- Southern California Coastal Water Research Project, Costa Mesa, CA, United States
Exfiltration from sanitary sewers has been researched for many years because of its potential impact on shallow groundwater or surface water, but measurements of exfiltration in situ are rare. Most previous measurements of sanitary sewer exfiltration have been done in the laboratory, in the field using natural, chemical or pharmaceutical tracers or modeled. Relatively few studies have employed physical measurements of volume loss in field settings. Here, we design, test, and apply at a watershed scale, a new methodology for measuring volume loss from sanitary sewer pipes that are currently in use and under typical operating conditions (i.e., not pressurized). The measurement system works by: (1) isolating a section of sanitary sewer between maintenance holes using a sewer bypass or equivalent, (2) introducing roughly 4,200 L of water at a controlled rate into the upstream inspection hole so that pipes remain one-third to one-half full, (3) using vacuum pumps to recover the introduced water at the downstream inspection hole, then (4) measuring differences in the volume from what was pumped into the inspection hole to what was recovered. This process is repeated up to six times to achieve a sensitivity of 0.95 L per experimental pipe segment. This technique was applied to 23 pipe segments of various ages and materials of construction that were selected to be a representative sample of the pipes throughout San Diego. Collectively, these pipes averaged averaged 3.78 × 10−2 L/s-km exfiltration rates (95%CI: 4.96 × 10−2, 2.60 × 10−2). Two of the pipe segments were infiltrating groundwater. Six pipe segments were not statistically different from zero (i.e., no exfiltration). There was no statistical difference between pipe segments of differing ages (p = 0.5) or materials of construction (p = 0.3). This study represents an initial effort at measuring exfiltration from in situ sanitary pipes. Future applications of this methodology should focus on method optimization, measurements at additional locations, and expanding measurements to collect data from additional types of pipe to better understand the geographic portability of the method and the relationship between exfiltration rates, pipe material, and pipe age.
Introduction
Exfiltration from the sanitary sewer has been the subject of research for more than 60 years (Amick and Burgess, 2000; Ducci et al., 2022; Ramseier, 1972; Rutsch et al., 2008; Selvakumar et al., 2004; Vazquez-Sune et al., 1990; Yang et al., 1999). The concern from watershed managers is the potential for exfiltrated sewage to impact shallow groundwater (Lee et al., 2015; Roehrdanz et al., 2017) or nearby surface waters (Delesantro et al., 2022; Sercu et al., 2011; Sercu et al., 2009) with a variety of potential contaminants including bacteria, nutrients, and emerging contaminants (Delesantro et al., 2022; Sercu et al., 2011; Sercu et al., 2009).
While exfiltration from the sanitary sewer has been identified as a potential environmental problem, it is a particularly challenging problem to quantify. Previous studies have employed a wide variety of methodologies to measure exfiltration rates, including ex situ testing in the laboratory (Ellis et al., 2009; Sercu et al., 2003; Vollertsen and Hvitved-Jacobsen, 2003), inferred leakage rates using chemical or naturally occurring tracers in situ (Barrett et al., 1999; Kobayashi et al., 2021; Prigiobbe and Giulianelli, 2011; Rieckermann et al., 2005; Rivers et al., 1996; Wolf et al., 2006), or by modelling (Karpf et al., 2009; Karpf and Krebs, 2011; Sercu et al., 2005; Nguyen et al., 2021; Selvakumar et al., 2004). Each of these techniques has advantages and disadvantages, but few previous studies (Amick and Burgess, 2000) have attempted to measure exfiltration from publicly operated sanitary sewer pipes in situ.
One watershed where exfiltration is a concern is the lower San Diego River (SDR) watershed in San Diego, California, United States. San Diego is the fifth most populous county in the United States (United States Census Bureau, 2024) and, as with most cities in semiarid climates, San Diego has separate sanitary and storm sewer systems. Yet, the SDR and its tributaries have a long history of exceeding water quality objectives for fecal indicator bacteria during wet weather throughout the watershed (Schiff et al., 2023). In addition to fecal indicator bacteria, wet weather is associated with concomitantly elevated levels of the HF183 human fecal marker (Steele et al., 2018) and human specific pathogens (Soller et al., 2017), demonstrating that some portion of fecal indicator bacteria in the separate stormwater system are derived from human sources of fecal contamination (Steele et al., 2018).
There are multiple potential sources of human fecal contamination in the SDR watershed during wet weather. These potential sources include unhoused persons (Hinds et al., 2024), illegal discharges and illicit dumping (Schiff et al., 2023), sanitary sewer overflows (Zimmer-Faust et al., 2021) and onsite wastewater treatment systems. However, measurement of human fecal pollutant inputs due to exfiltration does not exist.
Here we describe a study designed to measure volume loss through exfiltration from gravity pipelines of publicly operated sanitary sewer systems. The study describes the new technique used to measure exfiltration in situ, the operating parameters of the new methodology such as sensitivity, and application of the new technique in sanitary sewer pipes of various ages and materials of construction across San Diego.
Methods
We used a volumetric approach to measure exfiltration in situ, whereby a discreet volume of water could be passed through a section of underground pipe and recovered under controlled conditions, with the difference in measured volume attributed to exfiltration. In order to simulate typical water flows in underground test pipes, a system consisting of three 1,250 L holding tanks, a 757 L measuring tank with stilling well, a pump, a set of flow meters, and multiple valves was devised. Water moved through the system via hard piping constructed of laboratory-grade schedule 80 grey PVC and braided polypropylene tubing. Pipes were either glued together, per the manufacturer’s instructions or plumbed using threaded fittings and PTFE pipe sealant. Connections from hard pipe to braided polypropylene tubing were secured with crimped stainless steel band clamps. A schematic of the system is shown in Figure 1.
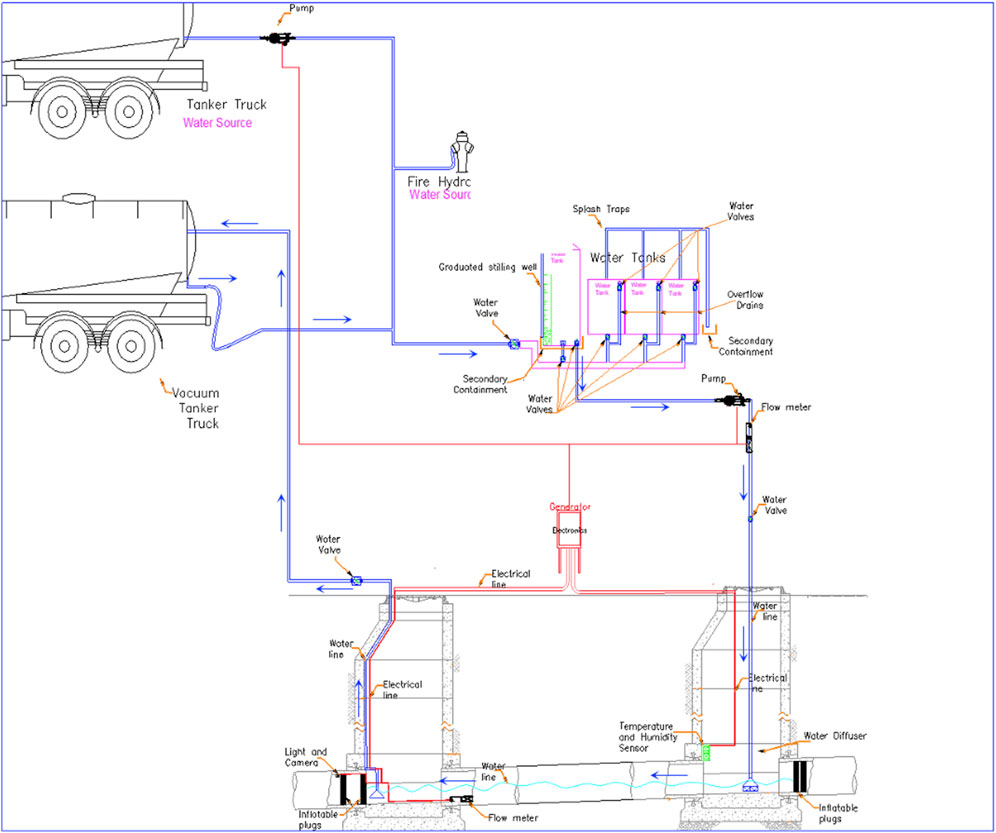
Figure 1. Schematic of in situ measurement system for quantifying exfiltration. Blue arrows indicate direction of water flow. See Supplemental Information for a detailed description of the measurement system and standard operating procedure.
Briefly, the pipe section being tested was first isolated using inflatable plugs. Water contained in the holding and measuring tanks was then pumped at a metered rate to exhaustion through the underground test pipe via the upstream maintenance hole and subsequently captured at the downstream maintenance hole using a vacuum truck. During the test, an above ground flow meter, two in-pipe flow meters, and two water level loggers were employed to monitor flow rate and to maintain the water level in the pipe between one-third and one-half full. The captured water in the vacuum truck was then returned to the test and measurement tanks by the vacuum truck and any volume loss (or gain) recorded. This procedure was repeated up to 6 times at each site. A detailed description of the system, its components, and standard operating procedure is provided in Supplementary Material.
Validating the system and power analysis
A test of the holding tanks and measurement methods was performed by filling the tanks, connecting the tanks to a PVC manifold, and suctioning the water into a vacuum truck (Ledwell Classic, Texarkana, TX). After approximately 4,200 L of water were transferred to the tank of the vacuum truck, the truck tank was then elevated 12–15° and pressurized, and the entire volume of water transferred back to the original tanks. An initial transfer prior to measurement was performed to prime the system and fill in any voids that would sequester water, resulting in a false loss measurement. To measure loss or gain of water from the tanks, level measurements were taken from the stilling well, which had a fixed scale measured in millimeters. The test was repeated 4 times.
To calibrate the measurement tank, known volumes of water (0.945, 3.78, and 37.85 L) were removed from the tank and the level of water in the stilling well was measured. This was repeated ten times at each volume and the mean and standard deviation were calculated. This test was performed both with the holding tanks connected to the measuring tank and with the measuring tank isolated.
To confirm the ability to detect a loss in volume during field tests, and to confirm the sensitivity of the measurement tank, known volumes ranging from 1.97 to 18.93 L were removed during the runs via a valve installed for this purpose on the PVC manifold. The volume removed during field tests was measured using a graduated cylinder and compared to the level loss measured in the stilling well.
Preliminary tests were also used to perform a power analysis to determine the number of repetitions needed to detect a small loss. A test of 6 runs which produced a standard deviation of 1.32 L was used to calculate the statistical power. The analysis was performed in R version 4.3.1 using the power.t.test function with a significance level of 0.05 and a power of 0.8.
Site selection
All sites were located on the coastal plain and foothills of southern San Diego County, CA. More than two-thirds of the soil in this region falls into Hydrogroup D: Soils with very low infiltration rates, typically made of finer particles such as silty or clay loams. Another 10% are classified as having low infiltration rates (Hydrogroup C), with only 17% classified as having moderate to high infiltration rates (Hydrogroups A & B) (SANDAG, 2024). The climate is semi-arid, with average rainfall of 9.79″ per year.
Sites were selected based on a defined set of criteria. The first criterion was that, due to the volume limitations of our equipment, the inner diameter of test pipes could be no larger than 10 inches. The second criterion was that there were no lateral connections coming into test pipes between maintenance holes. The third was the capability to either stop, capture with a vacuum truck, or bypass sewage flows around the test pipe segments. The fourth criterion was that there was ample room to locate and operate the equipment near the maintenance holes. The final and most important consideration was that, since these pipes were predominantly under roadways, traffic could be diverted and testing performed safely.
The materials with which sewers are constructed has changed over time. The first municipal sewers in San Diego date back to 1888 and were constructed of vitrified clay. Since that time, a range of additional materials has been used. These include vitrified clay, but also iron, concrete, PVC, and most recently, lined pipe, which includes cured-in-place pipe (CIPP) and to a lesser degree, Rib-Loc (a spiral-wound PVC liner), which is installed within existing pipes to rehabilitate older sewer lines without the need to dig them up. All segments available for testing included multiple pipe sections, joints, and the troughs of two maintenance holes. Our intention was to test the a representative cross section of the types of sewer construction materials and age classes of the existing pipes found in the watershed (Table 2). To facilitate this, GIS layers from the entire lower San Diego River watershed were utilized to determine the prevalence of each type of pipe material present. This data was then stratified by age into bins of <15 years, 15–30 years, 30–60 years, and >60 years, which is consistent with trends in construction practices.
Isolating the pipe being tested
Sewer pipes were tested by passing approximately 4,200 L of water through them under typical conditions (one-third to one-half full), which required isolating the test pipe from the rest of the collection system (Figure 1). To accomplish this, sewage in test pipes was diverted or interrupted by using maintenance holes up and downstream to pump sewage around the test pipe, capturing upstream flow with a vacuum truck, or shutting down access and water to buildings upstream of the test pipe. With sewage flow interrupted, three commercially-available inflatable plugs designed for this purpose were used to isolate each test pipe section; two in the pipe section just upstream and one plug in the pipe section just downstream of the pipe section to be tested. Prior to installing the downstream plug, a GoPro camera and light package was installed into the pipe, facing upstream, in order to capture video of any water leakage around the plug. Once all three plugs were in place, Signature® Bubbler flowmeter systems equipped with TIENet® Area Velocity sensors (Teledyne ISCO, Lincoln NE) were installed on spring rings into both the upstream and downstream ends of the test pipe.
With the test pipe plugged and meters in place, the next step was to fill the system tanks with water. The outlets of the three trailer mounted holding tanks were closed and the holding tanks filled from the top until the water rose just above the lower edge of the top bulkhead in each tank. Water was then added to the 757 L exterior measurement tank until it was about ¾ full. To ensure that the inflatable plugs were not leaking and the line to be tested was properly sealed, a static test was performed. Briefly, about 189 L of water was released from the 757 L exterior measurement tank and allowed to collect in the downstream inspection hole so that it just covered the plug at the downstream inspection hole. After waiting 15 min for the water level to stabilize, water depth was measured using a measuring stick devised for underground tanks which had been coated with Gasoila® water-finding paste (FedPro, OH, United States) which turns from brown to pink when wet. After another 15-min interval, water in the downstream maintenance hole was remeasured. If the water level was stable, then the plug was properly sealed and the test would proceed. If the water level had dropped, the plug was checked for air leaks or other damage and either reinserted or replaced, and the static test repeated. Only when the static test revealed no leakage could testing begin.
Priming the measurement system
With the static test complete, the measurement system was “primed” by running the entire volume of water tanks through the system to fill any low spots (sags) in the pipe and fill any voids in the vacuum truck or system piping. To begin the priming procedure the vacuum truck, which had been cleaned and emptied prior to arriving, was stationed at the downstream maintenance hole and its hose positioned to capture the water in the trough in the bottom of the vault with vacuum engaged. 22.68 L of 10% sodium hypochlorite solution were added to the water prior to the priming run. All valves on the water holding tanks were opened and water pumped into the upstream inspection hole at a metered rate, typically 50–70 L/min, to produce a one-third to one-half full pipe condition. Water was captured by the vacuum truck at the downstream maintenance hole. Once all water had been emptied from the holding tanks, the pump was halted, valves were closed, and the vacuum truck continued to operate for at least 15 min until no flow was observed in the vault at the downstream end of the test pipe.
The vacuum truck then returned the water to the holding and measuring tanks. To reduce variability, a box was drawn with spray paint or chalk on the ground around both rear wheels so that the truck would return to the same position after each test run reducing measurement variability introduced by the position of the truck from test run to test run. The discharge port on the truck was then connected to the inlet valve on the holding tank system. Valves at the top of the holding tanks were closed, as was the outlet valve to the measuring tank, while the holding tanks filled from the bottom through the PVC manifold. Each holding tank valve was closed individually when its level reached a predetermined point just above the outlet of the upper bulkhead valves.
With the holding tanks full, the upper bulkhead valves and outlet to the measuring tank were opened, allowing water to flow through the upper bulkhead valves to the measuring tank. After 15 min, upper bulkhead valves were closed, sequestering the majority of water in the holding tanks. With the outlet valve to the exterior tank still open and the vacuum hose still connected, the tank on the vacuum truck was elevated to 12–15° and pressurized to ∼5 psi, forcing the remainder of the water into the measuring tank. When large bubbles were observed, the inlet valve to the system was closed. The hose was then depressurized and carefully disconnected from the truck, making sure to capture any water still in the hose. Air was removed from the system through a valve installed for this purpose, ensuring any water that might accidentally escape was captured. All water captured during this process was added to the measuring tank. An initial measurement of water volume was taken by visually recording the level in the stilling well using the mm scale permanently affixed to the stilling well tube. Sodium thiosulfate was added to neutralize the residual chlorine. The measurement of water volume was taken again following the addition.
With the measurement system primed, a measurement of the water level that had collected at the downstream plug was recorded as described previously, effectively repeating the static leak test. This measurement was taken before each subsequent test run to ensure that there were no plug leaks that would allow volume loss or infiltration of groundwater into the test pipe between test runs. The vacuum truck was repositioned at the downstream inspection hole.
To initiate the test run, the vacuum truck at the downstream maintenance hole was activated to preclude the creation of a hydralic head, and all the water in the holding and measurement tanks was pumped into the test pipe at a controlled rate as described previously. When all water had left the holding and measurement tanks (0.75–1 h), the outlet valve was closed and the pump switched off. Once the flow at the downstream inspection hole ceased, which varied by location, the vacuum truck continued to operate at full power for a period of 15 min as described previously. Water was then returned to the tanks following the same procedure described above for priming the system. Once again, the water level in the exterior measuring tank was recorded using the stilling well. The difference between the starting height of the stilling well prior to pumping and the height of the stilling well after refilling the holding and measurement tanks at the end of the test run was used to calculate the loss or gain of volume from the test pipe. This procedure was repeated 3 to six times based on the results of our power analysis and the observed variability of the measurements in the field.
Data analysis
Data analysis required a three-step process. First, experimental results were summarized as average experimental volume loss by pipe segment (liters/experiment). Second, outliers were removed and the remaining data were normalized by the length of pipe segment tested and the duration of the experiment for more appropriate comparisons (liters/s-km). Third, normalized experimental results were statistically tested for significant differences using a two-way ANOVA based on pipe material and age group factors (R v 4.3.1, R Core Team, 2023).
Results
System validation
Initial validation testing of the system to see if the volume could be recovered from the vacuum truck, was performed for four runs. Across these four initial validation runs an average difference of 0.91 L (standard deviation of 2.3 L) was measured. Note that the standard deviation included zero. This was followed by laboratory calibration testing of the measurement variability of the stilling well using calibrated volumes. Thirty laboratory calibration tests found the level measurement in the stilling well was ∼0.2 cm (with the largest standard deviation of 0.075 cm) per liter of removed volume in the measurement tank (Table 1).
This sensitivity testing was repeated in the field with comparable results. Field sensitivity testing found an average level change in the stilling well of 0.76 cm (n = 10, standard deviation 0.09 cm) per 3.785 L or 0.2 cm per liter of removed volume. These measurements had increased variability compared to the laboratory calibration tests but were not statistically different from the laboratory calibration tests. The laboratory calibration measurement number was used to calculate volume loss in situ due to its greater precision. Power analysis performed on preliminary tests found 6 runs to be sufficient to detect a difference (i.e., effect size) of 2.3 L and 3 runs to be sufficient to detect a difference of 4.2 L (Figure 2).
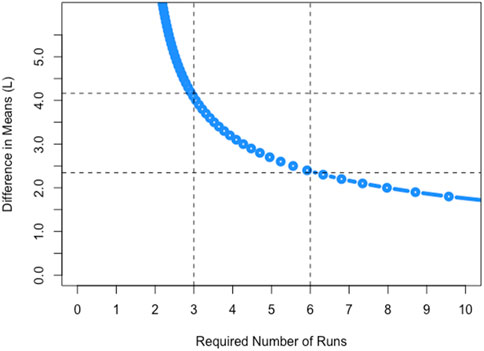
Figure 2. Power analysis curve showing the increase in measurement sensitivity based on increased number of test runs at a single site. Vertical lines show where the curve crosses at 3 and 6 runs, horizontal lines show where the power curve crosses at 2.3 L and 4.2 L.
In situ measurements of exfiltration
A total of 23 individual segments of public sanitary sewer pipe were tested during the study. Sites were located throughout the San Diego area (Figure 3; Table 2). Most sites were in the City of San Diego, while others were in surrounding communities including Lakeview, La Mesa or in unincorporated areas of San Diego County. Note that the isolated test segments include multiple pipe sections, joints, and the troughs of the maintenance holes. The pipes tested consisted of 3 different materials and all were 8″ or 10″ inner diameter. Pipe materials consisted of vitreous clay (n = 11), PVC (n = 8), and lined (CIPP and Rib-Loc, n = 4). No concrete or cast iron pipes were available for testing based on the site selection criteria; both materials were rare in this watershed. Pipes ranged in age from newly installed CIPP to 75-year-old vitreous clay. CIPP ranged in age from <1 to 20 years, PVC pipe ranged in age from <1 to 38 years, and vitreous clay pipe from 49 to 75 years, since installation. Pipe test sections ranged from 80 to 394 feet (Supplementary Table S1).
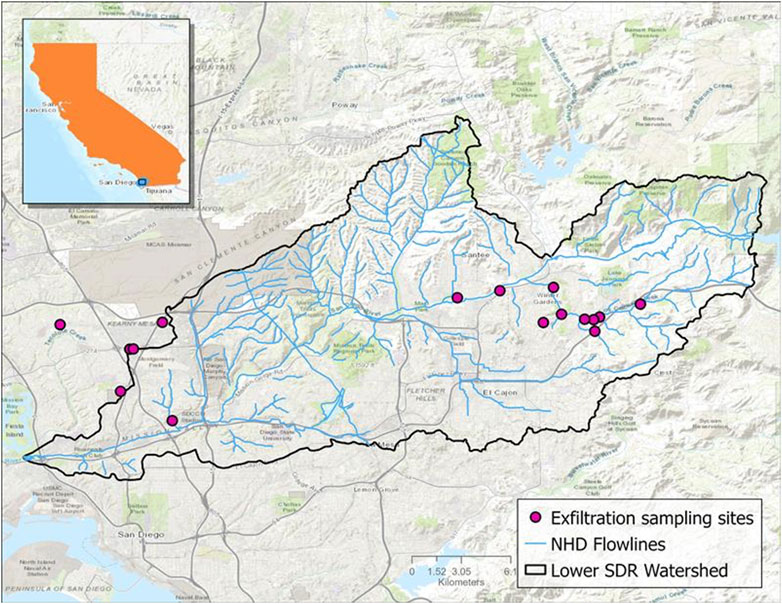
Figure 3. Map of sampling sites showing the flowlines and boundaries of the lower San Diego River watershed.
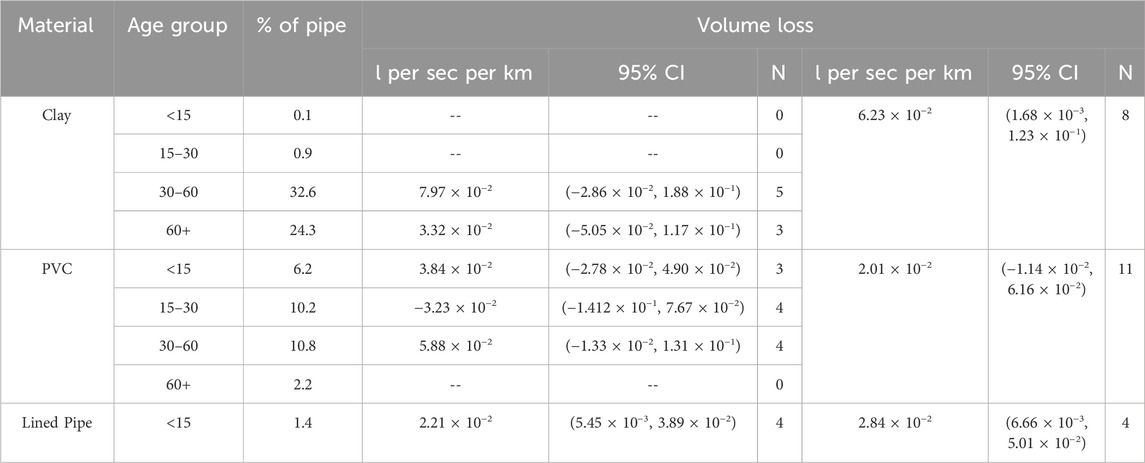
Table 2. Sewer pipe characteristics and in situ measured mean volume loss. The pipe characteristics are grouped by pipe material and age. Each material and age group is also expressed as a percent of total sewer pipe in the San Diego River watershed. In situ measured mean volume loss is shown in liters per second per kliometer, 95% confidence intervals, and number of pipes (N) in each category.
All but two of the pipes lost volume during testing (Figure 4). Average volume lost ranged from zero to more than 76 L. Of the 21 pipes that lost volume, measurements from 15 were statistically different from zero. Two pipes gained volume during testing due to infiltration: One test pipe segment near an embayment gained volume with the rising tide, and another gained volume due to an obvious irrigation leak. A third pipe lost four times as much volume as the next highest volume loss pipe. All three of these pipes were removed from the data set for purposes of estimating average volume loss due to exfiltration (Figure 5).
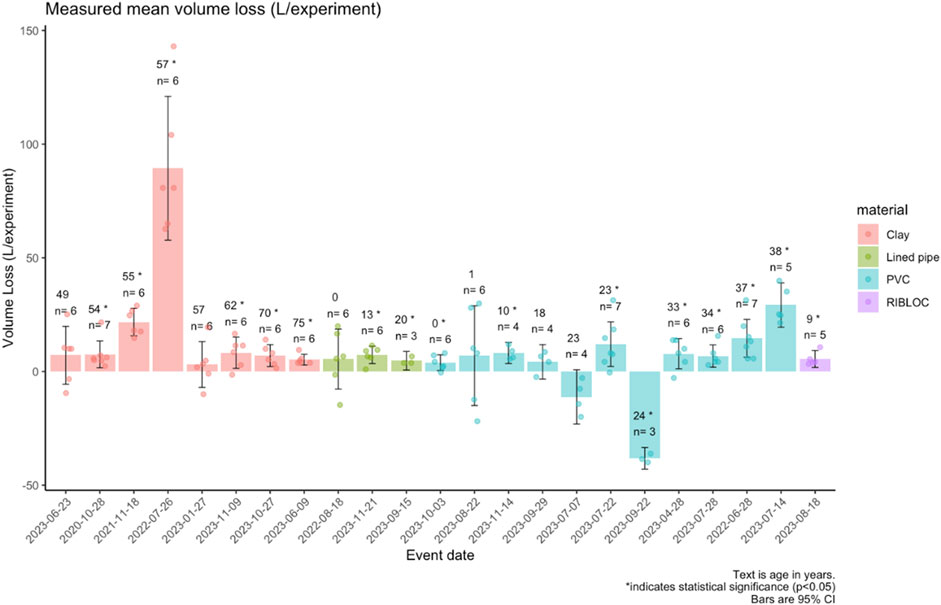
Figure 4. Mean volume loss (liters per experiment) from the 23 test pipe segments across San Diego, CA United States between 2021–2023.
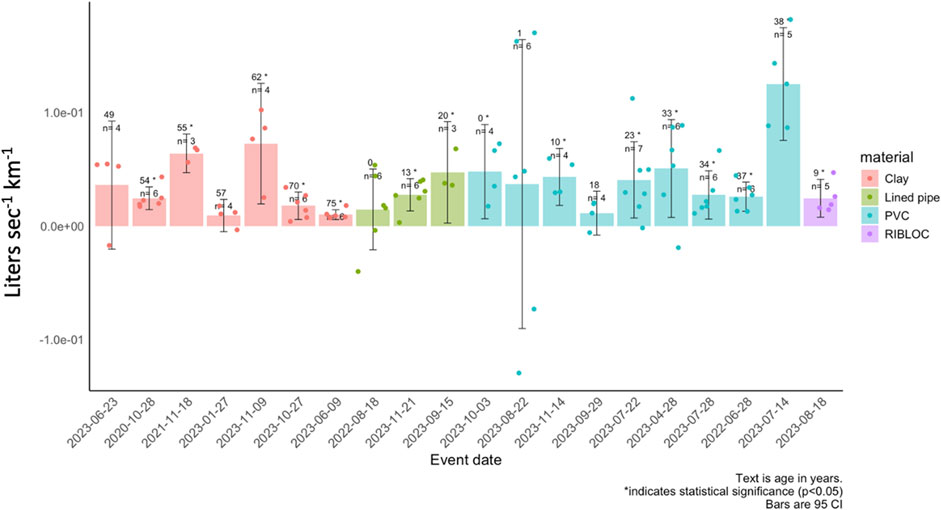
Figure 5. Mean volume loss (liters per second per km) from the 20 test pipe segments across San Diego, CA United States between 2021–2023 after removing outliers and normalizing across sites by the length of pipe and duration of testing.
After normalizing the test system results by the time and length of pipe during testing, the average volume loss varied by pipe material with lined pipe losing the least and PVC the most (Table 2; Figure 6A). There was no trend toward greater loss associated with pipe age; volume loss across all age groupings fell within a similar range (Figure 6B). Despite differences in mean volume loss between pipe materials and ages, the results of two-way ANOVA indicated no statistical difference in volume loss (Supplementary Table S2).
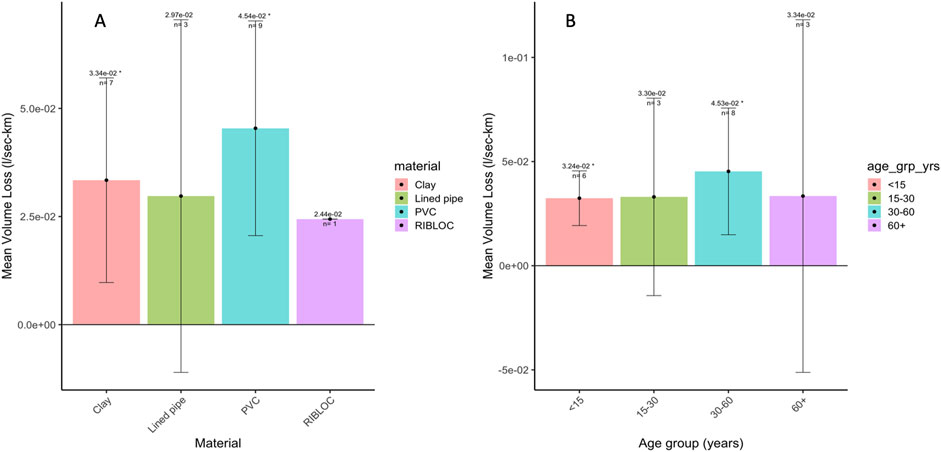
Figure 6. Mean volume loss by categories of (A) pipe material and (B) pipe age since installation. Error bars are 95% confidence intervals. Note that RIBLOC has no confidence interval, as it had a single pipe in the category.
Discussion
The methodology developed for this study was able to empirically measure volume loss in situ from public sanitary sewer pipes under simulated normal operating conditions and without pressure. Previous in situ studies of exfiltration at the pipe level incorporated pressure tests (Dohmann et al., 1999; Ullmann, 1994), which by their nature are likely to overestimate leakage, or employed a water balance approach that relied on in-pipe measurements of flow (Amick and Burgess, 2000). The main advantage of the volumetric loss methodology employed in this study is that it dispenses with the many assumptions and correction factors necessary to carry out sewer exfiltration modeling and replaces estimates with empirical results. Most previous studies of sewer exfiltration rates have relied on either data driven or physically-based models (see Nguyen et al., 2021 for a review). Data driven models include a variety of statistical model types (e.g., Baur and Herz, 2002; DeSilva et al., 2005; Roehrdanz et al., 2017), as well as artificial intelligence and machine learning models (e.g., Khan et al., 2010; Sousa et al., 2014; Hernández et al., 2018), while physically-based models rely on groundwater recharge estimates (Yang et al., 1999; Lerner, 2002; Wolf et al., 2012), water balance principles based on consumption, precipitation and discharge rates (e.g., Karpf and Krebs, 2005; Nakayama et al., 2006), sewer pipe models (e.g., DeSilva et al., 2005; Peche et al., 2017), and exfiltration estimates based on measurements of various sewage constituents and their concentration in subsurface ground water (e.g., Rieckermann et al., 2005; Roehrdanz et al., 2017; Kobayashi et al., 2021; Delesantro et al., 2022). These models, reviewed in Nguyen et al. (2021), have produced a wide range of estimated sewage exfiltration rates, from 2 to 1.4 × 10−9 of L/s-km with a median exfiltration rate of 7.5 × 10−2 L/s-km (25th percentile: 1.7 × 10−2; 75th percentile: 1.8 × 10−1). Our overall sewer exfiltration rate estimate of 3.78 × 10−2 L/s-km, derived from empirical measurements, falls within a similar range as the majority of results reported by previous studies (Table 3).
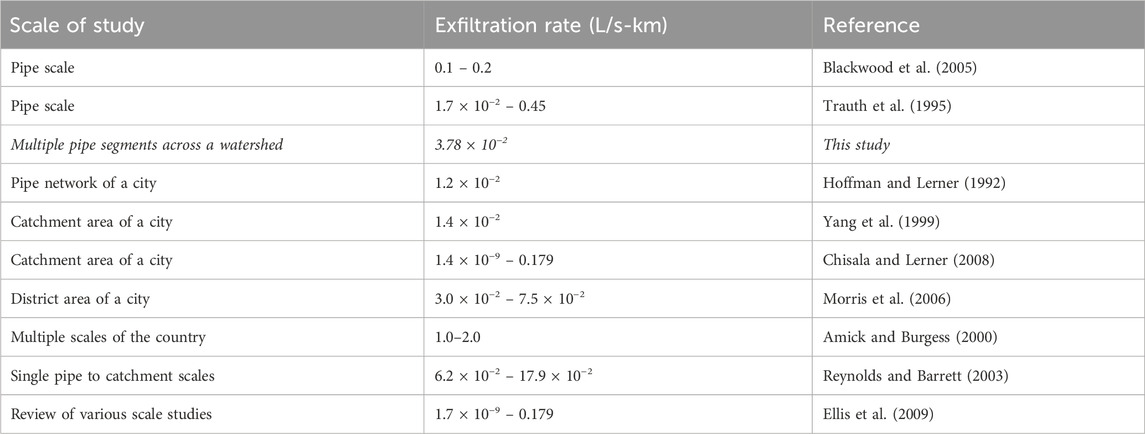
Table 3. Compilation of sewer exfiltration rate per pipe length in modeling studies (from Nguyen et al., 2021) compared to in situ measurements from this study.
Although our sewer exfiltration rates are within the same range as multiple other studies (Table 3), they are orders of magnitude higher than lowest rates reported by some (e.g., Chisala and Lerner, 2008). One possible reason for this disparity has to do with model assumptions surrounding the colmation layer that forms on the inside of sewer pipes. Many models of sewer exfiltration factor in the clogging nature of the colmation layer which has been observed in experimental settings to reduce flow through pipe defects over time (Vollertsen and Hvitved-Jacobsen, 2003). We employed paired in-pipe flow meters and water level sensors, and took great care to ensure that in-pipe flow did not exceed typical operating conditions so as not to scour the colmation layer. However, we still observed a greater volume loss than would be predicted by these models, which may suggest the colmation layer in situ may not behave similarly to laboratory conditions or that the less particle dense water used in our tests may have led to additional incremental volume loss.
Based on ex situ experiments, Vollertsen and Hvitved-Jacobsen (2003) suggest that sewer flushing and subsequent loss of the colmation layer may temporarily increase exfiltration rates through pipe defects. In our study, there were three occasions when the test pipe had to be flushed before testing could proceed (due to large non-sewage debris interfering with our ability to carry out measurements). However, our exfiltration measurements from these pipes were not dramatically different than for pipes that had not been flushed. Interestingly, the largest volume loss was observed on July 26, 2022, for a pipe which had been taken out of service years prior to the study (Figure 2). One hypothesis in that this pipe, which had been dry for many years prior to the study, had lost its colmation layer. Regardless, for data analysis, we eliminated the anomalous site tested on July 26, 2022, but retained the three sites that had been flushed of non-sewage debris.
One factor that may have influenced our measured in situ exfiltration rates is that we used potable water mixed with the residual solids in our test pipe segments from the priming runs. Thus, our test system utilized volumes with sewage particles, but possibly at concentrations of solids lower than what may be found in raw sewage from the tested segments. Further, the high chlorine content in the water in the priming run (∼500 ppt), although subsequently neutralized to <2 ppm, may have also disrupted the colmation layer. We employed flow meters, one at the pump discharge and two in-pipe, to ensure flow rates during testing mimicked typical operating conditions so as to prevent scouring of the pipe surface. Nonetheless, if solids content is an important factor limiting exfiltration in situ, then our system may be biasing exfiltration rates high. In situ studies using raw or synthetic sewage would be helpful in informing these results.
A second factor that may have influenced our measured in situ exfiltration rates is that we only tested public sewer pipe segments without lateral connections to private laterals. This was intentional since our test procedure could not account for additions of unknown volumes of water from private laterals and we could not intercept or stop water usage from private connections. Because defects at lateral connections have been identified as a location that can lead to exfiltration (Decker, 1994), it is possible that our methodology may actually underestimate exfiltration–or that private lateral exfiltration may be as large or larger than public sewer exfiltration–because some of these defects would have been missed by our methodology. A separate study in San Diego that employed static testing of leakage from private laterals estimated a mean rate of 0.33 L (sd = 0.55 L) per hour (Schiff et al., 2024). However, these measurements also excluded the connection between the private laterals and sanitary sewers and thus did not capture potential losses at these connections.
A variable which we were unable to control was the type of soil surrounding our test pipes. Laboratory experiments conducted using different soil types have shown that the composition of the soil surrounding a pipe may influence exfiltration rates (Karpf et al., 2009). Our test sites were primarily located on the large alluvial plain below San Diego’s coastal mountains, where the soil characteristics are primarily low infiltration throughout with only 17% of soils from high infiltration hydrogroups. However, because we made empirical measurements and had no way to examine the soil or the pipe bedding material surrounding our test pipes, we cannot know what, if any, influence soil type may have on exfiltration rates in this study. Because this method was tested exclusively at sites in San Diego, CA, its results are subject to the uncertainties associated with its application under the specific geographic, environmental and physical conditions present at the time of the study. For example, methodological limitations and logistical concerns excluded pipes more that 10″ in diameter, which excluded large trunk lines. Further, no concrete pipes, which may be more common in other locales have been largely replaced in this watershed and were therefore unavailable for testing. Thus, any application of this measurement technique outside the original study area will be subject to the set of uncertainties inherent to that location and should be assessed accordingly.
Our measurements were conducted during periods of dry weather over a two-year time span across all four seasons. It is possible that groundwater levels could have influenced our results since water saturation levels in soil have been shown to reduce exfiltration rates in experimental settings (Karpf et al., 2009). In fact, our test system quantified seawater infiltration at a coastal site due to the rising tide through both continual increases in volume and increasing conductivity of the test water. The test system also measured infiltration of subsurface groundwater at a non-coastal site. Further investigation identified a constant leak in a water main less than 10 m from our test pipe segment.
The methodology developed during this study has broad implications for managing exfiltration from sewer pipes (Steele et al. in review). Local sewer agencies typically use video inspections to monitor the condition of their collection systems. This standard practice works well for finding major defects and assessing capital improvement plans. However, many of the pipes we tested had minor or no defects identified by their most recent video inspection. Nonetheless, some of these pipe segments lost volume under test conditions. Wolf et al. (2006) identified that video inspections were not good predictors of public sewer exfiltration because video resolution is not sufficient to detect small cracks or joint separations that are still large enough for water to pass through. As such, future research should focus on new more sensitive technologies that could be used in routine inspections of collection systems.
Obtaining empirical measurements from in situ sanitary pipes is by its nature a challenging endeavor and would have been impossible without an extraordinary degree of cooperation between the research team and the sanitary collection system owners. The first hurdle was simply to identify pipes of the desired age, material, diameter and depth that had no lateral connections between manholes, an acceptable slope, and where the normal flow of wastewater could theoretically be halted, bypassed around the study site, or collected using vacuum trucks. This was followed by field visits to determine if sites were logistically suitable in terms of equipment placement and could be made safe for workers, who were often operating in roadways where they were exposed to vehicular traffic. Finally, it required weeks of planning for each sampling event to notify residents, control parking, set up traffic control and schedule the equipment and operators to be on-site from early morning until well into the early morning hours of the next day. Throughout the process, wastewater utilities supplied their equipment, personnel and operational and engineering expertise to make this study possible. Simply put, it literally could not have been done without them.
Despite the challenges associated with in situ exfiltration measurements, this new methodology has its place amongst the other tools used to estimate exfiltration and produced results that were very similar to the median value calculated from previous studies regardless of disparities between methods (Nguyen et al., 2021). Similar exfiltration rates were measured or modeled at the pipe scale (e.g., Trauth et al., 1995), the catchment scale (e.g., Yang et al., 1999; Reynolds and Barrett, 2003), or city scale (e.g., Morris et al., 2006; Chisala and Lerner, 2008). Each method has its own set of assumptions and bias, but together, the weight of evidence from in situ measurements, ex situ measurements, and modeling may help collection system managers understand exfiltration in their systems.
Data availability statement
The datasets presented in this study can be found in online repositories. The names of the repository/repositories and accession number(s) can be found in the article/Supplementary Material.
Author contributions
JG: Conceptualization, Investigation, Methodology, Project administration, Resources, Supervision, Validation, Writing–original draft, Writing–review and editing. JS: Conceptualization, Data curation, Formal Analysis, Investigation, Methodology, Project administration, Supervision, Validation, Writing–original draft, Writing–review and editing. AG-F: Data curation, Formal Analysis, Investigation, Methodology, Writing–review and editing. KS: Conceptualization, Investigation, Project administration, Resources, Visualization, Writing–review and editing.
Funding
The author(s) declare that financial support was received for the research, authorship, and/or publication of this article. This project was partially funded by the City of San Diego, County of San Diego, Padre Dam Municipal Water District, City of El Cajon and City of La Mesa under County of San Diego Contract 563356.
Acknowledgments
The authors are grateful for the guidance of the project Technical Review Committee: Martha Tremblay, Patricia Holden, Sandra McLellan, John Izbicki, Mia Mattioli, Jennifer Wolch. The authors wish to acknowledge the partnership and collaboration with the collection system professionals at the City of San Diego, County of San Diego, Padre Dam Municipal Water District, and the City of La Mesa including Daniel Carter, Jeff Van Every, Margaret Llagas, Terrell Powell, Bradley Burns, Sean Willis, Gary Harris, Ted Kautzman, Jimmy Vargas, and Daniel Lockhart. The authors appreciate discussions and suggestions provided by Steve Jepsen. The authors are grateful for the project Steering Committee. This project was partially funded by the City of San Diego, County of San Diego, Padre Dam Municipal Water District, City of El Cajon and City of La Mesa.
Conflict of interest
The authors declare that the research was conducted in the absence of any commercial or financial relationships that could be construed as a potential conflict of interest.
Publisher’s note
All claims expressed in this article are solely those of the authors and do not necessarily represent those of their affiliated organizations, or those of the publisher, the editors and the reviewers. Any product that may be evaluated in this article, or claim that may be made by its manufacturer, is not guaranteed or endorsed by the publisher.
Supplementary material
The Supplementary Material for this article can be found online at: https://www.frontiersin.org/articles/10.3389/fenvs.2024.1458146/full#supplementary-material
References
Amick, R. S., and Burgess, E. H. (2000). Exfiltration in sewer systems. Cincinnati OH: US EPA, Office of Research and Development.
Barrett, M. H., Hiscock, K. M., Pedley, S., Lerner, D. N., Tellam, J. H., and French, M. J. (1999). Marker species for identifying urban groundwater recharge sources: a review and case study in Nottingham, UK. UK. Water Res. 33, 3083–3097. doi:10.1016/s0043-1354(99)00021-4
Baur, R., and Herz, R. (2002). Selective inspection planning with ageing forecast for sewer types. Water Sci. Technol. 46, 389–396. doi:10.2166/wst.2002.0704
Blackwood, D. J., Ellis, J. B., Revitt, D. M., and Gilmour, D. J. (2005). Factors influencing exfiltration processes in sewers. Water Sci. Technol. 51, 147–154. doi:10.2166/wst.2005.0042
Chisala, B. N., and Lerner, D. N. (2008). Distribution of sewer exfiltration to urban groundwater. Proc. Institution Civ. Eng. - Water Manag. 161, 333–341. doi:10.1680/wama.2008.161.6.333
Decker, J. (1994). “Pollution load of subsoil, groundwater and surface water by leaky sewers,” in Proceedings from hydrotop (Marseille, France).
Delesantro, J. M., Duncan, J. M., Riveros-Iregui, D., Blaszczak, J. R., Bernhardt, E. S., Urban, D. L., et al. (2022). The nonpoint sources and transport of baseflow nitrogen loading across a developed rural-urban gradient. Water Resour. Res. 58. doi:10.1029/2021wr031533
DeSilva, D., Burn, S., Tjandraatmadja, G., Moglia, M., Davis, P., Wolf, L., et al. (2005). Sustainable management of leakage from wastewater pipelines. Water Sci. Technol. 52, 189–198. doi:10.2166/wst.2005.0459
Dohmann, M., Decker, J., and Menzenbach, B. (1999). Water contamination due to sewer leakage. Berlin, Heidelberg: Springer.
Ducci, L., Rizzo, P., Pinardi, R., Solfrini, A., Maggiali, A., Pizzati, M., et al. (2022). What is the impact of leaky sewers on groundwater contamination in urban semi-confined aquifers? A test study related to fecal matter and personal care products (PCPs). Hydrology 10, 3. doi:10.3390/hydrology10010003
Ellis, J. B., Revitt, D. M., Lister, P., Willgress, C., and Buckley, A. (2003). Experimental studies of sewer exfiltration. Water Sci. Technol. 47, 61–67. doi:10.2166/wst.2003.0221
Ellis, J. B., Revitt, D. M., Vollertsen, J., and Blackwood, D. J. (2009). Sewer exfiltration and the colmation layer. Water Sci. Technol. 59, 2273–2280. doi:10.2166/wst.2009.271
Hernández, N., Caradot, N., Sonnenberg, H., Rouault, P., and Torres, A. (2018). Support tools to predict the critical structural condition of uninspected pipes for case studies of Germany and Colombia. Water Pr. Technol. 13, 794–802. doi:10.2166/wpt.2018.085
Hinds, J. B., Garg, T., Hutmacher, S., Nguyen, A., Zheng, Z., Griffith, J., et al. (2024). Assessing the defecation practices of unsheltered individuals and their contributions to microbial water quality in an arid, urban watershed. Sci. Total Environ. 920, 170708. doi:10.1016/j.scitotenv.2024.170708
Hoffman, J. M., and Lerner, D. N. (1992). Leak free sewers – who needs them? Water Waste Treat. 35 (8), 18–19.
Karpf, C., and Krebs, P. (2011). A new sewage exfiltration model – parameters and calibration. Water Sci. Technol. 63, 2294–2299. doi:10.2166/wst.2011.167
Karpf, C., Traenckner, J., and Krebs, P. (2009). Hydraulic modelling of sewage exfiltration. Water Sci. Technol. 59, 1559–1565. doi:10.2166/wst.2009.172
Karpf, K., and Krebs, P. (2005). Application of a leakage model to assess exfiltration from sewers. Water Sci. and Technol. 52 (5), 225–231. doi:10.2166/wst.2005.0137
Khan, Z., Zayed, T., and Moselhi, O. (2010). Structural condition assessment of sewer pipelines. J. Perform. Constr. Facil. 24, 170–179. doi:10.1061/(asce)cf.1943-5509.0000081
Kobayashi, J., Kuroda, K., Miyamoto, C., Uchiyama, Y., Sankoda, K., and Nakajima, D. (2021). Evaluating sewer exfiltration in groundwater by pharmaceutical tracers after the 2016 Kumamoto earthquakes, Japan. J. Hazard. Mater. 411, 125183. doi:10.1016/j.jhazmat.2021.125183
Lee, D. G., Roehrdanz, P. R., Feraud, M., Ervin, J., Anumol, T., Jia, A., et al. (2015). Wastewater compounds in urban shallow groundwater wells correspond to exfiltration probabilities of nearby sewers. Water Res. 85, 467–475. doi:10.1016/j.watres.2015.08.048
Lerner, D. N. (2002). Identifying and quantifying urban recharge: a review. Hydrogeol. J. 10, 143–152. doi:10.1007/s10040-001-0177-1
Morris, B. L., Darling, W. G., Cronin, A. A., Rueedi, J., Whitehead, E. J., and Gooddy, D. C. (2006). Assessing the impact of modern recharge on a sandstone aquifer beneath a suburb of Doncaster, UK. Hydrogeol. J. 14, 979–997. doi:10.1007/s10040-006-0028-1
Nakayama, T., Watanabe, M., Tanji, K., and Morioka, T. (2006). Effect of underground urban structures on eutrophic coastal environment. Sci. total Environ. 373, 270–288. doi:10.1016/j.scitotenv.2006.11.033
Nguyen, H. H., Peche, A., and Venohr, M. (2021). Modelling of sewer exfiltration to groundwater in urban wastewater systems: a critical review. J. Hydrol. 596, 126130. doi:10.1016/j.jhydrol.2021.126130
Peche, A., Graf, T., Fuchs, L., and Neuweiler, I. (2017). A coupled approach for the three-dimensional simulation of pipe leakage in variably saturated soil. J. Hydrol. 555, 569–585. doi:10.1016/j.jhydrol.2017.10.050
Prigiobbe, V., and Giulianelli, M. (2011). Quantification of sewer leakage by a continuous tracer method. Water Sci. Technol. 64, 132–138. doi:10.2166/wst.2011.639
Ramseier, R. E. (1972). Testing new sewer pipe installations. Water Pollut. Control Fed. 44 (4), 557–564. Available at: http://www.jstor.org/stable/25037423.
R Core Team (2023). R: a language and environment for statistical computing. Vienna, Austria. Available at: https://www.R-project.org/.
Reynolds, J., and Barrett, M. (2003). A review of the effects of sewer leakage on groundwater quality. Water Environ. J. 1, 34–39. doi:10.1111/j.1747-6593.2003.tb00428.x
Rieckermann, J., Borsuk, M., Reichert, P., and Gujer, W. (2005). A novel tracer method for estimating sewer exfiltration. Water Resour. Res. 41. doi:10.1029/2004wr003699
Rivers, C. N., Barrett, M. H., Hiscock, K. M., Dennis, P. F., Feast, N. A., and Lerner, D. N. (1996). Use of nitrogen isotopes to identify nitrogen contamination of the sherwood sandstone aquifer beneath the city of nottingham, United Kingdom. Hydrogeol. J. 4, 90–102. doi:10.1007/s100400050099
Roehrdanz, P. R., Feraud, M., Lee, D. G., Means, J. C., Snyder, S. A., and Holden, P. A. (2017). Spatial models of sewer pipe leakage predict the occurrence of wastewater indicators in shallow urban groundwater. Environ. Sci. Technol. 51, 1213–1223. doi:10.1021/acs.est.6b05015
Rutsch, M., Rieckermann, J., Cullmann, J., Ellis, J. B., Vollertsen, J., and Krebs, P. (2008). Towards a better understanding of sewer exfiltration. Water Res. 42, 2385–2394. doi:10.1016/j.watres.2008.01.019
SANDAG (2024). San Diego association of governments regional data warehouse. Available at: https://geo.sandag.org/portal/apps/experiencebuilder/experience/?id=fad9e9c038c84f799b5378e4cc3ed068&page=Home#data_s=id%3AdataSource_1-0%3A204 (Accessed September 27, 2024).
Schiff, K., Griffith, J., Steele, J., and Zimmer-Faust, A. (2023). Dry and wet weather survey for human fecal sources in the San Diego River watershed. Water 15 (12), 2239. doi:10.3390/w15122239
Schiff, K. C., Griffith, J. F., Steele, J. A., and Gonzalez-Fernandez, A. (2024). Summary of technical research: quantifying sources of human fecal pollution in the lower San Diego River watershed. Technical Report 1380. Costa Mesa, CA: Southern California Coastal Water Research Project.
Selvakumar, A., Field, R., Burgess, E., and Amick, R. (2004). Exfiltration in sanitary sewer systems in the US. Urban Water J. 1, 227–234. doi:10.1080/15730620410001732017
Sercu, B., Werfhorst, L. C. V. D., Murray, J., and Holden, P. A. (2009). Storm drains are sources of human fecal pollution during dry weather in three urban southern California watersheds. Environ. Sci. Technol. 43, 293–298. doi:10.1021/es801505p
Sercu, B., Werfhorst, L. C. V. D., Murray, J. L. S., and Holden, P. A. (2011). Sewage exfiltration as a source of storm drain contamination during dry weather in urban watersheds. Environ. Sci. Technol. 45, 7151–7157. doi:10.1021/es200981k
Soller, J. A., Schoen, M., Steele, J. A., Griffith, J. F., and Schiff, K. C. (2017). Incidence of gastrointestinal illness following wet weather recreational exposures: harmonization of quantitative microbial risk assessment with an epidemiologic investigation of surfers. Water Res. 121, 280–289. doi:10.1016/j.watres.2017.05.017
Sousa, V., Matos, J. P., and Matias, N. (2014). Evaluation of artificial intelligence tool performance and uncertainty for predicting sewer structural condition. Autom. Constr. 44, 84–91. doi:10.1016/j.autcon.2014.04.004
Steele, J. A., Blackwood, A. D., Griffith, J. F., Noble, R. T., and Schiff, K. C. (2018). Quantification of pathogens and markers of fecal contamination during storm events along popular surfing beaches in San Diego, California. Water Res. 136, 137–149. doi:10.1016/j.watres.2018.01.056
Trauth, R., Hahn, H. H., and Xanthopoulos, C. (1995). In-situ method to determine water exchange between sewerage and groundwater. Abwassertechnik 4, 55–57.
Ullmann, F. (1994). Umweltorientierte Bewertung der Abwasserexfiltrationen bei undichten Kanälen dargestellt am Beispiel einer Bundeswehrkaserne. Dissertation. Aachen (Germany): Institut für Siedlungswasserwirtschaft.
United States Census Bureau (2024). County population totals and components of change: 2020-2023. Available at: https://www.census.gov/data/tables/time-series/demo/popest/2020s-counties-total.html (Accessed May 15, 2024).
Vazquez-Sune, E., Castillo, O., Sanchez-Vila, X., Alberich, C., and Carrera, J. (1990). “Use of natural and anthropogenic tracers to identify sources of groundwater recharge in urban areas on Barcelona,” in Tracers and modeling in hydrogeology, atmospheric environment. Part B. Urban atmosphere. Presented at the TraM’2000 (Liege, Belgium: IAHS), 7. doi:10.1016/0957-1272(90)90006-g
Vollertsen, J., and Hvitved-Jacobsen, T. (2003). Exfiltration from gravity sewers: a pilot scale study. Water Sci. Technol. 47, 69–76. doi:10.2166/wst.2003.0223
Wolf, L., Eiswirth, M., and Hötzl, H. (2006). Assessing sewer–groundwater interaction at the city scale based on individual sewer defects and marker species distributions. Environ. Geol. 49, 849–857. doi:10.1007/s00254-006-0180-x
Wolf, L., Zwiener, C., and Zemann, M. (2012). Tracking artificial sweeteners and pharmaceuticals introduced into urban groundwater by leaking sewer networks. Sci. Total Environ. 430, 8–19. doi:10.1016/j.scitotenv.2012.04.059
Yang, Y., Lerner, D. N., Barrett, M. H., and Tellam, J. H. (1999). Quantification of groundwater recharge in the city of Nottingham, UK. Environ. Geol. 38, 183–198. doi:10.1007/s002540050414
Zimmer-Faust, A., Schiff, K., and Nguyen, D. (2021). “Quantify sources of human fecal contamination loading to the San Diego river, technical memorandum, task 8A: compiling and analyzing existing data for sanitary sewer overflow events,” in Report prepared for the San Diego river investigative order steering committee (Costa Mesa, CA: Southern California Coastal Water Research Project), 28.
Keywords: sewer exfiltration, municipal separate storm sewer systems, sewer pipe material, sewer pipe age, sewage flow
Citation: Griffith JF, Steele JA, Gonzalez-Fernández A and Schiff KC (2025) Towards quantifying exfiltration from in situ sanitary sewer pipes. Front. Environ. Sci. 12:1458146. doi: 10.3389/fenvs.2024.1458146
Received: 02 July 2024; Accepted: 15 November 2024;
Published: 23 January 2025.
Edited by:
Vivek Pulikkal, Civil & Environmental Consultants, Inc., United StatesReviewed by:
Joseph Delesantro, The Pennsylvania State University (PSU), United StatesAbhisek Manikonda, Carollo Engineers, United States
Copyright © 2025 Griffith, Steele, Gonzalez-Fernández and Schiff. This is an open-access article distributed under the terms of the Creative Commons Attribution License (CC BY). The use, distribution or reproduction in other forums is permitted, provided the original author(s) and the copyright owner(s) are credited and that the original publication in this journal is cited, in accordance with accepted academic practice. No use, distribution or reproduction is permitted which does not comply with these terms.
*Correspondence: John F. Griffith, am9obmdAc2Njd3JwLm9yZw==; Joshua A. Steele, am9zaHVhc0BzY2N3cnAub3Jn