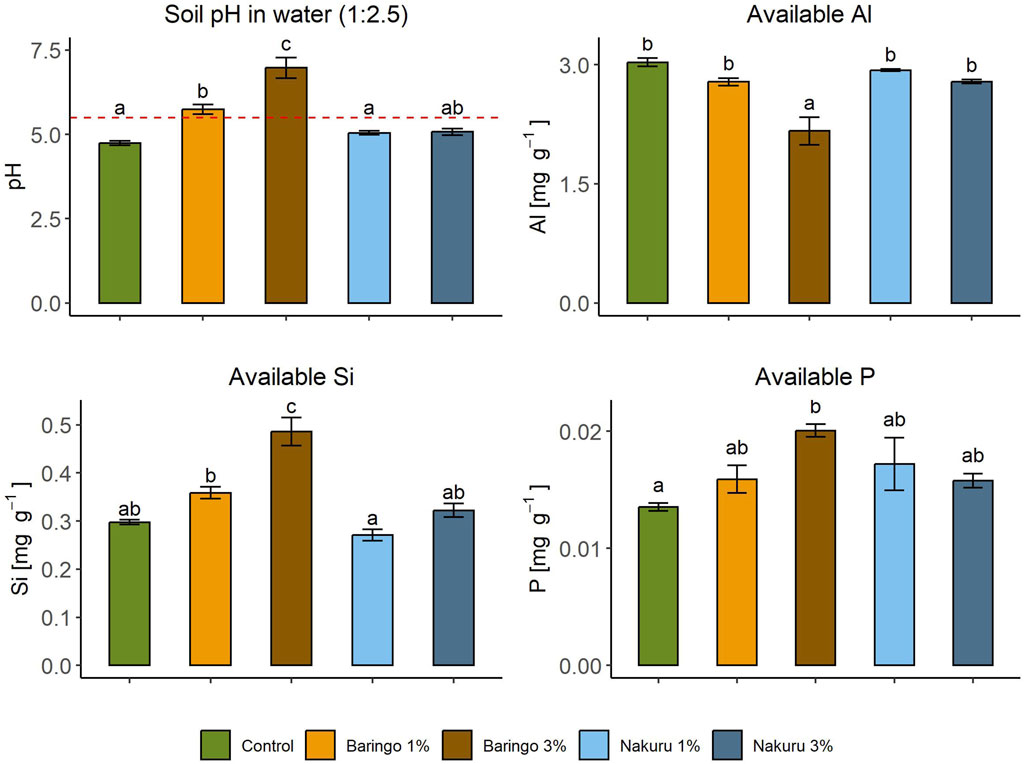
Figure 3. Sediment amendment effect on soil pH, available P, available Si and available Al in the soil. The red dotted line shows the pH threshold of 5.5. Error bars indicate standard errors. Significant differences between all treatments are shown as different letters, common letters indicate no significant difference (p < 0.05).
There were significant differences in nutrient availability between the control and the treatments (Figure 3). Only the 3% Baringo treatment, and not the 1%, significantly increased the contents of available P (0.02 ± 0.001 mg g−1) and Si (0.49 ± 0.6 mg g−1; for both p < 0.001, Tukey). Nakuru sediment amendment did not significantly increase the available P content in the soil (p = 0.213, F = 1.845, df = 2, ANOVA) and there is no significant difference for available Si between control and both Nakuru treatments. Only the 3% amendment of the Baringo sediment (2.17 ± 0.35 mg g−1) significantly decreased the available Al in soil (p < 0.001, Tukey). Again, there is no significant change with 1% Baringo treatment (p = 0.29, Tukey) and both Nakuru treatments (p = 0.916 for Nakuru 1%, p = 0.302 for Nakuru 3%, Tukey).
The amendment of sediments significantly increased CECeff (p < 0.001, F = 28.03, df = 4, ANOVA; Table 2), however only for the 3% Baringo treatment (p < 0.001, Tukey). Nevertheless, both Baringo treatments significantly increased exchangeable Ca (p = 0.008 for Baringo 1%, p < 0.001 for Baringo 3%, Tukey) and Mg (p = 0.001 for Baringo 1%, p < 0.001 for Baringo 3%, Tukey). Baringo 3% additionally increased Na significantly (p = 0.003, Tukey). The Nakuru amendment only significantly increased the exchangeable K (p = 0.004 for Nakuru 1%, p < 0.001 for Nakuru 3%, Tukey). Furthermore, the amendment of both sediments resulted in a significant decrease of exchangeable Al (p = 0.019 for Nakuru 1%, p < 0.001 for Nakuru 3% and bot Baringo, Tukey). A stronger increase (or decrease for Al) was observed for all mentioned elements with a higher amendment of sediment.
4 Discussion
4.1 Barley P and Si nutrition and aluminum accumulation after amendment of local sediments with volcanic influence
Our results indicate potential positive effects of amending local sediments including a certain share of volcanic ash on P, C/N and Si nutrition, as well as reduced aluminium availability in the soil using Baringo sediment at 3%. It is already known that volcanic tephra deposition can increase soil fertility in the surrounding areas, leading to better crop performance (Fiantis et al., 2019). However, in our study, sediments with volcanic influence were applied as a soil amendment in a less fertile region at field scale. The chemical composition of the sediments used in this study is comparable to that of several quaternary tephra deposits and bedded tuff members that have been analyzed in previous studies in the same regions (Tryon and McBrearty, 2006; Blegen et al., 2016).
Mineralogical evidence of the sediments using XRD was not carried out in this study. However, the high Ca content in the Baringo sediment fits to the geological map, which defines the materials at this location as lacustrine sediments (Hackman, 1987). The Pleistocene volcanism in the area north of Lake Baringo is characterized by alkali basalt-trachyte lava and may also have increased the Ca content in the sediments of the surrounding areas (Tryon and McBrearty, 2006). Both sediments have a high total Si content, consistent with volcanic materials (Baker et al., 1972; Blegen et al., 2016). Although the total Si content of the Baringo sediment is lower than that of the Nakuru sediment, it in particular showed higher Si mobilization. One possible explanation for this may be the difference in crystallinity of the Si between the two sediments. While the Baringo sediment has a higher ASi content, the Si in the Nakuru sediment is mostly bound in crystalline minerals. Hence, since weatherability increases with lower crystallinity, Baringo has a higher release of Si than Nakuru sediment (Wolff-Boenisch et al., 2006). This is in line with the results of the SEM analyses where the Nakuru sediment was characterized by splintery/molten Si particles. The total Si content is higher, but the release through dissolution is much lower. The weathering of foreign material applied to the soil not only releases Si in solution, it may also provide a new source of other nutrients (Manning, 2022). In this study, the Baringo sediment released essential nutrients like Ca, Mg and Na which became available to plants and promoted plant growth. In addition, it released mentionable amounts of P, which becomes plant available by dissolution. Furthermore, the highly mobilized Si from the Baringo sediment may promote P availability in the soil. Previous studies revealed an increase in P availability after Si addition. A possible mechanism is the exchange of P from mineral surfaces by silicic acid (Schaller et al., 2019; Schaller et al., 2021b). Hence, Si mobilizes P from typically unavailable sources and thus potentially enhancing its uptake by higher plants. Together with the P fertilizing effect from the Baringo sediment, this may explain the higher soil P availability and stronger P accumulation in plant tissues as shown for the grains yielded from the 3% Baringo treatment. However, these results were only significant for the 3% Baringo treatment. Besides the effect of Si on P availability, soil pH also controls P availability. Below a pH of 5.5, as in this study, P can become unavailable to plants due to binding to Fe and Al oxides and hydroxides and possible precipitation (Sanchez and Logan, 1992). Both sediments used in this study are characterized by alkaline pH of 8.6 (Baringo) or 9.4 (Nakuru), which is consistent with their characterization as lacustrine sediments (Baringo) or tuffs (Nakuru), both probably influenced by the alkaline volcanism of the EARS (Hackman, 1987). The increasing pH (>5.5) with Baringo amendment may be explained by dissolution of carbonates buffering the soil solution pH. This is in line with the high Ca contents released by extraction, which can be attributed to the dissolution of carbonates. An increasing pH promotes the availability of P which may also have occurred in our study. The Nakuru sediment had a much lower influence on the soil pH and thus most likely no agronomic benefit despite also having an alkaline pH. This may be due to the much lower Ca content in the Nakuru sediment and the lack of carbonates. In addition to the pH effects on P availability, a pH value of 5.5 is an important threshold for the mobility of Al in soil solution as pH controls Al speciation. At a pH below 5, Al3+ is the dominant species, which is solubilized in soil solution and is phytotoxic (Kochian et al., 2004). With increasing pH, the phytotoxicity and solubility decreases and the dominant species changes from Al(OH)2+ (pH 5.5–6.5) to Al(OH)3 at neutral pH (Bojórquez-Quintal et al., 2017). This change of speciation of Al results in a lower accumulation in plants and thus lower impact on plant growth (Bojórquez-Quintal et al., 2017). However, also the presence of silicic acid in soil solution may reduce the Al availability in soil solution (Exley et al., 2019). Silicic acid released into solution by weathering of the sediments may have interacted with Al forming short-range ordered aluminosilicates (SROAS) (Exley et al., 2019; Lenhardt et al., 2021). In addition, Si mediates physiological processes and induces changes in cellular metabolism that reduce the uptake and toxicity of heavy metals such as Al (Cocker et al., 1998; Gu et al., 2011; De Sousa et al., 2019). This is in line with the results of this study. In particular, Baringo 3% treatment increased pH and Si the most, resulting in a significant decrease of Al availability in soils and accumulation in plant tissues. Although the Nakuru treatments did not increase the pH above 5.5, the 3% amendment reduced Al availability and the proportion of exchangeable Al in the soil. This may indicate, that Si is also a driving factor in decreasing Al availability next to the soil pH. However, due to the experimental setup, we cannot separate the effects of Si versus the change in pH on the increasing P availability upon addition of the sediments. Nevertheless, only Baringo 3% treatment resulted in a lower Al accumulation in plant tissues. Baringo 1% and both Nakuru treatments did not decrease Al contents in plant tissues. In fact, these treatments resulted in an increased Al accumulation compared with the control. Although the soil in the control treatment had a high availability of Al, the plants showed a very low accumulation of Al in the harvested plant tissue, less than in Baringo 1% and in both Nakuru treatments. This can be attributed to the negative impact of Al toxicity on plant growth. Numerous studies have demonstrated that the primary effect of Al toxicity is the inhibition of root elongation, which negatively affects nutrient and water uptake (Foy et al., 2003; Kochian et al., 2004). As a result, the plants do not grow properly. The plants in the control plots probably suffered from Al toxicity because the soil pH was lower than in all sediment treatments resulting in a higher Al availability in the soil. This led to inhibition of root growth and lower biomass production and therefore lower Al uptake by the plants. This is consistent with plant N uptake in this study, which was also lower in the control treatments. Despite the fact that all the treatments were fertilized with the same amount of N, the plants of the control treatment had a lower uptake of N compared to the other treatments.
4.2 Plant performance and yield after soil sediment amendment
In some cases, amending local sediments improved plant performance resulting in higher barley yield and biomass production. While Nakuru 1% did not significantly increase barley yield compared to the control, Nakuru 3% and Baringo 1% resulted in yield increases of about 500% (450% for Nakuru 3% and 569% for Baringo 1%) and Baringo 3% increased yield by as much as 1061%. However, the yield of the control, Nakuru 1%, Nakuru 3% and also Baringo 1% is still below the 5-year average for 2018/19 - 2022/23 (3.4 t ha−1) (USDA, 2023). What stands out is the Baringo 3% treatment (4.72 t ha−1) showing a higher yield than the 5-year average and also higher than the yield average of 2022 (4.0 t ha−1) (USDA, 2023). These improvements of yield may be attributed to the new source of essential nutrients like Ca, Na, Mg and P, which are supplied by amending 3% of the Baringo sediment and the foliar P fertilizer. Another explanation may be the increased pH and Si availability resulting in an increase of P availability and a decrease of Al toxicity. These effects have caused better nutrient availability and growth conditions resulting in higher yields in the 3% Baringo treatment. The low yields of the control, Baringo 1% and the two Nakuru treatments may also be due to irregular rainfall with drought periods at the end of the growing season. Because of the less developed root system due to Al toxicity, plants in these treatments had a lower water uptake than plants in the Baringo 3% treatment. As a result, they senesced earlier and may have produced less grain.
Although the potential benefits of sediment amendments were demonstrated in this study, the underlying mechanisms are not yet fully understood. Further research on the used sediments and their interactions with the used soil is needed to improve knowledge on the underlying mechanisms. To find out whether some of our results can be explained more by the added Si or by the increased pH, another field experiment should be conducted. For this purpose, additional plots would need to be established that are limed only to compare whether it is only a pH effect or whether other mechanisms are also involved.
5 Conclusion
This study shows that amendment of volcanically influenced local sediments can improve plant growth and yield, but the improvement is dependent on the sediment source and the amendment rate. Of course, a new source of essential nutrients often has a fertilization effect in a soil. However, a release of Si in the soil and a potential increase of soil pH by the sediment amendment are most likely also important drivers for the improvement of yields in this study. Higher Si availability in soil can improve P availability and, in conjunction with higher pH, also reduce Al mobility and its phytotoxicity. These effects can lead to better nutrient uptake of P and Si and less accumulation of Al in plant tissues resulting in better growth and yield of arable crops. The yields of the 3% Baringo treatment resulted in a higher yield compared with the average barley yield in Kenya. In conclusion, amendments of local sediments with volcanic influence could make agriculture in sub-Saharan Africa more sustainable from a biophysical perspective. Importantly, our study demonstrates that the outcomes are, however, dependent on the individual sediment and its amendment rate. Further studies are now needed to investigate possible long-term effects of the amended sediments and to understand the underlying mechanisms.
Data availability statement
The original contributions presented in the study are included in the article/Supplementary Material, further inquiries can be directed to the corresponding author.
Author contributions
ES: Writing–original draft, Methodology, Formal Analysis, Data curation, Conceptualization, Writing–review and editing. MS: Writing–review and editing, Methodology. JhS: Supervision, Writing–review and editing. TB: Methodology, Writing–review and editing. JrS: Writing–review and editing, Supervision, Funding acquisition, Conceptualization.
Funding
The author(s) declare that financial support was received for the research, authorship, and/or publication of this article. The work was funded by Seeding the Future Foundation (US).
Acknowledgments
We thank our local farmer Felix Kipkorir Maiyo for providing the fields and help coordinating the work in Kenya. We acknowledge the central laboratory of ZALF Müncheberg for enabling ICP-OES analyses and Jaqueline Busse (ZALF Müncheberg) for enabling the SEM-EDX analysis.
Conflict of interest
The authors declare that the research was conducted in the absence of any commercial or financial relationships that could be construed as a potential conflict of interest.
Publisher’s note
All claims expressed in this article are solely those of the authors and do not necessarily represent those of their affiliated organizations, or those of the publisher, the editors and the reviewers. Any product that may be evaluated in this article, or claim that may be made by its manufacturer, is not guaranteed or endorsed by the publisher.
Supplementary material
The Supplementary Material for this article can be found online at: https://www.frontiersin.org/articles/10.3389/fenvs.2024.1458360/full#supplementary-material
References
Agegnehu, G., Amede, T., Erkossa, T., Yirga, C., Henry, C., Tyler, R., et al. (2021). Extent and management of acid soils for sustainable crop production system in the tropical agroecosystems: a review. Acta Agric. Scand. Sect. B — Soil and Plant Sci. 71 (9), 852–869. doi:10.1080/09064710.2021.1954239
Agegnehu, G., and Sommer, K. (2000). Optimisation of the efficiency of phosphate fertilisers in acidic-ferralitic soils of the humid tropics. Ethiop. J. Nat. Resour. 2, 63–77.
Ayodele, O., and Agboola, A. A. (1981). Evaluation of phosphorus fixation capacity of tropical savannah soils of western Nigeria. Soil Sci. Soc. Am. J. 45 (3), 462–464. doi:10.2136/sssaj1981.03615995004500030003x
Baker, B. H., Mohr, P. A., and Williams, L. A. J. (1972). “Geology of the eastern Rift System of Africa,” in Geology of the eastern Rift System of Africa (Boulder, Colorado: Geological Society of America), 0.
Balland-Bolou-Bi, C., Bolou-Bi, E. B., Livet, A., Alphonse, V., Giusti-Miller, S., Jusselme, M., et al. (2018). Impact of microbial activity on the mobility of metallic elements (Fe, Al and Hg) in tropical soils. Geoderma 334, 146–154. doi:10.1016/j.geoderma.2018.07.044
Beauchemin, S., Hesterberg, D., Chou, J., Beauchemin, M., Simard, R. R., and Sayers, D. E. (2003). Speciation of phosphorus in phosphorus-enriched agricultural soils using X-ray absorption near-edge structure spectroscopy and chemical fractionation. J. Environ. Qual. 32 (5), 1809–1819. doi:10.2134/jeq2003.1809
Blegen, N., Brown, F. H., Jicha, B. R., Binetti, K. M., Faith, J. T., Ferraro, J. V., et al. (2016). The Menengai Tuff: a 36 ka widespread tephra and its chronological relevance to Late Pleistocene human evolution in East Africa. Quat. Sci. Rev. 152, 152–168. doi:10.1016/j.quascirev.2016.09.020
Bojórquez-Quintal, E., Escalante-Magaña, C., Echevarría-Machado, I., and Martínez-Estévez, M. (2017). Aluminum, a friend or foe of higher plants in acid soils. Front. Plant Sci. 8, 1767. doi:10.3389/fpls.2017.01767
Cocker, K. M., Evans, D. E., and Hodson, M. J. (1998). The amelioration of aluminium toxicity by silicon in higher plants: solution chemistry or an in planta mechanism? Physiol. Plant. 104 (4), 608–614. doi:10.1034/j.1399-3054.1998.1040413.x
Dahlgren, R. A., Ugolini, F. C., and Casey, W. H. (1999). Field weathering rates of Mt. St. Helens tephra. Geochimica Cosmochimica Acta 63 (5), 587–598. doi:10.1016/s0016-7037(99)00067-8
DeMaster, D. J. (1981). The supply and accumulation of silica in the marine environment. Geochimica Cosmochimica Acta 45 (10), 1715–1732. doi:10.1016/0016-7037(81)90006-5
De Sousa, A., Saleh, A. M., Habeeb, T. H., Hassan, Y. M., Zrieq, R., Wadaan, M. A. M., et al. (2019). Silicon dioxide nanoparticles ameliorate the phytotoxic hazards of aluminum in maize grown on acidic soil. Sci. Total Environ. 693, 133636. doi:10.1016/j.scitotenv.2019.133636
Dietzel, M. (2000). Dissolution of silicates and the stability of polysilicic acid. Geochimica Cosmochimica Acta 64 (19), 3275–3281. doi:10.1016/s0016-7037(00)00426-9
Dietzel, M. (2002). “Interactions of polysilicic and monosilicic acid with mineral surfaces,” in Editor I. Stober, and K. Bucher Water-Rock Interaction. Water Science and Technology Library (Dordrecht: Springer) 40. doi:10.1007/978-94-010-0438-1_9
Du, E., Terrer, C., Pellegrini, A. F. A., Ahlström, A., Van Lissa, C. J., Zhao, X., et al. (2020). Global patterns of terrestrial nitrogen and phosphorus limitation. Nat. Geosci. 13 (3), 221–226. doi:10.1038/s41561-019-0530-4
Emamverdian, A., Ding, Y., Xie, Y., and Sangari, S. (2018). Silicon mechanisms to ameliorate heavy metal stress in plants. BioMed Res. Int. 2018, 1–10. doi:10.1155/2018/8492898
Exley, C., Guerriero, G., and Lopez, X. (2019). Silicic acid: the omniscient molecule. Sci. Total Environ. 665, 432–437. doi:10.1016/j.scitotenv.2019.02.197
Fiantis, D., Ginting, F., Gusnidar, G., Nelson, M., and Minasny, B. (2019). Volcanic ash, insecurity for the people but securing fertile soil for the future. Sustainability 11 (11), 3072. doi:10.3390/su11113072
Foy, C. D., Chaney, R., and White, M. (2003). The physiology of metal toxicity in plants. Annu. Rev. Plant Physiology 29, 511–566. doi:10.1146/annurev.pp.29.060178.002455
Gu, H.-H., Qiu, H., Tian, T., Zhan, S.-S., Deng, T.-H.-B., Chaney, R. L., et al. (2011). Mitigation effects of silicon rich amendments on heavy metal accumulation in rice (Oryza sativa L.) planted on multi-metal contaminated acidic soil. Chemosphere 83 (9), 1234–1240. doi:10.1016/j.chemosphere.2011.03.014
Hackman, B. D. (1987). Geological map Baringo - laikipia, degree square 35. Nairobi, Kenya: Ministry of Environment and Natural Recources, Mines and Geological Dept. Kenya.
Haysom, M. B. C., and Chapman, L. S. (1975). Some aspects of the calcium silicate trials at Mackay. Proc. Austr. Sugar Cane Technol. 42, 117–122.
Hengl, T., Leenaars, J. G. B., Shepherd, K. D., Walsh, M. G., Heuvelink, G. B. M., Mamo, T., et al. (2017). Soil nutrient maps of Sub-Saharan Africa: assessment of soil nutrient content at 250 m spatial resolution using machine learning. Nutrient Cycl. Agroecosyst. 109 (1), 77–102. doi:10.1007/s10705-017-9870-x
Horiguchi, T. (1988). Mechanism of manganese toxicity and tolerance of plants. Soil Sci. Plant Nutr. 34 (1), 65–73. doi:10.1080/00380768.1988.10415580
Kanyanjua, S. M., Ireri, L. N., Wambua, S. M., and Nandwa, S. M. (2002). Acidic soils in Kenya: constraints and remedial options.
Kellogg, C. E. (1956). World food and agricultural potentialities. J. Farm Econ. 38 (2), 250–257. doi:10.2307/1234360
Kochian, L., Hoekenga, O., and Piñeros, M. (2004). How do crop plants tolerate acid soils? Mechanisms of aluminum tolerance and phosphorous efficiency. Annu. Rev. plant Biol. 55, 459–493. doi:10.1146/annurev.arplant.55.031903.141655
Kodama, H., and Ross, G. J. (1991). Tiron dissolution method used to remove and characterize inorganic components in soils. Soil Sci. Soc. Am. J. 55 (4), 1180–1187. doi:10.2136/sssaj1991.03615995005500040047x
Lal, R., and Singh, B. R. (1998). Effects of soil degradation on crop productivity in East Africa. J. Sustain. Agric. 13 (1), 15–36. doi:10.1300/j064v13n01_04
Lenhardt, K., Breitzke, H., Buntkowsky, G., Reimhult, E., Willinger, M., and Rennert, T. (2021). Synthesis of short-range ordered aluminosilicates at ambient conditions. Sci. Rep. 11, 4207. doi:10.1038/s41598-021-83643-w
Li, L., Zheng, C., Fu, Y., Wu, D., Yang, X., and Shen, H. (2012). Silicate-mediated alleviation of Pb toxicity in banana grown in Pb-contaminated soil. Biol. Trace Elem. Res. 145 (1), 101–108. doi:10.1007/s12011-011-9165-z
Li, R. Y., Stroud, J. L., Ma, J. F., McGrath, S. P., and Zhao, F. J. (2009). Mitigation of arsenic accumulation in rice with water management and silicon fertilization. Environ. Sci. and Technol. 43 (10), 3778–3783. doi:10.1021/es803643v
Luyckx, M., Hausman, J. F., Lutts, S., and Guerriero, G. (2017). Silicon and plants: current knowledge and technological perspectives. Front. Plant Sci. 8, 411. doi:10.3389/fpls.2017.00411
Manning, D. A. C. (2022). Mineral stabilities in soils: how minerals can feed the world and mitigate climate change. Clay Miner. 57 (1), 31–40. doi:10.1180/clm.2022.17
McCall, G. J. H. (1966). Geol. Map Nakuru area, degree sheet no. 43, North-West Quart. Ministry Nat. Resour. Mines and Geol. Dep. Kenya.
Moody, L. E., and Graham, R. C. (1995). Geomorphic and pedogenic evolution in coastal sediments, central California. Geoderma 67 (3-4), 181–201. doi:10.1016/0016-7061(94)00078-o
Neu, S., Schaller, J., and Dudel, E. (2017). Silicon availability modifies nutrient use efficiency and content, C:N:P stoichiometry, and productivity of winter wheat (Triticum aestivum L.). Sci. Rep. 7, 40829. doi:10.1038/srep40829
Nyachiro, J. M., and Briggs, K. G. (1987). The effects of lime (Ca[OH]2) on the performance of wheat cultivars in plinthic Ferralsols (Eldoret soils) of Kenya. J. Agron. Crop Sci. 159 (4), 287–292. doi:10.1111/j.1439-037X.1987.tb00103.x
Nziguheba, G., Merckx, R., and Palm, C. A. (2002). Soil phosphorus dynamics and maize response to different rates of phosphorus fertilizer applied to an Acrisol in western Kenya. Plant Soil 243 (1), 1–10. doi:10.1023/a:1019926416211
Pačes, T. (1978). Reversible control of aqueous aluminum and silica during the irreversible evolution of natural waters. Geochimica Cosmochimica Acta 42 (10), 1487–1493. doi:10.1016/0016-7037(78)90019-4
Pandey, S., Ceballos, H., Magnavaca, R., Bahía Filho, A. F. C., Duque-Vargas, J., and Vinasco, L. E. (1994). Genetics of tolerance to soil acidity in tropical maize. Crop Sci. 34 (6), 1511–1514. doi:10.2135/cropsci1994.0011183X003400060018x
Pontigo, S., Ribera, A., Gianfreda, L., De La Luz Mora, M., Nikolic, M., and Cartes, P. (2015). Silicon in vascular plants: uptake, transport and its influence on mineral stress under acidic conditions. Planta 242 (1), 23–37. doi:10.1007/s00425-015-2333-1
R Core Team (2021). R: a language and environment for statistical computing. Vienna, Austria: R Foundation for Statistical Computing.
Reithmaier, G.-M. S., Knorr, K.-H., Arnhold, S., Planer-Friedrich, B., and Schaller, J. (2017). Enhanced silicon availability leads to increased methane production, nutrient and toxicant mobility in peatlands. Sci. Rep. 7 (1), 8728. doi:10.1038/s41598-017-09130-3
Russel, D. A., Joe Free, W., and McCune, D. L. (1974). “Potential for fertilizer use on tropical forages,” in Forage fertilization, 39–65.
Sade, H., Meriga, B., Surapu, V., Gadi, J., Sunita, M. S. L., Suravajhala, P., et al. (2016). Toxicity and tolerance of aluminum in plants: tailoring plants to suit to acid soils. BioMetals 29 (2), 187–210. doi:10.1007/s10534-016-9910-z
Sanchez, P. A., and Logan, T. J. (1992). “Myths and science about the chemistry and fertility of soils in the tropics,” in Myths and science of soils of the tropics, 35–46.
Sanchez, P. A., Shepherd, K. D., Soule, M. J., Place, F. M., Buresh, R. J., Izac, A.-M. N., et al. (1997). “Soil fertility replenishment in Africa: an investment in natural resource capital,” in Replenishing soil Fertility in Africa., 1–46.
Sanchez, P. A., and Uehara, G. (1980). “Management considerations for acid soils with high phosphorus fixation capacity,” in The Role of Phosphorus in agriculture., 471–514.
Schaller, J., Faucherre, S., Joss, H., Obst, M., Goeckede, M., Planer-Friedrich, B., et al. (2019). Silicon increases the phosphorus availability of Arctic soils. Sci. Rep. 9 (1), 449. doi:10.1038/s41598-018-37104-6
Schaller, J., Puppe, D., Kaczorek, D., Ellerbrock, R., and Sommer, M. (2021a). Silicon cycling in soils revisited. Plants 10 (2), 295. doi:10.3390/plants10020295
Schaller, J., Scherwietes, E., Gerber, L., Vaidya, S., Kaczorek, D., Pausch, J., et al. (2021b). Silica fertilization improved wheat performance and increased phosphorus concentrations during drought at the field scale. Sci. Rep. 11 (1), 20852. doi:10.1038/s41598-021-00464-7
Schaller, J., Wu, B., Amelung, W., Hu, Z., Stein, M., Lehndorff, E., et al. (2022). Silicon as a potential limiting factor for phosphorus availability in paddy soils. Sci. Rep. 12 (1), 16329. doi:10.1038/s41598-022-20805-4
Singh, V. P., Tripathi, D. K., Kumar, D., and Chauhan, D. K. (2011). Influence of exogenous silicon addition on aluminium tolerance in rice seedlings. Biol. Trace Elem. Res. 144 (1-3), 1260–1274. doi:10.1007/s12011-011-9118-6
Soil Survey Staff, U. (1999). Soil taxonomy: a basic system of soil classification for making and interpreting soil surveys. 2nd edition. U.S. Department of Agriculture Handbook, 436.
Sparks, D. L., and Bartels, J. M. (2009). Methods of soil analysis. Reprinted. Madison, Wis. SSSA Soil Sci. Soc. Am. Soil Sci. Am. book Ser. 5 (3).
Taylor, P. (2004). "Interactions of Silica with Iron Oxides: Effects on Oxide Transformations and Sorption", 27,5.
Tryon, C. A., and McBrearty, S. (2006). Tephrostratigraphy of the bedded tuff member (kapthurin formation, Kenya) and the nature of archaeological change in the later middle Pleistocene. Quat. Res. 65 (3), 492–507. doi:10.1016/j.yqres.2006.01.008
Tsuma, E. I., Odenyo, V. A., and Ouma-Odero, D. (2015). Rainwater harvesting potential at the university of Eldoret, Kenya. Afr. J. Educ. 2 (3), 261.
USDA (2023). Kenya barley area, yield and production. U.S. Department of agriculture (USDA). Int. Prod. Assessement Div. (IPAD). Available at: https://ipad.fas.usda.gov/countrysummary/Default.aspx?id=KE&crop=Barley (Accessed September 14 2023).
Vega, I., Nikolic, M., Pontigo, S., Godoy, K., Mora, M. D. L. L., and Cartes, P. (2019). Silicon improves the production of high antioxidant or structural phenolic compounds in barley cultivars under aluminum stress. Agronomy 9 (7), 388. doi:10.3390/agronomy9070388
von Uexküll, H. R., and Mutert, E. (1995). Global extent, development and economic impact of acid soils. Plant Soil 171 (1), 1–15. doi:10.1007/BF00009558
Wang, M., Gao, L., Dong, S., Sun, Y., Shen, Q., and Guo, S. (2017). Role of silicon on plant–pathogen interactions. Front. Plant Sci. 8, 701. doi:10.3389/fpls.2017.00701
Wedepohl, K. H. (1995). The composition of the continental-crust. Geochimica Cosmochimica Acta 59 (7), 1217–1232. doi:10.1016/0016-7037(95)00038-2
Keywords: aluminum toxicity, crop production, Phosphorus fertilizer, plant performance, silicon availability, yield
Citation: Scherwietes E, Stein M, Six J, Bawen TK and Schaller J (2024) Local sediment amendment can potentially increase barley yield and reduce the need for phosphorus fertilizer on acidic soils in Kenya. Front. Environ. Sci. 12:1458360. doi: 10.3389/fenvs.2024.1458360
Received: 02 July 2024; Accepted: 17 September 2024;
Published: 01 October 2024.
Edited by:
Sören Thiele-Bruhn, University of Trier, GermanyReviewed by:
Nadia Valentina Martínez-Villegas, Instituto Potosino de Investigación Científica y Tecnológica (IPICYT), MexicoThomas Scholten, University of Tübingen, Germany
Copyright © 2024 Scherwietes, Stein, Six, Bawen and Schaller. This is an open-access article distributed under the terms of the Creative Commons Attribution License (CC BY). The use, distribution or reproduction in other forums is permitted, provided the original author(s) and the copyright owner(s) are credited and that the original publication in this journal is cited, in accordance with accepted academic practice. No use, distribution or reproduction is permitted which does not comply with these terms.
*Correspondence: Eric Scherwietes, ZXJpY2xlb24uc2NoZXJ3aWV0ZXNAemFsZi5kZQ==